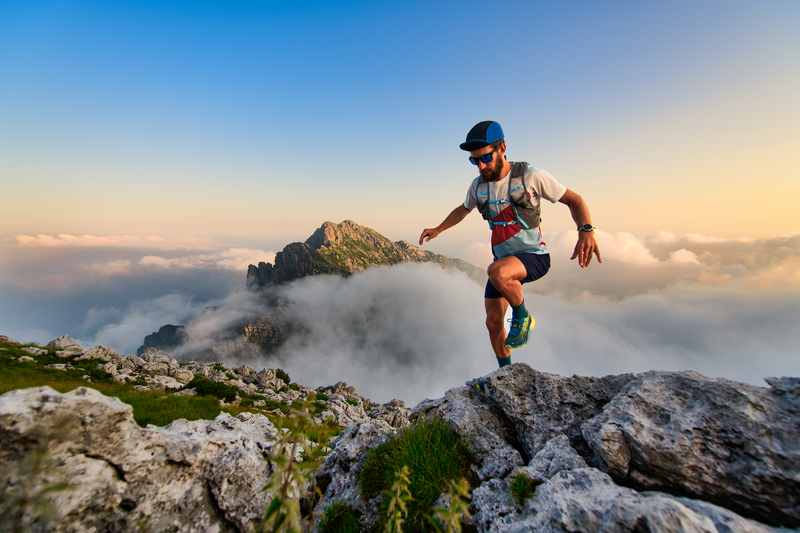
95% of researchers rate our articles as excellent or good
Learn more about the work of our research integrity team to safeguard the quality of each article we publish.
Find out more
REVIEW article
Front. Pharmacol. , 29 November 2021
Sec. Experimental Pharmacology and Drug Discovery
Volume 12 - 2021 | https://doi.org/10.3389/fphar.2021.777804
This article is part of the Research Topic Medicinal Cannabis: Evolution of Therapeutic Use, Future Approaches and Other Implications View all 12 articles
The medicinal use of Cannabis sativa L. can be traced back thousands of years to ancient China and Egypt. While marijuana has recently shown promise in managing chronic pain and nausea, scientific investigation of cannabis has been restricted due its classification as a schedule 1 controlled substance. A major breakthrough in understanding the pharmacology of cannabis came with the isolation and characterization of the phytocannabinoids trans-Δ9-tetrahydrocannabinol (Δ9-THC) and cannabidiol (CBD). This was followed by the cloning of the cannabinoid CB1 and CB2 receptors in the 1990s and the subsequent discovery of the endocannabinoid system. In addition to the major phytocannabinoids, Δ9-THC and CBD, cannabis produces over 120 other cannabinoids that are referred to as minor and/or rare cannabinoids. These cannabinoids are produced in smaller amounts in the plant and are derived along with Δ9-THC and CBD from the parent cannabinoid cannabigerolic acid (CBGA). While our current knowledge of minor cannabinoid pharmacology is incomplete, studies demonstrate that they act as agonists and antagonists at multiple targets including CB1 and CB2 receptors, transient receptor potential (TRP) channels, peroxisome proliferator-activated receptors (PPARs), serotonin 5-HT1a receptors and others. The resulting activation of multiple cell signaling pathways, combined with their putative synergistic activity, provides a mechanistic basis for their therapeutic actions. Initial clinical reports suggest that these cannabinoids may have potential benefits in the treatment of neuropathic pain, neurodegenerative diseases, epilepsy, cancer and skin disorders. This review focuses on the molecular pharmacology of the minor cannabinoids and highlights some important therapeutic uses of the compounds.
The marijuana plant has been grown and cultivated for medical, industrial and recreational uses throughout recorded history. Based on the physical characteristics of the plant, two main species of cannabis were originally described; Cannabis indica (short plant with broad leaves) and Cannabis sativa (tall plant with thin leaves) (Schultes, et al., 1974). However, numerous cannabis strains have been selected through breeding programs whose chemotaxonomic properties do not correlate with a Cannabis indica or Cannabis sativa lineage (Hillig and Mahlberg, 2004). More recently, the existence of only one species (Cannabis sativa L.), has been proposed (Small, 2015) with the strains categorized according to the content of the cannabinoids trans-Δ9-tetrahydrocannabinol (Δ9-THC) and cannabidiol (CBD). Δ9-THC dominant strains with low CBD content induce intoxicating, psychotropic effects including euphoria, enhancement of sensory perception and impairment in memory. In contrast, CBD dominant strains with low Δ9-THC content are considered to be nonpsychotropic.
Some of the earliest recorded medicinal uses of cannabis trace back to China and the pharmacopoeia of the Emperor Shen Nung (approximately 2500 BC), where the plant was indicated for the treatment of rheumatic pain, constipation, malaria and gynecological disorders (Russo, 2007; Pisanti and Bifulco, 2019). Along with China, cannabis medicine developed in India and then spread to Egypt, Greece and Rome. It was in Egypt that preparations of cannabis were first used in the treatment of glaucoma. By the 1800s extracts and tinctures of cannabis were recognized in the Western world for their relief of migraine headaches and their anti-emetic effects. In response to the perceived abuse of marijuana in the United States in the early 1900s, the Marijuana Tax Act was introduced which banned the sale and use of cannabis. This was followed in the 1970s by the classification of marijuana as a schedule 1 narcotic under the U.S. Controlled Substance Act. In Europe, the majority of countries have legalized the medical use of marijuana and decriminalized possession of small amounts of cannabis. However, the laws governing the use of cannabis can vary from one country to another with some countries having legalized only derivatives of the plant.
An important advancement in understanding the pharmacology of cannabis came with the isolation and structural determination of the phytocannabinoids CBD (Adams, et al., 1940a; Mechoulam and Shvo, 1963) and Δ9-THC (Gaoni and Mechoulam, 1971). Δ9-THC is the most abundant phytocannabinoid found in drug-type cannabis strains and the main psychotropic compound in the plant. In contrast, fiber-type strains have a higher content of CBD compared with Δ9-THC. CBD lacks psychotropic activity, but is reported to reduce the adverse effects (anxiety, psychosis, etc.) of Δ9-THC (Pennypacker and Romero-Sandoval, 2020). In addition to Δ9-THC and CBD, Cannabis sativa L. produces over 120 other phytocannabinoids as well as an abundance of related compounds including flavonoids, non-cannabinoid phenols, phenylpropanoids, fatty acids and terpenoids (Hanus, et al., 2016; Gülck and Möller, 2020). Phytocannabinoids are meroterpenoids (21- and 22-carbon terpenophenolic compounds with an alkyl side chain) produced in the plant’s glandular trichomes (Hanus, et al., 2016; Gülck and Möller, 2020). Cannabigerolic acid (CBGA), synthesized in cannabis from geranyl pyrophosphate and olivetolic acid, represents the parent cannabinoid from which the acidic and neutral minor cannabinoids are derived. In general, Δ9-THC and CBD are considered the major phytocannabinoids, while other phytocannabinoids, present in smaller amounts in the plant, are referred to as minor (or rare) cannabinoids.
More than 230 million people worldwide consume marijuana making it the most commonly used illicit substance (World Health Organization, 2016). In recent years, cannabis has become more accessible in the United States and Europe due to its legalization for medicinal and recreational purposes. While administered for a large number of medical conditions including nausea, anorexia, glaucoma, and muscle spasms, observational studies and user surveys indicate that pain management is the most common indication for the use of cannabis (Romero-Sandoval, et al., 2018). For this purpose, medical cannabis can be smoked or vaporized (using the floral buds of the plant), applied via oromucosal spray preparations [cannabis extract in Nabiximols (Sativex®)] or swallowed in capsule form as Nabilone (Cesamet®, synthetic cannabinoid) and Dronabinol (Marinol®, synthetic Δ9-THC). Anecdotal evidence indicates that the combination of the phytocannabinoids, terpenoids and other phytochemicals present in the whole cannabis plant provides a greater efficacy (called the “entourage effect”) in treating chronic pain when compared to oral cannabinoid formulations (Russo, 2011). However, definitive experimental data supporting this synergistic effect are currently lacking (Santiago, et al., 2019; Finlay, et al., 2020). Finally, in addition to its own direct anti-nociceptive effects, medical cannabis may have opioid drug-sparing actions: thus allowing lower doses of opioids to be used for pain relief (Khan, et al., 2019).
This review provides a brief description of the biosynthesis of the phytocannabinoids and an overview of the endocannabinoid system. This is followed by a discussion of the molecular pharmacology and potential therapeutic uses of the minor cannabinoids. Readers desiring information on Δ9-THC, CBD or synthetic cannabinoids are directed to these recent reviews (Banister, et al., 2019; de Almeida and Devi, 2020; Alves, et al., 2020; Walsh and Andersen, 2020).
Phytocannabinoids are meroterpenoids consisting of 21 or 22 carbon atoms that usually contain a propyl or pentyl side chain (Hanus, et al., 2016; Gülck and Möller, 2020). In Cannabis sativa L. the phytocannabinoids and terpenes are synthesized and stored in the glandular trichomes that are found in highest density in the female flowers of the plant (Hanus, et al., 2016; Gülck and Möller, 2020). The synthesis of the cannabinoids involves two pathways located in two separate sites within the glandular trichomes. In the first pathway olivetolic acid (OA) is produced in the cytosol of the gland cells from hexanoic acid. In the second, geranyl diphosphate (GPP) is generated in the plastidial organelles via the mevalonate-dependent isoprenoid (MEP) pathway. CBGA, the precursor of phytocannabinoids containing a pentyl side chain, is then synthesized from the GPP prenylation of olivetolic acid; a reaction catalyzed by olivetolate geranyltransferase (GOT) (Figure 1). Synthesis of tetrahydrocannabinolic acid (THCA), cannabidioloic acid (CBDA), and cannabichromenic acid (CBCA) proceeds through the appropriate oxidocyclases, THCA synthase, CBDA synthase and CBCA synthase, respectively (Figure 1). As described below, the neutral phytocannabinoids are then derived from the acidic forms through non-enzymatic decarboxylation during exposure to heat or light. Cannabigerovarin acid (CBGVA), the precursor to the variant cannabinoids (propyl side chain), is synthesized from divarinolic acid through geranyltransferase (Hanus, et al., 2016).
FIGURE 1. Biosynthesis pathways of phytocannabinoids. Abbreviations: CBN, cannabinol; CBC, cannabichromene; CBD, cannabidiol; CBG, cannabigerol; CBDA, cannabidioloic acid; CBGA, cannabigerolic acid; CBCA, cannabichromenic acid; GOT, olivetolate geranyltransferase; Δ9-THCA, Δ9-tetrahydrocannabinolic acid; Δ9-THC, Δ9-tetrahydrocannabinol.
Various methodologies including extraction/isolation, semi-synthesis or full synthesis and microbial engineering (E. coli, algae, yeast etc.,) are being utilized to obtain minor cannabinoids. Selective cannabis crossbreeding to enhance or decrease certain cannabinoids or terpenes is a common practice. For example, selective breeding has been used to yield cannabis varieties rich in CBG, CBC, THCV and CBDV (de Meijer and Hammond, 2005; de Meijer, et al., 2009). In a major advancement in the field, Luo et al. (2019) successfully introduced the MEP, GPP and hexanoic pathways along with THCA and CBDA synthases in yeast (Saccharomyces cerevisiae); establishing a platform for the large-scale fermentation of natural minor cannabinoids. Interestingly, unnatural cannabinoids with tailored alkyl side chains were produced by feeding different fatty acid precursors to the yeast (Luo, et al., 2019). Since the length and chemistry of the alkyl side chain modulates the affinity of the cannabinoids for the CB1 and CB2 receptors (Martin, et al., 1999), this platform could provide a novel method for discovering new and novel cannabinoid receptor specific agonists and antagonists.
Cannabinoid investigators initially hypothesized that Δ9-THC might act by disturbing cell membranes due to its lipophilic properties. However, binding assays obtained using the radio-labeled synthetic cannabinoid CP-55,940 [3(H)-CP-55,940], identified selective, high affinity binding sites for the compound in rat brain preparations (Devane, et al., 1988). This finding led to the cloning of the cannabinoid type 1 (CB1) (Matsuda, et al., 1990) and type 2 (CB2) (Munro, et al., 1993) receptors in the early 1990s. Both the CB1 and CB2 receptors are members of the G protein-coupled receptor (GPCR) super-family of proteins. CB1 receptors are primarily localized to presynaptic nerve terminals in the central and peripheral nervous system. Tissues expressing high levels of the CB1 receptor include the amygdala, hippocampus, cerebral cortex, cerebellum and spinal column (Herkenham, et al., 1990; Tsou, et al., 1998). In contrast, CB2 receptors are found in the cells of the immune system and in astrocytes and microglia of the CNS (Munro, et al., 1993; Galiégue, et al., 1995; Stella, 2010). As is the case with other Class A GPCRs, cannabinoid receptors contain seven transmembrane domains (TM1-7) with intracellular (ICLs) and extracellular loops (ECLs), an N-terminal ECL and an intracellular domain that interacts with pertussis toxin-sensitive G proteins (Gi/Go) (Figure 2). Using computational modeling with the CB1 receptor crystal structure, Hua et al. (2017) predicted that Δ9-THC interacts with the ECL2 and TM3, TM6 and TM7. Binding of cannabinoids is postulated to activate a toggle switch in the CB1 receptor (consisting of residues F200 and W356 in the TM3/TM6 binding pocket) that results in Gi/Go protein interaction (Hua, et al., 2017). The strong interaction of the indazole ring of the synthetic cannabinoid MDMB-FUBINACA (FUB) with the toggle switch stabilizes the active conformation of the receptor and brings about the high efficacy of this ligand (Kumar et al., 2019) (Figure 2). In contrast, (Kumar et al., 2019) suggested that the lack of toggle switch interaction by Δ9-THC may account for its partial agonist activity. Although cannabinoid receptors primarily couple to Gi/Go, they can also stimulate Gs and Gq proteins under certain conditions (Glass and Northup, 1999; Lauckner, et al., 2005).
FIGURE 2. Cannabinoid CB1 receptor structure and signaling. (A) Structural model of the CB1 receptor (CB1R)-Gi protein complex obtained from cryoelectron microscopy. The binding site for the synthetic cannabinoid MDMB-FUBINACA (FUB) is indicated by the magenta structure. The CB1-Gi receptor complex structure was obtained from the Protein Data Bank (code 6N4B). (B) Binding of cannabinoids to the neuronal CB1 receptor stimulates both neuronal Gi/Go and β-arrestin signaling pathways leading to an inhibition of adenylyl cyclase, activation of G protein-gated inward rectifier K+ (GIRK) channels and receptor internalization. In addition, activation of transient receptor potential vanilloid (TRPV) and ankyrin (TRPA) channels by cannabinoids causes Ca2+ influx that activates Ca2+-sensitive enzymes such as Ca2+/calmodulin-dependent protein kinase (CaM). Figure 2B was adapted from Walsh and Andersen (Walsh and Andersen, 2020).
Binding of Δ9-THC and synthetic cannabinoids (WIN 55,212-2, CP 55,940, etc.,) to the CB1 and CB2 receptors causes the dissociation of the βγ subunits of the G protein heterotrimer from the α subunit (Giα) (Figure 2). Giα inhibits adenylyl cyclase resulting in a decrease in intracellular levels of cAMP (Howlett, et al., 1986). In contrast, Giβγ inhibits the opening of voltage-gated Ca2+ channels (N and P/Q type) while activating G protein-gated inward rectifier K+ (GIRK) channels (Mackie, et al., 1995; Guo and Ikeda, 2004). In addition to GIRK channels, cannabinoid binding also couples to other K+ channels including M-type and A-type channels in cultured neurons (Schlicker and Kathmann, 2001). Together, these cannabinoid actions bring about an acute inhibition of synaptic neurotransmitter release and dampens neuronal excitability (Shen, et al., 1996; Vaughan, et al., 2000). These signaling effects are followed by receptor phosphorylation [by G protein receptor kinase (GRK)] that recruits β-arrestin1 (βarr1) and β-arrestin2 (βarr2) to the receptor and results in CB1 receptor desensitization and internalization (Figure 2) (Jin, et al., 1999; Ahn, et al., 2013). Both Gi and β-arrestin can also stimulate mitogen-activated protein kinases (MAPKs), including the extracellular signal-regulated kinases (ERK1/2), bringing about additional cellular effects (Bouaboula, et al., 1995; Galve-Roperh, et al., 2002; Derkinderen, et al., 2003).
Following the discovery of the CB1 and CB2 receptors, the endogenous cannabinoids (or endocannabinoids) anandamide [N-arachidonoylethanolamine (AEA)] and 2-arachidonoylglycerol (2-AG) were isolated (Devane, et al., 1992; Mechoulam, et al., 1995; Sugiura, et al., 1995). These endocannabinoids are synthesized from the cell membrane lipids N-arachidonoyl phosphatidyl ethanol (NAPE) (for AEA) and phosphatidyl inositol bis-phosphate (PIP2) (for 2-AG). Unlike the continuous cellular synthesis and storage of neurotransmitters and neuropeptides, AEA and 2-AG are produced through “on demand” cleavage of NAPE and PIP2. This provides for a temporal- and localization-dependent release of the endocannabinoids (Lu and Mackie, 2016). The actions of AEA and 2-AG are terminated following their cellular uptake and degradation by intracellular hydroxylase [fatty acid amide hydrolase (FAAH)] (for AEA) and lipase enzymes (monoacylglycerol lipase) (for 2-AG). Therefore, drugs that inhibit the cellular uptake of AEA and 2-AG or prevent their enzymatic degradation should result in a potentiation of endocannabinoid action. In addition to AEA and 2-AG, other putative endocannabinoids include N-arachidonoyldopamine (NADA), 2-arachidonoylglycerylether (noladin ether), N-oleoylethanolamine (OEA) and palmitoylethanolamide (PEA) (Tan, et al., 2006).
Endocannabinoids bring about their pharmacological effects through a number of mechanisms. Early studies demonstrated that while 2-AG acts as a full agonist at the CB1 and CB2 receptors, both AEA and Δ9-THC function as partial agonists when compared with the full cannabinoid agonist WIN 55,212-2 (Gonsiorek, et al., 2000; Luk, et al., 2004). Endocannabinoids can also act at receptor sites (“off targets”) other than the CB1 and CB2 receptors. Transient receptor potential (TRP) channels are a superfamily of inotropic channels that are activated by thermal, physical and electrochemical stimuli. The TRP channels are subdivided into several families including the vanilloid (TRPV), ankyrin (TRPA) and melastatin (TRPM) channels. Both AEA and 2-AG bind to and activate TRPV1 channels causing cell membrane potential depolarization and Ca2+ influx (Zygmunt, et al., 1999; Smart, et al., 2000) (Figure 2). AEA can also modulate the activity of TRPA1 and TRPM8 channels (De Petrocellis, et al., 2007; De Petrocellis, et al., 2012). In addition to the TRP channels, a number of other “off targets” for endocannabinoids have been identified. This includes the de-orphanized G protein-coupled receptors 18 (GPR18) and 55 (GPR55), as well as Peroxisome Proliferator-activated Receptors (PPARs). GPR18 and GPR55 are proposed to regulate acute and chronic pain pathways and are activated by the endogenous ligands N-arachidonoylglycine (NAgly) and lysophophatidylinositol (LPI), respectively (Kohno, et al., 2006; Henstidge, et al., 2009). Binding of endocannabinoids to these receptors stimulates cell signaling events through Gαi (GPR18), Gα12 and Gα12/13 (GPR55). PPARs are members of the nuclear hormone receptor family of proteins that function as ligand-inducible transcription factors. A number of endocannabinoids including AEA and 2-AG, as well as the phytocannabinoids Δ9-THC and CBD, are agonists at the PPARs (O'Sullivan, 2007).
Minor cannabinoids are divided into neutral, acidic and varinic phytocannabinoids. Minor cannabinoids include cannabinol (CBN), cannabichromene (CBC), cannabigerol (CBG), cannabidioloic acid (CBDA), cannabigerolic acid (CBGA), tetrahydrocannabinolic acid (THCA), cannabinolic acid (CBNA), cannabidivarin (CBDV), tetrahydrocannabivarin (THCV), cannabigerovarin (CBGV), cannabichromevarin (CBCV), and others (Hanus, et al., 2016; Gülck and Möller, 2020). Cannabinoids appear naturally in the cannabis plant in their acidic forms and are thought to confer antioxidant and defense mechanisms (insecticidal, antimicrobial, etc.,) to the plant. Acidic cannabinoids undergo decarboxylation during heating and are converted to the corresponding neutral cannabinoids (Figure 1). For example, THCA is converted to Δ9-THC when cannabis is smoked or vaporized. Some decarboxylation also occurs with passage of time at room temperature and during exposure to light. Cannabis products intended to contain the acidic forms of cannabinoids nearly universally also contain low levels of cannabinoids in their neutral forms. The varinic cannabinoids are considered rare but are now emerging as new targets of selective breeding. Varin compounds such as CBDV and THCV contain two fewer carbon atoms than their non-varin counterparts (CBD and Δ9-THC) endowing these cannabinoids with unique pharmacological properties (see below).
As described for the endocannabinoids, the overall pharmacological action of the minor cannabinoids often results from binding at both cannabinoid and “off target” receptors. This combination of receptor-mediated actions makes them well suited as multi-target therapeutic agents. While a number of minor cannabinoids including CBN and THCV bind to the CB1 receptor, they have significantly less binding activity when compared with Δ9-THC (Rhee, et al., 1997; Zagzoog, et al., 2020). To date, none of the minor cannabinoids have been clinically demonstrated to act as psychotropic drugs. The reported potencies of the minor cannabinoids at the CB1 and CB2 receptors and TRP channels are summarized in Table 1 and discussed in the sections below.
CBN was originally isolated from Indian hemp in 1896 making it the first phytocannabinoid identified in cannabis (Wood, et al., 1886). The structural determination and total synthesis of CBN was carried out by Adams and colleagues in the 1940s (Adams, et al., 1940b). CBN is not synthesized in the cannabis plant, but is derived during the degradation of Δ9-THC. Even under ideal storage conditions, exposure to UV light and/or heat over time results in the conversion of Δ9-THC to CBN. Using 3(H)-labelled synthetic cannabinoid (e.g., HU-243, CP-55,490) displacement assays it was determined that CBN has low binding affinities for the CB1 and CB2 receptors when compared with Δ9-THC (Rhee, et al., 1997; Mahadevan, et al., 2000; Rosenthaler, et al., 2014). In addition, CBN is less potent than Δ9-THC in CB1 receptor-mediated inhibition of adenylyl cyclase, but displays equal potency in CB2 receptor-mediated inhibition (Rhee, et al., 1997). CBN is an agonist at TRPV1, TRPV2, TRPV3 and TRPV4 channels stimulating cell Ca2+ influx with the activation of Ca2+-dependent pathways (De Petrocellis, et al., 2011). It is also a potent and efficacious agonist of the TRPA1 channel. In addition, CBN acts as a potent antagonist of icilin activation of the TRPM8 channel (De Petrocellis, et al., 2011).
CBN has been identified as a potential analgesic and anti-inflammatory agent. CBN isolate has been reported to relieve chronic muscle pain disorders such as temporomandibular disorders and fibromyalgia in a rat model of myofascial pain (Wong and Cairns, 2019). For example, CBN (1 mg/ml) reduces mechanical sensitivity induced by intramuscular injection of nerve growth factor in the masseter muscle (Wong and Cairns, 2019). While CBN is not as widely recognized as CBD and Δ9-THC for its anti-inflammatory properties, it may have therapeutic benefits in treating allergic airway diseases. CBN attenuates the production of interleukins 2, 4, 5, 13 and decreases allergen mucus production in OVA-sensitized and challenged A/J mice (Jan, et al., 2003). CBN and Δ9-THC (but not CBD) can also be used to treat glaucoma since they prevent inflammation that causes elevated intraocular pressure (ElSohly, et al., 1981). In addition, preliminary data indicate that CBN decreases cell damage and acts as an antioxidant in a cell culture model of Huntington’s disease (Aiken, et al., 2004).
CBN also shows promise as an antibacterial agent and an appetite stimulant. As with other cannabinoids (e.g., CBC and CBG), CBN has been found to be highly efficacious against multiple antibiotic-resistant bacteria, including methicillin-resistant Staphylococcus aureus (MRSA), making it a potentially viable treatment for staph infections (Appendino, et al., 2008). CBN also stimulates hyperphagia and increases food consumption and feeding time in rats (Farrimond et al., 2012a; Farrimond, et al., 2012b). Although CBN is not as potent an appetite stimulant as Δ9-THC, CBN administration is not associated with the psychotropic effects of Δ9-THC. Thus, CBN represents a non-intoxicating alternative to Δ9-THC as an appetite stimulant.
CBN-rich products are advertised for promoting sleep or relaxation without the impairment caused by Δ9-THC. Since CBN is a degradation product of Δ9-THC it is found in greater quantities in aged cannabis preparations. For this reason it is marketed as “the sleepy cannabinoid in old weed.” However, laboratory results obtained from sleep studies with CBN have been equivocal. In mice, CBN was reported to increase barbiturate-induced sleep time in one study (Yoshida, et al., 1995), while having no effects on sleep in another (Chesner, et al., 1974). When administered along with Δ9-THC in rats, CBN produces greater sedation compared with either cannabinoid alone (Fernandes, et al., 1974; Takahashi and Karniol, 1975). In one clinical study involving a small number of participants, the combination of Δ9-THC and CBN caused greater drowsiness than with Δ9-THC used alone (Karniol, et al., 1975). However, in a recent review of the CBN literature, Corroon (2021) found little evidence supporting a sleep promoting effect of CBN. Therefore, controlled studies are warranted to substantiate sleep-related claims of CBN containing products.
CBC is one of the most abundant minor cannabinoids found in cannabis. The structure of CBC was first determined using NMR spectroscopy by Gaoni and Mechoulam (Gaoni and Mechoulam, 1966). Although cannabinoid receptor studies using CBC are limited, the cannabinoid was initially identified as partial CB2 receptor agonist (Rosenthaler, et al., 2014). This was supported by experiments using a “hyperpolarization assay” with pituitary AtT20 cells in which CBC was found to be selective in stimulating the CB2 receptor over the CB1 receptor (Udoh, et al., 2019). In this same study, CBC was more potent and efficacious than Δ9-THC in causing cell hyperpolarization via the CB2 receptor. In contrast to these results, CBC was shown in a recent paper to display similar affinities for the CB1 and CB2 receptors and to cause both CB1 and CB2 receptor-mediated decreases in cellular cAMP levels (Zagzoog, et al., 2020). As is the case for CBN, CBC is a potent activator of the TRPA1 channel (De Petrocellis, et al., 2011). In addition, it activates TRPV3 and TRPV4 channels when applied at micromolar and submicromolar concentrations (De Petrocellis, et al., 2011). Other proposed sites of action of CBN are discussed below.
Anti-inflammatory effects of CBC were first reported in the 1980s using a rat model of edema. High doses of CBC were more efficacious than the nonsteroidal anti-inflammatory drug (NSAID) phenylbutazone in carrageenan-induced paw edema (Turner and ElSohly, 1981). CBC has been shown to reduce pain and inflammation associated with osteoarthritis in rats without the negative side effects of NSAIDs (Maione, et al., 2011). In addition, CBC attenuates lipopolysaccharide (LPS)-induced increases in nitric oxide levels in an in vitro model of colitis (Romano, et al., 2013) and reduces inflammation-induced GI motility (Izzo, et al., 2012). CBC also displays a modest anti-nociceptive effect in the mouse tail-withdrawal assay (Maione, et al., 2011; Zagzoog, et al., 2020). CBC regulates a number of cellular pathways involved in anti-nociception that include the stimulation of adenosine A1 receptors, CB1 receptors and TRPA1 channels (De Petrocellis, et al., 2011; Maione, et al., 2011). In addition, CBC has been proposed to inhibit AEA (anandamide) reuptake; thus potentiating the physiological effects of AEA (De Petrocellis, et al., 2011). Like other synergistic actions of cannabinoids, CBC has a greater anti-inflammatory response when combined with Δ9-THC than when either cannabinoid is used alone (DeLong, et al., 2010).
The anti-inflammatory actions of CBC may be important in its ability to function as a neuroprotective drug. CBC increases the viability of neural stem progenitor cells (NSPCs) in vitro through an ERK dependent mechanism (Shinjyo and Di Marzo, 2013). In addition, CBC inhibits astroglial differentiation of the NSPCs (Shinjyo and Di Marzo, 2013). NSPCs are modulated by surrounding microglial cells, brain immune cells, and astrocytes, which produce both pro- and anti-inflammatory factors. The potential anti-inflammatory and neuroprotective effects of CBC may occur through its suppression of reactive astrocytes (Covelo, et al., 2021). CBC inhibition of NSPCs differentiation into astrocytes may therefore offer a protective effect against neuro-inflammation, Alzheimer’s disease, and hepatic encephalopathy (Covelo, et al., 2021).
Δ9-THC possesses anti-tumor properties and is used for treating several different forms of cancer (Fraguas-Sánchez and Torres-Suárez, 2018). However, the psychotropic qualities of Δ9-THC limit its use as a chemotherapy agent. CBC may be beneficial in cancer treatment due to its ability to increase blood levels of AEA (see above). AEA has been shown to inhibit breast cancer cell proliferation and induce death of colon cancer cells (De Petrocellis, et al., 1998; Patsos, et al., 2005). CBC was also shown to inhibit cell migration and disrupt the cell cytoskeleton in an in vitro model of urothelial cancer (Anis, et al., 2021). In one study that examined the anti-tumor effects of several minor cannabinoids, only CBG was more potent than CBC at inhibiting the growth of several cancer cell lines (Ligresti, et al., 2006).
CBG is produced via decarboxylation of CBGA, the precursor molecule of the Δ9-THC and CBD branches of the cannabis synthesis pathway (Figure 1). Results obtained using cAMP assays revealed that CBG displays weak partial agonist activity at the CB1 and CB2 receptors (see Table 1) (Husni, et al., 2014; Navarro, et al., 2020; Zagzoog, et al., 2020). In CHO cells expressing both CB1 and CB2 receptors, CBG binds to the receptors with Kis in the low micromolar range (Navarro, et al., 2018). Of special note, in this same study CBG was found to compete with 3(H)-CP-55,490 for binding to the CB1 receptor, but not 3(H)-WIN-55,212-2 (Navarro, et al., 2018). This suggests the CBG and CP-55,490 (but not WIN-55,212-2) bind to the same orthosteric site on the receptor. CBG activates TRPV1, TRPV2, TRPV3, TRPV4 and TRPA1 channels at low micromolar concentrations (De Petrocellis, et al., 2011). CBG has also been shown to act through other off-target sites including the 5-HT1a receptor and the α2-adrenergic receptor. For example, CBG competitively antagonizes the ability of the 5-HT1a agonist 8-hydroxy-2-(di-n-propylamino)tetralin (8-OH-DPAT) to stimulate (35S)GTPγS binding in rat brain membranes (Cascio, et al., 2010). Furthermore, CBG inhibits electrically-induced contractions of the vas deferens and stimulates (35S)GTPγS binding in rat brain membranes through agonist activity at the α2-adrenergic receptor (Cascio, et al., 2010). CBG, along with acidic cannabinoids THCA and CBDA, also binds to and activates PPARγ (D'Aniello, et al., 2019) (see below).
As with other minor cannabinoids, CBG may reduce the severity of inflammatory diseases and peripheral pain. The anti-inflammatory properties of CBG are postulated to result from binding to the CB2 receptor, TRP channels, PPARγ and other targets (De Petrocellis, et al., 2011; Cascio, et al., 2010; Ruhaak, et al., 2011). There is anecdotal human and preclinical evidence for CBG having a benefit in cases of inflammatory bowel diseases including Crohn’s and ulcerative colitis. In a mouse model of colitis, CBG was found to reduce bowel inflammation, nitric oxide production [from increased nitric oxide synthase (iNOS) expression during inflammation] and oxidative stress in intestinal cells (Borrelli, et al., 2013; Pagano, et al., 2021). Similar to CBC, CBG (3 mg/kg and 10 mg/kg i.p.) produces a weak anti-nociceptive effect in mice (Zagzoog, et al., 2020). In one study this effect of CBG was inhibited by the α2-adrenergic receptor antagonist yohimbine, suggesting a role of α2-adrenergic regulation in CBG analgesia (Cascio, et al., 2010).
Inflammation and oxidative stress are both contributors to neurodegeneration, which is linked to Alzheimer’s and Huntington’s disease as well as Multiple Sclerosis (MS). CBG may protect against both neuroinflammation and oxidative stress, helping to prevent neuronal cell loss (Gugliandolo, et al., 2018). Carrillo-Salinas et al. (2014) examined the effect of the CBG analog VCE-003 on human T-cells and its efficacy in a mouse model of autoimmune MS. When tested in vitro, VCE-003 inhibited antigen-induced T-cell proliferation, cell cycle progression and the expression of surface activation markers. VCE-003 also prevented the expression of the pro-inflammatory enzyme iNOS in microglia. In animals, VCE-003 attenuated MS through activating CB2 and PPARγ receptors (Carrillo-Salinas, et al., 2014). CBG was also investigated in a mouse model of Huntington’s disease induced using 3-nitropropionate (3-NP) (Valdeolivas, et al., 2015). Treatment with CBG (10 mg/kg) reduced levels of the pro-inflammatory cytokines IL-6 and tumor necrosis factor α in the 3-NP treated mice. CBG also partially improved motor deficits and preserved striatum neurons in the R2/6 transgenic model of Huntington’s disease (Valdeolivas, et al., 2015).
As noted previously, CBG is effective in suppressing cancer cell growth (Ligresti, et al., 2006). In a murine colon cancer model, CBG was found to promote cancer cell death and inhibit the growth of tumors (Borrelli, et al., 2014). This inhibition was mimicked by TRPM8 channel antagonists (Borrelli, et al., 2014). Additionally, in vitro experiments using leukemia cell lines suggest this anti-cancer activity is enhanced when CBG is combined with other cannabinoids such as CBD (Scott, et al., 2013). Clinical studies are currently underway to determine if these results are translatable to treatment in humans. Individuals living with cancer and AIDS commonly experience anorexia and cachexia. CBG represents a non-psychoactive alternative to Δ9-THC for treating anorexia since it stimulates appetite and increases food consumption (Brierley, et al., 2017). Interestingly, CBG as part of a whole plant cannabis extract is more potent in stimulating appetite than CBG as an isolate (Brierley, et al., 2017). Thus, these results provide additional evidence that synergism of minor cannabinoids with other components of the cannabis plant may enhance their clinical efficacy (Russo, 2011).
CBDA was first isolated in 1955 and its structure elucidated in 1965 (Mechoulam and Gaoni, 1965). CBDA has a low affinity for both the CB1 and CB2 receptors based on 3[H]-CP-55,490 displacement assays (Zagzoog, et al., 2020). However, it shows moderate efficacy in inhibiting adenylyl cyclase through these receptors (Navarro, et al., 2020; Zagzoog, et al., 2020). In addition, CBDA is one of several minor cannabinoids (along with THCA and THCV) that functions as allosteric regulators at 5-HT1a receptors (Bolognini, et al., 2013). CBDA enhances 8-OH-DPAT-stimulated (35S)GTPγS binding to 5-HT1a receptors expressed in rat brain and CHO cell membranes possibly by binding to an allosteric site on the receptor (Bolognini, et al., 2013). CBDA was reported to be 1,000 times more potent than CBD in stimulating (35S)GTPγS binding at the 5-HT1a receptor. Based on in silico docking experiments, it was predicted that CBDA, along with the cannabinoids CBGA and CBG, bind to PPARs (D'Aniello, et al., 2019). In vitro reporter assays carried out with CHO cells confirmed that all three minor cannabinoids activate PPARα and PPARγ (D'Aniello, et al., 2019).
CBDA produces dose-dependent anti-hyperalgesia and anti-inflammatory effects in a rodent model of carrageenan-induced hind paw inflammation (Rock, et al., 2018). The anti-hyperalgesia effect of CBDA is blocked by AMG9810, an antagonist of the TRPV1 channel. In addition, when combined with Δ9-THC, low doses of CBDA are more effective in preventing hyperalgesia and reducing inflammation. CBDA may also produce anti-inflammatory effects via cyclooxygenase 2 (COX-2) enzyme inhibition; the same mechanism of action as the NSAID Celecoxib (Takeda, et al., 2008; Ruhaak, et al., 2011). CBDA inhibits the COX-2 enzyme in vitro with an EC50 of 2 µM and has 9-fold greater selectivity in inhibiting the COX-2 enzyme over the COX-1 enzyme. This selective inhibition is dependent on the presence of the carboxylic acid moiety in the CBDA molecule (Takeda, et al., 2008).
CBDA has anti-nausea effects at low doses in mice that are mediated via agonist activity at CNS 5-HT1A receptors (Pertwee, et al., 2018). CBDA was found to be 1000-fold more potent than CBD in reducing nausea-induced conditioned gaping disgust responses (Rock et al., 2020). The drug HU-580, a stable analogue of CBDA that is not metabolized to CBD, also reduces LiCl-induced conditioned gaping (Pertwee, et al., 2018). In addition to suppressing acute nausea, CBDA decreases anticipatory nausea and vomiting which occurs upon re-exposure to a contextual stimulus previously associated with acute nausea (e.g., a chemotherapy session) (Limebeer, et al., 2014). CBDA combined with ondansetron, a commonly used antiemetic drug, enhances ondansetron’s effect when applied at low doses (Rock and Parker, 2013).
Anderson et al. (2019) examined the anti-seizure activity of CBDA using Scn1aRX/+ mouse model of Dravet Syndrome. The Scn1aRX/+ mice develop generalized tonic-clonic seizures in response to elevated body temperature and thus recapitulate the seizures observed in children with Dravet Syndrome. When administered using i.p. injection (10 and 30 mg/kg), CBDA raised the temperature threshold required for seizures in the mice (Anderson, et al., 2019). CBDA also displayed dose-dependent protection in rats against electroshock-induced seizures (Goerl, et al., 2021). While clinical trials have not been reported, CBDA may be more effective than CBD in reducing seizures in humans. According to a patent application by GW Pharmaceuticals, the makers of Epidiolex® (a sublingual spray containing 100 mg of CBD/100 ml of solution), CBDA displays greater bioavailability and potency in treating epilepsy (GW PHARMA LTD, 2015).
CBGA is the precursor cannabinoid to THCA, CBDA, and CBCA (see Figure 1). Since CBGA is decarboxylated over time to CBG, it is rarely found in significant amounts in mature cannabis flowers. Thus, harvesting hemp very early yields higher levels of CBGA compared to later in the plant’s life. In addition, some cultivars have increased yields of CBGA through selective breeding to inhibit its transformation into other cannabinoids during the plant’s maturation (Garfinkel, et al., 2021). Similar to CBDA, CBGA displays low affinity for both the CB1 and CB2 receptors (Navarro, et al., 2020). Nonetheless, CBGA is equally as efficacious as Δ9-THC in decreasing intracellular cAMP levels though the CB1 receptor (Table 1) (Navarro, et al., 2020). However, unlike Δ9-THC, it is not effective in recruiting βarr2 to the CB1 receptor (Navarro, et al., 2020). CBGA has important off target effects including activating PPARs (D'Aniello, et al., 2019). In addition, fractions of Cannabis sativa containing high amounts of CBG/CBGA inhibit the aldose reductase enzyme (Smeriglio, et al., 2018).
While less in known about the therapeutic uses of CBGA compared with the other minor cannabinoids, it may play a role in controlling diabetes mellitus and preventing the cardiovascular complications that can accompany Type 2 diabetes (D'Aniello, et al., 2019). Through activation of PPARs, CBGA can improve lipid metabolism and reduce the accumulation of adipose tissue; thus reducing insulin resistance in the Type 2 patient (Gao, et al., 2015). Type 2 diabetes is considered a “coronary artery disease equivalent” and mortality in Type 2 diabetes primarily results from cardiovascular events including acute coronary syndrome (ACS). By inhibiting the enzyme aldose reductase, CBGA improves cardiac glucose metabolism and reduces the risk of ACS (Smeriglio, et al., 2018). Synthetic inhibitors of aldose reductase have severe side effects including elevations in blood liver enzymes from hepatotoxicity, as well as nausea and vomiting. Therefore, plant-derived CBGA offers a promising alternative to these inhibitors.
CBGA may also be beneficial in treating some types of cancer. A cannabis fraction containing high amounts of CBGA was reported to have cytotoxic activity against colon cancer cells (Nallathambi, et al., 2018). Interestingly, synergistic toxic effects were observed when CBGA was given with a cannabis fraction high in THCA. These two fractions also prevented the growth and proliferation of adenomatous colon polyps that are colon cancer precursors. When tested at micromolar concentrations, CBGA was also shown to have cytotoxic actions in human leukemia cancer cell lines (Scott, et al., 2013). In further support of cannabinoid synergism, the IC50 for CBGA leukemia cell toxicity was reduced when co-applied with CBD (Scott, et al., 2013; Scott, et al., 2017).
THCA is a non-psychotropic cannabinoid that is converted to Δ9-THC through decarboxylation by exposure to heat (Figure 1). Since THCA is a precursor to Δ9-THC, and because no sample of THCA is completely free of Δ9-THC, possession of this cannabinoid could be prosecuted under the U.S. government Federal Analogue Act. THCA displays roughly 60- and 125-fold lower affinity for the CB1 and CB2 receptors compared with Δ9-THC (McPartland, et al., 2017). While high concentrations of THCA inhibit forskolin-stimulated increases in cAMP through the CB1 receptor, it produces no inhibition through the CB2 receptor (McPartland, et al., 2017). Nagal et al. (2017) compared the effects of several cannabinoids, including CBDA, CBGA and THCA on PPAR activity. When compared with CBDA and CBGA, THCA has the highest binding affinity for PPARγ (Nadal, et al., 2017). In addition, THCA is more potent than other minor cannabinoids in inducing PPARγ-mediated transcriptional activity.
THCA was recently shown to possess potent anti-inflammatory activity in mice fed a high fat diet (HFD) (Palomares, et al., 2020; Carmona-Hidalgo, et al., 2021). THCA treatment reduced the expression of inflammatory molecules including tumor necrosis factor alpha (TNF-α4) and cytokine interleukin 10 (IL-10) in the HFD mice. This effect was mediated via PPARγ stimulation (Palomares, et al., 2020). THCA also improved glucose tolerance and attenuated liver fibrosis in the HFD mice (Carmona-Hidalgo, et al., 2021). Using an in vitro COX-1/COX-2 assay it was determined that Δ9-THCA inhibits both COX-1 and COX-2 enzymes with a concentration causing 50% inhibition (IC50) in the high micromolar range (Ruhaak, et al., 2011). Nallathambi et al. (2017) reported that cannabis fractions containing high amounts of THCA produce anti-inflammatory effects (e.g., reduction in IL-8) in several colon epithelial cell lines and in colon tissue biopsies. Anti-inflammatory effects of THCA were inhibited by treatment with the GPR55 antagonist CID16020046, but not by the CB1 and CB2 receptor antagonist rimonabant and SR144528 (Nallathambi, et al., 2017). In addition to its anti-inflammatory properties, THCA also has anti-nausea and antiemetic properties in mice at doses much lower than Δ9-THC (Rock, et al., 2013a). Thus, THCA may present a non-psychotropic alternative to Δ9-THC for treating nausea and vomiting.
As discussed previously for CBC and CBG, THCA may also exhibit neuroprotective properties that could be beneficial in the treatment of neurodegenerative diseases. THCA improved neuronal viability through a PPARγ-dependent pathway in an in vitro model of Huntington’s disease (Nadal, et al., 2017). THCA also caused an improvement in hind limb dystonia and locomotor activity in mice treated with 3-NPA. These neuroprotective actions of THCA were significantly reduced when mice were co-administered the PPARγ antagonist T0070903. In contrast, THCA had no effects on the survival of dopaminergic neurons in a 1-methyl-4-phenyl pyridinium (MPP+) cell culture model of Parkinson’s disease (Moldzio, et al., 2012). Since THCA undergoes decarboxylation to Δ9-THC, it is possible that the reported neuroprotective effects of THCA in Huntington’s disease may have resulted from contamination by Δ9-THC (Sagredo, et al., 2011). In addition, THCA displays poor brain penetration properties when tested using two vehicles (vegetable oil and Tween 80) (Anderson, et al., 2019); a limitation that could reduce its clinical efficacy.
Anecdotal reports have long suggested that THCA acts as an anticonvulsant. Over 40 years ago Karler and Turkanis reported that THCA (200 mg/kg) reduces seizures in the mouse maximal electroshock test (Karler and Turkanis, 1979). In a more recent mouse study the anticonvulsant effects of THCA were found to vary depending on the seizure model utilized and whether Δ9-THC was given along with the THCA (Benson, et al., 2020). When used alone, THCA (2, 30, and 100 mg/kg) was ineffective in the 6-Hz threshold (6-HzT) model of psychomotor seizures, but had anticonvulsant activity when given with Δ9-THC. Conversely, THCA used alone or with Δ9-THC did not reduce hyperthermia-induced seizures in the Scn1aRX/+ mice model (compared with the protective effects of CBDA described above). More encouraging results were reported with THCA in a clinical study. The frequency and duration of seizures were reduced in four case reports of children using low doses of THCA (0.1–1 mg/kg per day) in conjunction with conventional antiepileptic drugs and full spectrum cannabis (Sulak, et al., 2017). In contrast, Epidiolex® (CBD), which is approved by the U.S. Food and Drug Administration (FDA) for treating epilepsy, is dosed from 5 to 25 mg/kg per day. Thus, THCA may be ten to hundred times more potent in reducing seizures. However, increased doses of THCA did not improve efficacy in this clinical study (Sulak, et al., 2017). Furthermore, formulations of THCA containing high levels of the terpenoid α-linalool were more efficacious than formulations containing low levels of the terpenoid. Thus, other components of the THCA formulation may have accounted for the beneficial effects. Finally, symptoms and seizure activity worsened in one patient after increasing the THCA dose (Sulak, et al., 2017).
CBDV is found in landrace cannabis strains that have relatively high amounts of CBD and low amounts of Δ9-THC. Prior to its isolation in 1969, it was assumed that all naturally occurring cannabinoids contained a pentyl side chain, rather than the propyl chain found in CBDV and THCV. CBDV displays low binding affinity for the CB1 and CB2 receptors (Rosenthaler, et al., 2014; Husni, et al., 2014). Consistent with this, high concentrations of CBDV are needed for CB1 receptor stimulation of (35S)GTPγS binding, inhibition of cAMP synthesis and recruitment of βarr2 (Husni, et al., 2014; Navarro, et al., 2020; Zagzoog, et al., 2020). Overall, CBDV is a more potent and efficacious agonist at CB2 receptors (Navarro, et al., 2020; Zagzoog, et al., 2020). CBDV displays a similar pharmacological profile for TRP channels as CBN, CBG and THCV; activating TRPV1, TRPV2, TRPV3, TRPV4 and TRPA1 channels while inhibiting the TRPM8 channel (De Petrocellis, et al., 2008; De Petrocellis, et al., 2011). Other important off target sites for CBDV include the de-orphanized receptors GPR55 and GPR6. Binding of CBDV to the GPR55 receptor stimulates ERK1/2 phosphorylation and inhibits LPS-mediated signaling effects occurring through the GPR55 receptor (Anavi-Goffer, et al., 2012). These effects of CBDV are comparable to those of Δ9-THC. GPR6 is a constitutively active receptor that couples to Gs to stimulate adenylyl cyclase and recruits βarr2. CBDV acts as an inverse agonist at the GPR6 receptor causing significant inhibition of βarr2 recruitment at concentrations of 1 and 10 µM (Laun, et al., 2018).
CBD (marketed as Epidiolex®) was approved by the U.S. Food and Drug Administration (FDA) in 2018 for preventing epileptic seizures in Lennox-Gastaut syndrome and Dravet syndrome in children. CBDV, a structural homolog of CBD, possesses anti-epileptic properties when tested in animals and humans. When examined in vitro in rat brain slices, CBDV attenuates epileptiform local field potentials induced by 4-amino pyridine (Hill, et al., 2012). In vivo, CBDV (200 mg/kg per day) significantly reduces PTZ-induced seizure activity in the rats (Hill, et al., 2012). However, when used alone, CBDV has no effect on pilocarpine-induced seizures, but requires the co-administration of valproate or phenobarbital to be effective. Consistent with this, Amada et al. (2013) reported that CBDV significantly decreases PTZ-induced seizure severity. In addition, CBDV suppresses the expression of several epilepsy-related genes in animals that respond to CBDV anti-epileptic treatment (Amada, et al., 2013). A human trial to assess the efficacy, safety, and tolerability of CBDV in adults with focal seizures was recently conducted by GW Pharmaceuticals, the maker of Epidiolex® (Brodie, et al., 2021). The drug GPW42006 (800 mg b.i.d.), containing CBDV as its major component, reduced the frequency of seizures by 41%. However, similar reductions in focal seizure frequency were observed in the CBDV and placebo (38%) groups. There was also no differences between CBDV and placebo groups for any specific seizure type. Therefore, higher doses and longer durations of treatment of GPW42006 will be needed in future clinical trials to better access the benefits of CBDV.
CBDV has been investigated as a treatment for symptoms associated with autism spectrum disorder (ASD) such as repetitive behaviors, cognitive challenges and issues with communication and social functioning (Mouro, et al., 2019). Mice carrying mutations in the MeCP2 gene and MeCP2 null mice develop Rett Syndrome (RTT), a neurodevelopment disease related to ASD. CBDV treatment (2 mg/kg) was found to rescue both behavioral and phenotypic changes in the RTT mice model (Vigli, et al., 2018). These CBDV effects included improvements in motor coordination, locomotion and brain weight. When administered using a life-long treatment schedule, CBDV also prolonged survival and delayed the appearance of neurological and motor deficits in MeCP2 null mice (Zamberletti, et al., 2019a). CBDV also reversed memory deficits in these mice. Similar behavioral improvements were reported using CBDV in a valproic acid-induced model of ASD (Zamberletti, et al., 2019b).
CBDV may also have utility in the treatment of Duchenne muscular dystrophy (DMD) and in preventing nausea. In a recent study, CBDV was found to improve muscle quality and locomotion and to slow muscle degeneration in male dystrophic mdx mice (Iannotti, et al., 2019). Muscle improvement by CBDV (and CBD) was accompanied by anti-inflammatory and pro-autophagic effects. Both CBDV (200 mg/kg) and THCV (20 mg/kg) are effective in reducing LiCl-induced conditioned gaping in rats (Rock, et al., 2013b). In contrast, CB1 receptor inverse agonists such as SR141716 and AM251 are known to enhance nausea. Thus, CBDV and THCV do not function in vivo as CB1 receptor inverse agonists. In conclusion, CBDV has Δ9-THC-like, antiemetic effects in rodents consistent with a CB1 receptor agonist, but without the psychotropic activity of Δ9-THC.
THCV is derived from cannabigerovarin acid (CBGVA), one of the two primary minor cannabinoid precursors, the other being CBGA (Figure 1). THCA synthase converts CBGVA to THCVA, which is then decarboxylated to the neutral compound THCV when exposed to heat or light (Hanus, et al., 2016). THCV is typically found in very small amounts in cannabis flowers, though breeders are developing strains with higher concentrations. While THCV binds to both CB1 and CB2 receptors, its pharmacological effects remain controversial. In some studies, THCV has been reported to act as an antagonist/inverse agonist at the CB1 and CB2 receptors. In (35S)GTPγS binding assays measured with rodent brain preparations, THCV acts as an antagonist to WIN-55,212-2 (Dennis, et al., 2008). In addition, THCV antagonizes CP-55,940-induced stimulation of (35S)GTPγS binding in rodent brain and CHO cell membranes (Thomas, et al., 2005). In contrast, more recent studies using CHO cells have demonstrated that THCV functions as a partial agonist at the cannabinoid receptors to inhibit cAMP formation and to stimulate βarr2 recruitment (Zagzoog, et al., 2020). Computational docking experiments revealed that THCV interacts with the same residues as Δ9-THC in the orthosteric site of the CB1 receptor (Jung, et al., 2018). However, the pentyl side chain of Δ9-THC protrudes into a sub-pocket of the binding site. THCV containing a propyl side chain does not have this interaction (Jung, et al., 2018). This difference and the distinct binding energies of the two ligands might account for the higher affinity of Δ9-THC for the CB1 receptor.
As with other minor cannabinoids, THCV has off target actions at TRP channels and 5-HT1A receptors. THCV acts as an agonist at TRPV1-4 and TRPA1 channels, while acting as an antagonist at the TRPM8 channel (De Petrocellis, et al., 2011). THCV (at 100 nM) was found to enhance (H3)-8-OH-DPAT binding to the 5-HT1A receptor and to increase the potency of 8-OH-DPAT-stimulated (35S)GTPγS binding to cell membranes (Cascio, et al., 2015). Thus, both THCV and CBDA appear to function as positive allosteric regulators of the 5-HT1A receptor.
THCV has been shown to reduce inflammation and inflammatory pain in mice. THCV attenuated signs of inflammation induced by intraplantar injection of carrageenan in mouse hind paws and reduced hyperalgesia from formalin hind paw injection (Bolognini, et al., 2010). Surprisingly, the ability of THCV to relieve formalin-induced hyperalgesia was significantly attenuated by both the CB1 receptor-selective antagonist rimonabant, and CB2 receptor-selective antagonist SR144528 (Bolognini, et al., 2010). Thus, when tested in vivo, THCV exhibits both CB1 and CB2 receptor agonist activity. These anti-inflammatory actions of THCV were further supported by in vitro experiments using peritoneal-derived macrophages (Romano, et al., 2016). THCV was found to suppress inflammatory pathways by down-regulating LPS-induced expression of iNOS, COX-2 and interleukin 1β. THCV mediated suppression of nitrite production (from nitric oxide) was prevented by pretreatment of the macrophages with SR144528.
THCV has also shown promise as an anti-epileptic agent and in the treatment of neurodegeneration in Parkinson’s disease. Hill et al. (2010) used an extracellular multi-electrode array (MEA) assay to study the effects of THCV on spontaneous epileptiform bursting in rat brain slices. Pretreatment of the brain slices with THCV (20 µM) reduced burst complex incidence and the amplitude and frequency of paroxysmal depolarizing shifts (Hill, et al., 2010). In addition, in rats treated in vivo with pentylenetetrazole (PTZ) to induce seizures, THCV (0.25 mg/kg) reduced the incidence of seizures. Approximately 33% of animals treated with THCV exhibited a complete absence of PTZ seizures (compared with 13% of control treated rats). Garcia et al. (2011) reported that THCV reduces slow motor movements in rats with 6-hydroxydopamine (6-OHDA)-induced Parkinson’s disease. In addition, 2 weeks of treatment with THCV reduced microglial activation and preserved nigrostriatal dopaminergic neurons in the 6-OHDA Parkinson’s model (García, et al., 2011). Thus, THCV may be a useful treatment for Parkinson’s disease by preventing neuronal degradation and alleviating associated symptoms.
THCV regulates blood glucose levels suggesting it might be useful in weight reduction and treating diabetes. In mice with dietary-induced obesity (DIO), THCV improved fasting plasma glucose and glucose tolerance in a dose-dependent manner (Wargent, et al., 2013). In addition, THCV increased insulin sensitivity in genetically (ob/ob) obese mice. While THCV increased energy expenditure in the DIO and ob/ob mice, it did not reduce food intake or overall body weight (Wargent, et al., 2013). This contrasts with a previous study where intraperitoneal administration of THCV caused hypophagia and weight loss in rodents (Riedel, et al., 2009). Importantly, a clinical trial evaluated the effects of THCV and CBD on 62 subjects with type 2 diabetes (Jadoon, et al., 2016). Although THCV had no effect on plasma HDL levels, it significantly decreased fasting plasma glucose levels and improved pancreatic β-cell function in the type 2 patients. In addition to these findings, THCV was reported to increase the response to aversive stimuli in humans in regions of the brain (amygdala, insula and mid orbitofrontal cortex) involved in food aversion (Tudge, et al., 2015). This suggests that THCV may aid in appetite suppression and weight loss without the side effects (depression, anxiety, etc.,) caused by the CB1 receptor antagonist rimonabant (Mitchell and Morris, 2007).
Various minor cannabinoids including THCV, CBC, CBG and CBDV have shown promise in the treatment of skin disorders and are being investigated for the treatment of atopic dermatitis, psoriasis, scleroderma, acne hair growth and pigmentation disorders, keratin diseases, skin tumors, and pruritus (Tubaro, et al., 2010; Oláh, et al., 2016; Tóth, et al., 2019). It is postulated that these cannabinoids produce anti-acne effects by regulating homeostatic sebaceous lipogenesis and by exerting anti-proliferative and anti-inflammatory actions. In vitro experiments have shown that THCV inhibits the proliferation of human SZ95 sebocytes (Oláh, et al., 2016). This anti-proliferative effect of THCV occurs through a CBD-like mechanism of action; increasing intracellular Ca2+ and stimulating ERK1/2 following TRPV4 channel activation. In addition, THCV exhibits powerful anti-inflammatory properties by reducing levels of arachidonic acid (AA), needed for lipogenesis (Oláh, et al., 2016). THCV also suppresses lipid synthesis in the sebaceous glands, providing relief to acne sufferers whose condition is triggered by excessive oil production (Oláh, et al., 2016; Tóth, et al., 2019). In conclusion, THCV and other minor cannabinoids will continue to be evaluated for the management of acne.
Cannabitriol (CBT) and Δ8-tetrahydrocannabinol (Δ8-THC) are two rare cannabinoids that are gaining commercial popularity. Cannabitriol (CBT) was first isolated by Obata and Ishikawa, but its structure was not fully determined until 1977 (Obata and Ishikawa, 1966; ElSohly, et al., 1977). Although the pharmacology of CBT is largely unknown, recent virtual screening analysis of the estrogen receptor α (ER-α) indicate that CBT represents a novel estrogen antagonist that might be used for the prevention and treatment of breast cancer (Kikiowo, et al., 2021). Δ8-THC is an isomer of Δ9-THC that contains a double bond between carbon atoms 8 and 9. Unlike Δ9-THC, Δ8-THC is legally available in the U.S. through cannabis suppliers. The U.S. 2018 Farm Bill legalized cannabinoids such as CBD that are isolated from hemp. Since Δ8-THC can be derived from CBD, it is currently considered a legal natural product. While Δ8-THC displays roughly similar binding affinities as Δ9-THC to the CB1 and CB2 receptors (Husni, et al., 2014), preclinical results suggest that it is less potent in producing euphoric, anti-emetic and appetite-stimulating effects (Järbe and Henriksson, 1973; Hine, et al., 1977).
Preclinical data and early clinical studies support the continued investigation of phytocannabinoids for the treatment of pain, inflammation, neurodegeneration, cancer and other disorders (Figure 3). Natural products have historically been valuable sources of novel compounds developed into pharmaceuticals. Such was the case with the isolation of salicin from the bark of the Willow tree and the subsequent synthesis of aspirin. Δ9-THC (Dronabinol) is currently approved by the U.S. FDA for the treatment of nausea associated with cancer chemotherapy and as an appetite stimulant for patients with AIDS (Romero-Sandoval, et al., 2018; Fraguas-Sánchez and Torres-Suárez, 2018). Nabiximols (Sativex®) containing a mixture of Δ9-THC and CBD from the cannabis plant is approved in Canada and Europe for the treatment of MS spasticity (Fraguas-Sánchez and Torres-Suárez, 2018). It is also indicated for the treatment of neuropathic pain in MS and for pain relief in patients with advanced cancer (Fraguas-Sánchez and Torres-Suárez, 2018). However, use of Δ9-THC is associated with acute psychotropic effects including euphoria, sedation, anxiety, cognitive impairment, and in some patients, paranoia and hallucinations. Minor cannabinoids and their chemical homologs offer the potential medicinal benefits of Δ9-THC without adverse effects. Recently, Δ9-tetrahydrocannabiphorol (Δ9-THCP) and cannabidihexol (CBDH), homologs of Δ9-THC and CBD, were synthesized and shown to produce anti-nociceptive effects in mice at doses comparable to Δ9-THC (Citti, et al., 2019; Linciano, et al., 2020). Future studies will need to evaluate the risk versus benefit of these and other minor cannabinoids when compared to Δ9-THC and traditional analgesic drugs.
In addition to the CB1/CB2 receptors and “off target” binding sites described in this review, minor cannabinoids may bring about their pharmacological effects by interacting with other receptors and ion channels. Along with GPR55 and GPR18, de-orphanized receptors including GPR3, GPR6 and GPR12 are emerging as possible targets for minor cannabinoids (Laun and Song, 2017; Brown, et al., 2017). These receptors are highly expressed in neuronal tissues and are postulated to participate in neuroprotection, anti-nociception and brain development. Although the affinity of these receptors for minor cannabinoids has not yet been examined, CBD is known to function as an inverse agonist at all three receptors. However, it is unclear whether CBD binds to an orthostatic site on the receptor or if it modifies receptor activity via an allosteric site. While TRP channel agonism/antagonism provides a major mechanism of action for many of the minor cannabinoids, voltage-gated ion channels, such Na+ and Ca2+ channels are also regulated by cannabinoids. When tested in parathyroid cells, the synthetic cannabinoid WIN 55,212-2 and the endocannabinoid 2-AG reduce the peak Na+ current and shift the voltage-dependence of Na+ channel inactivation to more negative membrane potentials (Okada, et al., 2005). In addition, when applied at low micromolar concentrations, CBD inhibits the Na+ current in heterologous cells expressing various Na+ channel subunits (NaV1.1, NaV1.3 NaV1.6, etc.,) (Ghovanloo, et al., 2018). CBD also inhibits T-type Ca2+ channels (CaV3.x) in mouse sensory neurons (Ross, et al., 2008). Whether CBD acts directly to regulate the conduction of the Na+ and Ca2+ channels, or acts indirectly to alter the properties of the cell lipid membrane will require further investigation.
Advances in the bioengineering of cannabinoid synthesis enzymes in yeast and other microbial systems should expand the production of both natural and novel minor cannabinoids (Luo, et al., 2019). The ability to combine these cannabinoids with terpenes, flavonoids, polyphenols and other cannabis-based chemicals could create countless possibilities in the era of personalized healthcare. It is predicted that new cannabinoid products might be formulated to meet the therapeutic needs of different demographic groups and could be available in numerous delivery systems including topical creams, tablets, transdermal patches, vaporizers and more. Women represent one demographic group where cannabinoids could offer a variety of health care benefits. Cannabinoid receptors are ubiquitously distributed in reproductive tissues and AEA and the FAAH enzyme are found in the ovaries, oviducts and endometrium (Maia, et al., 2020). Cannabinoid-based suppositories containing Δ9-THC and CBD are already available for relieving menstrual cramps, and as drug discovery progresses, natural and unnatural cannabinoids may prove effective for reproductive system issues, from endometriosis and fibroids to perimenopause symptoms. Of course, the effectiveness of these cannabis products must first be confirmed through large, randomized and controlled clinical trials. Much of our current knowledge of the medicinal benefits of minor cannabinoids has come from subjective and anecdotal patient reporting, rather than through rigorous clinical trials. In order to move forward, researchers, clinicians and regulatory officials will need to work together to ensure that phytocannabinoid products meet the necessary therapeutic and safety standards.
KW, AM, and AH have all made a substantial, direct, and intellectual contribution to the work and approved it for publication.
This work was supported by U.S. Public Health Service award NS-071530 and U.S. National Science Foundation award CBET-1606882 to KW.
AH is employed by the company Precision Plant Molecules.
The remaining authors declare that the research was conducted in the absence of any commercial or financial relationships that could be construed as a potential conflict of interest.
All claims expressed in this article are solely those of the authors and do not necessarily represent those of their affiliated organizations, or those of the publisher, the editors and the reviewers. Any product that may be evaluated in this article, or claim that may be made by its manufacturer, is not guaranteed or endorsed by the publisher.
Adams, R., Hunt, M., and Clark, J. H. (1940a). Structure of Cannabidiol, a Product Isolated from the Marihuana Extract of Minnesota Wild Hemp. I. J. Am. Chem. Soc. 62, 196–200. doi:10.1021/ja01858a058
Adams, R., Pease, D. C., and Clark, J. H. (1940b). Isolation of Cannabinol, Cannabidiol and Quebrachitol from Red Oil of Minnesota Wild Hemp. J. Am. Chem. Soc. 62, 2194–2196. doi:10.1021/ja01865a080
Ahn, K. H., Mahmoud, M. M., Shim, J. Y., and Kendall, D. A. (2013). Distinct Roles of β-arrestin 1 and β-arrestin 2 in ORG27569-Induced Biased Signaling and Internalization of the Cannabinoid Receptor 1 (CB1). J. Biol. Chem. 288, 9790–9800. doi:10.1074/jbc.M112.438804
Aiken, C. T., Tobin, A. J., and Schweitzer, E. S. (2004). A Cell-Based Screen for Drugs to Treat Huntington's Disease. Neurobiol. Dis. 16, 546–555. doi:10.1016/j.nbd.2004.04.001
Alves, V. L., Gonçalves, J. L., Aguiar, J., Teixeira, H. M., and Câmara, J. S. (2020). The Synthetic Cannabinoids Phenomenon: from Structure to Toxicological Properties. A Review. Crit. Rev. Toxicol. 50, 359–382. doi:10.1080/10408444.2020.1762539
Amada, N., Yamasaki, Y., Williams, C. M., and Whalley, B. J. (2013). Cannabidivarin (CBDV) Suppresses Pentylenetetrazole (PTZ)-induced Increases in Epilepsy-Related Gene Expression. PeerJ 1, e214. doi:10.7717/peerj.214
Anavi-Goffer, S., Baillie, G., Irving, A. J., Gertsch, J., Greig, I. R., Pertwee, R. G., et al. (2012). Modulation of L-α-lysophosphatidylinositol/GPR55 Mitogen-Activated Protein Kinase (MAPK) Signaling by Cannabinoids. J. Biol. Chem. 287, 91–104. doi:10.1074/jbc.M111.296020
Anderson, L. L., Low, I. K., Banister, S. D., McGregor, I. S., and Arnold, J. C. (2019). Pharmacokinetics of Phytocannabinoid Acids and Anticonvulsant Effect of Cannabidiolic Acid in a Mouse Model of Dravet Syndrome. J. Nat. Prod. 82, 3047–3055. doi:10.1021/acs.jnatprod.9b00600
Anis, O., Vinayaka, A. C., Shalev, N., Namdar, D., Nadarajan, S., Anil, S. M., et al. (2021). Cannabis-Derived Compounds Cannabichromene and Δ9-Tetrahydrocannabinol Interact and Exhibit Cytotoxic Activity against Urothelial Cell Carcinoma Correlated with Inhibition of Cell Migration and Cytoskeleton Organization. Molecules 26, 465. doi:10.3390/molecules26020465
Appendino, G., Gibbons, S., Giana, A., Pagani, A., Grassi, G., Stavri, M., et al. (2008). Antibacterial Cannabinoids from Cannabis Sativa: a Structure-Activity Study. J. Nat. Prod. 71, 1427–1430. doi:10.1021/np8002673
Banister, S. D., Arnold, J. C., Connor, M., Glass, M., and McGregor, I. S. (2019). Dark Classics in Chemical Neuroscience: Δ9-Tetrahydrocannabinol. ACS Chem. Neurosci. 10, 2160–2175. doi:10.1021/acschemneuro.8b00651
Benson, M. J., Anderson, L. L., Low, I. K., Luo, J. L., Kevin, R. C., Zhou, C., et al. (2020). Evaluation of the Possible Anticonvulsant Effect of delta9-tetrahydrocannabinolic Acid in Murine Seizure Models. Cannabis Cannabinoid Res. online ahead of print.
Bolognini, D., Costa, B., Maione, S., Comelli, F., Marini, P., Di Marzo, V., et al. (2010). The Plant Cannabinoid delta9-tetrahydrocannabivarin Can Decrease Signs of Inflammation and Inflammatory Pain in Mice. Br. J. Pharmacol. 160, 677–687. doi:10.1111/j.1476-5381.2010.00756.x
Bolognini, D., Rock, E. M., Cluny, N. L., Cascio, M. G., Limebeer, C. L., Duncan, M., et al. (2013). Cannabidiolic Acid Prevents Vomiting in Suncus Murinus and Nausea-Induced Behaviour in Rats by Enhancing 5-HT1A Receptor Activation. Br. J. Pharmacol. 168, 1456–1470. doi:10.1111/bph.12043
Borrelli, F., Fasolino, I., Romano, B., Capasso, R., Maiello, F., Coppola, D., et al. (2013). Beneficial Effect of the Non-psychotropic Plant Cannabinoid Cannabigerol on Experimental Inflammatory Bowel Disease. Biochem. Pharmacol. 85, 1306–1316. doi:10.1016/j.bcp.2013.01.017
Borrelli, F., Pagano, E., Romano, B., Panzera, S., Maiello, F., Coppola, D., et al. (2014). Colon Carcinogenesis Is Inhibited by the TRPM8 Antagonist Cannabigerol, a Cannabis-Derived Non-psychotropic Cannabinoid. Carcinogenesis 35, 2787–2797. doi:10.1093/carcin/bgu205
Bouaboula, M., Poinot-Chazel, C., Bourrié, B., Canat, X., Calandra, B., Rinaldi-Carmona, M., et al. (1995). Activation of Mitogen-Activated Protein Kinases by Stimulation of the central Cannabinoid Receptor CB1. Biochem. J. 312 ( Pt 2), 637–641. doi:10.1042/bj3120637
Brierley, D. I., Samuels, J., Duncan, M., Whalley, B. J., and Williams, C. M. (2017). A Cannabigerol-Rich Cannabis Sativa Extract, Devoid of ∆9-tetrahydrocannabinol, Elicits Hyperphagia in Rats. Behav. Pharmacol. 28, 280–284. doi:10.1097/fbp.0000000000000285
Brodie, M. J., Czapinski, P., Pazdera, L., Sander, J. W., Toledo, M., Napoles, M., et al. (2021). A Phase 2 Randomized Controlled Trial of the Efficacy and Safety of Cannabidivarin as Add-On Therapy in Participants with Inadequately Controlled Focal Seizures. Cannabis Cannabinoid Res. online ahead of print. doi:10.1089/can.2020.0075
Brown, K. J., Laun, A. S., and Song, Z. H. (2017). Cannabidiol, a Novel Inverse Agonist for GPR12. Biochem. Biophys. Res. Commun. 493, 451–454. doi:10.1016/j.bbrc.2017.09.001
Carmona-Hidalgo, B., González-Mariscal, I., García-Martín, A., Prados, M. E., Ruiz-Pino, F., Appendino, G., et al. (2021). Δ9-Tetrahydrocannabinolic Acid Markedly Alleviates Liver Fibrosis and Inflammation in Mice. Phytomedicine 81, 153426. doi:10.1016/j.phymed.2020.153426
Carrillo-Salinas, F. J., Navarrete, C., Mecha, M., Feliú, A., Collado, J. A., Cantarero, I., et al. (2014). A Cannabigerol Derivative Suppresses Immune Responses and Protects Mice from Experimental Autoimmune Encephalomyelitis. PLoS ONE 9, e94733. doi:10.1371/journal.pone.0094733
Cascio, M. G., Gauson, L. A., Stevenson, L. A., Ross, R. A., and Pertwee, R. G. (2010). Evidence that the Plant Cannabinoid Cannabigerol Is a Highly Potent Alpha2-Adrenoceptor Agonist and Moderately Potent 5HT1A Receptor Antagonist. Br. J. Pharmacol. 159, 129–141. doi:10.1111/j.1476-5381.2009.00515.x
Cascio, M. G., Zamberletti, E., Marini, P., Parolaro, D., and Pertwee, R. G. (2015). The Phytocannabinoid, Δ9-tetrahydrocannabivarin, Can Act through 5-HT1Areceptors to Produce Antipsychotic Effects. Br. J. Pharmacol. 172, 1305–1318. doi:10.1111/bph.13000
Chesner, G. B., Jackson, D. M., and Stamer, G. A. (1974). Interaction of Cannabis and General Anesthetic Agents in Mice. Br. J Pharmacol 50, 593–599.
Citti, C., Linciano, P., Russo, F., Luongo, L., Iannotta, M., Maione, S., et al. (2019). A Novel Phytocannabinoid Isolated from Cannabis Sativa L. With an In Vivo Cannabimimetic Activity Higher Than Δ9-tetrahydrocannabinol: Δ9-Tetrahydrocannabiphorol. Sci. Rep. 9, 20335. doi:10.1038/s41598-019-56785-1
Corroon, J. (2021). Cannabinol and Sleep: Separating Fact from Fiction. Cannabis Cannabinoid Res6, 366–371. doi:10.1089/can.2021.0006
Covelo, A., Eraso-Pichot, A., Fernández-Moncada, I., Serrat, R., and Marsicano, G. (2021). CB1R-dependent Regulation of Astrocyte Physiology and Astrocyte-Neuron Interactions. Neuropharmacol 195, 108678. doi:10.1089/can.2021.0006
D'Aniello, E., Fellous, T., Iannotti, F. A., Gentile, A., Allarà, M., Balestrieri, F., et al. (2019). Identification and Characterization of Phytocannabinoids as Novel Dual PPARα/γ Agonists by a Computational and In Vitro Experimental Approach. Biochim. Biophys. Acta Gen. Subj 1863, 586–597. doi:10.1016/j.bbagen.2019.01.002
de Almeida, D. L., and Devi, L. A. (2020). Diversity of Molecular Targets and Signaling Pathways for CBD. Pharmacol. Res. Perspect. 8, e00682. doi:10.1002/prp2.682
de Meijer, E. P. M., Hammond, K. M., and Micheler, M. (2009). The Inheritance of Chemical Phenotype in Cannabis Sativa L. (III): Variation in Cannabichromene Proportion. Euphytica 165, 293–311. doi:10.1007/s10681-008-9787-1
de Meijer, E. P. M., and Hammond, K. M. (2005). The Inheritance of Chemical Phenotype in Cannabis Sativa L. (II): Cannabigerol Predominant Plants. Euphytica 145, 189–198. doi:10.1007/s10681-005-1164-8
De Petrocellis, L., Ligresti, A., Moriello, A. S., Allarà, M., Bisogno, T., Petrosino, S., et al. (2011). Effects of Cannabinoids and Cannabinoid-Enriched Cannabis Extracts on TRP Channels and Endocannabinoid Metabolic Enzymes. Br. J. Pharmacol. 163, 1479–1494. doi:10.1111/j.1476-5381.2010.01166.x
De Petrocellis, L., Melck, D., Palmisano, A., Bisogno, T., Laezza, C., Bifulco, M., et al. (1998). The Endogenous Cannabinoid Anandamide Inhibits Human Breast Cancer Cell Proliferation. Proc. Natl. Acad. Sci. U S A. 95, 8375–8380. doi:10.1073/pnas.95.14.8375
De Petrocellis, L., Schiano Moriello, A., Imperatore, R., Cristino, L., Starowicz, K., and Di Marzo, V. (2012). A Re-evaluation of 9-HODE Activity at TRPV1 Channels in Comparison with Anandamide: Enantioselectivity and Effects at Other TRP Channels and in Sensory Neurons. Br. J. Pharmacol. 167, 1643–1651. doi:10.1111/j.1476-5381.2012.02122.x
De Petrocellis, L., Starowicz, K., Moriello, A. S., Vivese, M., Orlando, P., and Di Marzo, V. (2007). Regulation of Transient Receptor Potential Channels of Melastatin Type 8 (TRPM8): Effect of cAMP, Cannabinoid CB(1) Receptors and Endovanilloids. Exp. Cel Res 313, 1911–1920. doi:10.1016/j.yexcr.2007.01.008
De Petrocellis, L., Vellani, V., Schiano-Moriello, A., Marini, P., Magherini, P. C., Orlando, P., et al. (2008). Plant-derived Cannabinoids Modulate the Activity of Transient Receptor Potential Channels of Ankyrin Type-1 and Melastatin Type-8. J. Pharmacol. Exp. Ther. 325, 1007–1015. doi:10.1124/jpet.107.134809
DeLong, G. T., Wolf, C. E., Poklis, A., and Lichtman, A. H. (2010). Pharmacological Evaluation of the Natural Constituent of Cannabis Sativa, Cannabichromene and its Modulation by Δ(9)-tetrahydrocannabinol. Drug Alcohol Depend 112, 126–133. doi:10.1016/j.drugalcdep.2010.05.019
Dennis, I., Whalley, B. J., and Stephens, G. J. (2008). Effects of Delta9-tetrahydrocannabivarin on [35S]GTPgammaS Binding in Mouse Brain Cerebellum and Piriform Cortex Membranes. Br. J. Pharmacol. 154, 1349–1358. doi:10.1038/bjp.2008.190
Derkinderen, P., Valjent, E., Toutant, M., Corvol, J. C., Enslen, H., Ledent, C., et al. (2003). Regulation of Extracellular Signal-Regulated Kinase by Cannabinoids in hippocampus. J. Neurosci. 23, 2371–2382. doi:10.1523/jneurosci.23-06-02371.2003
Devane, W. A., Dysarz, F. A., Johnson, M. R., Melvin, L. S., and Howlett, A. C. (1988). Determination and Characterization of a Cannabinoid Receptor in Rat Brain. Mol. Pharmacol. 34, 605–613.
Devane, W. A., Hanus, L., Breuer, A., Pertwee, R. G., Stevenson, L. A., Griffin, G., et al. (1992). Isolation and Structure of a Brain Constituent that Binds to the Cannabinoid Receptor. Science 258, 1946–1949. doi:10.1126/science.1470919
ElSohly, M. A., El-Feraly, F. S., and Turner, C. E. (1977). Isolation and Characterization of (+)-cannabitriol and (-)-10-Ethoxy-9-Hydroxy-delta 6a[10a]-Tetrahydrocannabinol: Two New Cannabinoids from Cannabis Sativa L. Extract. Lloydia 40, 275–280.
ElSohly, M. A., Harland, E., Murphy, J. C., Wirth, P., and Waller, C. W. (1981). Cannabinoids in Glaucoma: A Primary Screening Procedure. J. Clin. Pharmacol. 21, 472S–478S. doi:10.1002/j.1552-4604.1981.tb02627.x
Farrimond, J. A., Whalley, B. J., and Williams, C. M. (2012a). Cannabinol and Cannabidiol Exert Opposing Effects on Rat Feeding Patterns. Psychopharmacology (Berl) 223, 117–129. doi:10.1007/s00213-012-2697-x
Farrimond, J. A., Whalley, B. J., and Williams, C. M. (2012b). Non-Δ⁹tetrahydrocannabinol Phytocannabinoids Stimulate Feeding in Rats. Behav. Pharmacol. 23, 113–117. doi:10.1097/FBP.0b013e32834ed832
Fernandes, M., Schabarek, A., Coper, H., and Hill, R. (1974). Modification of delta9-THC-actions by Cannabinol and Cannabidiol in the Rat. Psychopharmacologia 38, 329–338. doi:10.1007/BF00429130
Finlay, D. B., Sircombe, K. J., Nimick, M., Jones, C., and Glass, M. (2020). Terpenoids from Cannabis Do Not Mediate an Entourage Effect by Acting at Cannabinoid Receptors. Front. Pharmacol. 11, 359. doi:10.3389/fphar.2020.00359
Fraguas-Sánchez, A. I., and Torres-Suárez, A. I. (2018). Medical Use of Cannabinoids. Drugs 78, 1665–1703.
Galiègue, S., Mary, S., Marchand, J., Dussossoy, D., Carrière, D., Carayon, P., et al. (1995). Expression of central and Peripheral Cannabinoid Receptors in Human Immune Tissues and Leukocyte Subpopulations. Eur. J. Biochem. 232, 54–61. doi:10.1111/j.1432-1033.1995.tb20780.x
Galve-Roperh, I., Rueda, D., Gómez del Pulgar, T., Velasco, G., and Guzmán, M. (2002). Mechanism of Extracellular Signal-Regulated Kinase Activation by the CB(1) Cannabinoid Receptor. Mol. Pharmacol. 62, 1385–1392. doi:10.1124/mol.62.6.1385
Gao, Q., Hanh, J., Váradi, L., Cairns, R., Sjöström, H., Liao, V. W., et al. (2015). Identification of Dual PPARα/γ Agonists and Their Effects on Lipid Metabolism. Bioorg. Med. Chem. 23, 7676–7684. doi:10.1016/j.bmc.2015.11.013
Gaoni, Y., and Mechoulam, R. (1971). The Isolation and Structure of delta-1-tetrahydrocannabinol and Other Neutral Cannabinoids from Hashish. J. Am. Chem. Soc. 93, 217–224. doi:10.1021/ja00730a036
Gaoni, Y., and Mechoulam, R. (1966). Cannabichromene, a New Active Principle in Hashish. Chem. Commun. (London) 1, 20–21. doi:10.1039/c19660000020
García, C., Palomo-Garo, C., García-Arencibia, M., Ramos, J. A., Pertwee, R. G., and Fernández-Ruiz, J. (2011). Symptom-relieving and Neuroprotective Effects of the Phytocannabinoid delta9-THCV in Animal Models of Parkinson's Disease. Br. J Pharmacol 163, 1495–1506.
Garfinkel, A. R., Otten, M., and Crawford, S. (2021). SNP in Potentially Defunct Tetrahydrocannabinolic Acid Synthase Is a Marker for Cannabigerolic Acid Dominance in Cannabis Sativa L. Genes (Basel) 12, 228. doi:10.3390/genes12020228
Ghovanloo, M. R., Shuart, N. G., Mezeyova, J., Dean, R. A., Ruben, P. C., and Goodchild, S. J. (2018). Inhibitory Effects of Cannabidiol on Voltage-dependent Sodium Currents. J. Biol. Chem. 293, 16546–16558. doi:10.1074/jbc.RA118.004929
Glass, M., and Northup, J. K. (1999). Agonist Selective Regulation of G Proteins by Cannabinoid CB(1) and CB(2) Receptors. Mol. Pharmacol. 56, 1362–1369. doi:10.1124/mol.56.6.1362
Goerl, B., Watkins, S., Metcalf, C., Smith, M., and Beenhakker, M. (2021). Cannabidiolic Acid Exhibits Entourage-like Improvements of Anticonvulsant Activity in an Acute Rat Model of Seizures. Epilepsy Res. 169, 106525. doi:10.1016/j.eplepsyres.2020.106525
Gonsiorek, W., Lunn, C., Fan, X., Narula, S., Lundell, D., and Hipkin, R. W. (2000). Endocannabinoid 2-arachidonyl Glycerol Is a Full Agonist through Human Type 2 Cannabinoid Receptor: Antagonism by Anandamide. Mol. Pharmacol. 57, 1045–1050.
Gugliandolo, A., Pollastro, F., Grassi, G., Bramanti, P., and Mazzon, E. (2018). In Vitro model of Neuroinflammation: Efficacy of Cannabigerol, a Non-psychoactive Cannabinoid. Int. J. Mol. Sci. 19, 1992. doi:10.3390/ijms19071992
Gülck, T., and Möller, B. L. (2020). Phytocannabinoids: Origins and Biosynthesis. Trends Plant Sci. 25, 985–1004.
Guo, J., and Ikeda, S. R. (2004). Endocannabinoids Modulate N-type Calcium Channels and G-Protein-Coupled Inwardly Rectifying Potassium Channels via CB1 Cannabinoid Receptors Heterologously Expressed in Mammalian Neurons. Mol. Pharmacol. 65, 665–674. doi:10.1124/mol.65.3.665
Hanus, L. O., Meyer, S. M., Muñoz, E., Taglialatela-Scafati, O., and Appendino, G. (2016). Phytocannabinoids: a Unified Critical Inventory. Nat. Prod. Rep. 33, 1357–1392.
Henstidge, C. M., Balenga, N. A. B., Ford, L. A., Ross, R. A., Waldhoer, M., and Irving, A. J. (2009). The GPR55 Ligand L-α-Lysophosphatidylinositol Promotes RhoA-dependent Ca2+ Signaling and NFAT Activation. FASEB J. 23, 183–193.
Herkenham, M., Lynn, A. B., Little, M. D., Johnson, M. R., Melvin, L. S., de Costa, B. R., et al. (1990). Cannabinoid Receptor Localization in Brain. Proc. Natl. Acad. Sci. U S A. 87, 1932–1936. doi:10.1073/pnas.87.5.1932
Hill, A. J., Mercier, M. S., Hill, T. D., Glyn, S. E., Jones, N. A., Yamasaki, Y., et al. (2012). Cannabidivarin Is Anticonvulsant in Mouse and Rat. Br. J. Pharmacol. 167, 1629–1642. doi:10.1111/j.1476-5381.2012.02207.x
Hill, A. J., Weston, S. E., Jones, N. A., Smith, I., Bevan, S. A., Williamson, E. M., et al. (2010). Δ⁹-Tetrahydrocannabivarin Suppresses In Vitro Epileptiform and In Vivo Seizure Activity in Adult Rats. Epilepsia 51, 1522–1532. doi:10.1111/j.1528-1167.2010.02523.x
Hillig, K. W., and Mahlberg, P. G. (2004). A Chemotaxonomic Analysis of Cannabinoid Variation in Cannabis (Cannabaceae). Am. J. Bot. 91, 966–975. doi:10.3732/ajb.91.6.966
Hine, B., Toreelio, M., and Gershon, S. (1977). Analgesic, Heart Rate, and Temperature Effects of delta8-THC during Acute and Chronic Administration to Conscious Rats. Pharmacology 15, 63–72. doi:10.1159/000136664
Howlett, A. C., Qualy, J. M., and Khachatrian, L. L. (1986). Involvement of Gi in the Inhibition of Adenylate Cyclase by Cannabimimetic Drugs. Mol. Pharmacol. 29, 307–313.
Hua, T., Vemuri, K., Nikas, S. P., Laprairie, R. B., Wu, Y., Qu, L., et al. (2017). Crystal Structures of Agonist-Bound Human Cannabinoid Receptor CB1. Nature 547, 468–471. doi:10.1038/nature23272
Husni, A. S., McCurdy, C. R., Radwan, M. M., Ahmed, S. A., Slade, D., Ross, S. A., et al. (2014). Evaluation of Phytocannabinoids from High Potency Cannabis Sativa Using In Vitro Bioassays to Determine Structure-Activity Relationships for Cannabinoid Receptor 1 and Cannabinoid Receptor 2. Med. Chem. Res. 23, 4295–4300. doi:10.1007/s00044-014-0972-6
Iannotti, F. A., Pagano, E., Moriello, A. S., Alvino, F. G., Sorrentino, N. C., D'Orsi, L., et al. (2019). Effects of Non-euphoric Plant Cannabinoids on Muscle Quality and Performance of Dystrophic Mdx Mice. Br. J. Pharmacol. 176, 1568–1584. doi:10.1111/bph.14460
Izzo, A. A., Capasso, R., Aviello, G., Borrelli, F., Romano, B., Piscitelli, F., et al. (2012). Inhibitory Effect of Cannabichromene, a Major Non-psychotropic Cannabinoid Extracted from Cannabis Sativa, on Inflammation-Induced Hypermotility in Mice. Br. J. Pharmacol. 166, 1444–1460. doi:10.1111/j.1476-5381.2012.01879.x
Jadoon, K. A., Ratcliffe, S. H., Barrett, D. A., Thomas, E. L., Stott, C., Bell, J. D., et al. (2016). Efficacy and Safety of Cannabidiol and Tetrahydrocannabivarin on Glycemic and Lipid Parameters in Patients with Type 2 Diabetes: A Randomized, Double-Blind, Placebo-Controlled, Parallel Group Pilot Study. Diabetes Care 39, 1777–1786. doi:10.2337/dc16-0650
Jan, T. R., Farraj, A. K., Harkema, J. R., and Kaminski, N. E. (2003). Attenuation of the Ovalbumin-Induced Allergic Airway Response by Cannabinoid Treatment in A/J Mice. Toxicol. Appl. Pharmacol. 188, 24–35. doi:10.1016/s0041-008x(03)00010-3
Järbe, T. U., and Henriksson, B. G. (1973). Effects of delta8-THC, and delta9-THC on the Acquisition of a Discriminative Positional Habit in Rats. Psychopharmacologia 31, 321–332.
Jin, W., Brown, S., Roche, J. P., Hsieh, C., Celver, J. P., Kovoor, A., et al. (1999). Distinct Domains of the CB1 Cannabinoid Receptor Mediate Desensitization and Internalization. J. Neurosci. 19, 3773–3780. doi:10.1523/jneurosci.19-10-03773.1999
Jung, S. W., Cho, A. E., and Yu, W. (2018). Exploring the Ligand Efficacy of Cannabinoid Receptor 1 (CB1) Using Molecular Dynamics Simulations. Sci. Rep. 8, 13787. doi:10.1038/s41598-018-31749-z
Karler, R., and Turkanis, S. A. (1979). “Cannabis and Epilepsy,” in Marihuana Biological Effects. Editors G. G. Nahas, and W. D. M. Paton (Pergamon), 619–641. doi:10.1016/b978-0-08-023759-6.50052-4
Karniol, I. G., Shirakawa, I., Takahashi, R. N., Knobel, E., and Musty, R. E. (1975). Effects of delta9-tetrahydrocannabinol and Cannabinol in Man. Pharmacology 13, 502–512. doi:10.1159/000136944
Khan, S. P., Pickens, T. A., and Berlau, D. J. (2019). Perspectives on Cannabis as a Substitute for Opioid Analgesics. Pain Manag. 9, 191–203. doi:10.2217/pmt-2018-0051
Kikiowo, B., Ogunleye, A. J., Iwaloye, O., Ijatuyi, T., Adelakun, N. S., and Alashe, W. O. (2021). Induced Fit Docking and Automated QSAR Studies Reveal the ER-α Inhibitory Activity of Cannabis Sativa in Breast Cancer. Recent Pat Anti-cancer Drug Discov. 16, 1–12. doi:10.2174/1574892816666210201115359
Kohno, M., Hasegawa, H., Inoue, A., Muraoka, M., Miyazaki, T., Oka, K., et al. (2006). Identification of N-Arachidonylglycine as the Endogenous Ligand for Orphan G-Protein-Coupled Receptor GPR18. Biochem. Biophys. Res. Commun. 347, 827–832. doi:10.1016/j.bbrc.2006.06.175
Kumar, K., Shalev-Benami, M., Robertson, M. J., Hu, H., Banister, S. D., Hollingsworth, S. A., et al. (2019). Structure of a Signaling Cannabinoid Receptor 1-G Protein Complex. Cell 176, 448–e12. doi:10.1016/j.cell.2018.11.040
Lauckner, J. E., Hille, B., and Mackie, K. (2005). The Cannabinoid Agonist WIN55,212-2 Increases Intracellular Calcium via CB1 Receptor Coupling to Gq/11 G Proteins. Proc. Natl. Acad. Sci. U S A. 102, 19144–19149. doi:10.1073/pnas.0509588102
Laun, A. S., Shrader, S. H., and Song, Z. H. (2018). Novel Inverse Agonists for the Orphan G Protein-Coupled Receptor 6. Heliyon 4, e00933. doi:10.1016/j.heliyon.2018.e00933
Laun, A. S., and Song, Z. H. (2017). GPR3 and GPR6, Novel Molecular Targets for Cannabidiol. Biochem. Biophys. Res. Commun. 490, 17–21. doi:10.1016/j.bbrc.2017.05.165
Ligresti, A., Moriello, A. S., Starowicz, K., Matias, I., Pisanti, S., De Petrocellis, L., et al. (2006). Antitumor Activity of Plant Cannabinoids with Emphasis on the Effect of Cannabidiol on Human Breast Carcinoma. J. Pharmacol. Exp. Ther. 318, 1375–1387. doi:10.1124/jpet.106.105247
Limebeer, C. L., Abdullah, R. A., Rock, E. M., Imhof, E., Wang, K., Lichtman, A. H., et al. (2014). Attenuation of Anticipatory Nausea in a Rat Model of Contextually Elicited Conditioned Gaping by Enhancement of the Endocannabinoid System. Psychopharmacology (Berl) 231, 603–612. doi:10.1007/s00213-013-3282-7
Linciano, P., Citti, C., Russo, F., Tolomeo, F., Laganà, A., Capriotti, A. L., et al. (2020). Identification of a New Cannabidiol N-Hexyl Homolog in a Medicinal Cannabis Variety with an Antinociceptive Activity in Mice: Cannabidihexol. Sci. Rep. 10, 22019. doi:10.1038/s41598-020-79042-2
Lu, H. C., and Mackie, K. (2016). An Introduction to the Endogenous Cannabinoid System. Biol. Psychiatry 79, 516–525. doi:10.1016/j.biopsych.2015.07.028
Luk, T., Jin, W., Zvonok, A., Lu, D., Lin, X. Z., Chavkin, C., et al. (2004). Identification of a Potent and Highly Efficacious, yet Slowly Desensitizing CB1 Cannabinoid Receptor Agonist. Br. J. Pharmacol. 142, 495–500. doi:10.1038/sj.bjp.0705792
Luo, X., Reiter, M. A., d'Espaux, L., Wong, J., Denby, C. M., Lechner, A., et al. (2019). Complete Biosynthesis of Cannabinoids and Their Unnatural Analogues in Yeast. Nature 567, 123–126. doi:10.1038/s41586-019-0978-9
Mackie, K., Lai, Y., Westenbroek, R., and Mitchell, R. (1995). Cannabinoids Activate an Inwardly Rectifying Potassium Conductance and Inhibit Q-type Calcium Currents in AtT20 Cells Transfected with Rat Brain Cannabinoid Receptor. J. Neurosci. 15, 6552–6561. doi:10.1523/jneurosci.15-10-06552.1995
Mahadevan, A., Siegel, C., Martin, B. R., Abood, M. E., Beletskaya, I., and Razdan, R. K. (2000). Novel Cannabinol Probes for CB1 and CB2 Cannabinoid Receptors. J. Med. Chem. 43, 3778–3785. doi:10.1021/jm0001572
Maia, J., Fonseca, B. M., Teixeira, N., and Correia-da-Silva, G. (2020). The Fundamental Role of the Endocannabinoid System in Endometrium and Placenta: Implications in Pathophysiological Aspects of Uterine and Pregnancy Disorders. Hum. Reprod. Update 26, 586–602. doi:10.1093/humupd/dmaa005
Maione, S., Piscitelli, F., Gatta, L., Vita, D., De Petrocellis, L., Palazzo, E., et al. (2011). Non-psychoactive Cannabinoids Modulate the Descending Pathway of Antinociception in Anaesthetized Rats through Several Mechanisms of Action. Br. J. Pharmacol. 162, 584–596. doi:10.1111/j.1476-5381.2010.01063.x
Martin, B. R., Jefferson, R., Winckler, R., Wiley, J. L., Huffman, J. W., Crocker, P. J., et al. (1999). Manipulation of the Tetrahydrocannabinol Side Chain Delineates Agonists, Partial Agonists, and Antagonists. J. Pharmacol. Exp. Ther. 290, 1065–1079.
Matsuda, L. A., Lolait, S. J., Brownstein, M. J., Young, A. C., and Bonner, T. I. (1990). Structure of a Cannabinoid Receptor and Functional Expression of the Cloned cDNA. Nature 346, 561–564. doi:10.1038/346561a0
McPartland, J. M., MacDonald, C., Young, M., Grant, P. S., Furkert, D. P., and Glass, M. (2017). Affinity and Efficacy Studies of Tetrahydrocannabinolic Acid A at Cannabinoid Receptor Types One and Two. Cannabis Cannabinoid Res. 2, 87–95. doi:10.1089/can.2016.0032
Mechoulam, R., Ben-Shabat, S., Hanus, L., Ligumsky, M., Kaminski, N. E., Schatz, A. R., et al. (1995). Identification of an Endogenous 2-monoglyceride, Present in Canine Gut, that Binds to Cannabinoid Receptors. Biochem. Pharmacol. 50, 83–90. doi:10.1016/0006-2952(95)00109-d
Mechoulam, R., and Gaoni, Y. (1965). Hashish. IV. The Isolation and Structure of Cannabinolic Cannabidiolic and Cannabigerolic Acids. Tetrahedron 21, 1223–1229. doi:10.1016/0040-4020(65)80064-3
Mechoulam, R., and Shvo, Y. (1963). Hashish. I. The Structure of Cannabidiol. Tetrahedron 19, 2073–2078. doi:10.1016/0040-4020(63)85022-x
Mitchell, P. B., and Morris, M. J. (2007). Depression and Anxiety with Rimonabant. Lancet 370, 1671–1672. doi:10.1016/S0140-6736(07)61705-X
Moldzio, R., Pacher, T., Krewenka, C., Kranner, B., Novak, J., Duvigneau, J. C., et al. (2012). Effects of Cannabinoids Δ(9)-tetrahydrocannabinol, Δ(9)-tetrahydrocannabinolic Acid and Cannabidiol in MPP+ Affected Murine Mesencephalic Cultures. Phytomedicine 19, 819–824. doi:10.1016/j.phymed.2012.04.002
Mouro, F. M., Miranda-Lourenço, C., Sebastião, A. M., and Diógenes, M. J. (2019). From Cannabinoids and Neurosteroids to Statins and the Ketogenic Diet: New Therapeutic Avenues in Rett Syndrome? Front. Neurosci. 13, 680. doi:10.3389/fnins.2019.00680
Munro, S., Thomas, K. L., and Abu-Shaar, M. (1993). Molecular Characterization of a Peripheral Receptor for Cannabinoids. Nature 365, 61–65. doi:10.1038/365061a0
Nadal, X., Del Río, C., Casano, S., Palomares, B., Ferreiro-Vera, C., Navarrete, C., et al. (2017). Tetrahydrocannabinolic Acid Is a Potent PPARγ Agonist with Neuroprotective Activity. Br. J. Pharmacol. 174, 4263–4276. doi:10.1111/bph.14019
Nallathambi, R., Mazuz, M., Ion, A., Selvaraj, G., Weininger, S., Fridlender, M., et al. (2017). Anti-Inflammatory Activity in Colon Models Is Derived from Δ9-Tetrahydrocannabinolic Acid that Interacts with Additional Compounds in Cannabis Extracts. Cannabis Cannabinoid Res. 2, 167–182. doi:10.1089/can.2017.0027
Nallathambi, R., Mazuz, M., Namdar, D., Shik, M., Namintzer, D., Vinayaka, A. C., et al. (2018). Identification of Synergistic Interaction between Cannabis-Derived Compounds for Cytotoxic Activity in Colorectal Cancer Cell Lines and colon Polyps that Induces Apoptosis-Related Cell Death and Distinct Gene Expression. Cannabis Cannabinoid Res. 3, 120–135. doi:10.1089/can.2018.0010
Navarro, G., Varani, K., Lillo, A., Vincenzi, F., Rivas-Santisteban, R., Raïch, I., et al. (2020). Pharmacological Data of Cannabidiol- and Cannabigerol-type Phytocannabinoids Acting on Cannabinoid CB1, CB2 and CB1/CB2 Heteromer Receptors. Pharmacol. Res. 159, 104940. doi:10.1016/j.phrs.2020.104940
Navarro, G., Varani, K., Reyes-Resina, I., Sánchez de Medina, V., Rivas-Santisteban, R., Sánchez-Carnerero Callado, C., et al. (2018). Cannabigerol Action at Cannabinoid CB1 and CB2 Receptors and at CB1-CB2 Heteroreceptor Complexes. Front. Pharmacol. 9, 632. doi:10.3389/fphar.2018.00632
O'Sullivan, S. E. (2007). Cannabinoids Go Nuclear: Evidence for Activation of Peroxisome Proliferator-Activated Receptors. Br. J. Pharmacol. 152, 576–582. doi:10.1038/sj.bjp.0707423
Obata, Y., and Ishikawa, Y. (1966). Studies on the Constituents of Hemp Plant (Cannabis sativaL). Agric. Biol. Chem. 30, 619–620. doi:10.1080/00021369.1966.10858651
Okada, Y., Imendra, K. G., Miyazaki, T., Hotokezaka, H., Fujiyama, R., Zeredo, J. L., et al. (2005). Biophysical Properties of Voltage-Gated Na+ Channels in Frog Parathyroid Cells and Their Modulation by Cannabinoids. J. Exp. Biol. 208, 4747–4756. doi:10.1242/jeb.01967
Oláh, A., Markovics, A., Szabó-Papp, J., Szabó, P. T., Stott, C., Zouboulis, C. C., et al. (2016). Differential Effectiveness of Selected Non-psychotropic Phytocannabinoids on Human Sebocyte Functions Implicates Their Introduction in Dry/seborrhoeic Skin and Acne Treatment. Exp. Dermatol. 25, 701–707.
Pagano, E., Iannotti, F. A., Piscitelli, F., Romano, B., Lucariello, G., Venneri, T., et al. (2021). Efficacy of Combined Therapy with Fish Oil and Phytocannabinoids in Murine Intestinal Inflammation. Phytother Res. 35, 517–529. doi:10.1002/ptr.6831
Palomares, B., Ruiz-Pino, F., Garrido-Rodriguez, M., Eugenia Prados, M., Sánchez-Garrido, M. A., Velasco, I., et al. (2020). Tetrahydrocannabinolic Acid A (THCA-A) Reduces Adiposity and Prevents Metabolic Disease Caused by Diet-Induced Obesity. Biochem. Pharmacol. 171, 113693. doi:10.1016/j.bcp.2019.113693
Patsos, H. A., Hicks, D. J., Dobson, R. R., Greenhough, A., Woodman, N., Lane, J. D., et al. (2005). The Endogenous Cannabinoid, Anandamide, Induces Cell Death in Colorectal Carcinoma Cells: a Possible Role for Cyclooxygenase 2. Gut 54, 1741–1750. doi:10.1136/gut.2005.073403
Pennypacker, S. D., and Romero-Sandoval, E. A. (2020). CBD and THC: Do They Complement Each Other like Yin and Yang? Pharmacotherapy 40, 1152–1165. doi:10.1002/phar.2469
Pertwee, R. G., Rock, E. M., Guenther, K., Limebeer, C. L., Stevenson, L. A., Haj, C., et al. (2018). Cannabidiolic Acid Methyl Ester, a Stable Synthetic Analogue of Cannabidiolic Acid, Can Produce 5-HT1A Receptor-Mediated Suppression of Nausea and Anxiety in Rats. Br. J. Pharmacol. 175, 100–112. doi:10.1111/bph.14073
Pisanti, S., and Bifulco, M. (2019). Medical Cannabis: A Plurimillennial History of an evergreen. J. Cel Physiol 234, 8342–8351. doi:10.1002/jcp.27725
Rhee, M. H., Vogel, Z., Barg, J., Bayewitch, M., Levy, R., Hanus, L., et al. (1997). Cannabinol Derivatives: Binding to Cannabinoid Receptors and Inhibition of Adenylylcyclase. J. Med. Chem. 40, 3228–3233. doi:10.1021/jm970126f
Riedel, G., Fadda, P., McKillop-Smith, S., Pertwee, R. G., Platt, B., and Robinson, L. (2009). Synthetic and Plant-Derived Cannabinoid Receptor Antagonists Show Hypophagic Properties in Fasted and Non-fasted Mice. Br. J. Pharmacol. 156, 1154–1166. doi:10.1111/j.1476-5381.2008.00107.x
Rock, E. M., Kopstick, R. L., Limebeer, C. L., and Parker, L. A. (2013a). Tetrahydrocannabinolic Acid Reduces Nausea-Induced Conditioned Gaping in Rats and Vomiting in Suncus Murinus. Br. J. Pharmacol. 170, 641–648. doi:10.1111/bph.12316
Rock, E. M., Limebeer, C. L., and Parker, L. A. (2018). Effect of Cannabidiolic Acid and ∆9-tetrahydrocannabinol on Carrageenan-Induced Hyperalgesia and Edema in a Rodent Model of Inflammatory Pain. Psychopharmacology (Berl) 235, 3259–3271. doi:10.1007/s00213-018-5034-1
Rock, E. M., and Parker, L. A. (2013). Suppression of Lithium Chloride-Induced Conditioned Gaping (A Model of Nausea-Induced Behaviour) in Rats (Using the Taste Reactivity Test) with Metoclopramide Is Enhanced by Cannabidiolic Acid. Pharmacol. Biochem. Behav. 111, 84–89. doi:10.1016/j.pbb.2013.08.012
Rock, E. M., Sticht, M. A., Duncan, M., Stott, C., and Parker, L. A. (2013b). Evaluation of the Potential of the Phytocannabinoids, Cannabidivarin (CBDV) and Δ(9) -tetrahydrocannabivarin (THCV), to Produce CB1 Receptor Inverse Agonism Symptoms of Nausea in Rats. Br. J. Pharmacol. 170, 671–678. doi:10.1111/bph.12322
Rock, E. M., Sullivan, M. T., Collins, S. A., Goodman, H., Limebeer, C. L., Mechoulam, R., et al. (2020). Evaluation of Repeated or Acute Treatment with Cannabidiol (CBD), Cannabidiolic Acid (CBDA) or CBDA Methyl Ester (HU-580) on Nausea And/or Vomiting in Rats and Shrews. Psychopharmacology (Berl) 237, 2621–2631. doi:10.1007/s00213-020-05559-z
Romano, B., Borrelli, F., Fasolino, I., Capasso, R., Piscitelli, F., Cascio, M., et al. (2013). The Cannabinoid TRPA1 Agonist Cannabichromene Inhibits Nitric Oxide Production in Macrophages and Ameliorates Murine Colitis. Br. J. Pharmacol. 169, 213–229. doi:10.1111/bph.12120
Romano, B., Pagano, E., Orlando, P., Capasso, R., Cascio, M. G., Pertwee, R., et al. (2016). Pure Δ9-tetrahydrocannabivarin and a Cannabis Sativa Extract with High Content in Δ9-tetrahydrocannabivarin Inhibit Nitrite Production in Murine Peritoneal Macrophages. Pharmacol. Res. 113, 199–208. doi:10.1016/j.phrs.2016.07.045
Romero-Sandoval, E. A., Fincham, J. E., Kolano, A. L., Sharpe, B. N., and Alvarado-Vázquez, P. A. (2018). Cannabis for Chronic Pain: Challenges and Considerations. Pharmacotherapy 38, 651–662. doi:10.1002/phar.2115
Rosenthaler, S., Pöhn, B., Kolmanz, C., Huu, C. N., Krewenka, C., Huber, A., et al. (2014). Differences in Receptor Binding Affinity of Several Phytocannabinoids Do Not Explain Their Effects on Neural Cell Cultures. Neurotoxicol Teratol 46, 49–56. doi:10.1016/j.ntt.2014.09.003
Ross, H. R., Napier, I., and Connor, M. (2008). Inhibition of Recombinant Human T-type Calcium Channels by delta9-tetrahydrocannabinol and Cannabidiol. J. Biol. Chem. 283, 16124–16134. doi:10.1074/jbc.M707104200
Ruhaak, L. R., Felth, J., Karlsson, P. C., Rafter, J. J., Verpoorte, R., and Bohlin, L. (2011). Evaluation of the Cyclooxygenase Inhibiting Effects of Six Major Cannabinoids Isolated from Cannabis Sativa. Biol. Pharm. Bull. 34, 774–778. doi:10.1248/bpb.34.774
Russo, E. B. (2007). History of Cannabis and its Preparations in Saga, Science, and Sobriquet. Chem. Biodivers 4, 1614–1648. doi:10.1002/cbdv.200790144
Russo, E. B. (2011). Taming THC: Potential Cannabis Synergy and Phytocannabinoid-Terpenoid Entourage Effects. Br. J. Pharmacol. 163, 1344–1364. doi:10.1111/j.1476-5381.2011.01238.x
Sagredo, O., Pazos, M. R., Satta, V., Ramos, J. A., Pertwee, R. G., and Fernández-Ruiz, J. (2011). Neuroprotective Effects of Phytocannabinoid-Based Medicines in Experimental Models of Huntington's Disease. J. Neurosci. Res. 89, 1509–1518. doi:10.1002/jnr.22682
Santiago, M., Sachdev, S., Arnold, J. C., McGregor, I. S., and Connor, M. (2019). Absence of Entourage: Terpenoids Commonly Found in Cannabis Sativa Do Not Modulate the Functional Activity of Δ9-THC at Human CB1 and CB2 Receptors. Cannabis Cannabinoid Res. 4, 165–176. doi:10.1089/can.2019.0016
Schlicker, E., and Kathmann, M. (2001). Modulation of Transmitter Release via Presynaptic Cannabinoid Receptors. Trends Pharmacol. Sci. 22, 565–572. doi:10.1016/s0165-6147(00)01805-8
Schultes, R. E., Klein, W. M., Plowman, T., and Lockwood, T. E. (1974). Cannabis: an Example of Taxonomic Neglect. Harv. Univ. Bot. Mus. Leaflets 23, 337–367. doi:10.5962/p.168565
Scott, K. A., Dalgleish, A. G., and Liu, W. M. (2017). Anticancer Effects of Phytocannabinoids Used with Chemotherapy in Leukaemia Cells Can Be Improved by Altering the Sequence of Their Administration. Int. J. Oncol. 51, 369–377. doi:10.3892/ijo.2017.4022
Scott, K. A., Shah, S., Dalgleish, A. G., and Liu, W. M. (2013). Enhancing the Activity of Cannabidiol and Other Cannabinoids In Vitro through Modifications to Drug Combinations and Treatment Schedules. Anticancer Res. 33, 4373–4380.
Shen, M., Piser, T. M., Seybold, V. S., and Thayer, S. A. (1996). Cannabinoid Receptor Agonists Inhibit Glutamatergic Synaptic Transmission in Rat Hippocampal Cultures. J. Neurosci. 16, 4322–4334. doi:10.1523/jneurosci.16-14-04322.1996
Shinjyo, N., and Di Marzo, V. (2013). The Effect of Cannabichromene on Adult Neural Stem/progenitor Cells. Neurochem. Int. 63, 432–437. doi:10.1016/j.neuint.2013.08.002
Small, E. (2015). Evolution and Classification of Cannabis Sativa (Marijuana, Hemp) in Relation to Human Utilization. Bot. Rev. 81, 189–294. doi:10.1007/s12229-015-9157-3
Smart, D., Gunthorpe, M. J., Jerman, J. C., Nasir, S., Gray, J., Muir, A. I., et al. (2000). The Endogenous Lipid Anandamide Is a Full Agonist at the Human Vanilloid Receptor (hVR1). Br. J. Pharmacol. 129, 227–230. doi:10.1038/sj.bjp.0703050
Smeriglio, A., Giofrè, S. V., Galati, E. M., Monforte, M. T., Cicero, N., D'Angelo, V., et al. (2018). Inhibition of Aldose Reductase Activity by Cannabis Sativa Chemotypes Extracts with High Content of Cannabidiol or Cannabigerol. Fitoterapia 127, 101–108. doi:10.1016/j.fitote.2018.02.002
Stella, N. (2010). Cannabinoid and Cannabinoid-like Receptors in Microglia, Astrocytes, and Astrocytomas. Glia 58, 1017–1030. doi:10.1002/glia.20983
Sugiura, T., Kondo, S., Sukagawa, A., Nakane, S., Shinoda, A., Itoh, K., et al. (1995). 2-Arachidonoylglycerol: a Possible Endogenous Cannabinoid Receptor Ligand in Brain. Biochem. Biophys. Res. Commun. 215, 89–97. doi:10.1006/bbrc.1995.2437
Sulak, D., Saneto, R., and Goldstein, B. (2017). The Current Status of Artisanal Cannabis for the Treatment of Epilepsy in the United States. Epilepsy Behav. 70, 328–333. doi:10.1016/j.yebeh.2016.12.032
Takahashi, R. N., and Karniol, I. G. (1975). Pharmacologic Interaction between Cannabinol and delta9-tetrahydrocannabinol. Psychopharmacologia 41, 277–284. doi:10.1007/BF00428937
Takeda, S., Misawa, K., Yamamoto, I., and Watanabe, K. (2008). Cannabidiolic Acid as a Selective Cyclooxygenase-2 Inhibitory Component in Cannabis. Drug Metab. Dispos 36, 1917–1921. doi:10.1124/dmd.108.020909
Tan, B., Bradshaw, H. B., Rimmerman, N., Srinivasan, H., Yu, Y. W., Krey, J. F., et al. (2006). Targeted Lipidomics: Discovery of New Fatty Acyl Amides. AAPS J. 8, E461–E465. doi:10.1208/aapsj080354
Thomas, A., Stevenson, L. A., Wease, K. N., Price, M. R., Baillie, G., Ross, R. A., et al. (2005). Evidence that the Plant Cannabinoid delta9-tetrahydrocannabivarin Is a Cannabinoid CB1 and CB2 Receptor Antagonist. Br. J. Pharmacol. 146, 917–926. doi:10.1038/sj.bjp.0706414
Tóth, K. F., Adám, D., Bíró, T., and Oláh, A. (2019). Cannabinoid Signaling in the Skin: Therapeutic Potential of the "c(ut)annabinoid System. Molecules 24, 918.
Tsou, K., Brown, S., Sañudo-Peña, M. C., Mackie, K., and Walker, J. M. (1998). Immunohistochemical Distribution of Cannabinoid CB1 Receptors in the Rat central Nervous System. Neuroscience 83, 393–411. doi:10.1016/s0306-4522(97)00436-3
Tubaro, A., Giangaspero, A., Sosa, S., Negri, R., Grassi, G., Casano, S., et al. (2010). Comparative Topical Anti-inflammatory Activity of Cannabinoids and Cannabivarins. Fitoterapia 81, 816–819. doi:10.1016/j.fitote.2010.04.009
Tudge, L., Williams, C., Cowen, P. J., and McCabe, C. (2015). Neural Effects of Cannabinoid CB1 Neutral Antagonist Tetrahydrocannabivarin on Food Reward and Aversion in Healthy Volunteers. Int. J. Neuropsychopharmacol. 18, pyu094. doi:10.1093/ijnp/pyu094
Turner, C. E., and ElSohly, M. A. (1981). Biological Activity of Cannabichromene, its Homologs and Isomers. J. Clin. Pharmacol. 21, 283S–291S. doi:10.1002/j.1552-4604.1981.tb02606.x
Udoh, M., Santiago, M., Devenish, S., McGregor, I. S., and Connor, M. (2019). Cannabichromene Is a Cannabinoid CB2 Receptor Agonist. Br. J. Pharmacol. 176, 4537–4547. doi:10.1111/bph.14815
Valdeolivas, S., Navarrete, C., Cantarero, I., Bellido, M. L., Muñoz, E., and Sagredo, O. (2015). Neuroprotective Properties of Cannabigerol in Huntington's Disease: Studies in R6/2 Mice and 3-Nitropropionate-Lesioned Mice. Neurotherapeutics 12, 185–199. doi:10.1007/s13311-014-0304-z
Vaughan, C. W., Connor, M., Bagley, E. E., and Christie, M. J. (2000). Actions of Cannabinoids on Membrane Properties and Synaptic Transmission in Rat Periaqueductal gray Neurons In Vitro. Mol. Pharmacol. 57, 288–295.
Vigli, D., Cosentino, L., Raggi, C., Laviola, G., Woolley-Roberts, M., and De Filippis, B. (2018). Chronic Treatment with the Phytocannabinoid Cannabidivarin (CBDV) Rescues Behavioural Alterations and Brain Atrophy in a Mouse Model of Rett Syndrome. Neuropharmacology 140, 121–129. doi:10.1016/j.neuropharm.2018.07.029
Walsh, K. B., and Andersen, H. K. (2020). Molecular Pharmacology of Synthetic Cannabinoids: Delineating CB1 Receptor-Mediated Cell Signaling. Ijms 21, 6115. doi:10.3390/ijms21176115
Wargent, E. T., Zaibi, M. S., Silvestri, C., Hislop, D. C., Stocker, C. J., Stott, C. G., et al. (2013). The Cannabinoid Δ(9)-tetrahydrocannabivarin (THCV) Ameliorates Insulin Sensitivity in Two Mouse Models of Obesity. Nutr. Diabetes 3, e68. doi:10.1038/nutd.2013.9
Wong, H., and Cairns, B. E. (2019). Cannabidiol, Cannabinol and Their Combinations Act as Peripheral Analgesics in a Rat Model of Myofascial Pain. Arch. Oral Biol. 104, 33–39. doi:10.1016/j.archoralbio.2019.05.028
Wood, T. B., Spivey, W. T. N., and Easterfield, T. H. (1886). Charas, the Resin of Indian Hemp. J. Chem. Soc. 69, 539–546.
World Health Organization (2016). The Health and Social Effects of Nonmedical Cannabis Use. Available at: http://www.who.int/substance_abuse/publications/cannabis/en/.
Yoshida, H., Usami, N., Ohishi, Y., Watanabe, K., Yamamoto, I., and Yoshimura, H. (1995). Synthesis and Pharmacological Effects in Mice of Halogenated Cannabinol Derivatives. Chem. Pharm. Bull. (Tokyo) 43, 335–337. doi:10.1248/cpb.43.335
Zagzoog, A., Mohamed, K. A., Kim, H. J. J., Kim, E. D., Frank, C. S., Black, T., et al. (2020). In Vitro and In Vivo Pharmacological Activity of Minor Cannabinoids Isolated from Cannabis Sativa. Sci. Rep. 10, 20405. doi:10.1038/s41598-020-77175-y
Zamberletti, E., Gabaglio, M., Piscitelli, F., Brodie, J. S., Woolley-Roberts, M., Barbiero, I., et al. (2019a). Cannabidivarin Completely Rescues Cognitive Deficits and Delays Neurological and Motor Defects in Male Mecp2 Mutant Mice. J. Psychopharmacol. 33, 894–907. doi:10.1177/0269881119844184
Zamberletti, E., Gabaglio, M., Woolley-Roberts, M., Bingham, S., Rubino, T., and Parolaro, D. (2019b). Cannabidivarin Treatment Ameliorates Autism-like Behaviors and Restores Hippocampal Endocannabinoid System and Glia Alterations Induced by Prenatal Valproic Acid Exposure in Rats. Front Cel Neurosci 13, 367. doi:10.3389/fncel.2019.00367
Keywords: Cannabis sativa, minor cannabinoids, TRP channel, endocannabinoids, therapeutics, CB1–CB2 cannabinoid receptors
Citation: Walsh KB, McKinney AE and Holmes AE (2021) Minor Cannabinoids: Biosynthesis, Molecular Pharmacology and Potential Therapeutic Uses. Front. Pharmacol. 12:777804. doi: 10.3389/fphar.2021.777804
Received: 15 September 2021; Accepted: 08 November 2021;
Published: 29 November 2021.
Edited by:
Kathia Honorio, Universidade de São Paulo, BrazilReviewed by:
Carl R. Lupica, National Institute on Drug Abuse (NIDA), United StatesCopyright © 2021 Walsh, McKinney and Holmes. This is an open-access article distributed under the terms of the Creative Commons Attribution License (CC BY). The use, distribution or reproduction in other forums is permitted, provided the original author(s) and the copyright owner(s) are credited and that the original publication in this journal is cited, in accordance with accepted academic practice. No use, distribution or reproduction is permitted which does not comply with these terms.
*Correspondence: Kenneth B. Walsh, d2Fsc2hAdXNjbWVkLnNjLmVkdQ==
Disclaimer: All claims expressed in this article are solely those of the authors and do not necessarily represent those of their affiliated organizations, or those of the publisher, the editors and the reviewers. Any product that may be evaluated in this article or claim that may be made by its manufacturer is not guaranteed or endorsed by the publisher.
Research integrity at Frontiers
Learn more about the work of our research integrity team to safeguard the quality of each article we publish.