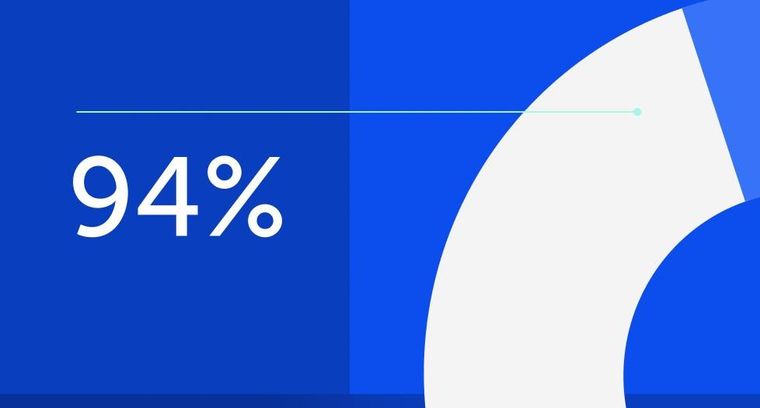
94% of researchers rate our articles as excellent or good
Learn more about the work of our research integrity team to safeguard the quality of each article we publish.
Find out more
ORIGINAL RESEARCH article
Front. Pharmacol., 13 January 2022
Sec. Inflammation Pharmacology
Volume 12 - 2021 | https://doi.org/10.3389/fphar.2021.785193
This article is part of the Research TopicStreaming Inflammation: From Damage to Healing and ResilienceView all 12 articles
In agriculture industries, workers are at increased risk for developing pulmonary diseases due to inhalation of agricultural dusts, particularly when working in enclosed confinement facilities. Agricultural dusts inhalation leads to unresolved airway inflammation that precedes the development and progression of lung disease. We have previously shown beneficial effects of the omega-3 polyunsaturated fatty acid (ω-3 PUFA) DHA in protecting against the negative inflammatory effects of repetitive dust exposure in the lung. Dietary manipulation of pulmonary disease risk is an attractive and timely approach given the contribution of an increased ω-6 to ω-3 PUFA ratio to low grade inflammation and chronic disease in the Western diet. To prevent any confounding factors that comes with dietary supplementation of ω-3 PUFA (different sources, purity, dose, and duration), we employed a Fat-1 transgenic mouse model that convert ω-6 PUFA to ω-3 PUFA, leading to a tissue ω-6 to ω-3 PUFA ratio of approximately 1:1. Building on our initial findings, we hypothesized that attaining elevated tissue levels of ω-3 PUFA would attenuate agricultural dust-induced lung inflammation and its resolution. To test this hypothesis, we compared wild-type (WT) and Fat-1 transgenic mice in their response to aqueous extracts of agricultural dust (DE). We also used a soluble epoxide hydrolase inhibitor (sEH) to potentiate the effects of ω-3 PUFA, since sEH inhibitors have been shown to stabilize the anti-inflammatory P450 metabolites derived from both ω-3 and ω-6 PUFA and promote generation of specialized pro-resolving lipid mediators from ω-3 PUFA. Over a three-week period, mice were exposed to a total of 15 intranasal instillations of DE obtained from swine confinement buildings in the Midwest. We observed genotype and sex-specific differences between the WT vs. Fat-1 transgenic mice in response to repetitive dust exposure, where three-way ANOVA revealed significant main effects of treatment, genotype, and sex. Also, Fat-1 transgenic mice displayed reduced lymphoid aggregates in the lung following DE exposure as compared to WT animals exposed to DE, suggesting improved resilience to the DE-induced inflammatory effects. Overall, our data implicate a protective role of ω-3 FA in the lung following repetitive dust exposure.
Agricultural workers are at increased risk for developing various respiratory diseases including chronic bronchitis, asthma, and COPD, due in part to exposure to respirable organic dusts associated with these environments (Von Essen and Romberger, 2003; Nordgren and Charavaryamath, 2018; Sigsgaard et al., 2020). Individuals that work in concentrated animal feeding operations, such as those housing swine, have appreciably increased risk for negative lung health outcomes (Iversen and Dahl, 2000; Kirkhorn and Garry, 2000; Pedersen et al., 2000; May et al., 2012; Pavilonis et al., 2013; Guillien et al., 2016; Nordgren and Bailey, 2016; Nordgren and Charavaryamath, 2018). Therapeutic options for affected individuals are limited, with no current treatments to reverse lung function decline associated with these ailments (American Thoracic, 1998; Kirkhorn and Garry, 2000; Kachan et al., 2012; Hoppin et al., 2014). Thus, novel treatment strategies that harness and/or promote reparative processes in the lung are necessary.
It is increasingly appreciated that inflammation resolution is an active process and regulated by a variety of pathways and mediators, some of which involve omega-3 (ω-3) and omega-6 (ω-6) polyunsaturated fatty acids (PUFA) (Levy and Serhan, 2014; Serhan et al., 2014; Hammock et al., 2020). As ω-3 PUFA are essential fatty acids that cannot be synthesized de novo by humans, dietary consumption of ω-3 PUFA dictates the tissue availability for these fatty acids and mediators derived from them. In a typical Western diet, ω-3 PUFA intakes are below recommended guidelines, while ω-6 PUFA intakes are high (Thompson et al., 2019). Conversely to ω-3 PUFA, ω-6 PUFA are metabolized into lipid mediators (e.g., leukotrienes, thromboxane, prostaglandins) that are largely involved in the induction of inflammatory processes (Calder et al., 2020). Thus, individuals consuming a diet with a high ω-6: ω-3 PUFA ratio may be at increased risk for inadequate control of inflammatory processes, with increased substrate to produce pro-inflammatory lipid mediators and a dearth of substrate for the production of specialized pro-resolving mediators (SPM).
We have recently assessed the efficacy of dietary supplementation with the ω-3 PUFA docosahexaenoic acid (DHA) on altering the lung inflammatory response and recovery following acute and repetitive organic dust exposure (Nordgren et al., 2014; Dominguez et al., 2020; Ulu et al., 2021a). Mice were fed a mouse chow supplemented with DHA for four consecutive weeks prior to challenge with a single DE exposure (acute model) or DE challenge over 3 weeks (repetitive model). In these investigations, we identified impacts of a high DHA diet on lung inflammation, including alterations in macrophage activation, that were overall protective against the deleterious impacts of DE exposure. However, these studies were limited in that they only assessed the impacts of one ω-3 PUFA, DHA, on male sex and on a limited dietary regimen of 4–7 weeks (Dominguez et al., 2020; Ulu et al., 2021a). Sex-specific differences in respiratory symptoms are observed among the asthmatic individuals and agricultural workers with asthma being more common in women than men and respiratory symptoms being more prevalent in men than women among the farmers (CDC, 2019; Fix et al., 2020). To better assess the impacts of a high ω-3 PUFA diet on the lung inflammatory response to DE and achieve a total tissue ω-6: ω-3 PUFA ratio of ∼1:1 that is considered ideal, we have now utilized the Fat-1 mouse transgenic model (Kang et al., 2004; Bilal et al., 2011) to better assess the sex-specific impacts of ω-3 PUFA on DE-induced inflammation. These mice express the Caenorhabditis elegans (C. elegans) fatty acid desaturase gene that converts ω-6 PUFA to ω-3 PUFA, thus yielding an overall tissue ratio of ∼1:1. We hypothesized that use of this model would enhance the protective effects identified in initial studies utilizing only DHA supplementation, while also overcoming study limitations that plague fatty acid supplementation investigations, including ambiguous outcomes due to different fatty acid sources, purity, doses, and duration of supplementation (Bradberry and Hilleman, 2013). In addition, we have also tested a strategy to further enhance the efficacy of ω-3 PUFAs through the use of a therapeutic inhibitor of soluble epoxide hydrolase (sEH) an enzyme that metabolizes lipid mediators such as SPM into inactive or less active forms (Ulu et al., 2013; Ulu et al., 2014; Ulu et al., 2016; Dileepan et al., 2019).
Through these investigations, we have clarified a role for ω-3 PUFA in regulating the initiation of lung inflammation following DE inhalation and identified differentially regulated genes in repair and recovery following these exposures. These studies warrant consideration of ω-3 PUFA supplementation as a complementary therapeutic strategy for protecting against the deleterious lung diseases associated with environmental dust exposures, such as those experienced by agriculture workers.
Settled dusts in closed swine confinement facilities (Nebraska) were collected one foot above the ground and kept at −20°C. Dust extracts were prepared as previously described (Romberger et al., 19852002). Briefly, 5 g dust was mixed with 50 ml Hank’s Balanced Salt Solution at room temperature for 1 h. The mixture was then centrifuged at 2,500 rpm for 20 min at 4°C, supernatant was centrifuged one more time and resultant supernatant was sterile filtered using a 0.22 μm filter. Extracts were aliquoted, labeled as 100% dust extract (DE) and kept frozen at −20°C. A 12.5% DE solution was prepared for use in mouse intranasal instillations by diluting the 100% extract with sterile saline. Detailed analyses of the DE have been performed previously (Poole et al., 2010; Boissy et al., 2014; Romberger et al., 2015). A previous study compared immune response to the agricultural dust administered via intranasal instillation and 100 µg LPS challenge in mice (Poole et al., 2011), which has been estimated to be approximately 250× more than the LPS in 12.5% DE. In this same study, the mean endotoxin levels have been reported to be 0.384 μg/ml. Given this finding, when we administer 50 µL DE via intranasal route, this would correspond to approximately 20 ng LPS. In addition, other studies report respirable LPS levels to be between 14–129 EU/mL (one EU is approximately 0.1–0.2 ng/ml) (Demanche et al., 2009).
All animal protocols were approved by the Institutional Animal Care and Use Committee of the University of California, Riverside. Male and female 10–12-week-old C57BL/6 WT (WT) and Fat-1 transgenic mice [C57BL/6-Tg (CAG-Fat-1)1Jxk/J] were purchased from Jackson Laboratories and used to obtain a mouse colony. Breeding pairs were set as WT x Fat-1 or Fat-1 x Fat-1 to obtain both the WT and Fat-1 genotypes. Mice had ad libitum access to food and water. Male and female mice were housed in separate cages with five mice/cage. Male and female pups reaching the age of 6–8 weeks were administered the sEH inhibitor TPPU [1-trifluoromethoxyphenyl-3-(1-propionylpiperidin-4-yl) urea] in their drinking water at 1 mg/kg dose for 1 week before intranasal dust exposure commences. For the repetitive dust exposure model, mice were instilled via the intranasal route with 50 µL of 12.5% DE for three-weeks (a total of 15 instillations, 5 days/week) as published before (Poole et al., 2009; Nordgren et al., 2015; Nordgren et al., 2019). Instillations were performed under light anesthesia using isoflurane.
At the end of the three-week period, mice were euthanized, and trachea were cannulated to obtain bronchoalveolar lavage fluid (BALF) from each mouse. Collection of BALF included three times washing with 1 ml PBS each time. All washes were centrifuged at 1,200 rpm for 5 min. While the first wash was kept separate, the second and third washes were combined before centrifugation. The supernatant from the first wash was aliquoted and stored in −80°C for cytokine profiling. The pelleted cells obtained from all the washes were combined and counted. Cytospin slides were prepared using 100,000 cells, stained with Diff-Quik kit (Siemens, Newark, DE) and differential cell counts were obtained as described before (Nordgren et al., 2015).
For histopathological assessments, lungs were inflated with 10% buffered formalin at 15 cm pressure. The same mouse lungs that were lavaged with PBS to obtain BALF was used for histology. Fixed lungs were transferred into 70% ethanol and then shipped to UC Irvine Pathology Research Services Core for paraffin embedding, sectioning, and Hematoxylin and Eosin (H and E) staining. The observer was blinded to the identity of each slide. A lymphoid aggregate was defined as close aggregation of ≥20 lymphocytes. Alveolar cellularity was evaluated by the number of cells in the alveolar spaces in the lung parenchyma in a total of five images obtained throughout the whole lung using 40× objective with 150% optical zoom. The resulting five values were averaged per tissue section. Each histopathological evaluation was represented as percentiles and a score between 0-to-4 was assigned for each percentile.
A mouse NanoString Immunology panel (NanoString Technologies, Seattle, WA, United States) was purchased for direct counting of 561 RNA transcripts using a nCounter Sprint Profiler. Each mouse lung was immediately put into 1 ml of RNA Later, kept at 4°C overnight, then stored in RNA Later at −80°C until RNA extraction. A total of three male mouse lungs per group obtained from three independent studies were thawed for RNA extraction. After lung samples were rinsed in sterile PBS, they were homogenized in 1 ml of Trizol using a 7 cm polypropylene pellet pestle in a microtube, then the extraction was performed as per manufacturer’s instructions using a PureLink RNA mini kit (Invitrogen, Carlsbad, California, United States). RNA integrity number was obtained for each sample (ranged from 7–9 on a scale of 1–10) at the UC Riverside Institute for Integrative Genome Biology Core Facility using an Agilent Bioanalyzer 2,100 (Agilent Technologies, Santa Clara, California, United States). Samples were prepared by a 16-h hybridization step of 50 ng RNA with the codeset probe provided in the Immunology panel. At the end of the hybridization, samples were diluted with nuclease-free water to 35 μL, and 32 µL of each sample was loaded onto a nCounter Sprint Cartridge. Given each cartridge can hold up to 12 samples, a total of 24 samples were run on two cartridges. All samples passed the QC test without any QC flags. The data resulting from each run were combined and analyzed together using nSolver 4.0 and NanoString Advanced analysis. On the nSolver software, gene expression data were normalized using ten housekeeping genes that showed strong correlation with each other, these included Rpl19, Alas1, Ppia, Oaz1, Sdha, Eef1g, Gusb, Gapdh, Hprt and Tbp. Heatmaps were generated using the agglomerative clustering analysis in nSolver software (nSolver 4.0, NanoString Technologies, Seattle, WA, United States, User Manual). For the advanced analysis, at least three housekeeping genes whose expression correlated well with each other (Alas1, Ppia, Tbp and Tubb5), and thus ideal for normalization of the data were used to normalize the raw data (NanoString, MAN-C0011-04 Gene Expression Data Analysis Guidelines), and a 77 transcript counts were taken as “count threshold”, which is two times the highest background-to-noise ratio (average of negative controls/sample + 2*standard deviation of negative controls/sample). Advanced analysis produced differential expression analysis, gene set analysis, and pathway scores. Differential expression outcomes identified the top 20 most upregulated genes among all the treatments, while gene set analysis displayed which pathways those most upregulated genes are related to.
To further explore the protein-protein interactions among differentially regulated proteins, we used the STRING database (https://string-db.org) of genes that were statistically significant based on the unadjusted p-values (Szklarczyk et al., 2021). Genes with low counts (counts < 79) were not included in any of the analyses. Raw and normalized NanoString data are deposited to https://www.ncbi.nlm.nih.gov/geo/info/spreadsheet.html.
GraphPad Prism software (Prism 9) was utilized to perform two-way ANOVA tests and version eight was used to perform three-way ANOVA on data to determine the main effects of exposure and treatments, and post-hoc comparisons were performed to reveal significant differences among all the groups. Differences between groups were considered significant if the p value ≤ 0.05. Data are represented with mean ± standard error of the mean on all figures.
To test the hypothesis that elevated tissue levels of ω-3 fatty acids are protective against agricultural dust induced lung inflammation and improve inflammation resolution, we compared WT and Fat-1 transgenic mice for their response to three-weeks repetitive dust exposure. As expected, mice exposed to aqueous dust extracts for the duration of three-weeks (5 instillations/week) had 2-3-fold increase in total BALF cell counts as compared to their corresponding saline controls. First, we examined the main effects of exposure, genotype, sex and TPPU treatment on the total infiltrating immune cell counts (Figure 1A). We found statistically significant differences among the groups that are driven by DE exposure, TPPU treatment (F = 51.2, p < 0.0001) and sex (F = 3.028, p = 0.0096). Further statistical analysis of the total cell counts also revealed that both sex and genotype plays a role in response to DE exposure and TPPU treatment (interaction between exposure/treatment x sex, F = 3.463, p = 0.052; genotype x sex, F = 1.417, p = 0.073). We observed similarly significant results for the infiltrating neutrophils but not for macrophages (Figures 1B,C). This increase in neutrophil numbers is consistent with the cellular signature of repetitive DE exposure (Nordgren et al., 2014; Nordgren et al., 2013; Ulu et al., 2021b; Warren et al., 2017). With regards to eosinophils, we did not detect any differences among the groups (Figure 1D). Lymphocyte counts showed a statistically significant increase only in Fat-1 females that were exposed to dust. These counts were lower in the presence of TPPU; however, this difference did not reach significance (Figure 1E). All the significant main effects and relevant multiple comparisons are shown in each figure. When administered alone, TPPU did not affect any immune cell infiltration into the lung, which is consistent with previously reported homeostatic effects of sEH inhibition (Morisseau and Hammock, 2013; Vanella et al., 2015; Kuo et al., 2019). At the dose we administered (1 mg/kg), TPPU reaches plasma concentrations of >50-fold of the mouse IC50 of 2.8 nM, suggesting good target engagement (Supplementary Figure S1). Among the DE-exposed mice, TPPU was most effective in reducing the number of infiltrating cells in Fat-1 male mice (Figures 1A,B). While this decrease with TPPU was not significant for total infiltrating neutrophils (p = 0.9, Fat-1+DE male mice vs. Fat-1 DE + TPPU), we observed a striking difference between the Fat-1 male versus Fat-1 female mice receiving the TPPU treatment during dust-exposure (p = 0.032 for total infiltrating cells and p = 0.0029 for neutrophils between Fat-1 male + DE + TPPU vs. Fat-1 female + DE + TPPU). Since TPPU can stabilize the P450 metabolites derived from the ω-3 fatty acids, EPA (eicosapentaenoic acid) and DHA and also promote generation of SPMs, these data suggest that elevated ω-3 fatty acids could be stabilized with a sEH inhibitor to contribute to the decrease in pro-inflammatory cell influx into the lung in male sex.
FIGURE 1. Genotype and sex-specific changes in infiltrating immune cells in the bronchoalveolar lavage fluid after a three-weeks repetitive dust exposure with or without TPPU treatment. (A) Total infiltrating cell counts, (B) neutrophil count, (C) macrophage count, (D) eosinophil count, (E) lymphocyte count; n = 6–8 mice WT male (except for WT TPPU n = 5), n = 6–8 WT female, n = 6 Fat-1 male, n = 6–7 Fat-1 female. Significant main effects of three-way ANOVA are shown. Statistical significance is shown with letters: (a) significantly different from WT male, (b) significantly different from WT female, (c) significantly different from Fat-1 male, (d) significantly different from Fat-1 female. Data are mean ± SEM. *p < 0.05, **p < 0.01, ***p < 0.001, ****p < 0.0001.
Similarly, pro-inflammatory cytokines in BALF decreased in Fat-1 transgenic mice receiving TPPU among the other Fat-1 mice exposed to dust (Figure 2). The three-way ANOVA analysis showed significant main effects of dust exposure/TPPU (F = 13.87, p = 0.0024) and a trend for the main effect of sex (F = 3.02, p = 0.068) on BALF IL-6 levels (Figure 2A). Regarding the BALF levels of TNF-α, we observed significant main effects of dust exposure or TPPU (F = 9.48, p = 0.037). For the neutrophil chemoattractant CXCL-1, we observed a statistically significant main effect of exposure/treatment (F = 29.8, p < 0.0001) and a statistical trend for interaction between dust exposure/TPPU x genotype (F = 5.4, p = 0.064). Since sex did not have a significant effect on CXCL-1 BALF levels, we also examined the data after combining both sexes. Similarly, we found a significant main effect of treatment (F = 30.36, p < 0.0001). When we investigated the specific differences among the groups, we found significant differences between WT + DE vs. WT + DE + TPPU as well as WT + DE vs. Fat-1 + DE + TPPU groups (Figure 2C, inset). Similarly, dust-exposed Fat-1 transgenic mice exhibited lower levels of CXCL-1 as compared to WT mice; however, this difference did not reach significance (p = 0.1).
FIGURE 2. Effects of three-weeks repetitive dust exposure on pro-inflammatory cytokine levels in the bronchoalveolar lavage fluid with or without TPPU treatment. (A) IL-6, n = 5–7 mice WT male, n = 6–8 WT female, n = 5–7 Fat-1 male, n = 6–7 Fat-1 female; (B) CXCL-1, n = 5–8 mice WT male, n = 5–6 WT female, n = 4–6 Fat-1 male, n = 5–7 Fat-1 female; (C) TNF-α, n = 5–8 mice WT male, n = 5–7 WT female, n = 5-6 Fat-1 male, n = 5–7 Fat-1 female. The inset shows data from both female and male sexes, n = 11–13 for WT and n = 9–12 for Fat-1 mice. Data are mean ± SEM. Significant main effects of three-way ANOVA are shown. Statistical significance is shown with letters: (a) significantly different from WT male, (b) significantly different from WT female, (c) significantly different from Fat-1 male, (d) significantly different from Fat-1 female. **p < 0.01, ***p < 0.001.
Lung histopathology was evaluated in H&E-stained paraffin-embedded tissue sections mounted on slides to evaluate dust-induced lung inflammation (Figure 3). We have previously shown that total number of lymphoid aggregates (defined as at least 20 closely aggregating cells) and alveolar inflammation evaluated as the number of macrophages in alveolar spaces increase with repetitive exposure to agricultural dust (Ulu et al., 2021b). The 2-3-fold increase in the number of BALF leukocytes following repetitive exposure to DE was reflected in approximately two fold increase in histopathological scores.
FIGURE 3. Representative images and scoring of lung histopathology in WT and Fat-1 transgenic mice following 3 weeks exposure. (A) Alveolar cellularity, n = 3–5 mice WT male, n = 2–5 WT female, n = 5–9 Fat-1 male, n = 2–6 Fat-1 female; (B) lymphoid aggregate formation, n = 5–8 mice WT male, n = 5–6 WT female, n = 4–6 Fat-1 male, n = 5–7 Fat-1 female; (C) quantification and pathological scoring of the alveolar cellularity and (D) lymphoid aggregate formation. Sample sizes for each group were shown on each graph. (a) significantly different from WT male, (b) significantly different from WT female. Data are mean ± SEM. *p < 0.05, **p < 0.01, ***p < 0.001, ****p < 0.0001.
As expected, the number of macrophages in alveolar spaces increased approximately two-fold with three-weeks repetitive exposure to DE in both WT and Fat-1 transgenic mice with a significant main effect of dust exposure and/or TPPU (F = 29.97, p < 0.0001) (Figures 3A,C). We also observed significant interaction between sex and genotype (F = 5.11, p = 0.018), which was reflected in a statistically significant difference in alveolar inflammation score in Fat-1 males and WT females that were receiving the three-weeks DE exposure as compared to their saline control counterparts.
Similarly, we observed a significant main effect of DE and/or TPPU (F = 32.15, p < 0.0001) and their interaction with genotype (F = 16.95, p < 0.0001) on lymphoid aggregate formation (Figures 3B,D). In WT mice receiving the three-weeks DE exposure, the number of lymphoid aggregates significantly increased as compared to WT saline controls (p < 0.0001). Among the mice exposed to DE, Fat-1 mice exhibited less lymphoid aggregates than the WT mice but only in the male sex (p = 0.0002). In addition, we observed a sex-dependent difference in TPPU treatment, which significantly reduced lymphoid aggregate formation in male mice receiving the three-weeks DE exposure regardless of the genotype (p = 0.0019, WT + DE male vs. WT + DE + TPPU male and p = 0.014, WT DE male vs. Fat-1 + DE + TPPU male).
ω-3 fatty acids are known to regulate gene expression (Ulven and Holven, 2020). To elucidate differences in lung gene expression between the WT and Fat-1 transgenic mice following repetitive dust exposure, we used a NanoString Mouse Immunology Panel as described in the Methods. We used only male sex for the gene expression study since we saw the most significant changes in the male sex as compared to the females. Following data acquisition, an automated QC test (quality control) was performed on nSolver software which showed that all samples passed this test with no warning flags. Then, samples were normalized to housekeeping genes and principal component analysis (PCA) of the data were performed. The most significantly altered genes after the three-weeks dust exposure are shown for both WT and Fat-1 mice in volcano plots on Figures 4A,B as compared to WT saline controls. The overall significant changes among all the groups are summarized with the heatmap in Supplementary Figure S2. Among these genes, several gene sets included in the Immunology panel showed high PC1 scores and a clear clustering of the saline vs. DE-exposed groups regardless of genotype or treatment with TPPU, suggesting that DE exposure is the main driver of the observed changes (Figure 4). Some of the gene sets with high PC1 scores include innate immune system, cell proliferation, transport, wound healing, and collagen. (Figures 4C–G, and Supplementary Figure S3).
FIGURE 4. Changes in gene expression and related pathways altered after three-weeks repetitive dust exposure. Volcano plots showing differential expression of genes in (A) WT and (B) Fat-1 transgenic mice as compared to WT saline controls. Heatmaps were generated for each sample for genes involved in (C) innate immune system, (D) cell proliferation, (E) transport, (F) wound healing and (G) collagen. (H) The top up-regulated gene, NFκBIA in Fat-1 mice as compared to WT mice receiving the repetitive DE exposure. (I) Expression of genes coding for proteins involved in autophosphorylation. n = 3 male mice/group.
To assess the effects of genotype and TPPU treatment on gene expression, we performed additional analyses that allowed us to make direct comparisons between the groups. These included advanced analyses of the raw NanoString data by selecting WT + DE, Fat-1 + DE, WT + DE + TPPU groups as reference instead of the WT saline group as selected in the initial analysis. When WT + DE group served as reference, nSolver software was able to directly compare WT + DE with Fat-1 + DE group. The most significantly altered gene between the two genotypes was NFκBIA, which acts a NFκB inhibitor by binding and confining it to the cytoplasm (Figure 4H). We found a significant interaction between the exposure/treatment and genotype (F = 39.56, p = 0.0068) and close to statistical significance with DE exposure and TPPU treatment (F = 19.86, p = 0.065). The Fat-1 transgenic mice had significantly higher log2 counts of NFκBIA as compared to Fat-1 saline controls (p = 0.0061) and to that of WT mice receiving the repetitive DE exposure (p = 0.025). When looking at the significant unadjusted p values, among the top 20 differentially regulated genes, we found Cd83, Tnfaip3, Il33, Xbp-1, Il4ra, S100a9, S100a8, Cebpb, Csf1, Il1rn, Ptpn2, Il10rb, Jak2, Il13ra1, Tmem173, Fcgr2b, and Ccrl2 to be upregulated in Fat-1 + DE, and Ahr and Cd2 to be down-regulated in Fat-1 + DE as compared to WT + DE groups. With this analysis, we also examined the differentially regulated genes between the WT + DE versus WT + DE + TPPU groups, and we observed only the LCP2 (Lymphocyte cytosolic protein 2) gene to be upregulated based on the significant unadjusted p values. Next, we repeated the analysis selecting Fat-1 + DE group as the reference which allowed us to compare gene expression in Fat-1 + DE group to Fat-1 + DE + TPPU. Based on the unadjusted p values, the top 20 upregulated genes in Fat-1 + DE + TPPU included Cxcr1, Cd2, Prkcd, H2-Dma, Itgb2, Fn1, H2-Eb1, Itgax, Csf1r, C1qb, Itga4, Lcp2, Ly86, Xbp-1, Irf8, Cd48, Npc1, Tlr8, C1qa, and Cd79b. After identifying differentially regulated genes for the hypotheses we wanted to test, we entered these genes into the STRING Database to identify any possible interactions and biological pathways that these cluster of genes would be related to. Results from these analyses are shown on Table 1. As expected, DE exposure was consistent with pathways related to immune cell activation, cell proliferation, neutrophil aggregation, and antigen presentation regardless of mouse genotype. To our surprise, this analysis revealed a role for DE exposure in positively regulating hematopoietic stem cell migration as well as negative regulation of hippocampal neuron apoptotic processes. Interestingly, the main differences between the WT and Fat-1 genotype were associated with response to macrophage colony stimulating factor, immune clearance, and neutrophil aggregation. Upon examining the different gene sets, we found that genes in the protein autophosphorylation pathway show a significant main effect of genotype (Figure 4I). The genes involved in this gene set include Jak 1/2, Irak 1/2, Mapk14, Csf1r, Ptk2, Fyn, Prkcd, and Src. With TPPU treatment, main differences appeared to be in positive regulation of T-cell differentiation in WT mice and positive regulation of PMN activation in Fat-1 mice. Consistent with these changes in upregulated genes, downregulated genes also showed a similar pattern. A list of upregulated and downregulated genes is provided separately in the Supplementary Table S1.
TABLE 1. STRING Database analysis of the differentially regulated genes and their association with biological pathways.
The lungs are continually exposed to harmful stimuli found in the air, including environmental dusts, diesel exhaust particles, and smoke exposures. The ability of the airways to respond to these stimuli and repair damage caused by the exposures is vital to respiratory health, because unrepaired damage can lead to debilitating airway diseases (Ehrlich, 1980; Kirkhorn and Garry, 2000; Smit et al., 2014; Franklin et al., 2015; Nordgren and Bailey, 2016; Watkins et al., 2017). Long-term particulate matter exposures have been consistently linked to negative cardiovascular and lung health outcomes and increased mortality (Franklin et al., 2015; Watkins et al., 2017). Disease susceptibility caused by chronic inhalation of particulates is clearly evidenced by occupational exposures such as those seen in agriculture workers; exposures to livestock farming operations are consistently linked to increased respiratory symptoms and inflammatory lung disease in not only workers, but in individuals living in the surrounding communities, including children and adults (Radon et al., 2007; Pavilonis et al., 2013; Nordgren and Bailey, 2016; van Dijk et al., 2016; Baliatsas et al., 2017; Borlee et al., 2017; Rasmussen et al., 2017; Burkes et al., 2018). Approximately two-thirds of agriculture workers report respiratory disease; 50% of agriculture industry workers experience asthma-like symptoms (American Thoracic, 1998), 25–35% of individuals working in concentrated animal feeding operations experience chronic bronchitis (American Thoracic, 1998), and the prevalence of chronic obstructive pulmonary disease (COPD) among agriculture workers is doubled compared to nonfarming working control subjects (Guillien et al., 2016). Curative options are not available for these workers, with current therapeutic options aimed primarily at symptom management and the prevention of lung disease.
To improve treatment options for this population, studies investigating therapeutic mechanisms to stimulate endogenous lung tissue repair mechanisms are warranted. To this end, we have assessed the impacts of a low (∼1:1) ω-6: ω-3 PUFA total body tissue ratio on lung inflammation following repetitive exposure to inhaled environmental dusts, using a well-described mouse model of DE inhalation. In addition, we have explored the therapeutic utility of an sEH inhibitor, TPPU, in enhancing the impacts of high ω-3 PUFA tissue levels, including exploring its effects in regulating SPM levels during inflammation resolution. Taken together, our results indicate sex-dependent protective effects associated with elevated tissue levels of ω-3 fatty acids in reducing the number of infiltrating immune cells (i.e., neutrophils) into the lung, lower pro-inflammatory cytokine levels and reduced overall histopathology of the lung.
The results identified herein model a long-term dietary intake of high ω-3 PUFA and reduced intake of ω-6 PUFA that achieves an ideal ω-6:ω-3 PUFA ratio throughout the body as reviewed before (Lands et al., 2018). This was achieved through the use of the Fat-1 transgenic mouse model; these mice express the Fat-1 gene from C. elegans encoding an ω-3 FA desaturase, converting ω-6 PUFA to ω-3 PUFA, leading to tissue ω-6:ω-3 PUFA ratios of ∼1:1 (Kang et al., 2004). This model is advantageous because it overcomes several issues that limit diet- and supplementation-based experimental strategies; current clinical and preclinical studies have no standardization in terms of dose, duration, or source and quality of ω-3 PUFA, and each of these factors has implications on outcomes (e.g., due to differences in tissue incorporation, lipid peroxide or aldehyde formation) (Yang et al., 2019). These inconsistencies are considered leading factors for discrepancies in ω-3 PUFA study outcomes (von Schacky, 2014; Calder, 2010; Deckelbaum and Torrejon, 2012). The Fat-1 mouse thus provides a preclinical model for assessing how elevated tissue levels of ω-3 PUFA and reduced ω-6 PUFA can influence health outcomes by minimizing variation based on supplementation, intake and absorption and distribution of ω-3 rich fatty acids. Using this model, we have found genotype and sex-specific differences in infiltrating cells (Figure 1), pro-inflammatory cytokines (Figure 2) and lung histopathology (Figure 3). The decreases in infiltrating PMNs and cytokines were consistent with each other within the Fat-1 male sex. Among the swine farm workers, sex-specific differences in lung function and TLR gene polymorphisms, which is a toll like receptor activated by swine farm dust, have been reported before (Senthilselvan et al., 2009; Gao et al., 2018). Gao et al. found that lung function is worse in males with the TLR9 gene polymorphism (rs187084) as compared to those males without the polymorphism, and females with the TLR2 gene polymorphism (rs4696480) exhibit better functioning lungs as compared to those females without the polymorphism in swine farm full-time workers (Gao et al., 2018). Another study by Senthilselvan et al. found increased plasma levels of TNF-α in males without any TLR4 gene polymorphisms (TLR4 299/399 polymorphism), and in females with the TLR4 gene polymorphism following 5 hours of swine farm exposure in naïve healthy subjects (Senthilselvan et al., 2009). We are not the first ones to report sex-specific differences in Fat-1 mice; a recent study investigating the role of elevated tissue levels of ω-3 fatty acids in obesity-associated post-traumatic osteoarthritis also reported sex-specific differences in Fat-1 transgenic mice (Kimmerling et al., 2020). It appears that such sex-dependent differences are disease model-specific where one sex in the Fat-1 transgenic background exhibits a greater response than the other sex. Another study reported on sex-and age-specific differences of Resolvin D1 (RvD1, an SPM derived from DHA) levels in the retina. This study found sex-dependent differences in retinal levels of RvD1 in aged mice (24-months) as compared to young mice (three-months old), with aged male mice showing a larger decrease in RvD1 levels (Trotta et al., 2021). Sex-dependent changes in the expression of genes involved in fatty acid synthesis, steroids and drug metabolizing enzymes have been identified before (Waxman and Holloway, 2009). Some of those genes encode for enzymes involved in the metabolism of ω-3 and ω-6 PUFA, thus identifying sex-dependent differences can inform pharmacokinetics, bioavailability and treatment options related to ω-3 and ω-6 PUFA-derived lipid mediators. The sEH inhibitor TPPU was used in this study as an indicator that to the inflammation resolving epoxide metabolites of ω-3 lipids might be a partial explanation for their beneficial effect. It is attractive to consider sEH inhibitors or mimics of ω-3 fatty acid epoxides as a prophylactic or therapeutic agent (Falck et al., 2011; Adebesin et al., 2019). Such mimics and sEH inhibitors are in clinical development by several companies but none are available on the market (Lazaar et al., 2016; Hammock et al., 2021). However, there are a number of sEH inhibitors from natural sources that are commercially available such as Maca (also known as Peruvian ginseng, Lepidium meyenii) (Kitamura et al., 2017; Singh et al., 2020).
One of the sex-dependent observations in our study was related to lymphoid aggregate formation in the lung. Male sex in the Fat-1 transgenic mouse had lesser number of aggregates as compared to their corresponding WT controls, which was further reduced in the presence of TPPU (Figures 3B,D). While we observed a similar trend for the female mice these differences did not reach significance. Lymphoid aggregates, which can be composed of T cells, B cells and dendritic cells (van der Strate et al., 2006) play different roles in different disease models such as COPD and tuberculosis. For example, in a chronic cigarette smoke-induced murine COPD model, lymphoid aggregates are associated with adverse outcomes and thus pathological, whereas in a tuberculosis model induced by the Mycobacterium tuberculosis, it is found to be a host defense mechanism (Hertz et al., 2020; Tam et al., 2020). To our knowledge sex-differences in lymphoid aggregate formation in the lung has not been fully explored. A recent study found that females are more susceptible to lymphoid aggregate formation as compared to males, and this was further confirmed by ovariectomy in a smoke-induced COPD model (Tam et al., 2020). This is consistent with the previous reports indicating that women are more susceptible to develop COPD than men (Martinez et al., 2007; de Torres et al., 2009; Perez et al., 2020). Overall, our results are consistent with the previously published literature on the effects of agricultural dust in males; and the improved histopathological effects seen in the male sex are novel.
We observed an elevated alveolar cellularity (approximately two-fold), in wild-type female mice as compared to male mice. A study investigated the differences in alveolar macrophage proteome between males and females, and found several proteins associated with inflammation and interact with estrogen receptor to be expressed higher in females than males (Phelps et al., 2012). Another study looking at sex-related differences in lung inflammation showed a higher baseline for lung histology score and number of infiltrating cells into the lungs in females compared to males in sham-operated controls (Vegeto et al., 2010). Both studies are consistent with the histological finding we observed in female wild-type mouse lungs. Because males responded TPPU treatment better, this might be related to differences in fatty acid metabolism between males and females given fatty acid epoxides regulate alveolar cell influx, as shown in a study demonstrating that pharmacological modulation of fatty acid epoxides affect inflammatory cell influx to the lungs (Yang et al., 2015). In addition, SPMs also modulate macrophage chemotaxis, trans-endothelial migration and cytokine release from macrophages as reviewed by Haworth and Levy (2007). More studies are needed to dissect differences in fatty acid metabolism between males and females.
It is well accepted that sex-specific differences that result in different biological responses between males and females stem from sex hormones. These differences affect storage and distribution of lipids thereby affect free fatty acid availability between sexes. Stable isotope studies report that ARA and DHA contribute more to blood lipids in women than in men and that there are differences in the conversion rate of fatty acids, for example conversion of ALA to omega-3 fatty acids is higher in women than in men (Decsi and Kennedy, 2011). Also, preclinical studies show that reproductive hormones affect the enzymes involved in the biosynthesis of fatty acids. Another study found that sex-related differences in COPD might be related in part to the increased production of leukotoxin-diol (linoleic acid derived diol) by goblet cell P450 and sEH activities (Balgoma et al., 2016). Overall, our results suggest a mechanism that 1) availability of free fatty acids from the elevated tissue levels of ω-3 acids in Fat-1 mice are sex-dependent and 2) females have different conversion rates of fatty acids as compared to males and this might lead to changes in gene expression of chemokines and cytokines, thereby affecting alveolar macrophage recruitment into the lung and thus creating sex-dependent host defense mechanism within the Fat-1 genotype.
In addition to dietary strategies aimed at promoting the healthful benefits of ω-3 PUFA, therapeutic strategies leveraging the endogenous repair SPM pathways to promote inflammation resolution and repair hold great clinical promise. For example, many investigations have identified positive outcomes in ameliorating lung inflammation/disease via therapeutic administration of SPM (Croasdell et al., 19502016; Seki et al., 19502010; Aoki et al., 2008; Haworth et al., 2011; Hsiao et al., 2013; Miyata and Arita, 2015; Kim et al., 2016), including our own previous studies identifying beneficial effects of the DHA-derived SPM maresin-1 in reducing the lung inflammatory effects of acute and repetitive organic dust exposure (Nordgren et al., 2013; Nordgren et al., 2015). While SPM can potently inhibit inflammation while promoting tissue repair, these bioactive metabolites are quickly deactivated by subsequent metabolism (Lopez-Vicario et al., 2015; Basil and Levy, 2016). Thus, another therapeutic strategy to leverage these endogenous inflammation resolution pathways is to combine ω-3 PUFA supplementation with pharmacologic inhibition of enzymes responsible for the deactivation of SPM. One such strategy has been the use of inhibitors of the sEH enzyme to prevent the deactivation of the cytochrome P450 family of SPM, thereby potentiating their protective effects (Guedes et al., 2013; Ulu et al., 2013; Ulu et al., 2014; Yang et al., 2015; Ulu et al., 2016; Zhou et al., 2016; Goswami et al., 2017; Zhou et al., 2017; Yang et al., 2020; Zhang et al., 2020). Previous studies have found enhanced protective effects of ω-3 PUFA when used in combination with inhibitors of sEH such as TPPU (Wang et al., 2012; Lopez-Vicario et al., 2015; Yang et al., 2015; Zhou et al., 2016; Zhou et al., 2017). This enzyme deactivates the epoxide SPM (e.g., 19, 20-EDP; produced through CYP450 metabolism) into less active diol forms (e.g., 19 (20)-DiHDPA). A recent study in a model of metabolic disease identified that a sEH inhibitor enhanced the protective effects identified in Fat-1 mice vs. WT mice (Lopez-Vicario et al., 2015), while previous studies also identify beneficial effects of sEH inhibition in murine models of acute lung injury (Zhou et al., 2017), pulmonary fibrosis (Zhou et al., 2016), asthma (Yang et al., 2015), and COPD (Wang et al., 2012). Corroborating these previous reports, when we utilized TPPU in the experiments described herein, we found that addition of TPPU lowered all outcomes examined in the DE-exposed animals, suggesting that TPPU not only enhanced the effects of ω-3 fatty acids as in Fat-1 + DE + TPPU animals, but also showed efficacy independent of the Fat-1 genotype. In addition, we observed a better response in the Fat-1 male sex receiving the TPPU treatment and three-weeks DE exposure. Consistent with our results sex-specific differences have been reported in sEH null mice and sEH activity in other mouse disease models (Vanella et al., 2015; Wagner et al., 2017; Jamieson et al., 2020).
While our results support a beneficial effect of maintaining a low omega6:omega3 ratio in response to agricultural dust, omega-6 PUFAs and their metabolites also modulate inflammation and participate in inflammation resolution and tissue homeostasis. The SPMs derived from arachidonic acid, such as LXA4 and metabolites generated by the P450 pathway (i.e., EETs or epoxyeicosatrienoic acids) have been repeatedly shown to have anti-inflammatory effects (Levy and Serhan, 2003; Morisseau and Hammock, 2013). Most surprisingly, the ARA metabolites generated by the COX-2 pathway, such as PGE2 have protective effects in the lung despite their infamous proinflammatory notion, as shown in asthma and allergic airway inflammation (Vancheri et al., 2004; Herrerias et al., 2009). Airway epithelial cells are the major source of PGE2 production in the lung, and PGE2 protects against airway hyperresponsiveness to allergens by inhibiting leukotriene and thromboxane synthesis that cause bronchoconstriction and by reducing eosinophil recruitment, both of which are anti-inflammatory effects of PGE2. Similarly, in asthma, it has been shown that the homeostatic balance between the COX and LOX pathways metabolizing ARA are altered due to damaged epithelium in asthmatic airways. Given this, it has been proposed that the dysregulation of these pathways leads to an imbalance between PGE2 and PGD2/LT which is in part responsible for increased bronchoconstriction. Other roles attributed to PGE2 in the lung include inflammatory cell recruitment, eosinophil degranulation, bronchodilation, T-cell recruitment and differentiation and adhesion molecule expression as reviewed before (Vancheri et al., 2004).
With regards to sex-related differences in response to environmental stimuli, a study examined changes in gene expression in the lung associated with inflammation and immunity after ozone exposure (Cabello et al., 2015). This study found increased lung histological scores and increased infiltrating PMN in the female sex as compared to males after ozone exposure. Among control mice exposed to filtered air alone, females displayed about 5% difference in gene expression of genes related to chemokines and cytokines as compared to males. These genes included Cxcl2 and Ccl19 (involved in activation of macrophages), Myd88 (involved in TLR activation), and C4b (associated with IL-6 response), all of which were associated with immune cell adhesion and recruitment. Considering the sex- and genotype-dependent differences in our model, we examined changes in gene expression as well. Since the most significant changes we observed in our model was in the male sex (as in Fat-1 mice with TPPU), we focused our gene expression studies to male sex. NanoString gene expression analysis identified differentially regulated pathways consistent with our previous results (Dominguez et al., 2020; Ulu et al., 2021b) indicating that immune cell activation, cell proliferation, wound healing and transport pathways were altered following 3-weeks DE exposure. In an In-depth analysis both using STRING database protein-protein interaction and NanoString advanced analyses, we also identified changes in NFκBIA, response to macrophage colony stimulating factor, immune clearance, and neutrophil aggregation between the two genotypes (Figure 4). In addition, TPPU treatment affected distinct cellular processes such as T-cell differentiation in WT mice and regulation of neutrophil activation in the Fat-1 genotype. This observed effect of TPPU is consistent with a previous report showing modulation of the Th1/Th17 response while elevating regulatory T-cells by TPPU in an arthritis model (Trindade-da-Silva et al., 2020). Evaluation of both upregulated and downregulated genes among the experimental groups were in the direction of inflammation resolution. For example, in the Fat-1 genotype the observed differences in the downregulated genes were associated with cytokine production in macrophages, regulation of tight junctions and ECM disassembly, suggesting a move towards resolution of inflammation in tissue (Table 1). Cytokine production and tight junctions contribute significantly to the maintenance of epithelial cell integrity and mucosal immunity of the lung in response to environmental insults as well as pathogens (Kyd et al., 2001; Brune et al., 2015). Similarly, changes in ECM are important for recruitment of immune cells into and within the lung (Wight et al., 2017). Altogether, changes in these processes promoted by elevated levels of ω-3 fatty acids as in Fat-1 transgenic mice might affect how the lung responds to DE exposure. Particularly with TPPU, we identified downregulation of genes involved in IL-33 mediated signaling, as well as IL-5, IL-13, and IL-6 secretion. Overall, these data identified differences in immune clearance between the two genotypes and TPPU appeared to contribute to T-cell differentiation and regulation of PMN activation in part by downregulating the IL-33-mediated signaling pathway. IL-33 has previously been reported as an important regulator of Th-2 immune response in allergic inflammation (Chan et al., 2019).
The studies described herein do have numerous limitations. As with all transgenic animal models, the use of the Fat-1 mice to increase total body tissue levels of ω-3 PUFA and achieve an ideal ∼1:1 ratio of ω-6:ω-3 PUFA does not fully recapitulate the human condition. For example, differences in PUFA intakes are seen not only between individuals, but temporal fluctuations in diet tendencies for each individual also undoubtedly alter daily to monthly PUFA levels, and the impacts of this will vary across different tissues based on PUFA uptake kinetics (Katan et al., 1997; Raphael and Sordillo, 2013; Gurzell et al., 2014). As PUFA substrate utilized during inflammatory events likely comes from both tissue sources as well as circulating blood and associated inflammatory cell infiltration, the impacts of recent dietary intake versus long-term dietary patterns on PUFA substrate availability are difficult to ascertain. Also, there are numerous recognized health benefits of diets high in ω-3 PUFA, due at least partly to their role in the endogenous production of SPM that regulate inflammation resolution and repair activities (Titos et al., 19502011; Schwab et al., 2007; Serhan et al., 2009; Levy, 2010; Serhan, 2014). SPM are produced temporally during an inflammatory response; their levels increase within hours to days following an inflammatory insult, and their production is critical to inflammation catabasis, including promoting neutrophil clearance, reducing inflammatory cytokine production, activating M2-like pro-resolution macrophages, promoting regulatory T cell recruitment, and activating tissue repair (Widgerow, 2012; Basil and Levy, 2016). Deficiencies in SPM generation pathways have been identified in asthma, and SPM are decreased in lavage, sputum, and/or exhaled breath condensates of COPD and asthma patients compared to individuals without lung disease (Levy et al., 2007; Bhavsar et al., 2010; Croasdell et al., 2015). Therefore, an in-depth analysis of both ω -3 and ω -6 PUFA derived SPMs is necessary, and lipid metabolomics analyses of our data are currently underway in our laboratory.
Another limitation is that we have used a model of organic dust exposure that utilizes a sterile-filtered aqueous extract of environmental dusts that is intranasally instilled to mice in a saline solution. This model will not fully recapitulate the inhalant injury that is experienced by an individual working in a swine confinement facility, as it does not consider certain components, including live pathogens or gaseous components that have recognized respiratory impacts in these workers (Von Essen and Donham, 1999; Kirkhorn and Garry, 2000; Charavaryamath et al., 2005; Charavaryamath et al., 2008; Langley, 2011; May et al., 2012; Madsen et al., 2015; Nordgren and Bailey, 2016; Schneberger et al., 2017; Nordgren and Charavaryamath, 2018; Schneberger et al., 2021). It has been reported that airway inflammation in swine confinement workers differ from naïve subjects even after repetitive exposures, one displaying neutrophilic airway inflammation and the other neutrophilic and eosinophilic inflammation, respectively (Larsson et al., 1994; Von Essen and Romberger, 2003). We have characterized a number of components that are likely to be involved in neutrophilic inflammation. Proteases are one of the components of agricultural dust that has been shown to be in part responsible for this type of inflammation (Von Essen and Romberger, 2003; Romberger et al., 2015). Our results are consistent with neutrophilic inflammation observed in people.
In addition, since we delivered agricultural dust extract under light isoflurane anesthesia, the previously reported sex-related differences of isoflurane might confound some of the sex-related effects we observed in our study; however, given all the mice in each group underwent this light anesthesia the significant differences between the groups would still stand.
Compelling data indicate the importance of having a balanced ω-6:ω-3 PUFA ratio for optimal health (∼1:1 is considered ideal), yet the typical Western diet has a ratio of ∼10–20:1 (Simopoulos, 2008; Patterson et al., 2012). This imbalance is considered a driving or compounding factor in chronic inflammatory diseases, with many pro-inflammatory lipids formed from the ω-6 PUFA arachidonic acid, such as leukotrienes, thromboxanes and prostaglandins (Calder, 2006; Calder, 2010; Patterson et al., 2012; Calder, 2013; Barden et al., 2016). It was recently reported that Veterans with COPD and an occupational history that include agricultural work had an ω-6:ω-3 PUFA ratio of ∼50:1 (Hanson et al., 2017), underscoring the potential vulnerability of this population. Supplementation with ω-3 PUFA has demonstrated health benefits in clinical studies of cystic fibrosis, COPD, and other lung diseases (Strasser et al., 1985; Pontes-Arruda et al., 2008; Giudetti and Cagnazzo, 2012; Oliver and Watson, 2013). Thus, increasing ω-3 FA intake, possibly in combination with a reduced intake of ω-6 PUFA, could be an accessible and effective means of preventing and ameliorating airway disease in patients with inflammatory lung diseases such as those caused by agricultural dust exposures. Taken together, our investigations utilizing the Fat-1 mouse model in conjunction with sEH inhibition highlight the potentials of targeting repair/resolution pathways therapeutically to promote lung protection from environmental dust exposures, and also highlight differences based on sex in the protectiveness offered by these interventions. In the case of agriculture workers who are chronically inhaling inflammatory dusts, these outcomes hold promise for improving lung health outcomes via both limiting lung inflammation but also promoting repair following injury in this vulnerable population.
The original contributions presented in the study are included in the article/Supplementary Material, further inquiries can be directed to the corresponding author.
The animal study was reviewed and approved by the Institutional Animal Care and Use Committee of the University of California, Riverside.
TN, AU, and BH conceptually contributed and designed experiments; AU, JV, AB, SS, JY, and CB collected samples, acquired, and analyzed data; AU and TN drafted the manuscript; AU, JV, AB, SS, JY, CB, BH, and TN reviewed and edited the manuscript.
This work was supported in part by the National Institute of Environmental Health Sciences (R00ES025819 to TN), the National Heart, Lung, and Blood Institute (R01HL158926 to TN), and with partial support from NIH/NIEHS (RIVER Award, R35 ES030443-01 to BH) and NIH/NIEHS (Superfund Award, P42 ES004699 to BH).
The authors declare that the research was conducted in the absence of any commercial or financial relationships that could be construed as a potential conflict of interest.
All claims expressed in this article are solely those of the authors and do not necessarily represent those of their affiliated organizations, or those of the publisher, the editors and the reviewers. Any product that may be evaluated in this article, or claim that may be made by its manufacturer, is not guaranteed or endorsed by the publisher.
Authors gratefully acknowledge Debra Romberger at the University of Nebraska Medical Center for the kind gift of the swine confinement facility dusts used for these investigations.
The Supplementary Material for this article can be found online at: https://www.frontiersin.org/articles/10.3389/fphar.2021.785193/full#supplementary-material
Adebesin, A. M., Wesser, T., Vijaykumar, J., Konkel, A., Paudyal, M. P., Lossie, J., et al. (2019). Development of Robust 17. J. Med. Chem. 62 (22), 10124–10143. doi:10.1021/acs.jmedchem.9b00952
American Thoracic, S. (1998). Respiratory Health Hazards in Agriculture. Am. J. Respir. Crit. Care Med. 158 (5 Pt 2), S1–S76. doi:10.1164/ajrccm.158.supplement_1.rccm1585s1
Aoki, H., Hisada, T., Ishizuka, T., Utsugi, M., Kawata, T., Shimizu, Y., et al. (2008). Resolvin E1 Dampens Airway Inflammation and Hyperresponsiveness in a Murine Model of Asthma. Biochem. biophysical Res. Commun. 367 (2), 509–515. doi:10.1016/j.bbrc.2008.01.012
Balgoma, D., Yang, M., Sjödin, M., Snowden, S., Karimi, R., Levänen, B., et al. (2016). Linoleic Acid-Derived Lipid Mediators Increase in a Female-Dominated Subphenotype of COPD. Eur. Respir. J. 47 (6), 1645–1656. doi:10.1183/13993003.01080-2015
Baliatsas, C., Borlee, F., van Dijk, C. E., van der Star, B., Zock, J. P., Smit, L. A. M., et al. (2017). Comorbidity and Coexisting Symptoms and Infections Presented in General Practice by COPD Patients: Does Livestock Density in the Residential Environment Play a Role? Int. J. Hyg. Environ. Health 220 (4), 704–710. doi:10.1016/j.ijheh.2017.02.005
Barden, A. E., Mas, E., and Mori, T. A. (2016). n-3 Fatty Acid Supplementation and Proresolving Mediators of Inflammation. Curr. Opin. Lipidol. 27 (1), 26–32. doi:10.1097/MOL.0000000000000262
Basil, M. C., and Levy, B. D. (2016). Specialized Pro-resolving Mediators: Endogenous Regulators of Infection and Inflammation. Nat. reviewsImmunology 16 (1), 51–67. doi:10.1038/nri.2015.4
Bhavsar, P. K., Levy, B. D., Hew, M. J., Pfeffer, M. A., Kazani, S., Israel, E., et al. (2010). Corticosteroid Suppression of Lipoxin A4 and Leukotriene B4from Alveolar Macrophages in Severe Asthma. Respir. Res. 11, 71. doi:10.1186/1465-9921-11-71
Bilal, S., Haworth, O., Wu, L., Weylandt, K. H., Levy, B. D., and Kang, J. X. (2011). Fat-1 Transgenic Mice with Elevated omega-3 Fatty Acids Are Protected from Allergic Airway Responses. Biochim. Biophys. Acta 1812 (9), 1164–1169. doi:10.1016/j.bbadis.2011.05.002
Boissy, R. J., Romberger, D. J., Roughead, W. A., Weissenburger-Moser, L., Poole, J. A., and LeVan, T. D. (2014). Shotgun Pyrosequencing Metagenomic Analyses of Dusts from Swine Confinement and Grain Facilities. PLoS One 9 (4), e95578. doi:10.1371/journal.pone.0095578
Borlee, F., Yzermans, C. J., Aalders, B., Rooijackers, J., Krop, E., Maassen, C. B. M., et al. (2017). Air Pollution from Livestock Farms Is Associated with Airway Obstruction in Neighboring Residents. Am. J. Respir. Crit. Care Med. 196 (9), 1152–1161. doi:10.1164/rccm.201701-0021OC
Bradberry, J. C., and Hilleman, D. E. (2013). Overview of omega-3 Fatty Acid Therapies. P T 38 (11), 681–691.
Brune, K., Frank, J., Schwingshackl, A., Finigan, J., and Sidhaye, V. K. (2015). Pulmonary Epithelial Barrier Function: Some New Players and Mechanisms. Am. J. Physiol. Lung Cel Mol Physiol 308 (8), L731–L745. doi:10.1152/ajplung.00309.2014
Burkes, R. M., Gassett, A. J., Ceppe, A. S., Anderson, W., O'Neal, W. K., Woodruff, P. G., et al. (2018). Rural Residence and COPD Exacerbations: Analysis of the SPIROMICS Cohort. Ann. Am. Thorac. Soc. 15, 808–816. doi:10.1513/AnnalsATS.201710-837OC
Cabello, N., Mishra, V., Sinha, U., DiAngelo, S. L., Chroneos, Z. C., Ekpa, N. A., et al. (2015). Sex Differences in the Expression of Lung Inflammatory Mediators in Response to Ozone. Am. J. Physiol. Lung Cel Mol Physiol 309 (10), L1150–L1163. doi:10.1152/ajplung.00018.2015
Calder, P. C. (2013). N-3 Fatty Acids, Inflammation and Immunity: New Mechanisms to Explain Old Actions. Proc. Nutr. Soc. 72 (3), 326–336. doi:10.1017/S0029665113001031
Calder, P. C. (2006). N-3 Polyunsaturated Fatty Acids, Inflammation, and Inflammatory Diseases. Am. J. Clin. Nutr. 83 (6 Suppl. l), 1505S–19S. doi:10.1093/ajcn/83.6.1505S
Calder, P. C. (2010). Omega-3 Fatty Acids and Inflammatory Processes. Nutrients 2 (3), 355–374. doi:10.3390/nu2030355
Calder, P. C., Waitzberg, D. L., Klek, S., and Martindale, R. G. (2020). Lipids in Parenteral Nutrition: Biological Aspects. JPEN J. Parenter. Enteral Nutr. 44 (Suppl. 1), S21–S7. doi:10.1002/jpen.1756
Cdc, (2019). CDC - Asthma - Most Recent Asthma Data. Available at: https://www.cdc.gov/asthma/most_recent_national_asthma_data.htm2018.
Chan, B. C. L., Lam, C. W. K., Tam, L. S., and Wong, C. K. (2019). IL33: Roles in Allergic Inflammation and Therapeutic Perspectives. Front. Immunol. 10, 364. doi:10.3389/fimmu.2019.00364
Charavaryamath, C., Janardhan, K. S., Townsend, H. G., Willson, P., and Singh, B. (2005). Multiple Exposures to Swine Barn Air Induce Lung Inflammation and Airway Hyper-Responsiveness. Respir. Res. 6, 50. doi:10.1186/1465-9921-6-50
Charavaryamath, C., Juneau, V., Suri, S. S., Janardhan, K. S., Townsend, H., and Singh, B. (2008). Role of Toll-like Receptor 4 in Lung Inflammation Following Exposure to Swine Barn Air. Exp. Lung Res. 34 (1), 19–35. doi:10.1080/01902140701807779
Croasdell, A., Lacy, S. H., Thatcher, T. H., Sime, P. J., and Phipps, R. P. (19502016). Resolvin D1 Dampens Pulmonary Inflammation and Promotes Clearance of Nontypeable Haemophilus Influenzae. J. Immunol. (Baltimore, Md 196 (6), 2742–2752. doi:10.4049/jimmunol.1502331
Croasdell, A., Thatcher, T. H., Kottmann, R. M., Colas, R. A., Dalli, J., Serhan, C. N., et al. (2015). Resolvins Attenuate Inflammation and Promote Resolution in Cigarette Smoke-Exposed Human Macrophages. Am. J. physiologyLung Cell. Mol. Physiol. 309 (8), L888–L901. doi:10.1152/ajplung.00125.2015
de Torres, J. P., Cote, C. G., López, M. V., Casanova, C., Díaz, O., Marin, J. M., et al. (2009). Sex Differences in Mortality in Patients with COPD. Eur. Respir. J. 33 (3), 528–535. doi:10.1183/09031936.00096108
Deckelbaum, R. J., and Torrejon, C. (2012). The omega-3 Fatty Acid Nutritional Landscape: Health Benefits and Sources. J. Nutr. 142 (3), 587S–91S. doi:10.3945/jn.111.148080
Decsi, T., and Kennedy, K. (2011). Sex-specific Differences in Essential Fatty Acid Metabolism. Am. J. Clin. Nutr. 94 (6 Suppl. l), 1914S–9S. doi:10.3945/ajcn.110.000893
Demanche, A., Bonlokke, J., Beaulieu, M. J., Assayag, E., and Cormier, Y. (2009). Swine Confinement Buildings: Effects of Airborne Particles and Settled Dust on Airway Smooth Muscles. Ann. Agric. Environ. Med. 16 (2), 233–238.
Dileepan, M., Rastle-Simpson, S., Greenberg, Y., Wijesinghe, D. S., Kumar, N. G., Yang, J., et al. (2019). Effect of Dual sEH/COX-2 Inhibition on Allergen-Induced Airway Inflammation. Front. Pharmacol. 10, 1118. doi:10.3389/fphar.2019.01118
Dominguez, E. C., Heires, A. J., Pavlik, J., Larsen, T. D., Guardado, S., Sisson, J. H., et al. (2020). A High Docosahexaenoic Acid Diet Alters the Lung Inflammatory Response to Acute Dust Exposure. Nutrients 12 (8). doi:10.3390/nu12082334
Ehrlich, R. (1980). Interaction between Environmental Pollutants and Respiratory Infections. Environ. Health Perspect. 35, 89–99. doi:10.1289/ehp.803589
Falck, J. R., Wallukat, G., Puli, N., Goli, M., Arnold, C., Konkel, A., et al. (2011). 17(R),18(S)-epoxyeicosatetraenoic Acid, a Potent Eicosapentaenoic Acid (EPA) Derived Regulator of Cardiomyocyte Contraction: Structure-Activity Relationships and Stable Analogues. J. Med. Chem. 54 (12), 4109–4118. doi:10.1021/jm200132q
Fix, J., Annesi-Maesano, I., Baldi, I., Boulanger, M., Cheng, S., Cortes, S., et al. (2020). Gender Differences in Respiratory Health Outcomes Among Farming Cohorts Around the globe: Findings from the AGRICOH Consortium. J. Agromedicine 26, 97–108. doi:10.1080/1059924X.2020.1713274
Franklin, B. A., Brook, R., and Arden Pope, C. (2015). Air Pollution and Cardiovascular Disease. Curr. Probl. Cardiol. 40 (5), 207–238. doi:10.1016/j.cpcardiol.2015.01.003
Gao, Z., Dosman, J. A., Rennie, D. C., Schwartz, D. A., Yang, I. V., Beach, J., et al. (2018). Gender-specific Associations between Polymorphisms in the Toll-like Receptor (TLR) Genes and Lung Function Among Workers in Swine Operations. J. Toxicol. Environ. Health A. 81 (22), 1186–1198. doi:10.1080/15287394.2018.1544523
Giudetti, A. M., and Cagnazzo, R. (2012). Beneficial Effects of N-3 PUFA on Chronic Airway Inflammatory Diseases. Prostaglandins & other lipid mediators 99 (3-4), 57–67. doi:10.1016/j.prostaglandins.2012.09.006
Goswami, S. K., Rand, A. A., Wan, D., Yang, J., Inceoglu, B., Thomas, M., et al. (2017). Pharmacological Inhibition of Soluble Epoxide Hydrolase or Genetic Deletion Reduces Diclofenac-Induced Gastric Ulcers. Life Sci. 180, 114–122. doi:10.1016/j.lfs.2017.05.018
Guedes, A. G., Morisseau, C., Sole, A., Soares, J. H., Ulu, A., Dong, H., et al. (2013). Use of a Soluble Epoxide Hydrolase Inhibitor as an Adjunctive Analgesic in a Horse with Laminitis. Vet. Anaesth. Analg 40 (4), 440–448. doi:10.1111/vaa.12030
Guillien, A., Puyraveau, M., Soumagne, T., Guillot, S., Rannou, F., Marquette, D., et al. (2016). Prevalence and Risk Factors for COPD in Farmers: a Cross-Sectional Controlled Study. Eur. Respir. J. 47 (1), 95–103. doi:10.1183/13993003.00153-2015
Gurzell, E. A., Wiesinger, J. A., Morkam, C., Hemmrich, S., Harris, W. S., and Fenton, J. I. (2014). Is the omega-3 index a Valid Marker of Intestinal Membrane Phospholipid EPA+DHA Content? Prostaglandins, Leukotrienes, and Essential Fatty Acids. Prostaglandins Leukot. Essent. Fatty Acids 91 (3), 87–96. doi:10.1016/j.plefa.2014.04.001
Hammock, B. D., McReynolds, C. B., Wagner, K., Buckpitt, A., Cortes-Puch, I., Croston, G., et al. (2021). Movement to the Clinic of Soluble Epoxide Hydrolase Inhibitor EC5026 as an Analgesic for Neuropathic Pain and for Use as a Nonaddictive Opioid Alternative. J. Med. Chem. 64 (4), 1856–1872. doi:10.1021/acs.jmedchem.0c01886
Hammock, B. D., Wang, W., Gilligan, M. M., and Panigrahy, D. (2020). Eicosanoids: The Overlooked Storm in Coronavirus Disease 2019 (COVID-19)? Am. J. Pathol. 190, 1782–1788. doi:10.1016/j.ajpath.2020.06.010
Hanson, C., Lyden, E., Weissenburger-Moser, L., Furtado, J., Hinds, J., and LeVan, T. (2017). Serum Level of Nutritional Antioxidants Are Decreased in Veteran Smokers with COPD. Mil. Veterans Health 25, 9.
Haworth, O., Cernadas, M., and Levy, B. D. (2011). NK Cells Are Effectors for Resolvin E1 in the Timely Resolution of Allergic Airway Inflammation. J. Immunol. (Baltimore, Md: 1950) 186 (11), 6129–6135. doi:10.4049/jimmunol.1004007
Haworth, O., and Levy, B. D. (2007). Endogenous Lipid Mediators in the Resolution of Airway Inflammation. Eur. Respir. J. 30 (5), 980–992. doi:10.1183/09031936.00005807
Herrerias, A., Torres, R., Serra, M., Marco, A., Pujols, L., Picado, C., et al. (2009). Activity of the Cyclooxygenase 2-Prostaglandin-E Prostanoid Receptor Pathway in Mice Exposed to House Dust Mite Aeroallergens, and Impact of Exogenous Prostaglandin E2. J. Inflamm. (Lond). 6, 30. doi:10.1186/1476-9255-6-30
Hertz, D., Dibbern, J., Eggers, L., von Borstel, L., and Schneider, B. E. (2020). Increased Male Susceptibility to Mycobacterium tuberculosis Infection Is Associated with Smaller B Cell Follicles in the Lungs. Sci. Rep. 10 (1), 5142. doi:10.1038/s41598-020-61503-3
Hoppin, J. A., Umbach, D. M., Long, S., Rinsky, J. L., Henneberger, P. K., Salo, P. M., et al. (2014). Respiratory Disease in United States Farmers. Occup. Environ. Med. 71 (7), 484–491. doi:10.1136/oemed-2013-101983
Hsiao, H. M., Sapinoro, R. E., Thatcher, T. H., Croasdell, A., Levy, E. P., Fulton, R. A., et al. (2013). A Novel Anti-inflammatory and Pro-resolving Role for Resolvin D1 in Acute Cigarette Smoke-Induced Lung Inflammation. PloS one 8 (3), e58258. doi:10.1371/journal.pone.0058258
Iversen, M., and Dahl, R. (2000). Working in Swine-Confinement Buildings Causes an Accelerated Decline in FEV1: a 7-yr Follow-Up of Danish Farmers. Eur. Respir. J. : official J. Eur. Soc. Clin. Respir. Physiol. 16 (3), 404–408. doi:10.1034/j.1399-3003.2000.016003404.x
Jamieson, K. L., Keshavarz-Bahaghighat, H., Darwesh, A. M., Sosnowski, D. K., and Seubert, J. M. (2020). Age and Sex Differences in Hearts of Soluble Epoxide Hydrolase Null Mice. Front. Physiol. 11, 48. doi:10.3389/fphys.2020.00048
Kachan, D., Fleming, L. E., LeBlanc, W. G., Goodman, E., Arheart, K. L., Caban-Martinez, A. J., et al. (2012). Worker Populations at Risk for Work-Related Injuries across the Life Course. Am. J. Ind. Med. 55 (4), 361–366. doi:10.1002/ajim.21994
Kang, J. X., Wang, J., Wu, L., and Kang, Z. B. (2004). Transgenic Mice: Fat-1 Mice Convert N-6 to N-3 Fatty Acids. Nature 427 (6974), 504. doi:10.1038/427504a
Katan, M. B., Deslypere, J. P., van Birgelen, A. P., Penders, M., and Zegwaard, M. (1997). Kinetics of the Incorporation of Dietary Fatty Acids into Serum Cholesteryl Esters, Erythrocyte Membranes, and Adipose Tissue: an 18-month Controlled Study. J. lipid Res. 38 (10), 2012–2022. doi:10.1016/s0022-2275(20)37132-7
Kim, K. H., Park, T. S., Kim, Y. S., Lee, J. S., Oh, Y. M., Lee, S. D., et al. (2016). Resolvin D1 Prevents Smoking-Induced Emphysema and Promotes Lung Tissue Regeneration. Int. J. Chron. Obstruct Pulmon Dis. 11, 1119–1128. doi:10.2147/COPD.S100198
Kimmerling, K. A., Oswald, S. J., Huebner, J. L., Little, D., Kraus, V. B., Kang, J. X., et al. (2020). Transgenic Conversion of ω-6 to ω-3 Polyunsaturated Fatty Acids via Fat-1 Reduces the Severity of post-traumatic Osteoarthritis. Arthritis Res. Ther. 22 (1), 83. doi:10.1186/s13075-020-02170-7
Kirkhorn, S. R., and Garry, V. F. (2000). Agricultural Lung Diseases. Environ. Health Perspect. 108 (Suppl. 4), 705–712. doi:10.1289/ehp.00108s4705
Kitamura, S., Morisseau, C., Harris, T. R., Inceoglu, B., and Hammock, B. D. (2017). Occurrence of Urea-Based Soluble Epoxide Hydrolase Inhibitors from the Plants in the Order Brassicales. PLoS One 12 (5), e0176571. doi:10.1371/journal.pone.0176571
Kuo, Y. M., Hsu, P. C., Hung, C. C., Hu, Y. Y., Huang, Y. J., Gan, Y. L., et al. (2019). Soluble Epoxide Hydrolase Inhibition Attenuates Excitotoxicity Involving 14,15-Epoxyeicosatrienoic Acid-Mediated Astrocytic Survival and Plasticity to Preserve Glutamate Homeostasis. Mol. Neurobiol. 56 (12), 8451–8474. doi:10.1007/s12035-019-01669-8
Kyd, J. M., Foxwell, A. R., and Cripps, A. W. (2001). Mucosal Immunity in the Lung and Upper Airway. Vaccine 19 (17-19), 2527–2533. doi:10.1016/s0264-410x(00)00484-9
Lands, B., Bibus, D., and Stark, K. D. (2018). Dynamic Interactions of N-3 and N-6 Fatty Acid Nutrients. Prostaglandins Leukot. Essent. Fatty Acids 136, 15–21. doi:10.1016/j.plefa.2017.01.012
Langley, R. L. (2011). Consequences of Respiratory Exposures in the Farm Environment. North Carolina Med. J. 72 (6), 477–480. doi:10.18043/ncm.72.6.477
Larsson, K. A., Eklund, A. G., Hansson, L. O., Isaksson, B. M., and Malmberg, P. O. (1994). Swine Dust Causes Intense Airways Inflammation in Healthy Subjects. Am. J. Respir. Crit. Care Med. 150 (4), 973–977. doi:10.1164/ajrccm.150.4.7921472
Lazaar, A. L., Yang, L., Boardley, R. L., Goyal, N. S., Robertson, J., Baldwin, S. J., et al. (2016). Pharmacokinetics, Pharmacodynamics and Adverse Event Profile of GSK2256294, a Novel Soluble Epoxide Hydrolase Inhibitor. Br. J. Clin. Pharmacol. 81 (5), 971–979. doi:10.1111/bcp.12855
Levy, B. D., Kohli, P., Gotlinger, K., Haworth, O., Hong, S., Kazani, S., et al. (2007). Protectin D1 Is Generated in Asthma and Dampens Airway Inflammation and Hyperresponsiveness. J. Immunol. 178 (1), 496–502. doi:10.4049/jimmunol.178.1.496
Levy, B. D. (2010). Resolvins and Protectins: Natural Pharmacophores for Resolution Biology. Prostaglandins, Leukot. Essent. fatty Acids 82 (4-6), 327–332. doi:10.1016/j.plefa.2010.02.003
Levy, B. D., and Serhan, C. N. (2003). Exploring New Approaches to the Treatment of Asthma: Potential Roles for Lipoxins and Aspirin-Triggered Lipid Mediators. Drugs Today (Barc) 39 (5), 373–384. doi:10.1358/dot.2003.39.5.740217
Levy, B. D., and Serhan, C. N. (2014). Resolution of Acute Inflammation in the Lung. Annu. Rev. Physiol. 76, 467–492. doi:10.1146/annurev-physiol-021113-170408
Lopez-Vicario, C., Alcaraz-Quiles, J., Garcia-Alonso, V., Rius, B., Hwang, S. H., Titos, E., et al. (2015). Inhibition of Soluble Epoxide Hydrolase Modulates Inflammation and Autophagy in Obese Adipose Tissue and Liver: Role for omega-3 Epoxides. Proc. Natl. Acad. Sci. U S A. 112 (2), 536–541. doi:10.1073/pnas.1422590112
Madsen, A. M., Zervas, A., Tendal, K., and Nielsen, J. L. (2015). Microbial Diversity in Bioaerosol Samples Causing ODTS Compared to Reference Bioaerosol Samples as Measured Using Illumina Sequencing and MALDI-TOF. Environ. Res. 140, 255–267. doi:10.1016/j.envres.2015.03.027
Martinez, F. J., Curtis, J. L., Sciurba, F., Mumford, J., Giardino, N. D., Weinmann, G., et al. (2007). Sex Differences in Severe Pulmonary Emphysema. Am. J. Respir. Crit. Care Med. 176 (3), 243–252. doi:10.1164/rccm.200606-828OC
May, S., Romberger, D. J., and Poole, J. A. (2012). Respiratory Health Effects of Large Animal Farming Environments. J. Toxicol. Environ. healthPart B, Crit. Rev. 15 (8), 524–541. doi:10.1080/10937404.2012.744288
Miyata, J., and Arita, M. (2015). Role of omega-3 Fatty Acids and Their Metabolites in Asthma and Allergic Diseases. Allergol. Int. : official J. Jpn. Soc. Allergol. 64 (1), 27–34. doi:10.1016/j.alit.2014.08.003
Morisseau, C., and Hammock, B. D. (2013). Impact of Soluble Epoxide Hydrolase and Epoxyeicosanoids on Human Health. Annu. Rev. Pharmacol. Toxicol. 53, 37–58. doi:10.1146/annurev-pharmtox-011112-140244
Nordgren, T. M., and Bailey, K. L. (2016). Pulmonary Health Effects of Agriculture. Curr. Opin. Pulm. Med. 22 (2), 144–149. doi:10.1097/MCP.0000000000000247
Nordgren, T. M., Bauer, C. D., Heires, A. J., Poole, J. A., Wyatt, T. A., West, W. W., et al. (2015). Maresin-1 Reduces Airway Inflammation Associated with Acute and Repetitive Exposures to Organic Dust. Transl Res. 166 (1), 57–69. doi:10.1016/j.trsl.2015.01.001
Nordgren, T. M., and Charavaryamath, C. (2018). Agriculture Occupational Exposures and Factors Affecting Health Effects. Curr. Allergy Asthma Rep. 18 (12), 65. doi:10.1007/s11882-018-0820-8
Nordgren, T. M., Friemel, T. D., Heires, A. J., Poole, J. A., Wyatt, T. A., and Romberger, D. J. (2014). The omega-3 Fatty Acid Docosahexaenoic Acid Attenuates Organic Dust-Induced Airway Inflammation. Nutrients 6 (12), 5434–5452. doi:10.3390/nu6125434
Nordgren, T. M., Heires, A. J., Wyatt, T. A., Poole, J. A., LeVan, T. D., Cerutis, D. R., et al. (2013). Maresin-1 Reduces the Pro-inflammatory Response of Bronchial Epithelial Cells to Organic Dust. Respir. Res. 14, 51. doi:10.1186/1465-9921-14-51
Nordgren, T. M., Heires, A. J., Zempleni, J., Swanson, B. J., Wichman, C., and Romberger, D. J. (2019). Bovine Milk-Derived Extracellular Vesicles Enhance Inflammation and Promote M1 Polarization Following Agricultural Dust Exposure in Mice. J. Nutr. Biochem. 64, 110–120. doi:10.1016/j.jnutbio.2018.10.017
Oliver, C., and Watson, H. (2013). Omega-3 Fatty Acids for Cystic Fibrosis. Cochrane database Syst. Rev. 11, CD002201. doi:10.1002/14651858.CD002201.pub4
Palmberg, L., Larssson, B. M., Malmberg, P., and Larsson, K. (2002). Airway Responses of Healthy Farmers and Nonfarmers to Exposure in a Swine Confinement Building. Scand. J. work, Environ. Health 28 (4), 256–263. doi:10.5271/sjweh.673
Patterson, E., Wall, R., Fitzgerald, G. F., Ross, R. P., and Stanton, C. (2012). Health Implications of High Dietary Omega-6 Polyunsaturated Fatty Acids. J. Nutr. Metab. 2012. doi:10.1155/2012/539426
Pavilonis, B. T., Sanderson, W. T., and Merchant, J. A. (2013). Relative Exposure to Swine Animal Feeding Operations and Childhood Asthma Prevalence in an Agricultural Cohort. Environ. Res. 122, 74–80. doi:10.1016/j.envres.2012.12.008
Pedersen, S., Nonnenmann, M., Rautiainen, R., Demmers, T. G., Banhazi, T., and Lyngbye, M. (2000). Dust in Pig Buildings. J. Agric. Saf. Health 6 (4), 261–274. doi:10.13031/2013.1909
Perez, T. A., Castillo, E. G., Ancochea, J., Pastor Sanz, M. T., Almagro, P., Martínez-Camblor, P., et al. (2020). Sex Differences between Women and Men with COPD: A New Analysis of the 3CIA Study. Respir. Med. 171, 106105. doi:10.1016/j.rmed.2020.106105
Phelps, D. S., Umstead, T. M., and Floros, J. (2012). Sex Differences in the Response of the Alveolar Macrophage Proteome to Treatment with Exogenous Surfactant Protein-A. Proteome Sci. 10 (1), 44. doi:10.1186/1477-5956-10-44
Pontes-Arruda, A., Demichele, S., Seth, A., and Singer, P. (2008). The Use of an Inflammation-Modulating Diet in Patients with Acute Lung Injury or Acute Respiratory Distress Syndrome: a Meta-Analysis of Outcome Data. JPENJournal Parenter. enteral Nutr. 32 (6), 596–605. doi:10.1177/0148607108324203
Poole, J. A., Dooley, G. P., Saito, R., Burrell, A. M., Bailey, K. L., Romberger, D. J., et al. (2010). Muramic Acid, Endotoxin, 3-hydroxy Fatty Acids, and Ergosterol Content Explain Monocyte and Epithelial Cell Inflammatory Responses to Agricultural Dusts. J. Toxicol. Environ. Health A. 73 (10), 684–700. doi:10.1080/15287390903578539
Poole, J. A., Wyatt, T. A., Kielian, T., Oldenburg, P., Gleason, A. M., Bauer, A., et al. (2011). Toll-like Receptor 2 Regulates Organic Dust-Induced Airway Inflammation. Am. J. Respir. Cel Mol Biol 45 (4), 711–719. doi:10.1165/rcmb.2010-0427OC
Poole, J. A., Wyatt, T. A., Oldenburg, P. J., Elliott, M. K., West, W. W., Sisson, J. H., et al. (2009). Intranasal Organic Dust Exposure-Induced Airway Adaptation Response Marked by Persistent Lung Inflammation and Pathology in Mice. Am. J. Physiol. Lung Cel Mol Physiol 296 (6), L1085–L1095. doi:10.1152/ajplung.90622.2008
Radon, K., Schulze, A., Ehrenstein, V., van Strien, R. T., Praml, G., and Nowak, D. (2007). Environmental Exposure to Confined Animal Feeding Operations and Respiratory Health of Neighboring Residents. Epidemiology (Cambridge, Mass) 18 (3), 300–308. doi:10.1097/01.ede.0000259966.62137.84
Raphael, W., and Sordillo, L. M. (2013). Dietary Polyunsaturated Fatty Acids and Inflammation: the Role of Phospholipid Biosynthesis. Int. J. Mol. Sci. 14 (10), 21167–21188. doi:10.3390/ijms141021167
Rasmussen, S. G., Casey, J. A., Bandeen-Roche, K., and Schwartz, B. S. (2017). Proximity to Industrial Food Animal Production and Asthma Exacerbations in Pennsylvania, 2005-2012. Int. J. Environ. Res. Public Health 14 (4). doi:10.3390/ijerph14040362
Romberger, D. J., Bodlak, V., Von Essen, S. G., Mathisen, T., and Wyatt, T. A. (19852002). Hog Barn Dust Extract Stimulates IL-8 and IL-6 Release in Human Bronchial Epithelial Cells via PKC Activation. J. Appl. Physiol. 93 (1), 289–296. doi:10.1152/japplphysiol.00815.2001
Romberger, D. J., Heires, A. J., Nordgren, T. M., Souder, C. P., West, W., Liu, X. D., et al. (2015). Proteases in Agricultural Dust Induce Lung Inflammation through PAR-1 and PAR-2 Activation. Am. J. Physiol. Lung Cel Mol Physiol 309 (4), L388–L399. doi:10.1152/ajplung.00025.2015
Schneberger, D., DeVasure, J., Bailey, K., Romberger, D., and Wyatt, T. (2017). Effect of Low-Level CO 2 on Innate Inflammatory Protein Response to Organic Dust from Swine Confinement Barns. J. Occup. Med. Toxicol. (London, England) 12 (9). doi:10.1186/s12995-017-0155-8
Schneberger, D., Pandher, U., Thompson, B., and Kirychuk, S. (2021). Effects of Elevated CO 2 Levels on Lung Immune Response to Organic Dust and Lipopolysaccharide. Respir. Res. 22 (1), 104. doi:10.1186/s12931-021-01700-4
Schwab, J. M., Chiang, N., Arita, M., and Serhan, C. N. (2007). Resolvin E1 and Protectin D1 Activate Inflammation-Resolution Programmes. Nature 447 (7146), 869–874. doi:10.1038/nature05877
Seki, H., Fukunaga, K., Arita, M., Arai, H., Nakanishi, H., Taguchi, R., et al. (19502010). The Anti-inflammatory and Proresolving Mediator Resolvin E1 Protects Mice from Bacterial Pneumonia and Acute Lung Injury. J. Immunol. (Baltimore, Md 184 (2), 836–843. doi:10.4049/jimmunol.0901809
Senthilselvan, A., Chénard, L., Kirychuk, S., Predicala, B., Schwartz, D. A., Burch, L. H., et al. (2009). Gender-related Tumor Necrosis Factor-Alpha Responses in Naïve Volunteers with Toll-like Receptor 4 Polymorphisms Exposed in a Swine Confinement Facility. J. Interferon Cytokine Res. 29 (12), 781–790. doi:10.1089/jir.2009.0002
Serhan, C. N., Chiang, N., Dalli, J., and Levy, B. D. (2014). Lipid Mediators in the Resolution of Inflammation. Cold Spring Harb Perspect. Biol. 7 (2), a016311. doi:10.1101/cshperspect.a016311
Serhan, C. N. (2014). Pro-resolving Lipid Mediators Are Leads for Resolution Physiology. Nature 510 (7503), 92–101. doi:10.1038/nature13479
Serhan, C. N., Yang, R., Martinod, K., Kasuga, K., Pillai, P. S., Porter, T. F., et al. (2009). Maresins: Novel Macrophage Mediators with Potent Antiinflammatory and Proresolving Actions. J. Exp. Med. 206 (1), 15–23. doi:10.1084/jem.20081880
Sigsgaard, T., Basinas, I., Doekes, G., de Blay, F., Folletti, I., Heederik, D., et al. (2020). Respiratory Diseases and Allergy in Farmers Working with Livestock: a EAACI Position Paper. Clin. Transl Allergy 10, 29. doi:10.1186/s13601-020-00334-x
Simopoulos, A. P. (2008). The Importance of the omega-6/omega-3 Fatty Acid Ratio in Cardiovascular Disease and Other Chronic Diseases. Exp. Biol. Med. (Maywood). 233 (6), 674–688. doi:10.3181/0711-MR-311
Singh, N., Barnych, B., Morisseau, C., Wagner, K. M., Wan, D., Takeshita, A., et al. (2020). -Benzyl-linoleamide, a Constituent of. J. Nat. Prod. 83 (12), 3689–3697. doi:10.1021/acs.jnatprod.0c00938
Smit, L. A., Hooiveld, M., van der Sman-de Beer, F., Opstal-van Winden, A. W., Beekhuizen, J., Wouters, I. M., et al. (2014). Air Pollution from Livestock Farms, and Asthma, Allergic Rhinitis and COPD Among Neighbouring Residents. Occup. Environ. Med. 71 (2), 134–140. doi:10.1136/oemed-2013-101485
Strasser, T., Fischer, S., and Weber, P. C. (1985). Leukotriene B5 Is Formed in Human Neutrophils after Dietary Supplementation with Icosapentaenoic Acid. Proc. Natl. Acad. Sci. United States America 82 (5), 1540–1543. doi:10.1073/pnas.82.5.1540
Szklarczyk, D., Gable, A. L., Nastou, K. C., Lyon, D., Kirsch, R., Pyysalo, S., et al. (2021). The STRING Database in 2021: Customizable Protein-Protein Networks, and Functional Characterization of User-Uploaded Gene/measurement Sets. Nucleic Acids Res. 49 (D1), D605–D12. doi:10.1093/nar/gkaa1074
Tam, A., Tanabe, N., Churg, A., Wright, J. L., Hogg, J. C., and Sin, D. D. (2020). Sex Differences in Lymphoid Follicles in COPD Airways. Respir. Res. 21 (1), 46. doi:10.1186/s12931-020-1311-8
Thompson, M., Hein, N., Hanson, C., Smith, L. M., Anderson-Berry, A., Richter, C. K., et al. (2019). Omega-3 Fatty Acid Intake by Age, Gender, and Pregnancy Status in the United States: National Health and Nutrition Examination Survey 2003(-)2014. Nutrients 11 (1). doi:10.3390/nu11010177
Titos, E., Rius, B., Gonzalez-Periz, A., Lopez-Vicario, C., Moran-Salvador, E., Martinez-Clemente, M., et al. (19502011). Resolvin D1 and its Precursor Docosahexaenoic Acid Promote Resolution of Adipose Tissue Inflammation by Eliciting Macrophage Polarization toward an M2-like Phenotype. J. Immunol. (Baltimore, Md 187 (10), 5408–5418. doi:10.4049/jimmunol.1100225
Trindade-da-Silva, C. A., Clemente-Napimoga, J. T., Abdalla, H. B., Rosa, S. M., Ueira-Vieira, C., Morisseau, C., et al. (2020). Soluble Epoxide Hydrolase Inhibitor, TPPU, Increases Regulatory T Cells Pathway in an Arthritis Model. FASEB J. 34, 9074–9086. doi:10.1096/fj.202000415R
Trotta, M. C., Gharbia, S., Herman, H., Mladin, B., Hermenean, A., Balta, C., et al. (2021). Sex and Age-Related Differences in Neuroinflammation and Apoptosis in. Int. J. Mol. Sci. 22 (12). doi:10.3390/ijms22126280
Ulu, A., Burr, A., Heires, A., Pavlik, J., Larsen, T., Perez, P., et al. (2021). A High Docosahexaenoic Acid Diet Alters Lung Inflammation and Recovery Following Repetitive Exposure to Aqueous Organic Dust Extracts: Role of DHA in Repetitive Organic Dust Exposure. J. Nutr. Biochem. 97, 108797. doi:10.1016/j.jnutbio.2021.108797
Ulu, A., Burr, A., Heires, A. J., Pavlik, J., Larsen, T., Perez, P. A., et al. (2021). A High Docosahexaenoic Acid Diet Alters Lung Inflammation and Recovery Following Repetitive Exposure to Aqueous Organic Dust Extracts. J. Nutr. Biochem. 97, 108797. doi:10.1016/j.jnutbio.2021.108797
Ulu, A., Harris, T. R., Morisseau, C., Miyabe, C., Inoue, H., Schuster, G., et al. (2013). Anti-inflammatory Effects of omega-3 Polyunsaturated Fatty Acids and Soluble Epoxide Hydrolase Inhibitors in Angiotensin-II-dependent Hypertension. J. Cardiovasc. Pharmacol. 62 (3), 285–297. doi:10.1097/FJC.0b013e318298e460
Ulu, A., Inceoglu, B., Yang, J., Singh, V., Vito, S., Wulff, H., et al. (2016). Inhibition of Soluble Epoxide Hydrolase as a Novel Approach to High Dose Diazepam Induced Hypotension. J. Clin. Toxicol. 6 (3). doi:10.4172/2161-0495.1000300
Ulu, A., Stephen Lee, K. S., Miyabe, C., Yang, J., Hammock, B. G., Dong, H., et al. (2014). An omega-3 Epoxide of Docosahexaenoic Acid Lowers Blood Pressure in Angiotensin-II-dependent Hypertension. J. Cardiovasc. Pharmacol. 64 (1), 87–99. doi:10.1097/FJC.0000000000000094
Ulven, S. M., and Holven, K. B. (2020). Metabolomic and Gene Expression Analysis to Study the Effects of Dietary Saturated and Polyunsaturated Fats. Curr. Opin. Lipidol. 31 (1), 15–19. doi:10.1097/MOL.0000000000000651
van der Strate, B. W., Postma, D. S., Brandsma, C. A., Melgert, B. N., Luinge, M. A., Geerlings, M., et al. (2006). Cigarette Smoke-Induced Emphysema: A Role for the B Cell? Am. J. Respir. Crit. Care Med. 173 (7), 751–758. doi:10.1164/rccm.200504-594OC
van Dijk, C. E., Garcia-Aymerich, J., Carsin, A. E., Smit, L. A., Borlee, F., Heederik, D. J., et al. (2016). Risk of Exacerbations in COPD and Asthma Patients Living in the Neighbourhood of Livestock Farms: Observational Study Using Longitudinal Data. Int. J. Hyg. Environ. Health 219 (3), 278–287. doi:10.1016/j.ijheh.2016.01.002
Vancheri, C., Mastruzzo, C., Sortino, M. A., and Crimi, N. (2004). The Lung as a Privileged Site for the Beneficial Actions of PGE2. Trends Immunol. 25 (1), 40–46. doi:10.1016/j.it.2003.11.001
Vanella, L., Canestraro, M., Lee, C. R., Cao, J., Zeldin, D. C., Schwartzman, M. L., et al. (2015). Soluble Epoxide Hydrolase Null Mice Exhibit Female and Male Differences in Regulation of Vascular Homeostasis. Prostaglandins Other Lipid Mediat 120, 139–147. doi:10.1016/j.prostaglandins.2015.04.004
Vegeto, E., Cuzzocrea, S., Crisafulli, C., Mazzon, E., Sala, A., Krust, A., et al. (2010). Estrogen Receptor-Alpha as a Drug Target Candidate for Preventing Lung Inflammation. Endocrinology 151 (1), 174–184. doi:10.1210/en.2009-0876
Von Essen, S., and Donham, K. (1999). Illness and Injury in Animal Confinement Workers. Occup. Med. (Philadelphia, Pa) 14 (2), 337–350.
Von Essen, S., and Romberger, D. (2003). The Respiratory Inflammatory Response to the Swine Confinement Building Environment: the Adaptation to Respiratory Exposures in the Chronically Exposed Worker. J. Agric. Saf. Health 9 (3), 185–196. doi:10.13031/2013.13684
von Schacky, C. (2014). Omega-3 index and Cardiovascular Health. Nutrients 6 (2), 799–814. doi:10.3390/nu6020799
Wagner, K., Gilda, J., Yang, J., Wan, D., Morisseau, C., Gomes, A. V., et al. (2017). Soluble Epoxide Hydrolase Inhibition Alleviates Neuropathy in Akita (Ins2 Akita) Mice. Behav. Brain Res. 326, 69–76. doi:10.1016/j.bbr.2017.02.048
Wang, L., Yang, J., Guo, L., Uyeminami, D., Dong, H., Hammock, B. D., et al. (2012). Use of a Soluble Epoxide Hydrolase Inhibitor in Smoke-Induced Chronic Obstructive Pulmonary Disease. Am. J. Respir. Cel Mol Biol 46 (5), 614–622. doi:10.1165/rcmb.2011-0359OC
Warren, K. J., Wyatt, T. A., Romberger, D. J., Ailts, I., West, W. W., Nelson, A. J., et al. (2017). Post-injury and Resolution Response to Repetitive Inhalation Exposure to Agricultural Organic Dust in Mice. Safety (Basel) 3 (1). doi:10.3390/safety3010010
Watkins, D. A., Dabestani, N., Mock, C. N., Cullen, M. R., Smith, K. R., and Nugent, R. (2017). “Trends in Morbidity and Mortality Attributable to Injuries and Selected Environmental Hazards,” in Injury Prevention and Environmental Health. Editors C. N. rdMock, R. Nugent, O. Kobusingye, and K. R. Smith (Washington (DC): The International Bank for Reconstruction and Development /The World Bank(c).). doi:10.1596/978-1-4648-0522-6_ch2
Waxman, D. J., and Holloway, M. G. (2009). Sex Differences in the Expression of Hepatic Drug Metabolizing Enzymes. Mol. Pharmacol. 76 (2), 215–228. doi:10.1124/mol.109.056705
Widgerow, A. D. (2012). Cellular Resolution of Inflammation-Ccatabasis. Wound Repair and Regeneration : Official Publication of the Wound Healing Society [and] the European Tissue Repair Society. Wound Repair Regen. 20 (1), 2–7. doi:10.1111/j.1524-475X.2011.00754.x
Wight, T. N., Frevert, C. W., Debley, J. S., Reeves, S. R., Parks, W. C., and Ziegler, S. F. (2017). Interplay of Extracellular Matrix and Leukocytes in Lung Inflammation. Cell Immunol 312, 1–14. doi:10.1016/j.cellimm.2016.12.003
Yang, B., Fritsche, K. L., Beversdorf, D. Q., Gu, Z., Lee, J. C., Folk, W. R., et al. (2019). Yin-Yang Mechanisms Regulating Lipid Peroxidation of Docosahexaenoic Acid and Arachidonic Acid in the Central Nervous System. Front. Neurol. 10, 642. doi:10.3389/fneur.2019.00642
Yang, H. H., Duan, J. X., Liu, S. K., Xiong, J. B., Guan, X. X., Zhong, W. J., et al. (2020). A COX-2/sEH Dual Inhibitor PTUPB Alleviates Lipopolysaccharide-Induced Acute Lung Injury in Mice by Inhibiting NLRP3 Inflammasome Activation. Theranostics 10 (11), 4749–4761. doi:10.7150/thno.43108
Yang, J., Bratt, J., Franzi, L., Liu, J. Y., Zhang, G., Zeki, A. A., et al. (2015). Soluble Epoxide Hydrolase Inhibitor Attenuates Inflammation and Airway Hyperresponsiveness in Mice. Am. J. Respir. Cel Mol Biol 52 (1), 46–55. doi:10.1165/rcmb.2013-0440OC
Zhang, Y. F., Sun, C. C., Duan, J. X., Yang, H. H., Zhang, C. Y., Xiong, J. B., et al. (2020). A COX-2/sEH Dual Inhibitor PTUPB Ameliorates Cecal Ligation and Puncture-Induced Sepsis in Mice via Anti-inflammation and Anti-oxidative Stress. Biomed. Pharmacother. 126, 109907. doi:10.1016/j.biopha.2020.109907
Zhou, Y., Liu, T., Duan, J. X., Li, P., Sun, G. Y., Liu, Y. P., et al. (2017). Soluble Epoxide Hydrolase Inhibitor Attenuates Lipopolysaccharide-Induced Acute Lung Injury and Improves Survival in Mice. Shock 47 (5), 638–645. doi:10.1097/SHK.0000000000000767
Keywords: fat-1 transgenic mice, omega-3 fatty acids, agricultural dust, resolution of inflammation, sex
Citation: Ulu A, Velazquez JV, Burr A, Sveiven SN, Yang J, Bravo C, Hammock BD and Nordgren TM (2022) Sex-Specific Differences in Resolution of Airway Inflammation in Fat-1 Transgenic Mice Following Repetitive Agricultural Dust Exposure. Front. Pharmacol. 12:785193. doi: 10.3389/fphar.2021.785193
Received: 28 September 2021; Accepted: 23 December 2021;
Published: 13 January 2022.
Edited by:
Pallavi R. Devchand, University of Calgary, CanadaReviewed by:
Gerard Bannenberg, Global Organization for EPA and DHA Omega-3s (GOED), United StatesCopyright © 2022 Ulu, Velazquez, Burr, Sveiven, Yang, Bravo, Hammock and Nordgren. This is an open-access article distributed under the terms of the Creative Commons Attribution License (CC BY). The use, distribution or reproduction in other forums is permitted, provided the original author(s) and the copyright owner(s) are credited and that the original publication in this journal is cited, in accordance with accepted academic practice. No use, distribution or reproduction is permitted which does not comply with these terms.
*Correspondence: Tara M. Nordgren, dG1ub3JkZ3JlbkBnbWFpbC5jb20=
Disclaimer: All claims expressed in this article are solely those of the authors and do not necessarily represent those of their affiliated organizations, or those of the publisher, the editors and the reviewers. Any product that may be evaluated in this article or claim that may be made by its manufacturer is not guaranteed or endorsed by the publisher.
Research integrity at Frontiers
Learn more about the work of our research integrity team to safeguard the quality of each article we publish.