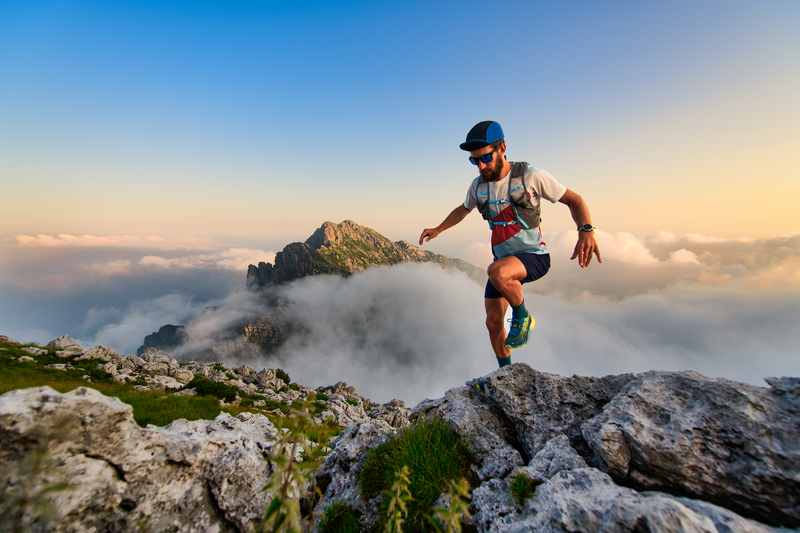
95% of researchers rate our articles as excellent or good
Learn more about the work of our research integrity team to safeguard the quality of each article we publish.
Find out more
ORIGINAL RESEARCH article
Front. Pharmacol. , 01 December 2021
Sec. Ethnopharmacology
Volume 12 - 2021 | https://doi.org/10.3389/fphar.2021.777689
This article is part of the Research Topic Filling the Void in Lung and Metabolic Inflammation Research: Novel Drug Targets and Addressing Knowledge Gaps View all 4 articles
Background: Respiratory syncytial virus (RSV) can cause varying degrees of lung inflammation in children. Qingfei Oral Liquid (QF) is effective in treating childhood RSV-induced lung inflammation (RSV-LI) in clinics, but its pharmacological profiles and mechanisms remain unclear.
Methods: This study combined network Pharmacology, lipidomics, pharmacodynamics, and pathway validation to evaluate the therapeutic mechanisms of QF. Using Cytoscape (v3.8.2) and enrichment analyses from the Kyoto Encyclopedia of Genes and Genomes (KEGG) and Gene Ontology (GO), a global view of the putative compound-target-pathway network was created. The corresponding lipidomic profiles were then used to detect differently activated lipids, revealing the metabolic pathway, using ultra-high-performance liquid chromatography linked to hybrid Quadrupole-Exactive Orbitrap mass spectrometry (UHPLC-Q-Exactive Orbitrap MS). Meanwhile, the in vivo efficiency of QF, the enrichment pathway, and the excessive autophagy inhibition mechanisms were validated in RSV-infected mice models.
Results: The network pharmacology results demonstrated 117 active compounds acted directly upon 101 core targets of QF against RSV-LI. The most significantly enriched pathway was the PI3K/Akt/mTOR signaling pathway (p < 0.05). In addition, untargeted lipidomics were performed, and it was revealed that higher lung levels of DAG 30:0, DAG 30:5, DAG 32:0, DAG 16:0_18:0, DAG 17:0_17:0, DAG 34:1, DAG 36:0, DAG 36:1 in the RSV-LI group were decreased after QF administration (FDR < 0.05, FC > 1.2). Lipin-1, a key enzyme in DAG synthesis, was increased in the RSV-LI mouse model. Animal experiments further validated that QF inhibited the PI3K/Akt/mTOR signaling pathway, with lower lung levels of phosphorylated PI3K, AKT and mTOR, as well as its related proteins of lipin-1 and VPS34 (p < 0.01). Finally, pharmacodynamic investigations indicated that QF reduced airway inflammation caused by excessive autophagy by decreasing lung levels of RSV F and G proteins, Beclin-1, Atg5, and LC3B II, IL-1 and TNF-α (p < 0.05).
Conclusion: Lipidomic-based network pharmacology, along with experimental validation, may be effective approaches for illustrating the therapeutic mechanism of QF in the treatment of RSV-LI.
Respiratory syncytial virus (RSV) is the most common causative agent leading to respiratory tract infection worldwide (Borchers et al., 2013). According to extensive epidemiological analysis, immunocompromised populations are more vulnerable to developing bronchiolitis and pneumonia induced by RSV infection, accounting for the high rates of hospitalizations and deaths among preterm babies and infants (Rey-Jurado and Kalergis, 2017; Seidenberg, 2019). Unlike mild symptoms, RSV- induced lung inflammation (RSV-LI) is closely associated with severe wheezing and hyperreactive airways, and asthma exacerbation at young ages (Tsukagoshi et al., 2013; Bianchini et al., 2020). Despite its remarkable threat to human health, population-based data on the practical and safe therapeutic options for treating this viral disease are lacking (Griffiths et al., 2017). To date, only palivizumab, a monoclonal antibody, has been applied to prevent severe illness caused by RSV infection at high cost (Perk and Özdil, 2018). Therefore, intense exploration should be focused on developing an effective and affordable strategy for the prevention and treatment of RSV-LI.
Traditional Chinese medicine (TCM), a unique and holistic theory system, has been employed for preventing and treating viral diseases for thousands of years (Oravecz and Mészáros, 2012). Qingfei Oral Liquid (QF) is derived from a classic prescription called “Maxin Shigan Decoction,” and composed of Ephedra sinica Stapf. (Ma Huang 5g), Prunus armeniaca L. (Ku Xingren 12g), Gypsum Fibrosum (Sheng Shigao 40g), Morus alba L. (Sang Baipi 12g), Lepidium virginicum L. (Ting Lizi 10g), Angelica decursiva (Zihua Qianhu 12g), Bistorta officinalis Delarbre (Quan Shen 15g), Reynoutria japonica Houtt. (Hu Zhang 15g), Bombyx mori Linnaeus. (Jiang Can 8g), and Salvia miltiorrhiza Bunge (Dan Shen 8g). Our previous research confirmed that QF can significantly relieve the symptoms and signs of fever, wheezing, and pulmonary rales in children with RSV pneumonia (Wang et al., 2016). In vitro experiments showed that QF prevented RSV proliferation by inhibiting virus membrane fusion with host cells (Yuan et al., 2009). Furthermore, it alleviated RSV-LI by reversing immune cell imbalance and decreasing inflammatory responses (Zhu et al., 2014; Zou et al., 2018). However, previous results only reflect the global effects of QF against RSV-LI, and targets for drug intervention have not been clarified.
Due to the multi-target and multi-component characteristics of TCM, it is difficult to identify the specific components and targets of TCM formulas (Li and Zhang, 2013). In recent years, bioinformatics in the form of network pharmacology has been widely applied to reveal interactions between targets and diseases through bioactive component identification, network construction, and pathway enrichment (Zhu et al., 2021). In addition, lipidomics, a system biology technique for large-scale determination of individual lipid species, has also been applied to investigate various human diseases. Given the systematic characteristics embodied in omics approaches, application of lipidomics can generate a complete atlas of the metabolic landscape, and enable comprehensive analysis to identify critical metabolic factors in disease pathology (Wang R. et al., 2020). Thus, systematic features of network pharmacology and lipidomic research can be combined for a comprehensive investigation of TCM formulas. By establishing an omics-based network, drug action can be unveiled through multi-layer information, thereby providing a promising alternative for accelerating rational drug design and better exploring the mechanism underlying TCM therapy (Caberlotto and Lauria, 2015). Despite several significant TCM achievements in blocking viral infection, the critical role of QF against RSV-LI has not been investigated using bioinformatics and omics analyses.
Here, integrated network pharmacology with lipidomics, we identified and validated the active compounds, core targets, signaling pathways, and disturbed lipids involved in QF treatment of RSV-LI in BALB/c mice models (Figure 1).
Chemical compounds of QF were obtained from the databases of traditional Chinese medicine systems pharmacology (TCMSP, http://tcmspw.com/tcmsp.php) and bioinformatics analysis tool for molecular mechanism of traditional Chinese medicine (BATMAN-TCM, http://bionet.ncpsb.org/batman-tcm) (Ru et al., 2014; Liu et al., 2016). Active compounds were screened with oral bioavailability (OB)≥30% and drug-likeness (DL)≥0.18. Protein targets of the active components in QF were converted to official symbols by checking with reviewed Homo sapiens gene list in the Protein Knowledgebase (UniProtKB, http://www.uniprot.org/) (Tao et al., 2020). The disease-related targets were collected by inputting keywords “respiratory syncytial virus pneumonia,” “RSV pneumonia” and “RSV-induced lung inflammation” into online platforms including GeneCards (http://www. genecards. org/) and online Mendelian inheritance in man (OMIM; http://www.omim.org/) (Stelzer et al., 2016; Amberger and Hamosh, 2017). The common target genes were selected out as QF targets in the treatment of RSV-LI. The core targets were further analyzed by STRING (https://string-db.org/cgi/input.pl) to construct Protein–Protein Interactions (PPI) network (Szklarczyk et al., 2017). The enrichment analysis in the Kyoto Encyclopedia of Genes and Genomes (KEGG) and Gene Ontology (GO) were performed to demonstrate the main biological functions and signal transductions involved by core targets. The top 20 KEGG pathways (p < 0.05) were displayed in a histogram using the MetaScape Bioinformatics Resource database (http://metascape.org/) (Zhou et al., 2019). GO enrichment was conducted by ClueGO, a plug-in of Cytoscape (v3.8.2) software (http://www.cytoscape.org/) to visualize biological process (BP), cellular component (CC) and molecular function (MF) enrichments of core targets.
QF was made up of the following ingredients: Ephedra sinica Stapf. (batch No. 190807, 5g), Prunus armeniaca L. (batch No.191321, 12g), Gypsum Fibrosum (batch No. 191201, 40g), Morus alba L. (batch No. 190407, 12g), Lepidium virginicum L. (batch No. 190312, 10g), Angelica decursiva (batch No. 190129, 12g), Bistorta officinalis Delarbre (batch No. 191227, 15g), Reynoutria japonica Houtt. (batch No. 191115, 15g), Bombyx mori Linnaeus. (batch No. 191104, 8g) and Salvia miltiorrhiza Bunge (batch No. 191226, 8g). The ten products were purchased from Tongling Hetian Chinese Medicine herbal tablets Co., Ltd. Anhui, China. QF was prepared in accordance with standard operating procedures (Wang et al., 2003). In brief, the ten drugs were mixed and decocted twice in filtered water for 1 hour at 100°C, and the filtrate was concentrated to a final concentration of 3.65 g/ml using a rotary evaporator (YRE-2000A, China). The concentrating agent was kept at −20°C for future use. The fingerprinting analysis of QF (30 ug/ml) was performed in both negative and positive ionization modes using a Linear Ion Trap Quadrupole-Orbitrap Mass Spectrometer (LTQ-Obitrap MS; Thermo Fisher Scientific, USA).
Hep2 cells (human laryngeal carcinoma cells; China Center for Type Culture Collection, Wuhan, China) were cultured with DMEM medium (10% FBS, 100 U/ml penicillin, 0.1 mg/ml streptomycin) to provide condition for RSV strain A2 (China Center for Type Culture Collection, Wuhan, China). The viral supernatant was collected after 4–5 days and a plaque assay was performed to ensure that the virulence reached 1*10^6 PFU/ml. The viral solution was stored in liquid nitrogen after being centrifuged for 10 min at 3,000 rpm at 4°C. The animal experiment in this study was approved by the ethics committee of the Laboratory Animal Center at Nanjing University of Chinese Medicine (ethical # 201912A009). The in vitro and in vivo experiments were conducted out in Yangzhou University’s biosafety level-2 laboratory. A total of 18 female BALB/c mice (aged 6–8 weeks, 18 ± 2 g) were purchased from the Laboratory Animal Department of Shanghai Family Planning Research Institute (permission number: 202116007) and randomly assigned to three groups: a control group (C), an RSV group (M) and a QF group (n = 6 per group). The RSV and QF groups received 80 μl RSV suspension intranasally under inhalation anesthesia on the first day of modeling, whereas the control group received equal DMEM medium. QF (27.6 g/kg/day) was delivered intragastrically for four consecutive days, equivalent to the clinical human dosage as assessed by host weight (Zhu et al., 2021). On the fifth day after intervention, mice were sacrificed. The body weight, energy level, diet, drinking water, fur color, and activity of the mice in each group were recorded daily.
After the mice were euthanized, middle lobe of right lung was fixed in 4% neutral buffered paraformaldehyde for 12 h dehydration before being embedded in paraffin wax and stained with hematoxylin and eosin (H&E). Tissue lesions and inflammatory cell infiltrates were measured and captured under a light microscope at high magnification (200×) (OLYMPUS, Japan). Lung tissue was divided into 20 mg aliquots for lipidomic analysis and the remaining was stored at −80°C for the biochemical indexes including PI3K, p-PI3K, AKT, p-AKT, mTOR, p-mTOR, Lipin-1, Beclin-1, Atg5, LC3B, VPS34, IL-1β, IL-6, and TNF-α.
To perform a comprehensive lipidomic profiling, the sample preparation strategy for lung tissue based on liquid-liquid MTBE extraction was previously established (Du et al., 2015). Briefly, 20 μl lung tissues homogenate was transferred into a clean tube and mixed with 225 μl cold methanol solution containing 10 μg/ml internal standards, including lyso PE (17:1) (batch number: LM171LPE-11), LPC17:0 (batch number: LM170LPC-30), PE (17:0/17:0) (batch number: LM170PE-19) purchased from Avanti Polar Lipids Company. After vortexing for 10 s, 750 μl Methyl tert-butyl ether (MTBE, ROE, USA) was added, and the mixture were shaken for 10 min at 4°C. The samples were vortexed for 10 s after adding 188 μl of deionized water, and then centrifuged at 18,000 rpm at 4°C in an Integrated SpeedVac apparatus (Thermo Fisher Scientific, USA). The upper (organic) phase, primarily lipids, was transferred to new tubes and dried in a Savant SPD1010 vacuum centrifuge concentrator (Thermo Fisher Scientific, USA). Finally, for LC-MS analysis, the upper phase lipids were reconstituted in 110 μl methanol: toluene (9:1) solution. The preparation was carried out by combining equal aliquots of 10 μl from each sample, and they were pretreated in the same way as the samples. Qulity control (QC) pool samples were injected before the samples, and one QC injection was placed per ten samples on a regular basis.
Untargeted lipidomics was conducted with a Dionex UltiMate 3000 ultra-high-performance liquid chromatography (UHPLC, Santa Clara, USA) coupled to Q Exactive Orbitrap Mass Spectrometers (Q-Exactive-MS; Thermo Fisher Scientific, USA). For gradient elution, a 2 μl sample solution was injected onto a Waters ACQUITY UPLC CSH C18 (100 mm, 2.1 mm, 1.7 mm) kept at 65°C. Mobile phase A was 40% acetonitrile in water, whereas mobile phase B was a 9:1 mixture of isopropanol and acetonitrile containing 5 mM ammonium formate and 0.1% formic acid. The specific elution gradient was as follows: 0–2 min, 15–30% B; 2–2.5 min, 30–48% B; 2.5–11 min, 48–82% B; 11–11.5 min, 82–99% B; 11.5–12 min, 99% B; 12–13 min, 99–15% B; 13–15 min, 15% B. The flow rate was 0.6 ml/min. The MS experiment was carried out in positive ion mode, with a heated electrospray ionization source (3.5 kV spray voltage). In full-scan mode, the mass analyzer had a resolving power of 60000 FWHM and a scan range of 150–1,500 m/z.
ABF converter (http://www.reifycs.com/AbfConverter) was used to convert raw data from the Xcalibur 2.2 program (Thermo Fisher Scientific, USA) to ABF formats. The files were then analyzed using the MS-DIAL (v4.10) software tool. The parameters of MS-DIAL were described in the previous study (Yang et al., 2014). For lipid identification, the public LipidBlast library was used with accurate mass and MS/MS matching. Adduct ions were set as + H, +NH4 and +Na for the positive ion mode. MetaboAnalyst 5.0 (http://www.metaboanalyst.ca) was used to further normalize multidimensional data following median and pareto scaling. The difference in lipidomic profiles between the control, model, and QF groups was represented in the principal component analysis (PCA) model. False discovery rate (FDR <0.05, Kruskal-Wallis test) and fold change (FC > 1.2 or <0.833, ratio of median) were used to screen for significant lipids. The number of screened lipids in each of the two groups was represented by a Venn diagram. Furthermore, heatmaps revealed the pattern of key lipids that were expressed in opposite directions between the two groups. In the boxplots, the FDR values were used to compare the relative concentrations of diacylglycerol (DAG).
Total RNA extractions were performed with a FastPure Tissue Total RNA Isolation Kit (Vazyme, China) in accordance with the manufacturer’s instructions. Total RNA concentration and purity were determined using a Bio Photometer (Eppendorf, Germany). Extracted RNA was reverse-transcribed into cDNA using a Reverse transcription and cDNA synthesis kit (Abm, China). Quantitative real-time PCR was conducted on a 384-well plate under QuantStudio 7 Flex Real-Time PCR System (Thermo Fisher Scientific, USA). Data was normalized and processed with 2−ΔΔCT method by taking the housekeeping gene GAPDH as reference standard.
Protein was extracted from lung tissues on ice for 30 min using RIPA lysis buffer with proteinase and phosphatase inhibitors (100:1:1). The tissue lysate was then centrifuged for 30 min at 13,000 rpm and 4°C. Using bovine serum albumin as a reference, protein content was determined using a BCA Protein Assay Kit (Thermo Fisher Scientific, USA). 30 μg total proteins of lung tissue lysate were run on 10% tris-glycine SDS-PAGE gel (Biosharp), 80 V for 0.5 h and 110 V for 1 h. Gels were transferred to a polyvinylidene difluoride (PVDF) membrane by Trans-Blot (Bio-Rad, USA), and blocked with 5% BSA for 1 h. The bands were incubated overnight at 4°C with primary antibodies: PI3K (1:1,000, CST, USA), p-PI3K (1:1,000, CST, USA), AKT (1:1,000, CST, USA), p-AKT (1:2000, CST, USA), mTOR (1:1,000, CST, USA), p-mTOR (1:1,000, CST, USA), Beclin-1 (1:1,000, CST, USA), Atg5 (1:1,000, CST, USA), LC3B (1:1,000, CST, USA), VPS34 (1:500, Proteintech, China), β-actin (1:20000, Proteintech, China). Proteins on the band were observed using a ChemiDoc XRS Gel Imaging System (Bio-Rad, USA) after 1 h at room temperature incubation with the appropriate secondary antibodies (Fcmacs, China). ImageJ software was used to examine the protein expression of each group (v1.8.0).
Lung tissue paraffin slides were deparaffinized in xylene, rehydrated using an ethanol gradient, antigen-retrieved in a 10 mM sodium citrate buffer (pH 6), blocked with 3% H2O2, and permeabilized with 1% BSA. The sections were then blocked with Lipin-1 antibody (1:500, Abcam, USA) overnight at 4°C. Afterwards, the secondary antibody (1:250, MaxVision, China) was added and incubated at room temperature for 20 min. After DAB staining, the slices were stained with hematoxylin and eosin for 10 min, dehydrated, and sealed in neutral resin. Lipin-1 protein expression levels were observed in different groups using a light microscope at high magnification (200×).
GraphPad Prism software (v8.0.2) was used to analyze and visualize all of the biochemical data. The standard deviation is shown by standard error of the mean (SEM). One-way analysis of variance (ANOVA) with the Student-Newman-Keuls post hoc test was used for multi-group comparisons, with p < 0.05 considered significant.
Detailed information on formula composition of QF was shown in Table 1. The chemical profile of QF was originally investigated using UPLC-ESI/LTQ-Orbitrap-MS. Based on the reference compounds, a total of six components from 10 crude materials were identified qualitatively. The typical total ion chromatograms (TICs) are shown in Supplementary Figure S1. QF’s primary bioactive components, including Catechin (C15H14O6), Luteolin (C15H10O6), Quercetin (C15H10O7), Naringenin (C15H12O5), Kaempferol (C15H10O6), and Tanshinone IIA (C19H18O3), were processed under quality control (Supplementary Table S1). The detail parameters of the quality control were listed in supplementary file.
To develop a BALB/c mouse model of RSV-LI, 80 μl RSV suspension (1*106 PFU/ml) intranasally under inhalation anesthesia on the first day of modeling (Figure 2A). Body weight (BW) changes revealed that RSV infection led to a significant weight loss within 24 h of nasal drip, with the mean value reaching the lowest level on the third day of modeling compared to controls (*p<0.01). Such weight loss, however, was successfully avoided in mice given QF therapy (*p>0.05 versus control, Figure 2B). The expression of RSV F and G proteins in lung tissues represented viral load. These two indicators’ transcription levels were evidently increased in the model group (*p<0.0001, versus control, Figure 2C), but they were significantly reduced in the QF group (#p<0.0001, versus model; Figure 2C). Figure 2D showed HE staining of pulmonary pathologies in the three groups. In RSV-LI mice model, there was clear evidence of alveolar wall damage and inflammatory cell infiltration surrounding the bronchi, which was significantly alleviated after QF treatment.
FIGURE 2. Evaluation of RSV-induced lung inflammation mouse model and efficacy of QF. (A) Schematic diagram of animal modeling and administration. (B) Weight changes of mice in control (C), model (M), and therapy (QF) groups from day 0 to day 4. Values are expressed as mean ± standard error of the mean (SEM; n = 6, * compared with control group, **p < 0.01, ns = not significant). (C) Transcription levels of RSV F and RSV G proteins in lung tissues of mice in control (C), model (M), and therapy (QF) groups. Values are expressed as mean ± SEM (n = 6, * compared with control group, # compared with model group, ****p < 0.0001, ####p < 0.0001. (D) H&E staining to evaluate pulmonary histopathological damage in control (C), model (M), and therapy (QF) groups (200× magnification). The black arrow indicated the evident inflammatory cell infiltration in lung tissue. Abbreviations: QF, Qingfei oral liquid; RSV, respiratory syncytial virus.
A total of 148 active compounds were identified in QF after integrating information from databases in TCMSP and BATMAN-TCM Specifically, 23 of them were found in Ephedra sinica Stapf (Ma Huang), 19 in Prunus armeniaca L. (Xingren), 12 in Lepidium virginicum L. (Ting Lizi), 27 in Morus alba L. (Sang Baipi), 8 in Angelica decursiva (Zihua Qianhu), 5 in Bistorta officinalis Delarbre (Quan Shen), 10 in Reynoutria japonica Houtt. (Hu Zhang), 1 in Bombyx mori Linnaeus. (Jiang Can), and 69 in Salvia miltiorrhiza Bunge (Dan Shen). Quercetin (MOL000098), Luteolin (MOL000006), Kaempferol (MOL000422), Naringenin (MOL004328), Tanshinone IIA (MOL007154), Beta-sitosterol (MOL000358), Cryptotanshinone (MOL007088), Ellagic acid (MOL001002), Isorhamnetin (MOL000354) and Dihydrotanshinlactone (MOL007100) were the top ten active chemicals for QF in the treatment of RSV-LI (Table 2). By referring to target mappings in TCMSP and translating protein names to official symbols on the UniProt database, 275 compound-related targets were identified. Furthermore, 859 disease-related targets were discovered in the GeneCards and OMIM databases. Finally, 101 symbols were identified as important targets for QF against RSV-LI by evaluating the shared parts in the two segments (Table 3). The network in Figure 3 constructed with 101 main targets and their matching 117 active molecules demonstrated the unique therapeutic benefits of QF working on RSV-LI. Based on interactions, active components primarily worked on targets such as Prostaglandin G/H synthase 2 (PGST2), Beta-2 adrenergic receptor (ADRB2), Muscarinic acetylcholine receptor M1 (CHRM1), Androgen receptor (AR), Nitric oxide synthase (NOS2), Peroxisome proliferator-activated receptor gamma (PPARG), and Phosphatidylinositol 4,5-bisphosphate 3-kinase catalytic subunit gamma isoform (PIK3CG).
FIGURE 3. Active compound-core target network of QF in the treatment of RSV-induced lung inflammation. Blue triangle nodes represent the molecular identification (MOL ID) number of active components in QF, and red circle nodes represent the core targets. Edges represent interactions between compounds and targets. The size of each node is positively correlated with the degree value. Abbreviations: QF, Qingfei oral liquid; RSV, respiratory syncytial virus.
The PPI network illustrated the relationship between core targets (Figure 4A). In the network, proteins including RAC-alpha serine/threonine-protein kinase (AKT1), Cellular tumor antigen p53 (TP53), Mitogen-activated protein kinase 3 (MAPK3), Vascular endothelial growth factor A (VEGFA), Tumor necrosis factor (TNF), Interleukin-6 (IL6), Transcription factor AP-1 (JUN), Caspase-3 (CASP3), Mitogen-activated protein kinase 8 (MAPK8), Signal transducer and activator of transcription 3 (STAT3), Prostaglandin G/H synthase 2 (PTGS2), Mitogen-activated protein kinase 1 (MAPK1), Pro-epidermal growth factor (EGF), Epidermal growth factor receptor (EGFR) and Myc proto-oncogene protein (MYC) were identified as hub nodes with a higher degree value.
FIGURE 4. PPI network and enrichment pathway analysis for QF in the treatment of RSV-induced lung inflammation. (A) PPI network of core targets. Circle nodes represent core targets and edges represent interactions between them. The size of each node is positively correlated with the degree value. (B) KEGG pathway analysis of core targets. The top 20 pathways are ranked according to gene count and adjusted p-value (ap<0.05). (C) GO enrichment analysis of core targets. Circle nodes represent sub-branches of the main pathways. Nodes of the same color are clustered into one functional group. The name of each biofunction is shown next to the group with the same color. Edges represent interactions between sub-branches of one cluster. Abbreviations: QF, Qingfei oral liquid; RSV, respiratory syncytial virus; PPI, protein interaction.
The signaling pathways were shown using KEGG and GO analysis to better study the biofunctions of key targets involved in the QF against RSV-LI process. The top 20 KEGG pathways, as shown in Figure 4B, were mostly related to immunological function, inflammatory response, and cell stress. Because of the lowest ap value, the phosphatidylinositol 3-kinase (PI3K)/protein kinase B (AKT) signaling pathway was rated top. The downstream of PI3K/AKT signal transduction including mammalian target of rapamycin (mTOR) and forkhead box (FoxO) were also shown in the list. Furthermore, ClueGO software was used to cluster fifteen significant GO terms in order to visualize the biological process (BP), cellular component (CC), and molecular function (MF) of core targets. With enrichment ratios greater than 10%, the apoptotic process, protein serine/threonine kinase activity, lipid response, and cellar response to chemical stress were found to be the most significant (Figure 4C).
Untargeted lipidomics were applied based on our previous study that revealed decreased levels of lipids including triglyceride (TG) and glycerophosphates in RSV-infected mice (Shan et al., 2018), as well as the GO enrichment results of network pharmacology (Figure 3C) that connected to a lipid response. The principal component analysis (PCA) model in Figure 5A demonstrated full separations of lipidomic profiles across the control (C), model (M), and treatment (QF) groups. There were 281 differential lipids detected in the M vs. C group, 158 in the QF vs. M group, and 134 in both comparisons (Figure 5B). The heatmaps in Figures 5C,D were established using 97 lipids that were expressed differently in the M vs. C and QF vs. M groups. Diacylglycerol (DAG), N-acylethanolamine (NAE), and ceramide (Cer) were higher expressed in lung tissues of RSV-infected mice (versus control, FDR<0.05, FC>1.2), whereas triglyceride (TG) and glycerophospholipids including phosphatidylcholine (PC), phosphatidylethanolamine (PE), phosphatidylinositol (PI), phosphatidylglycerol (PG), phosphatidylserine (PS) were lower expressed. (versus control, FDR<0.05, FC<0.833). After QF therapy, all of these lipid metabolites recover to normal levels at various degrees.
FIGURE 5. Effect of QF on lipid metabolism in lung tissues of mice with RSV-induced lung inflammation. (A) PCA score plot based on lipid profiles among control (C), model (M), and therapy (QF) groups. (B) Venn diagram of differential metabolites identified in M vs. C and QF vs. M comparisons. (C) Heatmaps of differential lipid metabolites up-regulated in M vs. C groups and down-regulated in QF vs. M groups. (D) Heatmaps of differential lipid metabolites down-regulated in M vs. C groups and up-regulated in QF vs. M group. (E) Boxplot of the relative concentrations of diglycerides (DAGs) in control (C), model (M), and therapy (QF) groups. * Compared with control group, # compared with model group, *p < 0.05, **p < 0.01, #p < 0.05, ##p < 0.01). Abbreviations: QF, Qingfei oral liquid; RSV, respiratory syncytial virus; DAG diacylglycerol.
In this investigation, however, there was no discernible change in the relative amounts of lysophospholipids and oxidized phospholipids (Figures 5C,D). Supplementary Table S3 provided detailed information of lipids in heatmaps. Above all, we found that QF controlled the lipidomic abnormalities produced by RSV infection in the lungs of mice, as evidenced by decreased DAG, Cer, NAE, and increased production of TG, PC, PE, PG, PS, and PI.
DAG was reported as the second messenger triggering signaling cascades in the previous study (Sim et al., 2020). We discovered that relative lung concentrations of DAG 30:0, DAG 30:5, DAG 32:0, DAG 16:0_18:0, DAG 17:0_17:0, DAG 34:1, DAG 36:0, DAG 36:1 and DAG 40:2 were elevated in model group, whereas QF suppressed lung levels of the DAGs in mice with RSV-induced LI (Figure 5E). As a result, we hypothesized that QF might have important therapeutic effects through modulating DAG synthesis. Lipin-1, a key enzyme in DAG production, was shown to be elevated in the lung tissue of mice with RSV-LI, and it was reduced following QF treatment (Figures 6B,C). In addition, lipin-1 serves as the downstream of the PI3K/AKT/mTOR signaling pathway (Peterson et al., 2011). The increased levels of phosphorylated versions of the proteins PI3K, AKT, and mTOR in the lung tissues of mice in the QF group were all reduced in our investigation (versus model, ##p<0.01; Figure 6A). In RSV-LI mouse models, the data showed that QF could inhibit DAG synthesis via modulating the PI3K/AKT/mTOR signaling pathway and its related protein levels of lipin-1.
FIGURE 6. Effect of QF on the activity of the PI3K/AKT/mTOR/Lipin-1 signaling pathway in lung tissues of mice with RSV-induced lung inflammation. (A) Protein expression of phosphatidylinositol 3-kinase (PI3K), phospho-phosphatidylinositol 3-kinase (p-PI3K), protein kinase B (AKT), phospho-protein kinase B (p-AKT), mammalian target of rapamycin (mTOR), phospho-mammalian target of rapamycin (p-mTOR), and β-actin in control (C), model (M), and therapy (QF) groups. The densitometry value of each phosphorylated protein was normalized against its total abundance. Values are expressed as mean ± SEM (n = 4; * compared with control group, # compared with model group, **p < 0.01, ***p < 0.001, ##p < 0.01). (B) Immunohistochemical staining of Lipin-1 protein in lung tissues of mice in control (C), model (M), and therapy (QF) groups (200 ×magnification). (C) mRNA expression level of lipin-1 protein in control (C), model (M), and therapy (QF) groups. Compared with control group, # compared with model group. **p < 0.01, ##p < 0.01. Abbreviations: QF, Qingfei oral liquid; RSV, respiratory syncytial virus.
Previous study discovered that Lipin-1-mediated DAG synthesis might activate excessive autophagy via stimulating type III phosphatidylinositol kinase (VPS34) signaling (Zhang et al., 2014). In our study, VPS34 was triggered by RSV-LI and suppressed after QF treatment (versus control, *p<0.01; versus model, #p<0.05, Figure 7B). The top-ranked list of KEGG analysis in Figure 3B included autophagy, a highly conserved cellular recycling mechanism that is intimately connected to viral replication and immune response during RSV infection (Li et al., 2018; Oh et al., 2020). To see if such a pathogenic process was activated by RSV-LI, we detected the expression of autophagy-related proteins including Beclin-1, Atg5, and LC3B. Figure 7A showed that protein levels of Beclin-1, Atg5, and LC3B II were all increased in the model group (versus control, *p<0.05, **p<0.01, ***p<0.001), but significantly decreased in the QF group (versus model, #p<0.05, ##p<0.01). Considering the close relationship between excessive autophagy and inflammation, our results demonstrated that QF effectively reduced high levels of proinflammatory cytokines, such as IL-1β, TNF-α and IL-6(versus control, *p<0.05, **p<0.01, ***p<0.001; versus model, #p<0.05, ###p<0.001, Figures 7C–E). As a consequence, we conclude that QF can suppress DAG-induced excessive autophagy and inflammation in lung tissues of RSV-LI.
FIGURE 7. Effect of QF on DAG-induced autophagy and inflammation in lung tissues of mice with RSV-induced lung inflammation. (A) Protein expression of Beclin-1, Atg5, LC3B (I, II), and β-actin in control (C), model (M), and therapy (QF) groups. Densitometry values of Beclin-1 and Atg5 were normalized against β-actin to determine relative protein expression values. The densitometry value of LC3BII was normalized against LC3BI. Values are expressed as mean ± SEM (n = 3; * compared with control group, # compared with model group, *p < 0.05, **p < 0.01, ***p < 0.001, #p < 0.05, ###p < 0.001). (B) VPS34 protein expression in control (C), model (M), and therapy (QF) groups. The densitometry value of VPS34 was normalized against β-actin. Values are expressed as mean ± SEM (n = 3; * compared with control group, # compared with model group, **p < 0.01, #p < 0.05). (C) Transcription levels of pro-inflammatory cytokines among control (C), model (M), and therapy (QF) groups. Values are expressed as mean ± SEM (n = 6; * compared with control group, # compared with model group, *p < 0.05, ***p < 0.001, #p < 0.05, ###p < 0.001). Abbreviations: QF, Qingfei oral liquid; RSV, respiratory syncytial virus.
Applying an omics strategy in network research can improve the accuracy and efficiency of exploring the mechanisms underlying TCM treatment. Our study systematically clarified the underlying mechanism of QF in the treatment of RSV-LI using lipidomic-based network pharmacology and validated by animal experiments. With the help of bioinformatics and omics analyses, we revealed that QF alleviated RSV-LI by mediating DAG-related autophagy, which is regulated by the PI3K/AKT/mTOR signaling pathway and its related proteins lipin-1 and VPS34.
In the pharmacokinetic system, compounds in QF with OB ≥ 30% and DL ≥ 0.18 were considered active ingredients that could be absorbed and utilized in the human body. By constructing an active compound-core target network, we found that Quercetin was the most effective ingredient in QF for the treatment of RSV-LI, followed by Luteolin, Kaempferol, Naringenin, Tanshinone IIA, Beta-sitosterol, Cryptotanshinone, Ellagic acid, Isorhamnetin, and Dihydrotanshinlactone (Table 2). In terms of chemical properties, most ingredients were identified as flavonoids, natural polyphenols with presumed beneficial effects on anti-inflammation, antioxidation, and immunomodulation (Yi, 2018; Zeng et al., 2019). Previous findings confirmed that the main flavone constituents in QF, such as Quercetin and Luteolin, could inhibit RSV replication by directly blocking viral adhesion or modulating microRNA-related signaling pathways (Wang S. et al., 2020; Lopes et al., 2020). Although there was no literature reporting direct links between RSV and Kaempferol, Naringenin, or Isorhamnetin, these compounds are reported to play critical roles in antiviral and anti-inflammatory procedures by reducing viral replication, inhibiting inflammatory cytokines, and eliminating free radicals in respiratory tract viral diseases (Yang et al., 2019; Huang YF. et al., 2020). Beta-sitosterol, a common phytosterol in six herbs of QF, exerts its therapeutic effect by ameliorating virus-induced proinflammatory responses and modulating immunologic activity (Fraile et al., 2012; Zhou et al., 2020). Tanshinone IIA and Cryptotanshinone, two active ingredients found in Radix Salviae, exert antiviral activity by suppressing autophagy and apoptosis during viral proliferation (Sun et al., 2019; Huang C. et al., 2020). During fingerprinting analysis of QF, Quercetin, Luteolin, Kaempferol, Naringenin, and Tanshinone IIA were also identified by LTQ-Orbitrap MS through comparison with standards (Supplementary Figure S1). Consequently, the existing literature and our experimental results supported the network prediction, and demonstrated the successful application of a network pharmacology approach in identifying active compounds in TCM formulas.
From PPI network and pathway enrichment analyses, core targets including AKT1, TP53, MAPK3, VEGFA, TNF, IL6, JUN, and CASP3 were identified as potential major points of action for QF against RSV-LI, and these targets are closely associated with inflammation, apoptosis, and carcinogenesis in the progressive course of disease. Protein kinase B (AKT), the most significant target, is a critical serine/threonine kinase that mediates cellular survival, proliferation, migration, metabolism, and angiogenesis, and it also participates in signal transduction in the PI3K/AKT/mTOR pathway, which was enriched according to KEGG analysis (Figure 3B). A recent study claimed that AKT is a promising drug target for reducing tissue damage and helping to eliminate virus infection (Xia et al., 2020). Meanwhile, through RNA interference, several genes in the PI3K/AKT/mTOR signaling pathway were demonstrated to support broad-spectrum viral replication, and Everolimus, an mTOR inhibitor, showed apparent antiviral activity against many kinds of viruses in vitro experiments (Murray et al., 2012). To further investigate the effect of QF on this signaling pathway during RSV infection, we measured the expression of crucial proteins by using western blotting. The results demonstrated the activation of this signaling pathway in lung tissues of mice in the treatment group, whereas QF exerted an inhibitory effect during treatment (Figure 6A).
The PI3K/AKT/mTOR signaling pathway involves upstream effectors that regulate a broad range of cellular processes including survival, proliferation, and growth (Ersahin et al., 2015). In primary metabolism, the PI3K/AKT/mTOR axis promotes various diseases by activating lipid synthesis (Chen et al., 2019; Yi et al., 2020). During viral infection, host invasion requires lipid modification for cell entry and trafficking, and the reprogramming of lipid metabolism and compartmentalization for assembly and egress (Mazzon and Mercer, 2014). In the present study, we also found that the lipid response was involved in the process by which QF treats RSV-LI, according to GO enrichment (Figure 4C). Through lipidomic analysis, 97 lipids were identified as metabolites that conversely expressed between M vs. C and QF vs. M groups. Among them, the abundance of DAG, NAE, and Cer in lung tissues was decreased after QF treatment, while the opposite was true for TG, PC, PE, PI, PS, and PG (Figures 5C,D). Moreover, boxplots of DAG 30:0, DAG 30:5, DAG 32:0, DAG 16:0_18:0, DAG 17:0_17:0, DAG 34:1, DAG 36:0, DAG 36:1, and DAG 40:2 among the three groups further confirmed that DAG synthesis was accelerated in the RSV-infected group but suppressed in the treatment group (Figure 5E). Regarding the link between the PI3K/AKT/mTOR pathway and DGA synthesis, lipin-1 is a key phosphatidic acid phosphatase (PAP) enzyme and the main factor in DAG production. In agreement with the mTOR signaling changes, the abundance of Lipin-1 protein was observed to be increased in mice with RSV-LI and decreased following QF therapy in immunohistochemical investigations, and this tendency was validated by PCR in mRNA levels (Figures 6B,C). These observations indicate that the PI3K/AKT/mTOR pathway may stimulate the synthesis of DAG by acting on the lipin-1 target.
Mammalian lipin proteins are involved in many cellular processes such as lipid storage, lipoprotein synthesis, autophagy, and gene expression (Reue and Wang, 2019). Previous studies on autophagy reported that QF could inhibit this process induced by RSV infection via the mTOR signaling pathway, but the specific regulatory mechanism has not been clarified (Yu et al., 2021). As a key enzyme mediated by mTOR signaling, the initiation of mature autophagosomes requires lipin-1 phosphatidic phosphatase activity to generate DAG at the surface of autophagosomes/lysosomes, which further activates the VPS34 signaling cascade to form functional autolysosomes (Zhang et al., 2014). This mechanism was verified via in vivo experiments showing that RSV-induced autophagy was accompanied by the activation of lipin-1 protein, DAG generation, and subsequent autophagy-related VPS34 signaling, and all were inhibited by QF treatment (Figures 7A,B).
Autophagy is a common intracellular self-digestion process in the human body that delivers intracellular cargo to lysosomes to generate autophagosomes, in which the contents are degraded or recycled (Painter et al., 2020). As protective or maladaptive responses, the roles of autophagy in the development of lung diseases remain poorly understood (Racanelli et al., 2018). Crosstalk between autophagy and the immune system helps maintain homeostasis and physiological functions to protect against infectious, autoimmune, and inflammatory diseases (Levine et al., 2011). However, once the balance is broken, excessive autophagy may lead to autophagic death of immune cells, which further aggravates the inflammatory response (Qiu et al., 2019). Recent findings proved that persistent or inefficient autophagy was detrimental to lung epithelial cells and promoted lung injury (Zhao et al., 2019). Consistently, both H&E staining and transcription measurements in the present work supported the inflammatory reaction in lung tissues in RSV-infected groups (Figures 2D, 7C), and this pathological progression may be closely related to dysregulated autophagy induced by RSV infection. Taken together, our findings suggest that QF may inhibit autophagy mainly via the regulation of DAG synthesis through the PI3K/AKT/mTOR signaling pathway and its related proteins lipin-1 and VPS34 (Figure 8). Detailed exploration of the underlying mechanism of QF against RSV-LI will be conducted in future studies.
FIGURE 8. Overall regulation mediated by QF in the treatment of RSV pneumonia. Abbreviations: QF, Qingfei oral liquid; RSV, respiratory syncytial virus.
In conclusion, this study investigated the pharmacological mechanism of QF in treating RSV-LI with the combination of network pharmacology, lipidomics and experimental validation. According to the observations, we suggested that QF may inhibit RSV-induced DAG synthesis via suppressing PI3K/Akt/mTOR signaling pathway and its related proteins lipin-1 and VPS34, and this process will further reduce the excessive autophagy combined with associated inflammation. Therefore, the roles of DAG synthesis and autophagy may provide potential avenues for the therapeutic targeting of RSV-LI.
The original contributions presented in the study are included in the article/Supplementary Material, further inquiries can be directed to the corresponding authors.
The animal study was reviewed and approved by the Ethics committee of Laboratory Animal Center in Nanjing university of Chinese medicine, China (ethical # 201912A009).
LL and LA designed the experiments and prepared the manuscript. ML, TX, and YL reviewed the experimental process. LA, HC, LF, and CC conducted the experiments, analyzed data, and prepared the manuscript. LL, SW, XW, and JS revised the manuscript. All authors confirmed the final manuscript.
This work was supported by National Natural Science Foundation of China (grant numbers 81904254); Natural Science Foundation of Jiangsu Province (grant numbers: BK20190808); Natural Science Foundation of the Jiangsu Higher Education Institutions of China (grant numbers: 19KJB360002); Priority Academic Program Development of Jiangsu Higher Education Institutions (PAPD): No. 87 (2018); The Open Projects of the Discipline of Chinese Medicine of Nanjing University of Chinese Medicine supported by the Subject of Academic priority discipline of Jiangsu Higher Education Institutions (grant numbers: ZYX03KF52); Young Scientists Fund of the National Natural Science Foundation of Nanjing University of Chinese medicine: (grant numbers: NZY81904254); Postgraduate Research & Practice Innovation Program of Jiangsu Province (grant numbers: SJCX21_0736). National College Students’ innovation and entrepreneurship training program (grant numbers: 202010315083Y).
The authors declare that the research was conducted in the absence of any commercial or financial relationships that could be construed as a potential conflict of interest.
All claims expressed in this article are solely those of the authors and do not necessarily represent those of their affiliated organizations, or those of the publisher, the editors and the reviewers. Any product that may be evaluated in this article, or claim that may be made by its manufacturer, is not guaranteed or endorsed by the publisher.
The researchers gratefully acknowledge the experimental conditions provided by Jiangsu Key Laboratory of Pediatric Respiratory Disease, Institute of Pediatrics, Medical Metabolomics Center, Nanjing University of Chinese Medicine.
The Supplementary Material for this article can be found online at: https://www.frontiersin.org/articles/10.3389/fphar.2021.777689/full#supplementary-material
Amberger, J. S., and Hamosh, A. (2017). Searching Online Mendelian Inheritance in Man (OMIM): A Knowledgebase of Human Genes and Genetic Phenotypes. Curr. Protoc. Bioinformatics 58, 11–121. doi:10.1002/cpbi.27
Bianchini, S., Silvestri, E., Argentiero, A., Fainardi, V., Pisi, G., and Esposito, S. (2020). Role of Respiratory Syncytial Virus in Pediatric Pneumonia. Microorganisms 8 (12), 2048–2114. doi:10.3390/microorganisms8122048
Borchers, A. T., Chang, C., Gershwin, M. E., and Gershwin, L. J. (2013). Respiratory Syncytial Virus-Aa Comprehensive Review. Clin. Rev. Allergy Immunol. 45 (3), 331–379. doi:10.1007/s12016-013-8368-9
Caberlotto, L., and Lauria, M. (2015). Systems Biology Meets -omic Technologies: Novel Approaches to Biomarker Discovery and Companion Diagnostic Development. Expert Rev. Mol. Diagn. 15 (2), 255–265. doi:10.1586/14737159.2015.975214
Chen, J., Chen, J., Huang, J., Li, Z., Gong, Y., Zou, B., et al. (2019). HIF-2α Upregulation Mediated by Hypoxia Promotes NAFLD-HCC Progression by Activating Lipid Synthesis via the PI3K-AKT-mTOR Pathway. Aging (Albany NY) 11 (23), 10839–10860. doi:10.18632/aging.102488
Du, L. N., Xie, T., Xu, J. Y., Kang, A., Di, L. Q., Shan, J. J., et al. (2015). A Metabolomics Approach to Studying the Effects of Jinxin Oral Liquid on RSV-Infected Mice Using UPLC/LTQ-Orbitrap Mass Spectrometry. J. Ethnopharmacol 174, 25–36. doi:10.1016/j.jep.2015.07.040
Ersahin, T., Tuncbag, N., and Cetin-Atalay, R. (2015). The PI3K/AKT/mTOR Interactive Pathway. Mol. Biosyst. 11 (7), 1946–1954. doi:10.1039/c5mb00101c
Fraile, L., Crisci, E., Córdoba, L., Navarro, M. A., Osada, J., and Montoya, M. (2012). Immunomodulatory Properties of Beta-Sitosterol in Pig Immune Responses. Int. Immunopharmacol 13 (3), 316–321. doi:10.1016/j.intimp.2012.04.017
Griffiths, C., Drews, S. J., and Marchant, D. J. (2017). Respiratory Syncytial Virus: Infection, Detection, and New Options for Prevention and Treatment. Clin. Microbiol. Rev. 30 (1), 277–319. doi:10.1128/cmr.00010-16
Huang, C., Zhu, J., Wang, L., Chu, A., Yin, Y., Vali, K., et al. (2020a). Cryptotanshinone Protects Porcine Alveolar Macrophages from Infection with Porcine Reproductive and Respiratory Syndrome Virus. Antivir. Res 183, 104937. doi:10.1016/j.antiviral.2020.104937
Huang, Y. F., Bai, C., He, F., Xie, Y., and Zhou, H. (2020b). Review on the Potential Action Mechanisms of Chinese Medicines in Treating Coronavirus Disease 2019 (COVID-19). Pharmacol. Res. 158, 104939. doi:10.1016/j.phrs.2020.104939
Levine, B., Mizushima, N., and Virgin, H. W. (2011). Autophagy in Immunity and Inflammation. Nature 469 (7330), 323–335. doi:10.1038/nature09782
Li, M., Li, J., Zeng, R., Yang, J., Liu, J., Zhang, Z., et al. (2018). Respiratory Syncytial Virus Replication Is Promoted by Autophagy-Mediated Inhibition of Apoptosis. J. Virol. 92 (8), 1–49. doi:10.1128/jvi.02193-17
Li, S., and Zhang, B. (2013). Traditional Chinese Medicine Network Pharmacology: Theory, Methodology and Application. Chin. J. Nat. Med. 11 (2), 110–120. doi:10.1016/s1875-5364(13)60037-0
Liu, Z., Guo, F., Wang, Y., Li, C., Zhang, X., Li, H., et al. (2016). BATMAN-TCM: a Bioinformatics Analysis Tool for Molecular mechANism of Traditional Chinese Medicine. Sci. Rep. 6, 21146. doi:10.1038/srep21146
Lopes, B. R. P., da Costa, M. F., Genova Ribeiro, A., da Silva, T. F., Lima, C. S., Caruso, I. P., et al. (2020). Quercetin Pentaacetate Inhibits In Vitro Human Respiratory Syncytial Virus Adhesion. Virus. Res. 276, 197805. doi:10.1016/j.virusres.2019.197805
Mazzon, M., and Mercer, J. (2014). Lipid Interactions during Virus Entry and Infection. Cell Microbiol 16 (10), 1493–1502. doi:10.1111/cmi.12340
Murray, J. L., McDonald, N. J., Sheng, J., Shaw, M. W., Hodge, T. W., Rubin, D. H., et al. (2012). Inhibition of Influenza A Virus Replication by Antagonism of a PI3K-AKT-mTOR Pathway Member Identified by Gene-Trap Insertional Mutagenesis. Antivir. Chem. Chemother. 22 (5), 205–215. doi:10.3851/imp2080
Oh, D. S., Park, J. H., Jung, H. E., Kim, H. J., and Lee, H. K. (2020). Autophagic Protein ATG5 Controls Antiviral Immunity via Glycolytic Reprogramming of Dendritic Cells against Respiratory Syncytial Virus Infection. Autophagy 28, 1–17. doi:10.1080/15548627.2020.1812218
Oravecz, M., and Mészáros, J. (2012). Traditional Chinese Medicine: Theoretical Background and its Use in China. Orv Hetil 153 (19), 723–731. doi:10.1556/oh.2012.29365
Painter, J. D., Galle-Treger, L., and Akbari, O. (2020). Role of Autophagy in Lung Inflammation. Front. Immunol. 11, 1337. doi:10.3389/fimmu.2020.01337
Perk, Y., and Özdil, M. (2018). Respiratory Syncytial Virüs Infections in Neonates and Infants. Turk Pediatri Ars 53 (2), 63–70. doi:10.5152/TurkPediatriArs.2018.6939
Qiu, P., Liu, Y., and Zhang, J. (2019). Review: the Role and Mechanisms of Macrophage Autophagy in Sepsis. Inflammation 42 (1), 6–19. doi:10.1007/s10753-018-0890-8
Racanelli, A. C., Kikkers, S. A., Choi, A. M. K., and Cloonan, S. M. (2018). Autophagy and Inflammation in Chronic Respiratory Disease. Autophagy 14 (2), 221–232. doi:10.1080/15548627.2017.1389823
Reue, K., and Wang, H. (2019). Mammalian Lipin Phosphatidic Acid Phosphatases in Lipid Synthesis and beyond: Metabolic and Inflammatory Disorders. J. Lipid Res. 60 (4), 728–733. doi:10.1194/jlr.S091769
Rey-Jurado, E., and Kalergis, A. M. (2017). Immunological Features of Respiratory Syncytial Virus-Caused Pneumonia-Implications for Vaccine Design. Int. J. Mol. Sci. 18 (3), 556. doi:10.3390/ijms18030556
Ru, J., Li, P., Wang, J., Zhou, W., Li, B., Huang, C., et al. (2014). TCMSP: a Database of Systems Pharmacology for Drug Discovery from Herbal Medicines. J. Cheminform 6, 13. doi:10.1186/1758-2946-6-13
Seidenberg, J. (2019). Respiratory Syncytial Virus. Internist (Berl) 60 (11), 1146–1150. doi:10.1007/s00108-019-00673-3
Shan, J., Qian, W., Shen, C., Lin, L., Xie, T., Peng, L., et al. (2018). High-resolution Lipidomics Reveals Dysregulation of Lipid Metabolism in Respiratory Syncytial Virus Pneumonia Mice. RSC Adv. 8 (51), 29368–29377. doi:10.1039/C8RA05640D
Sim, J. A., Kim, J., and Yang, D. (2020). Beyond Lipid Signaling: Pleiotropic Effects of Diacylglycerol Kinases in Cellular Signaling. Int. J. Mol. Sci. 21 (18), 6861. doi:10.3390/ijms21186861
Stelzer, G., Rosen, N., Plaschkes, I., Zimmerman, S., Twik, M., Fishilevich, S., et al. (2016). The GeneCards Suite: From Gene Data Mining to Disease Genome Sequence Analyses. Curr. Protoc. Bioinformatics 54, 13331–313033. doi:10.1002/cpbi.5
Sun, N., Sun, P., Yao, M., Khan, A., Sun, Y., Fan, K., et al. (2019). Autophagy Involved in Antiviral Activity of Sodium Tanshinone IIA Sulfonate against Porcine Reproductive and Respiratory Syndrome Virus Infection In Vitro. Antivir. Ther. 24 (1), 27–33. doi:10.3851/imp3268
Szklarczyk, D., Morris, J. H., Cook, H., Kuhn, M., Wyder, S., Simonovic, M., et al. (2017). The STRING Database in 2017: Quality-Controlled Protein-Protein Association Networks, Made Broadly Accessible. Nucleic Acids Res. 45 (D1), D362–d368. doi:10.1093/nar/gkw937
Tao, Q., Du, J., Li, X., Zeng, J., Tan, B., Xu, J., et al. (2020). Network Pharmacology and Molecular Docking Analysis on Molecular Targets and Mechanisms of Huashi Baidu Formula in the Treatment of COVID-19. Drug Dev. Ind. Pharm. 46 (8), 1345–1353. doi:10.1080/03639045.2020.1788070
Tsukagoshi, H., Ishioka, T., Noda, M., Kozawa, K., and Kimura, H. (2013). Molecular Epidemiology of Respiratory Viruses in Virus-Induced Asthma. Front. Microbiol. 4, 278. doi:10.3389/fmicb.2013.00278
Wang, R., Li, B., Lam, S. M., and Shui, G. (2020a). Integration of Lipidomics and Metabolomics for In-Depth Understanding of Cellular Mechanism and Disease Progression. J. Genet. Genomics 47 (2), 69–83. doi:10.1016/j.jgg.2019.11.009
Wang, S., Ling, Y., Yao, Y., Zheng, G., and Chen, W. (2020b). Luteolin Inhibits Respiratory Syncytial Virus Replication by Regulating the MiR-155/SOCS1/STAT1 Signaling Pathway. Virol. J. 17 (1), 187. doi:10.1186/s12985-020-01451-6
Wang, S.-C., Han, X.-M., Li, J.-Q., Ren, X.-Z., Da, Q.-G., Guo, L.-W., et al. (2003). Peparation of Qing Fei Oral Liquid and Preparation Method Treating Viral Pneumonia in Children. CN 1456341 (in Chinese).
Wang, S.-C., Sun, Y.-Q., Bian, G.-B., Xu, L., Ma, R., Wang, M.-Q., et al. (2016). Clinical Study on Qingfei Oral Liquid Treating 507 Cases of Phlegm-Heat Obstructing Lung Syndrome in Children Virus Pneumonia. World J. Traditional Chin. Med. 11 (9), 1649–1653+1658.
Xia, Q. D., Xun, Y., Lu, J. L., Lu, Y. C., Yang, Y. Y., Zhou, P., et al. (2020). Network Pharmacology and Molecular Docking Analyses on Lianhua Qingwen Capsule Indicate Akt1 Is a Potential Target to Treat and Prevent COVID-19. Cell Prolif 53 (12), e12949. doi:10.1111/cpr.12949
Yang, R., Zhang, Y., Qian, W., Peng, L., Lin, L., Xu, J., et al. (2019). Surfactant Lipidomics of Alveolar Lavage Fluid in Mice Based on Ultra-high-performance Liquid Chromatography Coupled to Hybrid Quadrupole-Exactive Orbitrap Mass Spectrometry. Metabolites 9 (4), 1–14. doi:10.3390/metabo9040080
Yang, Z. F., Bai, L. P., Huang, W. B., Li, X. Z., Zhao, S. S., Zhong, N. S., et al. (2014). Comparison of In Vitro Antiviral Activity of tea Polyphenols against Influenza A and B Viruses and Structure-Activity Relationship Analysis. Fitoterapia 93, 47–53. doi:10.1016/j.fitote.2013.12.011
Yi, J., Zhu, J., Wu, J., Thompson, C. B., and Jiang, X. (2020). Oncogenic Activation of PI3K-AKT-mTOR Signaling Suppresses Ferroptosis via SREBP-Mediated Lipogenesis. Proc. Natl. Acad. Sci. U S A. 117 (49), 31189–31197. doi:10.1073/pnas.2017152117
Yi, Y. S. (2018). Regulatory Roles of Flavonoids on Inflammasome Activation during Inflammatory Responses. Mol. Nutr. Food Res. 62 (13), e1800147. doi:10.1002/mnfr.201800147
Yu, L., Wang, J., Zou, Y., Zeng, H., Cheng, W., and Jing, X. (2021). Qingfei Oral Liquid Inhibited Autophagy to Alleviate Inflammation via mTOR Signaling Pathway in RSV-Infected Asthmatic Mice. Biomed. Pharmacother. 138, 111449. doi:10.1016/j.biopha.2021.111449
Yuan, B., Liao, H., Wang, S.-C., Xu, J.-Y., Li, F.-L., and Li, J.-Q. (2009). Experimental Study on Influence of Qingfei Oral Liquid Medicine-Contained Serum on Adhesion and Membrane Fusion Process of Respiratory Syncytial Virus. Chin. Pediatr. Integration Traditional West. Med. 1 (1), 51–53.
Zeng, X., Xi, Y., and Jiang, W. (2019). Protective Roles of Flavonoids and Flavonoid-Rich Plant Extracts against Urolithiasis: A Review. Crit. Rev. Food Sci. Nutr. 59 (13), 2125–2135. doi:10.1080/10408398.2018.1439880
Zhang, P., Verity, M. A., and Reue, K. (2014). Lipin-1 Regulates Autophagy Clearance and Intersects with Statin Drug Effects in Skeletal Muscle. Cell Metab 20 (2), 267–279. doi:10.1016/j.cmet.2014.05.003
Zhao, H., Chen, H., Yu, Y., Xiaoyin, G., Hu, Y., Xie, K., et al. (2019). Autophagy Activation Improves Lung Injury and Inflammation in Sepsis. Inflammation 42 (2), 426–439. doi:10.1007/s10753-018-00952-5
Zhou, B. X., Li, J., Liang, X. L., Pan, X. P., Hao, Y. B., Xie, P. F., et al. (2020). β-Sitosterol Ameliorates Influenza A Virus-Induced Proinflammatory Response and Acute Lung Injury in Mice by Disrupting the Cross-Talk between RIG-I and IFN/STAT Signaling. Acta Pharmacol. Sin 41 (9), 1178–1196. doi:10.1038/s41401-020-0403-9
Zhou, Y., Zhou, B., Pache, L., Chang, M., Khodabakhshi, A. H., Tanaseichuk, O., et al. (2019). Metascape Provides a Biologist-Oriented Resource for the Analysis of Systems-Level Datasets. Nat. Commun. 10 (1), 1523. doi:10.1038/s41467-019-09234-6
Zhu, D.-N., Chen, C., Wang, S.-M., and Wang, Y.-F. (2021). Application Progress of Network Pharmacology in Traditional Chinese Research. J. Nanjing Univ. Traditional Chin. Med. 37 (1), 156–160.
Zhu, Y.-Y., Yuan, B., Xu, J.-Y., Zhou, L.-H., and Zou, J.-D. (2014). Study on Effects of Qingfei Oral Liquid on Expression of T- Bet and GATA3 in RSV Infected Mice's Lung Tissue from Perspective of Sustaining Th1/Th2 Type Cytokines Balance. Liaoning J. Traditional Chin. Med. 41 (4), 805–807.
Keywords: respiratory syncytial virus, qingfei oral liquid, network pharmacology, lipidomics, excessive autophagy, PI3K/AKT/mTOR, lipin-1
Citation: Lin L, An L, Chen H, Feng L, Lu M, Liu Y, Chu C, Shan J, Xie T, Wang X and Wang S (2021) Integrated Network Pharmacology and Lipidomics to Reveal the Inhibitory Effect of Qingfei Oral Liquid on Excessive Autophagy in RSV-Induced Lung Inflammation. Front. Pharmacol. 12:777689. doi: 10.3389/fphar.2021.777689
Received: 27 September 2021; Accepted: 12 November 2021;
Published: 01 December 2021.
Edited by:
Vincent Kam Wai Wong, Macau University of Science and Technology, Macao SAR, ChinaReviewed by:
Wing Yan Wong, Hong Kong Polytechnic University, Hong Kong SAR, ChinaCopyright © 2021 Lin, An, Chen, Feng, Lu, Liu, Chu, Shan, Xie, Wang and Wang. This is an open-access article distributed under the terms of the Creative Commons Attribution License (CC BY). The use, distribution or reproduction in other forums is permitted, provided the original author(s) and the copyright owner(s) are credited and that the original publication in this journal is cited, in accordance with accepted academic practice. No use, distribution or reproduction is permitted which does not comply with these terms.
*Correspondence: Lili Lin, bGlubmpAbmp1Y20uZWR1LmNu; Xiaorong Wang, cmNyeXN0YWxAdG9tLmNvbQ==; Shouchuan Wang, d3NjbmpAbmp1Y20uZWR1LmNu
†These authors have contributed equally to this work and share first authorship
Disclaimer: All claims expressed in this article are solely those of the authors and do not necessarily represent those of their affiliated organizations, or those of the publisher, the editors and the reviewers. Any product that may be evaluated in this article or claim that may be made by its manufacturer is not guaranteed or endorsed by the publisher.
Research integrity at Frontiers
Learn more about the work of our research integrity team to safeguard the quality of each article we publish.