- 1Clinical Pharmacology, Pharmacy, and Environmental Medicine, Department of Public Health, University of Southern Denmark, Odense, Denmark
- 2Department of Clinical Pharmacology, Faculty of Medicine, University of Helsinki, Helsinki, Finland
- 3Individualized Drug Therapy Research Program, Faculty of Medicine, University of Helsinki, Helsinki, Finland
- 4Division of Pharmaceutical Biosciences, Faculty of Pharmacy, University of Helsinki, Helsinki, Finland
Glucuronidation and sulfation are the most typical phase II metabolic reactions of drugs. The resulting glucuronide and sulfate conjugates are generally considered inactive and safe. They may, however, be the most prominent drug-related material in the circulation and excreta of humans. The glucuronide and sulfate metabolites of drugs typically have limited cell membrane permeability and subsequently, their distribution and excretion from the human body requires transport proteins. Uptake transporters, such as organic anion transporters (OATs and OATPs), mediate the uptake of conjugates into the liver and kidney, while efflux transporters, such as multidrug resistance proteins (MRPs) and breast cancer resistance protein (BCRP), mediate expulsion of conjugates into bile, urine and the intestinal lumen. Understanding the active transport of conjugated drug metabolites is important for predicting the fate of a drug in the body and its safety and efficacy. The aim of this review is to compile the understanding of transporter-mediated disposition of phase II conjugates. We review the literature on hepatic, intestinal and renal uptake transporters participating in the transport of glucuronide and sulfate metabolites of drugs, other xenobiotics and endobiotics. In addition, we provide an update on the involvement of efflux transporters in the disposition of glucuronide and sulfate metabolites. Finally, we discuss the interplay between uptake and efflux transport in the intestine, liver and kidneys as well as the role of transporters in glucuronide and sulfate conjugate toxicity, drug interactions, pharmacogenetics and species differences.
1 Introduction
Metabolic enzymes and membrane transporters that are expressed in the intestine, liver and kidney have a significant impact on the absorption, distribution, metabolism and excretion of drugs and other compounds. Drug metabolism is mediated primarily by cytochrome P450 (CYP) enzymes (phase I metabolism) and conjugation reactions (phase II metabolism) catalyzed by uridine 5′-diphospho-glucuronosyltransferases (UGTs) and sulfotransferases (SULTs). UGT-mediated glucuronidation is a major metabolic pathway for 12% of drugs, while SULTs contribute major metabolites for ≈1% of drugs (Cerny, 2016). Furthermore, UGTs contribute to some extent to the metabolism of >50% of the 200 most prescribed drugs (Guillemette et al., 2014), evidencing the potential significance of UGTs on drug clearance and pharmacokinetics. Conjugation reactions are also important in steroid homeostasis in humans (Rižner, 2013) and in the elimination of natural compounds (e.g. flavonoids) consumed in food or as dietary supplements (Manach et al., 2004).
UGT- and SULT-mediated conjugation reactions introduce a negative charge and reduce lipid partitioning of the substrate by 2-5 logP units (Smith et al., 1985; Manners et al., 1988; Smith and Dalvie, 2012), which typically results in negligible passive permeability of the formed metabolite. This is in contrast to most phase I metabolites that possess higher lipophilicity than conjugates (Loi et al., 2013), and therefore the disposition of glucuronide and sulfate metabolites depends on organic anion transporters. Conjugated drug metabolites are considered to have a small impact on drug therapy, because they are typically pharmacologically inactive and facilitate drug excretion from the body. However, some phase II conjugates, such as reactive acyl glucuronide metabolites or glucuronides capable of enzyme inhibition, are known to affect drug efficacy and safety (Ogilvie et al., 2006; Regan et al., 2010; Tornio et al., 2014). Human esterases are capable of cleaving acyl glucuronides and releasing the parent compound from the conjugate (Fukami and Yokoi, 2012). Furthermore, the glucuronide or sulfate groups of drug conjugates can be cleaved by human β-glucuronidases or different sulfatases expressed in tissues, resulting in a release of the parent drug in tissues (Pang et al., 1994; Sperker et al., 1997; Mueller et al., 2015). For example, flavonoid glucuronides may be deconjugated in tissues and subsequently increase local parent exposure (Perez-Vizcaino et al., 2012). The highest deglucuronidation and desulfation activity in the body is found in the intestine within bacteria that express numerous different β-glucuronidases and sulfatases (Pollet et al., 2017; Ervin et al., 2020). Intestinal deconjugation may therefore also prolong parent drug exposure via enterohepatic recycling (Roberts et al., 2002). Thus, understanding the combined effects of uptake and efflux transport on phase II conjugates in different organs is important for predicting drug disposition and possible changes related to altered transporter function.
The aim of this review is to summarize knowledge on the uptake and efflux transport of glucuronide and sulfate conjugates. These conjugates are abbreviated as -G for glucuronide, -AG for acyl glucuronide and -S for sulfate. We focus on drug conjugates, but also include endogenous compounds and natural products. The review highlights the interplay between uptake and efflux in the liver and kidneys, and the role of transporters in glucuronide and sulfate conjugate toxicity, drug-drug interactions (DDIs), pharmacogenetics and species differences are also discussed.
2 UGTs, SULTs and Transporters in Human Tissues
Several UGT and SULT isoforms (e.g. UGT1A1, UGT1A10, UGT2B7, SULT1A1 and SULT1B1) are involved in drug conjugation in human tissues (Oda et al., 2015; Coughtrie, 2016; Basit et al., 2020) (Figure 1). Once formed, these conjugates rely on transporters to cross cell membranes. Transporters are typically divided into two families: Members of the solute carrier (SLC) transporter family function mainly as uptake transporters, whereas ATP-binding cassette (ABC) transporters are primarily efflux transporters (Figure 1). Several members of both families are recognized to play a role in drug disposition and may affect drug safety (Giacomini et al., 2010; Hillgren et al., 2013). These include efflux transporters breast cancer resistance protein (BCRP, ABCG2), P-glycoprotein (P-gp, ABCB1), multidrug and toxin extrusion proteins (MATEs) and multidrug-resistance associated proteins (MRPs) as well as uptake transporters organic anion transporting polypeptides (OATPs), organic anion transporters (OATs) and organic cation transporters (OCTs). The effects of these transporters on drug and conjugate disposition is dependent on the direction of transport and their polarized localization especially in tissues where metabolism takes place.
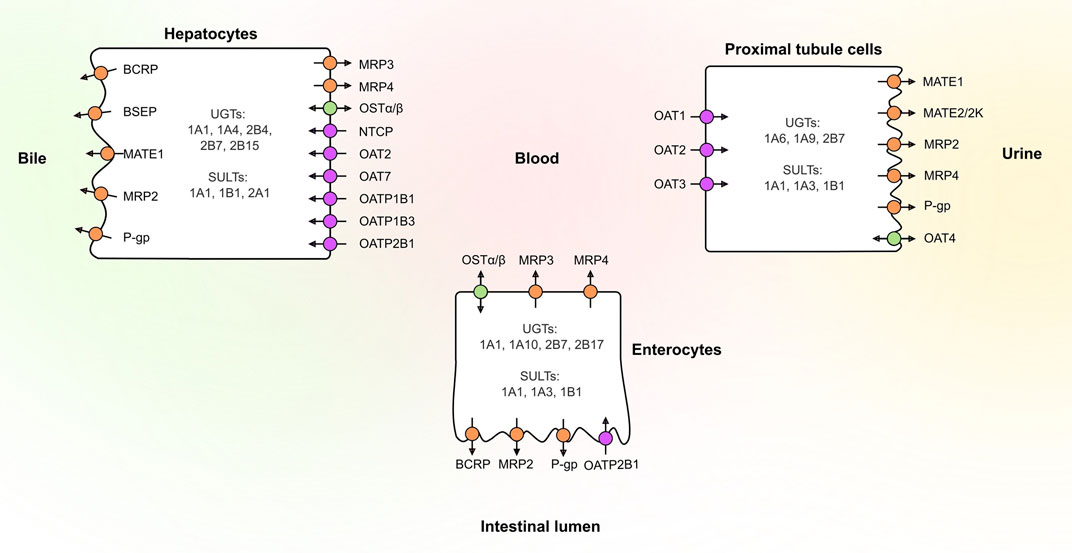
FIGURE 1. Localization of the main drug transporters in the human liver, kidney and intestine as well as the main phase II enzymes involved in glucuronidation and sulfation reactions of drugs. Uptake transporters are colored in purple, efflux transporters in orange, and bidirectional transporters in green. BCRP, breast cancer resistance protein; BSEP, bile salt export pump; MATE, multidrug and toxin extrusion protein; MRP, multidrug-resistance associated protein; NTCP, sodium/taurocholate cotransporting polypeptide; OAT, organic anion transporter; OATP, organic anion transporting polypeptide; OSTα/β, organic solute transporter α/β; P-gp, P-glycoprotein; SULT, sulfotransferase; UGT, uridine 5′-diphospho-glucuronosyltransferase.
2.1 Expression in the Intestine
Intestinal metabolism can markedly decrease the bioavailability of drugs. For instance, the bioavailability of testosterone is limited by intestinal UGT2B17 (Zhang et al., 2018). Other major intestinal UGTs are UGT1A1, UGT1A10 and UGT2B7 (Sato Y. et al., 2014; Zhang et al., 2020). The presence of UGT1A10 in the intestine is notable, since it is absent from the human liver and kidney, but can still contribute significantly to glucuronidation (Cubitt et al., 2011; Troberg et al., 2017). The three main SULTs in the intestine are SULT1A1, SULT1A3 and SULT1B1 (Riches et al., 2009). The uptake transporter that is most likely to affect conjugate absorption is OATP2B1 (SLCO2B1). OATP2B1 is expressed on the apical membrane of enterocytes, but there is controversy on its localization, as some studies suggest basolateral expression (Keiser et al., 2017). The main efflux transporters in the intestine are BCRP, MRP2 (ABCC2), MRP3 (ABCC3), MRP4 (ABCC4) and P-gp (Drozdzik et al., 2019; Harwood et al., 2019). BCRP, MRP2 and P-gp are expressed at the apical membrane, whereas MRP3 is located at the basolateral membrane of enterocytes. MRP4 appears to show basolateral expression alongside MRP3 (Ming and Thakker, 2010). The relative transport rates of conjugates by basolateral and apical transporters determine the fraction of intestinally formed conjugates that reaches the portal vein or is pumped back to intestinal lumen, respectively. These rates may vary along the intestine, with P-gp and BCRP levels increasing towards the distal end of the small intestine, whereas the levels of MRP2, MRP3, and OATP2B1 are similar in different intestinal sections (Drozdzik et al., 2019).
2.2 Expression in the Liver
The liver is a major site of biotransformation and excretion of drugs. Uptake of circulating compounds from the blood into the liver is facilitated on the basolateral (sinusoidal) membranes of hepatocytes by OATP1B1 (SLCO1B1), OATP1B3 (SLCO1B3) and OATP2B1, sodium/taurocholate cotransporting polypeptide (NTCP, SLC10A1), OAT2 (SLC22A7), OAT7 (SLC22A9) and OCT1 (SLC22A1). In the liver, the most abundant UGT is UGT2B7, followed by UGT1A1, UGT1A4, UGT2B4 and UGT2B15 (Fallon et al., 2013; Sato Y. et al., 2014). UGT1A4, and the less abundant UGT2B10, are particularly significant hepatic enzymes, because, unlike most other UGTs, they can catalyze N-glucuronidation of amines. The two main SULTS in the liver are SULT1A1 and SULT2A1 (Riches et al., 2009; Ladumor et al., 2019). Compounds transported into the liver or formed by hepatic metabolism can be effluxed either into blood or excreted into bile. Biliary excretion enables enterohepatic recycling where glucuronide conjugates excreted into bile can be deconjugated by intestinal bacteria and subsequently reabsorbed. On the basolateral membrane of hepatocytes, efflux is mediated by MRP3 and MRP4, whereas biliary excretion is mediated by BCRP, bile salt export pump (BSEP, ABCB11), MRP2, P-gp and MATE1 (SLC47A1) (Giacomini et al., 2010; Hillgren et al., 2013). BSEP, MRP2, MRP3 and P-gp are found at high levels in the liver, while BCRP and MRP4 abundances are typically low in healthy livers (Burt et al., 2016; Kurzawski et al., 2019; Vildhede et al., 2020). However, MRP3 and MRP4 levels have been shown to increase in several liver diseases (Drozdzik et al., 2020; Vildhede et al., 2020), possibly as an alternative efflux route in hepatocytes in the case of dysfunctional MRP2. MRP3/MRP4 efflux is also a likely prerequisite for hydrophilic conjugates, such as diclofenac-AG, cabotegavir-G and sulfonyloxyaristolactam, formed in the liver, to reach the blood circulation and be excreted into urine (Zhang et al., 2016; Chang et al., 2017; Patel et al., 2019). The interplay between basolateral efflux and uptake transporters in the liver results in a phenomenon called hepatocyte hopping, where transporter substrates are shuttled back and forth between the sinusoidal blood and hepatocytes along the sinusoids (Iusuf et al., 2012). This co-operation between transporters could promote the excretion of various compounds by distributing them between hepatocytes and preventing the saturation of metabolism and canalicular efflux. Hepatocyte hopping is thought to protect hepatocytes from the accumulation of metabolites produced in the liver and is important for endogenous toxins such bilirubin and its glucuronides (Iusuf et al., 2012; Sticova and Jirsa, 2013).
2.3 Expression in the Kidney
The kidneys excrete drugs and drug metabolites, in particular hydrophilic conjugates, both through glomerular filtration and transporter-mediated secretion. Conjugates can either be transported into the proximal tubule cells by renal uptake transporters or in some cases formed in the kidney. UGT1A6, UGT1A9 and UGT2B7 are the major renal UGTs (Sato Y. et al., 2014). SULTs (primarily SULT1A1, SULT1A3 and SULT1B1) are expressed in the kidney, but the abundance is much lower than in the intestine or liver (Riches et al., 2009). In the kidney, uptake into proximal tubule cells is primarily mediated by OAT1 (SLC22A6), OAT3 (SLC22A8) and OCT2 (SLC22A2) (Prasad et al., 2016; Li et al., 2019b; Oswald et al., 2019). On the apical membranes of the proximal tubules, MATE1, MRP2, MRP4 and P-gp, are responsible for efflux into urine (Prasad et al., 2016). For example, MRP2 and MRP4 participate in the renal excretion of endogenous glucuronide and sulfate conjugates and may also facilitate excretion of drug conjugates such as glucuronides of non-steroidal anti-inflammatory drugs (NSAIDs), cabotegravir-G and mycophenolic acid glucuronide (MPA-G) (Regan et al., 2010; Matsunaga et al., 2014; Järvinen et al., 2018; Li et al., 2019a; Patel et al., 2019). BCRP, MRP3, MATE2/2K (SLC47A2) and OAT2 are present only at low levels, but may contribute to active renal secretion (Fallon et al., 2016; Prasad et al., 2016; Li et al., 2019b; Cheung et al., 2019; Oswald et al., 2019). Notably, in contrast to OAT1 and OAT3, OAT4 (SLC22A11) is expressed on the apical membrane of the proximal tubules and may mediate reabsorption of organic anions, such as estrone-S, dehydroepiandrosterone-S (DHEAS) and ethinylestradiol-S (Ugele et al., 2008; Han et al., 2010a).
3 Transport of Glucuronide and Sulfate Conjugates
The impact of efflux transporters on the disposition of glucuronide and sulfate conjugates has been reviewed previously (Zamek-Gliszczynski et al., 2006), while an in-depth review of conjugates that interact with uptake transporters is missing. A few examples of phase II drug metabolites as substrates for hepatic and renal uptake transporters have previously been discussed (Zamek-Gliszczynski et al., 2014; Patel et al., 2016). Here we provide an updated comprehensive review of both uptake and efflux transporter interactions with sulfate and glucuronide conjugates. We searched SciFinder and Pubmed databases for literature reports on in vitro data on uptake and efflux transporter interactions with glucuronide and sulfate conjugates of drugs and other compounds from studies in transporter overexpression systems. For efflux transporters, the search was limited to articles published after 2006 as earlier data has been compiled by Zamek-Gliszczynski et al. (2006). The complete results of our search, including reports on animal transporters, are reported in Supplementary Table S1.
3.1 Uptake Transporters in Humans
In the literature search, a high number of drug as well as other xenobiotic or endogenous glucuronides and sulfates were identified as substrates for human organic anion uptake transporters (Table 1, Supplementary Table S1). Hepatic uptake transporters OATP1B1, OATP1B3, OATP2B1 and NTCP, and renal uptake transporters OAT1, OAT3 and OAT4 all transport both glucuronide and sulfate metabolites of small molecule compounds. Other important uptake transporters OAT2, OAT7, OATP1A2 (SLCO1A2), OATP4C1 (SLCO4C1) and organic solute transporter α/β (OSTα/β, SLC51A/B) are not as well characterized in the transport of conjugated drug metabolites and their significance in this context remains to be fully explored (Supplementary Table S1).
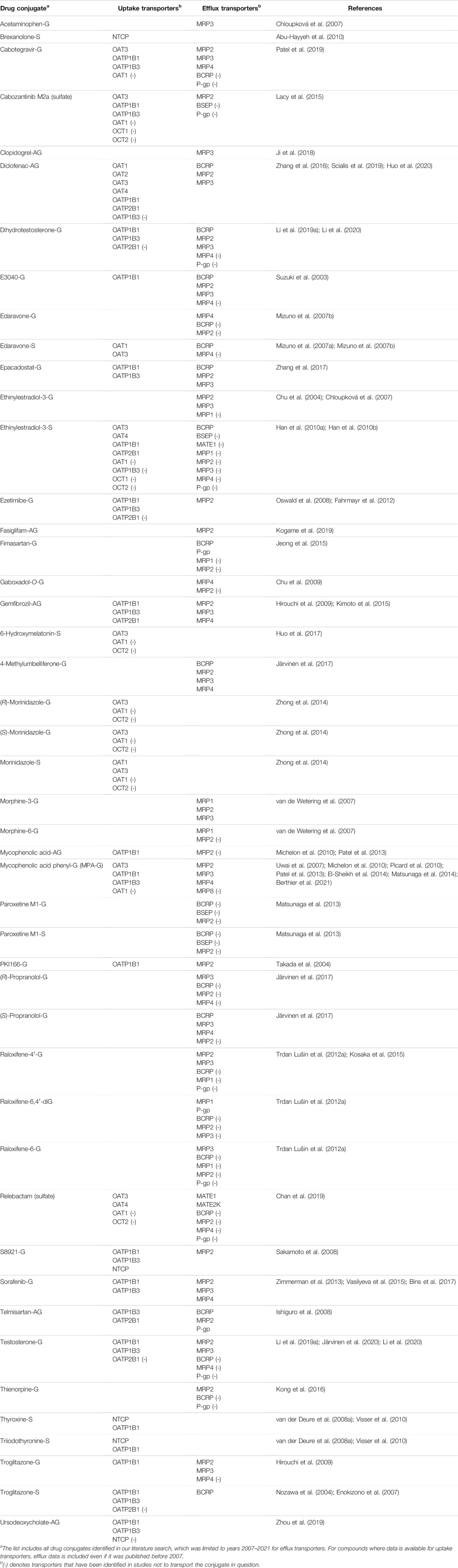
TABLE 1. Drug glucuronides (-G and -AG) and sulfates (-S) studied as substrates in transporter overexpression systems.
Organic cation uptake transporters, OCTs and OCTNs, do not appear to interact with conjugate metabolites. For example, neither glucuronides of estradiol, glycochenodeoxycholate (GCDCA) or morinidazole, nor sulfates of estrone, ethinyl-estradiol or morinidazole are substrates for OCTs (Han et al., 2010a, 2010b; Zhong et al., 2014; Bi et al., 2019; Neuvonen et al., 2021). Furthermore, several glucuronides, such fevipiprant-G, baicalein-7-G and epacadostat-G, were reported not to inhibit OCTs and MATEs, although uremic toxin indoxyl-G inhibits OCT2 (Xu et al., 2013; Cheung et al., 2017; Zhang et al., 2017; Poller et al., 2019). A few reports found in the literature search investigated glucuronide and sulfate conjugates as substrates or inhibitors for other human transporters, such as sodium-dependent organic anion transporter (SOAT, SLC10A6), orphan transporter SLC22A24, apical sodium-dependent bile acid transporter (ASBT, SLC10A2) and other OATPs (e.g. OATP1C1 (SLCO1C1) and OATP4A1 (SLCO4A1)) (Craddock et al., 1998; Tamai et al., 2000; Pizzagalli et al., 2002; Geyer et al., 2007; Sakamoto et al., 2007; van der Deure et al., 2008b; Fietz et al., 2013; Grosser et al., 2015, 2018; Yee et al., 2019).
3.1.1 Hepatic Uptake Transporters OATP1B1, OATP1B3 and OATP2B1
Uptake transporters OATP1B1 and OATP1B3 transport glucuronide conjugates of several drugs and other xenobiotics, such as flavonoids (Table 1, Supplementary Table S1). Both transporters also play a key role in homeostasis of conjugates of endogenous compounds in the liver. Most importantly, bilirubin glucuronides are high affinity substrates for both OATP1B1 and OATB1B3 and thus, these transporters are partly responsible for controlling plasma levels of conjugated bilirubin (König et al., 2000; Cui et al., 2001; van de Steeg et al., 2010, 2012). Other endogenous conjugate substrates for these transporters include bile acid and steroid conjugates, such as ursodeoxycholate-AG, GCDCA-G and GCDCA-S, glycodeoxycholate-G (GDCA-G) and estradiol-17-G (Takehara et al., 2017; Bi et al., 2019; Zhou et al., 2019; Neuvonen et al., 2021). Glucuronides of several drugs, such as ezetimibe, gemfibrozil and sorafenib, are transported by OATP1B1 and OATP1B3, which may contribute to the enterohepatic recycling of these drugs by directing the excretion of metabolites to the bile and feces, instead of excretion into the urine (Oswald et al., 2008; Hirouchi et al., 2009; Zimmerman et al., 2013; Kimoto et al., 2015; Bins et al., 2017). Similarly, metabolites of therapeutically used hormones, including testosterone-G, dihydrotestosterone-G and ethinylestradiol-S are transported by OATP1B1 or OATP1B3 (Han et al., 2010b; Li et al., 2020).
The transport of drug conjugates by OATP2B1 is not as extensively characterized as for OATP1Bs. OATP2B1 is more widely expressed than the OATP1Bs, and it may therefore affect drug disposition also in the intestine and blood vessels of the heart, brain and other tissues (McFeely et al., 2019). In our literature search, only a few drug glucuronides or sulfates were identified as substrates of OATP2B1 (Table 1). These include ethinylestradiol-S, gemfibrozil-AG, telmisartan-AG and diclofenac-AG (Ishiguro et al., 2008; Han et al., 2010b; Kimoto et al., 2015; Zhang et al., 2016). Estrone-S is an excellent substrate for OATP2B1, while this transporter does not transport estradiol-17-G (Tamai et al., 2001; Bi et al., 2019). Within natural compounds, scutellarein-7-G in particular is a good substrate for OATP2B1, and could even be a specific substrate for this transporter as this compound is not transported by other hepatic organic anion transporters (Gao et al., 2012). Similarly, resveratrol-3-G is highly transported by OATP2B1, weakly by OATP1B1 and OATP1B3 but not by OAT2 or NTCP (Bi et al., 2019). Lastly, some drug conjugates that are transported by OATP1B1 and OATP1B3, such as ezetimibe-G and troglitazone-S, are not OATP2B1 substrates (Nozawa et al., 2004; Oswald et al., 2008).
3.1.2 Hepatic Transporters NTCP and OSTα/β
NTCP is primarily a bile acid transporter, but it can also transport several conjugate metabolites with steroid structures. For example, sulfates of estrone, ethinylestradiol, GCDCA and brexanolone are substrates for NTCP (Abu-Hayyeh et al., 2010; Han et al., 2010b; Takehara et al., 2017). Most studies with NTCP, regarding conjugates, have focused on sulfate metabolites and only one glucuronide, chenodeoxycholate-AG (CDCA-AG), is reported to be a substrate for NTCP (Takehara et al., 2017) (Supplementary Table S1). Similarly, the bidirectional bile acid transporter OSTα/β may have a role in the transport of sulfated metabolites. DHEAS, estrone-S and pregnenolone-S are substrates of OSTα/β, but estradiol-17-G is not (Seward et al., 2003; Ballatori et al., 2005; Fang et al., 2010; Malinen et al., 2019).
3.1.3 Hepatic Uptake Transporters OAT2 and OAT7
Only three conjugate substrates of OAT2 were identified in our literature review. DHEAS and diclofenac-AG are weakly transported by OAT2 (Kobayashi et al., 2005; Zhang et al., 2016), while other studies could not identify OAT2-mediated transport of DHEAS (Hotchkiss et al., 2015; Mathialagan et al., 2018). In contrast, estrone-S was identified as a rather good substrate for OAT2 (Kobayashi et al., 2005; Xu et al., 2013; Mathialagan et al., 2018; Bi et al., 2019). Several glucuronides and sulfates of natural and endogenous compounds, such as quercetin-S and resveratrol-G, estradiol-17-G and glucuronides of GCDCA and GDCA are not transported by OAT2 (Wong et al., 2011a, 2012; Xu et al., 2013; Bi et al., 2019; Neuvonen et al., 2021).
Little is known about OAT7-mediated transport of conjugates. The only identified conjugate substrates of OAT7 are DHEAS and estrone-S (Shin et al., 2007; Ahn et al., 2015; Mathialagan et al., 2018). Inhibition studies also indicate low interaction between conjugates and OAT7. For example, acetaminophen-G, 4-methylumbelliferone-G and vincristine-S did not inhibit OAT7, while minoxidil-S, vinblastine-S and 4-methylumbelliferone-S inhibited OAT7 moderately at best (Shin et al., 2007).
3.1.4 Renal Uptake Transporters OAT1, OAT3 and OAT4
OAT1 and OAT3 transport some glucuronide and sulfate conjugates of endogenous compounds and xenobiotics, but only a small number of drug conjugates have been identified as their substrates (Table 1, Supplementary Table S1). Morinidazole-S, edaravone-S and diclofenac-AG are transported by both OAT1 and OAT3 (Mizuno et al., 2007a; Zhong et al., 2014; Zhang et al., 2016; Huo et al., 2020). On the other hand, OAT3, but not OAT1, transports ethinylestradiol-S and glucuronides such as of cabotegravir-G, curcumin-G, genistein-7-G, steviol-G and MPA-G (Uwai et al., 2007; Han et al., 2010a; Wong et al., 2011b; Wang M. et al., 2015; Zhou et al., 2017; Patel et al., 2019). Moreover, OAT3, but not OAT1, transports endogenous sulfates estrone-S and DHEAS (Ueo et al., 2005) and relebactam, which is a drug molecule containing a sulfate group (Chan et al., 2019). Taken together, it appears that OAT3 may have a more significant role in the renal uptake of glucuronide and sulfate conjugates than OAT1.
The transport profile of OAT4 towards glucuronide or sulfate conjugates has been examined only in a few studies. Of drug conjugates, OAT4 transports ethinylestradiol-S, diclofenac-AG and relebactam (Han et al., 2010a; Zhang et al., 2016; Chan et al., 2019). Other sulfate conjugates transported by OAT4 include quercetin-3′-S and uremic toxin indoxyl-S (Enomoto et al., 2003; Wong et al., 2012). In particular, endogenous sulfates DHEAS, 16α-hydroxy-DHEAS and estrone-S are good substrates for OAT4 (Ugele et al., 2008; Schweigmann et al., 2014). Since OAT4 is localized in the apical membranes of proximal tubule cells, this transporter may have a role in the renal reabsorption of sulfate conjugates. Interestingly, an orphan transporter encoded by the SLC22A24 gene, and highly homologous to OAT4, was recently identified as a potential renal apical reabsorption transporter for glucuronide and sulfate conjugates of steroids (Yee et al., 2019).
3.2 Efflux Transporters in Humans
The role of efflux transporters on phase II conjugate disposition in the liver has been reviewed previously (Zamek-Gliszczynski et al., 2006), but the understanding of conjugate transport has increased within the last 15 years. At the time of the previous review, many endogenous and natural compound conjugates were well-characterized substrates of BCRP, MRP2 and MRP3, but only a handful of drug conjugates had been identified as their substrates in vitro (Zamek-Gliszczynski et al., 2006). Since then, numerous drug conjugates as well as conjugated natural compounds that are substrates of these and other efflux transporters have emerged and are discussed below.
3.2.1 MRPs
The majority of drug glucuronides reported after 2006 as efflux substrates are transported by MRP2 and MRP3 (Table 1). MRP2 plays an important role in the biliary excretion of many endogenous organic anions, including estradiol-17-G and bilirubin-Gs (Cui et al., 1999; Kamisako et al., 1999). Based on the current literature, MRP2 may also participate in the disposition of several drug glucuronides. Like OATP1Bs, MRP2 transports ezetimibe-G, MPA-G, sorafenib-G and telmisartan-G, and may contribute to their enterohepatic recycling (Ishiguro et al., 2008; Fahrmayr et al., 2012; Patel et al., 2013; Vasilyeva et al., 2015). In line with previous findings (Zamek-Gliszczynski et al., 2006), MRP2 does not appear to be a prominent sulfate conjugate transporter as only a single sulfated drug metabolite (cabozantinib M2a) and two sulfated natural compounds were identified as substrates of MRP2 in our literature review (Table 1, Supplementary Table S1). In addition to drug metabolites, MRP2 is able to transport flavonoid glucuronides present in herbal medicines (e.g. baicalein-7-G, scutellarein-Gs and wogonin-7-G). MRP2 also transports glucuronides and sulfates of resveratrol, which is found in foods, such as grapes, and used as a herbal supplement (Li et al., 2006; Novelle et al., 2015).
MRP3 is an important transporter for many glucuronide conjugates: Altogether 18 drug glucuronides were identified as MRP3 substrates and only one tested drug glucuronide was reported not to be transported (Table 1). MRP3 transports a wide number of sulfated bile acids (Zelcer et al., 2003; Murai et al., 2013), but otherwise the disposition of sulfated xenobiotics by MRP3 is poorly characterized, and we identified only a single sulfate conjugate (sulfonyloxyaristolactam) as an MRP3 substrate (Chang et al., 2017) (Supplementary Table S1). There is a high degree of overlap between substrates of MRP2 and MRP3, but MRP3 is the only transporter identified to transport acetaminophen-G, clopidogrel-AG and (R-)-propranolol-G (Chloupková et al., 2007; Järvinen et al., 2017; Ji et al., 2018). In addition to drug conjugates, MRP3 transports a wide range of glucuronidated endogenous and natural compounds (Supplementary Table S1). Since MRP3 expels its substrates towards the blood, it can facilitate the entry of conjugates into the systemic circulation and increase their plasma concentrations. For instance, MRP3 substrates epacadostat-G, raloxifene-Gs and scutellarein-6-G all have plasma levels an order of magnitude higher than that of their parent compounds (Chen et al., 2006; Sun et al., 2013; Boer et al., 2016).
MRP4 is reported to transport glucuronides as well as several sulfate conjugates. Since the previous review (Zamek-Gliszczynski et al., 2006), eight glucuronidated drug metabolites have been identified as MRP4 substrates, including several substrates shared with MRP2 and MRP3 (e.g. cabotegravir-G, gemfibrozil-AG, MPA-G and sorafenib-G) (Table 1). In addition, MRP4 transports edaravone-G and gaboxadol-O-G, neither of which are transported by MRP2 (Mizuno et al., 2007b; Chu et al., 2009). With respect to sulfated drug conjugates, no substrates have been reported for MRP4 in vitro. In contrast, several sulfate conjugates of flavonoids (e.g. chrysin-S) are transported by MRP4, and DHEAS is also an MRP4 substrate (Li et al., 2015; Sun et al., 2015; Järvinen et al., 2017; Kanamitsu et al., 2017). The capability of MRP4 to transport various sulfate conjugates suggests that MRP4 might participate in the efflux of other, yet unidentified sulfate metabolites of drugs.
Limited information is available regarding phase II conjugate transport of other MRPs expressed in different tissues. There are few studies on the ability of MRP1 to transport phase II drug conjugates. Morphine-3-G and morphine-6-G have been shown to be transported by MRP1 in vitro (van de Wetering et al., 2007). MRP1 also transports wogonin-7-G, estrone-S and estradiol-17-G (Maeno et al., 2009; Wang et al., 2018). On the other hand, no drug sulfate conjugates were reported to be transported by or inhibit MRP1. Additionally, a limited number of studies investigated the involvement of MRP5 (ABCC5), MRP6 (ABCC6), MRP7 (ABCC10), and MRP8 (ABCC11) in the transport of various glucuronide and sulfate conjugates (Supplementary Table S1), but only MRP7 was found to transport a phase II conjugate, estradiol-17-G (Malofeeva et al., 2012).
3.2.2 BCRP
BCRP was previously reported to transport both glucuronides and sulfates of 4-methylumbelliferone and E3040 (Zamek-Gliszczynski et al., 2006). Several new drug conjugate substrates have been identified, such as diclofenac-AG, raloxifene-Gs, telmisartan-AG and troglitazone-S (Table 1). Furthermore, numerous flavonoid and endogenous compound glucuronides and sulfates are known to be transported by BCRP (Supplementary Table S1). Compared to MRPs, BCRP appears to have more sulfate conjugate substrates (Supplementary Table S1). Furthermore, whereas estradiol-17-G is a typical in vitro probe substrate of MRPs, estrone-S is preferred for BCRP (Elsby et al., 2011; Brouwer et al., 2013; Pedersen et al., 2017). Similarly, 17α-ethinylestradiol-3-S is a substrate of BCRP, but it is not transported by any of the tested MRPs (Han et al., 2010b). BCRP is, however, capable of transporting several glucuronide conjugates of estrogens (Järvinen et al., 2018).
3.2.3 BSEP
BSEP is expressed exclusively in hepatocytes, where it excretes bile acids into the bile canaliculi and maintains the bile flow. Only few drugs (pravastatin, fexofenadine) are known substrates of BSEP (Hirano et al., 2005; Matsushima et al., 2008), and no glucuronide or sulfate conjugated drugs have been reported to be transported by BSEP. However, glycyrrhizin, which is a diglucuronide of enoxolone extracted from licorice root, has been identified as a BSEP substrate (Dong et al., 2018).
3.2.4 P-gp
P-gp has a negatively charged binding pocket, which is thought to repel anionic compounds (Li et al., 2014; Deng et al., 2020) and is therefore an unlikely candidate for glucuronide and sulfate transport. This is supported by a plethora of studies where conjugates were shown not to be transported by P-gp (Table 1). While a few in vitro reports suggest that some endogenous compound and drug glucuronides are P-gp substrates, the involvement of P-gp in the disposition of conjugates is likely low (Supplementary Table S1).
3.2.5 MATEs
Similarly to P-gp, the anionic properties of glucuronide and sulfate conjugates are outside the substrate preferences of MATE1 and MATE2K. One sulfate, relebactam, has been shown to be transported by MATE1 and MATE2K, and not by other drug efflux transporters (e.g. BCRP) (Chan et al., 2019). Additionally, estrone-S is transported by MATE2K, but data on MATE1 transport is contradictory (Tanihara et al., 2007; Shen et al., 2016).
4 Interplay of Uptake and Efflux Transport in Drug Conjugate Disposition
Phase II metabolism in the liver, intestine and kidney can greatly affect drug exposure. The systemic exposure of conjugated metabolites depends on the metabolic clearance of a parent drug in tissues and the interplay of different transporters expressed on the basolateral and apical membranes of cells (Figure 1 and Figure 2). For instance, some conjugates formed in the intestine may reach the systemic circulation only at low levels if they have high net transport into the intestinal lumen from enterocytes or into hepatocytes over the hepatic basolateral membrane (i.e. high uptake and low efflux). Moreover, high net transport into hepatocytes combined with high biliary efflux typically leads to biliary excretion of glucuronides, which contributes to enterohepatic recycling and prolonged half-life of the parent compound (Figure 2A). In contrast, low hepatic uptake and biliary efflux, together with high basolateral efflux, leads to glucuronide levels that may exceed the levels of the parent drug in plasma, and typically result in urinary excretion of the drug conjugate (Figure 2B). Using four examples, below we highlight how consideration of the interplay of uptake and efflux transporters in conjugate transport can help to explain the observed pharmacokinetic properties of drugs.
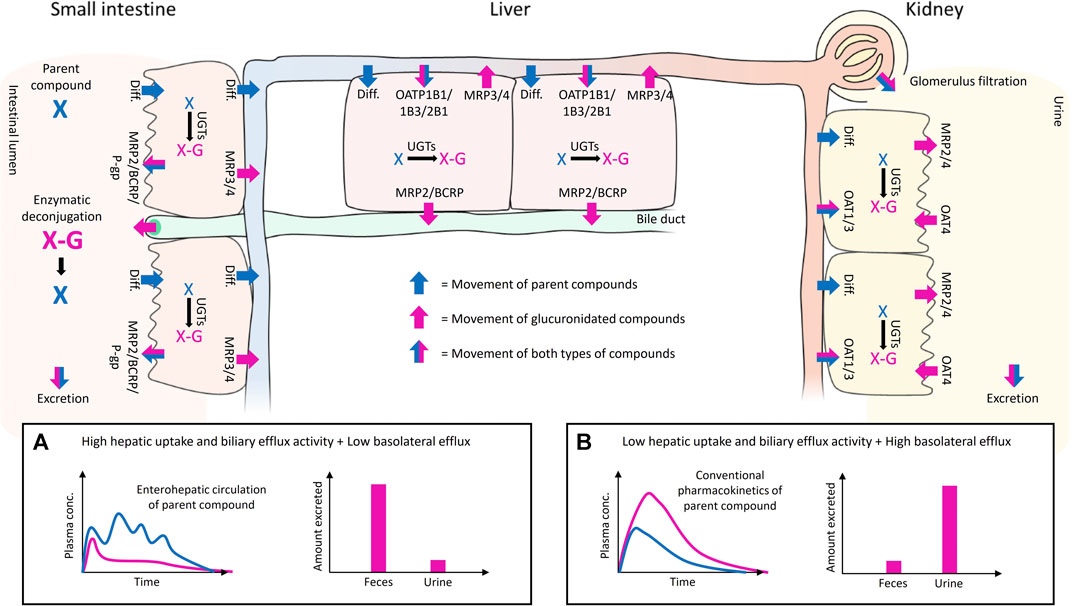
FIGURE 2. Illustration of the interplay of uptake and efflux transporters in the small intestine, liver, and kidney after oral administration of a parent compound that undergoes glucuronidation. The movement of the parent and its glucuronide conjugate are shown with blue and pink arrows, respectively. The movement of both compounds is shown with half-blue/half-pink arrows. The UGT-mediated glucuronidation of the parent and the enzymatic deconjugation of glucuronidated compounds by bacterial β-D-glucuronidases in the gut are indicated with black arrows. The passive diffusion of compounds over membranes is abbreviated as Diff., and the transporters mentioned in the illustration are considered to be the most relevant transporters for glucuronide disposition in that particular tissue. Examples of uptake and efflux transporter interplay on the disposition of the parent and its glucuronide conjugate are presented in subpanels (A,B). High hepatic uptake and biliary efflux activity, and low hepatic basolateral efflux (A) leads to enterohepatic recycling of parent compound (blue line) and favors the fecal excretion of glucuronide conjugate (pink bar). Low hepatic uptake and biliary efflux activity, and high basolateral efflux (B) leads to elevated plasma concentration and renal excretion of the glucuronidated compound (pink line and bar).
4.1 Sorafenib-G
Sorafenib is a tyrosine kinase inhibitor that is primarily metabolized by CYP3A4 to sorafenib N-oxide and by UGT1A9 to sorafenib-G (Food and Drug Administration, 2005; Lathia et al., 2006). However, sorafenib exposure was unaltered by ketoconazole in humans, indicating a minor role for oxidative metabolism in its clearance. Approximately 15% of orally administered sorafenib is excreted as sorafenib-G in urine in humans, but most of the dose (77%) is recovered in feces primarily as unchanged drug (50%). Enterohepatic recycling contributes to the pharmacokinetics and long half-life (25–48 h) of sorafenib. Sorafenib-G is a substrate for human MRP2, MRP3 and MRP4 (Table 1), which means that it can both be effluxed into the blood and undergo biliary excretion in the liver, as demonstrated in sandwich-cultured human hepatocytes (Swift et al., 2013). Sorafenib-G is also a substrate of OATP1B1 and OATP1B3 (Table 1), which together with MRP3-mediated hepatocyte hopping may explain the low levels of sorafenib-G found in human plasma (European Medicines Agency, 2006; Vasilyeva et al., 2015). Moreover, UGT1A9 is highly expressed in the kidney and it is likely that direct renal glucuronidation may contribute to the urinary excretion of sorafenib-G. The low levels of sorafenib-G in feces may be explained by bacteria-mediated deconjugation of the conjugate in the intestine. This is supported by a 54% reduction in sorafenib area under the concentration time curve (AUC) after administration of the antibiotic neomycin in humans (European Medicines Agency, 2006), which also highlights the role of sorafenib-G in the enterohepatic recycling and prolonged exposure of sorafenib.
4.2 Raloxifene-Gs
Raloxifene is a selective oestrogen receptor modulator indicated for osteoporosis treatment and prevention in postmenopausal women. It has low absolute bioavailability (2%) due to extensive first-pass glucuronidation and most of the raloxifene in human plasma is in the form of raloxifene-4′-G, raloxifene-6-G and raloxifene-6,4′-di-G (Hochner-Celnikier, 1999; Snyder et al., 2000; Trdan Lušin et al., 2012b). Unconjugated raloxifene represents less than 1% of total drug material in human plasma. The half-life of raloxifene is long (30 h) and secondary plasma peaks of raloxifene appear in human plasma indicating enterohepatic recycling. The main excretion pathway of raloxifene and its glucuronides is in feces and less than 6% of the dose is excreted as glucuronides in urine. Intestinal metabolism contributes significantly to raloxifene clearance and even exceeds the clearance measured in human liver microsomes in vitro (Gufford et al., 2015). From enterocytes, raloxifene-4′-G and raloxifene-6-G can reach the portal circulation via MRP3-mediated transport, whereas MRP2 could efflux raloxifene-4′-G to the intestinal lumen (Trdan Lušin et al., 2012a; Kosaka et al., 2015). No reports on uptake transport of raloxifene glucuronides are available, but raloxifene-4′G and raloxifene-6,4′-di-G strongly inhibit OATP1B1 and OATP1B3 (Table 2), showing that these glucuronides interact with OATPs, which may mediate their hepatic uptake. In the liver, raloxifene-Gs are likely to be excreted into bile by MRP2. While MRP3 can efflux raloxifene-Gs into blood from hepatocytes, the high recovery of administered raloxifene in feces supports predominant biliary excretion as a consequence of high MRP2 transport and high net uptake into hepatocytes, likely mediated by OATPs. Although raloxifene undergoes sulfate conjugation in human enterocytes, intestinal microsomes and liver microsomes, raloxifene sulfates were not detected in human plasma (Food and Drug Administration, 1999; Hui et al., 2015; Davies et al., 2020). The systemic absorption of raloxifene sulfates may be limited by apical efflux transporters (Jeong et al., 2004; Zhou et al., 2015).
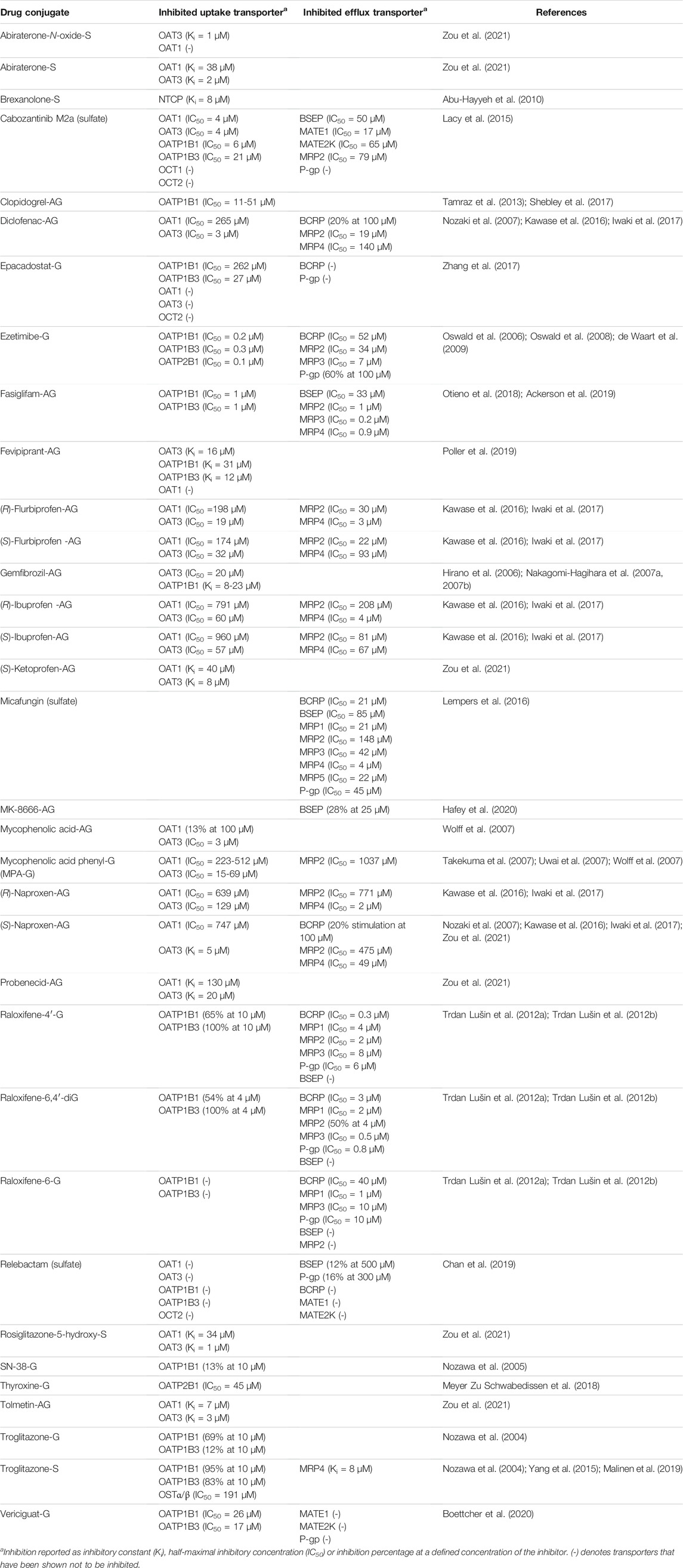
TABLE 2. Drug glucuronides (-G and -AG) and sulfates (-S) studied as inhibitors in transporter overexpression systems.
4.3 Epacadostat-G
Epacadostat is an indoleamine 2,3-dioxygenase inhibitor being developed for cancer treatment. Its most abundant metabolite is epacadostat-G, which is formed by UGT1A9 in the liver. At steady state, epacadostat-G is found at 8-fold levels compared to epacadostat in human plasma, which can be explained by efficient basolateral efflux by MRP3 (Boer et al., 2016; Zhang et al., 2017). Epacadostat exhibits a pharmacokinetic profile with double peaking that indicates enterohepatic recycling of the drug. Epacadostat-G is likely involved in this recycling as it is excreted into bile by MRP2 and BCRP and hydrolyzed completely in incubations with human feces (Boer et al., 2016; Zhang et al., 2017). Epacadostat-G is also a substrate of OATP1B1 and OATP1B3, which may enhance its biliary excretion and contribution to the enterohepatic recycling of epacadostat (Zhang et al., 2017). The renal clearance of epacadostat and its metabolites is minimal at least in preclinical species (Zhang et al., 2017), but human excretion data is not available.
4.4 Cabotegravir-G
Cabotegravir is a newly approved integrase strand transfer inhibitor for HIV treatment, which is glucuronidated primarily in the liver by UGT1A1 and UGT1A9 to form cabotegravir-G (Bowers et al., 2016). Cabotegravir-G is a substrate of MRP2, and cabotegravir-G was found in the bile of some human subjects, whereas the parent drug was found in all bile samples in line with its primary excretion in feces (47%) in humans (Bowers et al., 2016; Patel et al., 2019). Cabotegravir-G also undergoes sinusoidal efflux by MRP3 and MRP4 (Patel et al., 2019). Approximately 20% of the oral dose is recovered in urine as cabotegravir-G, but cabotegravir-G levels in the systemic circulation are negligible (Bowers et al., 2016). This behavior is explained by efficient renal elimination of cabotegravir-G that is mediated by OAT3 on the apical and MRP2 and MRP4 on the basolateral membranes of renal proximal tubule cells (Patel et al., 2019). In addition, renal glucuronidation of cabotegravir by UGT1A9 and direct efflux of the metabolite into urine may contribute to the renal excretion of cabotegravir-G.
5 Conjugates as Inhibitors of Transporters
Transporters are known to mediate DDIs (Gessner et al., 2019). In vitro inhibition studies can identify compounds that affect transporter activity and might cause DDIs or other transporter-mediated toxicity. A list of transporter inhibitors within glucuronide and sulfate conjugates are compiled in Table 2 for drug conjugates and the full list is available in Supplementary Table S1. Among phase II conjugates, some strong inhibitors of hepatic uptake and efflux transporters have been identified. For example, ezetimibe-G, a substrate of OATPs and MRP2, is also a strong inhibitor of OATPs (half maximal inhibitory concentration (IC50) <0.5 µM) and can inhibit BCRP, MRP2 and MRP3 (Oswald et al., 2008; de Waart et al., 2009). Another drug conjugate with strong inhibition potential for both OATP1Bs and MRPs is fasiglifam-G, which has IC50 values ≤1 µM for these transporters (Otieno et al., 2018; Ackerson et al., 2019). Raloxifene-4′-G and raloxifene-6,4′-di-G appear able to inhibit all of the major hepatic drug transporters with IC50 values <10 µM, but interpretation warrants caution as values for the efflux transporters are based on changes in ATPase activity and not inhibition of transport (Trdan Lušin et al., 2012a; 2012b). Interestingly, the third raloxifene glucuronide, raloxifene-6-G, did not inhibit OATP1B1, OATP1B3 or MRP2.
Reports on transporter inhibition by drug sulfates are scarce, but the sulfate conjugate of a metabolite of tyrosine kinase inhibitor cabozantinib (cabozantinib M2a) inhibits efflux transporters BSEP, MATE1, MATE2K and MRP2 with IC50 values of 17–79 µM (Lacy et al., 2015). Cabozantinib M2a also inhibits renal and hepatic uptake transporters OAT1, OAT3, OATP1B1 and OATP1B3 with IC50 values of 4–21 µM (Lacy et al., 2015). Micafungin, a large antifungal compound which contains a sulfate group, inhibits BCRP, BSEP, P-gp, and several MRPs (Lempers et al., 2016). The strongest inhibition was towards MRP4 with an IC50 value of 4 µM. MRP4 and rat Bsep are inhibited by troglitazone-S, which contributes to the hepatotoxicity of troglitazone (Funk et al., 2001; Masubuchi, 2006; Yang et al., 2015). Importantly, despite having a low number of drug substrates, BSEP is susceptible to drug-induced inhibition, which may lead to intrahepatic accumulation of bile acids and drug-induced liver injury (Kenna et al., 2018). Therefore, the inhibitory potential of conjugate metabolites should be considered.
In the kidney, acyl glucuronides of flurbiprofen, ibuprofen and naproxen inhibit OAT3 with IC50 values 19–129 µM (Iwaki et al., 2017). Stronger inhibition (IC50 <3 µM) was observed by diclofenac-AG, tolmetin-AG and mycophenolic acid-AG (Wolff et al., 2007; Iwaki et al., 2017; Zou et al., 2021). Sulfate conjugates may also strongly inhibit OAT3. Sulfates of abiraterone, abiraterone-N-oxide and 5-hydroxy-rosiglitazone inhibit OAT3 with inhibitory constant (Ki) values below 2 µM (Zou et al., 2021). Although OAT1 has fewer conjugate substrates than OAT3, conjugated metabolites can inhibit OAT1. Diclofenac-AG, flurbiprofen-AG, naproxen-AG and ibuprofen-AG have IC50 values between 174–960 µM towards OAT1. More potent OAT1 inhibitors are abiraterone-S, (S)-ketoprofen-AG, rosiglitazone-5-hydroxy-S and tolmetin-AG with Ki values between 7–40 µM (Zou et al., 2021).
Many natural compounds found either in foods or herbal supplements are known to inhibit transporters (Supplementary Table S1). BCRP and MRP2, in particular, are inhibited by several glucuronide and sulfate conjugated natural compounds. For example, chrysin-7-G, hesperitin-3′-G, hesperitin-7-G and quercetin-3-G all inhibit BCRP and MRP2 with IC50 <50 µM. Quercetin-Gs also inhibit OAT1 and OAT3 with IC50 values even below 1 µM (Wong et al., 2011b). Baicalein-7-G is a well-characterized inhibitor of both uptake and efflux transporters, with IC50 values <20 µM for BCRP, MRP3, MRP4 OATP1B3, OATP2B1, OAT3 and OAT4 (Xu et al., 2013; Kalapos-Kovács et al., 2015). Scutellarein-7-G (scutellarin) is an inhibitor of OATP2B1 with IC50 of 2–5 µM (Wen et al., 2016; Iijima et al., 2018) and it also inhibits BCRP and MRP2 (Gao et al., 2012). Chrysin-7-S is a potent inhibitor of BCRP, OATP1B1 and OATP2B1 (IC50 <1 µM) and inhibits MRP2, but not as well as chrysin-7-G (Mohos et al., 2020a). Furthermore, quercetin-3′-S inhibits OATP1B1, OATP2B1, OAT1 and OAT3 with IC50 <1 µM (Wong et al., 2011b; Mohos et al., 2020b).
6 Clinical Drug Interactions Involving Conjugates
To date, little data is available on clinically significant DDIs, in which conjugates act as victims or perpetrators. Given the role of transporters in drug disposition and the potential of glucuronide and sulfate metabolites to inhibit these transporters in vitro (Table 2), clinical DDIs might be mediated by phase II metabolites. Inhibition of conjugate transport, on the other hand, may reduce excretion or enterohepatic recycling of the drug conjugates, which can increase conjugate levels, but decrease exposure of the parent and thus reduce treatment efficacy. DDIs can be of special concern for phase II conjugates as victim drugs for those drugs with potentially serious side effects, such as opioid or kinase inhibitor conjugates (e.g. morphine-6-G and sorafenib-G). Several of the known drug interactions involving drug conjugates (e.g. disruption of enterohepatic recycling of MPA-G by cyclosporine or inhibition of BSEP mediated biliary excretion of bile acids by troglitazone-S) have been reviewed previously (Zamek-Gliszczynski et al., 2014; Patel et al., 2016), but some new findings are discussed below.
6.1 Sorafenib-G: Altered Conjugate Disposition due to Transporter Inhibition
As described in Section 4.1, sorafenib-G is excreted into bile by MRP2 and undergoes OATP1B1- and OATP1B3-mediated reuptake into hepatocytes, after basolateral efflux by MRP3, to enhance biliary excretion. When this re-uptake was interrupted with the OATP inhibitor rifampicin (600 mg once daily twice) in healthy volunteers, the systemic exposure to sorafenib-G rose over 2-fold, while there were no significant differences in sorafenib parameters (Bins et al., 2017). Increased sorafenib-G exposure was also observed in Oatp1b2-deficient mice, indicating that OATP inhibition was the mechanism of the observed rifampicin interaction (Bins et al., 2017). This serves as an example of in vivo OATP inhibition affecting conjugate disposition. Long-term treatment with rifampicin leads to a decrease in sorafenib AUC by 37% (Food and Drug Administration, 2005), which may be caused by several reasons, such as induction of glucuronidation and hepatic efflux transport. However, many compounds can inhibit OATPs (Karlgren et al., 2012) and not all of them are inducers of metabolic enzymes or transporters. Recently, administration of probenecid with sorafenib was shown to increase the ratio of sorafenib-G to sorafenib in plasma due to a reduction in sorafenib exposure (Hussaarts et al., 2020). This was suggested to be caused by disrupted enterohepatic recycling caused by inhibition of OATP1B1, as with rifampicin, but surprisingly the plasma concentrations of the glucuronide were unaffected.
6.2 Clopidogrel-AG: Inhibition of Transport by a Drug Conjugate
Even though conjugate-mediated transporter inhibition might not be the primary mechanistic source of many DDIs, they can be complicit in adding to complex DDIs. Clopidogrel-AG is a mechanism-based inhibitor of CYP2C8, but also a substrate and inhibitor of transporters (Table 1 and Table 2, (Tornio et al., 2014)). Co-administration of clopidogrel increased repaglinide AUC by 5.1-fold (Tornio et al., 2014). While mechanism-based inhibition of CYP2C8 by clopidogrel-AG is the primary contributor to this interaction, OAT1B1 inhibition by clopidogrel and its acyl glucuronide increases the severity of the interaction by 1.5-fold based on pharmacokinetic simulations. Although there are no clinical reports, MRP3 function may also affect this interaction by modulating the intracellular concentrations of clopidogrel-AG. Indeed, the liver-to-plasma ratio of clopidogrel-AG was 11-fold higher in Mrp3 knockout mice compared to wild type, supporting the role of MRP3 in sinusoidal efflux (Ji et al., 2018). This was observed also in a clinical setting, where the plasma AUC of clopidogrel-AG was 1000 times higher than of the parent (Tornio et al., 2014). It is unknown if clopidogrel-AG itself undergoes re-uptake into hepatocytes by uptake transporters from the blood circulation.
6.3 Conjugates as Biomarkers of Drug Inhibition
The use of endogenous transporter substrates as biomarkers of transporter function can help to avoid dedicated DDI studies and be used to assess DDI risks of drugs that have ethical and practical limitations when it comes to conventional DDI trials (Chu et al., 2018). Many endogenous conjugates, primarily bile acid conjugates, have been studied for their utility as biomarkers to assess the inhibitory effects of drugs on transporters. Although this may be challenging considering the overlap between transporters, some promising conjugates have been identified, especially for OATP1Bs.
The bile acid conjugate GCDCA-S has been proposed as a biomarker for several transporters. As a biomarker of OAT1/OAT3-mediated DDIs, probenecid reduced the renal clearance of GCDCA-S in a dose-dependent manner. The degree of reduction was similar to changes for the OAT1/OAT3 probe drug benzyl penicillin, even though apically expressed MRP2 in the proximal tubules might also be involved in the decreased GCDCA-S excretion (Tsuruya et al., 2016). GCDCA-S and other bile acid conjugates have also been studied as OATP1B biomarkers for detecting DDIs. A 600 mg single dose of rifampicin increased GCDCA-S AUC over 20-fold (Takehara et al., 2018). A dose-dependent effect of rifampicin on the AUCs of GCDCA-S, GCDCA-G and CDCA-AG was later confirmed (Mori et al., 2020b). The utility of bile acid conjugates as biomarkers for OATP1B-mediated DDIs was additionally investigated with paclitaxel at therapeutic doses in non-small cell lung cancer patients (Mori et al., 2020a). In this study, the AUC of several sulfate and glucuronide conjugates (e.g. GCDCA-S, GCDCA-G, CDCA-AG and GDCA-S) increased over 2.5-fold with paclitaxel administration. It should be noted however, that some of these compounds are also substrates of efflux transporters (Neuvonen et al., 2021), which may complicate interpretation. Clinical studies to identify biomarkers for OATP1B3 have not yet been conducted, but testosterone-G and androsterone-G were recently identified to be primarily transported by OATP1B3 and have been proposed as potential OATP1B3 biomarkers (Li et al., 2020). Plasma levels of DHEAS, a testosterone precursor, appear to be insensitive towards OATP1B inhibition by rifampicin in humans (Shen et al., 2017).
7 Conjugate Toxicity Involving Transporter-Mediated Disposition
Some phase II conjugates have been linked to drug toxicity, since molecules containing carboxylic acid moieties can be metabolized into reactive, electrophilic acyl glucuronides and may thus require safety assessment (Food and Drug Administration, 2020). Acyl glucuronides are formed from many widely used drugs (e.g. NSAIDs, mycophenolic acid, valproic acid and gemfibrozil) and they can covalently bind to proteins and DNA, increase oxidative stress or trigger an immune response contributing to the risk of idiosyncratic drug toxicity (Regan et al., 2010). Transporters play a key role in regulating intracellular acyl glucuronide levels and transporter function may therefore influence their toxicity risk. Furthermore, transporters may also affect the toxicity of environmental toxins that form reactive sulfate conjugates (Glatt, 2000).
7.1 Fasiglifam-AG
Fasiglifam (TAK-875) is a free fatty acid receptor 1 agonist that was in development for the treatment of type 2 diabetes (Kaku et al., 2015). It is a recent example of a drug with an acyl glucuronide that was withdrawn during phase III clinical trials due to safety concerns regarding drug-induced liver injury (DILI). Formation of fasiglifam-AG is the major metabolic pathway for fasiglifam in humans and in vitro studies have indicated that its high risk for DILI is attributable mainly to this metabolite (Kaku, 2013) (Thompson et al., 2012). Fasiglifam-AG showed nonlinear accumulation in rat livers with dose escalation, suggestive of saturation of biliary efflux, likely from inhibition of Mrp2 (Otieno et al., 2018; Kogame et al., 2019). The role of uptake transporters in fasiglifam-AG disposition is unknown, but it is an inhibitor of several hepatic uptake and efflux transporters (Table 2). Based on observed plasma and liver concentrations of fasiglifam-AG in rats, it is likely to inhibit hepatic efflux transporters in vivo, but not uptake transporters. Moreover, fasiglifam-AG is a more potent inhibitor of human than rat MRPs/Mrps, as the IC50 values were over 10- and 50-fold higher for rat Mrp2 and Mrp4 than for human MRP2 and MRP4, while MRP3 inhibition was more similar (Otieno et al., 2018). In addition to direct fasiglifam-AG-mediated liver toxicity, fasiglifam and fasiglifam-AG can alter bile acid homeostasis by inhibiting MRP2, MRP3, MRP4, NTCP and BSEP, possibly contributing to DILI (Wolenski et al., 2017).
7.2 Diclofenac-AG
Diclofenac is used widely (e.g. in the treatment of osteoarthritis) and has been associated with enteropathy, kidney toxicity and rare but severe idiosyncratic hepatotoxicity (Banks et al., 1995; Douros et al., 2018; Watanabe et al., 2020). Histological samples collected from patients having adverse reactions to diclofenac showed hepatocellular injury in over 70% of the samples (Banks et al., 1995). Diclofenac-AG is formed in the liver by UGT2B7 (King et al., 2001) and transporters play a key role in its disposition (Table 1). In the liver, diclofenac-AG is excreted into bile by BCRP and MRP2, whereas MRP3 serves as an alternative pathway if biliary excretion is impaired (Lagas et al., 2010). In humans, diclofenac-AG is primarily excreted in urine. After efflux into the blood by MRP3 in hepatocytes, diclofenac-AG is taken up by OAT1/3 in the kidney and can be transported on the apical side by OAT4 (Zhang et al., 2016). This uptake can lead to renal accumulation and predispose the tubular cells to direct cytotoxicity, which in addition to the reduction in afferent arterial flow caused by diclofenac is considered to be one of the main mechanisms of diclofenac nephrotoxicity. In vitro, the toxicity of diclofenac-AG could be reduced by OAT1/3 inhibition by cilastatin (Huo et al., 2020). Diclofenac-AG can increase diclofenac exposure, as acyl glucuronides can be deconjugated non-enzymatically or by esterases both in the liver and plasma (Suzuki et al., 2010; Ito et al., 2014). Due to this conversion, cilastatin increased the AUC of both diclofenac and diclofenac-AG in mice, even though diclofenac itself is not an OAT1/3 substrate (Huo et al., 2020). MRP3 in the intestine may also protect against diclofenac induced enteropathy. Intestinal ulceration is a classic adverse effect of NSAIDs caused by their pharmacological mode of action and the accumulation of AG metabolites can aggravate it. Studies in Mrp3 knockout mice showed that intestinal injuries caused by diclofenac were consistently more severe in knockout compared to wild type mice (Niu et al., 2015). Ulceration was also reduced in Mrp2 knockout rats compared with wild type rats, presumably due to decreased diclofenac-AG biliary clearance (Seitz and Boelsterli, 1998).
7.3 Other Toxins
Aristolochic acids are a group of toxic phytochemicals found in the Aristolochiaceae plant family. These herbs used in Chinese medicine are associated with urothelial carcinoma and can cause end-stage renal failure. Aristolochic acid I, a potent nephrotoxin, is metabolized by several enzymes, including SULTs in the liver. This hepatic bioactivation has been shown in vitro in a microphysiological system to be the key factor in aristolochic acid I toxicity through the formation of the sulfate conjugate sulfonyloxyaristolactam (Chang et al., 2017). Even more importantly, transporters play a critical role in the renal toxicity of this compound. Sulfonyloxyaristolactam is transported out of hepatocytes by MRP3 and MRP4 and concentrated by OAT1 and OAT3 from blood, and by OAT4 from urine, into the proximal tubular epithelial cells, where this toxin reacts to form DNA adducts at high levels. Inhibition of OATs by probenecid, decreased the toxicity in kidney cells by 50–60% in the microphysiological in vitro system (Chang et al., 2017).
Transporter-mediated enhancement of toxicity has also been observed with other reactive sulfate conjugates. 1-sulfooxymethylpyrene is a sulfate metabolite of 1-methylpyrene, a procarcinogen present for example in cigarette smoke. In vitro, 1-sulfooxymethylpyrene accumulated into OAT1-or OAT3-overexpressing cells resulting in 4.6- and 3.0-fold higher DNA adduct formation, respectively, compared to control cells (Bakhiya et al., 2006). OAT-inhibitor probenecid abolished this effect, indicating that the OAT-mediated uptake of 1-sulfooxymethylpyrene is important for its renal toxicity. Similar results have been seen with 5-sulfooxymethylfurfural, a reactive sulfate metabolite of inactive 5-hydroxymethylfurfural, which is found in many foods (Bakhiya et al., 2009). Like 1-sulfooxymethylpyrene, 5-sulfooxymethylfurfural is a substrate for OAT1 and OAT3. OAT-mediated uptake was found to significantly increase its cytotoxicity, whereas the inhibition of OATs by probenecid reduced the cytotoxic effects (Bakhiya et al., 2009).
8 Effects of Pharmacogenetics on Conjugate Disposition
Genetic variation in transporter genes can cause alterations in transporter abundance, localization or function, leading to altered disposition of their substrates, including glucuronide and sulfate conjugates. Transporter variants have a well-described role in two benign bilirubin syndromes involving bilirubin conjugates: 1) In people with Rotor syndrome, decreased hepatic uptake by OATP1B1 and OATP1B3 loss-of function variants increases circulating levels of total bilirubin (van de Steeg et al., 2012) and 2) loss-of-function variants of MRP2 cause the Dubin-Johnson syndrome, where decreased biliary excretion increases conjugated bilirubin levels in the serum and liver (Kartenbeck et al., 1996). Transporter genotype also influences endogenous sulfate conjugates, as healthy volunteers with OATP1B1 *15/*15 haplotype (c.388A>G, rs2306283; and c.521T>C, rs4149056) had >1.7-fold higher AUCs of GCDCA-S, lithocholate-S, glycolithocholate-S and taurolithocholate-S compared to OATP1B1 *1b/*1b (c.388A>G) (Mori et al., 2019). Furthermore, in a recent genome-wide association study on metabolomic data, genetic variants of an orphan transporter, SLC22A24, were found to be associated with androsterone-G and etiocholanolone-G levels (Yee et al., 2019). In addition to affecting endogenous compounds, there is increasing evidence showing that transporter pharmacogenetics contribute to interindividual variability in drug pharmacokinetics (Giacomini et al., 2013). Although less is known about the effect of genetic variation on the disposition of phase II conjugates than on parent drugs, several cases where transporter variants cause altered drug glucuronide or sulfate disposition have been found.
8.1 Ezetimibe-G
Ezetimibe, a cholesterol-lowering drug acting primarily in the intestine, is extensively glucuronidated in enterocytes to form ezetimibe-G. Ezetimibe-G is a substrate of several transporters including OATP1B1, OATP2B1, and MRP2 (Zamek-Gliszczynski et al., 2014) (Table 1). Since ezetimibe-G is formed in the intestine, hepatic uptake could be a rate-limiting step for enterohepatic recycling as only the glucuronide is a substrate of OATP1B1 (Oswald et al., 2008). The cellular uptake of ezetimibe-G was reduced in cells expressing the OATP1B1 *1b and *5 (c.521T>C, rs4149056) haplotypes compared to OATP1B1 *1a (reference genotype). Healthy volunteers carrying OATP1B1 *1b/*1b had a 50% lower AUC of ezetimibe compared to carriers of *1a/*1a, and a trend for a higher ezetimibe-G AUC as well as increased amount of ezetimibe-G excreted in urine. In addition, *5 and *15 carriers had decreased excretion of ezetimibe into feces. These results suggest that decreased OATP1B1 function shifts ezetimibe excretion from biliary to renal and reduces enterohepatic recycling. However, these changes did not significantly affect the pharmacodynamic effect of ezetimibe in a study with healthy volunteers (Oswald et al., 2008).
8.2 Morphine-Gs
The opioid analgesic morphine is primarily eliminated by glucuronidation in the liver and the subsequent glucuronides, morphine-3-G and morphine-6-G, are substrates of MRP2 and MRP3, which transport the glucuronides to the bile and the systemic circulation, respectively (Zelcer et al., 2005). The disposition of morphine-6-G is of particular interest since it is an active metabolite. The appearance of morphine glucuronides in the systemic circulation appears to be associated with a promoter region variant of ABCC3 (c.-211C>T, rs4793665) in pediatric patients, with the CC genotypes having a higher glucuronide level than the CT and TT genotypes (Venkatasubramanian et al., 2014; Chidambaran et al., 2017). Even though morphine is not known to be a substrate of MRP3, a link between apparent decreased morphine clearance and the c.-211C>T variant has been found in pediatric patients (Hahn et al., 2020). This is suggested to be due to a shift from the urinary excretion pathway to biliary excretion: Patients with the CT or TT genotypes had lower MRP3 activity than those with the CC genotype, leading to increased excretion of the glucuronides to the bile by MRP2, and a subsequent increase in enterohepatic recycling and morphine exposure. Furthermore, some ABCC3 intronic variants were found to be associated with a longer postoperative unit care stay, due to respiratory depression as a side effect of morphine treatment (Chidambaran et al., 2017).
8.3 Raloxifene-Gs
As described in Section 4.2, raloxifene undergoes high intestinal and hepatic metabolism to several glucuronide conjugates. Although direct in vitro evidence is still missing, it is expected that OATP1B1 and OATP1B3 are required for the uptake of raloxifene-Gs into hepatocytes and subsequent biliary excretion by apical efflux transporters (Trdan Lušin et al., 2012a, 2012b; Kosaka et al., 2015). The role of OATP1B1 is supported by the association of OATP1B1 *1b haplotype with higher serum concentrations of raloxifene species (raloxifene, raloxifene-6,4′-di-G and total raloxifene) compared with OATP1B1 *1a in a study with 57 postmenopausal women treated with raloxifene for 12 months (Trdan Lušin et al., 2012b). Decreased hepatic uptake of raloxifene-Gs by the low-function *1b haplotype would explain the observed increase in the systemic concentrations of the raloxifene species. This haplotype was also linked to a higher decrease in serum C-terminal telopeptide fragments of type I collagen, a bone resorption marker, indicating a better therapeutic effect, probably due to the increased total systemic raloxifene exposure (Trdan Lušin et al., 2012b). The SLCO1B1 c.521T>C polymorphism, which is included in the *5 and *15 haplotypes, did not significantly influence the pharmacokinetics or pharmacodynamics of raloxifene species in this study. No significant association was found either for SLCO1B3 int7C>G (rs17680137) or for efflux transporter polymorphisms ABCB1 c.3435C>T (rs1045642) or ABCC2 c.3972C>T (rs3740066) (Trdan Lušin et al., 2012a; 2012b).
8.4 MPA-G
Mycophenolate mofetil is an immunosuppressive pro-drug that is commonly used in solid organ transplantations. The active form, mycophenolic acid, undergoes extensive glucuronidation in the liver, and the main metabolite, MPA-G, is pharmacologically inactive (Lamba et al., 2014). Additionally, a pharmacologically active acyl glucuronide is formed as a minor metabolite. Active secretion in the kidney is the main elimination pathway of MPA-G, but a significant portion of MPA-G is excreted to the bile and undergoes enterohepatic recycling, so that only a small portion of MPA-G is found in feces (Bullingham et al., 1998). Systemic MPA-G is taken up by OATP1B1 and OATP1B3 in hepatocytes, while MRP2 mediates MPA-G excretion into bile and is involved in secreting MPA-G into urine (Naesens et al., 2006; Picard et al., 2010; Matsunaga et al., 2014). OATP1B1 and OATP1B3 genotypes were predictive for MPA-G exposure in a study with 80 Japanese renal transplant patients (Miura et al., 2008). Furthermore, the decreased function ABCG2 polymorphism c.421C>A (rs2231142) caused ≈30% increase in median MPA-G AUC, but MPA-G transport by BCRP remains to be verified in vitro. The OATP1B3 haplotype c.334T>G–c.699G>A (rs4149117 and rs7311358, respectively) resulted in decreased MPA AUC and increased MPA-G/MPA AUC ratio in renal transplant patients, while studied OATP1B1 polymorphisms had no effect on MPA or MPA-G (Picard et al., 2010). The decrease in MPA AUC is suggested to result from reduced hepatic uptake of MPA-G and subsequent enterohepatic recycling, leading to lower AUC and less frequent adverse reactions. In vitro and in vivo studies support this hypothesis, as uptake of MPA-G was reduced in OATP1B3 c.334T>G–c.699G>A expressing cells (Picard et al., 2010) and this haplotype was associated with lower survival and increased risk for non-minimal acute rejection (Tague et al., 2020). Although in vivo pharmacokinetic evidence is missing, MPA-G uptake is also reduced in OATP1B1 *5 expressing cells in vitro and the OATP1B1 *5 haplotype protected patients from MPA-related adverse reactions after renal transplantation (Michelon et al., 2010). The effect of MRP2 polymorphisms on MPA-G exposure is more contradictory (Lamba et al., 2014).
9 Effects of Disease on Conjugate Disposition
Diseases affecting the intestine, liver or kidney can alter the absorption and excretion of drugs and their metabolites, either through physiological changes (e.g. in blood flow, protein binding), or through altered function of metabolic enzymes and transporters. The pharmacokinetic impact of disease-mediated changes in drug transporters has recently been reviewed by Evers et al. (2018). However, clinical evidence for transporter-mediated alterations in drug conjugate exposure in disease is still scarce even though conjugates may be sensitive to changes in transport due to their low passive permeability.
9.1 Liver Disease
Liver disease is known to alter the metabolic capacity of hepatocytes and hepatic clearance may be impacted by changes in liver blood flow rates or even intra-hepatic shunting of blood in severe cases of liver impairment (Drozdzik et al., 2021). In addition, there is increasing evidence for changes in transporter protein levels in different types of liver disease (Drozdzik et al., 2020). For example, levels of several hepatic uptake transporters, as well as MRP2, tend to decrease with increasing liver disease severity, whereas OCT1, MRP3, MRP4 and P-gp levels tend to increase. Some changes, however, appear to be disease specific. The clinical significance of these changes in transporter levels is poorly characterized and many studies have relied on data from animal models of liver disease (Thakkar et al., 2017). Animal models have been useful, for example, to elucidate the role of MRP2 in estradiol-17-G induced cholestasis (Huang et al., 2000).
Two examples of altered conjugate disposition in hepatic disease come from studies of nononalcoholic steatohepatitis (NASH), the advanced stage of nonalcoholic liver disease. In patients with NASH, systemic exposure to morphine-3-G, morphine-6-G and acetaminophen-G is increased (Canet et al., 2015; Ferslew et al., 2015). This is likely due to the increased abundance of hepatic MRP3 and mislocalization of MRP2 in NASH, which results in increased efflux into the blood circulation and reduced biliary excretion (Hardwick et al., 2011; Vildhede et al., 2020; Sjöstedt et al., 2021). On the other hand, an observed trend for decreasing acetaminophen sulfate levels could be due to altered sulfonation activity caused by impaired sulfur activation in NASH (Canet et al., 2015), which highlights the importance (and challenge) of distinguishing changes in metabolite formation from transporter-mediated alterations. More studies are needed to provide further evidence on how conjugate disposition is impacted by liver disease and to elucidate, for example, whether the observed shift from biliary to sinusoidal clearance in NASH and other diseases has a significant effect on enterohepatic recycling and drug exposure.
9.2 Renal Disease
There is little information on transporter levels in renal disease in humans, although a decrease in OAT1 expression at the mRNA level has been reported (Sakurai et al., 2004). Renal disease and decreased kidney function are known to increase the exposure to several drug glucuronides (Verbeeck, 1982). For example, oxazepam and oxazepam-G are typically found at comparable levels in healthy subjects, but the oxazepam-G to oxazepam ratio was shown to increase to up to 50 due to an increase in oxazepam-G in patients with renal insufficiency (Odar-Cederlöf et al., 1977). Further, in a study with renal failure patients, the concentrations of propranolol-Gs were up to 18-fold higher compared with patients with normal renal function (Stone and Walle, 1980). These two examples clearly highlight the possibility of high accumulation of drug conjugates in renal diseases. Moreover, fecal excretion and enterohepatic recycling may become more pronounced for conjugates primarily eliminated via the kidney in healthy individuals if excretion is shifted to the biliary route, as shown for example for oxazepam (Odar-Cederlöf et al., 1977).
An age-related decrease in active renal secretion was suggested to result in a 1.9- to 2.5-fold reduction in the renal clearance of propafenone-Gs in older compared to younger subjects with normal renal function (Fromm et al., 1995), but the transporters involved in secretion have not been identified. Zhong et al. (2014) showed that severe renal impairment increased exposure to sulfate and glucuronide conjugates of morinidazole ≥15-fold and identified them as OAT1 and OAT3 substrates. Therefore, decreased transporter function could explain the reduction of their renal clearance. The challenge in renal disease is to distinguish whether pharmacokinetic changes are caused solely by changes in glomerular filtration rate or by alterations in transporter-mediated renal excretion. The distinction is further complicated by the use of serum creatinine to estimate glomerular filtration rate. Transporters are involved in creatinine clearance and changes in serum creatinine may in some cases be attributed to altered transport activity (Chu et al., 2016). Furthermore, transporter function may be altered in kidney disease both due to altered expression as well as inhibition by the uremic toxins that accumulate with declined kidney function (Table 3).
Uremic toxins include several glucuronide and sulfate conjugates. The most noteworthy of these are indoxyl-S and p-cresol-S because of their role in the progression of chronic kidney disease, cardiovascular disease and interactions with transport proteins (Sirich et al., 2014). Both also exist as glucuronide conjugates. Indoxyl-S and p-cresol-S are eliminated via renal excretion and impaired kidney function can elevate their plasma levels by more than 40- and 10-fold, respectively (Duranton et al., 2012). Renal excretion of uremic toxins is facilitated by OAT1, OAT3, OAT4 and BCRP and they can inhibit multiple transporters in vitro (Table 3). The ratio of plasma concentrations to IC50 values of indoxyl-S and p-cresyl-S suggest potential for clinically significant inhibition. For example, inhibition by uremic toxins was proposed to be the mechanism for reduced clearance of morinidazole conjugates in a rat model of chronic renal failure (Kong et al., 2017). Notably, the effects of uremic toxins are not limited to renal transporters, but may also alter hepatic clearance by inhibiting OATP-mediated uptake into the liver (Tan et al., 2018), as indoxyl-S and p-cresyl-S have been shown in vitro to inhibit OATPs (Table 3). Furthermore, the accumulation of uremic toxins can possibly lead to displacement of other drugs from albumin and this combined with hypoalbuminemia common in chronic kidney disease patients may increase the unbound fraction of drugs.
9.3 Intestinal Disease
Drug absorption is affected greatly by intestinal physiology, which can be altered in disease (Stillhart et al., 2020). Transporters in the intestine may have a smaller role than in the liver and kidney on conjugate disposition except if the conjugation occurs in the enterocytes. In this case, disease-associated changes in transporter levels or function may alter the fraction of conjugate reaching the systemic circulation, but limited information on transporter changes is available. For example, inflammation in ulcerative colitis has been shown to decrease mRNA, but not protein, levels of P-gp and BCRP and increase SLCO2B1 mRNA levels when compared with non-inflamed tissue (Erdmann et al., 2019). Jahnel et al. (2014) reported decreased ABCG2 mRNA levels in Crohn’s disease compared to healthy controls, whereas both ABCC3 and ABCC4 mRNA was decreased in ulcerative colitis in their study. However, the in vivo effect of these transporter changes on conjugate kinetics remains to be elucidated. Besides transporter changes, any diseases affecting the gut microbiome and bacterial β-glucuronidase activity could affect conjugate and parent drug disposition by decreasing intestinal deconjugation and leading to impaired enterohepatic recycling.
10 Conjugate Disposition Studies in Preclinical Animals
In vivo studies are needed to understand how the complex interplay of metabolism and transport affects the overall disposition and elimination patterns of drugs and their metabolites. However, plasma, urine and fecal samples from human subjects are often insufficient for resolving detailed mechanisms in disposition. Studies in preclinical animals allow more invasive sampling, such as bile collection, that is not typically feasible in human subjects. Important mechanistic information can also be obtained when performing studies in animals where specific transporters have been knocked out or by using humanized rodent models (Durmus et al., 2016). Examples of transporter-mediated conjugate disposition studies in efflux knockout rodents have been discussed previously by (Zamek-Gliszczynski et al., 2006) and examples for uptake transporters are summarized in Table 4.
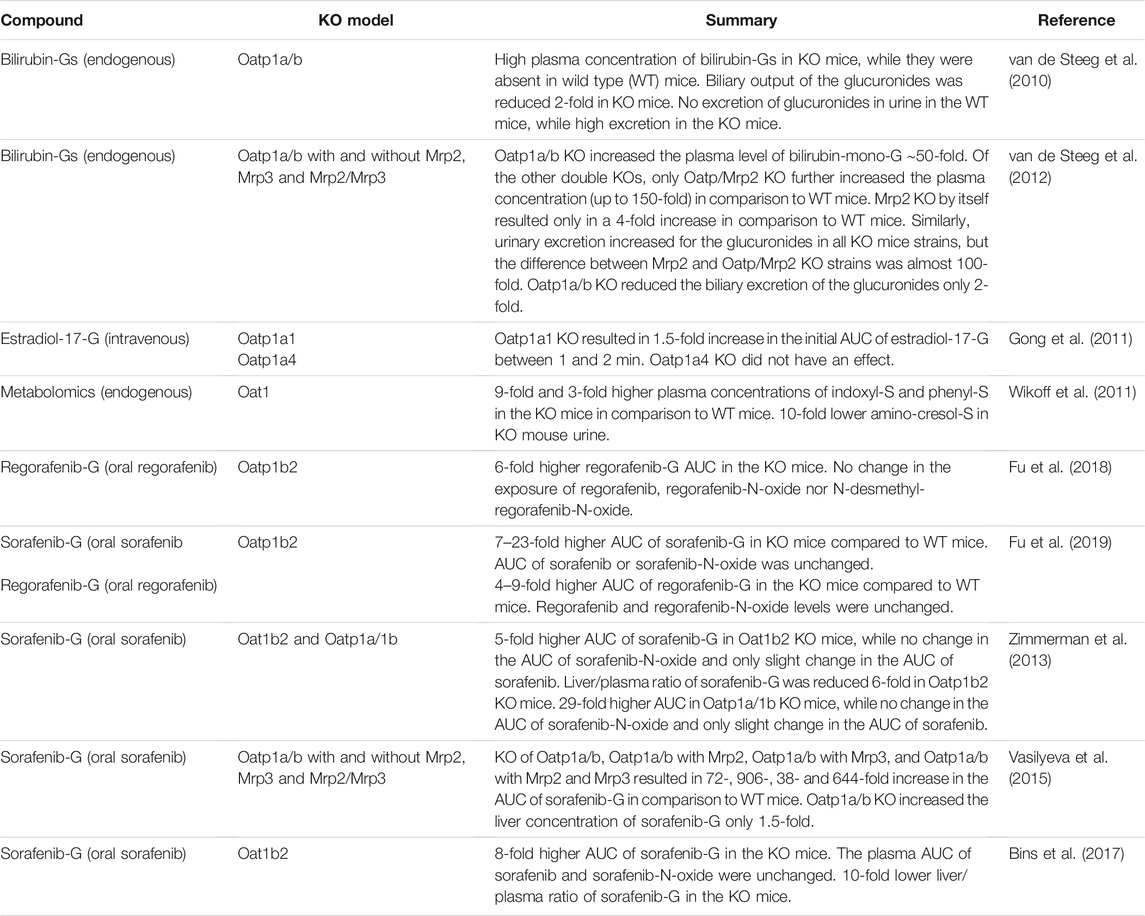
TABLE 4. Studies in knockout (KO) mice supporting the significance of uptake transporters in the disposition of glucuronide and sulfate metabolites of drugs and other compounds.
Knockout animal studies have been instrumental in clarifying the roles and interplay of uptake and efflux transporters in the liver. Studies in Oatp1a/b knockout mice revealed that the glucuronides of bilirubin undergo Oatp1a/b-mediated shuttling between hepatocytes, which was termed hepatocyte hopping (van de Steeg et al., 2010). Later, sorafenib-G was also found to exhibit similar behavior (Vasilyeva et al., 2015). In mice, Mrp2, Oatp, Oatp;Mrp2 and Oatp;Mrp2;Mrp3 knockouts all led to a substantial (38–906-fold) increase in sorafenib-G plasma concentrations, while the increase in liver concentrations was more modest (1.5 – 3-fold). Furthermore, knockout mice were used to resolve the roles of sinusoidal and canalicular transporters in diclofenac-AG disposition (Lagas et al., 2010).
The above examples highlight the utility of preclinical in vivo studies, especially in knockout animals. However, compensatory changes in knockout animal models should be considered as it is well known, for example, that decreased MRP2/Mrp2 function in humans and rodents leads to an increase in hepatic MRP3/Mrp3 protein levels (König et al., 1999; Kuroda et al., 2004). Inherent interspecies differences may also complicate the interpretation and extrapolation of preclinical data on drug conjugates to humans (Zamek-Gliszczynski et al., 2006). For example, rats do not have a gall bladder and human OATP isoforms do not have direct orthologs in the rat. Based on its specific expression in the liver, rat Oatp1b2 is considered to correspond to OATP1B1 and OATP1B3, even though there are differences in their substrate specificity (Cattori et al., 2001). Differences also exist in transporter abundance. According to a proteomics study across liver samples from human, monkey, dog and rat, OATPs/Oatps were the most abundant transporters, but their contribution to the total abundance varied from 29% in humans to 69% in dogs, with the total Oatp expression being approximately 4-fold higher in rat than in human (Wang L. et al., 2015). In rat livers, Mrp2 protein levels were over 5-fold compared to human livers, whereas Bcrp and Mrp3 abundances were below the detection limit in rat livers, unlike in humans. High expression of Oatps and Mrp2 in rats may contribute to the pronounced biliary excretion observed in rats compared with humans (Grime and Paine, 2013). For the kidney, transporters tended to have higher abundance in kidney cortices in monkey, dog, rat and mice than in humans (Basit et al., 2019). Importantly, regarding localization differences, Oat2 is found only on the apical membrane of renal proximal tubule cells in rats, while human OAT2 is found primarily on the basolateral membrane (Shen et al., 2015).
In addition to differences in transporter levels, substrate specificity can vary between species. Data for species differences in transport of sulfate and glucuronide conjugates is sparse. In our literature search, only a handful of drug conjugates had data available for humans and preclinical species, but the data suggests that drug conjugates that are substrates of human transporters are generally also substrates of the ortholog proteins (Supplementary Table S1). An exception to this is, for example, raloxifene-6-G, which is a substrate of rat Mrp2 and its disposition is altered in Mrp2-deficient EHBR rats (Kosaka et al., 2015). However, it does not appear to be a substrate of human MRP2, as determined by an indirect in vitro assay measuring ATP hydrolysis in the presence of raloxifene-6-G (Trdan Lušin et al., 2012a). Similarly, paroxetine M1-G is a substrate of rat MRP2, but not human MRP2 (Matsunaga et al., 2013). Species differences in transporter affinity and inhibition potency may be significant when evaluating drug safety. For example, the lower inhibition potency of fasiglifam-AG towards transporters in rats than in humans may have contributed to the late identification of fasiglifam DILI risk (Otieno et al., 2018).
Differences in UGTs and SULTs also need to be considered when studying drugs that undergo conjugation, since alterations in both transport and metabolism can affect the observed exposure of conjugated metabolites. In the case of fasiglifam, lower formation of fasiglifam-AG in rats may have further contributed to differences in observed DILI risk (Otieno et al., 2018). Species differences in metabolism can also change the overall disposition patterns. Maribavir, a novel agent for the management of human cytomegalovirus infection, is primarily metabolized via glucuronidation in non-human primates and undergoes biliary excretion and enterohepatic recycling, whereas only 20% of the dose is glucuronidated in human hepatocytes and no direct glucuronides were found in human feces (Sun and Welty, 2021).
Species differences in UGTs have been characterized (Oda et al., 2015; Fujiwara et al., 2018). The UGT1A gene family is mostly conserved among species and the isoforms are generally expressed in the same tissues in humans and rodents, whereas assigning orthologs of human UGT2 genes in other species is more difficult. Even for UGT1As, it should be noted that Ugt1a8 and Ugt1a10 are not intestine-specific isoforms in mice as they are in humans (Fujiwara et al., 2018). More importantly, N-glucuronidation of tertiary amines is very low in mice and rats (Kaivosaari et al., 2011). Most substrates that are metabolized by human UGT1 isoforms in humans are also glucuronidated in rodents, but the clearance may vary several fold. For instance, the intrinsic formation clearance of furosemide-AG and naproxen-AG are approximately 5-fold and 3-fold, respectively, in mice compared to humans (Kutsuno et al., 2013). Conversely, MPA glucuronidation occurs at a 5-fold rate in humans compared to rat (Shiratani et al., 2008). Differences also exist for UGT2B7, which catalyzes morphine glucuronidation in humans. In rats and mice, morphine-3-G is formed at approximately 17- and 29-fold higher rates, respectively, than in humans, whereas morphine-6-G, which is formed in humans, could not be detected in rats and mice (Soars et al., 2001; Shiratani et al., 2008). Less data is available comparing SULT expression and activity in preclinical species, but some evidence of differences exists. For example, the O-demethyl phase I metabolite of apixaban is sulfated in human S9 liver fractions with over 50-fold higher rate than in rat and over 600-fold higher than in mouse S9 fractions, whereas the sulfate formation rate was more similar to humans in dogs and monkeys (Wang et al., 2009).
11 Discussion
Phase II glucuronide and sulfate metabolites are typically less active than their parent drugs, but these metabolites can still contribute significantly to drug exposure, for instance through enterohepatic recycling or DDIs, and may even cause toxicity. Since transporters are vital determinants of the disposition of poorly permeable phase II conjugates, it is important to understand the interactions between transporters and these conjugates. By characterizing the role of different transporters and the sum of uptake and efflux transport across membranes, the fate of a compound in the body can be explained (see Section 4). Despite increased study within the last decade, there are still many unanswered questions regarding transport of sulfate and glucuronide conjugates. Interactions with efflux transporters have been better characterized than with uptake transporters, but there are still many conjugates with a paucity of information regarding both uptake and efflux. The large number of endogenous and natural compound conjugates also identified in our literature search further highlight the prevalence of interactions between phase II conjugates and drug transporters (Supplementary Table S1).
Among efflux transporters, BCRP, MRP2 and MRP3 appear to have the most conjugate substrates, while P-gp, which transports many parent drugs, does not seem to influence the disposition of conjugates (Table 1). The relative transport activity of MRP2 on the apical and MRP3 and OATP1Bs on the basolateral membranes of hepatocytes appears particularly important for determining the excretion routes for conjugated drugs. Even for glucuronide conjugates undergoing biliary excretion, hepatic uptake may be the rate-limiting factor, especially for glucuronides formed in the intestine, such as ezetimibe and raloxifene glucuronides (Oswald et al., 2008; Trdan Lušin et al., 2012b). Due to the complex interplay between metabolism and transport, alterations in transporter activity can shift the excretion pathway and exposure of the parent drug and the metabolite, thereby affecting the safety and efficacy of the drug.
The role of phase II metabolites in DDIs is poorly characterized, partly since it can be difficult to distinguish the role of inhibition by the conjugate versus the parent drug in the clinical setting. Evaluating the likelihood of inhibition is especially challenging for efflux transporters as inhibition is likely related to intracellular concentrations, whereas uptake transporter inhibition is typically driven by extracellular (systemic) concentrations. Furthermore, both uptake and efflux transporters may play a role in determining intracellular concentrations of conjugates and may thus affect not only transporter inhibition, but also the likelihood of inhibition of metabolism. Conjugated drug metabolites may have been overlooked as possible perpetrators for DDIs (Table 2), particularly for renal uptake transporters, as a recent study identified several drug conjugate inhibitors of OAT1 and OAT3 (Zou et al., 2021). The clinical significance of this in vitro OAT inhibition by conjugates remains to be determined, but some conjugates have already been implicated in clinical DDIs (Zamek-Gliszczynski et al., 2014; Patel et al., 2016). Furthermore, endogenous glucuronide and sulfate bile acid conjugates have in recent years received interest as endogenous biomarkers for transporter inhibition in clinical studies for identifying DDI risk (Section 6.3).
Overall, there are still many challenges to solve when determining the mechanisms behind the disposition and effects of phase II conjugates in the body. As the potential impact of transporter interactions is recognized, the interactions of new drugs and their conjugates with transporters are extensively studied. However, the interactions and impact of conjugates of older drugs, natural products and many other compounds with transporters are unknown. In vitro studies can be used to identify transporter substrates and inhibitors, but other methods are needed in order to evaluate the in vivo impact. Due to the large substrate overlap between transporters and metabolic enzymes there is a lack of specific inhibitors, biomarkers and probe substrates to use for mechanistic in vivo studies. Although knockout animal models are useful to elucidate transporter impact, they have significant limitations due to species differences and compensatory changes in transporter levels. Pharmacogenetic studies can also help to gain mechanistic information on conjugate disposition, but should ideally include genotyping of all relevant transporters instead of focusing on a single pathway. Finally, physiologically-based pharmacokinetic modeling has the potential to integrate metabolism and transport data to elucidate their roles in different tissues and predict conjugate disposition and tissue concentrations even in complex cases, including e.g. those involving enterohepatic recycling.
In conclusion, the number of identified interactions between uptake and efflux transporters and phase II conjugates continues to increase. This increased understanding of transporter involvement will further improve our ability of predict drug disposition and possible interindividual variability due to intrinsic (e.g. genotype, disease) and extrinsic factors (e.g. concomitant medication).
Author Contributions
EJ, FD, and AS performed the literature search for in vitro transporter studies in overexpression systems. All authors contributed to the conceptualization, writing and revision of the manuscript.
Funding
FD was supported by the Academy of Finland and Cancer Foundation Finland. AS and WK were supported by the Finnish Cultural Foundation.
Conflict of Interest
The authors declare that the research was conducted in the absence of any commercial or financial relationships that could be construed as a potential conflict of interest.
Publisher’s Note
All claims expressed in this article are solely those of the authors and do not necessarily represent those of their affiliated organizations, or those of the publisher, the editors and the reviewers. Any product that may be evaluated in this article, or claim that may be made by its manufacturer, is not guaranteed or endorsed by the publisher.
Supplementary Material
The Supplementary Material for this article can be found online at: https://www.frontiersin.org/articles/10.3389/fphar.2021.802539/full#supplementary-material
References
Abu-Hayyeh, S., Martinez-Becerra, P., Sheikh Abdul Kadir, S. H., Selden, C., Romero, M. R., Rees, M., et al. (2010). Inhibition of Na+-Taurocholate Co-transporting Polypeptide-Mediated Bile Acid Transport by Cholestatic Sulfated Progesterone Metabolites. J. Biol. Chem. 285, 16504–16512. doi:10.1074/jbc.M109.072140
Ackerson, T., Amberg, A., Atzrodt, J., Arabeyre, C., Defossa, E., Dorau, M., et al. (2019). Mechanistic Investigations of the Liver Toxicity of the Free Fatty Acid Receptor 1 Agonist Fasiglifam (TAK875) and its Primary Metabolites. J. Biochem. Mol. Toxicol. 33, e22345. doi:10.1002/jbt.22345
Ahn, S. K., Suh, C. K., and Cha, S. H. (2015). Polymorphisms of SLC22A9 (hOAT7) in Korean Females with Osteoporosis. Korean J. Physiol. Pharmacol. 19, 319–325. doi:10.4196/kjpp.2015.19.4.319
Bakhiya, N., Monien, B., Frank, H., Seidel, A., and Glatt, H. (2009). Renal Organic Anion Transporters OAT1 and OAT3 Mediate the Cellular Accumulation of 5-sulfooxymethylfurfural, a Reactive, Nephrotoxic Metabolite of the Maillard Product 5-hydroxymethylfurfural. Biochem. Pharmacol. 78, 414–419. doi:10.1016/j.bcp.2009.04.017
Bakhiya, N., Stephani, M., Bahn, A., Ugele, B., Seidel, A., Burckhardt, G., et al. (2006). Uptake of Chemically Reactive, DNA-Damaging Sulfuric Acid Esters into Renal Cells by Human Organic Anion Transporters. J. Am. Soc. Nephrol. 17, 1414–1421. doi:10.1681/ASN.2005080801
Ballatori, N., Christian, W. V., Lee, J. Y., Dawson, P. A., Soroka, C. J., Boyer, J. L., et al. (2005). OSTalpha-OSTbeta: a Major Basolateral Bile Acid and Steroid Transporter in Human Intestinal, Renal, and Biliary Epithelia. Hepatology 42, 1270–1279. doi:10.1002/hep.20961
Banks, A. T., Zimmerman, H. J., Ishak, K. G., and Harter, J. G. (1995). Diclofenac-associated Hepatotoxicity: Analysis of 180 Cases Reported to the Food and Drug Administration as Adverse Reactions. Hepatology 22, 820–827. doi:10.1002/hep.1840220320
Basit, A., Neradugomma, N. K., Wolford, C., Fan, P. W., Murray, B., Takahashi, R. H., et al. (2020). Characterization of Differential Tissue Abundance of Major Non-CYP Enzymes in Human. Mol. Pharm. 17, 4114–4124. doi:10.1021/acs.molpharmaceut.0c00559
Basit, A., Radi, Z., Vaidya, V. S., Karasu, M., and Prasad, B. (2019). Kidney Cortical Transporter Expression across Species Using Quantitative Proteomics. Drug Metab. Dispos 47, 802–808. doi:10.1124/dmd.119.086579
Berthier, J., Benmameri, M., Sauvage, F. L., Fabre, G., Chantemargue, B., Arnion, H., et al. (2021). MRP4 Is Responsible for the Efflux Transport of Mycophenolic Acid β-d Glucuronide (MPAG) from Hepatocytes to Blood. Xenobiotica 51, 105–114. doi:10.1080/00498254.2020.1813352
Bi, Y. A., Costales, C., Mathialagan, S., West, M., Eatemadpour, S., Lazzaro, S., et al. (2019). Quantitative Contribution of Six Major Transporters to the Hepatic Uptake of Drugs: "SLC-Phenotyping" Using Primary Human Hepatocytes. J. Pharmacol. Exp. Ther. 370, 72–83. doi:10.1124/jpet.119.257600
Bins, S., van Doorn, L., Phelps, M. A., Gibson, A. A., Hu, S., Li, L., et al. (2017). Influence of OATP1B1 Function on the Disposition of Sorafenib-β-D-Glucuronide. Clin. Transl Sci. 10, 271–279. doi:10.1111/cts.12458
Boer, J., Young-Sciame, R., Lee, F., Bowman, K. J., Yang, X., Shi, J. G., et al. (2016). Roles of UGT, P450, and Gut Microbiota in the Metabolism of Epacadostat in Humans. Drug Metab. Dispos 44, 1668–1674. doi:10.1124/dmd.116.070680
Boettcher, M., Gerisch, M., Lobmeyer, M., Besche, N., Thomas, D., Gerrits, M., et al. (2020). Metabolism and Pharmacokinetic Drug-Drug Interaction Profile of Vericiguat, A Soluble Guanylate Cyclase Stimulator: Results from Preclinical and Phase I Healthy Volunteer Studies. Clin. Pharmacokinet. 59, 1407–1418. doi:10.1007/s40262-020-00895-x
Bowers, G. D., Culp, A., Reese, M. J., Tabolt, G., Moss, L., Piscitelli, S., et al. (2016). Disposition and Metabolism of Cabotegravir: a Comparison of Biotransformation and Excretion between Different Species and Routes of Administration in Humans. Xenobiotica 46, 147–162. doi:10.3109/00498254.2015.1060372
Brouwer, K. L., Keppler, D., Hoffmaster, K. A., Bow, D. A., Cheng, Y., Lai, Y., et al. (2013). In Vitro Methods to Support Transporter Evaluation in Drug Discovery and Development. Clin. Pharmacol. Ther. 94, 95–112. doi:10.1038/clpt.2013.81
Bullingham, R. E., Nicholls, A. J., and Kamm, B. R. (1998). Clinical Pharmacokinetics of Mycophenolate Mofetil. Clin. Pharmacokinet. 34, 429–455. doi:10.2165/00003088-199834060-00002
Burt, H. J., Riedmaier, A. E., Harwood, M. D., Crewe, H. K., Gill, K. L., and Neuhoff, S. (2016). Abundance of Hepatic Transporters in Caucasians: A Meta-Analysis. Drug Metab. Dispos 44, 1550–1561. doi:10.1124/dmd.116.071183
Canet, M. J., Merrell, M. D., Hardwick, R. N., Bataille, A. M., Campion, S. N., Ferreira, D. W., et al. (2015). Altered Regulation of Hepatic Efflux Transporters Disrupts Acetaminophen Disposition in Pediatric Nonalcoholic Steatohepatitis. Drug Metab. Dispos 43, 829–835. doi:10.1124/dmd.114.062703
Cattori, V., van Montfoort, J. E., Stieger, B., Landmann, L., Meijer, D. K., Winterhalter, K. H., et al. (2001). Localization of Organic Anion Transporting Polypeptide 4 (Oatp4) in Rat Liver and Comparison of its Substrate Specificity with Oatp1, Oatp2 and Oatp3. Pflugers Arch. 443, 188–195. doi:10.1007/s004240100697
Cerny, M. A. (2016). Prevalence of Non-cytochrome P450-Mediated Metabolism in Food and Drug Administration-Approved Oral and Intravenous Drugs: 2006-2015. Drug Metab. Dispos 44, 1246–1252. doi:10.1124/dmd.116.070763
Chan, G., Houle, R., Lin, M., Yabut, J., Cox, K., Wu, J., et al. (2019). Role of Transporters in the Disposition of a Novel β-lactamase Inhibitor: Relebactam (MK-7655). J. Antimicrob. Chemother. 74, 1894–1903. doi:10.1093/jac/dkz101
Chang, S. Y., Weber, E. J., Sidorenko, V. S., Chapron, A., Yeung, C. K., Gao, C., et al. (2017). Human Liver-Kidney Model Elucidates the Mechanisms of Aristolochic Acid Nephrotoxicity. JCI Insight 2, 95978. doi:10.1172/jci.insight.95978
Chen, X., Cui, L., Duan, X., Ma, B., and Zhong, D. (2006). Pharmacokinetics and Metabolism of the Flavonoid Scutellarin in Humans after a Single Oral Administration. Drug Metab. Dispos 34, 1345–1352. doi:10.1124/dmd.106.009779
Cheung, K. W. K., Hsueh, C. H., Zhao, P., Meyer, T. W., Zhang, L., Huang, S. M., et al. (2017). The Effect of Uremic Solutes on the Organic Cation Transporter 2. J. Pharm. Sci. 106, 2551–2557. doi:10.1016/j.xphs.2017.04.076
Cheung, K. W. K., van Groen, B. D., Spaans, E., van Borselen, M. D., de Bruijn, A. C. J. M., Simons-Oosterhuis, Y., et al. (2019). A Comprehensive Analysis of Ontogeny of Renal Drug Transporters: mRNA Analyses, Quantitative Proteomics, and Localization. Clin. Pharmacol. Ther. 106, 1083–1092. doi:10.1002/cpt.1516
Chidambaran, V., Venkatasubramanian, R., Zhang, X., Martin, L. J., Niu, J., Mizuno, T., et al. (2017). ABCC3 Genetic Variants Are Associated with Postoperative Morphine-Induced Respiratory Depression and Morphine Pharmacokinetics in Children. Pharmacogenomics J. 17, 162–169. doi:10.1038/tpj.2015.98
Chloupková, M., Pickert, A., Lee, J. Y., Souza, S., Trinh, Y. T., Connelly, S. M., et al. (2007). Expression of 25 Human ABC Transporters in the Yeast Pichia pastoris and Characterization of the Purified ABCC3 ATPase Activity. Biochemistry 46, 7992–8003. doi:10.1021/bi700020m
Chu, X., Bleasby, K., Chan, G. H., Nunes, I., and Evers, R. (2016). The Complexities of Interpreting Reversible Elevated Serum Creatinine Levels in Drug Development: Does a Correlation with Inhibition of Renal Transporters Exist? Drug Metab. Dispos 44, 1498–1509. doi:10.1124/dmd.115.067694
Chu, X., Liao, M., Shen, H., Yoshida, K., Zur, A. A., Arya, V., et al. (2018). Clinical Probes and Endogenous Biomarkers as Substrates for Transporter Drug-Drug Interaction Evaluation: Perspectives from the International Transporter Consortium. Clin. Pharmacol. Ther. 104, 836–864. doi:10.1002/cpt.1216
Chu, X. Y., Huskey, S. E., Braun, M. P., Sarkadi, B., Evans, D. C., and Evers, R. (2004). Transport of Ethinylestradiol Glucuronide and Ethinylestradiol Sulfate by the Multidrug Resistance Proteins MRP1, MRP2, and MRP3. J. Pharmacol. Exp. Ther. 309, 156–164. doi:10.1124/jpet.103.062091
Chu, X. Y., Liang, Y., Cai, X., Cuevas-Licea, K., Rippley, R. K., Kassahun, K., et al. (2009). Metabolism and Renal Elimination of Gaboxadol in Humans: Role of UDP-Glucuronosyltransferases and Transporters. Pharm. Res. 26, 459–468. doi:10.1007/s11095-008-9799-5
Coughtrie, M. W. H. (2016). Function and Organization of the Human Cytosolic Sulfotransferase (SULT) Family. Chem. Biol. Interact 259, 2–7. doi:10.1016/j.cbi.2016.05.005
Craddock, A. L., Love, M. W., Daniel, R. W., Kirby, L. C., Walters, H. C., Wong, M. H., et al. (1998). Expression and Transport Properties of the Human Ileal and Renal Sodium-dependent Bile Acid Transporter. Am. J. Physiol. 274, G157–G169. doi:10.1152/ajpgi.1998.274.1.G157
Cubitt, H. E., Houston, J. B., and Galetin, A. (2011). Prediction of Human Drug Clearance by Multiple Metabolic Pathways: Integration of Hepatic and Intestinal Microsomal and Cytosolic Data. Drug Metab. Dispos 39, 864–873. doi:10.1124/dmd.110.036566
Cui, Y., König, J., Buchholz, J. K., Spring, H., Leier, I., and Keppler, D. (1999). Drug Resistance and ATP-dependent Conjugate Transport Mediated by the Apical Multidrug Resistance Protein, MRP2, Permanently Expressed in Human and Canine Cells. Mol. Pharmacol. 55, 929–937.
Cui, Y., König, J., Leier, I., Buchholz, U., and Keppler, D. (2001). Hepatic Uptake of Bilirubin and its Conjugates by the Human Organic Anion Transporter SLC21A6. J. Biol. Chem. 276, 9626–9630. doi:10.1074/jbc.M004968200
Davies, M., Peramuhendige, P., King, L., Golding, M., Kotian, A., Penney, M., et al. (2020). Evaluation of In Vitro Models for Assessment of Human Intestinal Metabolism in Drug Discovery. Drug Metab. Dispos 48, 1169–1182. doi:10.1124/dmd.120.000111
de Waart, D. R., Vlaming, M. L., Kunne, C., Schinkel, A. H., and Oude Elferink, R. P. (2009). Complex Pharmacokinetic Behavior of Ezetimibe Depends on Abcc2, Abcc3, and Abcg2. Drug Metab. Dispos 37, 1698–1702. doi:10.1124/dmd.108.026146
Deguchi, T., Kusuhara, H., Takadate, A., Endou, H., Otagiri, M., and Sugiyama, Y. (2004). Characterization of Uremic Toxin Transport by Organic Anion Transporters in the Kidney. Kidney Int. 65, 162–174. doi:10.1111/j.1523-1755.2004.00354.x
Deng, F., Ghemtio, L., Grazhdankin, E., Wipf, P., Xhaard, H., and Kidron, H. (2020). Binding Site Interactions of Modulators of Breast Cancer Resistance Protein, Multidrug Resistance-Associated Protein 2, and P-Glycoprotein Activity. Mol. Pharm. 17, 2398–2410. doi:10.1021/acs.molpharmaceut.0c00155
Dong, J., Olaleye, O. E., Jiang, R., Li, J., Lu, C., Du, F., et al. (2018). Glycyrrhizin Has a High Likelihood to Be a Victim of Drug-Drug Interactions Mediated by Hepatic Organic Anion-Transporting Polypeptide 1B1/1B3. Br. J. Pharmacol. 175, 3486–3503. doi:10.1111/bph.14393
Douros, A., Bronder, E., Klimpel, A., Erley, C., Garbe, E., and Kreutz, R. (2018). Drug-induced kidney injury: A large case series from the Berlin Case-Control Surveillance Study. Clin. Nephrol. 89 (2018), 18–26. doi:10.5414/CN109212
Drozdzik, M., Busch, D., Lapczuk, J., Müller, J., Ostrowski, M., Kurzawski, M., et al. (2019). Protein Abundance of Clinically Relevant Drug Transporters in the Human Liver and Intestine: A Comparative Analysis in Paired Tissue Specimens. Clin. Pharmacol. Ther. 105, 1204–1212. doi:10.1002/cpt.1301
Drozdzik, M., Lapczuk-Romanska, J., Wenzel, C., Szelag-Pieniek, S., Post, M., Skalski, Ł., et al. (2021). Gene Expression and Protein Abundance of Hepatic Drug Metabolizing Enzymes in Liver Pathology. Pharmaceutics 13, 1334. doi:10.3390/pharmaceutics13091334
Drozdzik, M., Szelag-Pieniek, S., Post, M., Zeair, S., Wrzesinski, M., Kurzawski, M., et al. (2020). Protein Abundance of Hepatic Drug Transporters in Patients with Different Forms of Liver Damage. Clin. Pharmacol. Ther. 107, 1138–1148. doi:10.1002/cpt.1717
Duranton, F., Cohen, G., De Smet, R., Rodriguez, M., Jankowski, J., Vanholder, R., et al. (2012). Normal and Pathologic Concentrations of Uremic Toxins. J. Am. Soc. Nephrol. 23, 1258–1270. doi:10.1681/ASN.2011121175
Durmus, S., van Hoppe, S., and Schinkel, A. H. (2016). The Impact of Organic Anion-Transporting Polypeptides (OATPs) on Disposition and Toxicity of Antitumor Drugs: Insights from Knockout and Humanized Mice. Drug Resist. Updat 27, 72–88. doi:10.1016/j.drup.2016.06.005
El-Sheikh, A. A., Koenderink, J. B., Wouterse, A. C., van den Broek, P. H., Verweij, V. G., Masereeuw, R., et al. (2014). Renal Glucuronidation and Multidrug Resistance Protein 2-/Multidrug Resistance Protein 4-mediated Efflux of Mycophenolic Acid: Interaction with Cyclosporine and Tacrolimus. Transl Res. 164, 46–56. doi:10.1016/j.trsl.2014.01.006
Elsby, R., Smith, V., Fox, L., Stresser, D., Butters, C., Sharma, P., et al. (2011). Validation of Membrane Vesicle-Based Breast Cancer Resistance Protein and Multidrug Resistance Protein 2 Assays to Assess Drug Transport and the Potential for Drug-Drug Interaction to Support Regulatory Submissions. Xenobiotica 41, 764–783. doi:10.3109/00498254.2011.578761
Enokizono, J., Kusuhara, H., and Sugiyama, Y. (2007). Involvement of Breast Cancer Resistance Protein (BCRP/ABCG2) in the Biliary Excretion and Intestinal Efflux of Troglitazone Sulfate, the Major Metabolite of Troglitazone with a Cholestatic Effect. Drug Metab. Dispos 35, 209–214. doi:10.1124/dmd.106.012567
Enomoto, A., Takeda, M., Taki, K., Takayama, F., Noshiro, R., Niwa, T., et al. (2003). Interactions of Human Organic Anion as Well as Cation Transporters with Indoxyl Sulfate. Eur. J. Pharmacol. 466, 13–20. doi:10.1016/s0014-2999(03)01530-9
Erdmann, P., Bruckmueller, H., Martin, P., Busch, D., Haenisch, S., Müller, J., et al. (2019). Dysregulation of Mucosal Membrane Transporters and Drug-Metabolizing Enzymes in Ulcerative Colitis. J. Pharm. Sci. 108, 1035–1046. doi:10.1016/j.xphs.2018.09.024
Ervin, S. M., Simpson, J. B., Gibbs, M. E., Creekmore, B. C., Lim, L., Walton, W. G., et al. (2020). Structural Insights into Endobiotic Reactivation by Human Gut Microbiome-Encoded Sulfatases. Biochem. 59, 3939–3950. doi:10.1021/acs.biochem.0c00711
European Medicines Agency (EMA) (2006). Nexavar : European Public Assessment Report - Product information.
Evers, R., Piquette-Miller, M., Polli, J. W., Russel, F. G. M., Sprowl, J. A., Tohyama, K., et al. (2018). Disease-Associated Changes in Drug Transporters May Impact the Pharmacokinetics And/or Toxicity of Drugs: A White Paper from the International Transporter Consortium. Clin. Pharmacol. Ther. 104, 900–915. doi:10.1002/cpt.1115
Fahrmayr, C., König, J., Auge, D., Mieth, M., and Fromm, M. F. (2012). Identification of Drugs and Drug Metabolites as Substrates of Multidrug Resistance Protein 2 (MRP2) Using Triple-Transfected MDCK-Oatp1b1-Ugt1a1-MRP2 Cells. Br. J. Pharmacol. 165, 1836–1847. doi:10.1111/j.1476-5381.2011.01672.x
Fallon, J. K., Neubert, H., Hyland, R., Goosen, T. C., and Smith, P. C. (2013). Targeted Quantitative Proteomics for the Analysis of 14 UGT1As and -2Bs in Human Liver Using NanoUPLC-MS/MS with Selected Reaction Monitoring. J. Proteome Res. 12, 4402–4413. doi:10.1021/pr4004213
Fallon, J. K., Smith, P. C., Xia, C. Q., and Kim, M. S. (2016). Quantification of Four Efflux Drug Transporters in Liver and Kidney across Species Using Targeted Quantitative Proteomics by Isotope Dilution NanoLC-MS/MS. Pharm. Res. 33, 2280–2288. doi:10.1007/s11095-016-1966-5
Fang, F., Christian, W. V., Gorman, S. G., Cui, M., Huang, J., Tieu, K., et al. (2010). Neurosteroid Transport by the Organic Solute Transporter OSTα-OSTβ. J. Neurochem. 115, 220–233. doi:10.1111/j.1471-4159.2010.06920.x
Ferslew, B. C., Johnston, C. K., Tsakalozou, E., Bridges, A. S., Paine, M. F., Jia, W., et al. (2015). Altered Morphine Glucuronide and Bile Acid Disposition in Patients with Nonalcoholic Steatohepatitis. Clin. Pharmacol. Ther. 97, 419–427. doi:10.1002/cpt.66
Fietz, D., Bakhaus, K., Wapelhorst, B., Grosser, G., Günther, S., Alber, J., et al. (2013). Membrane Transporters for Sulfated Steroids in the Human Testis-Ccellular Localization, Expression Pattern and Functional Analysis. PLoS One 8, e62638. doi:10.1371/journal.pone.0062638
Food and Drug Administration (FDA) (2020). Safety Testing of Drug Metabolites Guidance for Industry.
Fromm, M. F., Botsch, S., Heinkele, G., Evers, J., and Kroemer, H. K. (1995). Influence of Renal Function on the Steady-State Pharmacokinetics of the Antiarrhythmic Propafenone and its Phase I and Phase II Metabolites. Eur. J. Clin. Pharmacol. 48, 279–283. doi:10.1007/BF00198312
Fu, Q., Chen, M., Anderson, J. T., Sun, X., Hu, S., Sparreboom, A., et al. (2019). Interaction between Sex and Organic Anion-Transporting Polypeptide 1b2 on the Pharmacokinetics of Regorafenib and its Metabolites Regorafenib-N-Oxide and Regorafenib-Glucuronide in Mice. Clin. Transl Sci. 12, 400–407. doi:10.1111/cts.12630
Fu, Q., Chen, M., Hu, S., McElroy, C. A., Mathijssen, R. H., Sparreboom, A., et al. (2018). Development and Validation of an Analytical Method for Regorafenib and its Metabolites in Mouse Plasma. J. Chromatogr. B Analyt Technol. Biomed. Life Sci. 1090, 43–51. doi:10.1016/j.jchromb.2018.05.005
Fujiwara, R., Yoda, E., and Tukey, R. H. (2018). Species Differences in Drug Glucuronidation: Humanized UDP-Glucuronosyltransferase 1 Mice and Their Application for Predicting Drug Glucuronidation and Drug-Induced Toxicity in Humans. Drug Metab. Pharmacokinet. 33, 9–16. doi:10.1016/j.dmpk.2017.10.002
Fukami, T., and Yokoi, T. (2012). The Emerging Role of Human Esterases. Drug Metab. Pharmacokinet. 27, 466–477. doi:10.2133/dmpk.dmpk-12-rv-042
Funk, C., Pantze, M., Jehle, L., Ponelle, C., Scheuermann, G., Lazendic, M., et al. (2001). Troglitazone-induced Intrahepatic Cholestasis by an Interference with the Hepatobiliary export of Bile Acids in Male and Female Rats. Correlation with the Gender Difference in Troglitazone Sulfate Formation and the Inhibition of the Canalicular Bile Salt export Pump (Bsep) by Troglitazone and Troglitazone Sulfate. Toxicology 167, 83–98. doi:10.1016/s0300-483x(01)00460-7
Gao, C., Zhang, H., Guo, Z., You, T., Chen, X., and Zhong, D. (2012). Mechanistic Studies on the Absorption and Disposition of Scutellarin in Humans: Selective OATP2B1-Mediated Hepatic Uptake Is a Likely Key Determinant for its Unique Pharmacokinetic Characteristics. Drug Metab. Dispos 40, 2009–2020. doi:10.1124/dmd.112.047183
Gessner, A., König, J., and Fromm, M. F. (2019). Clinical Aspects of Transporter-Mediated Drug-Drug Interactions. Clin. Pharmacol. Ther. 105, 1386–1394. doi:10.1002/cpt.1360
Geyer, J., Döring, B., Meerkamp, K., Ugele, B., Bakhiya, N., Fernandes, C. F., et al. (2007). Cloning and Functional Characterization of Human Sodium-dependent Organic Anion Transporter (SLC10A6). J. Biol. Chem. 282, 19728–19741. doi:10.1074/jbc.M702663200
Giacomini, K. M., Balimane, P. V., Cho, S. K., Eadon, M., Edeki, T., Hillgren, K. M., et al. (2013). International Transporter Consortium Commentary on Clinically Important Transporter Polymorphisms. Clin. Pharmacol. Ther. 94, 23–26. doi:10.1038/clpt.2013.12
Giacomini, K. M., Giacomini, K. M., Huang, S. M., Tweedie, D. J., Benet, L. Z., Brouwer, K. L., et al. (2010). Membrane Transporters in Drug Development. Nat. Rev. Drug Discov. 9, 215–236. doi:10.1038/nrd3028
Glatt, H. (2000). Sulfotransferases in the Bioactivation of Xenobiotics. Chem. Biol. Interact 129, 141–170. doi:10.1016/S0009-2797(00)00202-7
Gong, L., Aranibar, N., Han, Y. H., Zhang, Y., Lecureux, L., Bhaskaran, V., et al. (2011). Characterization of Organic Anion-Transporting Polypeptide (Oatp) 1a1 and 1a4 Null Mice Reveals Altered Transport Function and Urinary Metabolomic Profiles. Toxicol. Sci. 122, 587–597. doi:10.1093/toxsci/kfr114
Grime, K., and Paine, S. W. (2013). Species Differences in Biliary Clearance and Possible Relevance of Hepatic Uptake and Efflux Transporters Involvement. Drug Metab. Dispos 41, 372–378. doi:10.1124/dmd.112.049312
Grosser, G., Bennien, J., Sánchez-Guijo, A., Bakhaus, K., Döring, B., Hartmann, M., et al. (2018). Transport of Steroid 3-sulfates and Steroid 17-sulfates by the Sodium-dependent Organic Anion Transporter SOAT (SLC10A6). J. Steroid Biochem. Mol. Biol. 179, 20–25. doi:10.1016/j.jsbmb.2017.09.013
Grosser, G., Döring, B., Ugele, B., Geyer, J., Kulling, S. E., and Soukup, S. T. (2015). Transport of the Soy Isoflavone Daidzein and its Conjugative Metabolites by the Carriers SOAT, NTCP, OAT4, and OATP2B1. Arch. Toxicol. 89, 2253–2263. doi:10.1007/s00204-014-1379-3
Gufford, B. T., Chen, G., Vergara, A. G., Lazarus, P., Oberlies, N. H., and Paine, M. F. (2015). Milk Thistle Constituents Inhibit Raloxifene Intestinal Glucuronidation: A Potential Clinically Relevant Natural Product-Drug Interaction. Drug Metab. Dispos 43, 1353–1359. doi:10.1124/dmd.115.065086
Guillemette, C., Lévesque, É., and Rouleau, M. (2014). Pharmacogenomics of Human Uridine Diphospho-Glucuronosyltransferases and Clinical Implications. Clin. Pharmacol. Ther. 96, 324–339. doi:10.1038/clpt.2014.126
Hafey, M. J., Houle, R., Tanis, K. Q., Knemeyer, I., Shang, J., Chen, Q., et al. (2020). A Two-Tiered In Vitro Approach to De-risk Drug Candidates for Potential Bile Salt Export Pump Inhibition Liabilities in Drug Discovery. Drug Metab. Dispos 48, 1147–1160. doi:10.1124/dmd.120.000086
Hahn, D., Fukuda, T., Euteneuer, J. C., Mizuno, T., Vinks, A. A., Sadhasivam, S., et al. (2020). Influence of MRP3 Genetics and Hepatic Expression Ontogeny for Morphine Disposition in Neonatal and Pediatric Patients. J. Clin. Pharmacol. 60, 992–998. doi:10.1002/jcph.1592
Han, Y. H., Busler, D., Hong, Y., Tian, Y., Chen, C., and Rodrigues, A. D. (2010a). Transporter Studies with the 3-O-Sulfate Conjugate of 17alpha-Ethinylestradiol: Assessment of Human Kidney Drug Transporters. Drug Metab. Dispos 38, 1064–1071. doi:10.1124/dmd.109.031526
Han, Y. H., Busler, D., Hong, Y., Tian, Y., Chen, C., and Rodrigues, A. D. (2010b). Transporter Studies with the 3-O-Sulfate Conjugate of 17alpha-Ethinylestradiol: Assessment of Human Liver Drug Transporters. Drug Metab. Dispos 38, 1072–1082. doi:10.1124/dmd.109.031518
Hardwick, R. N., Fisher, C. D., Canet, M. J., Scheffer, G. L., and Cherrington, N. J. (2011). Variations in ATP-Binding Cassette Transporter Regulation during the Progression of Human Nonalcoholic Fatty Liver Disease. Drug Metab. Dispos 39, 2395–2402. doi:10.1124/dmd.111.041012
Harwood, M. D., Zhang, M., Pathak, S. M., and Neuhoff, S. (2019). The Regional-specific Relative and Absolute Expression of Gut Transporters in Adult Caucasians: A Meta-Analysis. Drug Metab. Dispos 47, 854–864. doi:10.1124/dmd.119.086959
Hillgren, K. M., Keppler, D., Zur, A. A., Giacomini, K. M., Stieger, B., Cass, C. E., et al. (2013). Emerging Transporters of Clinical Importance: an Update from the International Transporter Consortium. Clin. Pharmacol. Ther. 94, 52–63. doi:10.1038/clpt.2013.74
Hirano, M., Maeda, K., Hayashi, H., Kusuhara, H., and Sugiyama, Y. (2005). Bile Salt export Pump (BSEP/ABCB11) Can Transport a Nonbile Acid Substrate, Pravastatin. J. Pharmacol. Exp. Ther. 314, 876–882. doi:10.1124/jpet.105.084830
Hirano, M., Maeda, K., Shitara, Y., and Sugiyama, Y. (2006). Drug-drug Interaction between Pitavastatin and Various Drugs via OATP1B1. Drug Metab. Dispos 34, 1229–1236. doi:10.1124/dmd.106.009290
Hirouchi, M., Kusuhara, H., Onuki, R., Ogilvie, B. W., Parkinson, A., and Sugiyama, Y. (2009). Construction of Triple-Transfected Cells [organic Anion-Transporting Polypeptide (OATP) 1B1/multidrug Resistance-Associated Protein (MRP) 2/MRP3 and OATP1B1/MRP2/MRP4] for Analysis of the Sinusoidal Function of MRP3 and MRP4. Drug Metab. Dispos 37, 2103–2111. doi:10.1124/dmd.109.027193
Hochner-Celnikier, D. (1999). Pharmacokinetics of Raloxifene and its Clinical Application. Eur. J. Obstet. Gynecol. Reprod. Biol. 85, 23–29. doi:10.1016/s0301-2115(98)00278-4
Hotchkiss, A. G., Berrigan, L., and Pelis, R. M. (2015). Organic Anion Transporter 2 Transcript Variant 1 Shows Broad Ligand Selectivity when Expressed in Multiple Cell Lines. Front. Pharmacol. 6, 216. doi:10.3389/fphar.2015.00216
Hsueh, C. H., Yoshida, K., Zhao, P., Meyer, T. W., Zhang, L., Huang, S. M., et al. (2016). Identification and Quantitative Assessment of Uremic Solutes as Inhibitors of Renal Organic Anion Transporters, OAT1 and OAT3. Mol. Pharm. 13, 3130–3140. doi:10.1021/acs.molpharmaceut.6b00332
Huang, L., Smit, J. W., Meijer, D. K., and Vore, M. (2000). Mrp2 Is Essential for Estradiol-17beta(beta-D-Glucuronide)-Induced Cholestasis in Rats. Hepatology 32, 66–72. doi:10.1053/jhep.2000.8263
Hui, Y., Luo, L., Zhang, L., Kurogi, K., Zhou, C., Sakakibara, Y., et al. (2015). Sulfation of Afimoxifene, Endoxifen, Raloxifene, and Fulvestrant by the Human Cytosolic Sulfotransferases (SULTs): A Systematic Analysis. J. Pharmacol. Sci. 128, 144–149. doi:10.1016/j.jphs.2015.06.004
Huo, X., Meng, Q., Wang, C., Wu, J., Wang, C., Zhu, Y., et al. (2020). Protective Effect of Cilastatin against Diclofenac-Induced Nephrotoxicity through Interaction with Diclofenac Acyl Glucuronide via Organic Anion Transporters. Br. J. Pharmacol. 177, 1933–1948. doi:10.1111/bph.14957
Huo, X., Wang, C., Yu, Z., Peng, Y., Wang, S., Feng, S., et al. (2017). Human Transporters, PEPT1/2, Facilitate Melatonin Transportation into Mitochondria of Cancer Cells: An Implication of the Therapeutic Potential. J. Pineal Res. 62. doi:10.1111/jpi.12390
Hussaarts, K. G. A. M., van Doorn, L., Eechoute, K., Damman, J., Fu, Q., van Doorn, N., et al. (2020). Influence of Probenecid on the Pharmacokinetics and Pharmacodynamics of Sorafenib. Pharmaceutics 12, E788. doi:10.3390/pharmaceutics12090788
Iijima, R., Watanabe, T., Ishiuchi, K., Matsumoto, T., Watanabe, J., and Makino, T. (2018). Interactions between Crude Drug Extracts Used in Japanese Traditional Kampo Medicines and Organic Anion-Transporting Polypeptide 2B1. J. Ethnopharmacol 214, 153–159. doi:10.1016/j.jep.2017.12.016
Ishiguro, N., Maeda, K., Saito, A., Kishimoto, W., Matsushima, S., Ebner, T., et al. (2008). Establishment of a Set of Double Transfectants Coexpressing Organic Anion Transporting Polypeptide 1B3 and Hepatic Efflux Transporters for the Characterization of the Hepatobiliary Transport of Telmisartan Acylglucuronide. Drug Metab. Dispos 36, 796–805. doi:10.1124/dmd.107.018903
Ito, Y., Fukami, T., Yokoi, T., and Nakajima, M. (2014). An Orphan Esterase ABHD10 Modulates Probenecid Acyl Glucuronidation in Human Liver. Drug Metab. Dispos 42, 2109–2116. doi:10.1124/dmd.114.059485
Iusuf, D., van de Steeg, E., and Schinkel, A. H. (2012). Hepatocyte Hopping of OATP1B Substrates Contributes to Efficient Hepatic Detoxification. Clin. Pharmacol. Ther. 92, 559–562. doi:10.1038/clpt.2012.143
Iwaki, M., Shimada, H., Irino, Y., Take, M., and Egashira, S. (2017). Inhibition of Methotrexate Uptake via Organic Anion Transporters OAT1 and OAT3 by Glucuronides of Nonsteroidal Anti-inflammatory Drugs. Biol. Pharm. Bull. 40, 926–931. doi:10.1248/bpb.b16-00970
Jahnel, J., Fickert, P., Hauer, A. C., Högenauer, C., Avian, A., and Trauner, M. (2014). Inflammatory Bowel Disease Alters Intestinal Bile Acid Transporter Expression. Drug Metab. Dispos 42, 1423–1431. doi:10.1124/dmd.114.058065
Järvinen, E., Deng, F., Kidron, H., and Finel, M. (2018). Efflux Transport of Estrogen Glucuronides by Human MRP2, MRP3, MRP4 and BCRP. J. Steroid Biochem. Mol. Biol. 178, 99–107. doi:10.1016/j.jsbmb.2017.11.007
Järvinen, E., Kidron, H., and Finel, M. (2020). Human Efflux Transport of Testosterone, Epitestosterone and Other Androgen Glucuronides. J. Steroid Biochem. Mol. Biol. 197, 105518. doi:10.1016/j.jsbmb.2019.105518
Järvinen, E., Troberg, J., Kidron, H., and Finel, M. (2017). Selectivity in the Efflux of Glucuronides by Human Transporters: MRP4 Is Highly Active toward 4-Methylumbelliferone and 1-Naphthol Glucuronides, while MRP3 Exhibits Stereoselective Propranolol Glucuronide Transport. Mol. Pharm. 14, 3299–3311. doi:10.1021/acs.molpharmaceut.7b00366
Jeong, E. J., Lin, H., and Hu, M. (2004). Disposition Mechanisms of Raloxifene in the Human Intestinal Caco-2 Model. J. Pharmacol. Exp. Ther. 310, 376–385. doi:10.1124/jpet.103.063925
Jeong, E. S., Kim, Y. W., Kim, H. J., Shin, H. J., Shin, J. G., Kim, K. H., et al. (2015). Glucuronidation of Fimasartan, a New Angiotensin Receptor Antagonist, Is Mainly Mediated by UGT1A3. Xenobiotica 45, 10–18. doi:10.3109/00498254.2014.942810
Ji, J. Z., Tai, T., Huang, B. B., Gu, T. T., Mi, Q. Y., and Xie, H. G. (2018). Mrp3 Transports Clopidogrel Acyl Glucuronide from the Hepatocytes into Blood. Drug Metab. Dispos 46, 151–154. doi:10.1124/dmd.117.078329
Kaivosaari, S., Finel, M., and Koskinen, M. (2011). N-glucuronidation of Drugs and Other Xenobiotics by Human and Animal UDP-Glucuronosyltransferases. Xenobiotica 41, 652–669. doi:10.3109/00498254.2011.563327
Kaku, K., Enya, K., Nakaya, R., Ohira, T., and Matsuno, R. (2015). Efficacy and Safety of Fasiglifam (TAK-875), a G Protein-Coupled Receptor 40 Agonist, in Japanese Patients with Type 2 Diabetes Inadequately Controlled by Diet and Exercise: a Randomized, Double-Blind, Placebo-Controlled, Phase III Trial. Diabetes Obes. Metab. 17, 675–681. doi:10.1111/dom.12467
Kaku, K. (2013). Fasiglifam as a New Potential Treatment Option for Patients with Type 2 Diabetes. Expert Opin. Pharmacother. 14, 2591–2600. doi:10.1517/14656566.2013.851668
Kalapos-Kovács, B., Magda, B., Jani, M., Fekete, Z., Szabó, P. T., Antal, I., et al. (2015). Multiple ABC Transporters Efflux Baicalin. Phytother Res. 29, 1987–1990. doi:10.1002/ptr.5477
Kamisako, T., Leier, I., Cui, Y., König, J., Buchholz, U., Hummel-Eisenbeiss, J., et al. (1999). Transport of Monoglucuronosyl and Bisglucuronosyl Bilirubin by Recombinant Human and Rat Multidrug Resistance Protein 2. Hepatology 30, 485–490. doi:10.1002/hep.510300220
Kanamitsu, K., Kusuhara, H., Schuetz, J. D., Takeuchi, K., and Sugiyama, Y. (2017). Investigation of the Importance of Multidrug Resistance-Associated Protein 4 (Mrp4/Abcc4) in the Active Efflux of Anionic Drugs across the Blood-Brain Barrier. J. Pharm. Sci. 106, 2566–2575. doi:10.1016/j.xphs.2017.04.040
Karlgren, M., Vildhede, A., Norinder, U., Wisniewski, J. R., Kimoto, E., Lai, Y., et al. (2012). Classification of Inhibitors of Hepatic Organic Anion Transporting Polypeptides (OATPs): Influence of Protein Expression on Drug-Drug Interactions. J. Med. Chem. 55, 4740–4763. doi:10.1021/jm300212s
Kartenbeck, J., Leuschner, U., Mayer, R., and Keppler, D. (1996). Absence of the Canalicular Isoform of the MRP Gene-Encoded Conjugate export Pump from the Hepatocytes in Dubin-Johnson Syndrome. Hepatology 23, 1061–1066. doi:10.1053/jhep.1996.v23.pm0008621134
Katsube, Y., Tsujimoto, M., Koide, H., Ochiai, M., Hojyo, A., Ogawa, K., et al. (2017). Cooperative Inhibitory Effects of Uremic Toxins and Other Serum Components on OATP1B1-Mediated Transport of SN-38. Cancer Chemother. Pharmacol. 79, 783–789. doi:10.1007/s00280-017-3276-y
Kawase, A., Yamamoto, T., Egashira, S., and Iwaki, M. (2016). Stereoselective Inhibition of Methotrexate Excretion by Glucuronides of Nonsteroidal Anti-inflammatory Drugs via Multidrug Resistance Proteins 2 and 4. J. Pharmacol. Exp. Ther. 356, 366–374. doi:10.1124/jpet.115.229104
Keiser, M., Kaltheuner, L., Wildberg, C., Müller, J., Grube, M., Partecke, L. I., et al. (2017). The Organic Anion-Transporting Peptide 2B1 Is Localized in the Basolateral Membrane of the Human Jejunum and Caco-2 Monolayers. J. Pharm. Sci. 106, 2657–2663. doi:10.1016/j.xphs.2017.04.001
Kenna, J. G., Taskar, K. S., Battista, C., Bourdet, D. L., Brouwer, K. L. R., Brouwer, K. R., et al. (2018). Can Bile Salt Export Pump Inhibition Testing in Drug Discovery and Development Reduce Liver Injury Risk? an International Transporter Consortium Perspective. Clin. Pharmacol. Ther. 104, 916–932. doi:10.1002/cpt.1222
Kimoto, E., Li, R., Scialis, R. J., Lai, Y., and Varma, M. V. (2015). Hepatic Disposition of Gemfibrozil and its Major Metabolite Gemfibrozil 1-O-β-Glucuronide. Mol. Pharm. 12, 3943–3952. doi:10.1021/acs.molpharmaceut.5b00411
King, C., Tang, W., Ngui, J., Tephly, T., and Braun, M. (2001). Characterization of Rat and Human UDP-Glucuronosyltransferases Responsible for the In Vitro Glucuronidation of Diclofenac. Toxicol. Sci. 61, 49–53. doi:10.1093/toxsci/61.1.49
Kobayashi, Y., Ohshiro, N., Sakai, R., Ohbayashi, M., Kohyama, N., and Yamamoto, T. (2005). Transport Mechanism and Substrate Specificity of Human Organic Anion Transporter 2 (hOat2 [SLC22A7]). J. Pharm. Pharmacol. 57, 573–578. doi:10.1211/0022357055966
Kogame, A., Lee, R., Pan, L., Sudo, M., Nonaka, M., Moriya, Y., et al. (2019). Disposition and Metabolism of the G Protein-Coupled Receptor 40 Agonist TAK-875 (Fasiglifam) in Rats, Dogs, and Humans. Xenobiotica 49, 433–445. doi:10.1080/00498254.2018.1453100
Kong, F., Pang, X., Zhong, K., Guo, Z., Li, X., Zhong, D., et al. (2017). Increased Plasma Exposures of Conjugated Metabolites of Morinidazole in Renal Failure Patients: A Critical Role of Uremic Toxins. Drug Metab. Dispos 45, 593–603. doi:10.1124/dmd.116.074492
Kong, L. L., Shen, G. L., Wang, Z. Y., Zhuang, X. M., Xiao, W. B., Yuan, M., et al. (2016). Inhibition of P-Glycoprotein and Multidrug Resistance-Associated Protein 2 Regulates the Hepatobiliary Excretion and Plasma Exposure of Thienorphine and its Glucuronide Conjugate. Front. Pharmacol. 7, 242. doi:10.3389/fphar.2016.00242
König, J., Cui, Y., Nies, A. T., and Keppler, D. (2000). A Novel Human Organic Anion Transporting Polypeptide Localized to the Basolateral Hepatocyte Membrane. Am. J. Physiol. Gastrointest. Liver Physiol. 278, G156–G164. doi:10.1152/ajpgi.2000.278.1.G156
König, J., Rost, D., Cui, Y., and Keppler, D. (1999). Characterization of the Human Multidrug Resistance Protein Isoform MRP3 Localized to the Basolateral Hepatocyte Membrane. Hepatology 29, 1156–1163. doi:10.1002/hep.510290404
Kosaka, K., Watanabe, T., Susukida, T., Aoki, S., Sekine, S., Kume, T., et al. (2015). Key Determinants of the Circulatory Exposure of Organic Anions: Differences in Hepatic Uptake between Multidrug Resistance-Associated Protein 2 (Mrp2)-Deficient Rats and Wild-type Rats. Xenobiotica 45, 556–562. doi:10.3109/00498254.2014.997820
Kuroda, M., Kobayashi, Y., Tanaka, Y., Itani, T., Mifuji, R., Araki, J., et al. (2004). Increased Hepatic and Renal Expressions of Multidrug Resistance-Associated Protein 3 in Eisai Hyperbilirubinuria Rats. J. Gastroenterol. Hepatol. 19, 146–153. doi:10.1111/j.1440-1746.2004.03275.x
Kurzawski, M., Szeląg-Pieniek, S., Łapczuk-Romańska, J., Wrzesiński, M., Sieńko, J., Oswald, S., et al. (2019). The Reference Liver - ABC and SLC Drug Transporters in Healthy Donor and Metastatic Livers. Pharmacol. Rep. 71, 738–745. doi:10.1016/j.pharep.2019.04.001
Kutsuno, Y., Sumida, K., Itoh, T., Tukey, R. H., and Fujiwara, R. (2013). Glucuronidation of Drugs in Humanized UDP-Glucuronosyltransferase 1 Mice: Similarity with Glucuronidation in Human Liver Microsomes. Pharmacol. Res. Perspect. 1, e00002. doi:10.1002/prp2.2
Lacy, S., Hsu, B., Miles, D., Aftab, D., Wang, R., and Nguyen, L. (2015). Metabolism and Disposition of Cabozantinib in Healthy Male Volunteers and Pharmacologic Characterization of its Major Metabolites. Drug Metab. Dispos 43, 1190–1207. doi:10.1124/dmd.115.063610
Ladumor, M. K., Bhatt, D. K., Gaedigk, A., Sharma, S., Thakur, A., Pearce, R. E., et al. (2019). Ontogeny of Hepatic Sulfotransferases and Prediction of Age-dependent Fractional Contribution of Sulfation in Acetaminophen Metabolism. Drug Metab. Dispos 47, 818–831. doi:10.1124/dmd.119.086462
Lagas, J. S., Sparidans, R. W., Wagenaar, E., Beijnen, J. H., and Schinkel, A. H. (2010). Hepatic Clearance of Reactive Glucuronide Metabolites of Diclofenac in the Mouse Is Dependent on Multiple ATP-Binding Cassette Efflux Transporters. Mol. Pharmacol. 77, 687–694. doi:10.1124/mol.109.062364
Lamba, V., Sangkuhl, K., Sanghavi, K., Fish, A., Altman, R. B., and Klein, T. E. (2014). PharmGKB Summary: Mycophenolic Acid Pathway. Pharmacogenet Genomics 24, 73–79. doi:10.1097/FPC.0000000000000010
Lathia, C., Lettieri, J., Cihon, F., Gallentine, M., Radtke, M., and Sundaresan, P. (2006). Lack of Effect of Ketoconazole-Mediated CYP3A Inhibition on Sorafenib Clinical Pharmacokinetics. Cancer Chemother. Pharmacol. 57, 685–692. doi:10.1007/s00280-005-0068-6
Lempers, V. J., van den Heuvel, J. J., Russel, F. G., Aarnoutse, R. E., Burger, D. M., Brüggemann, R. J., et al. (2016). Inhibitory Potential of Antifungal Drugs on ATP-Binding Cassette Transporters P-Glycoprotein, MRP1 to MRP5, BCRP, and BSEP. Antimicrob. Agents Chemother. 60, 3372–3379. doi:10.1128/AAC.02931-15
Li, C. Y., Basit, A., Gupta, A., Gáborik, Z., Kis, E., and Prasad, B. (2019a). Major Glucuronide Metabolites of Testosterone Are Primarily Transported by MRP2 and MRP3 in Human Liver, Intestine and Kidney. J. Steroid Biochem. Mol. Biol. 191, 105350. doi:10.1016/j.jsbmb.2019.03.027
Li, C. Y., Gupta, A., Gáborik, Z., Kis, E., and Prasad, B. (2020). Organic Anion Transporting Polypeptide-Mediated Hepatic Uptake of Glucuronide Metabolites of Androgens. Mol. Pharmacol. 98, 234–242. doi:10.1124/mol.120.119891
Li, C. Y., Hosey-Cojocari, C., Basit, A., Unadkat, J. D., Leeder, J. S., and Prasad, B. (2019b). Optimized Renal Transporter Quantification by Using Aquaporin 1 and Aquaporin 2 as Anatomical Markers: Application in Characterizing the Ontogeny of Renal Transporters and its Correlation with Hepatic Transporters in Paired Human Samples. AAPS J. 21, 88. doi:10.1208/s12248-019-0359-1
Li, D., Chen, L., Li, Y., Tian, S., Sun, H., and Hou, T. (2014). ADMET Evaluation in Drug Discovery. 13. Development of In Silico Prediction Models for P-Glycoprotein Substrates. Mol. Pharm. 11, 716–726. doi:10.1021/mp400450m
Li, W., Sun, H., Zhang, X., Wang, H., and Wu, B. (2015). Efflux Transport of Chrysin and Apigenin Sulfates in HEK293 Cells Overexpressing SULT1A3: The Role of Multidrug Resistance-Associated Protein 4 (MRP4/ABCC4). Biochem. Pharmacol. 98, 203–214. doi:10.1016/j.bcp.2015.08.090
Li, X., Wu, B., Wang, L., and Li, S. (2006). Extractable Amounts of Trans-resveratrol in Seed and berry Skin in Vitis Evaluated at the Germplasm Level. J. Agric. Food Chem. 54, 8804–8811. doi:10.1021/jf061722y
Lin, S. P., Yu, C. P., Hou, Y. C., Huang, C. Y., Ho, L. C., and Chan, S. L. (2018). Transporter-mediated Interaction of Indican and Methotrexate in Rats. J. Food Drug Anal. 26, S133–S140. doi:10.1016/j.jfda.2017.11.006
Loi, C. M., Smith, D. A., and Dalvie, D. (2013). Which Metabolites Circulate? Drug Metab. Dispos 41, 933–951. doi:10.1124/dmd.112.050278
Maeno, K., Nakajima, A., Conseil, G., Rothnie, A., Deeley, R. G., and Cole, S. P. (2009). Molecular Basis for Reduced Estrone Sulfate Transport and Altered Modulator Sensitivity of Transmembrane helix (TM) 6 and TM17 Mutants of Multidrug Resistance Protein 1 (ABCC1). Drug Metab. Dispos 37, 1411–1420. doi:10.1124/dmd.109.026633
Malinen, M. M., Kauttonen, A., Beaudoin, J. J., Sjöstedt, N., Honkakoski, P., and Brouwer, K. L. R. (2019). Novel In Vitro Method Reveals Drugs that Inhibit Organic Solute Transporter Alpha/Beta (OSTα/β). Mol. Pharm. 16, 238–246. doi:10.1021/acs.molpharmaceut.8b00966
Malofeeva, E. V., Domanitskaya, N., Gudima, M., and Hopper-Borge, E. A. (2012). Modulation of the ATPase and Transport Activities of Broad-Acting Multidrug Resistance Factor ABCC10 (MRP7). Cancer Res. 72, 6457–6467. doi:10.1158/0008-5472.CAN-12-1340
Manach, C., Scalbert, A., Morand, C., Rémésy, C., and Jiménez, L. (2004). Polyphenols: Food Sources and Bioavailability. Am. J. Clin. Nutr. 79, 727–747. doi:10.1093/ajcn/79.5.727
Manners, C. N., Payling, D. W., and Smith, D. A. (1988). Distribution Coefficient, a Convenient Term for the Relation of Predictable Physico-Chemical Properties to Metabolic Processes. Xenobiotica 18, 331–350. doi:10.3109/00498258809041669
Masubuchi, Y. (2006). Metabolic and Non-metabolic Factors Determining Troglitazone Hepatotoxicity: a Review. Drug Metab. Pharmacokinet. 21, 347–356. doi:10.2133/dmpk.21.347
Mathialagan, S., Costales, C., Tylaska, L., Kimoto, E., Vildhede, A., Johnson, J., et al. (2018). In Vitro studies with Two Human Organic Anion Transporters: OAT2 and OAT7. Xenobiotica 48, 1037–1049. doi:10.1080/00498254.2017.1384595
Matsunaga, N., Nunoya, K., Okada, M., Ogawa, M., and Tamai, I. (2013). Evaluation of Hepatic Disposition of Paroxetine Using sandwich-cultured Rat and Human Hepatocytes. Drug Metab. Dispos 41, 735–743. doi:10.1124/dmd.112.049817
Matsunaga, N., Wada, S., Nakanishi, T., Ikenaga, M., Ogawa, M., and Tamai, I. (2014). Mathematical Modeling of the In Vitro Hepatic Disposition of Mycophenolic Acid and its Glucuronide in sandwich-cultured Human Hepatocytes. Mol. Pharm. 11, 568–579. doi:10.1021/mp400513k
Matsushima, S., Maeda, K., Hayashi, H., Debori, Y., Schinkel, A. H., Schuetz, J. D., et al. (2008). Involvement of Multiple Efflux Transporters in Hepatic Disposition of Fexofenadine. Mol. Pharmacol. 73, 1474–1483. doi:10.1124/mol.107.041459
McFeely, S. J., Wu, L., Ritchie, T. K., and Unadkat, J. (2019). Organic Anion Transporting Polypeptide 2B1 - More Than a Glass-Full of Drug Interactions. Pharmacol. Ther. 196, 204–215. doi:10.1016/j.pharmthera.2018.12.009
Meyer Zu Schwabedissen, H. E., Ferreira, C., Schaefer, A. M., Oufir, M., Seibert, I., Hamburger, M., et al. (2018). Thyroid Hormones Are Transport Substrates and Transcriptional Regulators of Organic Anion Transporting Polypeptide 2B1. Mol. Pharmacol. 94, 700–712. doi:10.1124/mol.117.111161
Michelon, H., König, J., Durrbach, A., Quteineh, L., Verstuyft, C., Furlan, V., et al. (2010). SLCO1B1 Genetic Polymorphism Influences Mycophenolic Acid Tolerance in Renal Transplant Recipients. Pharmacogenomics 11, 1703–1713. doi:10.2217/pgs.10.132
Ming, X., and Thakker, D. R. (2010). Role of Basolateral Efflux Transporter MRP4 in the Intestinal Absorption of the Antiviral Drug Adefovir Dipivoxil. Biochem. Pharmacol. 79, 455–462. doi:10.1016/j.bcp.2009.08.029
Miura, M., Kagaya, H., Satoh, S., Inoue, K., Saito, M., Habuchi, T., et al. (2008). Influence of Drug Transporters and UGT Polymorphisms on Pharmacokinetics of Phenolic Glucuronide Metabolite of Mycophenolic Acid in Japanese Renal Transplant Recipients. Ther. Drug Monit. 30, 559–564. doi:10.1097/FTD.0b013e3181838063
Mizuno, N., Takahashi, T., Iwase, Y., Kusuhara, H., Niwa, T., and Sugiyama, Y. (2007a). Human Organic Anion Transporters 1 (hOAT1/SLC22A6) and 3 (hOAT3/SLC22A8) Transport Edaravone (MCI-186; 3-Methyl-1-Phenyl-2-Pyrazolin-5-One) and its Sulfate Conjugate. Drug Metab. Dispos 35, 1429–1434. doi:10.1124/dmd.106.013912
Mizuno, N., Takahashi, T., Kusuhara, H., Schuetz, J. D., Niwa, T., and Sugiyama, Y. (2007b). Evaluation of the Role of Breast Cancer Resistance Protein (BCRP/ABCG2) and Multidrug Resistance-Associated Protein 4 (MRP4/ABCC4) in the Urinary Excretion of Sulfate and Glucuronide Metabolites of Edaravone (MCI-186; 3-Methyl-1-Phenyl-2-Pyrazolin-5-One). Drug Metab. Dispos 35, 2045–2052. doi:10.1124/dmd.107.016352
Mohos, V., Fliszár-Nyúl, E., Ungvári, O., Bakos, É., Kuffa, K., Bencsik, T., et al. (2020a). Effects of Chrysin and its Major Conjugated Metabolites Chrysin-7-Sulfate and Chrysin-7-Glucuronide on Cytochrome P450 Enzymes and on OATP, P-Gp, BCRP, and MRP2 Transporters. Drug Metab. Dispos 48, 1064–1073. doi:10.1124/dmd.120.000085
Mohos, V., Fliszár-Nyúl, E., Ungvári, O., Kuffa, K., Needs, P. W., Kroon, P. A., et al. (2020b). Inhibitory Effects of Quercetin and its Main Methyl, Sulfate, and Glucuronic Acid Conjugates on Cytochrome P450 Enzymes, and on OATP, BCRP and MRP2 Transporters. Nutrients 12, E2306. doi:10.3390/nu12082306
Mori, D., Ishida, H., Mizuno, T., Kusumoto, S., Kondo, Y., Izumi, S., et al. (2020a). Alteration in the Plasma Concentrations of Endogenous Organic Anion-Transporting Polypeptide 1B Biomarkers in Patients with Non-small Cell Lung Cancer Treated with Paclitaxel. Drug Metab. Dispos 48, 387–394. doi:10.1124/dmd.119.089474
Mori, D., Kashihara, Y., Yoshikado, T., Kimura, M., Hirota, T., Matsuki, S., et al. (2019). Effect of OATP1B1 Genotypes on Plasma Concentrations of Endogenous OATP1B1 Substrates and Drugs, and Their Association in Healthy Volunteers. Drug Metab. Pharmacokinet. 34, 78–86. doi:10.1016/j.dmpk.2018.09.003
Mori, D., Kimoto, E., Rago, B., Kondo, Y., King-Ahmad, A., Ramanathan, R., et al. (2020b). Dose-Dependent Inhibition of OATP1B by Rifampicin in Healthy Volunteers: Comprehensive Evaluation of Candidate Biomarkers and OATP1B Probe Drugs. Clin. Pharmacol. Ther. 107, 1004–1013. doi:10.1002/cpt.1695
Morimoto, K., Tominaga, Y., Agatsuma, Y., Miyamoto, M., Kashiwagura, S., Takahashi, A., et al. (2018). Intestinal Secretion of Indoxyl Sulfate as a Possible Compensatory Excretion Pathway in Chronic Kidney Disease. Biopharm. Drug Dispos 39, 328–334. doi:10.1002/bdd.2149
Motojima, M., Hosokawa, A., Yamato, H., Muraki, T., and Yoshioka, T. (2002). Uraemic Toxins Induce Proximal Tubular Injury via Organic Anion Transporter 1-mediated Uptake. Br. J. Pharmacol. 135, 555–563. doi:10.1038/sj.bjp.0704482
Mueller, J. W., Gilligan, L. C., Idkowiak, J., Arlt, W., and Foster, P. A. (2015). The Regulation of Steroid Action by Sulfation and Desulfation. Endocr. Rev. 36, 526–563. doi:10.1210/er.2015-1036
Murai, T., Oda, K., Toyo, T., Nittono, H., Takei, H., Muto, A., et al. (2013). Measurement of Transport Activities of 3β-Hydroxy-Δ(5)-Bile Acids in Bile Salt export Pump and Multidrug Resistance-Associated Proteins Using LC-MS/MS. Chem. Pharm. Bull. (Tokyo) 61, 559–566. doi:10.1248/cpb.c13-00001
Mutsaers, H. A., Caetano-Pinto, P., Seegers, A. E., Dankers, A. C., van den Broek, P. H., Wetzels, J. F., et al. (2015). Proximal Tubular Efflux Transporters Involved in Renal Excretion of P-Cresyl Sulfate and P-Cresyl Glucuronide: Implications for Chronic Kidney Disease Pathophysiology. Toxicol. Vitro 29, 1868–1877. doi:10.1016/j.tiv.2015.07.020
Mutsaers, H. A., van den Heuvel, L. P., Ringens, L. H., Dankers, A. C., Russel, F. G., Wetzels, J. F., et al. (2011). Uremic Toxins Inhibit Transport by Breast Cancer Resistance Protein and Multidrug Resistance Protein 4 at Clinically Relevant Concentrations. PLoS One 6, e18438. doi:10.1371/journal.pone.0018438
Naesens, M., Kuypers, D. R., Streit, F., Armstrong, V. W., Oellerich, M., Verbeke, K., et al. (2006). Rifampin Induces Alterations in Mycophenolic Acid Glucuronidation and Elimination: Implications for Drug Exposure in Renal Allograft Recipients. Clin. Pharmacol. Ther. 80, 509–521. doi:10.1016/j.clpt.2006.08.002
Nakagomi-Hagihara, R., Nakai, D., and Tokui, T. (2007). Inhibition of Human Organic Anion Transporter 3 Mediated Pravastatin Transport by Gemfibrozil and the Metabolites in Humans. Xenobiotica 37, 416–426. doi:10.1080/00498250601188808
Nakagomi-Hagihara, R., Nakai, D., Tokui, T., Abe, T., and Ikeda, T. (2007b). Gemfibrozil and Its Glucuronide Inhibit the Hepatic Uptake of Pravastatin Mediated by OATP1B1. Xenobiotica 37, 474–486. doi:10.1080/00498250701278442
Neuvonen, M., Hirvensalo, P., Tornio, A., Rago, B., West, M., Lazzaro, S., et al. (2021). Identification of Glycochenodeoxycholate 3-O-Glucuronide and Glycodeoxycholate 3-O-Glucuronide as Highly Sensitive and Specific OATP1B1 Biomarkers. Clin. Pharmacol. Ther. 109, 646–657. doi:10.1002/cpt.2053
Niu, X., de Graaf, I. A., van de Vegte, D., Langelaar-Makkinje, M., Sekine, S., and Groothuis, G. M. (2015). Consequences of Mrp2 Deficiency for Diclofenac Toxicity in the Rat Intestine Ex Vivo. Toxicol. Vitro 29, 168–175. doi:10.1016/j.tiv.2014.10.004
Novelle, M. G., Wahl, D., Diéguez, C., Bernier, M., and de Cabo, R. (2015). Resveratrol Supplementation: Where Are We Now and where Should We Go? Ageing Res. Rev. 21, 1–15. doi:10.1016/j.arr.2015.01.002
Nozaki, Y., Kusuhara, H., Kondo, T., Iwaki, M., Shiroyanagi, Y., Nakayama, H., et al. (2007). Species Difference in the Inhibitory Effect of Nonsteroidal Anti-inflammatory Drugs on the Uptake of Methotrexate by Human Kidney Slices. J. Pharmacol. Exp. Ther. 322, 1162–1170. doi:10.1124/jpet.107.121491
Nozawa, T., Minami, H., Sugiura, S., Tsuji, A., and Tamai, I. (2005). Role of Organic Anion Transporter OATP1B1 (OATP-C) in Hepatic Uptake of Irinotecan and its Active Metabolite, 7-Ethyl-10-Hydroxycamptothecin: In Vitro Evidence and Effect of Single Nucleotide Polymorphisms. Drug Metab. Dispos 33, 434–439. doi:10.1124/dmd.104.001909
Nozawa, T., Sugiura, S., Nakajima, M., Goto, A., Yokoi, T., Nezu, J., et al. (2004). Involvement of Organic Anion Transporting Polypeptides in the Transport of Troglitazone Sulfate: Implications for Understanding Troglitazone Hepatotoxicity. Drug Metab. Dispos 32, 291–294. doi:10.1124/dmd.32.3.291
Oda, S., Fukami, T., Yokoi, T., and Nakajima, M. (2015). A Comprehensive Review of UDP-Glucuronosyltransferase and Esterases for Drug Development. Drug Metab. Pharmacokinet. 30, 30–51. doi:10.1016/j.dmpk.2014.12.001
Odar-Cederlöf, I., Vessman, J., Alván, G., and Sjöqvist, F. (1977). Oxazepam Disposition in Uremic Patients. Acta Pharmacol. Toxicol. (Copenh) 40 (Suppl. 1), 52–62.
Ogilvie, B. W., Zhang, D., Li, W., Rodrigues, A. D., Gipson, A. E., Holsapple, J., et al. (2006). Glucuronidation Converts Gemfibrozil to a Potent, Metabolism-dependent Inhibitor of CYP2C8: Implications for Drug-Drug Interactions. Drug Metab. Dispos 34, 191–197. doi:10.1124/dmd.105.007633
Oswald, S., Haenisch, S., Fricke, C., Sudhop, T., Remmler, C., Giessmann, T., et al. (2006). Intestinal Expression of P-Glycoprotein (ABCB1), Multidrug Resistance Associated Protein 2 (ABCC2), and Uridine Diphosphate-Glucuronosyltransferase 1A1 Predicts the Disposition and Modulates the Effects of the Cholesterol Absorption Inhibitor Ezetimibe in Humans. Clin. Pharmacol. Ther. 79, 206–217. doi:10.1016/j.clpt.2005.11.004
Oswald, S., König, J., Lütjohann, D., Giessmann, T., Kroemer, H. K., Rimmbach, C., et al. (2008). Disposition of Ezetimibe Is Influenced by Polymorphisms of the Hepatic Uptake Carrier OATP1B1. Pharmacogenet Genomics 18, 559–568. doi:10.1097/FPC.0b013e3282fe9a2c
Oswald, S., Müller, J., Neugebauer, U., Schröter, R., Herrmann, E., Pavenstädt, H., et al. (2019). Protein Abundance of Clinically Relevant Drug Transporters in the Human Kidneys. Int. J. Mol. Sci. 20, 5303. doi:10.3390/ijms20215303
Otieno, M. A., Snoeys, J., Lam, W., Ghosh, A., Player, M. R., Pocai, A., et al. (2018). Fasiglifam (TAK-875): Mechanistic Investigation and Retrospective Identification of Hazards for Drug Induced Liver Injury. Toxicol. Sci. 163, 374–384. doi:10.1093/toxsci/kfx040
Pang, K. S., Schwab, A. J., Goresky, C. A., and Chiba, M. (1994). Transport, Binding, and Metabolism of Sulfate Conjugates in the Liver. Chem. Biol. Interact 92, 179–207. doi:10.1016/0009-2797(94)90063-9
Patel, C. G., Ogasawara, K., and Akhlaghi, F. (2013). Mycophenolic Acid Glucuronide Is Transported by Multidrug Resistance-Associated Protein 2 and This Transport Is Not Inhibited by Cyclosporine, Tacrolimus or Sirolimus. Xenobiotica 43, 229–235. doi:10.3109/00498254.2012.713531
Patel, M., Eberl, H. C., Wolf, A., Pierre, E., Polli, J. W., and Zamek-Gliszczynski, M. J. (2019). Mechanistic Basis of Cabotegravir-Glucuronide Disposition in Humans. J. Pharmacol. Exp. Ther. 370, 269–277. doi:10.1124/jpet.119.258384
Patel, M., Taskar, K. S., and Zamek-Gliszczynski, M. J. (2016). Importance of Hepatic Transporters in Clinical Disposition of Drugs and Their Metabolites. J. Clin. Pharmacol. 56 (Suppl. 7), S23–S39. doi:10.1002/jcph.671
Pedersen, J. M., Khan, E. K., Bergström, C. A. S., Palm, J., Hoogstraate, J., and Artursson, P. (2017). Substrate and Method Dependent Inhibition of Three ABC-Transporters (MDR1, BCRP, and MRP2). Eur. J. Pharm. Sci. 103, 70–76. doi:10.1016/j.ejps.2017.03.002
Perez-Vizcaino, F., Duarte, J., and Santos-Buelga, C. (2012). The Flavonoid Paradox: Conjugation and Deconjugation as Key Steps for the Biological Activity of Flavonoids. J. Sci. Food Agric. 92, 1822–1825. doi:10.1002/jsfa.5697
Picard, N., Yee, S. W., Woillard, J. B., Lebranchu, Y., Le Meur, Y., Giacomini, K. M., et al. (2010). The Role of Organic Anion-Transporting Polypeptides and Their Common Genetic Variants in Mycophenolic Acid Pharmacokinetics. Clin. Pharmacol. Ther. 87, 100–108. doi:10.1038/clpt.2009.205
Pizzagalli, F., Hagenbuch, B., Stieger, B., Klenk, U., Folkers, G., and Meier, P. J. (2002). Identification of a Novel Human Organic Anion Transporting Polypeptide as a High Affinity Thyroxine Transporter. Mol. Endocrinol. 16, 2283–2296. doi:10.1210/me.2001-0309
Poller, B., Woessner, R., Barve, A., Tillmann, H. C., Vemula, J., Nica, A., et al. (2019). Fevipiprant Has a Low Risk of Influencing Co-medication Pharmacokinetics: Impact on Simvastatin and Rosuvastatin in Different SLCO1B1 Genotypes. Pulm. Pharmacol. Ther. 57, 101809. doi:10.1016/j.pupt.2019.101809
Pollet, R. M., D'Agostino, E. H., Walton, W. G., Xu, Y., Little, M. S., Biernat, K. A., et al. (2017). An Atlas of β-Glucuronidases in the Human Intestinal Microbiome. Structure 25, 967-e5. doi:10.1016/j.str.2017.05.003
Prasad, B., Johnson, K., Billington, S., Lee, C., Chung, G. W., Brown, C. D., et al. (2016). Abundance of Drug Transporters in the Human Kidney Cortex as Quantified by Quantitative Targeted Proteomics. Drug Metab. Dispos 44, 1920–1924. doi:10.1124/dmd.116.072066
Regan, S. L., Maggs, J. L., Hammond, T. G., Lambert, C., Williams, D. P., and Park, B. K. (2010). Acyl Glucuronides: the Good, the Bad and the Ugly. Biopharm. Drug Dispos 31, 367–395. doi:10.1002/bdd.720
Reyes, M., and Benet, L. Z. (2011). Effects of Uremic Toxins on Transport and Metabolism of Different Biopharmaceutics Drug Disposition Classification System Xenobiotics. J. Pharm. Sci. 100, 3831–3842. doi:10.1002/jps.22640
Riches, Z., Stanley, E. L., Bloomer, J. C., and Coughtrie, M. W. (2009). Quantitative Evaluation of the Expression and Activity of Five Major Sulfotransferases (SULTs) in Human Tissues: the SULT "pie". Drug Metab. Dispos 37, 2255–2261. doi:10.1124/dmd.109.028399
Rižner, T. L. (2013). Estrogen Biosynthesis, Phase I and Phase II Metabolism, and Action in Endometrial Cancer. Mol. Cell Endocrinol. 381, 124–139. doi:10.1016/j.mce.2013.07.026
Roberts, M. S., Magnusson, B. M., Burczynski, F. J., and Weiss, M. (2002). Enterohepatic Circulation: Physiological, Pharmacokinetic and Clinical Implications. Clin. Pharmacokinet. 41, 751–790. doi:10.2165/00003088-200241100-00005
Sakamoto, S., Kusuhara, H., Horie, K., Takahashi, K., Baba, T., Ishizaki, J., et al. (2008). Identification of the Transporters Involved in the Hepatobiliary Transport and Intestinal Efflux of Methyl 1-(3,4-Dimethoxyphenyl)-3-(3-Ethylvaleryl)-4-Hydroxy-6,7,8-Trimethoxy-2-Naphthoate (S-8921) Glucuronide, a Pharmacologically Active Metabolite of S-8921. Drug Metab. Dispos 36, 1553–1561. doi:10.1124/dmd.108.020511
Sakamoto, S., Kusuhara, H., Miyata, K., Shimaoka, H., Kanazu, T., Matsuo, Y., et al. (2007). Glucuronidation Converting Methyl 1-(3,4-Dimethoxyphenyl)-3-(3-Ethylvaleryl)-4-Hydroxy-6,7,8-Trimethoxy-2-Naphthoate (S-8921) to a Potent Apical Sodium-dependent Bile Acid Transporter Inhibitor, Resulting in a Hypocholesterolemic Action. J. Pharmacol. Exp. Ther. 322, 610–618. doi:10.1124/jpet.106.116426
Sakurai, Y., Motohashi, H., Ueo, H., Masuda, S., Saito, H., Okuda, M., et al. (2004). Expression Levels of Renal Organic Anion Transporters (OATs) and Their Correlation with Anionic Drug Excretion in Patients with Renal Diseases. Pharm. Res. 21, 61–67. doi:10.1023/b:pham.0000012153.71993.cb
Sato, T., Yamaguchi, H., Kogawa, T., Abe, T., and Mano, N. (2014). Organic Anion Transporting Polypeptides 1B1 and 1B3 Play an Important Role in Uremic Toxin Handling and Drug-Uremic Toxin Interactions in the Liver. J. Pharm. Pharm. Sci. 17, 475–484. doi:10.18433/j3m89q
Sato, Y., Nagata, M., Tetsuka, K., Tamura, K., Miyashita, A., Kawamura, A., et al. (2014). Optimized Methods for Targeted Peptide-Based Quantification of Human Uridine 5'-Diphosphate-Glucuronosyltransferases in Biological Specimens Using Liquid Chromatography-Tandem Mass Spectrometry. Drug Metab. Dispos 42, 885–889. doi:10.1124/dmd.113.056291
Schweigmann, H., Sánchez-Guijo, A., Ugele, B., Hartmann, K., Hartmann, M. F., Bergmann, M., et al. (2014). Transport of the Placental Estriol Precursor 16α-Hydroxy-Dehydroepiandrosterone Sulfate (16α-OH-DHEAS) by Stably Transfected OAT4-, SOAT-, and NTCP-HEK293 Cells. J. Steroid Biochem. Mol. Biol. 143, 259–265. doi:10.1016/j.jsbmb.2014.03.013
Scialis, R. J., Aleksunes, L. M., Csanaky, I. L., Klaassen, C. D., and Manautou, J. E. (2019). Identification and Characterization of Efflux Transporters that Modulate the Subtoxic Disposition of Diclofenac and its Metabolites. Drug Metab. Dispos 47, 1080–1092. doi:10.1124/dmd.119.086603
Seitz, S., and Boelsterli, U. A. (1998). Diclofenac Acyl Glucuronide, a Major Biliary Metabolite, Is Directly Involved in Small Intestinal Injury in Rats. Gastroenterology 115, 1476–1482. doi:10.1016/s0016-5085(98)70026-5
Seward, D. J., Koh, A. S., Boyer, J. L., and Ballatori, N. (2003). Functional Complementation between a Novel Mammalian Polygenic Transport Complex and an Evolutionarily Ancient Organic Solute Transporter, OSTalpha-OSTbeta. J. Biol. Chem. 278, 27473–27482. doi:10.1074/jbc.M301106200
Shebley, M., Fu, W., Badri, P., Bow, D., and Fischer, V. (2017). Physiologically Based Pharmacokinetic Modeling Suggests Limited Drug-Drug Interaction between Clopidogrel and Dasabuvir. Clin. Pharmacol. Ther. 102, 679–687. doi:10.1002/cpt.689
Shen, H., Chen, W., Drexler, D. M., Mandlekar, S., Holenarsipur, V. K., Shields, E. E., et al. (2017). Comparative Evaluation of Plasma Bile Acids, Dehydroepiandrosterone Sulfate, Hexadecanedioate, and Tetradecanedioate with Coproporphyrins I and III as Markers of OATP Inhibition in Healthy Subjects. Drug Metab. Dispos 45, 908–919. doi:10.1124/dmd.117.075531
Shen, H., Liu, T., Jiang, H., Titsch, C., Taylor, K., Kandoussi, H., et al. (2016). Cynomolgus Monkey as a Clinically Relevant Model to Study Transport Involving Renal Organic Cation Transporters: In Vitro and In Vivo Evaluation. Drug Metab. Dispos 44, 238–249. doi:10.1124/dmd.115.066852
Shen, H., Liu, T., Morse, B. L., Zhao, Y., Zhang, Y., Qiu, X., et al. (2015). Characterization of Organic Anion Transporter 2 (SLC22A7): A Highly Efficient Transporter for Creatinine and Species-dependent Renal Tubular Expression. Drug Metab. Dispos 43, 984–993. doi:10.1124/dmd.114.062364
Shin, H. J., Anzai, N., Enomoto, A., He, X., Kim, D. K., Endou, H., et al. (2007). Novel Liver-specific Organic Anion Transporter OAT7 that Operates the Exchange of Sulfate Conjugates for Short Chain Fatty Acid Butyrate. Hepatology 45, 1046–1055. doi:10.1002/hep.21596
Shiratani, H., Katoh, M., Nakajima, M., and Yokoi, T. (2008). Species Differences in UDP-Glucuronosyltransferase Activities in Mice and Rats. Drug Metab. Dispos 36, 1745–1752. doi:10.1124/dmd.108.021469
Sirich, T. L., Meyer, T. W., Gondouin, B., Brunet, P., and Niwa, T. (2014). Protein-bound Molecules: a Large Family with a Bad Character. Semin. Nephrol. 34, 106–117. doi:10.1016/j.semnephrol.2014.02.004
Sjöstedt, N., Neuhoff, S., and Brouwer, K. L. R. (2021). Physiologically‐Based Pharmacokinetic Model of Morphine and Morphine‐3‐Glucuronide in Nonalcoholic Steatohepatitis. Clin. Pharmacol. Ther. 109, 676–687. doi:10.1002/cpt.2037
Smith, D. A., Brown, K., and Neale, M. G. (1985). Chromone-2-carboxylic Acids: Roles of Acidity and Lipophilicity in Drug Disposition. Drug Metab. Rev. 16, 365–388. doi:10.3109/03602538508991440
Smith, D. A., and Dalvie, D. (2012). Why Do Metabolites Circulate? Xenobiotica 42, 107–126. doi:10.3109/00498254.2011.630110
Snyder, K. R., Sparano, N., and Malinowski, J. M. (2000). Raloxifene Hydrochloride. Am. J. Health Syst. Pharm. 57, 1669–1678. doi:10.1093/ajhp/57.18.1669
Soars, M. G., Riley, R. J., Findlay, K. A., Coffey, M. J., and Burchell, B. (2001). Evidence for Significant Differences in Microsomal Drug Glucuronidation by Canine and Human Liver and Kidney. Drug Metab. Dispos 29, 121–126.
Sperker, B., Backman, J. T., and Kroemer, H. K. (1997). The Role of Beta-Glucuronidase in Drug Disposition and Drug Targeting in Humans. Clin. Pharmacokinet. 33, 18–31. doi:10.2165/00003088-199733010-00003
Sticova, E., and Jirsa, M. (2013). New Insights in Bilirubin Metabolism and Their Clinical Implications. World J. Gastroenterol. 19, 6398–6407. doi:10.3748/wjg.v19.i38.6398
Stillhart, C., Vučićević, K., Augustijns, P., Basit, A. W., Batchelor, H., Flanagan, T. R., et al. (2020). Impact of Gastrointestinal Physiology on Drug Absorption in Special Populations--An UNGAP Review. Eur. J. Pharm. Sci. 147, 105280. doi:10.1016/j.ejps.2020.105280
Stone, W. J., and Walle, T. (1980). Massive Propranolol Metabolite Retention during Maintenance Hemodialysis. Clin. Pharmacol. Ther. 28, 449–455. doi:10.1038/clpt.1980.187
Sun, D., Jones, N. R., Manni, A., and Lazarus, P. (2013). Characterization of Raloxifene Glucuronidation: Potential Role of UGT1A8 Genotype on Raloxifene Metabolism In Vivo. Cancer Prev. Res. (Phila) 6, 719–730. doi:10.1158/1940-6207.CAPR-12-0448
Sun, H., Wang, X., Zhou, X., Lu, D., Ma, Z., and Wu, B. (2015). Multidrug Resistance-Associated Protein 4 (MRP4/ABCC4) Controls Efflux Transport of Hesperetin Sulfates in Sulfotransferase 1A3-Overexpressing Human Embryonic Kidney 293 Cells. Drug Metab. Dispos 43, 1430–1440. doi:10.1124/dmd.115.065953
Sun, K., and Welty, D. (2021). Elucidation of Metabolic and Disposition Pathways for Maribavir in Nonhuman Primates through Mass Balance and Semi-physiologically Based Modeling Approaches. Drug Metab. Dispos 49, 1025–1037. doi:10.1124/dmd.121.000493
Suzuki, E., Yamamura, N., Ogura, Y., Nakai, D., Kubota, K., Kobayashi, N., et al. (2010). Identification of Valproic Acid Glucuronide Hydrolase as a Key Enzyme for the Interaction of Valproic Acid with Carbapenem Antibiotics. Drug Metab. Dispos 38, 1538–1544. doi:10.1124/dmd.110.032938
Suzuki, M., Suzuki, H., Sugimoto, Y., and Sugiyama, Y. (2003). ABCG2 Transports Sulfated Conjugates of Steroids and Xenobiotics. J. Biol. Chem. 278, 22644–22649. doi:10.1074/jbc.M212399200
Swift, B., Nebot, N., Lee, J. K., Han, T., Proctor, W. R., Thakker, D. R., et al. (2013). Sorafenib Hepatobiliary Disposition: Mechanisms of Hepatic Uptake and Disposition of Generated Metabolites. Drug Metab. Dispos 41, 1179–1186. doi:10.1124/dmd.112.048181
Tague, L. K., Byers, D. E., Hachem, R., Kreisel, D., Krupnick, A. S., Kulkarni, H. S., et al. (2020). Impact of SLCO1B3 Polymorphisms on Clinical Outcomes in Lung Allograft Recipients Receiving Mycophenolic Acid. Pharmacogenomics J. 20, 69–79. doi:10.1038/s41397-019-0086-0
Takada, T., Weiss, H. M., Kretz, O., Gross, G., and Sugiyama, Y. (2004). Hepatic Transport of PKI166, an Epidermal Growth Factor Receptor Kinase Inhibitor of the Pyrrolo-Pyrimidine Class, and its Main Metabolite, ACU154. Drug Metab. Dispos 32, 1272–1278. doi:10.1124/dmd.104.000497
Takada, T., Yamamoto, T., Matsuo, H., Tan, J. K., Ooyama, K., Sakiyama, M., et al. (2018). Identification of ABCG2 as an Exporter of Uremic Toxin Indoxyl Sulfate in Mice and as a Crucial Factor Influencing CKD Progression. Sci. Rep. 8, 11147. doi:10.1038/s41598-018-29208-w
Takehara, I., Terashima, H., Nakayama, T., Yoshikado, T., Yoshida, M., Furihata, K., et al. (2017). Investigation of Glycochenodeoxycholate Sulfate and Chenodeoxycholate Glucuronide as Surrogate Endogenous Probes for Drug Interaction Studies of OATP1B1 and OATP1B3 in Healthy Japanese Volunteers. Pharm. Res. 34, 1601–1614. doi:10.1007/s11095-017-2184-5
Takehara, I., Yoshikado, T., Ishigame, K., Mori, D., Furihata, K. I., Watanabe, N., et al. (2018). Comparative Study of the Dose-Dependence of OATP1B Inhibition by Rifampicin Using Probe Drugs and Endogenous Substrates in Healthy Volunteers. Pharm. Res. 35, 138. doi:10.1007/s11095-018-2416-3
Takekuma, Y., Kakiuchi, H., Yamazaki, K., Miyauchi, S., Kikukawa, T., Kamo, N., et al. (2007). Difference between Pharmacokinetics of Mycophenolic Acid (MPA) in Rats and that in Humans Is Caused by Different Affinities of MRP2 to a Glucuronized Form. J. Pharm. Pharm. Sci. 10, 71–85.
Tamai, I., Nezu, J., Uchino, H., Sai, Y., Oku, A., Shimane, M., et al. (2000). Molecular Identification and Characterization of Novel Members of the Human Organic Anion Transporter (OATP) Family. Biochem. Biophys. Res. Commun. 273, 251–260. doi:10.1006/bbrc.2000.2922
Tamai, I., Nozawa, T., Koshida, M., Nezu, J., Sai, Y., and Tsuji, A. (2001). Functional Characterization of Human Organic Anion Transporting Polypeptide B (OATP-B) in Comparison with Liver-specific OATP-C. Pharm. Res. 18, 1262–1269. doi:10.1023/a:1013077609227
Tamraz, B., Fukushima, H., Wolfe, A. R., Kaspera, R., Totah, R. A., Floyd, J. S., et al. (2013). OATP1B1-related Drug-Drug and Drug-Gene Interactions as Potential Risk Factors for Cerivastatin-Induced Rhabdomyolysis. Pharmacogenet Genomics 23, 355–364. doi:10.1097/FPC.0b013e3283620c3b
Tan, M. L., Yoshida, K., Zhao, P., Zhang, L., Nolin, T. D., Piquette-Miller, M., et al. (2018). Effect of Chronic Kidney Disease on Nonrenal Elimination Pathways: A Systematic Assessment of CYP1A2, CYP2C8, CYP2C9, CYP2C19, and OATP. Clin. Pharmacol. Ther. 103, 854–867. doi:10.1002/cpt.807
Tanihara, Y., Masuda, S., Sato, T., Katsura, T., Ogawa, O., and Inui, K. (2007). Substrate Specificity of MATE1 and MATE2-K, Human Multidrug and Toxin extrusions/H(+)-organic Cation Antiporters. Biochem. Pharmacol. 74, 359–371. doi:10.1016/j.bcp.2007.04.010
Thakkar, N., Slizgi, J. R., and Brouwer, K. L. R. (2017). Effect of Liver Disease on Hepatic Transporter Expression and Function. J. Pharm. Sci. 106, 2282–2294. doi:10.1016/j.xphs.2017.04.053
Thompson, R. A., Isin, E. M., Li, Y., Weidolf, L., Page, K., Wilson, I., et al. (2012). In Vitro approach to Assess the Potential for Risk of Idiosyncratic Adverse Reactions Caused by Candidate Drugs. Chem. Res. Toxicol. 25, 1616–1632. doi:10.1021/tx300091x
Tornio, A., Filppula, A. M., Kailari, O., Neuvonen, M., Nyrönen, T. H., Tapaninen, T., et al. (2014). Glucuronidation Converts Clopidogrel to a strong Time-dependent Inhibitor of CYP2C8: a Phase II Metabolite as a Perpetrator of Drug-Drug Interactions. Clin. Pharmacol. Ther. 96, 498–507. doi:10.1038/clpt.2014.141
Trdan Lušin, T., Mrhar, A., Stieger, B., Kullak-Ublick, G. A., Marc, J., Ostanek, B., et al. (2012a). Influence of Hepatic and Intestinal Efflux Transporters and Their Genetic Variants on the Pharmacokinetics and Pharmacodynamics of Raloxifene in Osteoporosis Treatment. Transl Res. 160, 298–308. doi:10.1016/j.trsl.2012.03.002
Trdan Lušin, T., Stieger, B., Marc, J., Mrhar, A., Trontelj, J., Zavratnik, A., et al. (2012b). Organic Anion Transporting Polypeptides OATP1B1 and OATP1B3 and Their Genetic Variants Influence the Pharmacokinetics and Pharmacodynamics of Raloxifene. J. Transl Med. 10, 76. doi:10.1186/1479-5876-10-76
Troberg, J., Järvinen, E., Ge, G. B., Yang, L., and Finel, M. (2017). UGT1A10 Is a High Activity and Important Extrahepatic Enzyme: Why Has its Role in Intestinal Glucuronidation Been Frequently Underestimated? Mol. Pharm. 14, 2875–2883. doi:10.1021/acs.molpharmaceut.6b00852
Tsuruya, Y., Kato, K., Sano, Y., Imamura, Y., Maeda, K., Kumagai, Y., et al. (2016). Investigation of Endogenous Compounds Applicable to Drug-Drug Interaction Studies Involving the Renal Organic Anion Transporters, OAT1 and OAT3, in Humans. Drug Metab. Dispos 44, 1925–1933. doi:10.1124/dmd.116.071472
Ueo, H., Motohashi, H., Katsura, T., and Inui, K. (2005). Human Organic Anion Transporter hOAT3 Is a Potent Transporter of Cephalosporin Antibiotics, in Comparison with hOAT1. Biochem. Pharmacol. 70, 1104–1113. doi:10.1016/j.bcp.2005.06.024
Ugele, B., Bahn, A., and Rex-Haffner, M. (2008). Functional Differences in Steroid Sulfate Uptake of Organic Anion Transporter 4 (OAT4) and Organic Anion Transporting Polypeptide 2B1 (OATP2B1) in Human Placenta. J. Steroid Biochem. Mol. Biol. 111, 1–6. doi:10.1016/j.jsbmb.2008.04.001
Uwai, Y., Motohashi, H., Tsuji, Y., Ueo, H., Katsura, T., and Inui, K. (2007). Interaction and Transport Characteristics of Mycophenolic Acid and its Glucuronide via Human Organic Anion Transporters hOAT1 and hOAT3. Biochem. Pharmacol. 74, 161–168. doi:10.1016/j.bcp.2007.03.024
van de Steeg, E., Stránecký, V., Hartmannová, H., Nosková, L., Hřebíček, M., Wagenaar, E., et al. (2012). Complete OATP1B1 and OATP1B3 Deficiency Causes Human Rotor Syndrome by Interrupting Conjugated Bilirubin Reuptake into the Liver. J. Clin Invest 122, 519–28. doi:10.1172/JCI59526
van de Steeg, E., Wagenaar, E., van der Kruijssen, C. M., Burggraaff, J. E., de Waart, D. R., Elferink, R. P., et al. (2010). Organic anion transporting polypeptide 1a/1b-knockout mice provide insights into hepatic handling of bilirubin, bile acids, and drugs. J Clin Invest 120, 2942–52. doi:10.1172/JCI42168
van de Wetering, K., Zelcer, N., Kuil, A., Feddema, W., Hillebrand, M., Vlaming, M. L., et al. (2007). Multidrug resistance proteins 2 and 3 provide alternative routes for hepatic excretion of morphine-glucuronides. Mol Pharmacol 72, 387–94. doi:10.1124/mol.107.035592
van der Deure, W. M., Friesema, E. C., de Jong, F. J., de Rijke, Y. B., de Jong, F. H., Uitterlinden, A. G., et al. (2008a). Organic anion transporter 1B1: an important factor in hepatic thyroid hormone and estrogen transport and metabolism. Endocrinology 149, 4695–701. doi:10.1210/en.2008-0169
van der Deure, W. M., Hansen, P. S., Peeters, R. P., Kyvik, K. O., Friesema, E. C., Hegedüs, L., et al. (2008b). Thyroid hormone transport and metabolism by organic anion transporter 1C1 and consequences of genetic variation. Endocrinology 149, 5307–14. doi:10.1210/en.2008-0430
Vasilyeva, A., Durmus, S., Li, L., Wagenaar, E., Hu, S., Gibson, A. A., et al. (2015). Hepatocellular Shuttling and Recirculation of Sorafenib-Glucuronide Is Dependent on Abcc2, Abcc3, and Oatp1a/1b. Cancer Res 75, 2729–36. doi:10.1158/0008-5472.CAN-15-0280
Venkatasubramanian, R., Fukuda, T., Niu, J., Mizuno, T., Chidambaran, V., Vinks, A. A., et al. (2014). ABCC3 and OCT1 genotypes influence pharmacokinetics of morphine in children. Pharmacogenomics 15, 1297–309. doi:10.2217/pgs.14.99
Verbeeck, R. K. (1982). Glucuronidation and disposition of drug glucuronides in patients with renal failure. A review. Drug Metab Dispos 10, 87–9.
Vildhede, A., Kimoto, E., Pelis, R. M., Rodrigues, A. D., and Varma, M. V. S. (2020). Quantitative Proteomics and Mechanistic Modeling of Transporter-Mediated Disposition in Nonalcoholic Fatty Liver Disease. Clin Pharmacol Ther 107, 1128–1137. doi:10.1002/cpt.1699
Visser, W. E., Wong, W. S., van Mullem, A. A., Friesema, E. C., Geyer, J., and Visser, T. J. (2010). Study of the transport of thyroid hormone by transporters of the SLC10 family. Mol Cell Endocrinol 315, 138–45. doi:10.1016/j.mce.2009.08.003
Wang, L., Prasad, B., Salphati, L., Chu, X., Gupta, A., Hop, C. E., et al. (2015). Interspecies Variability in Expression of Hepatobiliary Transporters across Human, Dog, Monkey, and Rat as Determined by Quantitative Proteomics. Drug Metab Dispos 43, 367–74. doi:10.1124/dmd.114.061580
Wang, L., Raghavan, N., He, K., Luettgen, J. M., Humphreys, W. G., Knabb, R. M., et al. (2009). Sulfation of O-Demethyl Apixaban: Enzyme Identification and Species Comparison. Drug Metab Dispos 37, 802–8. doi:10.1124/dmd.108.025593
Wang, M., Qi, H., Li, J., Xu, Y., and Zhang, H. (2015). Transmembrane transport of steviol glucuronide and its potential interaction with selected drugs and natural compounds. Food Chem Toxicol 86, 217–24. doi:10.1016/j.fct.2015.10.011
Wang, Q., Shi, R., Dai, Y., Li, Y., Wang, T., Ma, Y., et al. (2018). Mechanism in the existent difference in form of wogonin/wogonoside between plasma and intestine/liver in rats. RSC Adv. 8, 3364–3373. doi:10.1039/C7RA08270C
Watanabe, H., Sakaguchi, Y., Sugimoto, R., Kaneko, K., Iwata, H., Kotani, S., et al. (2014). Human organic anion transporters function as a high-capacity transporter for p-cresyl sulfate, a uremic toxin. Clin Exp Nephrol 18, 814–20. doi:10.1007/s10157-013-0902-9
Watanabe, T., Fujiwara, Y., and Chan, F. K. L. (2020). Current knowledge on non-steroidal anti-inflammatory drug-induced small-bowel damage: a comprehensive review. J Gastroenterol 55, 481–495. doi:10.1007/s00535-019-01657-8
Weigand, K. M., Schirris, T. J. J., Houweling, M., van den Heuvel, J. J. M. W., Koenderink, J. B., Dankers, A. C. A., et al. (2019). Uremic solutes modulate hepatic bile acid handling and induce mitochondrial toxicity. Toxicol In Vitro 56, 52–61. doi:10.1016/j.tiv.2019.01.003
Wen, F., Shi, M., Bian, J., Zhang, H., and Gui, C. (2016). Identification of natural products as modulators of OATP2B1 using LC-MS/MS to quantify OATP-mediated uptake. Pharm Biol 54, 293–302. doi:10.3109/13880209.2015.1034326
Wikoff, W. R., Nagle, M. A., Kouznetsova, V. L., Tsigelny, I. F., and Nigam, S. K. (2011). Untargeted metabolomics identifies enterobiome metabolites and putative uremic toxins as substrates of organic anion transporter 1 (Oat1). J Proteome Res 10, 2842–51. doi:10.1021/pr200093w
Wolenski, F. S., Zhu, A. Z. X., Johnson, M., Yu, S., Moriya, Y., Ebihara, T., et al. (2017). Fasiglifam (TAK-875) Alters Bile Acid Homeostasis in Rats and Dogs: A Potential Cause of Drug Induced Liver Injury. Toxicol Sci 157, 50–61. doi:10.1093/toxsci/kfx018
Wolff, N. A., Burckhardt, B. C., Burckhardt, G., Oellerich, M., and Armstrong, V. W. (2007). Mycophenolic acid (MPA) and its glucuronide metabolites interact with transport systems responsible for excretion of organic anions in the basolateral membrane of the human kidney. Nephrol Dial Transplant 22, 2497–503. doi:10.1093/ndt/gfm219
Wong, C. C., Akiyama, Y., Abe, T., Lippiat, J. D., Orfila, C., and Williamson, G. (2012). Carrier-mediated transport of quercetin conjugates: involvement of organic anion transporters and organic anion transporting polypeptides. Biochem Pharmacol 84, 564–70. doi:10.1016/j.bcp.2012.05.011
Wong, C. C., Barron, D., Orfila, C., Dionisi, F., Krajcsi, P., and Williamson, G. (2011a). Interaction of hydroxycinnamic acids and their conjugates with organic anion transporters and ATP-binding cassette transporters. Mol Nutr Food Res 55, 979–88. doi:10.1002/mnfr.201000652
Wong, C. C., Botting, N. P., Orfila, C., Al-Maharik, N., and Williamson, G. (2011b). Flavonoid conjugates interact with organic anion transporters (OATs) and attenuate cytotoxicity of adefovir mediated by organic anion transporter 1 (OAT1/SLC22A6). Biochem Pharmacol 81, 942–9. doi:10.1016/j.bcp.2011.01.004
Xu, F., Li, Z., Zheng, J., Gee Cheung, F. S., Chan, T., Zhu, L., et al. (2013). The inhibitory effects of the bioactive components isolated from Scutellaria baicalensis on the cellular uptake mediated by the essential solute carrier transporters. J Pharm Sci 102, 4205–11. doi:10.1002/jps.23727
Yang, K., Pfeifer, N. D., Köck, K., and Brouwer, K. L. (2015). Species differences in hepatobiliary disposition of taurocholic acid in human and rat sandwich-cultured hepatocytes: implications for drug-induced liver injury. J Pharmacol Exp Ther 353, 415–23. doi:10.1124/jpet.114.221564
Yee, S. W., Stecula, A., Chien, H. C., Zou, L., Feofanova, E. V., van Borselen, M., et al. (2019). Unraveling the functional role of the orphan solute carrier, SLC22A24 in the transport of steroid conjugates through metabolomic and genome-wide association studies. PLoS Genet 15, e1008208. doi:10.1371/journal.pgen.1008208
Zamek-Gliszczynski, M. J., Chu, X., Polli, J. W., Paine, M. F., and Galetin, A. (2014). Understanding the transport properties of metabolites: case studies and considerations for drug development. Drug Metab Dispos 42, 650–64. doi:10.1124/dmd.113.055558
Zamek-Gliszczynski, M. J., Hoffmaster, K. A., Nezasa, K., Tallman, M. N., and Brouwer, K. L. (2006). Integration of hepatic drug transporters and phase II metabolizing enzymes: mechanisms of hepatic excretion of sulfate, glucuronide, and glutathione metabolites. Eur J Pharm Sci 27, 447–86. doi:10.1016/j.ejps.2005.12.007
Zelcer, N., Saeki, T., Bot, I., Kuil, A., and Borst, P. (2003). Transport of bile acids in multidrug-resistance-protein 3-overexpressing cells co-transfected with the ileal Na+-dependent bile-acid transporter. Biochem J 369, 23–30. doi:10.1042/BJ20021081
Zelcer, N., van de Wetering, K., Hillebrand, M., Sarton, E., Kuil, A., Wielinga, P. R., et al. (2005). Mice lacking multidrug resistance protein 3 show altered morphine pharmacokinetics and morphine-6-glucuronide antinociception. Proc Natl Acad Sci U S A 102, 7274–9. doi:10.1073/pnas.0502530102
Zhang, H., Basit, A., Busch, D., Yabut, K., Bhatt, D. K., Drozdzik, M., et al. (2018). Quantitative characterization of UDP-glucuronosyltransferase 2B17 in human liver and intestine and its role in testosterone first-pass metabolism. Biochem Pharmacol 156, 32–42. doi:10.1016/j.bcp.2018.08.003
Zhang, H., Wolford, C., Basit, A., Li, A. P., Fan, P. W., Murray, B. P., et al. (2020). Regional Proteomic Quantification of Clinically Relevant Non-Cytochrome P450 Enzymes along the Human Small Intestine. Drug Metab Dispos 48, 528–536. doi:10.1124/dmd.120.090738
Zhang, Q., Zhang, Y., Boer, J., Shi, J. G., Hu, P., Diamond, S., et al. (2017). In Vitro Interactions of Epacadostat and its Major Metabolites with Human Efflux and Uptake Transporters: Implications for Pharmacokinetics and Drug Interactions. Drug Metab. Dispos 45, 612–623. doi:10.1124/dmd.116.074609
Zhang, Y., Han, Y. H., Putluru, S. P., Matta, M. K., Kole, P., Mandlekar, S., et al. (2016). Diclofenac and its Acyl Glucuronide: Determination of In Vivo Exposure in Human Subjects and Characterization as Human Drug Transporter Substrates In Vitro. Drug Metab. Dispos 44, 320–328. doi:10.1124/dmd.115.066944
Zhong, K., Li, X., Xie, C., Zhang, Y., Zhong, D., and Chen, X. (2014). Effects of Renal Impairment on the Pharmacokinetics of Morinidazole: Uptake Transporter-Mediated Renal Clearance of the Conjugated Metabolites. Antimicrob. Agents Chemother. 58, 4153–4161. doi:10.1128/AAC.02414-14
Zhou, D., Kong, L., Jiang, Y., Wang, C., Ni, Y., Wang, Y., et al. (2019). UGT-dependent Regioselective Glucuronidation of Ursodeoxycholic Acid and Obeticholic Acid and Selective Transport of the Consequent Acyl Glucuronides by OATP1B1 and 1B3. Chem. Biol. Interact 310, 108745. doi:10.1016/j.cbi.2019.108745
Zhou, X., Wang, S., Sun, H., and Wu, B. (2015). Sulfonation of Raloxifene in HEK293 Cells Overexpressing SULT1A3: Involvement of Breast Cancer Resistance Protein (BCRP/ABCG2) and Multidrug Resistance-Associated Protein 4 (MRP4/ABCC4) in Excretion of Sulfate Metabolites. Drug Metab. Pharmacokinet. 30, 425–433. doi:10.1016/j.dmpk.2015.09.001
Zhou, X., Zhang, F., Chen, C., Guo, Z., Liu, J., Yu, J., et al. (2017). Impact of Curcumin on the Pharmacokinetics of Rosuvastatin in Rats and Dogs Based on the Conjugated Metabolites. Xenobiotica 47, 267–275. doi:10.1080/00498254.2016.1183060
Zimmerman, E. I., Hu, S., Roberts, J. L., Gibson, A. A., Orwick, S. J., Li, L., et al. (2013). Contribution of OATP1B1 and OATP1B3 to the Disposition of Sorafenib and Sorafenib-Glucuronide. Clin. Cancer Res. 19, 1458–1466. doi:10.1158/1078-0432.CCR-12-3306
Keywords: ABC transporter, acyl glucuronide, drug-drug interaction (DDI), enterohepatic recycling, solute carrier, sulfotransferase (SULT), transporter inhibition, UDP-glucuronosyltransferase (UGT)
Citation: Järvinen E, Deng F, Kiander W, Sinokki A, Kidron H and Sjöstedt N (2022) The Role of Uptake and Efflux Transporters in the Disposition of Glucuronide and Sulfate Conjugates. Front. Pharmacol. 12:802539. doi: 10.3389/fphar.2021.802539
Received: 26 October 2021; Accepted: 06 December 2021;
Published: 13 January 2022.
Edited by:
Michael Zimmermann, European Molecular Biology Laboratory (EMBL) Heidelberg, GermanyReviewed by:
Csilla Özvegy-Laczka, Hungarian Academy of Sciences (MTA), HungaryWalter Jäger, University of Vienna, Austria
Copyright © 2022 Järvinen, Deng, Kiander, Sinokki, Kidron and Sjöstedt. This is an open-access article distributed under the terms of the Creative Commons Attribution License (CC BY). The use, distribution or reproduction in other forums is permitted, provided the original author(s) and the copyright owner(s) are credited and that the original publication in this journal is cited, in accordance with accepted academic practice. No use, distribution or reproduction is permitted which does not comply with these terms.
*Correspondence: Noora Sjöstedt, bm9vcmEuc2pvc3RlZHRAaGVsc2lua2kuZmk=
†ORCID: Erkka Järvinen, orcid.org/0000-0001-8970-5194; Feng Deng, orcid.org/0000-0002-9173-6923; Wilma Kiander, orcid.org/0000-0001-9523-7139; Alli Sinokki, orcid.org/0000-0002-9712-6552; Heidi Kidron, orcid.org/0000-0001-6427-8042; Noora Sjöstedt, orcid.org/0000-0001-6960-7757
‡These authors have contributed equally to this work