- 1Department of Pharmaceutical Sciences, Faculty of Pharmacy, Universitas Airlangga, Surabaya, Indonesia
- 2Stem Cell Research and Development Center, Universitas Airlangga, Surabaya, Indonesia
- 3Study Program of Pharmacy, Faculty of Pharmacy, Universitas Airlangga, Surabaya, Indonesia
Ursolic acid is a natural pentacyclic triterpenoid that exerts a potent anticancer effect. Furthermore, it is classified as a BCS class IV compound possessing low permeability and water solubility, consequently demonstrating limited bioavailability in addition to low therapeutic effectiveness. Nanoparticles are developed to modify the physical characteristics of drug and can often be produced in the range of 30–200 nm, providing highly effective cancer therapy due to the Enhanced Permeation and Retention (EPR) Effect. This study aims to provide a review of the efficacy and safety of various types of Ursolic Acid-loading nanoparticles within the setting of preclinical and clinical anticancer studies. This literature study used scoping review method, where the extracted data must comply with the journal inclusion criteria of within years of 2010–2020. The identification stage produced 237 suitable articles. Duplicate screening was then conducted followed by the initial selection of 18 articles that had been reviewed and extracted for data analysis. Based on this review, the use of nanoparticles can be seen to increase the anticancer efficacy of Ursolic Acid in terms of several parameters including pharmacokinetic data, survival rates and inhibition rates, as well as the absence of serious toxicity in preclinical and clinical trials in terms of several parameters including body weight, blood clinical chemistry, and organ histipathology. Based on this review, the use of nanoparticles has been able to increase the anticancer efficacy of Ursolic Acid, as well as show the absence of serious toxicity in preclinical and clinical trials. Evenmore, the liposome carrier provides development data that has reached the clinical trial phase I. The use of nanoparticle provides high potential for Ursolic Acid delivery in cancer therapy.
Introduction
Cancer is a disease that can occur in almost any organ or tissue of the body when abnormal cells grow uncontrollably beyond their usual limits to invade adjacent parts of the body and/or spread to other organs (Kamińska et al., 2015). Data from the Global Burden of Cancer released by the World Health Organization (WHO) reported that the number of cases and deaths from cancer in 2018 totaled 18.1 million and 9.6 million respectively. Cancer-related deaths are predicted to increase to more than 13.1 million by 2030 (Internatioanl Agency for Research on Cancer, 2018). The most common types affecting men include lung, prostate, colorectal, and liver cancer, while in women, they comprise breast, colorectal, lung, cervical and thyroid cancers. In 2018, the incidence rate of liver cancer in Indonesia ranked eighth highest in Southeast Asia, while in Asia as a whole it occupied 23rd position with 348,809 cases (Globocan. Indonesia - Global Cancer Observatory WHO, 2018).
The first-line options for cancer treatment include surgery, radiotherapy and the administering of biological and chemical drugs (chemotherapy, hormones, and biological therapy). However, such forms of treatment fail to control metastatic tumors that have spread to other organs (Kumari et al., 2016). Chemotherapeutic agents are predominantly toxic compounds that primarily inhibit the rapid proliferation of cancer cells, while also potentially restricting the growth of hair follicle, bone marrow and gastrointestinal cells culminating in severe undesired side effects (Pérez-Herrero and Fernández-Medarde, 2015). Consequently, the effect of certain natural compounds have been widely explored and it has been scientifically proven that Ursolic Acid (UA), an active agent, inhibits the proliferation of cancer cells (Kashyap et al., 2016).
UA is a natural pentacyclic triterpene of the cyclosqualenoid family present in many plants which can modulate cellular transcription factors, growth factor receptors, inflammatory cytokines, and numerous other molecular targets that regulate cell proliferation, metastasis, apoptosis, angiogenesis, and autophagy. The anticancer effects of UA have been reported for many types of cancer, one of which is liver cancer (Khwaza et al., 2020). The mechanisms of UA which produce such effects include nuclear-kappa B (NF-kB) factors and apoptosis signaling in cancer cells (Seo et al., 2018). The protease activity involving urokinase and cathepsin B, both of which are known to be associated with tumor invasion and metastasis, is also significantly inhibited by UA, interleukin-1 β (IL-1β), Tumor necrotic factor-α (TNF-α), and the expression of MMP-2 and MMP-9 (Mitochondria-Mediated Pathway) (Kashyap et al., 2016; Ali et al., 2019). Prolonged administration of excessive doses of UA, with an LD50 value of 9.26 g/kg in acute toxicity tests on mice (Sun et al., 2020), has the potential to cause liver cytotoxicity which is not classified as genetic toxicity. Within the Biopharmaceutical Classification System (BCS), UA is categorized as a BCS class IV compound demonstrating low permeability and solubility (Sun et al., 2020) which, consequently, requires a nanotechnology-based drug delivery system to reach the desired target (Biopharmaceutical, 2011). In particular, the development of drug delivery systems entails the use of nanoparticles targeted at cancer cells which significantly improve therapeutic and diagnostic efficacy as well as reducing unwanted side effects.
Nanotechnology represents a new therapeutic platform that employs nanoparticles (NPs) in the diagnosis and treatment of cancer. NPs are used in cancer therapy due to their relatively small size, 1–1,000 nm, the fact that they frequently fall within the range of 10–200 nm, and the presence of the EPR effect (Rajesh, 2000; Reddy, 2005; Fang et al., 2011). Nano-drug delivery systems have been lauded for their excellent biocompatibility properties, low toxicity, increased solubility in water, in addition to their ability to deliver targeted drugs to tissues which limits their accumulation in the kidneys, liver, spleen, and other non-targeted organs, while improving therapeutic efficacy (Kumari et al., 2016; Li et al., 2017). The delivery of nanoparticles to tumor tissues through systemic circulation can be executed through two targeting strategies, including passive targeting, when nanoparticles entering circulation will accumulate at the tumor site due to enhanced permeation and retention (EPR). In contrast, active targeting, generally employs ligand molecules such as antibodies and peptides to recognize specific antigens expressed in tumor cells or the microenvironment (Kumari et al., 2016).
Many types of nanoparticles exist, including polymeric therapy (polymer-protein and polymer-drug conjugates) in which drugs are covalently bound or conjugated to polymer structures and nanoparticulate drugs. The drugs are physically trapped in assembled particles composed of different materials such as polymers (polymer micelles, dendrimers and polymer nanoparticles), lipids (liposomes), or organometallic compounds (carbon nanotubes). The first generation of anticancer nanoparticles approved by the FDA include liposomal drugs and polymer conjugates (Barenholz, 2012; Pérez-Herrero and Fernández-Medarde, 2015). However, certain products can be subjected to in vivo and clinical trials, while others remain limited to in vitro studies. Therefore, the effectiveness and safety of the nanoparticle drug constitute important assessed parameters.
As for the development of drugs with nanoparticle carriers, one example is Doxil®, the first nano-drug using liposomes approved by the FDA, which demonstrates the clinical performance advantages of doxorubicin in a variety of neoplastic conditions due to pharmacokinetics and the unique EPR-related biodistribution of liposomal doxorubicin (Barenholz, 2012; Wei et al., 2016). Doxil® can reduce side effects, especially that of cardiac toxicity, and improve patients’ adherence and quality of life (Barenholz, 2012). Cisplatin is an anticancer drug prepared with a polymeric micelle through the formation of a metal-polymer complex between cisplatin and poly-(ethylene glycol)-poly(glutamic acid) block copolymers (Nishiyama et al., 2003; Uchino et al., 2005). These micelles are 28 nm in size with a very narrow distribution, demonstrate extremely extended blood circulation, and accumulate effectively in solid tumor models of Lewis lung carcinoma cells. However, because they undergo chemical synthesis, toxicity and scale-up production become major issues (Mizumura et al., 2001; Plummer et al., 2011; Mitchell et al., 2021). In addition, the development of Abraxane®, a paclitaxel-albumin-bound nanoparticle ∼130 nm in size, was approved by the FDA in 2005 for the treatment of metastatic breast cancer (Miele et al., 2009; Ma and Mumper, 2013). This formulation has been shown to have several advantages in terms of toxicity reduction compared to Taxol®. Moreover, the total dose can be given within 30 min without pre-treatment. However, the manner in which Abraxane® can improve survival rates and overcome P-GP-mediated drug resistance remains unclear (Ma and Mumper, 2013).
Certain nanoparticles have been used in the delivery of UA as a cancer therapy including liposomes, polymeric nanoparticles, and polymeric micelles (Zaimy et al., 2017). However, at the time of writing, in contrast to other chemotherapy drugs such as Doxorubicin (Doxil®), Cisplatin, Paclitaxel (Taxol®), or Amphotericin B (Ambisome®), no review of the effectiveness and safety of several types of nanoparticles for the delivery of UA for cancer therapy has been conducted (Zaimy et al., 2017). This study aims to provide a literature review related to the anticancer effectiveness and safety of UA delivered with various types of nanoparticles based on pre-clinical and clinical trial results from existing research published between 2011 and 2021.
Methods
Article Selection Criteria
This study uses the scoping review method involving literature accessible through the PubMed, Sciencedirect, Scopus, and Google Scholar databases consisting of online research publications dating from 2011 to 2021. Clinical trial articles were sourced using the keywords"Clinical trial”, “Ursolic Acid”, “Cancer”, and “OR Nanoparticle Liposome”. As for the search for articles relating to in vivo studies, this employed the keywords “Pre-Clinical OR in vivo OR Animal”, Ursolic Acid”, “Cancer”, “Nanoparticle”. Within this study, several inclusion and exclusion criteria were applied to select and screen articles for review as shown in Table 1.
Evaluation of Physical Characteristics of UA Nanoparticles
Data analysis involved comparing the physical characteristics of different types of nanoparticles identified in the selected articles.
Analysis of Particle Size
Particle size and particle size distribution produce significant impacts on drug loading variation, drug release, bioavailability, and efficacy (Kharia et al., 2012). In addition, particle sizes of 150 nm to 4.5 μm will be easily recognized by macrophages and dendritic cells during phagositosis (Koppolu and Zaharoff, 2013). Instruments used in nanoparticle size and morphology determination include Dynamic Light Scattering (DLS), Scanning Electron Microscopy (SEM), Transmission Electron Microscopy (TEM), and Atomic Force Microscopy (AFM).
Analysis of ζ-Potential
Zeta potential is used to predict the stability of colloidal dispersion systems during storage. Generally, ζ-potential values above ± 30 mV resulted in more stable particles because the repulsing force between particles can prevent aggregation. The ζ-potential is affected by surfactants, polymers, the surface active agent component of nanoparticles, the presence of absorbing compounds, dispersed phase media, ionic strength, and pH (Kharia et al., 2012). The ζ-potential can be analyzed using the Electrophoretic Light Scattering (ELS) method (Miatmoko et al., 2017; Wang et al., 2017).
Analysis of Encapsulation Efficiency
Entrapment Efficiency (EE%), or encapsulation efficiency, is defined as the portion of drugs encapsulated in nanoparticles. Free drugs that are not encapsulated in the drug delivery carriers or nanoparticles can be separated by means of centrifugation, dialysis, or gel chromatography. The concentration of entrapped and un-entrapped drugs can be determined through the use of instruments such as spectrophotometers or high-performance liquid chromatography (HPLC). The encapsulation efficiency percentage is calculated using the following equation:
where,
Pharmacokinetic Evaluation of UA Nanoparticles
Plasma concentration versus time data was analyzed using non-compartmental methods. Peak plasma concentration
Evaluation of the Effectiveness of Ursolic Acid Nanoparticles
The analysis was conducted by comparing the results of efficacy studies including survival rate, tumor growth inhibition, tumor weight, and tumor volume, as well as tumor tissue histopathology extracted from reviewed articles.
Cancerous Tissue Histopathology
The histological section of the liver was stained with haematoxylin and eosin (H&E) staining and subsequently compared to the histopathological appearance of each organ in the control and treatment groups (Melo et al., 2020). The microstructure and morphology of tissues were observed using a light microscope (Wang et al., 2017). Hematoxylin is a base dye that has an affinity for the acidic components of cells, primarily the nucleic acids contained in the nucleus, while eosin is an acidic dye that binds to the cell cytoplasm. As a result, H&E stained the core blue and cytoplasm orange-red (Slaoui and Fiette, 2011).
Analysis of Relative Tumor Volume
In the articles, the size of the tumor is determined by means of a calliper, while its volume is calculated using the following equation:
where x is the longest and y the shortest diameter (Hattori et al., 2014; Yang et al., 2014; Wang et al., 2016; Miatmoko et al., 2017; Liu et al., 2021). In this study, the relative tumor volume is calculated using the following formulas:
or
where
Analysis of Relative Tumor Weight and Growth Inhibition Rate
The antitumor activity of nanoparticles is assessed through the tumor growth inhibition rate (IR) or tumor growth inhibition (TGI) at the experimental endpoint, calculated using the following equations:
where
where
where
Analysis of Relative Survival Rate
Survival rates can be used as a standard assessment of effective therapy. The survival period is usually calculated from the date of diagnosis or commencement of the treatment period. The survival curve of each group was estimated using the Kaplan-Meier method with the average survival time difference being assessed by means of a log-rank test (Yang et al., 2014). This method involves non-parametric estimation of the survival function commonly used to describe the survival of a single population or compare the survival of two populations. The Kaplan-Meier estimate is one of the most effective statistical methods of measuring the probability of a patient’s survival observed during a post-treatment period (Etikan, 2017). In this study, the relative survival rate was calculated using the following formula:
where
Evaluation of Toxicity of UA Nanoparticles in Pre-Clinical Trials
Weight Analysis
Weight loss represents a significant parameter of biological safety analysis or drug safety. The weight of the mice subjects is measured on the day of tumor inoculation and continues daily, or at least several times per week, during treatment. Each treatment group mouse is quantitatively weighed with the result being compared to that of a normal mouse in order to identify any significant difference between the two groups (Valcourt et al., 2020). In this study, the weight of the mice was calculated using the following formulas:
Where
Other Toxicity Analysis
Other toxicity data on the in vivo studies was derived by data recapitulation that included: tissue histopathology, increased levels of ALT and AST, and the amount of WBC as an indicator of hematological toxicity (Zhao et al., 2018; Li et al., 2019; Liu et al., 2021).
Evaluation of AU-NP Toxicity in Clinical Trials
Analysis of Clinical Chemistry Data
Toxicity was evaluated in all subjects treated with at least one cycle of UA Liposome therapy. Hematological parameters (red blood cells, WBC, hemoglobin, ANC, and platelets), urinary routines (urine protein, glucose, erythrocytes, leukocytes, and urine bilirubin), and stool routines (stool erythrocytes and stool leukocytes) were evaluated. Blood biochemistry including ALT, AST, ALP, gamma-glutamyl transpeptidase (GGT) were further analyzed (Qian et al., 2015). In this study, the analysis was conducted by comparing clinical laboratory data (ALT, AST, GGT, DBIL, and TBIL) extracted from reviewed articles.
Analysis of Adverse Events
Adverse events are used to assess unintended events (AE) in healthy adult volunteers and patients with advanced solid tumor disease. In addition, the toxicity can be seen from the value of the maximum tolerated dose (MTD) used to determine the highest dose of the drug that can be administered without adverse events. The adverse events documented during the study were classified as mild, moderate, or severe based on the dosage (Valcourt et al., 2020). In this study, the analysis was conducted by comparing adverse events or side effects occurring in subjects who had received the treatment mentioned in reviewed articles.
Results and Discussion
This study provides a literature review focusing on the anticancer effectiveness and safety of UA delivered with various types of nanoparticles to increase its anticancer effects as confirmed by both pre-clinical and clinical trials. Literature searches of all four databases using pre-determined keywords identified 237 articles in the prescreening stage as can be seen in Figure 1. Of the total literature reviewed, duplication screening was conducted using the Mendeley application to produce a final body of 226 articles. Application of exclusion criteria resulting in a body of 196 selected articles which were subsequently subjected to inclusion criteria to produce a final total of 30. The initial selection process identified 24 articles which were subsequently reviewed, culminating in 18 which satisfied the inclusion criteria. The summary of reviewed articles is presented in Table 2.
The data extraction of the literature used can be seen in Table 2.
Nanoparticle Characterization Results
From the review of the 18 articles, it was clear that three types of drug represent the most frequent delivery carriers of UA as an anticancer agent, namely; Liposome (50%), Nanosphere (39%) and Polymeric Misel (11%), (see Figure 2A).
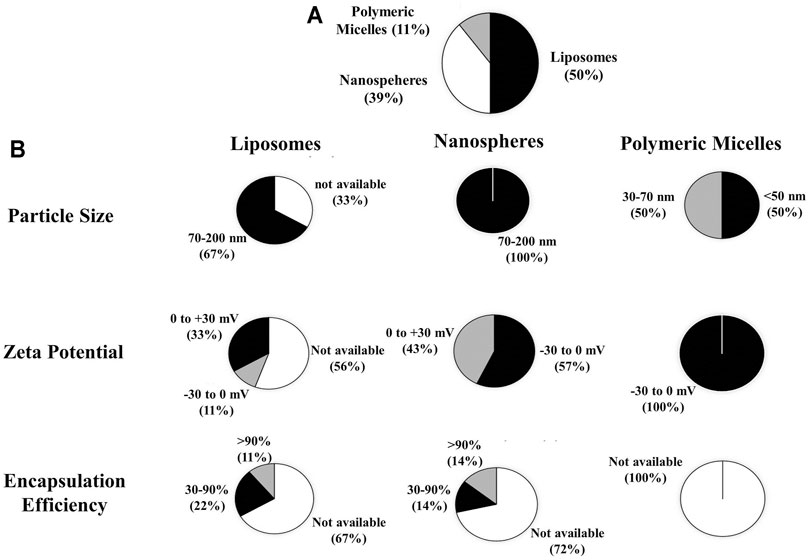
FIGURE 2. (A) Types of drug carrier extracted from the article review regarding the preclinical and clinical studies of nanoparticle use for UA delivery within cancer therapy, (B) the physical characteristics of UA-loaded nanoparticles including particle size, zeta potential, and efficiency of encapsulation.
According to the review results, several characterization parameters of liposomes, nanospheres, and polymeric micelles exist, including particle size, ζ-potential, and encapsulation efficiency (EE). From the data analysis of the 18 articles, the size of liposome particles was found to range from 70 to 200 nm (67%); nanosphere particle size to be between 70 and 200 nm (100%); and micelle polymeric particle size to be between 30 and 70 nm (50%). The ζ-potential of liposomes ranged from (−)30 to 0 mV (11%) and 0 to (+)30 mV (33%), while the nanosphere ζ-potentials were between (-)30 to 0 mV (57%), 0 to (+)30 mV (43%); and ζ liposomes of (−)30 to 0 mV (100%). For the EE of liposomes ≥90% (11%) and 30–90% (22%); EE nanospheres ≥90% (14%) and 30–90% (14%); as for EE polymeric micelles, these are not mentioned in the article, as presented in Figure 2B.
Characterization of liposome particle size is important because it affects the interaction of liposomes with target cells as well as the elimination, penetration and retention of drugs in the target sites (Monteiro et al., 2014). Phospholipids represent the main constituents of liposomal membranes and the use of lipid types and ratios within different preparation methods can affect the size of liposomes (Monteiro et al., 2014; Shaker et al., 2017). From Figure 2A it can be seen that liposomes prepared with ethanol injection and thin-film hydration methods have particle sizes ranging from 70 to 200 nm. This finding is in accordance with that of previous research arguing that, with the ethanol injection method, liposome could be generated as SUVs with diameters of 30–110 nm (Akbarzadeh et al., 2013; Monteiro et al., 2014), while with the adoption of thin film hydration methods, continued use of sonication or extrusion processes can produce liposomes as 25 nm to 1 μm-sized ULVs (Monteiro et al., 2014). Liposome size depends on that of the phospholipid molecule assembly whose average dimensions depend on their lipid composition, while it is supposed that the size of liposome particles increases slightly with a reduction in the molar ratio of HSPC/SPC in the range of 119–143 nm (Chen et al., 2006). On the other hand, liposomes made from DMPC, DSPC, and HSPC (at a weight ratio of 2:1 to cholesterol) experienced different increases in particle size, e.g., DMPC:Chol liposomes increased in size from 149 to 190 nm, DSPC:Chol expanded from 83 to 104 nm, and HSPC:Chol liposomes from 88 to 122 nm (Webb et al., 2019).
For nanospheres, particle size, which is greatly affected by lipid type, ranges from 70 to 200 nm. This is in accordance with a previous report stating that nanospheres have a diameter of 10–200 nm (Baldim et al., 2020). With regard to polymeric micelles, studies show that particle sizes ranging from 30–70 nm are affected by polymer types based on the characteristics of hydrophilic and hydrophobic block copolymers. This finding is in keeping with that of earlier research which reported that the size of polymeric micelle particles is determined by the ratio of hydrophobic and hydrophilic block chains and can produce particle sizes of ≤50 nm (Cabral et al., 2011). Increased targeting of drugs to cancer cells within the tumor tissues with the use of long-circulating polymeric micelles depends on the size of the micelle and the vascular permeability of the tumor tissues. In hypervascular tumors with highly permeable vascular structures, sub-100 nm polymeric micelles show no limits for drug extravasation and tumor penetration. In contrast, only micelles smaller than 50 nm can penetrate hipovascular tumors whose vascular permeability is poor (Cabral et al., 2011).
The zeta potentials which reflect the liposome surface charges were evaluated (Alves et al., 2017). Figure 2B shows that liposomes and nanospheres had zeta potentials ranging from (−30) to 0 mV and 0 to (+)30 mV, while those of polymeric micelles varied from (−30) to 0 mV. If the system has a strong negative or positive zeta potential the particles will tend to repulse each other and no aggregation occurs. Therefore, if the system has zeta potential >+30 mV or <−30 mV, then it can be considered stable (Laouini et al., 2012; Lunardi et al., 2021). The positive or negative charges measured in nanoparticles are highly dependent on lipid components. Analysis of the composition and intracellular delivery mechanisms confirmed that conventional liposomes had a relative neutral charge due to their neutral phospholipid composition such as HSPC and became negatively charged when added to cholesterol. pH sensitive liposomes contained a DOPE-like phospholipid component with CHEMS causing their negative charges; cationic liposomes had a cationic lipid composition such as DDAB, DOGS, DOTAP, DOTMA, DMRIE, DORIE with DOPE; Long-circulating liposomes (LCL) had a high TC neutral lipid composition, cholesterol, added to approximately 5–10 mol % of PEG-DSPE rendering these liposomes stable when under protein opsonization (Sharma and Sharma, 1997; Gabizon et al., 2012).
The tendency of a drug to interact with polar or non-polar bonds and/or electrostatic interactions with lipid bilayer will determine whether it will be encapsulated into inner aqueous compartments or the lipid bilayer membrane, or whether it will be closely related to the polar head group of the bilayer membrane through electrostatic interactions. It will correlate to encapsulation efficiency (EE) or loading capacity, which is usually defined as a fraction of the percentage of the total encapsulated drug, in the bilayer membrane or aqueous intravesicular compartments or the matrix of nanoparticles (Kulkarni et al., 1995). UA has poor permeability and low water (Sun et al., 2020), thus causing possibly encapsulated within membrane bilayer of lipid vesicles. As can be seen in Figure 2B, the EE of liposomes and nanospheres ranges from 30% to ≥90%, while the EE of polymeric micelles is not mentioned. This suggests that drugs are successfully encapsulated in nanoparticles in order to increase the amount of drugs delivered to the target sites.
Pharmacokinetic Data in Clinical Trials
From the review of pharmacokinetic data relating to clinical trials, it was found that in Lipo A the average
Pharmacokinetic Data Review on In Vivo Studies
The pharmacokinetic data on in vivo studies of Lipo E shows that the highest plasma UA concentration in the PEGylated liposome treatment group was 19.87 ug/mL, which exceeded that of both the Ursolic Acid Liposomes (UAL) and Ursolic Acid (UA) solution groups. In addition, as seen from Table 4, PEG-modified liposomes have the longest
In Nano A, after administration of a hydrophobic drug, i.e., hydroxycamptothecin (HCPT), conjugated to folic acid-pectin-eight-arm PEG-UA (F-Pt-PU) the concentration of UA and HCPT in plasma decreases slowly, resulting in the longer circulation period of native UA, which may be due to the breaking of ester bonds between 8 arm-PEG and UA. The concentration of NP HCPT@F-Pt-PU in the bloodstream, still detectable at 80 h, is higher than that of native UA (7 h) and HCPT (8 h). The concentration of NP HCPT @F-Pt-PU in plasma is higher than that of np F-Pt-PU, possibly because the conjugation of HCPT into polymers increases the strength of the hydrophobic bonds in the particle cores, thereby reducing the hydrolysis rate of nanoparticles (Liu et al., 2021).
In Nano G, UA blood circulation in pectin-eight-arm polyethylene glycol-ursolic acid/hydrooxycampothecin nanoparticles (NP Pec-8PUH) at 80 h can be maintained at a higher concentration in plasma, while native UA and HCPT rapidly disappear from plasma. The half-life of UA blood circulation in NP Pec-8PUH is 8.7 h which is 7.3 times longer than in native UA (Liu et al., 2018).
In Poly B, polymeric drug conjugates are synthesized by conjugating UA into polyethylene glycol using disulfide bonds (U-SS-M), while UA is eliminated relatively slowly and maintained at high concentrations in plasma for up to 48 h after administration. U-SS-M exhibits a similar pattern of biodistribution and accumulates mainly in the liver and kidneys before being subsequently eliminated by these organs. In tumor tissue, the concentration of UA decreases over time, although the amount delivered by the polymer-drug conjugate gradually increases. The concentration of U-SS-M in tumor tissue is significantly higher than that of native UA at both 6 and 12 h after administration (Fu et al., 2021).
Recapitulation of Pre-Clinical and Clinical Research Relating to UA Nanoparticles
The results indicate that the available articles which discuss pre-clinical/in vivo trials amounted of 83%, including the use of nanoparticle carrier types of nanospheres (47%), liposomes (40%), and polymeric micelles (13%). As for those that discussed clinical trials (17%), as seen in Figure 3A–C, these featured only the use of liposomes (100%). Clinical trials are still being conducted in phase 1, indicating that they remain at the stage of evaluating dose levels, acute toxicity, and drug distribution in humans (Valcourt et al., 2020).
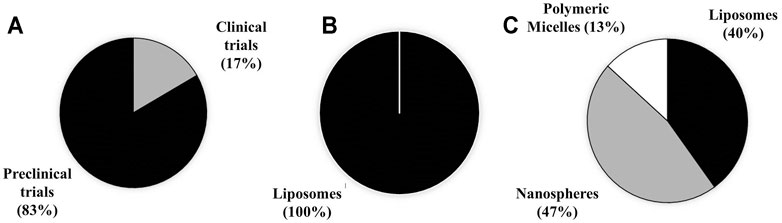
FIGURE 3. Research recapitulation of (A) clincial and preclinical studies, (B) types of nanoparticle use in clinical trials, (C) and pre-clinical trials.
In vivo Anti-Cancer Efficacy of Nanoparticles Containing UA
Analysis of Tumor Tissue Histopathology
The anticancer effectiveness of nanoparticles containing UA compared to negative control and free UA are shown to have a significant effect on tumor growth inhibition as shown in Table 5.
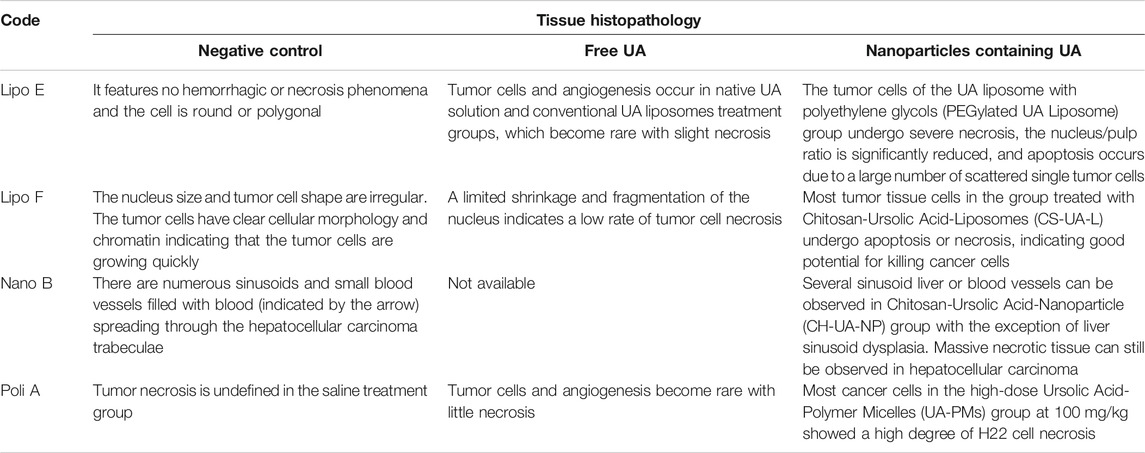
TABLE 5. Tissue histopathology of liver cancer after administration of negative control, native UA and nanoparticles containing UA.
Comparative Analysis of Tumor Growth Inhibition
The results indicate that the normal tumor volume when compared to administration of UA-loaded liposomes (Lipo D, E, F, G, H, I), nanospheres (Nano A, B, D, E, F, G) and polymeric micelles (Poly A) decreased in relative tumor volume by approximately 2.0–21.2 times. The tumor volume of native UA compared to the administration of UA liposomes (Lipo D, E, F, G, H, I), nanospheres (Nano A, D, E, F, G) and polymeric micelle (Poly A) showed a relative reduction in tumor volume of about 1.6–15.9 times lower than that of the native UA group, as presented in Figure 5A. This suggests that nanoparticles can improve UA effectiveness in inhibiting expansions in tumor volume.
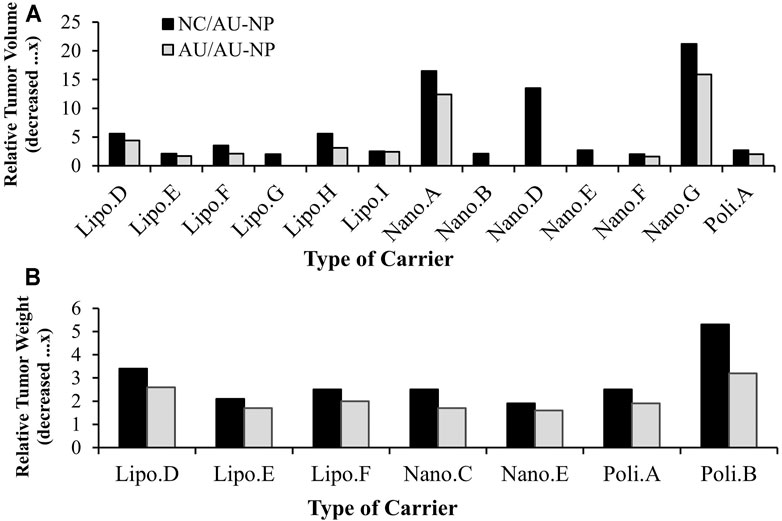
FIGURE 5. (A) Relative tumor volume in animal models treated with UA-loaded nanoparticles compared to negative control (black bars) and UA-free treatment groups (grey bars), (B) relative tumor tissue weight of animal models treated with UA-loaded nanoparticles compared to negative control (black bars) and native UA-treatment groups (grey bars).
The relative tumor weight analysis results relating to groups treated with UA liposomes (Lipo D, E, F), nanospheres (Nano C, E) and polymeric micelles (Poly A,B) indicated a relative reduction in tumor weight approximately 1.9–5.3 times that of the negative control group. Tumor weight in the native UA group compared to that of groups administered with UA liposomes (Lipo D, E, F), nanospheres (Nano C,E) and polymeric micelles (Poly A,B) showed a relative reduction of about 1.6-3.2x, as shown in Figure 5B. This suggests that nanoparticles may increase the effectiveness of UA in inhibiting tumor growth resulted in reduction of tumor weight.
The relative inhibition rate analysis results indicate that the administration of UA liposomes (Lipo E, F), nanospheres (Nano A) and polymeric micelles (Poly B) produces an increase in the relative tumor inhibition rate of approximately 1.9-3.4x compared to the native UA group. Of the three types of drug carriers, liposomes (Lipo F) experienced the highest relative inhibition rate increase of 3.4x the native UA group, as seen in Figure 4A. This suggests that nanoparticles may increase the effectiveness of UA in inhibiting tumor growth.
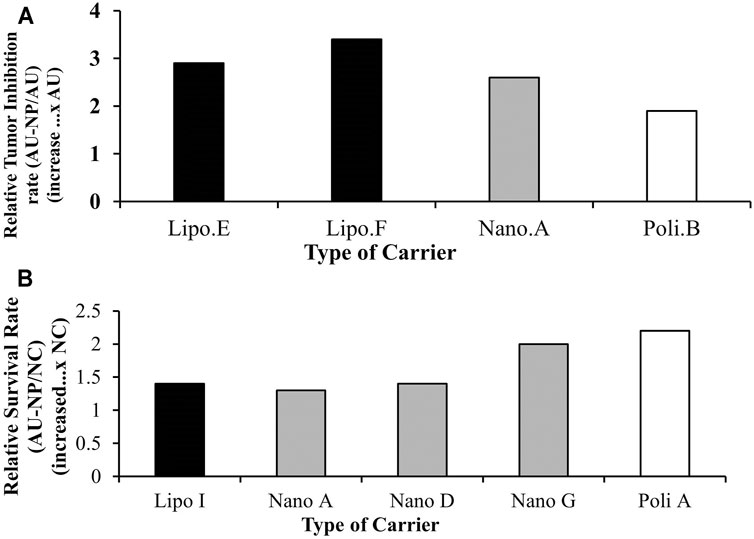
FIGURE 4. (A) Relative tumor growth inhibition rate of animal models treated with nanoparticles loading UA compared to native UA treatment groups, (B) Relative survival rate of animal models treated with UA-loaded nanoparticles compared to the negative control.
Analysis of Survival Rate
Based on the results, the administration of liposomes (Lipo E, F), nanospheres (Nano A) and polymeric micelles (Poly B) produced an increase in the relative survival rate about 1.3-2.2x higher when compared to that of the negative control group, as seen in Figure 4B. This suggests that nanoparticles may increase the effectiveness of UA associated with improved survival rate.
The increased anti-tumor activity observed from the volume and weight of the tumor was associated with necrosis in the tumor tissues caused by large dose exposures of UA reaching cancer cells due to the increased permeability of small nanoparticles with high drug loading due to the EPR effect. Furthermore, the drug will be released into the extracellular and/or intracellular matrix. In the extracellular fluids, the drug will leak from nanoparticles and subsequently diffuse into cancer cells, while in intracellular fluids nanoparticles will experience endocytosis and the matrix will be destroyed in the endosome and release free drugs which then diffuse into the cytoplasm and nucleus subsequently causing cell necrosis. These results show that the use of nanoparticles as carriers within anticancer drug delivery can increase the in vivo survival rate.
Other studies have suggested that when nanoparticles such as liposomes interact with cells, drug delivery and diffusion into target cells can occur in several ways. Liposomes can penetrate the tumor tissue matrix resulting in degradation of carrier lipids by enzymes, such as lipase, or by mechanical strain inducing release of active substances into the extracellular fluid. This process induces drug diffusion into cell membranes culminating in cytoplasm and nucleus delivery. However, the latter process cannot easily be achieved by the use of hydrophilic drugs. Secondly, liposome membranes will fuse with those of the target cell leading to the release of liposomes directly into the cytoplasm. The third and most frequent method is that of receptor-mediated endocytosis. This process involves only vesicles with a maximum diameter of 150 nm and active substances demonstrating significant stability in such an acidic lysosome environment where liposomes are metabolized enzymatically. Phagocytosis may also ensue but involving large size nanoparticles affected by specialized immune system cells, such as macrophages, monocytes, and Kupffer cells. This process may eliminate the nanoparticles from the circulatory system (Bozzuto and Molinari, 2015).
The survival rate of liposomes is higher than that of other nanoparticles indicating the stability of the system in the blood circulation which ensures that the trapped drug is carried by the nanoparticles for further release into the cancer cells. In addition, because of the biomimetic property of liposome components that resemble phospholipid cell membranes it is easier for them to be absorbed by the cell.
In vivo Toxicity Studies of Nanoparticles Containing UA
Pre-Clinical Toxicity Based on the Analysis of Relative Body Weight
From the results of relative body weight calculations contained in Table 6, no significant differences existed in the weight of the mice, proving that nanoparticle administration neither caused side effects nor produced symptoms of toxicity (Poudel et al., 2020; Liu et al., 2021; Fu et al., 2021). This result is also supported by other toxicity data presented in Table 7, which shows that there was no clear cell damage and no morphological changes in the major organs i.e. heart, liver, spleen, lungs, and kidneys. However, ALT, AST and WBC levels all decreased after administration of UA nanoparticles when compared to native UA (Zhang et al., 2015; Zhao et al., 2018). This suggested that UA nanoparticles do not cause serious toxicity, indeed, do not even produce toxicity. Rather, the effectt is mild and of short duration (Li et al., 2019).
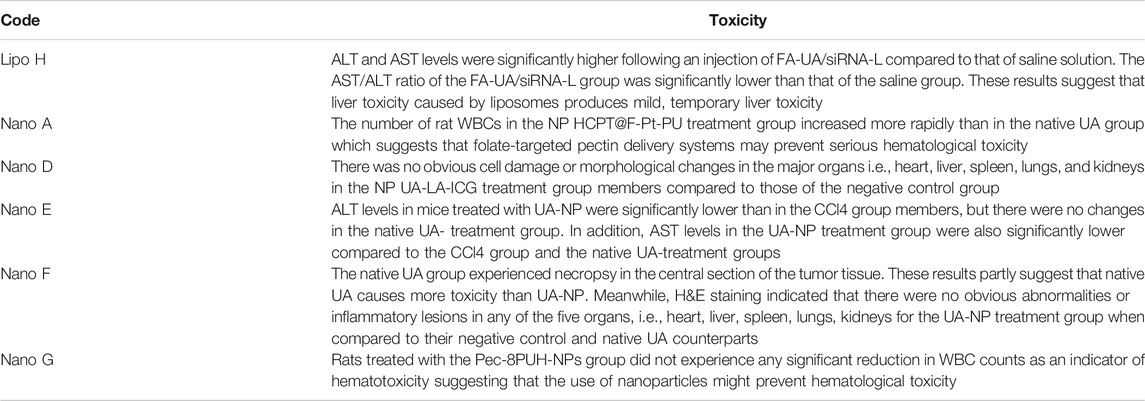
TABLE 6. The relative body weight of animal models treated with UA-loaded nanoparticles compared to negative control and native UA-treatment groups.
Safety Aspects of the Use of Nanoparticles Containing UA Based on the Clinical Trials
Toxicity Based on Clinical Laboratory Parameters
The content of the graphs contained in Figure 6A, confirm an increase in levels of AST, ALT, GGT, TG, DBIL, and TBIL after UA liposome (Lipo A, B, C) administration occurred. It can also be seen that Dose Limiting Toxicity (DLT) related to hepatotoxicity, which was monitored for substantial side effect parameters, was at a moderate level (Valcourt et al., 2020).
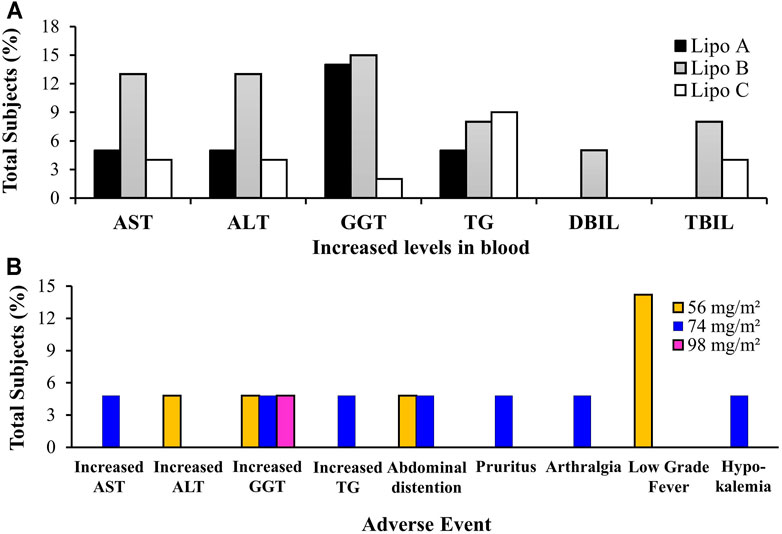
FIGURE 6. (A) Total subjects with increasing blood marker levels on clinical trials of Lipo A, Lipo B, and Lipo C, (B) Adverse events of Lipo A during phase I clinical trials. Notes: AST, Alanine Aminotransferase/SGPT (serum glutamic pyruvic transaminase); ALT, Aspartate Aminotransferase/SGOT (serum glutamic oxaloacetic transaminase); GGT, Gamma Glutamyl Transpeptidase; TG, Triglycerides; DBIL, Direct Bilirubin; TBIL, Total Bilirubin.
In Lipo A, an increase in levels of AST (5%), ALT (5%), GGT (14%), TG (5%) was observed in 21 subjects who received doses of 56, 74, and 98 mg/m2. In Lipo B, elevated levels of AST (13%), ALT (13%), GGT (15%), TG (8%), DBIL (5%) and TBIL (8%) were recorded in 39 subjects receiving doses of 11, 22, 37, 56, 74, 98, and 130 mg/m2. In Lipo C, there was an increase in AST (4%), ALT (4%), GGT (2%), TG (9%), and TBIL (4%) levels observed in 24 subjects who received doses of 74 mg/m2 as a single dose and 98 and 74 mg/m2 as multiple doses.
Clinical Toxicity Based on the Occurences of Adverse Events
According to the data contained in Figure 6B, three subjects (14%) treated with a dose of 56 mg/m2 of Lipo A experienced a mild fever but recovered after 2 hours without receiving treatment. Moreover, three subjects (14%) treated with sequential doses of 56, 74, and 98 mg/m2 of Lipo A experienced an increase in GGT, two subjects (10%) administered with doses of 56 and 74 mg/m2 of Lipo A experienced abdominal distension, and one patient (5%) experienced a rise in ALT levels. Other mild symptoms included increased AST and TG, pruritus, arthralgia, and hypokalemia. The most common adverse conditions included fever, increased GGT, and flatulence. These results indicated that a 4-h intravenous administration of Lipo A was tolerable and safe if a timetable of three doses per day for 14 consecutive days followed by a break lasting 7 days within each 21-days cycle was adhered to. Therefore, a 98 mg/m2 dose of Lipo A is the recommended dose for phase II trials (Qian et al., 2015).
In addition, from the contents of Figure 7A, it can be seen that one patient treated with a 11 mg/m2 dose experienced a first degree skin rash which healed untreated after 3 days. In addition, two patients who had been administered with a 98 mg/m2 dose experienced vascular stimulation. First degree microscopic hematuria was observed in three subjects (7.7%) suffering from hepatoma malignancy who had received 11 doses of 11, 74, and 130 mg/m2, respectively. However, these side effects disappeared after 3 days without any treatment being administered. Elevated levels of AST, ALT, GGT, DBIL, and TBIL were observed in several subjects receiving doses of 74, 98, and 130 mg/m2. Dose Limiting Toxicity (DLT) resulted in hepatotoxicity: two subjects (5.1%) experienced an increase in AST, four subjects (10.3%) an increase in ALT, one subject (2.6%) an increase in GGT, and one subject (2.6%) an increase in DBIL. Diarrhea (2.6%) constitutes another DLT. Other drug-related side effects included nausea reported by one subject (2.6%), abdominal distension observed in another (2.6%), vascular stimulation occurred in two subjects (5.1%), while elevated TG was reported in three subjects (7.7%). Other reported adverse events included one subject (2.6%) suffering a skin rash and another (2.6%) experiencing higher serum sodium levels. At a dose of 74 mg/m2, one of six subjects experienced DLT, which is a form of non-hematological toxicity, including increased AST/ALT and diarrhea. At a dose of 98 mg/m2, one of the eleven subjects experienced DLT, i.e., non-hematological toxicity including increased ALT/GGT). At a dose of 130 mg/m2, two thirds of the subjects experienced DLT (increased ALT, AST, and DBIL). Therefore, the increased dosage was suspended and MTD was confirmed to be 98 mg/m2. Double administration of trial doses of UAL at recommended levels of 56, 74, and 98 mg/m2 was completed (Wang et al., 2013).
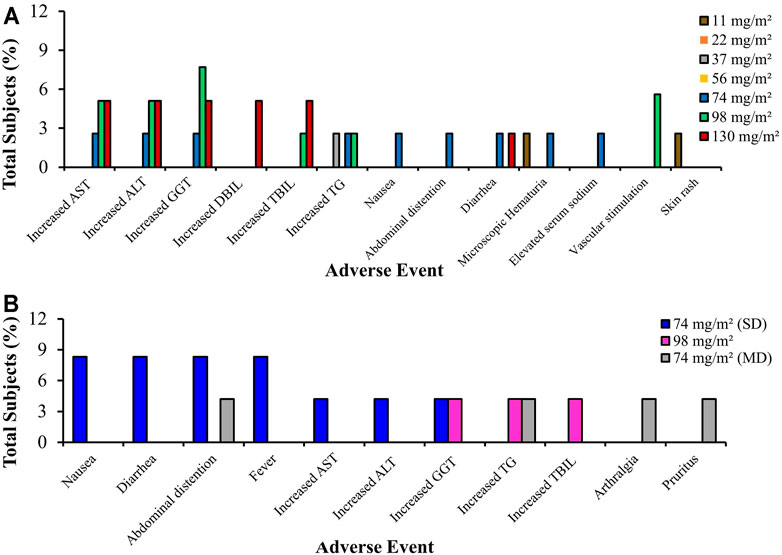
FIGURE 7. Total subjects with adverse events of (A) Lipo B, and (B) Lipo C in phase I clinical trials. AST, Alanine Aminotransferase/SGPT (serum glutamic pyruvic transaminase); ALT, Aspartate Aminotransferase/SGOT (serum glutamic oxaloacetic transaminase); GGT, Gamma Glutamyl Transpeptidase; DBIL, Direct Bilirubin; TBIL, Total Bilirubin; TG, Triglycerides
From the graph in Figure 7B, it is clear that all subjects in the study tolerated the Lipo C treatment. Most adverse events varying from mild to moderate related to Lipo C, which is Ursolic Acid Nanoliposome (UANL), were non-dose dependent. The most commonly observed adverse events included abdominal distension, nausea, and diarrhea. The adverse events after a 14-days continuous infusion of UANL comprised skin pruritus, arthrisgia, and increased triglycerides levels. UANL has minimal toxic effects. The limiting toxicity of UANL dose is hepatotoxicity. In this study, intravenous UANL infusions were well tolerated both by healthy volunteers and patients with advanced tumors (Zhu et al., 2013).
Based on the review analysis, only three articles which focused on liposomes as the drug carrier discussed clinical trials of UA. Although UA is classified as a BCS class IV drug, its permeability and solubility can be enhanced with the use of liposomes. It is related to the natural phase properties of the liposomal membrane that significantly affect permeability, aggregation, protein binding and liposome fusion. Membrane permeability largely depends on lipid components. Lipids that contain saturated chains or do not have carbon double bonds are more stable because they demonstrate greater resistance to oxidation. Lipid bilayer and liposome membranes possess a good lipid-packing order or gel phase below the lipid phase transition temperature (Tc), where the temperature is in a balanced proportion in the two phases. The fluidity of the lipid bilayer can be controlled by the selection and combined use of lipids, as the various Tcs depend on the length and origin sources (saturated or unsaturated) of fatty acid chains. For example, the incorporation of cholesterol at low concentrations into the lipid bilayer leads to increased trans-membrane permeability, where the incorporation of large amounts (>30 mol%) of cholesterol can reduce the transition phase and decrease membrane permeability at higher Tc temperatures (Corvera et al., 1992). Liposome permeability is related to the rate of solute diffusion through the lipid bilayer. The liposome membrane will achieve the highest permeability in the transition temperature phase, while its permeability is lower in gel form than in the fluid phase. The temperature of the bilayer phase transition is determined by the composition of the liposome. In the transition temperature phase, the permeability of liposomes to molecules such as protons and water increases (Koda et al., 2008; Yoshimoto et al., 2013; Hansen et al., 2015). In addition, the in vivo biodistribution and disposition of liposomes varies depending on the composition of the lipids, particle size, potential charge and degree of steric surface/hydration. In addition, the administration route may affect the in vivo disposition of liposomes. During intravenous administration, liposomes usually interact with serum proteins and are absorbed by RES cells, thus accumulating in the liver or spleen (Sercombe et al., 2015).
The development of nanoparticles for drug delivery, one of which is Doxil® (Doxorubicin HCl liposome injection), the first nanoliposomal drug approved by FDA in 1995, was based on three principles: 1) prolonging drug circulation time and RES avoidance due to the PEGylation of nanoliposomes; 2) higher stable loading of doxorubicin driven by the transmembrane ammonium sulfate gradient which also allows the re-release of the drug in tumors; and 3) having lipid bilayer liposomes in a “liquid ordered” phase consisting of phosphatidylcholine with a high melting temperature (
As for the development of cisplatin therapy, which incorporates the use of an anticancer drug, this involves a polymeric micelle delivery system. Polymeric micelles were prepared through the formation of a metal-polymer complex between cisplatin (CDDP) and poly-(ethylene glycol)-poly(glutamic acid) block copolymers. Cisplatin polymeric micelles (CDDP/m) are 28 nm in size with the ability to distribute themselves through narrow spaces such as blood vessels in pancreatic tissue. These micelles undergo lengthy blood circulation and accumulate effectively in solid tumors of Lewis lung carcinoma cells. However, because they are produced synthetically, the toxicity and safety aspects as well as manufacturing production scale constitute extremely important issues (Plummer et al., 2011).
Abraxane®, a paclitaxel albumin-bound nanoparticle with a particle size of ∼130 nm which received FDA approval in 2005 for the treatment of metastatic breast cancer succesfully reduces toxicities in comparison to Taxol®. Moreover, it enables a complete dose to be administered within 30 min without the need for any pre-treatment. Nevertheless, the mechanism of Abraxane® in improving survival rate and overcoming P-GP-mediated drug resistance remains unclear (Ma and Mumper, 2013).
The findings of this scoping review suggest that liposomes provide more comprehensive data than other forms of nanoparticles. This is demonstrated by the existence of in vivo studies of anticancer effectiveness assessed using several parameters such as increasing relative survival rate; more robust tumor growth inhibition (increasing relative inhibition rate, decreasing relative tumor weight, and reducing tumor volume); and improvements in tumor tissue histopathology. In addition, in vivo studies related to safety were also evaluated employing several parameters, i.e., weight loss, and other toxicity (lowering AST, ALT, and WBC), and well-tolerated toxicity by healthy volunteers and patients with advanced tumors.
There needs multi-faceted views of the use of nanoparticles for reviewing drug delivery. The components of the nanoparticle formulation would greatly affect the characteristics of the nanoparticles including particle size, potential charges, stealth and biomimetic properties, and others, which are closely related to drug delivery to cancer tissue, due to the Enhanced Permeation and Retention (EPR) effects. In addition, in vivo analysis of different types of cancer, where each type of cancer cell has different biological properties, also requires an in-depth study to provide data on supporting the effectiveness of drug delivery to target cancerous tissues. Moreover, the route of administration, dose, and frequency of drug administration related to the physicochemical properties and pharmacokinetic profile of the drug also greatly affect the systemic bioavailability and effective drug amount capable of reaching cancer tissue as the target of drug delivery. All these aspects provide important views for comprehensive study of the drug delivery system in cancer therapy.
Conclusion
Based on the scoping review of the relevant literature, it can be concluded that UA loaded into nanoparticles is effective as a form of anticancer therapy. Pre-clinical trials confirm that it increases the relative survival rate; tumor resistance (increasing the relative inhibition rate, lowering the relative tumor weight, and decreasing tumor volume); and improves tumor tissue histopathology. In addition, UA-loaded nanoparticles have been proven safe for anticancer therapy based on the evaluation of weight loss and other toxicity (decreased AST/ALT). The results from the last 10-years analysis have indicated that, compared to nanospheres and polymeric micelles, liposomes have been assessed as more effective and safer during more comprehensive pre-clinical and clinical trials. This finding highlights the potential for liposomes to be further developed as a means of delivering UA as an anticancer therapy.
Author Contributions
AM: 1) conception and design of the work, data acquisition, data analysis and interpretation; 2) critically revising the article for important intellectual content; 3) Final approval of the version to be published; 4) Agreement to be accountable for all aspects of the work in ensuring that questions related to the accuracy or integrity of the work are appropriately investigated and resolved. EM: 1) data acquisition; 2) Drafting the article; 3) Final approval of the version to be published; 4) Agreement to be accountable for all aspects of the work in ensuring that questions related to the accuracy or integrity of the work are appropriately investigated and resolved. RS: 1) data analysis and interpretation; 2) Final approval of the version to be published; 3) Agreement to be accountable for all aspects of the work in ensuring that questions related to the accuracy or integrity of the work are appropriately investigated and resolved. EH: 1) data analysis and interpretation; 2) Final approval of the version to be published; 3) Agreement to be accountable for all aspects of the work in ensuring that questions related to the accuracy or integrity of the work are appropriately investigated.
Funding
This study was supported by a Preliminary Research on Excellence in Higher Education Institutions (Penelitian Dasar Unggulan Perguruan Tinggi, PDUPT) Grant No. 8/E1/KPT/2021, No. 4/E1/KP.PTNBH/2021, and No. 385/UN3.15PT/2021 provided by the Ministry of Research and Technology-National Research and Innovation Agency of Republic of Indonesia.
Conflict of Interest
The authors declare that the research was conducted in the absence of any commercial or financial relationships that could be construed as a potential conflict of interest.
Publisher’s Note
All claims expressed in this article are solely those of the authors and do not necessarily represent those of their affiliated organizations, or those of the publisher, the editors and the reviewers. Any product that may be evaluated in this article, or claim that may be made by its manufacturer, is not guaranteed or endorsed by the publisher.
References
Akbarzadeh, A., Rezaei-Sadabady, R., Davaran, S., Joo, S. W., Zarghami, N., Hanifehpour, Y., et al. (2013). Liposome: Classification, Preparation, and Applications. Nanoscale Res. Lett. 8 (1), 102. doi:10.1186/1556-276X-8-102
Ali, S. A., Ibrahim, N. A., Mohammed, M. M. D., El-Hawary, S., and Refaat, E. A. (2019). The Potential Chemo Preventive Effect of Ursolic Acid Isolated from Paulownia Tomentosa, against N-Diethylnitrosamine: Initiated and Promoted Hepatocarcinogenesis. Heliyon 5 (5), e01769. doi:10.1016/j.heliyon.2019.e01769
Alves, R. F., Favaro, M. T. P., Balbino, T. A., de Toledo, M. A. S., de la Torre, L. G., and Azzoni, A. R. (2017). Recombinant Protein-Based Nanocarriers and Their Association with Cationic Liposomes: Characterization and In Vitro Evaluation. Colloids Surf. A: Physicochemical Eng. Aspects 513, 1–10. doi:10.1016/j.colsurfa.2016.11.019
Anselmo, A. C., and Mitragotri, S. (2019). Nanoparticles in the Clinic: An Update. Bioeng. Transl Med. 4 (3), e10143–16. doi:10.1002/btm2.10143
Baldim, I., Oliveira, W. P., Kadian, V., Rao, R., Yadav, N., Mahant, S., et al. (2020). Natural Ergot Alkaloids in Ocular Pharmacotherapy: Known Molecules for Novel Nanoparticle-Based Delivery Systems. Biomolecules 10 (7), 1–21. doi:10.3390/biom10070980
Barenholz, Y. (2012). Doxil®--the First FDA-Approved Nano-Drug: Lessons Learned. J. Control. Release 160 (2), 117–134. doi:10.1016/j.jconrel.2012.03.020
Bozzuto, G., and Molinari, A. (2015). Liposomes as Nanomedical Devices. Int. J. Nanomedicine 10, 975–999. doi:10.2147/IJN.S68861
Cabral, H., Matsumoto, Y., Mizuno, K., Chen, Q., Murakami, M., Kimura, M., et al. (2011). Accumulation of Sub-100 Nm Polymeric Micelles in Poorly Permeable Tumours Depends on Size. Nat. Nanotechnol 6 (12), 815–823. doi:10.1038/nnano.2011.166
Chastagner, P., Devictor, B., Geoerger, B., Aerts, I., Leblond, P., Frappaz, D., et al. (2015). Phase I Study of Non-pegylated Liposomal Doxorubicin in Children with Recurrent/refractory High-Grade Glioma. Cancer Chemother. Pharmacol. 76 (2), 425–432. doi:10.1007/s00280-015-2781-0
Chen, J., Ping, Q. N., Guo, J. X., Chu, X. Z., and Song, M. M. (2006). Effect of Phospholipid Composition on Characterization of Liposomes Containing 9-nitrocamptothecin. Drug Dev. Ind. Pharm. 32 (6), 719–726. Jul. doi:10.1080/03639040500529077
Corvera, E., Mouritsen, O. G., Singer, M. A., and Zuckermann, M. J. (1992). The Permeability and the Effect of Acyl-Chain Length for Phospholipid Bilayers Containing Cholesterol: Theory and experiment. Biochim. Biophys. Acta 1107 (2), 261–270. doi:10.1016/0005-2736(92)90413-g
Dawidczyk, C. M., Kim, C., Park, J. H., Russell, L. M., Lee, K. H., Pomper, M. G., et al. (2014). State-of-the-art in Design Rules for Drug Delivery Platforms: Lessons Learned from FDA-Approved Nanomedicines. J. Control. Release 187, 133–144. doi:10.1016/j.jconrel.2014.05.036
Etikan, I. (2017). The Kaplan Meier Estimate in Survival Analysis. Biometrics Biostat Int. J. 5 (2), 55–59. doi:10.15406/bbij.2017.05.00128
Fan, L., Zhang, B., Xu, A., Shen, Z., Guo, Y., Zhao, R., et al. (2018). Carrier-Free, Pure Nanodrug Formed by the Self-Assembly of an Anticancer Drug for Cancer Immune Therapy. Mol. Pharm. 15 (6), 2466–2478. Jun. doi:10.1021/acs.molpharmaceut.8b00444
Fang, J., Nakamura, H., and Maeda, H. (2011). The EPR Effect: Unique Features of Tumor Blood Vessels for Drug Delivery, Factors Involved, and Limitations and Augmentation of the Effect. Adv. Drug Deliv. Rev. 63 (3), 136–151. Available from:. doi:10.1016/j.addr.2010.04.009
Forssen, E. A. (1997). The Design and Development of DaunoXome® for Solid Tumor Targeting In Vivo. Adv. Drug Deliv. Rev. 24 (2), 133–150. doi:10.1016/s0169-409x(96)00453-x
Fu, D., Ni, Z., Wu, K., Cheng, P., Ji, X., Li, G., et al. (2021). A Novel Redox-Responsive Ursolic Acid Polymeric Prodrug Delivery System for Osteosarcoma Therapy. Drug Deliv. 28 (1), 195–205. doi:10.1080/10717544.2020.1870583
Gabizon, A., Shmeeda, H., and Grenader, T. (2012). Pharmacological Basis of Pegylated Liposomal Doxorubicin: Impact on Cancer Therapy. Eur. J. Pharm. Sci. 45 (4), 388–398. doi:10.1016/j.ejps.2011.09.006
Globocan. Indonesia - Global Cancer Observatory WHO. International Agency for Research on Cancer, 2018. Available at: Https://GcoIarcFr/Today/Data/Factsheets/Populations/360-Indonesia-Fact-SheetsPdf. 256. (Accessed 2020 1 2).
Hansen, A. E., Petersen, A. L., Henriksen, J. R., Boerresen, B., Rasmussen, P., Elema, D. R., et al. (2015). Positron Emission Tomography Based Elucidation of the Enhanced Permeability and Retention Effect in Dogs with Cancer Using Copper-64 Liposomes. ACS Nano 9 (7), 6985–6995. doi:10.1021/acsnano.5b01324
Hattori, Y., Yamashita, J., Sakaida, C., Kawano, K., and Yonemochi, E. (2014). Evaluation of Antitumor Effect of Zoledronic Acid Entrapped in Folate-Linked Liposome for Targeting to Tumor-Associated Macrophages. J. Liposome Res. 25 (2), 131–140. doi:10.3109/08982104.2014.954128
Internatioanl Agency for Research on Cancer (2018). Latest Global Cancer Data : Cancer burden Rises to 18.1 Million New Cases and 9 . 6 Million Cancer Deaths in 2018.
Jin, H., Pi, J., Yang, F., Jiang, J., Wang, X., Bai, H., et al. (2016). Folate-Chitosan Nanoparticles Loaded with Ursolic Acid Confer Anti-breast Cancer Activities In Vitro and In Vivo. Sci. Rep. 6, 30782. July 2016. doi:10.1038/srep30782
Jin, H., Pi, J., Yang, F., Wu, C., Cheng, X., Bai, H., et al. (2016). Ursolic Acid-Loaded Chitosan Nanoparticles Induce Potent Anti-angiogenesis in Tumor. Appl. Microbiol. Biotechnol. 100, 6643–6652. doi:10.1007/s00253-016-7360-8
Kamińska, K., Szczylik, C., Bielecka, Z. F., Bartnik, E., Porta, C., Lian, F., et al. (2015). The Role of the Cell-Cell Interactions in Cancer Progression. J. Cel Mol Med 19 (2), 283–296. doi:10.1111/jcmm.12408
Kashyap, D., Tuli, H. S., and Sharma, A. K. (2016). Ursolic Acid (UA): A Metabolite with Promising Therapeutic Potential. Life Sci. 146, 201–213. doi:10.1016/j.lfs.2016.01.017
Kharia, A. A., Singhai, A. K., and Verma, R. (2012). Formulation and Evaluation of Polymeric Nanoparticles of an Antiviral Drug for Gastroretention. PCI- Approved-IJPSN 4 (4), 1557–1562. doi:10.37285/ijpsn.2011.4.4.6
Khwaza, V., Oyedeji, O. O., and Aderibigbe, B. A. (2020). Ursolic Acid-Based Derivatives as Potential Anti-cancer Agents : An Update.
Koda, Y., Liang, M. T., Blanchfield, J. T., and Toth, I. (2008). In Vitro stability and Permeability Studies of Liposomal Delivery Systems for a Novel Lipophilic Endomorphin 1 Analogue. Int. J. Pharm. 356 (1–2), 37–43. doi:10.1016/j.ijpharm.2007.12.036
Koppolu, B., and Zaharoff, D. A. (2013). The Effect of Antigen Encapsulation in Chitosan Particles on Uptake, Activation and Presentation by Antigen Presenting Cells. Biomaterials 34 (9), 2359–2369. Mar. doi:10.1016/j.biomaterials.2012.11.066
Kulkarni, S. B., Betageri, G. V., and Singh, M. (1995). Factors Affecting Microencapsulation of Drugs in Liposomes. J. Microencapsul 12 (3), 229–246. doi:10.3109/02652049509010292
Kumari, P., Ghosh, B., and Biswas, S. (2016). Nanocarriers for Cancer-Targeted Drug Delivery. J. Drug Target. 24 (3), 179–191. doi:10.3109/1061186X.2015.1051049
Laouini, A., Jaafar-Maalej, C., Limayem-Blouza, I., Sfar, S., Charcosset, C., Fessi, H., et al. (2012). Preparation, Characterization and Applications of Liposomes: State of the Art. J Coll. Sci. Biotechnol. 1, 147–168. doi:10.1166/jcsb.2012.1020
Li, W., Yan, R., Liu, Y., He, C., Zhang, X., Lu, Y., et al. (2019). Co-delivery of Bmi1 Small Interfering RNA with Ursolic Acid by Folate Receptor-Targeted Cationic Liposomes Enhances Anti-tumor Activity of Ursolic Acid In Vitro and In Vivo. Drug Deliv. 26 (1), 794–802. doi:10.1080/10717544.2019.1645244
Li, Z., Tan, S., Li, S., Shen, Q., and Wang, K. (2017). Cancer Drug Delivery in the Nano Era: An Overview and Perspectives (Review). Oncol. Rep. 38 (2), 611–624. doi:10.3892/or.2017.5718
Liu, Y., Kong, T., Yang, Z., Zhang, Y., Lei, J., and Zhao, P. (2021). Self-Assembled Folic Acid-Targeted Pectin-Multi-Arm Polyethylene Glycol Nanoparticles for Tumor Intracellular Chemotherapy. ACS omega 6 (2), 1223–1234. Jan. doi:10.1021/acsomega.0c04350
Liu, Y., Liu, K., Li, X., Xiao, S., Zheng, D., Zhu, P., et al. (2018). A Novel Self-Assembled Nanoparticle Platform Based on Pectin-Eight-Arm Polyethylene Glycol-Drug Conjugates for Co-delivery of Anticancer Drugs. Mater. Sci. Eng. C Mater. Biol. Appl. 86, 28–41. doi:10.1016/j.msec.2017.12.018
Lunardi, C. N., Gomes, A. J., Rocha, F. S., De Tommaso, J., and Patience, G. S. (2021). Experimental Methods in Chemical Engineering: Zeta Potential. Can. J. Chem. Eng. 99 (3), 627–639. Mar 1. doi:10.1002/cjce.23914
Ma, P., and Mumper, R. J. (2013). Paclitaxel Nano-Delivery Systems: A Comprehensive Review. J. Nanomed Nanotechnol 4 (2), 1000164. doi:10.4172/2157-7439.1000164
Melo, R. C. N., Raas, M. W. D., Palazzi, C., Neves, V. H., Malta, K. K., and Silva, T. P. (2020). Whole Slide Imaging and its Applications to Histopathological Studies of Liver Disorders. Front. Med. (Lausanne) 6 (January), 310–313. doi:10.3389/fmed.2019.00310
Miatmoko, A., Kawano, K., Yoda, H., Yonemochi, E., and Hattori, Y. (2017). Tumor Delivery of Liposomal Doxorubicin Prepared with Poly-L-Glutamic Acid as a Drug-Trapping Agent. J. Liposome Res. 27 (2), 99–107. doi:10.3109/08982104.2016.1166511
Miele, E., Spinelli, G. P., Miele, E., Tomao, F., and Tomao, S. (2009). Albumin-bound Formulation of Paclitaxel (Abraxane ABI-007) in the Treatment of Breast Cancer. Int. J. Nanomedicine 4, 99–105. 2009/04/20. doi:10.2147/ijn.s3061
Mitchell, M. J., Billingsley, M. M., Haley, R. M., Wechsler, M. E., Peppas, N. A., and Langer, R. (2021). Engineering Precision Nanoparticles for Drug Delivery. Nat. Rev. Drug Discov. 20, 101–124. doi:10.1038/s41573-020-0090-8
Mizumura, Y., Matsumura, Y., Hamaguchi, T., Nishiyama, N., Kataoka, K., Kawaguchi, T., et al. (2001). Cisplatin-incorporated Polymeric Micelles Eliminate Nephrotoxicity, while Maintaining Antitumor Activity. Jpn. J. Cancer Res. 92 (March), 328–336. doi:10.1111/j.1349-7006.2001.tb01099.x
Monteiro, N., Martins, A., Reis, R. L., and Neves, N. M. (2014). Liposomes in Tissue Engineering and Regenerative Medicine. J. R. Soc. Interf. 11 (101), 20140459. doi:10.1098/rsif.2014.0459
Nishiyama, N., Okazaki, S., Cabral, H., Miyamoto, M., Kato, Y., Sugiyama, Y., et al. (2003). Novel Cisplatin-Incorporated Polymeric Micelles Can Eradicate Solid Tumors in Mice. Cancer Res. 63 (24), 8977–8983.
Pérez-Herrero, E., and Fernández-Medarde, A. (2015). Advanced Targeted Therapies in Cancer: Drug Nanocarriers, the Future of Chemotherapy. Eur. J. Pharm. Biopharm. 93, 52–79.
Plummer, R., Wilson, R. H., Calvert, H., Boddy, A. V., Griffin, M., Sludden, J., et al. (2011). A Phase I Clinical Study of Cisplatin-Incorporated Polymeric Micelles (NC-6004) in Patients with Solid Tumours. Br. J. Cancer 104 (4), 593–598. doi:10.1038/bjc.2011.6
Poudel, K., Gautam, M., Maharjan, S., Jeong, J. H., Choi, H. G., Khan, G. M., et al. (2020). Dual Stimuli-Responsive Ursolic Acid-Embedded Nanophytoliposome for Targeted Antitumor Therapy. Int. J. Pharm. 582 (December 2019), 119330. doi:10.1016/j.ijpharm.2020.119330
Qian, Z., Wang, X., Song, Z., Zhang, H., Zhou, S., Zhao, J., et al. (2015). A Phase I Trial to Evaluate the Multiple-Dose Safety and Antitumor Activity of Ursolic Acid Liposomes in Subjects with Advanced Solid Tumors. Biomed. Res. Int. 2015, 809714–809717. doi:10.1155/2015/809714
Rajesh, S. J. W. L. (2000). Nanoparticle-based Targeted Drug Delivery. Exp. Mol. Pathol. 86 (3), 215–223.
Reddy, L. H. (2005). Drug Delivery to Tumours: Recent Strategies, Internet. J. Pharm. Pharmacol. 57 (10), 1231–1242. Available from: http://www.ncbi.nlm.nih.gov/pubmed/16259751. doi:10.1211/jpp.57.10.0001
Seo, D. Y., Lee, S. R., Heo, J. W., No, M. H., Rhee, B. D., Ko, K. S., et al. (2018). Ursolic Acid in Health and Disease. Korean J. Physiol. Pharmacol. 22 (3), 235–248. doi:10.4196/kjpp.2018.22.3.235
Sercombe, L., Veerati, T., Moheimani, F., Wu, S. Y., Sood, A. K., and Hua, S. (2015). Advances and Challenges of Liposome Assisted Drug Delivery. Front. Pharmacol. 6 (DEC), 286. doi:10.3389/fphar.2015.00286
Shaker, S., Gardouh, A. R., and Ghorab, M. M. (2017). Factors Affecting Liposomes Particle Size Prepared by Ethanol Injection Method. Res. Pharm. Sci. 12 (5), 346–352. doi:10.4103/1735-5362.213979
Sharma, A., and Sharma, U. S. (1997). Liposomes in Drug Delivery: Progress and Limitations. Int. J. Pharmaceutics 154 (2), 123–140. doi:10.1016/s0378-5173(97)00135-x
Shen, Z., Li, B., Liu, Y., Zheng, G., Guo, Y., Zhao, R., et al. (2018). A Self-Assembly Nanodrug Delivery System Based on Amphiphilic Low Generations of PAMAM Dendrimers-Ursolic Acid Conjugate Modified by Lactobionic Acid for HCC Targeting Therapy. Nanomedicine 14 (2), 227–236. doi:10.1016/j.nano.2017.10.007
Silverman, J. A., and Deitcher, S. R. (2012). Marqibo® (Vincristine Sulfate Liposome Injection) Improves the Pharmacokinetics and Pharmacodynamics of Vincristine. Cancer Chemother. Pharmacol. 71 (3), 555–564./12/05. 2013 Mar. doi:10.1007/s00280-012-2042-4
Slaoui, M., and Fiette, L. (2011). Histopathology Procedures: from Tissue Sampling to Histopathological Evaluation. Methods Mol. Biol. 691 (December 2014), 69–82. doi:10.1007/978-1-60761-849-2_4
Sun, Q., He, M., Zhang, M., Zeng, S., Chen, L., Zhou, L., et al. (2020). Ursolic Acid: A Systematic Review of its Pharmacology, Toxicity and Rethink on its Pharmacokinetics Based on PK-PD Model. Fitoterapia 147, 104735. doi:10.1016/j.fitote.2020.104735
Swenson, C. E., Perkins, W. R., Roberts, P., and Janoff, A. S. (2001). Liposome Technology and the Development of Myocet (Liposomal Doxorubicin Citrate). The Breast 10, 1–7. doi:10.1016/s0960-9776(01)80001-1
Uchino, H., Matsumura, Y., Negishi, T., Koizumi, F., Hayashi, T., Honda, T., et al. (2005). Cisplatin-incorporating Polymeric Micelles (NC-6004) Can Reduce Nephrotoxicity and Neurotoxicity of Cisplatin in Rats. Br. J. Cancer 93 (6), 678–687. doi:10.1038/sj.bjc.6602772
Valcourt, D. M., Kapadia, C. H., Scully, M. A., Dang, M. N., and Day, E. S. (2020). Best Practices for Preclinical In Vivo Testing of Cancer Nanomedicines. Adv. Healthc. Mater. 9 (12), e2000110–15. doi:10.1002/adhm.202000110
Ventola, C. L. (2017). Progress in Nanomedicine: Approved and Investigational Nanodrugs. P T 42 (12), 742–755.
Wang, M., Zhao, T., Liu, Y., Wang, Q., Xing, S., Li, L., et al. (2017). Ursolic Acid Liposomes with Chitosan Modification: Promising Antitumor Drug Delivery and Efficacy. Mater. Sci. Eng. C Mater. Biol. Appl. 71, 1231–1240. doi:10.1016/j.msec.2016.11.014
Wang, Q., Zhao, T., Liu, Y., Xing, S., Li, L., and Gao, D. (2016). An Evaluation of Anti-tumor Effect and Toxicity of PEGylated Ursolic Acid Liposomes. J. Nanoparticle Res. 18 (2), 1–13. doi:10.1007/s11051-016-3339-8
Wang, X. H., Zhou, S. Y., Qian, Z. Z., Zhang, H. L., Qiu, L. H., Song, Z., et al. (2013). Evaluation of Toxicity and Single-Dose Pharmacokinetics of Intravenous Ursolic Acid Liposomes in Healthy Adult Volunteers and Patients with Advanced Solid Tumors. Expert Opin. Drug Metab. Toxicol. 9 (2), 117–125. doi:10.1517/17425255.2013.738667
Webb, C., Khadke, S., Schmidt, S. T., Roces, C. B., Forbes, N., Berrie, G., et al. (2019). The Impact of Solvent Selection: Strategies to Guide the Manufacturing of Liposomes Using Microfluidics. Pharmaceutics 11 (12), 653. doi:10.3390/pharmaceutics11120653
Wei, X., Cohen, R., and Barenholz, Y. (2016). Insights into Composition/structure/function Relationships of Doxil® Gained from "High-Sensitivity" Differential Scanning Calorimetry, Internet. Eur. J. Pharm. Biopharm. 104, 260–270. Available from: http://linkinghub.elsevier.com/retrieve/pii/S0939641116301357. doi:10.1016/j.ejpb.2016.04.011
Yang, G., Yang, T., Zhang, W., Lu, M., Ma, X., and Xiang, G. (2014). Vitro and In Vivo Antitumor E Ff Ects of Folate-Targeted Ursolic AcidIn Vitro and In Vivo Antitumor Effects of Folate-Targeted Ursolic Acid Stealth Liposome. J. Agric. Food Chem. 62 (10), 2207–2215. doi:10.1021/jf405675g
Yoshimoto, M., Tamura, R., and Natsume, T. (2013). Liposome Clusters with Shear Stress-Induced Membrane Permeability. Chem. Phys. Lipids 174, 8–16. doi:10.1016/j.chemphyslip.2013.06.001
Zaimy, M. A., Saffarzadeh, N., Mohammadi, A., Pourghadamyari, H., Izadi, P., Sarli, A., et al. (2017). New Methods in the Diagnosis of Cancer and Gene Therapy of Cancer Based on Nanoparticles. Cancer Gene Ther. 24 (6), 233–243. doi:10.1038/cgt.2017.16
Zhang, H. (2016). Onivyde for the Therapy of Multiple Solid Tumors. Onco Targets Ther. 9, 3001–3007. doi:10.2147/OTT.S105587
Zhang, H., Zheng, D., Ding, J., Xu, H., Li, X., and Sun, W. (2015). Efficient Delivery of Ursolic Acid by poly(N-Vinylpyrrolidone)-Block-Poly (ε-Caprolactone) Nanoparticles for Inhibiting the Growth of Hepatocellular Carcinoma In Vitro and In Vivo. Int. J. Nanomedicine 10, 1909–1920. doi:10.2147/IJN.S77125
Zhang, N., Liu, S., Shi, S., Chen, Y., Xu, F., Wei, X., et al. (2020). Solubilization and Delivery of Ursolic-Acid for Modulating Tumor Microenvironment and Regulatory T Cell Activities in Cancer Immunotherapy. J. Control. Release 320 (January), 168–178. doi:10.1016/j.jconrel.2020.01.015
Zhao, R., Zheng, G., Fan, L., Shen, Z., Jiang, K., Guo, Y., et al. (2018). Carrier-free Nanodrug by Co-assembly of Chemotherapeutic Agent and Photosensitizer for Cancer Imaging and Chemo-Photo Combination Therapy. Acta Biomater. 70, 197–210. doi:10.1016/j.actbio.2018.01.028
Zhou, M., Yi, Y., Liu, L., Lin, Y., Li, J., Ruan, J., et al. (2019). Polymeric Micelles Loading with Ursolic Acid Enhancing Anti-tumor Effect on Hepatocellular Carcinoma. J. Cancer 10 (23), 5820–5831. doi:10.7150/jca.30865
Keywords: ursolic acid, cancer, nanoparticle, liposome, nanosphere, polymeric micelle, efficacy, toxicity
Citation: Miatmoko A, Mianing EA, Sari R and Hendradi E (2021) Nanoparticles use for Delivering Ursolic Acid in Cancer Therapy: A Scoping Review. Front. Pharmacol. 12:787226. doi: 10.3389/fphar.2021.787226
Received: 30 September 2021; Accepted: 29 November 2021;
Published: 24 December 2021.
Edited by:
Michael Whittaker, Monash University, AustraliaReviewed by:
Khalid Saad Alharbi, Jouf University, Saudi ArabiaYong Sze Ong, Monash University Malaysia, Malaysia
Copyright © 2021 Miatmoko, Mianing, Sari and Hendradi. This is an open-access article distributed under the terms of the Creative Commons Attribution License (CC BY). The use, distribution or reproduction in other forums is permitted, provided the original author(s) and the copyright owner(s) are credited and that the original publication in this journal is cited, in accordance with accepted academic practice. No use, distribution or reproduction is permitted which does not comply with these terms.
*Correspondence: Andang Miatmoko, YW5kYW5nLW1AZmYudW5haXIuYWMuaWQ=