- 1Department of Respiratory and Critical Care Medicine, Aerospace Center Hospital, Peking University Aerospace School of Clinical Medicine, Beijing, China
- 2Department of Neurology, Tianjin Fourth Central Hospital, The Fourth Central Hospital Affiliated to Nankai University, The Fourth Central Clinical College, Tianjin Medical University, Tianjin, China
- 3Department of Neurology, The Affiliated Hospital of Chifeng University, Chifeng, China
- 4Department of Pediatric Neurology, Shengjing Hospital of China Medical University, Shenyang, China
- 5Department of Neurology, Aerospace Center Hospital, Peking University Aerospace School of Clinical Medicine, Beijing, China
Parkinson’s disease (PD) is a complex neurodegenerative disorder featuring both motor and nonmotor symptoms associated with a progressive loss of dopaminergic neurons in the substantia nigra pars compacta. Oxidative stress (OS) has been implicated in the pathogenesis of PD. Genetic and environmental factors can produce OS, which has been implicated as a core contributor to the initiation and progression of PD through the degeneration of dopaminergic neurons. The transcription factor nuclear factor erythroid 2-related factor 2 (Nrf2) orchestrates activation of multiple protective genes, including heme oxygenase-1 (HO-1), which protects cells from OS. Nrf2 has also been shown to exert anti-inflammatory effects and modulate both mitochondrial function and biogenesis. Recently, a series of studies have reported that different bioactive compounds were shown to be able to activate Nrf2/antioxidant response element (ARE) and can ameliorate PD-associated neurotoxin, both in animal models and in tissue culture. In this review, we briefly overview the sources of OS and the association between OS and the pathogenesis of PD. Then, we provided a concise overview of Nrf2/ARE pathway and delineated the role played by activation of Nrf2/HO-1 in PD. At last, we expand our discussion to the neuroprotective effects of pharmacological modulation of Nrf2/HO-1 by bioactive compounds and the potential application of Nrf2 activators for the treatment of PD. This review suggests that pharmacological modulation of Nrf2/HO-1 signaling pathway by bioactive compounds is a therapeutic target of PD.
Introduction
Parkinson’s disease (PD) is defined primarily as a movement disorder, with the typical symptoms being resting tremor, rigidity, bradykinesia, and postural instability (Rai et al., 2020; Rai and Singh, 2020; Rai et al., 2021). PD is pathologically characterized by degeneration of nigrostriatal dopaminergic neurons and the presence of Lewy bodies (LBs), which mainly consist of misfolded α-synuclein, ubiquitin, Parkin, PTEN-induced kinase-1 (PINK1), and other proteins in the surviving neurons (Rai et al., 2017, 2019a; Zahra et al., 2020; Oliveira et al., 2021). PD is the second most common age-related neurodegenerative disease, affecting more than 2% of the population older than 65 years old (Aarsland et al., 2017). PD is becoming a major age-related health problem (Zou Y. et al., 2015; Hirsch et al., 2016; Savica et al., 2016).
The majority of PD cases are idiopathic or sporadic, and approximately 10% of PD cases are associated with a genetic component. Even though familial PD is the less frequent form as only 10% of cases comprise only a minor subset of the overall PD pool, these are of high relevance since they have provided extended information about pathogenesis (Cuenca et al., 2018; Deng et al., 2018; Domingo and Klein, 2018). Since the first PD-associated substitution mutation of alanine in position 53 for threonine (A53T) in α-synuclein was identified more than 20 years ago (Polymeropoulos et al., 1997), many other genes with Mendelian inheritance have been identified, and the number of PD-related genes as risk factors has exponentially increased (Brás and Outeiro, 2021; Oliveira et al., 2021). Twenty-three loci and nineteen genes have been directly linked to the cause of genetic PD (Deng et al., 2018) (Table 1). PINK1, leucine-rich repeat kinase 2 (LRRK2), Parkin, DJ-1, and α-synuclein are the proteins that have been strongly linked to the familial PD (Polymeropoulos et al., 1997; Bonifati et al., 2003; Valente et al., 2004; Di Fonzo et al., 2005; Nichols et al., 2005). Of note, because of its predominance in LBs, α-synuclein is most commonly associated with PD pathogenesis (Spillantini et al., 1997). These different mutation genes are involved in the regulation of different pathways, Parkin, and UCHL-1 for proteasomal degradation pathways; PINK1, Omi/Htra, DJ-1, and LRRK2 for mitochondrial homeostasis; DJ-1 for antioxidant response pathways; ATP13A2 for lysosome function; and PINK1 and Parkin for mitophagy.
Despite all the efforts that have been directed to interpret which mechanisms are responsible for neuronal degeneration in PD, its origin and the cause of PD remain unknown in most patients and remain to be fully elucidated (Przedborski, 2017), leading to unsustainable treatment options that only provide symptomatic relief, and there are no preventative or curative therapies that slow the neurodegenerative process. Most PD cases have a multifactorial etiology and a complicated interplay of genetic and environmental factors, which affect numerous fundamental cellular processes. Since 1992, the oxidative stress (OS) hypothesis came into existence with an observation of postmortem brain of PD patients (Fahn and Cohen, 1992); accumulating evidence indicates that OS leads to the neurodegeneration of these DA neurons (Dias et al., 2013; Blesa et al., 2015; Sarrafchi et al., 2016; Puspita et al., 2017; Guo et al., 2018). It is now believed that OS plays an important role during the pathogenesis of PD (Subramaniam and Chesselet, 2013). Ample evidence has supported the OS hypothesis, which prompted an investigation into the efficacy of nonenzymatic exogenous antioxidants to treat PD (Todorovic et al., 2016). More recently, much attention and interest have been centered on targeting antioxidant gene transcription through pharmacological modulation, which leads to mitigating OS-dependent neuronal injury (Buendia et al., 2016). The common target is Nrf2, which is a transcription factor and “master regulator.” Cells have been equipped with a complex endogenous protection system against OS through the antioxidant response element (ARE) pathway, which renders neuronal cells resistant to OS. The nuclear factor E2-related factor 2 (Nrf2) regulates this coordinated induction of detoxifying and antioxidative enzymes through the binding of the ARE within the regulatory region of target genes. Nrf2 regulates the coordinated expression of cytoprotective genes, including heme oxygenase-1 (HO-1), among other enzymes (Jiang L. et al., 2016; Deshmukh et al., 2017). Thus, considering the neuroprotective role of the Nrf2/HO-1 pathway, pharmacological modulation of the activation of Nrf2/HO-1 may represent a novel therapeutic target for the treatments of PD (Cuadrado et al., 2018). Currently, ongoing investigations have been focused on the potential of natural compounds targeting the Nrf2/HO-1 signaling pathway as a neuroprotective agent for the therapeutic treatment of PD. Therefore, it will be vital to summarize the current literature on Nrf2/HO-1 signaling pathway in PD.
Here, we briefly overview the sources of OS and the association between OS and the pathogenesis of PD. Then, we provided a concise overview of the Keap1/Nrf2/ARE pathway and delineated the role played by activation of Nrf2/HO-1 in PD. Following this background, we expand our discussion to the neuroprotective effects of pharmacological modulation of Nrf2/HO-1 by bioactive compounds and the potential application of Nrf2 activators for the treatment of PD. This review suggests that pharmacological modulation of Nrf2/HO-1 signaling pathway by bioactive compounds is a therapeutic target of PD.
The Role of Oxidative Stress in Parkinson’s Disease
General Aspects Regarding Oxidative Stress
OS was first introduced by Helmut Sies in 1985, who stated “A disturbance in the prooxidant/antioxidant systems in favor of the former may be denoted as an OS” (Sies, 2020a; Lushchak and Storey, 2021). More recently, OS was defined as a disequilibrium between the levels of produced reactive oxygen species (ROS) and the ability of a biological system to readily detoxify the reactive intermediates or to repair the resulting damage, creating a perilous state contributing to cellular damage (Ji and Yeo, 2021). Many complex mechanisms maintained the delicate balance between ROS generation and elimination. The dysfunction of any of these mechanisms could result in alterations in cellular redox status. An increase in ROS production or a decrease in ROS-scavenging capacity resulting from exogenous stimuli or endogenous metabolic alterations can disrupt redox homeostasis, leading to OS.
ROS is a collective term that describes the oxygen-derived small molecules that are formed upon incomplete reduction of oxygen. ROS includes oxygen radicals and certain nonradicals that either are oxidizing agents or are easily converted into radicals. Oxygen radicals include O2•– (superoxide anion), HO• (hydroxyl radical), RO2• (peroxyl), and RO• (alkoxyl), and certain nonradicals include HOCl (hypochlorous acid), O3 (ozone),1O2 (singlet oxygen), and H2O2 (hydrogen peroxide) (Bedard and Krause, 2007; D'Autréaux and Toledano, 2007). The greater chemical reactivity of ROS with regard to oxygen mediates the toxicity of oxygen (Gutowski and Kowalczyk, 2013).
O2•– is considered the “primary” ROS, which is produced mainly by mitochondrial complexes I and III of the electron transport chain (ETC), is highly reactive, and can easily cross the inner mitochondrial membrane (IMM), where it can be reduced to H2O2 (Elfawy and Das, 2019).
O2•– can further interact with other molecules to generate “secondary” ROS either directly or prevalently through enzyme- or metal-catalyzed processes. The “secondary” ROS are highly reactive and can attack and damage DNA, purines, pyrimidines, deoxyribose backbone, leading to mutation (Pisoschi et al., 2021). OS causes injury to macromolecular components (DNA, proteins, and lipids), which lead to various pathological conditions and human diseases, such as PD.
ROS can be either harmful or beneficial to living systems, which make them play a dual role as both deleterious and beneficial species. ROS exerts beneficial effects at low to moderate concentrations, which involve physiological roles in cellular responses to noxia, such as in defense against infectious agents and cellular signaling systems (Sachdev et al., 2021). The balance between harmful and beneficial effects of free radicals is a very important aspect of living organisms. This balance is achieved by mechanisms called “redox regulation.” The process of “redox regulation” maintains “redox homeostasis” and protects living organisms from various OS and by controlling the redox status in vivo (Sies, 2020b).
In response to OS, cells have developed and are equipped with an antioxidant defense system, which uses enzymatic and nonenzymatic antioxidant systems to eliminate ROS and maintain redox homeostasis, thereby protecting cells from damage (Trachootham et al., 2008). Nonenzymatic defenses are the thiol-containing small molecules, including compounds of intrinsic antioxidant properties, such as thioredoxin (Txn), glutathione (GSH), vitamins C and E, and β-carotene. Purely enzymatic defenses ROS-inactivating enzymes, such as glutathione peroxide (GPx), superoxide dismutases (SOD), catalases (CAT), and peroxidases, can exert a protective effect through directly scavenging superoxide radicals and hydrogen peroxide, therefore converting them to less reactive species (Jung and Kwak, 2010). CAT, SOD, and GPx directly neutralize ROS. GSH and Txn neutralize ROS via direct interactions serving as substrates for GPx and peroxiredoxins (Prxs). CAT, GPx, and Prxs reduce hydrogen peroxide to water. Antioxidants can be classified into endogenous and exogenous or direct antioxidants, indirect antioxidants, and bifunctional antioxidants according to source, nature, and mechanism of action (Dinkova-Kostova and Talalay, 2008; Magesh et al., 2012). Direct antioxidants are redox-active and short-lived, and they are consumed during the process and need to be regenerated to offer further protection. Indirect antioxidants show with or without redox activity and exert their antioxidant effects through upregulating various antioxidant genes such as HO-1, NAD(P)H, NAD(P)H:quinone oxidoreductase 1 (NQO1), glutathione S-transferase (GST), glutamate-cysteine ligase (GCL), SOD, GPx, CAT, and Txn (Talalay, 2000). These protective proteins have relatively long half-lives, are not consumed during their antioxidant actions, are members of this antioxidant system, and are referred to as the “ultimate antioxidants.” They catalyze various chemical detoxification reactions related to the regeneration of some direct antioxidants (Dinkova-Kostova and Talalay, 2008; Magesh et al., 2012). The Keap1, Nrf2, and ARE are the three main cellular components involved in regulating antioxidant response. The Keap1/Nrf2/ARE is a major signaling pathway that regulates the basal and inducible expression of a wide array of antioxidant genes (Cuadrado et al., 2019). The Keap1/Nrf2/ARE signaling pathway induces an adaptive response for OS that can otherwise lead to PD. Thus, targeting the Keap1/Nrf2/ARE pathway is being regarded as a rational strategy to prevent and treat PD.
Evidence of Oxidative Stress in PD
OS leads to cellular dysfunction and eventual cell death in both familial and sporadic forms of PD. Both postmortem studies, modeling of PD in animals with toxins in neuronal degeneration of the DAergic nigral neurons (Gilgun-Sherki et al., 2001; Mythri et al., 2011), and in vivo observations of patients with PD supported the occurrence of OS in PD (Vinish et al., 2011).
Ample of studies on postmortem brain tissues of PD patients has shown decreased levels of antioxidant enzyme activity (including GPx and CAT), reduced levels of GSH, elevated free iron levels, an augmented activity of SOD, and a decreased mitochondrial complex I activity in the SN of PD patients (Morris and Edwardson, 1994; Pearce et al., 1997; Blum et al., 2001; Jenner, 2003). Evidence showed a selective loss of GSH in the SN (Sian et al., 1994), which is thought of to be one of the earliest biochemical changes in PD (Perry et al., 1982, 1984; Riederer et al., 1989; Danielson and Andersen, 2008) and is not found in other parts of the brain (Sian et al., 1994). Studies have also demonstrated a reduction in mitochondrial complex I activity in PD compared to controls (Mizuno et al., 1989; Parker et al., 1989; Schapira et al., 1989; Mann et al., 1992).
Accumulating evidence indicates that OS markers, such as high levels of oxidatively modified lipids, proteins, and DNA/RNA, are all found in the SNpc of postmortem brains of PD patients. Compared with other brain regions and age-matched controls, cholesterol lipid hydroperoxides and malondialdehyde, the lipid peroxidation products, are 10-fold higher (Dexter et al., 1989). The amounts of nitrotyrosine (3-NT), a marker of damage to protein, have been identified in peripheral polymorphonuclear cells in PD patients and increased in their brains in LBs (Good et al., 1998; Gatto et al., 2000). Increased levels of carbonyl modifications of soluble proteins are also found throughout the brain in PD (Alam et al., 1997a). Meanwhile, the byproduct of lipid peroxidation, 4-hydroxyl-2-nonenal (HNE), is also increased in the SN of PD patients (Yoritaka et al., 1996). Lastly, DNA and RNA oxidation products 8-hydroxydeoxyguanosine (8-OHdG) and 8-hydroxy-guanosine (8-OHG) are also increased in the SN and cerebrospinal fluid of PD patients (Alam et al., 1997b; Zhang et al., 1999; Kikuchi et al., 2002; Isobe et al., 2010).
Evidence of OS existing in PD is further supported by PD animals modeled with toxins that can cause OS, which includes 1-methyl-4-phenyl-1,2,3,6-tetrahydropyridine (MPTP) (Dong et al., 2021), rotenone (Sharma et al., 2021), paraquat (Ahmad et al., 2021), and 6-hydroxydopamine (6-OHDA) (Zou et al., 2021). Moreover, in vivo observations revealed that several markers of OS are altered in the cerebrospinal fluid and blood samples of PD patients (Vinish et al., 2011).
The Sources of Oxidative Stress in Parkinson’s Disease
Numerous pieces of evidence suggest that a number of sources and mechanisms for OS are recognized in PD. The major sources of OS in PD include mitochondrial dysfunction, nicotinamide adenine dinucleotide phosphate (NADPH) oxidases (NOX) activation, the metabolism of dopamine by autooxidation, and iron (Fe2+) accumulation (Figure 1). We will discuss how these PD-associated factors induce ROS and how ROS results in cell death in dopaminergic neurons in PD.
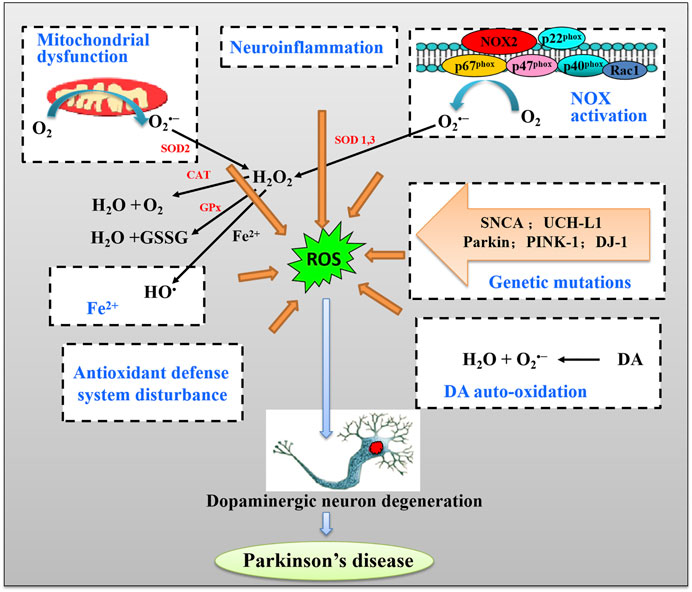
FIGURE 1. The schematic pathway of major sources of oxidative stress (OS) and induction of DA neuron death in Parkinson’s disease (PD).
Mitochondrial Dysfunction and Oxidative Stress in PD
Mitochondria are an organelle for their cellular function essential for their role in ATP production, calcium homeostasis, and apoptotic signaling. In eukaryotic cells, mitochondria are the primary source of energy through the process of respiration and oxidative phosphorylation (OXPH) to produce adenosine triphosphate (ATP). The process of OXPH involves coupling of both redox and phosphorylation reactions in the IMM, leading to effective ATP synthesis. During this process, electrons donated from nicotinamide adenine dinucleotide (NADH) or flavin adenine dinucleotide (FADH2) are transported through the ETC, which is comprised of complexes I–IV, to produce water and create a proton electrochemical gradient across the IMM (Subramaniam and Chesselet, 2013). The ETC constitutes electron carriers that transport electrons from reduced cofactors, which are reduced during the catabolism of energy nutrients, to molecular oxygen. This comprises the primary energy transformation step. The designated protonmotive force, i.e., the dual gradient across the IMM, is composed of a pH and electrical potential, which provides the driving force for ATP synthesis through the backflow of protons into the mitochondrial matrix through the ATP synthase complex (Figure 2). Protons flow back into the mitochondrial matrix providing energy for the ATP synthase to phosphorylate ADP into ATP. This metabolic process is a critical means of energy production and the main source of O2•– and H2O2 as a major byproduct, leading to propagation of free radicals, thereby contributing to the disease (Boveris and Chance, 1973; Turrens, 2003; Figueira et al., 2013) (Figure 2).
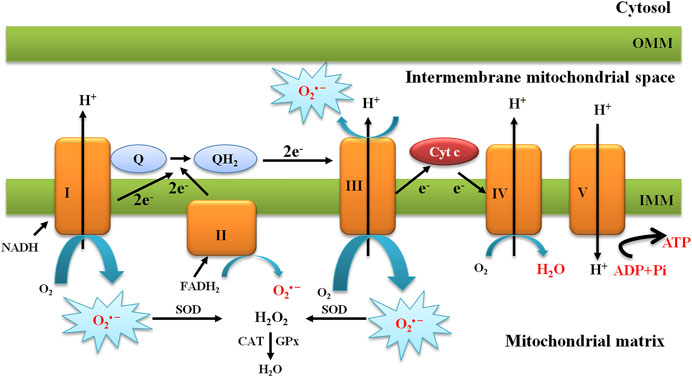
FIGURE 2. Schematic presentation of the mitochondrial electron transport chain and production of mitochondrial O2•−. The mitochondrial electron transport chain produces ROS. Mitochondrial complexes I and II use electrons donated from NADH and FADH2 to reduce coenzyme Q. Coenzyme Q shuttles these electrons to complex III, where they are transferred to cytochrome c. Complex IV uses electrons from cytochrome c to reduce molecular oxygen to water. The action of complexes I, III, and IV produce a proton electrochemical potential gradient, the free energy of which is used to phosphorylate ADP at ATP synthase. Complexes I, II, and III produce superoxide through the incomplete reduction of oxygen to superoxide, whereas complexes I and II produce superoxide only into the mitochondrial matrix and complex III produces superoxide into both the matrix and the intermembrane space.
The main sites of ROS production in mitochondria are considered to be complexes I III in the ETC. The primary ROS produced in mitochondria is O2•–, which results from a single electron transfer to O2 in the respiratory chain. Superoxide dismutase 2 (SOD2) or MnSOD converts O2•– to H2O2, which is further detoxified by the CAT. Redox-active metals such as Fe2+ also contribute to ROS generation. The highly reactive HO• can be generated through the Fenton reaction or Haber-Weiss reaction in the presence of Fe2+, causing severe oxidative damage to the cellular components and leading to DNA damage and lipid damage (Kehrer, 2000) (Figure 2).
Many lines of evidence provide substantial evidence that mitochondrial dysfunction involves in the pathogenesis of PD. Histology of postmortem brains of PD patients, which supports the notion of mitochondrial dysfunction, is a common pathological mechanism employed in PD pathology (Chaturvedi and Beal, 2008; Isobe et al., 2010). Accidental administration of MPTP in young drug users, who eventually developed parkinsonism, reveals significant lesions of DAergic neurons in the SNpc (Langston et al., 1983). It was reported that deficiency in mitochondrial complex I was identified for the first time in PD brains but remains normal in other neuronal regions (Schapira et al., 1989, 1990). Since then, ample evidence has been well documented on the role of mitochondrial dysfunction in the pathogenesis of PD. Mounting evidence has shown that mitochondrial dysfunction is one unique feature observed in PD (Figure 3) (Prasuhn et al., 2020). Numerous studies have suggested that mitochondria are the primary source of ROS and contribute to the intracellular OS in PD (Onyango, 2008; Hauser and Hastings, 2013; Subramaniam and Chesselet, 2013). Complex I deficiencies of the ETC account for the majority of sources of ROS in PD. Premature electron leakage from complex I and complex III of ETC to oxygen is the main source of mitochondrial O2•–(Kussmaul and Hirst, 2006). The dysfunction of ETC in damaged mitochondrial leads to excessive ROS production, which is quite detrimental to cells, resulting in dopaminergic neuron death. ROS also triggers the autophagy/mitophagy process, with the consequent removal of damaged mitochondria, and in turn enhances cellular survival (Elfawy and Das, 2019). However, once the accumulation of ROS results from OS, proteins and toxic wastes can be deposited in the brain, thereby leading to dysfunction of the brain. Along with increased production of ROS, decreased production of antioxidant enzymes can together lead to neurodegeneration in PD. ROS can damage mtDNA by inducing mutations, leading to more dysfunction of OXPH and mitochondrial morphology, resulting in the vicious cycle of the mitochondria in PD (Elfawy and Das, 2019). Mitochondrial dysfunction also causes the decreased production of ATP, an influx of calcium, and the opening of the mitochondrial permeability pore, eventually resulting in apoptosis.
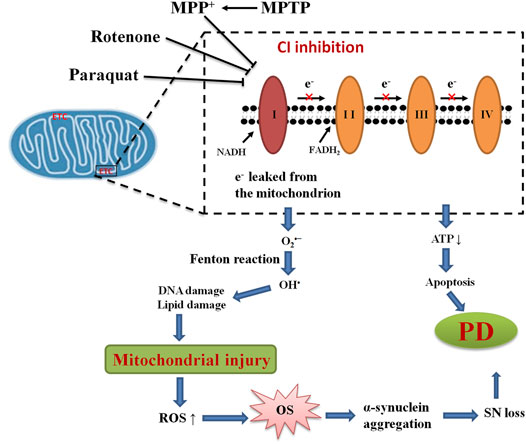
FIGURE 3. Mitochondrial dysfunction and OS in PD. The mechanism of mitochondrial dysfunction and OS highlights the inhibition of mitochondrial complex I (CI) and associated ROS production leading to loss of dopaminergic neurons in PD.
NADPH Oxidases Activation and Oxidative Stress in PD
NADPH oxidases are a family of membrane-bound, multisubunit enzyme complexes. The primary function of NADPH oxidases is to transfer electrons across the plasma membrane from NADPH to molecular oxygen via their “Nox” catalytic subunit to generate O2•− and subsequently ROS, including H2O2 and HO (Figure 4) (Tarafdar and Pula, 2018). NADPH oxidases consist of two membrane-bound components and three components in the cytosol, plus rac 1 or rac 2. The NADPH oxidase family of enzymes, consisting of seven members in mammalian species (NOX2, NOX1, NOX3, NOX4, NOX5, DUOX1, and DUOX2-containing NADPH oxidases), was a major source of ROS that is important in diverse cellular functions, including antimicrobial defense, inflammation, and redox signaling (Bedard and Krause, 2007). According to the new terminology, the catalytic subunit of NADPH oxidases includes NOX2 (gp91phox), and its six homologs (NOX1, NOX3, NOX4, NOX5, DUOX1, and DUOX2) are referred to as the NOX family. These seven isoforms, sharing not only conserved functions but also conserved structural properties, are transmembrane proteins and primarily distinguished by the presence of the distinct membrane-spanning catalytic “Nox” (Nox1-Nox5) or “Duox” subunit (Duox1-Duox 2), which mediate the electron transfer process from NADPH to molecular oxygen (Vermot et al., 2021). The catalytic NOX subunits have unique distribution patterns and are widely expressed in different tissues throughout the body. Many cells express several NOX isoforms; differences in subcellular distributions and activation mechanisms of different NOX isoforms might explain the nonredundancy in their functions [for a review see: references (Bedard and Krause, 2007; Ma et al., 2017)].
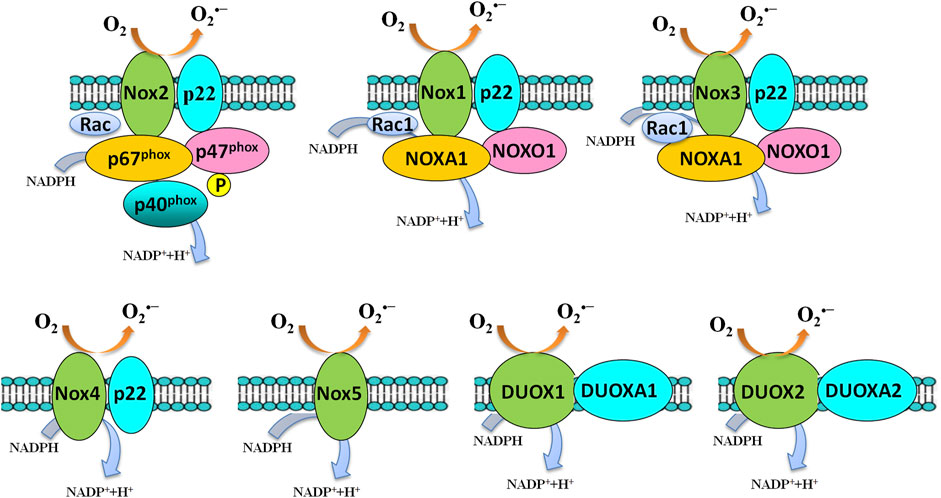
FIGURE 4. Activation of the NADPH oxidase family members. Several components and domains make up the transmembrane-active enzyme complexes of NADPH oxidase isoforms. NOX1-5 and DUOX1/DUOX2 are shown. Upon activation, an electron will be transferred from NADPH to O2 to form superoxide. NOX4-generated superoxide undergoes rapid conversion into hydrogen peroxide, which mediates many of its downstream effects. NOX5 and the DUOX enzymes are sensitive to cellular Ca2+ concentrations.
NOX2-containing NADPH oxidases are the best-characterized member of the NADPH oxidase family of enzymes (Figure 4). NOX2-containing NADPH oxidases were firstly identified and discovered in studying a process called “respiratory burst” in neutrophils (Berendes et al., 1957; Sbarra and Karnovsky, 1959; Rossi and Zatti, 1964; Babior et al., 1973, 1975; Segal et al., 1978; Segal and Jones, 1978). Two research groups cloned the gene coding for the catalytic subunit of the phagocyte NADPH oxidase, i.e., the gp91phox in the late 1980s (Royer-Pokora et al., 1986; Teahan et al., 1987). gp91phox is now called NOX2 in the novel NOX terminology.
NADPH oxidases are activity-dependent, which activation usually requires the translocation of cytosolic subunits to the membrane-bound subunits p22phox and NOX isoforms. NOX2 (gp91phox) is the best-characterized member of the NOX family. Once stimulation, the cytosolic subunits of NADPH oxidases, i.e., p47phox, p67phox, p40phox, and the small Rho GTPase, Rac1, or Rac2, translocate to the membrane-bound p22phox/NOX2 heterodimer to assemble the active NADPH oxidases complexes, which catalyzes the reduction of O2 to generate O2•− and subsequently H2O2 and HO•.
Mounting evidence has shown that microglial NOX2 contributes to CNS OS and neuronal damage. NOX2-containing NADPH oxidases have emerged as a major source of OS in PD (Gao et al., 2003a; Qin et al., 2004; Wu et al., 2005; Kim et al., 2007; Gao et al., 2012; Marrali et al., 2018; Sun et al., 2020) (Figure 5). NOX2 is expressed in several regions of the brain and various cell types, including neurons at the striatum (McCann et al., 2008; Guemez-Gamboa et al., 2011), substantia nigra (Zawada et al., 2011; Qin et al., 2013), and midbrain (Qin et al., 2013), and is heavily expressed in microglia than in neurons and astroglia. Postmortem SN samples from brains of patients with PD had higher NOX2 protein content than samples from control individuals, and an increase of NOX2 was also observed in microglia in the ventral midbrain of MPTP-treated mice (Wu et al., 2003). The same study also showed that NOX2 is upregulated in SNpc of mice after repeated intraperitoneal injections of MPTP. The upregulation of NOX2 coincides with the local production of ROS, microglial activation, and DA neuronal loss. NOX2 knockdown abates MPTP-associated ROS production and shows less SNpc DA neuronal loss than their WT littermates (Wu et al., 2003). These findings support that microglial NOX2 is a common pathway for selective DA neurotoxicity. Since then, ample evidence has been well documented on the role of microglial NADPH oxidase activation in the pathogenesis of PD. This study was corroborated by numerous in vitro cell cultures lacking functional NOX2 failing to produce neurotoxicity induced by MPP+ (Kim et al., 2007; Zhang et al., 2008; Jiang T. et al., 2016), MPTP (Gao et al., 2003b, 2003c; Kim et al., 2007), paraquat (Wu et al., 2005), or rotenone (Gao et al., 2003a) and in vivo studies show that mice lacking NOX2 receiving MPTP (Kim et al., 2007), paraquat (Purisai et al., 2007), and 6-OHDA (Hernandes et al., 2013a; 2013b) are less sensitive to dopaminergic degeneration. Many studies have suggested that NADPH oxidase has been linked to microglia-derived OS after a variety of PD-related neurotoxin, for example, 6-OHDA (Rodriguez-Pallares et al., 2007), rotenone (Gao et al., 2002), paraquat (Wu et al., 2005), and α-synuclein (Zhang et al., 2005), which suggest that microglia are the major NOX2-expressing cells in PD and in PD experimental models. Microglial NADPH oxidase activation and NOX2-containing NADPH oxidases-derived ROS have been suggested to contribute to the injury to DA neurons in PD, which may be a common denominator associated with neurotoxicity in PD, and could contribute to its pathophysiology.
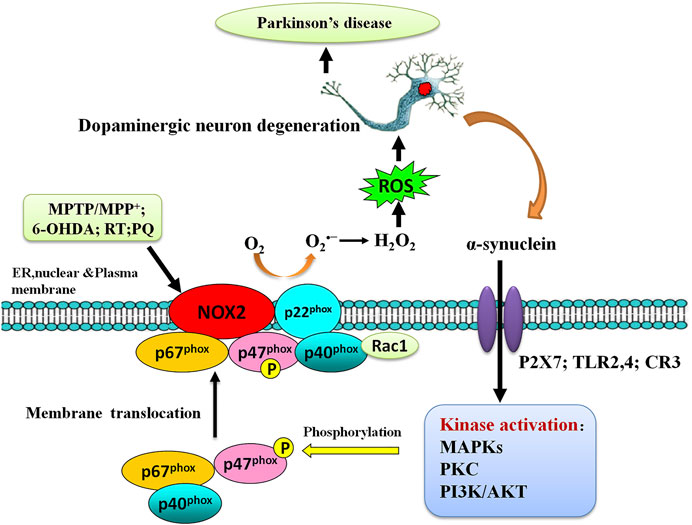
FIGURE 5. Alpha-synuclein and microglial Nox2 activation. The activation of microglia by alpha-synuclein can implicate several cell-surface receptors, such as P2X7, TLR2/4, and CR3, and subsequent activation of several kinases, such as PKC, Akt, MAPKs, PAK, and ERK1/2. This in turn could promote the phosphorylation and translocation of p47phox and subsequent Nox2 activation. Released oxygen species appear to promote microglia chemoattraction, activation, and OS. Neuronal damage leads to the release of alpha-synuclein and the TLR-agonist high mobility group box protein 1 (HMGB1).
Oxidative Stress Caused by Dopamine Autooxidation
The pathological hallmarks of PD are selective degeneration of the DA neurons of the SN, which is more vulnerable ROS generated by the nigral DA neurons during dopamine metabolism (Chinta and Andersen, 2008), suggesting the possibility that dopamine itself may lead to the neurodegenerative process (Hastings, 2009). Under normal conditions, dopamine is synthesized from tyrosine in the cytosol, in which the phenol ring undergoes hydroxylation to levodopa under the catalysis of tyrosine hydroxylase (TH). The TH is the rate-limiting enzyme in dopamine biosynthesis (Figure 6). Levodopa is then decarboxylated to DA by the enzyme aromatic L-amino acid decarboxylase (AADC) (Napolitano et al., 2011; Segura-Aguilar et al., 2016). Once formed, dopamine is safely stored in high millimolar concentrations in synaptic vesicles after uptake by vesicular monoamine transporter 2 (VMAT2) (Miesenböck et al., 1998; Staal et al., 2004). TH and AADC are associated with VMAT-2 generating a complex.
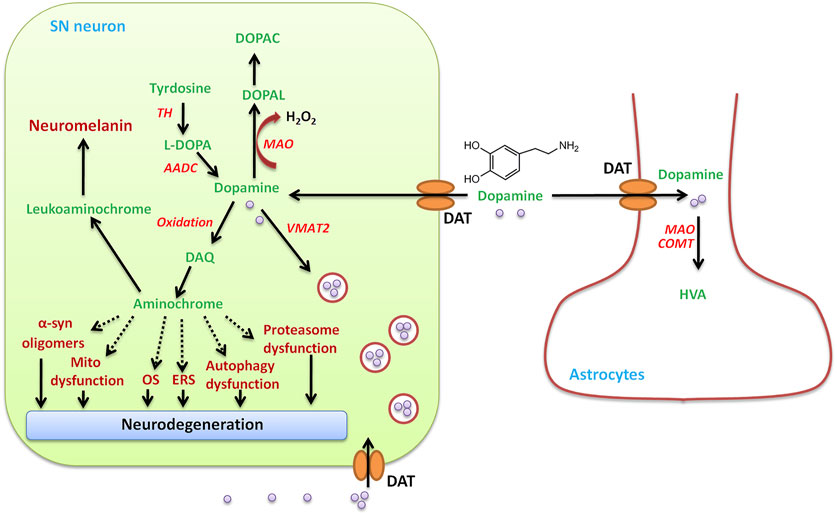
FIGURE 6. SN neuron DA metabolism. In addition to the uptake of DA by the DA transporter (DAT) from outside, DAergic neurons in the SN produce DA under the action of tyrosine hydroxylase (TH) and aromatic amino acid decarboxylase (AADC) in the cytosol. The amino acid tyrosine is converted to L-dopa using TH, and the second L-dopa is converted to dopamine using AADC. The newly synthesized or taken up DA is immediately transported to and stored in the monoaminergic vesicles by VMAT-2, preventing the existence of free dopamine in the cytosol. Cytosolic DA oxidizes to dopamine o-quinone (DAQ), which is immediately cyclized to aminochrome, which induces mitochondrial dysfunction, endoplasmic reticulum stress (ERS), oxidative stress (OS), the aggregation of alpha-synuclein, and the dysfunction of protein degradation. Dopamine in the cytosol can be degraded by monoamine oxidase-mediated degradation to 3,4-dihydroxyphenylacetaldehyde (DOPAL), hydrogen peroxide, and ammonia, which is converted to 3,4-dihydroxyphenylacetic acid (DOPAC) by aldehyde dehydrogenase. DA can be taken up into glial cells and degraded by catechol-o-methyltransferase (CMOT) or monoamine oxidase (MAO) to form homovanillic acid (HVA).
Excess DA that is not stored in vesicles by VMAT2 will undergo either degradation or oxidation in the cytosol (Zhang B. et al., 2019). The MAO-mediated degradation of DA produces H2O2, which leads to OS in PD. DA eventually degrades to homovanillic acid (HVA) under the action of monoamine oxidase-B (MAO-B) and catechol-o-methyltransferase (COMT), producing H2O2 (Zhang S. et al., 2019). Once the excitation of DAergic neurons, dopamine in synaptic vesicles is released into the synaptic cleft from dopaminergic axon terminals and then binds to its receptors that are localized in postsynaptic dendrites/neurons (Werkman et al., 2006; Zhang B. et al., 2019). At a later stage, the excitation signal is terminated and the extracellular free DA is removed from the synaptic cleft by the dopamine transporter (DAT) expressed on the dopaminergic nerve endings and can be reutilized by DAergic neurons or taken up by astrocytes. The DA that DAT-mediated took up in DAergic neurons is sequestered by VMAT2 into synaptic vesicles. DA leaking from synaptic vesicles accumulates in the cytosol and then is degraded by MAO-B, producing hydrogen peroxide and 3,4-dihydroxyphenylacetaldehyde (DOPAL) (Zucca et al., 2017), which is then reduced to inactive 3,4-dihydroxyphenylethanol (DOPET) or further oxidized to 3,4-dihydroxyphenylacetic acid (DOPAC) by alcohol dehydrogenase (ADH) or acetaldehyde dehydrogenase (ALDH) (Herrera et al., 2017). Astrocytes can also take up DA in the synaptic cleft and easily degrade DA by MAO and COMT, which catalyzes the methylation of DOPAC to finally form HVA, the main product of DA degradation (Inyushin et al., 2012).
DA autooxidation, another source of OS to DAergic neurons, forms ROS and reactive o-quinones, which include DA-o-quinone (DAQ) and aminochrome (Zucca et al., 2017). When free DA in the cytosol of DA neurons exceeds the physiological content, DA can oxidize to DAQ, where they finally polymerize, producing neuromelanin (Segura-Aguilar et al., 2014; Herrera et al., 2017), which immediately cyclizes to aminochrome (Herrera et al., 2017). Aminochrome then participates in neurotoxic reactions by inducing chronic neurotoxicity in the dopaminergic neurons. Aminochrome can result in α-synuclein modification (generating neurotoxic oligomers), mitochondrial dysfunction, OS, autophagy dysfunction, proteasomal dysfunction, and endoplasmic reticulum stress (Herrera et al., 2017), all of which are related to cellular changes in PD.
Iron Accumulation (Fe2+) and Oxidative Stress in PD
Specifically increased content of iron in SN is another common hallmark of PD brains, suggesting the possibility that iron may contribute to the selective degeneration of the DA neurons in the SN. Lhermitte’s pioneering study has shown the occurrence of abnorma1 iron deposits in the brain of PD patients (Lhermitte et al., 1924). After that pioneering study, accumulating evidence suggests that iron accumulation results in OS in PD. A more detailed description of the molecular mechanism by which iron leads to OS in PD is seen in other reviews (Ke and Qian, 2007; Weinreb et al., 2013; Belaidi and Bush, 2016; Xu et al., 2017; Zucca et al., 2017; Chen et al., 2019).
Nrf2/ARE Pathway and PD
Concise Overview of Keap1/Nrf2/ARE Pathway
Based on previous works, targeting Keap1/Nrf2/ARE pathway is becoming a strong candidate for therapy for neurodegenerative disease (Zgorzynska et al., 2021). As a core factor, Nrf2 orchestrates the cytoprotective pathway and regulates the expression of several protective genes containing AREs in their promoters, which function to restore homeostasis after combatting OS (Bento-Pereira and Dinkova-Kostova, 2021). Nrf2 was discovered as a member of the human cap’n’collar (CNC) basic-region leucine zipper transcription factor family in 1994 (Moi et al., 1994). In the nucleus, NRF2 forms complexes with small musculoaponeurotic fibrosarcoma protein (MAF) K, G, and F, which recognizes and is bound to an enhancer sequence termed ARE; the latter is present in the regulatory regions of over 250 genes (i.e., ARE genes) (Cuadrado et al., 2018). In unstressed conditions, KEAP1 and CULLIN3 (CUL3) form a ubiquitin E3 ligase complex in the cytoplasm, which polyubiquitinates NRF2 for rapid degradation through the proteasome system (Yamamoto et al., 2018). NRF2 is synthesized but constantly degraded. KEAP1 was identified as a repressor of Nrf2 in 1999 (Itoh et al., 1999). KEAP1 functions were identified as a sensor, while NRF2 plays a role as an effector for the coordinated activation of cytoprotective genes in the KEAP1/NRF2 system. Nrf2 regulates the expression of a battery of cytoprotective genes involved in several cellular processes, such as xenobiotic metabolism and detoxification, ROS scavenging, glutathione, NADPH homeostasis, and autophagy (Bento-Pereira and Dinkova-Kostova, 2021).
The Keap1/Nrf2/ARE signaling pathway is primarily regulated by Keap1-dependent and Keap1-independent mechanisms [more detail seen in other reviews (Bryan et al., 2013; Zhang et al., 2013; Tebay et al., 2015; Zenkov et al., 2017)]. In brief, the activity of Nrf2 is primarily regulated by Keap1, through its interaction with Keap1 which directs the transcription factor for proteasomal degradation. OS or exposure to electrophilic agents can react with Keap1 and stabilize Nrf2, leading to nuclear accumulation of Nrf2 and upregulated Nrf2 protein levels. Once in the nucleus, Nrf2 dimerizes with small Maf proteins and binds to the ARE, leading to transcriptionally driving the expression of several protective genes. The alternative Keap1-independent regulation mechanisms of Nrf2 include protein kinases-induced phosphorylation of Nrf2, interaction with other protein partners, and epigenetic factors (Zhou et al., 2019). Human Nrf2 contains a large number of serine, threonine, and tyrosine residues (17%), which can be phosphorylated by the protein kinases, which belong to various families, including PKC, JNK, PI3K, ERK, p38 MAPK, PERK, AMPK, and GSK-3β, all of which participate in regulating Nrf2 stability and translocation into the nucleus and bind to ARE (Zenkov et al., 2017; Rai et al., 2019b).
Growing experimental evidence implicates that the Keap1/Nrf2 system serves as an attractive drug development target in PD. Nrf2 may play several significant roles in mitochondrial function, which provides a potential therapeutic target for mitochondrial dysfunction in PD. Activation of Nrf2 by natural bioactive compounds is a promising approach for PD.
Role Played by Nrf2 During PD
The pioneering studies of Johnson and colleagues have provided the proof of concept that activation of Nrf2 protects cells and animal models against OS-associated neurodegeneration and revealed appropriate strategies for induction of Nrf2 through pharmacologic modulation to combat OS (Lee et al., 2003a; Shih et al., 2003; Kraft et al., 2004; Johnson et al., 2008; Calkins et al., 2009). Systematic Nrf2 deficiency sensitizes neurons to 3-NP toxicity in cell culture and in whole animals (Calkins et al., 2005). Nrf2 knockout mice are significantly more sensitive to mitochondrial complex I and II inhibitors (Johnson et al., 2008).
Recent evidence has proven that Nrf2 is a novel neuroprotective platform that rendered resistance to a variety of PD-related OS-dependent neurotoxin insults. Regarding PD, evidence from Nrf2 deficiency in cell and animal models supports the functional importance of Nrf2 during PD. Nrf2 protects mixed primary astrocytes and neurons through coordinate upregulation of ARE-driven genes. Nrf2-/- neurons in primary neuronal cultures containing both astrocytes and neurons were more sensitive to MPTP or rotenone (Lee et al., 2003b). This observation was corroborated by further studies, which reported that Nrf2 deficiency exacerbates vulnerability to the 6-OHDA both in vitro and in vivo (Jakel et al., 2007). They further showed that tert-butylhydroquinone activates the Nrf2/ARE pathway and protects against 6-OHDA in vitro. Induction of Nrf2/ARE by transplantation of astrocytes overexpressed Nrf2 can protect living mice against 6-OHDA-induced damage (Jakel et al., 2007). Nrf2 deficiency increases the vulnerability to PD-related neurotoxin MPTP sensitivity in vivo (Chen et al., 2009). Using siRNA knockdown of Keap1, activation of the Nrf2/ARE pathway can reduce OS and partially provide protection against MPTP-mediated neurotoxicity (Williamson et al., 2012). Overexpression of Nrf2 in astrocyte delays synuclein aggregation and motor deficit throughout the CNS in the alpha-synuclein mutant (A53T) mouse model, suggesting that Nrf2 in astrocytes exerts neuroprotection from hSYN(A53T)-mediated toxicity through promoting the degradation of hSYN(A53T) via autophagy-lysosome pathway in vivo. Thus, activation of the astrocytes Nrf2 is a potential target to develop therapeutic strategies for treating PD (Gan et al., 2012). Collectively, these studies suggest that the Nrf2/ARE pathway is a promising target for therapeutics in PD (Jakel et al., 2007).
Nrf2/ARE/HO-1 Pathway and Therapeutic Modulation of Parkinson’s Disease
Neuroprotective Role of the Activation of Nrf2 in PD
Mounting evidence indicates that activators of the Nrf2/ARE pathway displayed significantly greater resistance to neurotoxicity induced by 6-OHDA (Table 2), MPP+ (Table 3), MPTP (Table 4), paraquat (Table 5), and rotenone-induced (Table 6) in vitro or in vivo model. The presence of activation of Nrf2 by pharmacologic compounds was shown to exert neuroprotection, or conversely, Nrf2 deficiency led to exacerbating neuron sensitivity to the neurotoxin. It is becoming evident from the published literature that activation of Nrf2 can protect against PD-related neurotoxin-induced neurotoxicity when activated before or coincident with neurotoxin exposure. Targeting Nrf2 activity is emerging as a strong candidate for the treatment of PD.
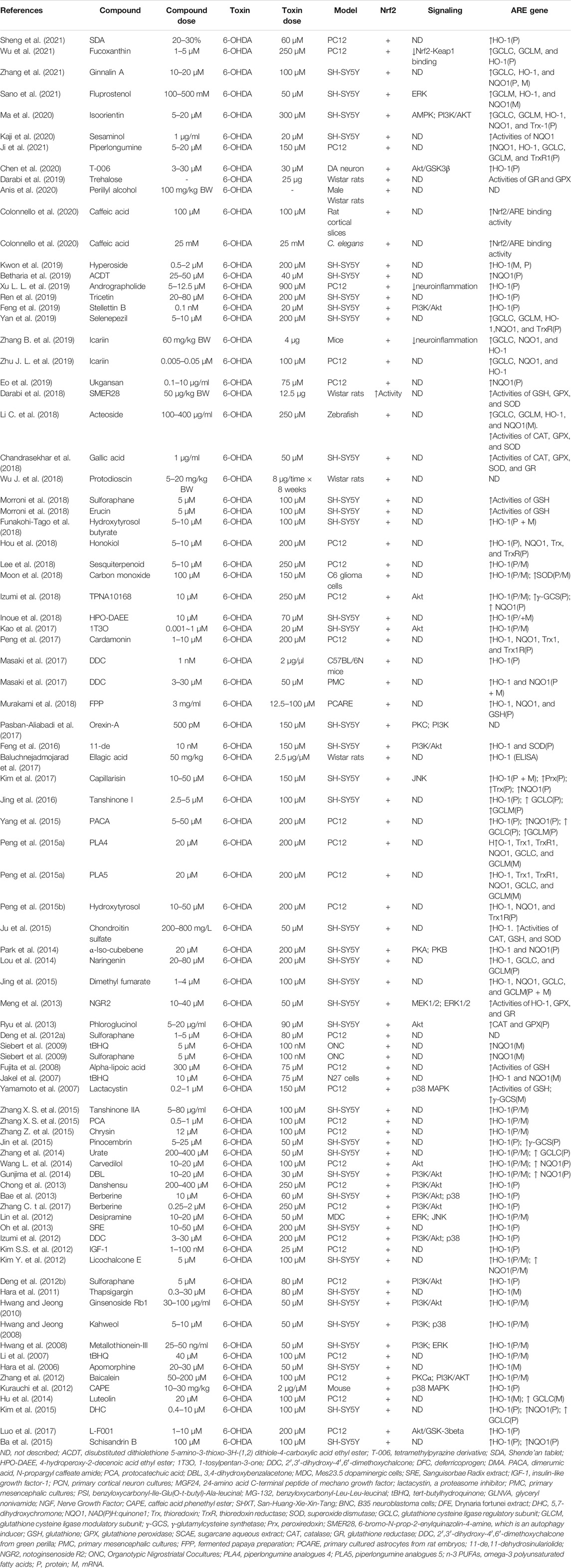
TABLE 2. Summary of the experimental studies involving compounds able to modulate the Nrf2 pathway in 6-OHDA-induced PD models.
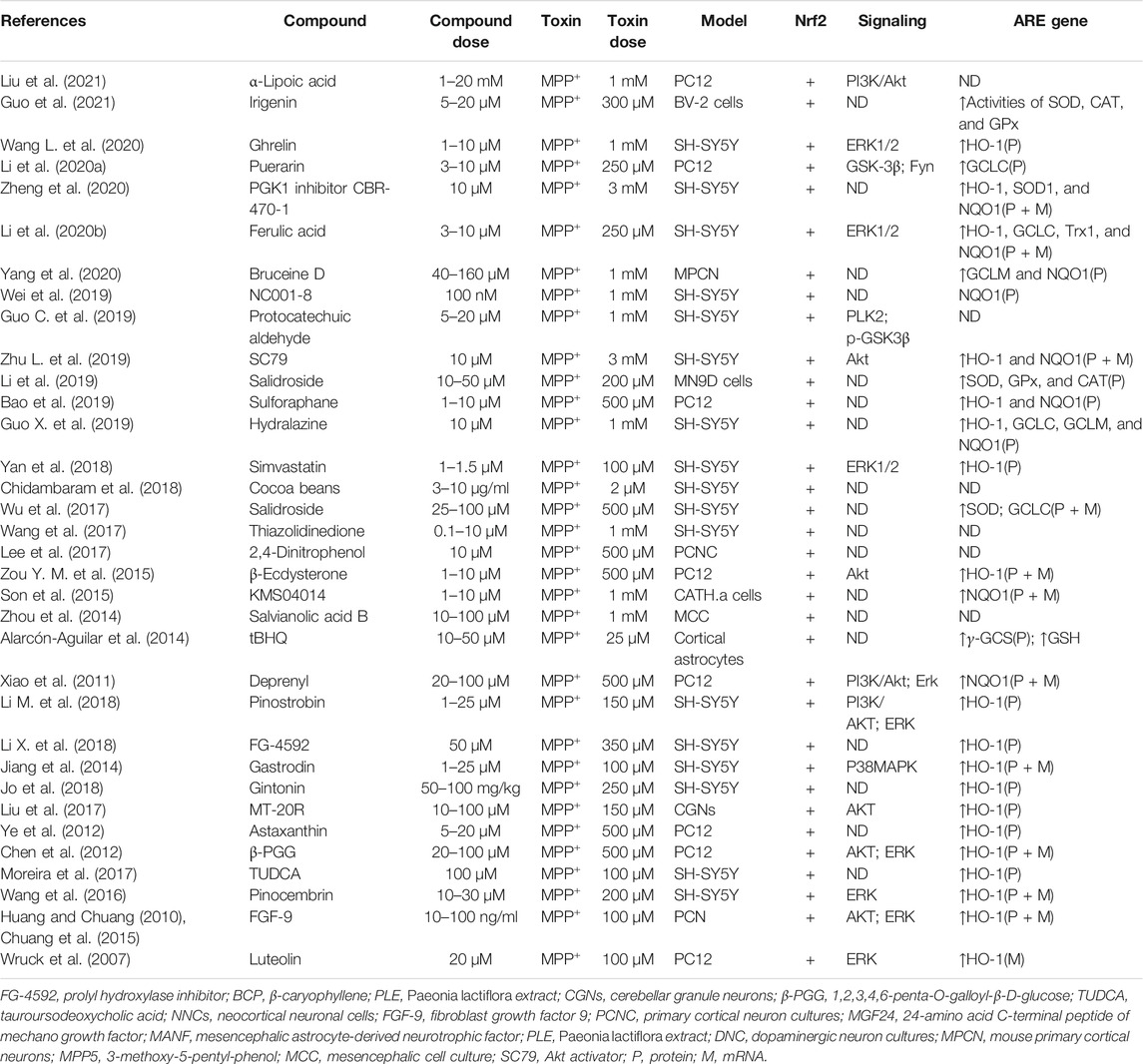
TABLE 3. Summary of the experimental studies involving compounds able to modulate Nrf2 pathway in MPP+-induced PD models.
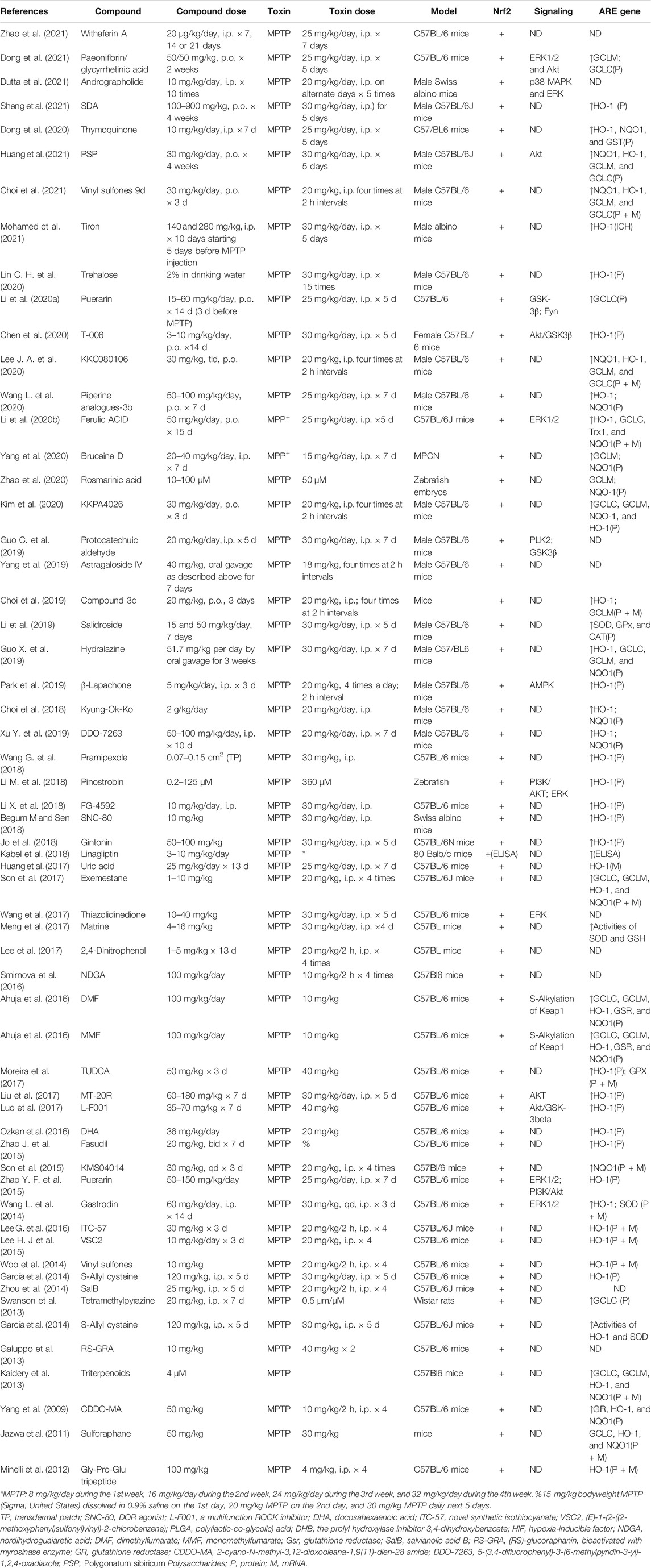
TABLE 4. Summary of the experimental studies involving compounds able to modulate Nrf2 pathway in MPTP-induced PD models.
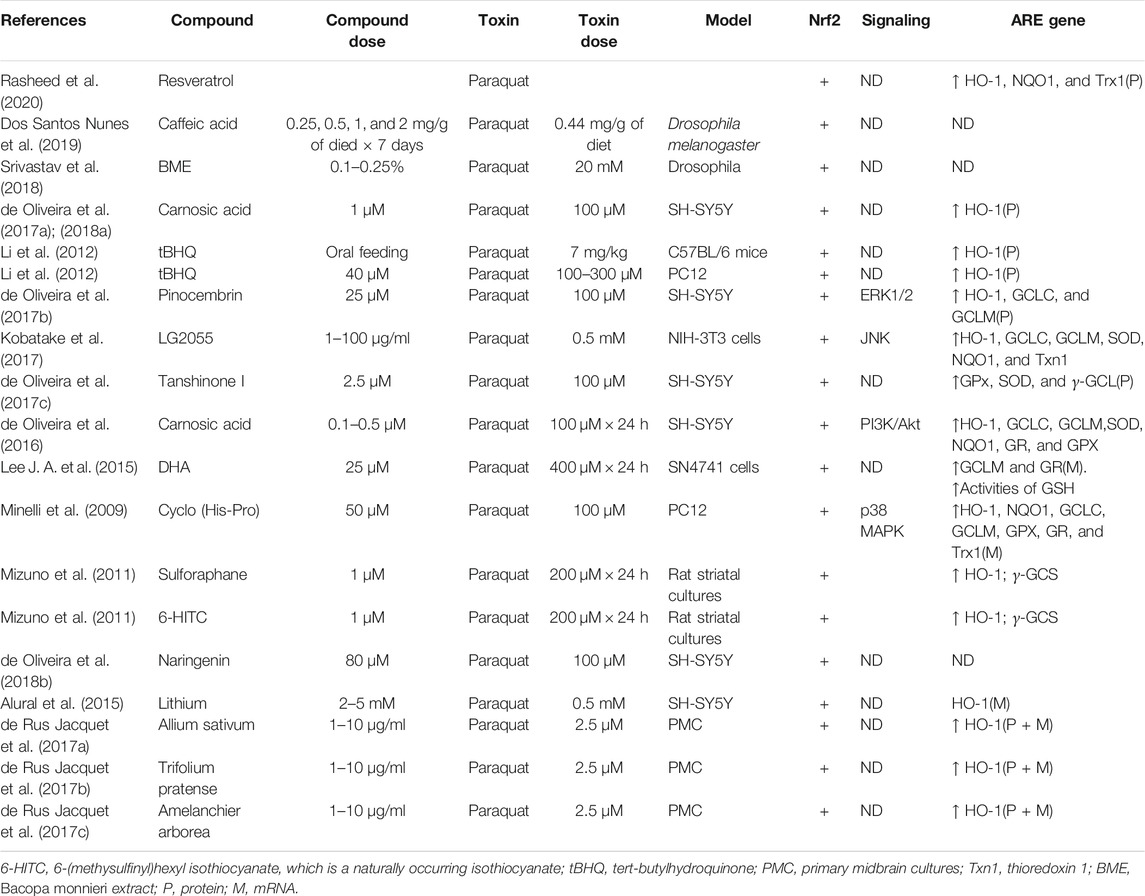
TABLE 5. Summary of the experimental studies involving compounds able to modulate Nrf2 pathway in paraquat-induced PD models.
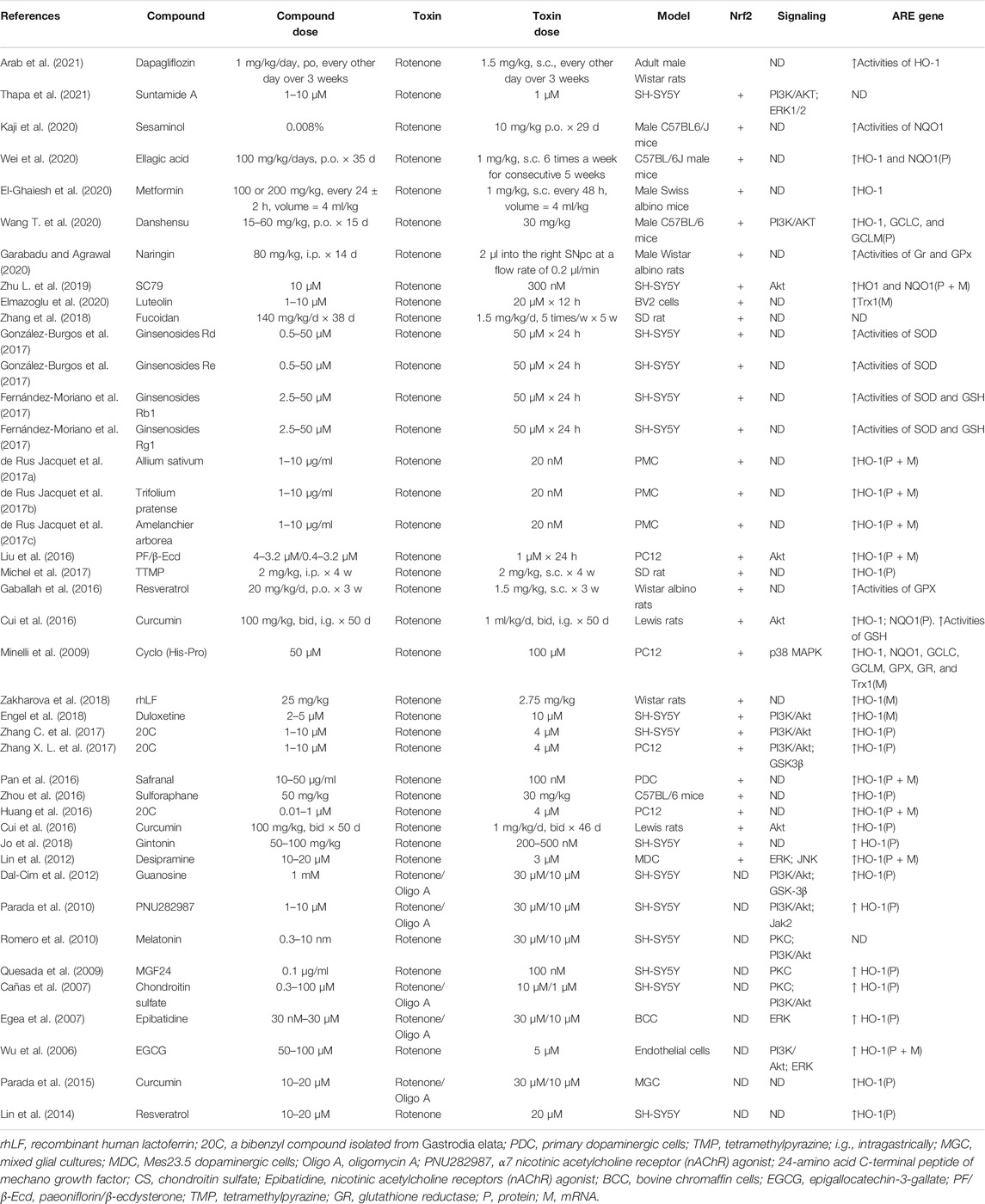
TABLE 6. Summary of the experimental studies involving compounds able to modulate Nrf2 pathway in Rotenone-induced PD models.
Neuroprotective Role of the Induction of HO-1 in PD
The list of genes regulated by Nrf2/ARE includes over 250 genes, which encode proteins and enzymes involved in antioxidant defense and detoxification (Cores et al., 2020). These genes include classical phase II detoxification enzymes like NQO1, GSTs, etc., and the enzymes involved in GSH biosynthesis, antioxidant defense (e.g., GSH-Px and HO-1), and inflammation (e.g., COX-2 and HO-1) (van Muiswinkel and Kuiperij, 2005; Tebay et al., 2015).
Heme oxygenase-1 (HO-1), a potent antioxidant enzyme regulated by Nrf2, degrades heme to carbon monoxide, free iron, and biliverdin (Consoli et al., 2021). HO-1 has been found at higher concentrations in serum in patients with PD (Sun et al., 2021). HO-1 participates in neuroprotection against OS-dependent injury and has been speculated as a new therapeutic target for PD (Jazwa and Cuadrado, 2010). Tyrrell and others first revealed the cytoprotective effect of HO-1, demonstrating that induction of HO-1 expression mediates an adaptive cytoprotective response to OS in cultured human fibroblasts (Vile et al., 1994; Reeve and Tyrrell, 1999). Particularly interesting is the role played by HO-1 in PD (Schipper et al., 2019). HO-1 induction has been seen to implicate a neuroprotective role on exposure to a variety of PD-associated neurotoxins, both in animal models and in tissue culture (Kwon et al., 2019; Inose et al., 2020). Pharmacological induction of HO-1 by administration of bioactive compounds can exert therapeutic effects against 6-OHDA (Table 7), MPP+ (Table 8), MPTP (Table 9), paraquat (Table 10), and rotenone-induced (Table 11) neurotoxicity in vitro or in vivo PD models.
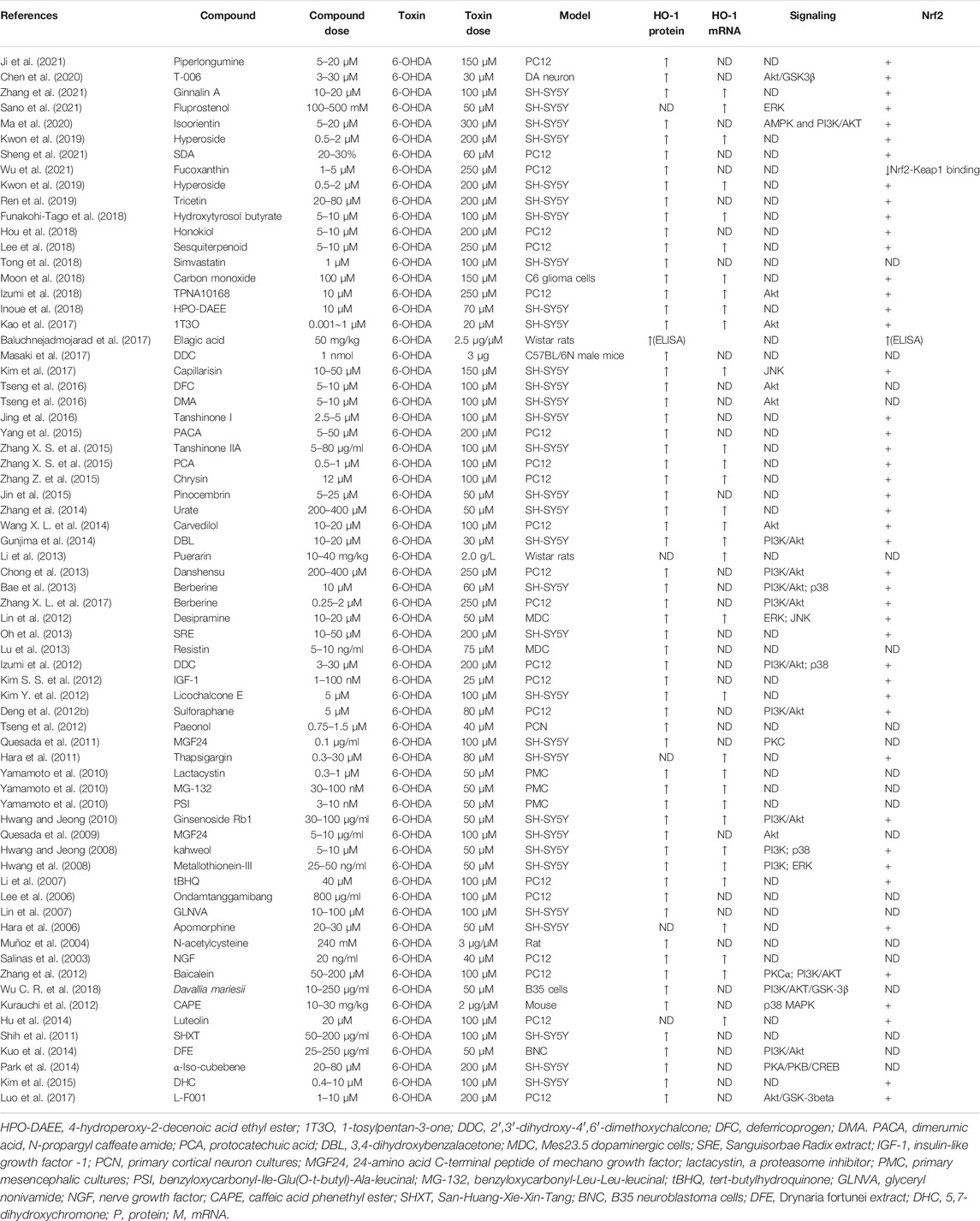
TABLE 7. Summary of the experimental studies involving HO-1 inducer against 6-OHDA-induced PD models.
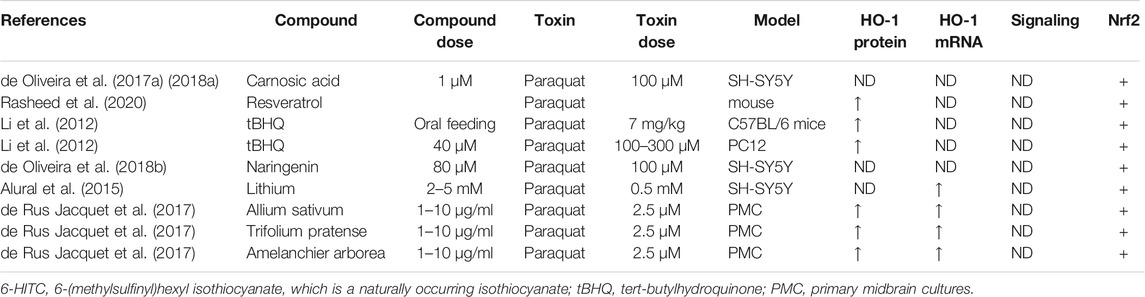
TABLE 10. Summary of the experimental studies involving HO-1 inducer against paraquat-induced PD models.
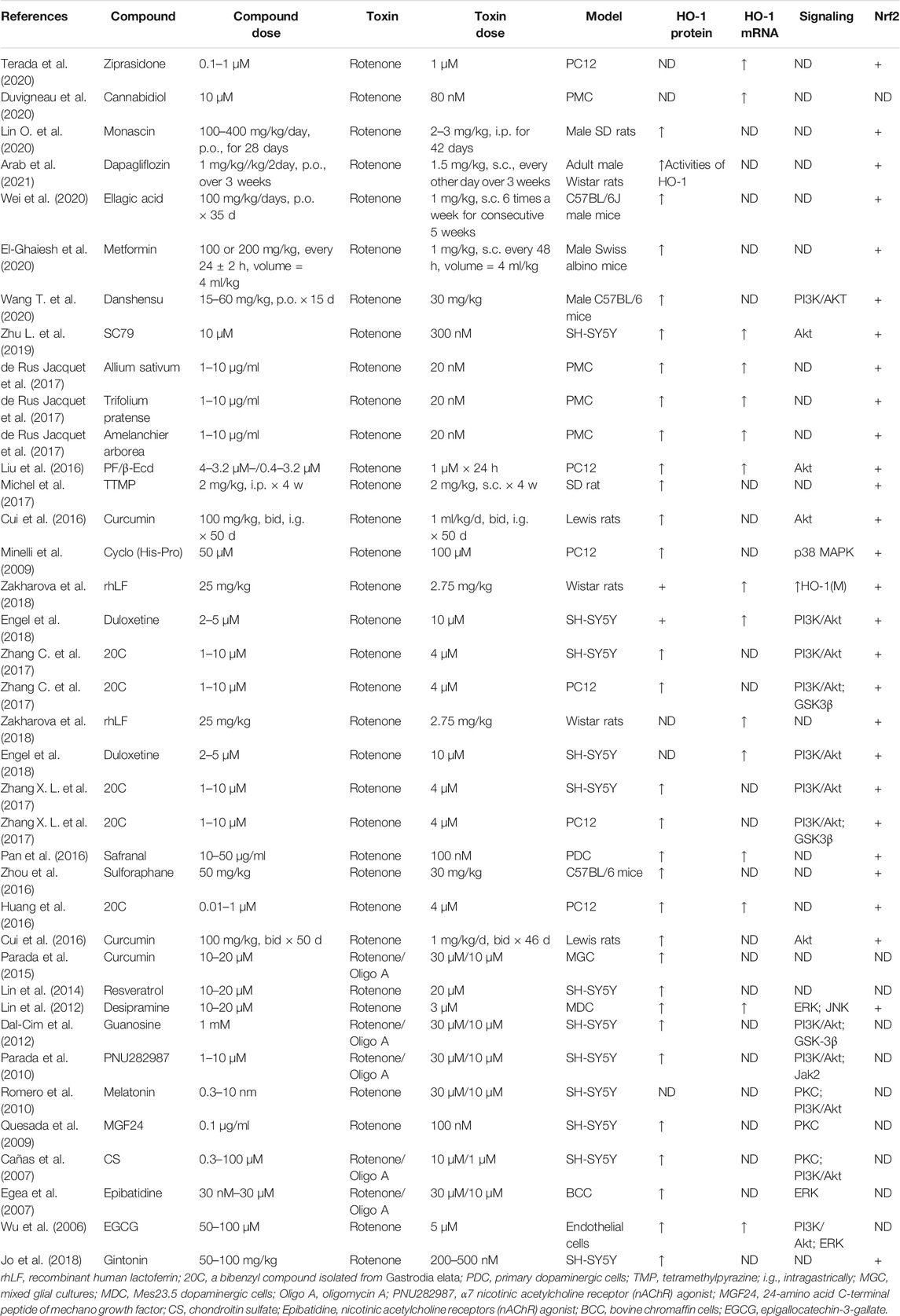
TABLE 11. Summary of the experimental studies involving HO-1 inducer against rotenone-induced PD models.
Future Perspectives
In the last decade, many research groups have developed induced pluripotent stem cell-based protocols to generate three-dimensional, multicellular, neural organoids to study the pathophysiology of PD (Lázaro et al., 2017; Rai and Singh, 2020; Costamagna et al., 2021; Outeiro et al., 2021). Organoids provide almost full features of PD pathology and physiology. The main advantage of using organoids as a PD model is that it shows very close association with in vivo conditions; thus, organoids are very easy to recapitulate all the features of PD. As compared to the conventional two-dimensional culture model, these new three-dimensional organoids provide new hope for drug screening. Recently, Outeiro and others developed microfluidic platforms to investigate specific molecular mechanisms associated with PD (Fernandes et al., 2016). Microfluidic platforms have shown PD-relevant phenotypes, including ROS production and mitochondrial dysfunction. Fernandes et al. designed a microfluidic device to understand their cell-cell and biochemical communication. The connected chambers allowed rapid diffusion of molecules from one camber to another. The device was integrated with pneumatic valves, which helped in controlling the fluid routing and cellular microenvironment and simulating the paracrine signaling. The authors studied the spreading of α-Syn and mutual communication between different cell types (neurons and glia). They observed diffusion of ROS from a chamber containing activated microglia to the other chamber that contained healthy neuroglioma cells indicating the role of ROS for neuronal functional impairment (Fernandes et al., 2016). The microfluidic device was used to study the transport of mitochondria along dopaminergic axons isolated from mice (Lu et al., 2012). A recent study used a microfluidic platform to dissect the mitochondrial dysfunctions associated with a genetic form of PD with dynamin-related GTPase optic atrophy type 1 (OPA1) mutations (Iannielli et al., 2019), revealing that axons of OPA1 mutant dopaminergic neurons exhibit a significant reduction of mitochondrial mass. This defect causes a significant loss of dopaminergic synapses, which worsens in long-term cultures. Therefore, PD-associated depletion of mitochondria at synapses might precede loss of neuronal connectivity and neurodegeneration. Seidi and others used microfluidic platforms to study the effect of 6-OHDA that induces neuronal apoptosis in PC12 cells. This represented an in vitro model of PD, which revealed that low and high concentrations of 6-ODHA decreased the viability of neuronal cells due to apoptosis and necrosis, respectively. Thus, these concentration gradient studies were considered as useful information for creating an in vitro model of PD to induce the highest level of apoptosis in cells (Seidi et al., 2011). They may provide a useful approach for generating in vitro models of disease for drug discovery applications.
Microfluidics is a rising star in the development of innovative approaches in drug discovery and screening, particularly in screening natural product drugs based on chemical properties, pharmacological effects, and drug cytotoxicity. But in the present stage, these newly developed in vitro models of PD and microfluidic platforms were not used to study the effect of the Nrf2/HO-1 activator (Lee J. A. et al., 2020). Future research is expected to elucidate the detailed molecular mechanism of Nrf2/HO-1 activator which regulates Nrf2 activation and HO-1 induction in these newly developed in vitro models of PD leading to the development of novel drugs that target Nrf2/ARE/HO-1.
Conclusion
Emerging evidence has suggested that the Nrf2/ARE pathway plays a crucial role in cellular adaption by controlling orchestrated cytoprotective proteins, including HO-1, to counteract OS in PD, thereby providing a promising optimal therapeutic target against PD (Figure 7). By using various PD-related neurotoxin-induced in vitro and in vivo models, recent preclinical studies provide direct compelling evidence that the contribution of the pharmacological modulation of the Nrf2/ARE/HO-1 pathway exerts neuroprotection in PD.
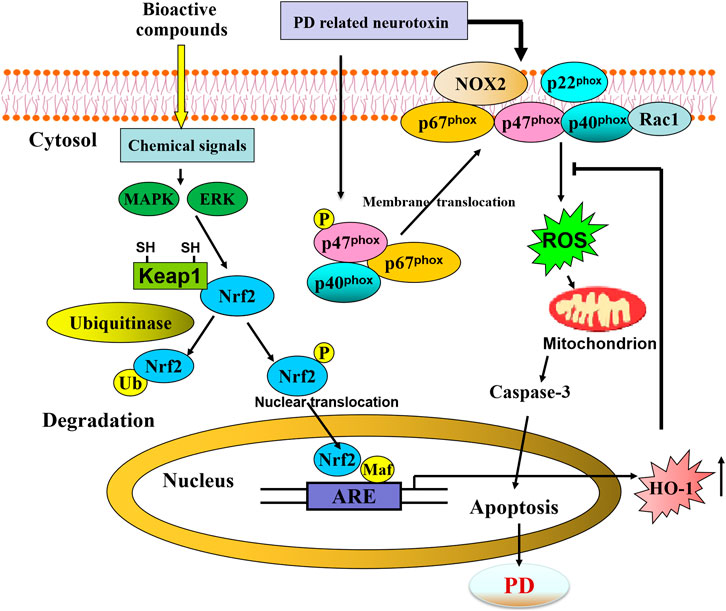
FIGURE 7. Schematic representation of bioactive compounds-mediated neuroprotective against PD through activating Nrf2/ARE/HO-1 pathway.
Author Contributions
YUW and HW: Conceptualization, Writing—original draft, Visualization. LG, JC, QL, LH, and YAW: Writing—original draft (table). YUM and HW: Conceptualization, Writing—review and, editing, Supervision. JD: review and editing, Supervision.
Funding
This work was supported in part by the National Natural Science Foundation of China (61971011, 71704053, 81260196, and 81450036), Natural Science Foundation of Inner Mongolia Autonomous Region (IMAR) (2021MS08131, 2020MS08175, and 2021LHMS08024), Science Foundation of AMHT (2020YK02), Science Foundation of CASIC (2020-LCYL-009), Science Foundation of ASCH (YN202104), Cultivation Plan of Scientific Research Committee for Health Development of Haidian District of Beijing (HP2021-19-50701), Science Foundation of Universities of IMAR (NJZY19218), and Program for Young Talents of Science and Technology in Universities of IMAR (NJYT-17-B23).
Conflict of Interest
The authors declare that the research was conducted in the absence of any commercial or financial relationships that could be construed as a potential conflict of interest.
Publisher’s Note
All claims expressed in this article are solely those of the authors and do not necessarily represent those of their affiliated organizations, or those of the publisher, the editors and the reviewers. Any product that may be evaluated in this article, or claim that may be made by its manufacturer, is not guaranteed or endorsed by the publisher.
References
Aarsland, D., Creese, B., Politis, M., Chaudhuri, K. R., Ffytche, D. H., and Weintraub, D. (2017). Cognitive Decline in Parkinson Disease. Nat. Rev. Neurol. 13, 217–231. doi:10.1038/nrneurol.2017.27
Ahmad, M. H., Fatima, M., Ali, M., Rizvi, M. A., and Chandra Mondal, A. (2021). Naringenin Alleviates Paraquat-Induced Dopaminergic Neuronal Loss in SH-Sy5y Cells and a Rat Model of Parkinson's Disease. Neuropharmacology 21, 108831. doi:10.1016/j.neuropharm.2021.108831
Ahuja, M., Ammal Kaidery, N., Yang, L., Calingasan, N., Smirnova, N., Gaisin, A., et al. (2016). Distinct Nrf2 Signaling Mechanisms of Fumaric Acid Esters and Their Role in Neuroprotection against 1-Methyl-4-Phenyl-1,2,3,6-Tetrahydropyridine-Induced Experimental Parkinson's-like Disease. J. Neurosci. 36, 6332–6351. doi:10.1523/JNEUROSCI.0426-16.2016
Alam, Z. I., Daniel, S. E., Lees, A. J., Marsden, D. C., Jenner, P., and Halliwell, B. (1997a). A Generalised Increase in Protein Carbonyls in the Brain in Parkinson's but Not Incidental Lewy Body Disease. J. Neurochem. 69, 1326–1329. doi:10.1046/j.1471-4159.1997.69031326.x
Alam, Z. I., Jenner, A., Daniel, S. E., Lees, A. J., Cairns, N., Marsden, C. D., et al. (1997b). Oxidative DNA Damage in the Parkinsonian Brain: an Apparent Selective Increase in 8-hydroxyguanine Levels in Substantia Nigra. J. Neurochem. 69, 1196–1203. doi:10.1046/j.1471-4159.1997.69031196.x
Alarcón-Aguilar, A., Luna-López, A., Ventura-Gallegos, J. L., Lazzarini, R., Galván-Arzate, S., González-Puertos, V. Y., et al. (2014). Primary Cultured Astrocytes from Old Rats Are Capable to Activate the Nrf2 Response against MPP+ Toxicity after tBHQ Pretreatment. Neurobiol. Aging 35, 1901–1912. doi:10.1016/j.neurobiolaging.2014.01.143
Alural, B., Ozerdem, A., Allmer, J., Genc, K., and Genc, S. (2015). Lithium Protects against Paraquat Neurotoxicity by NRF2 Activation and miR-34a Inhibition in SH-Sy5y Cells. Front. Cell. Neurosci. 9, 209. doi:10.3389/fncel.2015.00209
Anis, E., Zafeer, M. F., Firdaus, F., Islam, S. N., Khan, A. A., and Hossain, M. M. (2020). Perillyl Alcohol Mitigates Behavioural Changes and Limits Cell Death and Mitochondrial Changes in Unilateral 6-OHDA Lesion Model of Parkinson's Disease through Alleviation of Oxidative Stress. Neurotoxicity Res. 38, 461–477. doi:10.1007/s12640-020-00213-0
Arab, H. H., Safar, M. M., and Shahin, N. N. (2021). Targeting ROS-dependent AKT/GSK-3β/nf-Κb and DJ-1/Nrf2 Pathways by Dapagliflozin Attenuates Neuronal Injury and Motor Dysfunction in Rotenone-Induced Parkinson's Disease Rat Model. ACS Chem. Neurosci. 12, 689–703. doi:10.1021/acschemneuro.0c00722
Ba, Q., Cui, C., Wen, L., Feng, S., Zhou, J., and Yang, K. (2015). Schisandrin B Shows Neuroprotective Effect in 6-OHDA-Induced Parkinson's Disease via Inhibiting the Negative Modulation of miR-34a on Nrf2 Pathway. Biomed. Pharmacother. = Biomédecine pharmacothérapie 75, 165–172. doi:10.1016/j.biopha.2015.07.034
Babior, B. M., Curnutte, J. T., and Kipnes, B. S. (1975). Pyridine Nucleotide-dependent Superoxide Production by a Cell-free System from Human Granulocytes. J. Clin. Invest. 56, 1035–1042. doi:10.1172/JCI108150
Babior, B. M., Kipnes, R. S., and Curnutte, J. T. (1973). Biological Defense Mechanisms. The Production by Leukocytes of Superoxide, a Potential Bactericidal Agent. J. Clin. Invest. 52, 741–744. doi:10.1172/JCI107236
Bae, J., Lee, D., Kim, Y. K., Gil, M., Lee, J. Y., and Lee, K. J. (2013). Berberine Protects 6-Hydroxydopamine-Induced Human Dopaminergic Neuronal Cell Death through the Induction of Heme Oxygenase-1. Mol. Cell 35, 151–157. doi:10.1007/s10059-013-2298-5
Baluchnejadmojarad, T., Rabiee, N., Zabihnejad, S., and Roghani, M. (2017). Ellagic Acid Exerts Protective Effect in Intrastriatal 6-hydroxydopamine Rat Model of Parkinson's Disease: Possible Involvement of ERβ/Nrf2/HO-1 Signaling. Brain Res. 1662, 23–30. doi:10.1016/j.brainres.2017.02.021
Bao, B., Zhang, M. Q., Chen, Z. Y., Wu, X. B., Xia, Z. B., Chai, J. Y., et al. (2019). Sulforaphane Prevents PC12 Cells from Oxidative Damage via the Nrf2 Pathway. Mol. Med. Rep. 19, 4890–4896. doi:10.3892/mmr.2019.10148
Bedard, K., and Krause, K. H. (2007). The NOX Family of ROS-Generating NADPH Oxidases: Physiology and Pathophysiology. Physiol. Rev. 87, 245–313. doi:10.1152/physrev.00044.2005
Begum M, E. T., and Sen, D. (2018). DOR Agonist (SNC-80) Exhibits Anti-parkinsonian Effect via Downregulating UPR/oxidative Stress Signals and Inflammatory Response In Vivo. Neurosci. Lett. 678, 29–36. doi:10.1016/j.neulet.2018.04.055
Belaidi, A. A., and Bush, A. I. (2016). Iron Neurochemistry in Alzheimer's Disease and Parkinson's Disease: Targets for Therapeutics. J. Neurochem. 139 (Suppl. 1), 179–197. doi:10.1111/jnc.13425
Bento-Pereira, C., and Dinkova-Kostova, A. T. (2021). Activation of Transcription Factor Nrf2 to Counteract Mitochondrial Dysfunction in Parkinson's Disease. Med. Res. Rev. 41, 785–802. doi:10.1002/med.21714
Berendes, H., Bridges, R. A., and Good, R. A. (1957). A Fatal Granulomatosus of Childhood: the Clinical Study of a New Syndrome. Minn. Med. 40, 309–312.
Betharia, S., Rondόn-Ortiz, A. N., and Brown, D. A. (2019). Disubstituted Dithiolethione ACDT Exerts Neuroprotective Effects against 6-Hydroxydopamine-Induced Oxidative Stress in SH-Sy5y Cells. Neurochem. Res. 44, 1878–1892. doi:10.1007/s11064-019-02823-3
Blesa, J., Trigo-Damas, I., Quiroga-Varela, A., and Jackson-Lewis, V. R. (2015). Oxidative Stress and Parkinson's Disease. Front. Neuroanat. 9, 91. doi:10.3389/fnana.2015.00091
Blum, D., Torch, S., Lambeng, N., Nissou, M., Benabid, A. L., Sadoul, R., et al. (2001). Molecular Pathways Involved in the Neurotoxicity of 6-OHDA, Dopamine and MPTP: Contribution to the Apoptotic Theory in Parkinson's Disease. Prog. Neurobiol. 65, 135–172. doi:10.1016/s0301-0082(01)00003-x
Bonifati, V., Rizzu, P., van Baren, M. J., Schaap, O., Breedveld, G. J., Krieger, E., et al. (2003). Mutations in the DJ-1 Gene Associated with Autosomal Recessive Early-Onset Parkinsonism. Science 299, 256–259. doi:10.1126/science.1077209
Boveris, A., and Chance, B. (1973). The Mitochondrial Generation of Hydrogen Peroxide. General Properties and Effect of Hyperbaric Oxygen. Biochem. J. 134, 707–716. doi:10.1042/bj1340707
Brás, I. C., and Outeiro, T. F. (2021). Alpha-Synuclein: Mechanisms of Release and Pathology Progression in Synucleinopathies. Cells 10, 375. doi:10.3390/cells10020375
Bryan, H. K., Olayanju, A., Goldring, C. E., and Park, B. K. (2013). The Nrf2 Cell Defence Pathway: Keap1-dependent and -independent Mechanisms of Regulation. Biochem. Pharmacol. 85, 705–717. doi:10.1016/j.bcp.2012.11.016
Buendia, I., Michalska, P., Navarro, E., Gameiro, I., Egea, J., and León, R. (2016). Nrf2-ARE Pathway: An Emerging Target against Oxidative Stress and Neuroinflammation in Neurodegenerative Diseases. Pharmacol. Ther. 157, 84–104. doi:10.1016/j.pharmthera.2015.11.003
Calkins, M. J., Jakel, R. J., Johnson, D. A., Chan, K., Kan, Y. W., and Johnson, J. A. (2005). Protection from Mitochondrial Complex II Inhibition In Vitro and In Vivo by Nrf2-Mediated Transcription. Proc. Natl. Acad. Sci. United States America 102, 244–249. doi:10.1073/pnas.0408487101
Calkins, M. J., Johnson, D. A., Townsend, J. A., Vargas, M. R., Dowell, J. A., Williamson, T. P., et al. (2009). The Nrf2/ARE Pathway as a Potential Therapeutic Target in Neurodegenerative Disease. Antioxid. Redox signaling 11, 497–508. doi:10.1089/ars.2008.2242
Cañas, N., Valero, T., Villarroya, M., Montell, E., Vergés, J., García, A. G., et al. (2007). Chondroitin Sulfate Protects SH-Sy5y Cells from Oxidative Stress by Inducing Heme Oxygenase-1 via Phosphatidylinositol 3-kinase/Akt. J. Pharmacol. Exp. Ther. 323, 946–953. doi:10.1124/jpet.107.123505
Chandrasekhar, Y., Phani Kumar, G., Ramya, E. M., and Anilakumar, K. R. (2018). Gallic Acid Protects 6-OHDA Induced Neurotoxicity by Attenuating Oxidative Stress in Human Dopaminergic Cell Line. Neurochem. Res. 43, 1150–1160. doi:10.1007/s11064-018-2530-y
Chaturvedi, R. K., and Beal, M. F. (2008). Mitochondrial Approaches for Neuroprotection. Ann. N.Y Acad. Sci. 1147, 395–412. doi:10.1196/annals.1427.027
Chen, H., Cao, J., Zha, L., Wang, P., Liu, Z., Guo, B., et al. (2020). Neuroprotective and Neurogenic Effects of Novel Tetramethylpyrazine Derivative T-006 in Parkinson's Disease Models through Activating the MEF2-Pgc1α and BDNF/CREB Pathways. Aging 12, 14897–14917. doi:10.18632/aging.103551
Chen, H., Li, H., Cao, F., Zhen, L., Bai, J., Yuan, S., et al. (2012). 1,2,3,4,6-penta-O-galloyl-β-D-glucose Protects PC12 Cells from MPP(+)-mediated Cell Death by Inducing Heme Oxygenase-1 in an ERK- and Akt-dependent Manner. J. Huazhong Univ. Sci. Technolog Med. Sci. 32, 737–745. doi:10.1007/s11596-012-1027-1
Chen, L. L., Huang, Y. J., Cui, J. T., Song, N., and Xie, J. (2019). Iron Dysregulation in Parkinson's Disease: Focused on the Autophagy-Lysosome Pathway. ACS Chem. Neurosci. 10, 863–871. doi:10.1021/acschemneuro.8b00390
Chen, P. C., Vargas, M. R., Pani, A. K., Smeyne, R. J., Johnson, D. A., Kan, Y. W., et al. (2009). Nrf2-mediated Neuroprotection in the MPTP Mouse Model of Parkinson's Disease: Critical Role for the Astrocyte. Proc. Natl. Acad. Sci. United States America 106, 2933–2938. doi:10.1073/pnas.0813361106
Cheng, B., Guo, Y., Li, C., Ji, B., Pan, Y., Chen, J., et al. (2014). Edaravone Protected PC12 Cells against MPP(+)-cytoxicity via Inhibiting Oxidative Stress and Up-Regulating Heme Oxygenase-1 Expression. J. Neurol. Sci. 343, 115–119. doi:10.1016/j.jns.2014.05.051
Chidambaram, S. B., Bhat, A., Ray, B., Sugumar, M., Muthukumar, S. P., Manivasagam, T., et al. (2018). Cocoa Beans Improve Mitochondrial Biogenesis via PPARγ/PGC1α Dependent Signalling Pathway in MPP+ Intoxicated Human Neuroblastoma Cells (SH-Sy5y). Nutr. Neurosci. 23, 471. doi:10.1080/1028415X.2018.1521088
Chinta, S. J., and Andersen, J. K. (2008). Redox Imbalance in Parkinson's Disease. Biochim. Biophys. Acta 1780, 1362–1367. doi:10.1016/j.bbagen.2008.02.005
Chinta, S. J., Rajagopalan, S., Ganesan, A., and Andersen, J. K. (2012). A Possible Novel Anti-inflammatory Mechanism for the Pharmacological Prolyl Hydroxylase Inhibitor 3,4-dihydroxybenzoate: Implications for Use as a Therapeutic for Parkinson's Disease. Parkinson's Dis. 2012, 364684. doi:10.1155/2012/364684
Choi, J. H., Jang, M., Lee, J. I., Chung, W. S., and Cho, I. H. (2018). Neuroprotective Effects of a Traditional Multi-Herbal Medicine Kyung-Ok-Ko in an Animal Model of Parkinson's Disease: Inhibition of MAPKs and NF-Κb Pathways and Activation of Keap1-Nrf2 Pathway. Front. Pharmacol. 9, 1444. doi:10.3389/fphar.2018.01444
Choi, J. W., Kim, S., Yoo, J. S., Kim, H. J., Kim, H. J., Kim, B. E., et al. (2021). Development and Optimization of Halogenated Vinyl Sulfones as Nrf2 Activators for the Treatment of Parkinson's Disease. Eur. J. Med. Chem. 212, 113103. doi:10.1016/j.ejmech.2020.113103
Choi, J. W., Shin, S. J., Kim, H. J., Park, J. H., Kim, H. J., Lee, E. H., et al. (2019). Antioxidant, Anti-inflammatory, and Neuroprotective Effects of Novel Vinyl Sulfonate Compounds as Nrf2 Activator. ACS Med. Chem. Lett. 10, 1061–1067. doi:10.1021/acsmedchemlett.9b00163
Chong, C. M., Zhou, Z. Y., Razmovski-Naumovski, V., Cui, G. Z., Zhang, L. Q., Sa, F., et al. (2013). Danshensu Protects against 6-Hydroxydopamine-Induced Damage of PC12 Cells In Vitro and Dopaminergic Neurons in Zebrafish. Neurosci. Lett. 543, 121–125. doi:10.1016/j.neulet.2013.02.069
Chuang, J. I., Huang, J. Y., Tsai, S. J., Sun, H. S., Yang, S. H., Chuang, P. C., et al. (2015). FGF9-induced Changes in Cellular Redox Status and HO-1 Upregulation Are FGFR-dependent and Proceed through Both ERK and AKT to Induce CREB and Nrf2 Activation. Free Radic. Biol. Med. 89, 274–286. doi:10.1016/j.freeradbiomed.2015.08.011
Colonnello, A., Aguilera-Portillo, G., Rubio-López, L. C., Robles-Bañuelos, B., Rangel-López, E., Cortez-Núñez, S., et al. (2020). Comparing the Neuroprotective Effects of Caffeic Acid in Rat Cortical Slices and Caenorhabditis elegans: Involvement of Nrf2 and SKN-1 Signaling Pathways. Neurotoxicity Res. 37, 326–337. doi:10.1007/s12640-019-00133-8
Consoli, V., Sorrenti, V., Grosso, S., and Vanella, L. (2021). Heme Oxygenase-1 Signaling and Redox Homeostasis in Physiopathological Conditions. Biomolecules 11, 589. doi:10.3390/biom11040589
Cores, Á., Piquero, M., Villacampa, M., León, R., and Menéndez, J. C. (2020). NRF2 Regulation Processes as a Source of Potential Drug Targets against Neurodegenerative Diseases. Biomolecules 10, 904. doi:10.3390/biom10060904
Costamagna, G., Comi, G. P., and Corti, S. (2021). Advancing Drug Discovery for Neurological Disorders Using iPSC-Derived Neural Organoids. Int. J. Mol. Sci. 22, 2659. doi:10.3390/ijms22052659
Cuadrado, A., Manda, G., Hassan, A., Alcaraz, M. J., Barbas, C., Daiber, A., et al. (2018). Transcription Factor NRF2 as a Therapeutic Target for Chronic Diseases: A Systems Medicine Approach. Pharmacol. Rev. 70, 348–383. doi:10.1124/pr.117.014753
Cuadrado, A., Rojo, A. I., Wells, G., Hayes, J. D., Cousin, S. P., Rumsey, W. L., et al. (2019). Therapeutic Targeting of the NRF2 and KEAP1 Partnership in Chronic Diseases. Nat. Rev. Drug Discov. 18, 295–317. doi:10.1038/s41573-018-0008-x
Cuenca, L., Gil-Martinez, A. L., Cano-Fernandez, L., Sanchez-Rodrigo, C., Estrada, C., Fernandez-Villalba, E., et al. (2018). Parkinson's Disease: a Short story of 200 Years. Histology and histopathology 34, 573. doi:10.14670/HH-18-073
Cui, Q., Li, X., and Zhu, H. (2016). Curcumin Ameliorates Dopaminergic Neuronal Oxidative Damage via Activation of the Akt/Nrf2 Pathway. Mol. Med. Rep. 13, 1381–1388. doi:10.3892/mmr.2015.4657
D'Autréaux, B., and Toledano, M. B. (2007). ROS as Signalling Molecules: Mechanisms that Generate Specificity in ROS Homeostasis. Nat. Rev. Mol. Cel. Biol. 8, 813–824. doi:10.1038/nrm2256
Dal-Cim, T., Molz, S., Egea, J., Parada, E., Romero, A., Budni, J., et al. (2012). Guanosine Protects Human Neuroblastoma SH-Sy5y Cells against Mitochondrial Oxidative Stress by Inducing Heme Oxigenase-1 via PI3K/Akt/GSK-3β Pathway. Neurochem. Int. 61, 397–404. doi:10.1016/j.neuint.2012.05.021
Danielson, S. R., and Andersen, J. K. (2008). Oxidative and Nitrative Protein Modifications in Parkinson's Disease. Free Radic. Biol. Med. 44, 1787–1794. doi:10.1016/j.freeradbiomed.2008.03.005
Darabi, S., Noori-Zadeh, A., Abbaszadeh, H. A., Rajaei, F., and Bakhtiyari, S. (2019). Trehalose Neuroprotective Effects on the Substantia Nigra Dopaminergic Cells by Activating Autophagy and Non-canonical Nrf2 Pathways. Iranian J. Pharm. Res. 18, 1419–1428. doi:10.22037/ijpr.2019.2387
Darabi, S., Noori-Zadeh, A., Rajaei, F., Abbaszadeh, H. A., Bakhtiyari, S., and Roozbahany, N. A. (2018). SMER28 Attenuates Dopaminergic Toxicity Mediated by 6-Hydroxydopamine in the Rats via Modulating Oxidative Burdens and Autophagy-Related Parameters. Neurochem. Res. 43, 2313–2323. doi:10.1007/s11064-018-2652-2
de Oliveira, M. R., Andrade, C., and Fürstenau, C. R. (2018a). Naringenin Exerts Anti-inflammatory Effects in Paraquat-Treated SH-Sy5y Cells through a Mechanism Associated with the Nrf2/HO-1 Axis. Neurochem. Res. 43, 894–903. doi:10.1007/s11064-018-2495-x
de Oliveira, M. R., de Souza, I., and Fürstenau, C. R. (2018b). Carnosic Acid Induces Anti-inflammatory Effects in Paraquat-Treated SH-Sy5y Cells through a Mechanism Involving a Crosstalk between the Nrf2/HO-1 Axis and NF-Κb. Mol. Neurobiol. 55, 890–897. doi:10.1007/s12035-017-0389-6
de Oliveira, M. R., Ferreira, G. C., and Schuck, P. F. (2016). Protective Effect of Carnosic Acid against Paraquat-Induced Redox Impairment and Mitochondrial Dysfunction in SH-Sy5y Cells: Role for PI3K/Akt/Nrf2 Pathway. Toxicol. vitro 32, 41–54. doi:10.1016/j.tiv.2015.12.005
de Oliveira, M. R., Peres, A., Ferreira, G. C., Schuck, P. F., Gama, C. S., and Bosco, S. (2017a). Carnosic Acid Protects Mitochondria of Human Neuroblastoma SH-Sy5y Cells Exposed to Paraquat through Activation of the Nrf2/HO-1Axis. Mol. Neurobiol. 54, 5961–5972. doi:10.1007/s12035-016-0100-3
de Oliveira, M. R., Peres, A., Gama, C. S., and Bosco, S. (2017b). Pinocembrin Provides Mitochondrial Protection by the Activation of the Erk1/2-Nrf2 Signaling Pathway in SH-Sy5y Neuroblastoma Cells Exposed to Paraquat. Mol. Neurobiol. 54, 6018–6031. doi:10.1007/s12035-016-0135-5
de Oliveira, M. R., Schuck, P. F., and Bosco, S. (2017c). Tanshinone I Induces Mitochondrial Protection through an Nrf2-dependent Mechanism in Paraquat-TreatedHuman Neuroblastoma SH-Sy5y Cells. Mol. Neurobiol. 54, 4597–4608. doi:10.1007/s12035-016-0009-x
de Rus Jacquet, A., Tambe, M. A., Ma, S. Y., McCabe, G. P., Vest, J., and Rochet, J. C. (2017). Pikuni-Blackfeet Traditional Medicine: Neuroprotective Activities of Medicinal Plants Used to Treat Parkinson's Disease-Related Symptoms. J. ethnopharmacology 206, 393–407. doi:10.1016/j.jep.2017.01.001
Deng, C., Tao, R., Yu, S. Z., and Jin, H. (2012a). Inhibition of 6-Hydroxydopamine-Induced Endoplasmic Reticulum Stress by Sulforaphane through the Activation of Nrf2 Nuclear Translocation. Mol. Med. Rep. 6, 215–219. doi:10.3892/mmr.2012.894
Deng, C., Tao, R., Yu, S. Z., and Jin, H. (2012b). Sulforaphane Protects against 6-Hydroxydopamine-Induced Cytotoxicity by Increasing Expression of Heme Oxygenase-1 in a PI3K/Akt-dependent Manner. Mol. Med. Rep. 5, 847–851. doi:10.3892/mmr.2011.731
Deng, H., Wang, P., and Jankovic, J. (2018). The Genetics of Parkinson Disease. Ageing Res. Rev. 42, 72–85. doi:10.1016/j.arr.2017.12.007
Deshmukh, P., Unni, S., Krishnappa, G., and Padmanabhan, B. (2017). The Keap1-Nrf2 Pathway: Promising Therapeutic Target to Counteract ROS-Mediated Damage in Cancers and Neurodegenerative Diseases. Biophysical Rev. 9, 41–56. doi:10.1007/s12551-016-0244-4
Dexter, D. T., Carter, C. J., Wells, F. R., Javoy-Agid, F., Agid, Y., Lees, A., et al. (1989). Basal Lipid Peroxidation in Substantia Nigra Is Increased in Parkinson's Disease. J. Neurochem. 52, 381–389. doi:10.1111/j.1471-4159.1989.tb09133.x
Di Fonzo, A., Rohé, C. F., Ferreira, J., Chien, H. F., Vacca, L., Stocchi, F., et al. (2005). A Frequent LRRK2 Gene Mutation Associated with Autosomal Dominant Parkinson's Disease. Lancet 365, 412–415. doi:10.1016/S0140-6736(05)17829-5
Dias, V., Junn, E., and Mouradian, M. M. (2013). The Role of Oxidative Stress in Parkinson's Disease. J. Parkinson's Dis. 3, 461–491. doi:10.3233/JPD-130230
Dinkova-Kostova, A. T., and Talalay, P. (2008). Direct and Indirect Antioxidant Properties of Inducers of Cytoprotective Proteins. Mol. Nutr. Food Res. 52 (Suppl. 1), S128–S138. doi:10.1002/mnfr.200700195
Domingo, A., and Klein, C. (2018). Genetics of Parkinson Disease. Handbook Clin. Neurol. 147, 211–227. doi:10.1016/B978-0-444-63233-3.00014-2
Dong, H., Zhang, J., Rong, H., Zhang, X., and Dong, M. (2021). Paeoniflorin and Plycyrrhetinic Acid Synergistically Alleviate MPP(+)/MPTP-Induced Oxidative Stress through Nrf2-dependent Glutathione Biosynthesis Mechanisms. ACS Chem. Neurosci. 12, 1100–1111. doi:10.1021/acschemneuro.0c00544
Dong, J., Zhang, X., Wang, S., Xu, C., Gao, M., Liu, S., et al. (2020). Thymoquinone Prevents Dopaminergic Neurodegeneration by Attenuating Oxidative Stress via the Nrf2/ARE Pathway. Front. Pharmacol. 11, 615598. doi:10.3389/fphar.2020.615598
Dos Santos Nunes, R. G., Pereira, P. S., Elekofehinti, O. O., Fidelis, K. R., da Silva, C. S., Ibrahim, M., et al. (2019). Possible Involvement of Transcriptional Activation of Nuclear Factor Erythroid 2-related Factor 2 (Nrf2) in the Protective Effect of Caffeic Acid on Paraquat-Induced Oxidative Damage in Drosophila melanogaster. Pestic. Biochem. Physiol. 157, 161–168. doi:10.1016/j.pestbp.2019.03.017
Dutta, N., Ghosh, S., Nelson, V. K., Sareng, H. R., Majumder, C., Mandal, S. C., et al. (2021). Andrographolide Upregulates Protein Quality Control Mechanisms in Cell and Mouse through Upregulation of mTORC1 Function. Biochim. Biophys. Acta Gen. subjects 1865, 129885. doi:10.1016/j.bbagen.2021.129885
Duvigneau, J. C., Trovato, A., Müllebner, A., Miller, I., Krewenka, C., Krenn, K., et al. (2020). Cannabidiol Protects Dopaminergic Neurons in Mesencephalic Cultures against the Complex I Inhibitor Rotenone via Modulation of Heme Oxygenase Activity and Bilirubin. Antioxidants 9. doi:10.3390/antiox9020135
Egea, J., Rosa, A. O., Cuadrado, A., García, A. G., and López, M. G. (2007). Nicotinic Receptor Activation by Epibatidine Induces Heme Oxygenase-1 and Protects Chromaffin Cells against Oxidative Stress. J. Neurochem. 102, 1842–1852. doi:10.1111/j.1471-4159.2007.04665.x
El-Ghaiesh, S. H., Bahr, H. I., Ibrahiem, A. T., Ghorab, D., Alomar, S. Y., Farag, N. E., et al. (2020). Metformin Protects from Rotenone-Induced Nigrostriatal Neuronal Death in Adult Mice by Activating AMPK-FOXO3 Signaling and Mitigation of Angiogenesis. Front. Mol. Neurosci. 13, 84. doi:10.3389/fnmol.2020.00084
Elfawy, H. A., and Das, B. (2019). Crosstalk between Mitochondrial Dysfunction, Oxidative Stress, and Age Related Neurodegenerative Disease: Etiologies and Therapeutic Strategies. Life Sci. 218, 165–184. doi:10.1016/j.lfs.2018.12.029
Elmazoglu, Z., Yar Saglam, A. S., Sonmez, C., and Karasu, C. (2020). Luteolin Protects Microglia against Rotenone-Induced Toxicity in a Hormetic Manner through Targeting Oxidative Stress Response, Genes Associated with Parkinson's Disease and Inflammatory Pathways. Drug Chem. Toxicol. 43, 96–103. doi:10.1080/01480545.2018.1504961
Engel, D. F., de Oliveira, J., Lieberknecht, V., Rodrigues, A., de Bem, A. F., and Gabilan, N. H. (2018). Duloxetine Protects Human Neuroblastoma Cells from Oxidative Stress-Induced Cell Death through Akt/Nrf-2/HO-1 Pathway. Neurochem. Res. 43, 387–396. doi:10.1007/s11064-017-2433-3
Eo, H., Huh, E., Sim, Y., and Oh, M. S. (2019). Ukgansan Protects Dopaminergic Neurons from 6-hydroxydopamine Neurotoxicity via Activation of the Nuclear Factor (Erythroid-derived 2)-like 2 Factor Signaling Pathway. Neurochem. Int. 122, 208–215. doi:10.1016/j.neuint.2018.11.021
Fahn, S., and Cohen, G. (1992). The Oxidant Stress Hypothesis in Parkinson's Disease: Evidence Supporting it. Ann. Neurol. 32, 804–812. doi:10.1002/ana.410320616
Farrer, M., Gwinn-Hardy, K., Muenter, M., DeVrieze, F. W., Crook, R., Perez-Tur, J., et al. (1999). A Chromosome 4p Haplotype Segregating with Parkinson's Disease and Postural Tremor. Hum. Mol. Genet. 8, 81–85. doi:10.1093/hmg/8.1.81
Feng, C. W., Chen, N. F., Wen, Z. H., Yang, W. Y., Kuo, H. M., Sung, P. J., et al. (2019). In Vitro and In Vivo Neuroprotective Effects of Stellettin B through Anti-apoptosis and the Nrf2/HO-1 Pathway. Mar. Drugs 17. doi:10.3390/md17060315
Feng, C. W., Hung, H. C., Huang, S. Y., Chen, C. H., Chen, Y. R., Chen, C. Y., et al. (2016). Neuroprotective Effect of the Marine-Derived Compound 11-Dehydrosinulariolide through DJ-1-Related Pathway in In Vitro and In Vivo Models of Parkinson's Disease. Mar. Drugs 14, 187. doi:10.3390/md14100187
Fernandes, J. T., Chutna, O., Chu, V., Conde, J. P., and Outeiro, T. F. (2016). A Novel Microfluidic Cell Co-culture Platform for the Study of the Molecular Mechanisms of Parkinson's Disease and Other Synucleinopathies. Front. Neurosci. 10, 511. doi:10.3389/fnins.2016.00511
Fernández-Moriano, C., González-Burgos, E., Iglesias, I., Lozano, R., and Gómez-Serranillos, M. P. (2017). Evaluation of the Adaptogenic Potential Exerted by Ginsenosides Rb1 and Rg1 against Oxidative Stress-Mediated Neurotoxicity in an In Vitro Neuronal Model. PloS one 12, e0182933. doi:10.1371/journal.pone.0182933
Figueira, T. R., Barros, M. H., Camargo, A. A., Castilho, R. F., Ferreira, J. C., Kowaltowski, A. J., et al. (2013). Mitochondria as a Source of Reactive Oxygen and Nitrogen Species: from Molecular Mechanisms to Human Health. Antioxid. Redox signaling 18, 2029–2074. doi:10.1089/ars.2012.4729
Fujita, H., Shiosaka, M., Ogino, T., Okimura, Y., Utsumi, T., Sato, E. F., et al. (2008). Alpha-lipoic Acid Suppresses 6-Hydroxydopamine-Induced ROS Generation and Apoptosis through the Stimulation of Glutathione Synthesis but Not by the Expression of Heme Oxygenase-1. Brain Res. 1206, 1–12. doi:10.1016/j.brainres.2008.01.081
Funakohi-Tago, M., Sakata, T., Fujiwara, S., Sakakura, A., Sugai, T., Tago, K., et al. (2018). Hydroxytyrosol Butyrate Inhibits 6-OHDA-Induced Apoptosis through Activation of the Nrf2/HO-1 axis in SH-Sy5y Cells. Eur. J. Pharmacol. 834, 246–256. doi:10.1016/j.ejphar.2018.07.043
Gaballah, H. H., Zakaria, S. S., Elbatsh, M. M., and Tahoon, N. M. (2016). Modulatory Effects of Resveratrol on Endoplasmic Reticulum Stress-Associated Apoptosis and Oxido-Inflammatory Markers in a Rat Model of Rotenone-Induced Parkinson's Disease. Chemico-biological interactions 251, 10–16. doi:10.1016/j.cbi.2016.03.023
Galuppo, M., Iori, R., De Nicola, G. R., Bramanti, P., and Mazzon, E. (2013). Anti-inflammatory and Anti-apoptotic Effects of (RS)-Glucoraphanin Bioactivated with Myrosinase in Murine Sub-acute and Acute MPTP-Induced Parkinson's Disease. Bioorg. Med. Chem. 21, 5532–5547. doi:10.1016/j.bmc.2013.05.065
Gan, L., Vargas, M. R., Johnson, D. A., and Johnson, J. A. (2012). Astrocyte-specific Overexpression of Nrf2 Delays Motor Pathology and Synuclein Aggregation throughout the CNS in the Alpha-Synuclein Mutant (A53T) Mouse Model. J. Neurosci. 32, 17775–17787. doi:10.1523/JNEUROSCI.3049-12.2012
Gao, H. M., Hong, J. S., Zhang, W., and Liu, B. (2002). Distinct Role for Microglia in Rotenone-Induced Degeneration of Dopaminergic Neurons. J. Neurosci. 22, 782–790. doi:10.1523/jneurosci.22-03-00782.2002
Gao, H. M., Liu, B., and Hong, J. S. (2003a). Critical Role for Microglial NADPH Oxidase in Rotenone-Induced Degeneration of Dopaminergic Neurons. J. Neurosci. 23, 6181–6187. doi:10.1523/jneurosci.23-15-06181.2003
Gao, H. M., Liu, B., Zhang, W., and Hong, J. S. (2003b). Critical Role of Microglial NADPH Oxidase-Derived Free Radicals in the In Vitro MPTP Model of Parkinson's Disease. FASEB J. 17, 1954–1956. doi:10.1096/fj.03-0109fje
Gao, H. M., Liu, B., Zhang, W., and Hong, J. S. (2003c). Synergistic Dopaminergic Neurotoxicity of MPTP and Inflammogen Lipopolysaccharide: Relevance to the Etiology of Parkinson's Disease. FASEB J. 17, 1957–1959. doi:10.1096/fj.03-0203fje
Gao, H. M., Zhou, H., and Hong, J. S. (2012). NADPH Oxidases: Novel Therapeutic Targets for Neurodegenerative Diseases. Trends Pharmacological Sciences 33, 295–303. doi:10.1016/j.tips.2012.03.008
Garabadu, D., and Agrawal, N. (2020). Naringin Exhibits Neuroprotection against Rotenone-Induced Neurotoxicity in Experimental Rodents. Neuromolecular Med. 22, 314–330. doi:10.1007/s12017-019-08590-2
García, E., Santana-Martínez, R., Silva-Islas, C. A., Colín-González, A. L., Galván-Arzate, S., Heras, Y., et al. (2014). S-allyl Cysteine Protects against MPTP-Induced Striatal and Nigral Oxidative Neurotoxicity in Mice: Participation of Nrf2. Free Radic. Res. 48, 159–167. doi:10.3109/10715762.2013.857019
Gatto, E. M., Riobó, N. A., Carreras, M. C., Cherñavsky, A., Rubio, A., Satz, M. L., et al. (2000). Overexpression of Neutrophil Neuronal Nitric Oxide Synthase in Parkinson's Disease. Nitric oxide 4, 534–539. doi:10.1006/niox.2000.0288
Gilgun-Sherki, Y., Melamed, E., and Offen, D. (2001). Oxidative Stress Induced-Neurodegenerative Diseases: the Need for Antioxidants that Penetrate the Blood Brain Barrier. Neuropharmacology 40, 959–975. doi:10.1016/s0028-3908(01)00019-3
González-Burgos, E., Fernández-Moriano, C., Lozano, R., Iglesias, I., and Gómez-Serranillos, M. P. (2017). Ginsenosides Rd and Re Co-treatments Improve Rotenone-Induced Oxidative Stress and Mitochondrial Impairment in SH-Sy5y Neuroblastoma Cells. Food Chem. Toxicol. 109, 38–47. doi:10.1016/j.fct.2017.08.013
Good, P. F., Hsu, A., Werner, P., Perl, D. P., and Olanow, C. W. (1998). Protein Nitration in Parkinson's Disease. J. Neuropathol. Exp. Neurol. 57, 338–342. doi:10.1097/00005072-199804000-00006
Guemez-Gamboa, A., Estrada-Sánchez, A. M., Montiel, T., Páramo, B., Massieu, L., and Morán, J. (2011). Activation of NOX2 by the Stimulation of Ionotropic and Metabotropic Glutamate Receptors Contributes to Glutamate Neurotoxicity In Vivo through the Production of Reactive Oxygen Species and Calpain Activation. J. Neuropathol. Exp. Neurol. 70, 1020–1035. doi:10.1097/NEN.0b013e3182358e4e
Gunjima, K., Tomiyama, R., Takakura, K., Yamada, T., Hashida, K., Nakamura, Y., et al. (2014). 3,4-dihydroxybenzalacetone Protects against Parkinson's Disease-Related Neurotoxin 6-OHDA through Akt/Nrf2/glutathione Pathway. J. Cell. Biochem. 115, 151–160. doi:10.1002/jcb.24643
Guo, C., Zhu, J., Wang, J., Duan, J., Ma, S., Yin, Y., et al. (2019). Neuroprotective Effects of Protocatechuic Aldehyde through PLK2/p-GSK3β/Nrf2 Signaling Pathway in Both In Vivo and In Vitro Models of Parkinson's Disease. Aging 11, 9424–9441. doi:10.18632/aging.102394
Guo, F., Wang, X., and Liu, X. (2021). Protective Effects of Irigenin against 1-Methyl-4-Phenylpyridinium-Induced Neurotoxicity through Regulating the Keap1/Nrf2 Pathway. Phytotherapy Res. 35, 1585–1596. doi:10.1002/ptr.6926
Guo, J. D., Zhao, X., Li, Y., Li, G. R., and Liu, X. L. (2018). Damage to Dopaminergic Neurons by Oxidative Stress in Parkinson's Disease (Review). Int. J. Mol. Med. 41, 1817–1825. doi:10.3892/ijmm.2018.3406
Guo, X., Han, C., Ma, K., Xia, Y., Wan, F., Yin, S., et al. (2019). Hydralazine Protects Nigrostriatal Dopaminergic Neurons from MPP(+) and MPTP Induced Neurotoxicity: Roles of Nrf2-ARE Signaling Pathway. Front. Neurol. 10, 271. doi:10.3389/fneur.2019.00271
Gutowski, M., and Kowalczyk, S. (2013). A Study of Free Radical Chemistry: Their Role and Pathophysiological Significance. Acta Biochim. Pol. 60, 1–16. doi:10.18388/abp.2013_1944
Hara, H., Kamiya, T., and Adachi, T. (2011). Endoplasmic Reticulum Stress Inducers Provide protection against 6-Hydroxydopamine-Induced Cytotoxicity. Neurochem. Int. 58, 35–43. doi:10.1016/j.neuint.2010.10.006
Hara, H., Ohta, M., and Adachi, T. (2006). Apomorphine Protects against 6-Hydroxydopamine-Induced Neuronal Cell Death through Activation of the Nrf2-ARE Pathway. J. Neurosci. Res. 84, 860–866. doi:10.1002/jnr.20974
Hastings, T. G. (2009). The Role of Dopamine Oxidation in Mitochondrial Dysfunction: Implications for Parkinson's Disease. J. Bioenerg. biomembranes 41, 469–472. doi:10.1007/s10863-009-9257-z
Hauser, D. N., and Hastings, T. G. (2013). Mitochondrial Dysfunction and Oxidative Stress in Parkinson's Disease and Monogenic Parkinsonism. Neurobiol. Dis. 51, 35–42. doi:10.1016/j.nbd.2012.10.011
Hernandes, M. S., Café-Mendes, C. C., and Britto, L. R. (2013a). NADPH Oxidase and the Degeneration of Dopaminergic Neurons in Parkinsonian Mice. Oxidative Med. Cell. longevity 2013, 157857. doi:10.1155/2013/157857
Hernandes, M. S., Santos, G. D., Café-Mendes, C. C., Lima, L. S., Scavone, C., Munhoz, C. D., et al. (2013b). Microglial Cells Are Involved in the Susceptibility of NADPH Oxidase Knockout Mice to 6-Hydroxy-Dopamine-Induced Neurodegeneration. PloS one 8, e75532. doi:10.1371/journal.pone.0075532
Herrera, A., Muñoz, P., Steinbusch, H., and Segura-Aguilar, J. (2017). Are Dopamine Oxidation Metabolites Involved in the Loss of Dopaminergic Neurons in the Nigrostriatal System in Parkinson's Disease. ACS Chem. Neurosci. 8, 702–711. doi:10.1021/acschemneuro.7b00034
Hirsch, L., Jette, N., Frolkis, A., Steeves, T., and Pringsheim, T. (2016). The Incidence of Parkinson's Disease: A Systematic Review and Meta-Analysis. Neuroepidemiology 46, 292–300. doi:10.1159/000445751
Hou, Y., Peng, S., Li, X., Yao, J., Xu, J., and Fang, J. (2018). Honokiol Alleviates Oxidative Stress-Induced Neurotoxicity via Activation of Nrf2. ACS Chem. Neurosci. 9, 3108–3116. doi:10.1021/acschemneuro.8b00290
Hu, L. W., Yen, J. H., Shen, Y. T., Wu, K. Y., and Wu, M. J. (2014). Luteolin Modulates 6-Hydroxydopamine-Induced Transcriptional Changes of Stress Response Pathways in PC12 Cells. PloS one 9, e97880. doi:10.1371/journal.pone.0097880
Huang, J. Y., and Chuang, J. I. (2010). Fibroblast Growth Factor 9 Upregulates Heme Oxygenase-1 and Gamma-Glutamylcysteine Synthetase Expression to Protect Neurons from 1-Methyl-4-Phenylpyridinium Toxicity. Free Radic. Biol. Med. 49, 1099–1108. doi:10.1016/j.freeradbiomed.2010.06.026
Huang, J. Y., Yuan, Y. H., Yan, J. Q., Wang, Y. N., Chu, S. F., Zhu, C. G., et al. (2016). 20C, a Bibenzyl Compound Isolated from Gastrodia Elata, Protects PC12 Cells against Rotenone-Induced Apoptosis via Activation of the Nrf2/ARE/HO-1 Signaling Pathway. Acta pharmacologica Sinica 37, 731–740. doi:10.1038/aps.2015.154
Huang, S., Yuan, H., Li, W., Liu, X., Zhang, X., Xiang, D., et al. (2021). Polygonatum Sibiricum Polysaccharides Protect against MPP-Induced Neurotoxicity via the Akt/mTOR and Nrf2 Pathways. Oxidative Med. Cell. longevity 2021, 8843899. doi:10.1155/2021/8843899
Huang, T. T., Hao, D. L., Wu, B. N., Mao, L. L., and Zhang, J. (2017). Uric Acid Demonstrates Neuroprotective Effect on Parkinson's Disease Mice through Nrf2-ARE Signaling Pathway. Biochem. biophysical Res. Commun. 493, 1443–1449. doi:10.1016/j.bbrc.2017.10.004
Hwang, Y. P., and Jeong, H. G. (2010). Ginsenoside Rb1 Protects against 6-Hydroxydopamine-Induced Oxidative Stress by Increasing Heme Oxygenase-1 Expression through an Estrogen Receptor-Related PI3K/Akt/Nrf2-dependent Pathway in Human Dopaminergic Cells. Toxicol. Appl. Pharmacol. 242, 18–28. doi:10.1016/j.taap.2009.09.009
Hwang, Y. P., and Jeong, H. G. (2008). The Coffee Diterpene Kahweol Induces Heme Oxygenase-1 via the PI3K and p38/Nrf2 Pathway to Protect Human Dopaminergic Neurons from 6-Hydroxydopamine-Derived Oxidative Stress. FEBS Lett. 582, 2655–2662. doi:10.1016/j.febslet.2008.06.045
Hwang, Y. P., Kim, H. G., Han, E. H., and Jeong, H. G. (2008). Metallothionein-III Protects against 6-Hydroxydopamine-Induced Oxidative Stress by Increasing Expression of Heme Oxygenase-1 in a PI3K and ERK/Nrf2-dependent Manner. Toxicol. Appl. Pharmacol. 231, 318–327. doi:10.1016/j.taap.2008.04.019
Iannielli, A., Ugolini, G. S., Cordiglieri, C., Bido, S., Rubio, A., Colasante, G., et al. (2019). Reconstitution of the Human Nigro-Striatal Pathway On-A-Chip Reveals OPA1-dependent Mitochondrial Defects and Loss of Dopaminergic Synapses. Cel Rep. 29, 4646–4656.e4. doi:10.1016/j.celrep.2019.11.111
Inose, Y., Izumi, Y., Takada-Takatori, Y., Akaike, A., Koyama, Y., Kaneko, S., et al. (2020). Protective Effects of Nrf2-ARE Activator on Dopaminergic Neuronal Loss in Parkinson Disease Model Mice: Possible Involvement of Heme Oxygenase-1. Neurosci. Lett. 736, 135268. doi:10.1016/j.neulet.2020.135268
Inoue, Y., Hara, H., Mitsugi, Y., Yamaguchi, E., Kamiya, T., Itoh, A., et al. (2018). 4-Hydroperoxy-2-decenoic Acid Ethyl Ester Protects against 6-Hydroxydopamine-Induced Cell Death via Activation of Nrf2-ARE and eIF2α-ATF4 Pathways. Neurochem. Int. 112, 288–296. doi:10.1016/j.neuint.2017.08.011
Inyushin, M. Y., Huertas, A., Kucheryavykh, Y. V., Kucheryavykh, L. Y., Tsydzik, V., Sanabria, P., et al. (2012). L-DOPA Uptake in Astrocytic Endfeet Enwrapping Blood Vessels in Rat Brain. Parkinson's Dis. 2012, 321406. doi:10.1155/2012/321406
Isobe, C., Abe, T., and Terayama, Y. (2010). Levels of Reduced and Oxidized Coenzyme Q-10 and 8-Hydroxy-2'-Deoxyguanosine in the Cerebrospinal Fluid of Patients with Living Parkinson's Disease Demonstrate that Mitochondrial Oxidative Damage And/or Oxidative DNA Damage Contributes to the Neurodegenerative Process. Neurosci. Lett. 469, 159–163. doi:10.1016/j.neulet.2009.11.065
Itoh, K., Wakabayashi, N., Katoh, Y., Ishii, T., Igarashi, K., Engel, J. D., et al. (1999). Keap1 Represses Nuclear Activation of Antioxidant Responsive Elements by Nrf2 through Binding to the Amino-Terminal Neh2 Domain. Genes Dev. 13, 76–86. doi:10.1101/gad.13.1.76
Izumi, Y., Kataoka, H., Inose, Y., Akaike, A., Koyama, Y., and Kume, T. (2018). Neuroprotective Effect of an Nrf2-ARE Activator Identified from a Chemical Library on Dopaminergic Neurons. Eur. J. Pharmacol. 818, 470–479. doi:10.1016/j.ejphar.2017.11.023
Izumi, Y., Matsumura, A., Wakita, S., Akagi, K., Fukuda, H., Kume, T., et al. (2012). Isolation, Identification, and Biological Evaluation of Nrf2-ARE Activator from the Leaves of green Perilla (Perilla Frutescens Var. Crispa F. Viridis). Free Radic. Biol. Med. 53, 669–679. doi:10.1016/j.freeradbiomed.2012.06.021
Jakel, R. J., Townsend, J. A., Kraft, A. D., and Johnson, J. A. (2007). Nrf2-mediated protection against 6-hydroxydopamine. Brain Res. 1144, 192–201. doi:10.1016/j.brainres.2007.01.131
Jazwa, A., and Cuadrado, A. (2010). Targeting Heme Oxygenase-1 for Neuroprotection and Neuroinflammation in Neurodegenerative Diseases. Curr. Drug Targets 11, 1517–1531. doi:10.2174/1389450111009011517
Jazwa, A., Rojo, A. I., Innamorato, N. G., Hesse, M., Fernández-Ruiz, J., and Cuadrado, A. (2011). Pharmacological Targeting of the Transcription Factor Nrf2 at the Basal Ganglia Provides Disease Modifying Therapy for Experimental Parkinsonism. Antioxid. Redox signaling 14, 2347–2360. doi:10.1089/ars.2010.3731
Jenner, P. (2003). Oxidative Stress in Parkinson's Disease. Ann. Neurol. 53 (Suppl. 3), S26–S36. discussion S36–38. doi:10.1002/ana.10483
Ji, L. L., and Yeo, D. (2021). Oxidative Stress: an Evolving Definition. Fac. Rev. 10, 13. doi:10.12703/r/10-13
Ji, L., Qu, L., Wang, C., Peng, W., Li, S., Yang, H., et al. (2021). Identification and Optimization of Piperlongumine Analogues as Potential Antioxidant and Anti-inflammatory Agents via Activation of Nrf2. Eur. J. Med. Chem. 210, 112965. doi:10.1016/j.ejmech.2020.112965
Jiang, G., Hu, Y., Liu, L., Cai, J., Peng, C., and Li, Q. (2014). Gastrodin Protects against MPP(+)-induced Oxidative Stress by up Regulates Heme Oxygenase-1 Expression through P38 MAPK/Nrf2 Pathway in Human Dopaminergic Cells. Neurochem. Int. 75, 79–88. doi:10.1016/j.neuint.2014.06.003
Jiang, L., Wu, X., Wang, S., Chen, S. H., Zhou, H., Wilson, B., et al. (2016). Clozapine Metabolites Protect Dopaminergic Neurons through Inhibition of Microglial NADPH Oxidase. J. neuroinflammation 13, 110. doi:10.1186/s12974-016-0573-z
Jiang, T., Sun, Q., and Chen, S. (2016). Oxidative Stress: A Major Pathogenesis and Potential Therapeutic Target of Antioxidative Agents in Parkinson's Disease and Alzheimer's Disease. Prog. Neurobiol. 147, 1–19. doi:10.1016/j.pneurobio.2016.07.005
Jin, X., Liu, Q., Jia, L., Li, M., and Wang, X. (2015). Pinocembrin Attenuates 6-OHDA-Induced Neuronal Cell Death through Nrf2/ARE Pathway in SH-Sy5y Cells. Cell Mol. Neurobiol. 35, 323–333. doi:10.1007/s10571-014-0128-8
Jing, X., Shi, H., Zhang, C., Ren, M., Han, M., Wei, X., et al. (2015). Dimethyl Fumarate Attenuates 6-OHDA-Induced Neurotoxicity in SH-Sy5y Cells and in Animal Model of Parkinson's Disease by Enhancing Nrf2 Activity. Neuroscience 286, 131–140. doi:10.1016/j.neuroscience.2014.11.047
Jing, X., Wei, X., Ren, M., Wang, L., Zhang, X., and Lou, H. (2016). Neuroprotective Effects of Tanshinone I against 6-OHDA-Induced Oxidative Stress in Cellular and Mouse Model of Parkinson's Disease through Upregulating Nrf2. Neurochem. Res. 41, 779–786. doi:10.1007/s11064-015-1751-6
Jo, M. G., Ikram, M., Jo, M. H., Yoo, L., Chung, K. C., Nah, S. Y., et al. (2018). Gintonin Mitigates MPTP-Induced Loss of Nigrostriatal Dopaminergic Neurons and Accumulation of α-Synuclein via the Nrf2/HO-1 Pathway. Mol. Neurobiol. 56, 39. doi:10.1007/s12035-018-1020-1
Johnson, J. A., Johnson, D. A., Kraft, A. D., Calkins, M. J., Jakel, R. J., Vargas, M. R., et al. (2008). The Nrf2-ARE Pathway: an Indicator and Modulator of Oxidative Stress in Neurodegeneration. Ann. N.Y Acad. Sci. 1147, 61–69. doi:10.1196/annals.1427.036
Ju, C., Hou, L., Sun, F., Zhang, L., Zhang, Z., Gao, H., et al. (2015). Anti-oxidation and Antiapoptotic Effects of Chondroitin Sulfate on 6-Hydroxydopamine-Induced Injury through the Up-Regulation of Nrf2 and Inhibition of Mitochondria-Mediated Pathway. Neurochem. Res. 40, 1509–1519. doi:10.1007/s11064-015-1628-8
Jung, K. A., and Kwak, M. K. (2010). The Nrf2 System as a Potential Target for the Development of Indirect Antioxidants. Molecules 15, 7266–7291. doi:10.3390/molecules15107266
Kabel, A. M., Omar, M. S., Alhadhrami, A., Alharthi, S. S., and Alrobaian, M. M. (2018). Linagliptin Potentiates the Effect of L-Dopa on the Behavioural, Biochemical and Immunohistochemical Changes in Experimentally-Induced Parkinsonism: Role of Toll-like Receptor 4, TGF-Β1, NF-κB and Glucagon-like Peptide 1. Physiol. Behav. 188, 108–118. doi:10.1016/j.physbeh.2018.01.028
Kaidery, N. A., Banerjee, R., Yang, L., Smirnova, N. A., Hushpulian, D. M., Liby, K. T., et al. (2013). Targeting Nrf2-Mediated Gene Transcription by Extremely Potent Synthetic Triterpenoids Attenuate Dopaminergic Neurotoxicity in the MPTP Mouse Model of Parkinson's Disease. Antioxid. Redox signaling 18, 139–157. doi:10.1089/ars.2011.4491
Kaji, H., Matsui-Yuasa, I., Matsumoto, K., Omura, A., Kiyomoto, K., and Kojima-Yuasa, A. (2020). Sesaminol Prevents Parkinson's Disease by Activating the Nrf2-ARE Signaling Pathway. Heliyon 6, e05342. doi:10.1016/j.heliyon.2020.e05342
Kao, C. J., Chen, W. F., Guo, B. L., Feng, C. W., Hung, H. C., Yang, W. Y., et al. (2017). The 1-Tosylpentan-3-One Protects against 6-Hydroxydopamine-Induced Neurotoxicity. Int. J. Mol. Sci. 18. doi:10.3390/ijms18051096
Ke, Y., and Qian, Z. M. (2007). Brain Iron Metabolism: Neurobiology and Neurochemistry. Prog. Neurobiol. 83, 149–173. doi:10.1016/j.pneurobio.2007.07.009
Kehrer, J. P. (2000). The Haber-Weiss Reaction and Mechanisms of Toxicity. Toxicology 149, 43–50. doi:10.1016/s0300-483x(00)00231-6
Kikuchi, A., Takeda, A., Onodera, H., Kimpara, T., Hisanaga, K., Sato, N., et al. (2002). Systemic Increase of Oxidative Nucleic Acid Damage in Parkinson's Disease and Multiple System Atrophy. Neurobiol. Dis. 9, 244–248. doi:10.1006/nbdi.2002.0466
Kim, D. W., Lee, K. T., Kwon, J., Lee, H. J., Lee, D., and Mar, W. (2015). Neuroprotection against 6-OHDA-Induced Oxidative Stress and Apoptosis in SH-Sy5y Cells by 5,7-Dihydroxychromone: Activation of the Nrf2/ARE Pathway. Life Sci. 130, 25–30. doi:10.1016/j.lfs.2015.02.026
Kim, J., Lim, J., Kang, B. Y., Jung, K., and Choi, H. J. (2017). Capillarisin Augments Anti-oxidative and Anti-inflammatory Responses by Activating Nrf2/HO-1 Signaling. Neurochem. Int. 105, 11–20. doi:10.1016/j.neuint.2017.01.018
Kim, S., Indu Viswanath, A. N., Park, J. H., Lee, H. E., Park, A. Y., Choi, J. W., et al. (2020). Nrf2 Activator via Interference of Nrf2-Keap1 Interaction Has Antioxidant and Anti-inflammatory Properties in Parkinson's Disease Animal Model. Neuropharmacology 167, 107989. doi:10.1016/j.neuropharm.2020.107989
Kim, S. S., Lim, J., Bang, Y., Gal, J., Lee, S. U., Cho, Y. C., et al. (2012). Licochalcone E Activates Nrf2/antioxidant Response Element Signaling Pathway in Both Neuronal and Microglial Cells: Therapeutic Relevance to Neurodegenerative Disease. J. Nutr. Biochem. 23, 1314–1323. doi:10.1016/j.jnutbio.2011.07.012
Kim, Y., Li, E., and Park, S. (2012). Insulin-like Growth Factor-1 Inhibits 6-Hydroxydopamine-Mediated Endoplasmic Reticulum Stress-Induced Apoptosis via Regulation of Heme Oxygenase-1 and Nrf2 Expression in PC12 Cells. Int. J. Neurosci. 122, 641–649. doi:10.3109/00207454.2012.702821
Kim, Y. S., Choi, D. H., Block, M. L., Lorenzl, S., Yang, L., Kim, Y. J., et al. (2007). A Pivotal Role of Matrix Metalloproteinase-3 Activity in Dopaminergic Neuronal Degeneration via Microglial Activation. FASEB J. 21, 179–187. doi:10.1096/fj.06-5865com
Kitada, T., Asakawa, S., Hattori, N., Matsumine, H., Yamamura, Y., Minoshima, S., et al. (1998). Mutations in the Parkin Gene Cause Autosomal Recessive Juvenile Parkinsonism. Nature 392, 605–608. doi:10.1038/33416
Kobatake, E., Nakagawa, H., Seki, T., and Miyazaki, T. (2017). Protective Effects and Functional Mechanisms of Lactobacillus Gasseri SBT2055 against Oxidative Stress. PloS one 12, e0177106. doi:10.1371/journal.pone.0177106
Kraft, A. D., Johnson, D. A., and Johnson, J. A. (2004). Nuclear Factor E2-Related Factor 2-dependent Antioxidant Response Element Activation by Tert-Butylhydroquinone and Sulforaphane Occurring Preferentially in Astrocytes Conditions Neurons against Oxidative Insult. J. Neurosci. 24, 1101–1112. doi:10.1523/JNEUROSCI.3817-03.2004
Kuo, H. C., Chang, H. C., Lan, W. C., Tsai, F. H., Liao, J. C., and Wu, C. R. (2014). Protective Effects of Drynaria Fortunei against 6-Hydroxydopamine-Induced Oxidative Damage in B35 Cells via the PI3K/AKT Pathway. Food Funct. 5, 1956–1965. doi:10.1039/c4fo00219a
Kurauchi, Y., Hisatsune, A., Isohama, Y., Mishima, S., and Katsuki, H. (2012). Caffeic Acid Phenethyl Ester Protects Nigral Dopaminergic Neurons via Dual Mechanisms Involving Haem Oxygenase-1 and Brain-Derived Neurotrophic Factor. Br. J. Pharmacol. 166, 1151–1168. doi:10.1111/j.1476-5381.2012.01833.x
Kussmaul, L., and Hirst, J. (2006). The Mechanism of Superoxide Production by NADH:ubiquinone Oxidoreductase (Complex I) from Bovine Heart Mitochondria. Proc. Natl. Acad. Sci. United States America 103, 7607–7612. doi:10.1073/pnas.0510977103
Kwon, S. H., Lee, S. R., Park, Y. J., Ra, M., Lee, Y., Pang, C., et al. (2019). Suppression of 6-Hydroxydopamine-Induced Oxidative Stress by Hyperoside via Activation of Nrf2/HO-1 Signaling in Dopaminergic Neurons. Int. J. Mol. Sci. 20, 5832. doi:10.3390/ijms20235832
Langston, J. W., Ballard, P., Tetrud, J. W., and Irwin, I. (1983). Chronic Parkinsonism in Humans Due to a Product of Meperidine-Analog Synthesis. Science 219, 979–980. doi:10.1126/science.6823561
Lázaro, D. F., Pavlou, M., and Outeiro, T. F. (2017). Cellular Models as Tools for the Study of the Role of Alpha-Synuclein in Parkinson's Disease. Exp. Neurol. 298, 162–171. doi:10.1016/j.expneurol.2017.05.007
Lee, D. W., Rajagopalan, S., Siddiq, A., Gwiazda, R., Yang, L., Beal, M. F., et al. (2009). Inhibition of Prolyl Hydroxylase Protects against 1-Methyl-4-Phenyl-1,2,3,6-Tetrahydropyridine-Induced Neurotoxicity: Model for the Potential Involvement of the Hypoxia-Inducible Factor Pathway in Parkinson Disease. J. Biol. Chem. 284, 29065–29076. doi:10.1074/jbc.M109.000638
Lee, G., Joo, J. C., Choi, B. Y., Lindroth, A. M., Park, S. J., and Park, Y. J. (2016). Neuroprotective Effects of Paeonia Lactiflora Extract against Cell Death of Dopaminergic SH-Sy5y Cells Is Mediated by Epigenetic Modulation. BMC Complement. Altern. Med. 16, 208. doi:10.1186/s12906-016-1205-y
Lee, H. J., Han, J., Jang, Y., Kim, S. J., Park, J. H., Seo, K. S., et al. (2015). Docosahexaenoic Acid Prevents Paraquat-Induced Reactive Oxygen Species Production in Dopaminergic Neurons via Enhancement of Glutathione Homeostasis. Biochem. biophysical Res. Commun. 457, 95–100. doi:10.1016/j.bbrc.2014.12.085
Lee J. A., J. A., Son, H. J., Kim, J. H., Park, K. D., Shin, N., Kim, H. R., et al. (2016). A Novel Synthetic Isothiocyanate ITC-57 Displays Antioxidant, Anti-inflammatory, and Neuroprotective Properties in a Mouse Parkinson's Disease Model. Free Radic. Res. 50, 1188–1199. doi:10.1080/10715762.2016.1223293
Lee, J. A., Kim, H. R., Son, H. J., Shin, N., Han, S. H., Cheong, C. S., et al. (2020). A Novel Pyrazolo [3,4-d] Pyrimidine, KKC080106, Activates the Nrf2 Pathway and Protects Nigral Dopaminergic Neurons. Exp. Neurol. 332, 113387. doi:10.1016/j.expneurol.2020.113387
Lee, J. A., Kim, J. H., Woo, S. Y., Son, H. J., Han, S. H., Jang, B. K., et al. (2015). A Novel Compound VSC2 Has Anti-inflammatory and Antioxidant Properties in Microglia and in Parkinson's Disease Animal Model. Br. J. Pharmacol. 172, 1087–1100. doi:10.1111/bph.12973
Lee, J. E., Sim, H., Yoo, H. M., Lee, M., Baek, A., Jeon, Y. J., et al. (2020). Neuroprotective Effects of Cryptotanshinone in a Direct Reprogramming Model of Parkinson's Disease. Molecules 25, 3602. doi:10.3390/molecules25163602
Lee, J. M., Calkins, M. J., Chan, K., Kan, Y. W., and Johnson, J. A. (2003a). Identification of the NF-E2-Related Factor-2-dependent Genes Conferring protection against Oxidative Stress in Primary Cortical Astrocytes Using Oligonucleotide Microarray Analysis. J. Biol. Chem. 278, 12029–12038. doi:10.1074/jbc.M211558200
Lee, J. M., Shih, A. Y., Murphy, T. H., and Johnson, J. A. (2003b). NF-E2-related Factor-2 Mediates Neuroprotection against Mitochondrial Complex I Inhibitors and Increased Concentrations of Intracellular Calcium in Primary Cortical Neurons. J. Biol. Chem. 278, 37948–37956. doi:10.1074/jbc.M305204200
Lee, J., Song, K., Huh, E., Oh, M. S., and Kim, Y. S. (2018). Neuroprotection against 6-OHDA Toxicity in PC12 Cells and Mice through the Nrf2 Pathway by a Sesquiterpenoid from Tussilago Farfara. Redox Biol. 18, 6–15. doi:10.1016/j.redox.2018.05.015
Lee, M. S., Lee, J., Kwon, D. Y., and Kim, M. S. (2006). Ondamtanggamibang Protects Neurons from Oxidative Stress with Induction of Heme Oxygenase-1. J. ethnopharmacology 108, 294–298. doi:10.1016/j.jep.2006.05.012
Lee, Y., Heo, G., Lee, K. M., Kim, A. H., Chung, K. W., Im, E., et al. (2017). Neuroprotective Effects of 2,4-dinitrophenol in an Acute Model of Parkinson's Disease. Brain Res. 1663, 184–193. doi:10.1016/j.brainres.2017.03.018
Lhermitte, J., Kraus, W. M., and McAlpine, D. (1924). Original Papers: On the Occurrence of Abnomal Deposits of Iron in the Brain in Parkinsonism with Special Refeence to its Localisation. The J. Neurol. psychopathology 5, 195–208. doi:10.1136/jnnp.s1-5.19.195
Li, C., Tang, B., Feng, Y., Tang, F., Pui-Man Hoi, M., Su, Z., et al. (2018). Pinostrobin Exerts Neuroprotective Actions in Neurotoxin-Induced Parkinson's Disease Models through Nrf2 Induction. J. Agric. Food Chem. 66, 8307–8318. doi:10.1021/acs.jafc.8b02607
Li, H., Wu, S., Wang, Z., Lin, W., Zhang, C., and Huang, B. (2012). Neuroprotective Effects of Tert-Butylhydroquinone on Paraquat-Induced Dopaminergic Cell Degeneration in C57BL/6 Mice and in PC12 Cells. Arch. Toxicol. 86, 1729–1740. doi:10.1007/s00204-012-0935-y
Li, H. Y., Zhong, Y. F., Wu, S. Y., and Shi, N. (2007). NF-E2 Related Factor 2 Activation and Heme Oxygenase-1 Induction by Tert-Butylhydroquinone Protect against Deltamethrin-Mediated Oxidative Stress in PC12 Cells. Chem. Res. Toxicol. 20, 1242–1251. doi:10.1021/tx700076q
Li, M., Zhou, F., Xu, T., Song, H., and Lu, B. (2018). Acteoside Protects against 6-OHDA-Induced Dopaminergic Neuron Damage via Nrf2-ARE Signaling Pathway. Food Chem. Toxicol. 119, 6–13. doi:10.1016/j.fct.2018.06.018
Li, R., Wang, S., Li, T., Wu, L., Fang, Y., Feng, Y., et al. (2019). Salidroside Protects Dopaminergic Neurons by Preserving Complex I Activity via DJ-1/Nrf2-Mediated Antioxidant Pathway. Parkinson's Dis. 2019, 6073496. doi:10.1155/2019/6073496
Li, R., Zheng, N., Liang, T., He, Q., and Xu, L. (2013). Puerarin Attenuates Neuronal Degeneration and Blocks Oxidative Stress to Elicit a Neuroprotective Effect on Substantia Nigra Injury in 6-OHDA-Lesioned Rats. Brain Res. 1517, 28–35. doi:10.1016/j.brainres.2013.04.013
Li, X., Cui, X. X., Chen, Y. J., Wu, T. T., Xu, H., Yin, H., et al. (2018). Therapeutic Potential of a Prolyl Hydroxylase Inhibitor FG-4592 for Parkinson's Diseases In Vitro and In Vivo: Regulation of Redox Biology and Mitochondrial Function. Front. Aging Neurosci. 10, 121. doi:10.3389/fnagi.2018.00121
Li, X., Zhang, J., Rong, H., Zhang, X., and Dong, M. (2020a). Ferulic Acid Ameliorates MPP(+)/MPTP-Induced Oxidative Stress via ERK1/2-dependent Nrf2 Activation: Translational Implications for Parkinson Disease Treatment. Mol. Neurobiol. 57, 2981–2995. doi:10.1007/s12035-020-01934-1
Li, X., Zhang, J., Zhang, X., and Dong, M. (2020b). Puerarin Suppresses MPP(+)/MPTP-induced Oxidative Stress through an Nrf2-dependent Mechanism. Food Chem. Toxicol. 144, 111644. doi:10.1016/j.fct.2020.111644
Lin, C. H., Wei, P. C., Chen, C. M., Huang, Y. T., Lin, J. L., Lo, Y. S., et al. (2020). Lactulose and Melibiose Attenuate MPTP-Induced Parkinson's Disease in Mice by Inhibition of Oxidative Stress, Reduction of Neuroinflammation and Up-Regulation of Autophagy. Front. Aging Neurosci. 12, 226. doi:10.3389/fnagi.2020.00226
Lin, H. Y., Yeh, W. L., Huang, B. R., Lin, C., Lai, C. H., Lin, H., et al. (2012). Desipramine Protects Neuronal Cell Death and Induces Heme Oxygenase-1 Expression in Mes23.5 Dopaminergic Neurons. PloS one 7, e50138. doi:10.1371/journal.pone.0050138
Lin, Q., Hou, S., Dai, Y., Jiang, N., and Lin, Y. (2020). Monascin Exhibits Neuroprotective Effects in Rotenone Model of Parkinson's Disease via Antioxidation and Anti-neuroinflammation. Neuroreport 31, 637–643. doi:10.1097/WNR.0000000000001467
Lin, T. K., Chen, S. D., Chuang, Y. C., Lin, H. Y., Huang, C. R., Chuang, J. H., et al. (2014). Resveratrol Partially Prevents Rotenone-Induced Neurotoxicity in Dopaminergic SH-Sy5y Cells through Induction of Heme Oxygenase-1 Dependent Autophagy. Int. J. Mol. Sci. 15, 1625–1646. doi:10.3390/ijms15011625
Lin, Y. C., Uang, H. W., Lin, R. J., Chen, I. J., and Lo, Y. C. (2007). Neuroprotective Effects of Glyceryl Nonivamide against Microglia-like Cells and 6-Hydroxydopamine-Induced Neurotoxicity in SH-Sy5y Human Dopaminergic Neuroblastoma Cells. J. Pharmacol. Exp. Ther. 323, 877–887. doi:10.1124/jpet.107.125955
Liu, H., Yu, C., Xu, T., Zhang, X., and Dong, M. (2016). Synergistic Protective Effect of Paeoniflorin and β-ecdysterone against Rotenone-Induced Neurotoxicity in PC12 Cells. Apoptosis 21, 1354–1365. doi:10.1007/s10495-016-1293-7
Liu, L., Yang, S., and Wang, H. (2021). α-Lipoic Acid Alleviates Ferroptosis in the MPP+ -induced PC12 Cells via Activating the PI3K/Akt/Nrf2 Pathway. Cel Biol. Int. 45, 422–431. doi:10.1002/cbin.11505
Liu, Y., Zhang, J., Jiang, M., Cai, Q., Fang, J., and Jin, L. (2018). MANF Improves the MPP+/MPTP-Induced Parkinson's Disease via Improvement of Mitochondrial Function and Inhibition of Oxidative Stress. Am. J. translational Res. 10, 1284–1294.
Liu, Z., Cai, W., Lang, M., Yan, R., Li, Z., Zhang, G., et al. (2017). Neuroprotective Effects and Mechanisms of Action of Multifunctional Agents Targeting Free Radicals, Monoamine Oxidase B and Cholinesterase in Parkinson's Disease Model. J. Mol. Neurosci. 61, 498–510. doi:10.1007/s12031-017-0891-3
Lou, H., Jing, X., Wei, X., Shi, H., Ren, D., and Zhang, X. (2014). Naringenin Protects against 6-OHDA-Induced Neurotoxicity via Activation of the Nrf2/ARE Signaling Pathway. Neuropharmacology 79, 380–388. doi:10.1016/j.neuropharm.2013.11.026
Lu, D. Y., Chen, J. H., Tan, T. W., Huang, C. Y., Yeh, W. L., and Hsu, H. C. (2013). Resistin Protects against 6-Hydroxydopamine-Induced Cell Death in Dopaminergic-like MES23.5 Cells. J. Cell. Physiol. 228, 563–571. doi:10.1002/jcp.24163
Lu, X., Kim-Han, J. S., O'Malley, K. L., and Sakiyama-Elbert, S. E. (2012). A Microdevice Platform for Visualizing Mitochondrial Transport in Aligned Dopaminergic Axons. J. Neurosci. Methods 209, 35–39. doi:10.1016/j.jneumeth.2012.05.021
Luo, L., Chen, J., Su, D., Chen, M., Luo, B., Pi, R., et al. (2017). L-F001, a Multifunction ROCK Inhibitor Prevents 6-OHDA Induced Cell Death through Activating Akt/GSK-3beta and Nrf2/HO-1 Signaling Pathway in PC12 Cells and Attenuates MPTP-Induced Dopamine Neuron Toxicity in Mice. Neurochem. Res. 42, 615–624. doi:10.1007/s11064-016-2117-4
Lushchak, V. I., and Storey, K. B. (2021). Oxidative Stress Concept Updated: Definitions, Classifications, and Regulatory Pathways Implicated. EXCLI J. 20, 956–967. doi:10.17179/excli2021-3596
Ma, L., Zhang, B., Liu, J., Qiao, C., Liu, Y., Li, S., et al. (2020). Isoorientin Exerts a Protective Effect against 6-OHDA-Induced Neurotoxicity by Activating the AMPK/AKT/Nrf2 Signalling Pathway. Food Funct. 11, 10774–10785. doi:10.1039/d0fo02165b
Ma, M. W., Wang, J., Zhang, Q., Wang, R., Dhandapani, K. M., Vadlamudi, R. K., et al. (2017). NADPH Oxidase in Brain Injury and Neurodegenerative Disorders. Mol. neurodegeneration 12, 7. doi:10.1186/s13024-017-0150-7
Magesh, S., Chen, Y., and Hu, L. (2012). Small Molecule Modulators of Keap1-Nrf2-ARE Pathway as Potential Preventive and Therapeutic Agents. Med. Res. Rev. 32, 687–726. doi:10.1002/med.21257
Mann, V. M., Cooper, J. M., Krige, D., Daniel, S. E., Schapira, A. H., and Marsden, C. D. (1992). Brain, Skeletal Muscle and Platelet Homogenate Mitochondrial Function in Parkinson's Disease. Brain 115 (Pt 2), 333–342. doi:10.1093/brain/115.2.333
Marrali, G., Casale, F., Salamone, P., Fuda, G., Ilardi, A., Manera, U., et al. (2018). NADPH Oxidases 2 Activation in Patients with Parkinson's Disease. Parkinsonism Relat. Disord. 49, 110–111. doi:10.1016/j.parkreldis.2018.01.003
Masaki, Y., Izumi, Y., Matsumura, A., Akaike, A., and Kume, T. (2017). Protective Effect of Nrf2-ARE Activator Isolated from green Perilla Leaves on Dopaminergic Neuronal Loss in a Parkinson's Disease Model. Eur. J. Pharmacol. 798, 26–34. doi:10.1016/j.ejphar.2017.02.005
McCann, S. K., Dusting, G. J., and Roulston, C. L. (2008). Early Increase of Nox4 NADPH Oxidase and Superoxide Generation Following Endothelin-1-Induced Stroke in Conscious Rats. J. Neurosci. Res. 86, 2524–2534. doi:10.1002/jnr.21700
Meng, F., Wang, J., Ding, F., Xie, Y., Zhang, Y., and Zhu, J. (2017). Neuroprotective Effect of Matrine on MPTP-Induced Parkinson's Disease and on Nrf2 Expression. Oncol. Lett. 13, 296–300. doi:10.3892/ol.2016.5383
Meng, X. B., Sun, G. B., Wang, M., Sun, J., Qin, M., and Sun, X. B. (2013). P90RSK and Nrf2 Activation via MEK1/2-Erk1/2 Pathways Mediated by Notoginsenoside R2 to Prevent 6-Hydroxydopamine-Induced Apoptotic Death in SH-Sy5y Cells. Evid Based. Complement. Altern. Med. 2013, 971712. doi:10.1155/2013/971712
Michel, H. E., Tadros, M. G., Esmat, A., Khalifa, A. E., and Abdel-Tawab, A. M. (2017). Tetramethylpyrazine Ameliorates Rotenone-Induced Parkinson's Disease in Rats: Involvement of its Anti-inflammatory and Anti-apoptotic Actions. Mol. Neurobiol. 54, 4866–4878. doi:10.1007/s12035-016-0028-7
Miesenböck, G., De Angelis, D. A., and Rothman, J. E. (1998). Visualizing Secretion and Synaptic Transmission with pH-Sensitive green Fluorescent Proteins. Nature 394, 192–195. doi:10.1038/28190
Minelli, A., Conte, C., Cacciatore, I., Cornacchia, C., and Pinnen, F. (2012). Molecular Mechanism Underlying the Cerebral Effect of Gly-Pro-Glu Tripeptide Bound to L-Dopa in a Parkinson's Animal Model. Amino acids 43, 1359–1367. doi:10.1007/s00726-011-1210-x
Minelli, A., Conte, C., Grottelli, S., Bellezza, I., Cacciatore, I., and Bolaños, J. P. (2009). Cyclo(His-Pro) Promotes Cytoprotection by Activating Nrf2-Mediated Up-Regulation of Antioxidant Defence. J. Cell. Mol. Med. 13, 1149–1161. doi:10.1111/j.1582-4934.2008.00326.x
Mizuno, K., Kume, T., Muto, C., Takada-Takatori, Y., Izumi, Y., Sugimoto, H., et al. (2011). Glutathione Biosynthesis via Activation of the Nuclear Factor E2-Related Factor 2 (Nrf2)–Antioxidant-Response Element (ARE) Pathway Is Essential for Neuroprotective Effects of Sulforaphane and 6-(methylsulfinyl) Hexyl Isothiocyanate. J. Pharmacol. Sci. 115, 320–328. doi:10.1254/jphs.10257fp
Mizuno, Y., Ohta, S., Tanaka, M., Takamiya, S., Suzuki, K., Sato, T., et al. (1989). Deficiencies in Complex I Subunits of the Respiratory Chain in Parkinson's Disease. Biochem. biophysical Res. Commun. 163, 1450–1455. doi:10.1016/0006-291x(89)91141-8
Mohamed, S. A., El-Kashef, D. H., and Nader, M. A. (2021). Tiron Alleviates MPTP-Induced Parkinsonism in Mice via Activation of Keap-1/Nrf2 Pathway. J. Biochem. Mol. Toxicol. 35, e22685. doi:10.1002/jbt.22685
Moi, P., Chan, K., Asunis, I., Cao, A., and Kan, Y. W. (1994). Isolation of NF-E2-Related Factor 2 (Nrf2), a NF-E2-like Basic Leucine Zipper Transcriptional Activator that Binds to the Tandem NF-E2/ap1 Repeat of the Beta-Globin Locus Control Region. Proc. Natl. Acad. Sci. United States America 91, 9926–9930. doi:10.1073/pnas.91.21.9926
Moon, H., Jang, J. H., Jang, T. C., and Park, G. H. (2018). Carbon Monoxide Ameliorates 6-Hydroxydopamine-Induced Cell Death in C6 Glioma Cells. Biomolecules Ther. 26, 175–181. doi:10.4062/biomolther.2018.009
More, S., and Choi, D. K. (2017a). Neuroprotective Role of Atractylenolide-I in an In Vitro and In Vivo Model of Parkinson's Disease. Nutrients 9, 451. doi:10.3390/nu9050451
More, S. V., and Choi, D. K. (2017b). Atractylenolide-I Protects Human SH-Sy5y Cells from 1-Methyl-4-Phenylpyridinium-Induced Apoptotic Cell Death. Int. J. Mol. Sci. 18, 1012. doi:10.3390/ijms18051012
Moreira, S., Fonseca, I., Nunes, M. J., Rosa, A., Lemos, L., Rodrigues, E., et al. (2017). Nrf2 Activation by Tauroursodeoxycholic Acid in Experimental Models of Parkinson's Disease. Exp. Neurol. 295, 77–87. doi:10.1016/j.expneurol.2017.05.009
Morris, C. M., and Edwardson, J. A. (1994). Iron Histochemistry of the Substantia Nigra in Parkinson's Disease. Neurodegeneration 3, 277–282.
Morroni, F., Sita, G., Djemil, A., D'Amico, M., Pruccoli, L., Cantelli-Forti, G., et al. (2018). Comparison of Adaptive Neuroprotective Mechanisms of Sulforaphane and its Interconversion Product Erucin in In Vitro and In Vivo Models of Parkinson's Disease. J. Agric. Food Chem. 66, 856–865. doi:10.1021/acs.jafc.7b04641
Muñoz, A. M., Rey, P., Soto-Otero, R., Guerra, M. J., and Labandeira-Garcia, J. L. (2004). Systemic Administration of N-Acetylcysteine Protects Dopaminergic Neurons against 6-Hydroxydopamine-Induced Degeneration. J. Neurosci. Res. 76, 551–562. doi:10.1002/jnr.20107
Murakami, S., Miyazaki, I., and Asanuma, M. (2018). Neuroprotective Effect of Fermented Papaya Preparation by Activation of Nrf2 Pathway in Astrocytes. Nutr. Neurosci. 21, 176–184. doi:10.1080/1028415X.2016.1253171
Mythri, R. B., Venkateshappa, C., Harish, G., Mahadevan, A., Muthane, U. B., Yasha, T. C., et al. (2011). Evaluation of Markers of Oxidative Stress, Antioxidant Function and Astrocytic Proliferation in the Striatum and Frontal Cortex of Parkinson's Disease Brains. Neurochem. Res. 36, 1452–1463. doi:10.1007/s11064-011-0471-9
Napolitano, A., Manini, P., and d'Ischia, M. (2011). Oxidation Chemistry of Catecholamines and Neuronal Degeneration: an Update. Curr. Med. Chem. 18, 1832–1845. doi:10.2174/092986711795496863
Nichols, W. C., Pankratz, N., Hernandez, D., Paisán-Ruíz, C., Jain, S., Halter, C. A., et al. (2005). Genetic Screening for a Single Common LRRK2 Mutation in Familial Parkinson's Disease. Lancet 365, 410–412. doi:10.1016/S0140-6736(05)17828-3
Oh, H., Hur, J., Park, G., Kim, H. G., Kim, Y. O., and Oh, M. S. (2013). Sanguisorbae Radix Protects against 6-Hydroxydopamine-Induced Neurotoxicity by Regulating NADPH Oxidase and NF-E2-Related Factor-2/heme Oxygenase-1 Expressions. Phytotherapy Res. 27, 1012–1017. doi:10.1002/ptr.4802
Oliveira, L., Gasser, T., Edwards, R., Zweckstetter, M., Melki, R., Stefanis, L., et al. (2021). Alpha-synuclein Research: Defining Strategic Moves in the Battle against Parkinson's Disease. NPJ Parkinson's Dis. 7, 65. doi:10.1038/s41531-021-00203-9
Onyango, I. G. (2008). Mitochondrial Dysfunction and Oxidative Stress in Parkinson's Disease. Neurochem. Res. 33, 589–597. doi:10.1007/s11064-007-9482-y
Outeiro, T. F., Heutink, P., Bezard, E., and Cenci, A. M. (2021). From iPS Cells to Rodents and Nonhuman Primates: Filling Gaps in Modeling Parkinson's Disease. Move. Disord. 36, 832–841. doi:10.1002/mds.28387
Ozkan, A., Parlak, H., Tanriover, G., Dilmac, S., Ulker, S. N., Birsen, I., et al. (2016). The Protective Mechanism of Docosahexaenoic Acid in Mouse Model of Parkinson: The Role of Hemeoxygenase. Neurochem. Int. 101, 110. doi:10.1016/j.neuint.2016.10.012
Paisán-Ruíz, C., Jain, S., Evans, E. W., Gilks, W. P., Simón, J., van der Brug, M., et al. (2004). Cloning of the Gene Containing Mutations that Cause PARK8-Linked Parkinson's Disease. Neuron 44, 595–600. doi:10.1016/j.neuron.2004.10.023
Pan, P. K., Qiao, L. Y., and Wen, X. N. (2016). Safranal Prevents Rotenone-Induced Oxidative Stress and Apoptosis in an In Vitro Model of Parkinson's Disease through Regulating Keap1/Nrf2 Signaling Pathway. Cell Mol. Biol. 62, 11–17. doi:10.14715/cmb/2016.62.14.2
Parada, E., Buendia, I., Navarro, E., Avendaño, C., Egea, J., and López, M. G. (2015). Microglial HO-1 Induction by Curcumin Provides Antioxidant, Antineuroinflammatory, and Glioprotective Effects. Mol. Nutr. Food Res. 59, 1690–1700. doi:10.1002/mnfr.201500279
Parada, E., Egea, J., Romero, A., del Barrio, L., García, A. G., and López, M. G. (2010). Poststress Treatment with PNU282987 Can rescue SH-Sy5y Cells Undergoing Apoptosis via α7 Nicotinic Receptors Linked to a Jak2/Akt/HO-1 Signaling Pathway. Free Radic. Biol. Med. 49, 1815–1821. doi:10.1016/j.freeradbiomed.2010.09.017
Park, J. S., Leem, Y. H., Park, J. E., Kim, D. Y., and Kim, H. S. (2019). Neuroprotective Effect of β-Lapachone in MPTP-Induced Parkinson's Disease Mouse Model: Involvement of Astroglial P-AMPK/Nrf2/HO-1 Signaling Pathways. Biomolecules Ther. 27, 178–184. doi:10.4062/biomolther.2018.234
Park, S. Y., Son, B. G., Park, Y. H., Kim, C. M., Park, G., and Choi, Y. W. (2014). The Neuroprotective Effects of α-iso-cubebene on Dopaminergic Cell Death: Involvement of CREB/Nrf2 Signaling. Neurochem. Res. 39, 1759–1766. doi:10.1007/s11064-014-1371-6
Parker, W. D., Boyson, S. J., and Parks, J. K. (1989). Abnormalities of the Electron Transport Chain in Idiopathic Parkinson's Disease. Ann. Neurol. 26, 719–723. doi:10.1002/ana.410260606
Pasban-Aliabadi, H., Esmaeili-Mahani, S., and Abbasnejad, M. (2017). Orexin-A Protects Human Neuroblastoma SH-Sy5y Cells against 6-Hydroxydopamine-Induced Neurotoxicity: Involvement of PKC and PI3K Signaling Pathways. Rejuvenation Res. 20, 125–133. doi:10.1089/rej.2016.1836
Pearce, R. K., Owen, A., Daniel, S., Jenner, P., and Marsden, C. D. (1997). Alterations in the Distribution of Glutathione in the Substantia Nigra in Parkinson's Disease. J. Neural Transm. 104, 661–677. doi:10.1007/BF01291884
Peng, S., Hou, Y., Yao, J., and Fang, J. (2017). Activation of Nrf2-Driven Antioxidant Enzymes by Cardamonin Confers Neuroprotection of PC12 Cells against Oxidative Damage. Food Funct. 8, 997–1007. doi:10.1039/c7fo00054e
Peng, S., Zhang, B., Meng, X., Yao, J., and Fang, J. (2015a). Synthesis of Piperlongumine Analogues and Discovery of Nuclear Factor Erythroid 2-related Factor 2 (Nrf2) Activators as Potential Neuroprotective Agents. J. Med. Chem. 58, 5242–5255. doi:10.1021/acs.jmedchem.5b00410
Peng, S., Zhang, B., Yao, J., Duan, D., and Fang, J. (2015b). Dual protection of Hydroxytyrosol, an Olive Oil Polyphenol, against Oxidative Damage in PC12 Cells. Food Funct. 6, 2091–2100. doi:10.1039/c5fo00097a
Perry, T. L., Godin, D. V., and Hansen, S. (1982). Parkinson's Disease: a Disorder Due to Nigral Glutathione Deficiency. Neurosci. Lett. 33, 305–310. doi:10.1016/0304-3940(82)90390-1
Perry, T. L., Yong, V. W., Ito, M., Foulks, J. G., Wall, R. A., Godin, D. V., et al. (1984). Nigrostriatal Dopaminergic Neurons Remain Undamaged in Rats Given High Doses of L-DOPA and Carbidopa Chronically. J. Neurochem. 43, 990–993. doi:10.1111/j.1471-4159.1984.tb12834.x
Pisoschi, A. M., Pop, A., Iordache, F., Stanca, L., Predoi, G., and Serban, A. I. (2021). Oxidative Stress Mitigation by Antioxidants - an Overview on Their Chemistry and Influences on Health Status. Eur. J. Med. Chem. 209, 112891. doi:10.1016/j.ejmech.2020.112891
Polymeropoulos, M. H., Lavedan, C., Leroy, E., Ide, S. E., Dehejia, A., Dutra, A., et al. (1997). Mutation in the Alpha-Synuclein Gene Identified in Families with Parkinson's Disease. Science 276, 2045–2047. doi:10.1126/science.276.5321.2045
Prasuhn, J., Davis, R. L., and Kumar, K. R. (2020). Targeting Mitochondrial Impairment in Parkinson's Disease: Challenges and Opportunities. Front. Cel. Dev. Biol. 8, 615461. doi:10.3389/fcell.2020.615461
Przedborski, S. (2017). The Two-century Journey of Parkinson Disease Research. Nat. Rev. Neurosci. 18, 251–259. doi:10.1038/nrn.2017.25
Purisai, M. G., McCormack, A. L., Cumine, S., Li, J., Isla, M. Z., and Monte, Di. (2007). Microglial Activation as a Priming Event Leading to Paraquat-Induced Dopaminergic Cell Degeneration. Neurobiol. Dis. 25, 392–400. doi:10.1016/j.nbd.2006.10.008
Puspita, L., Chung, S. Y., and Shim, J. W. (2017). Oxidative Stress and Cellular Pathologies in Parkinson's Disease. Mol. Brain 10, 53. doi:10.1186/s13041-017-0340-9
Qin, L., Liu, Y., Hong, J. S., and Crews, F. T. (2013). NADPH Oxidase and Aging Drive Microglial Activation, Oxidative Stress, and Dopaminergic Neurodegeneration Following Systemic LPS Administration. Glia 61, 855–868. doi:10.1002/glia.22479
Qin, L., Liu, Y., Wang, T., Wei, S. J., Block, M. L., Wilson, B., et al. (2004). NADPH Oxidase Mediates Lipopolysaccharide-Induced Neurotoxicity and Proinflammatory Gene Expression in Activated Microglia. J. Biol. Chem. 279, 1415–1421. doi:10.1074/jbc.M307657200
Qu, L., Xu, H., Jia, W., Jiang, H., and Xie, J. (2019). Rosmarinic Acid Protects against MPTP-Induced Toxicity and Inhibits Iron-Induced α-synuclein Aggregation. Neuropharmacology 144, 291–300. doi:10.1016/j.neuropharm.2018.09.042
Quesada, A., Micevych, P., and Handforth, A. (2009). C-terminal Mechano Growth Factor Protects Dopamine Neurons: a Novel Peptide that Induces Heme Oxygenase-1. Exp. Neurol. 220, 255–266. doi:10.1016/j.expneurol.2009.08.029
Quesada, A., Ogi, J., Schultz, J., and Handforth, A. (2011). C-terminal Mechano-Growth Factor Induces Heme Oxygenase-1-Mediated Neuroprotection of SH-Sy5y Cells via the Protein Kinase Cϵ/Nrf2 Pathway. J. Neurosci. Res. 89, 394–405. doi:10.1002/jnr.22543
Rai, S. N., Birla, H., Singh, S. S., Zahra, W., Patil, R. R., Jadhav, J. P., et al. (2017). Mucuna Pruriens Protects against MPTP Intoxicated Neuroinflammation in Parkinson's Disease through NF-κB/pAKT Signaling Pathways. Front. Aging Neurosci. 9, 421. doi:10.3389/fnagi.2017.00421
Rai, S. N., Chaturvedi, V. K., Singh, P., Singh, B. K., and Singh, M. P. (2020). Mucuna Pruriens in Parkinson's and in Some Other Diseases: Recent Advancement and Future Prospective. 3 Biotech. 10, 522. doi:10.1007/s13205-020-02532-7
Rai, S. N., Dilnashin, H., Birla, H., Singh, S. S., Zahra, W., Rathore, A. S., et al. (2019a). The Role of PI3K/Akt and ERK in Neurodegenerative Disorders. Neurotoxicity Res. 35, 775–795. doi:10.1007/s12640-019-0003-y
Rai, S. N., and Singh, P. (2020). Advancement in the Modelling and Therapeutics of Parkinson's Disease. J. Chem. Neuroanat. 104, 101752. doi:10.1016/j.jchemneu.2020.101752
Rai, S. N., Singh, P., Varshney, R., Chaturvedi, V. K., Vamanu, E., Singh, M. P., et al. (2021). Promising Drug Targets and Associated Therapeutic Interventions in Parkinson's Disease. Neural Regen. Res. 16, 1730–1739. doi:10.4103/1673-5374.306066
Rai, S. N., Zahra, W., Singh, S. S., Birla, H., Keswani, C., Dilnashin, H., et al. (2019b). Anti-inflammatory Activity of Ursolic Acid in MPTP-Induced Parkinsonian Mouse Model. Neurotoxicity Res. 36, 452–462. doi:10.1007/s12640-019-00038-6
Rasheed, M., Tripathi, M. K., Patel, D. K., and Singh, M. P. (2020). Resveratrol Regulates Nrf2-Mediated Expression of Antioxidant and Xenobiotic Metabolizing Enzymes in Pesticides-Induced Parkinsonism. Protein Pept. Lett. 27, 1038–1045. doi:10.2174/0929866527666200403110036
Reeve, V. E., and Tyrrell, R. M. (1999). Heme Oxygenase Induction Mediates the Photoimmunoprotective Activity of UVA Radiation in the Mouse. Proc. Natl. Acad. Sci. United States America 96, 9317–9321. doi:10.1073/pnas.96.16.9317
Ren, J., Yuan, L., Wang, W., Zhang, M., Wang, Q., Li, S., et al. (2019). Tricetin Protects against 6-OHDA-Induced Neurotoxicity in Parkinson's Disease Model by Activating Nrf2/HO-1 Signaling Pathway and Preventing Mitochondria-dependent Apoptosis Pathway. Toxicol. Appl. Pharmacol. 378, 114617. doi:10.1016/j.taap.2019.114617
Riederer, P., Sofic, E., Rausch, W. D., Schmidt, B., Reynolds, G. P., Jellinger, K., et al. (1989). Transition Metals, Ferritin, Glutathione, and Ascorbic Acid in Parkinsonian Brains. J. Neurochem. 52, 515–520. doi:10.1111/j.1471-4159.1989.tb09150.x
Rodriguez-Pallares, J., Parga, J. A., Muñoz, A., Rey, P., Guerra, M. J., and Labandeira-Garcia, J. L. (2007). Mechanism of 6-hydroxydopamine Neurotoxicity: the Role of NADPH Oxidase and Microglial Activation in 6-Hydroxydopamine-Induced Degeneration of Dopaminergic Neurons. J. Neurochem. 103, 145–156. doi:10.1111/j.1471-4159.2007.04699.x
Romero, A., Egea, J., García, A. G., and López, M. G. (2010). Synergistic Neuroprotective Effect of Combined Low Concentrations of Galantamine and Melatonin against Oxidative Stress in SH-Sy5y Neuroblastoma Cells. J. pineal Res. 49, 141–148. doi:10.1111/j.1600-079X.2010.00778.x
Rossi, F., and Zatti, M. (1964). Biochemical Aspects of Phagocytosis in Polymorphonuclear Leucocytes. NADH and NADPH Oxidation by the Granules of Resting and Phagocytizing Cells. Experientia 20, 21–23. doi:10.1007/BF02146019
Royer-Pokora, B., Kunkel, L. M., Monaco, A. P., Goff, S. C., Newburger, P. E., Baehner, R. L., et al. (1986). Cloning the Gene for an Inherited Human Disorder–Chronic Granulomatous Disease–On the Basis of its Chromosomal Location. Nature 322, 32–38. doi:10.1038/322032a0
Ryu, J., Zhang, R., Hong, B. H., Yang, E. J., Kang, K. A., Choi, M., et al. (2013). Phloroglucinol Attenuates Motor Functional Deficits in an Animal Model of Parkinson's Disease by Enhancing Nrf2 Activity. PloS one 8, e71178. doi:10.1371/journal.pone.0071178
Sachdev, S., Ansari, S. A., Ansari, M. I., Fujita, M., and Hasanuzzaman, M. (2021). Abiotic Stress and Reactive Oxygen Species: Generation, Signaling, and Defense Mechanisms. Antioxidants 10, 277. doi:10.3390/antiox10020277
Salinas, M., Diaz, R., Abraham, N. G., Ruiz de Galarreta, C. M., and Cuadrado, A. (2003). Nerve Growth Factor Protects against 6-Hydroxydopamine-Induced Oxidative Stress by Increasing Expression of Heme Oxygenase-1 in a Phosphatidylinositol 3-kinase-dependent Manner. J. Biol. Chem. 278, 13898–13904. doi:10.1074/jbc.M209164200
Sano, A., Maehara, T., and Fujimori, K. (2021). Protection of 6-OHDA Neurotoxicity by PGF(2α) through FP-ERK-Nrf2 Signaling in SH-Sy5y Cells. Toxicology 450, 152686. doi:10.1016/j.tox.2021.152686
Sarrafchi, A., Bahmani, M., Shirzad, H., and Rafieian-Kopaei, M. (2016). Oxidative Stress and Parkinson's Disease: New Hopes in Treatment with Herbal Antioxidants. Curr. Pharm. Des. 22, 238–246. doi:10.2174/1381612822666151112151653
Savica, R., Grossardt, B. R., Bower, J. H., Ahlskog, J. E., and Rocca, W. A. (2016). Time Trends in the Incidence of Parkinson Disease. JAMA Neurol. 73, 981–989. doi:10.1001/jamaneurol.2016.0947
Sbarra, A. J., and Karnovsky, M. L. (1959). The Biochemical Basis of Phagocytosis. I. Metabolic Changes during the Ingestion of Particles by Polymorphonuclear Leukocytes. J. Biol. Chem. 234, 1355–1362. doi:10.1016/s0021-9258(18)70011-2
Schapira, A. H., Cooper, J. M., Dexter, D., Clark, J. B., Jenner, P., and Marsden, C. D. (1990). Mitochondrial Complex I Deficiency in Parkinson's Disease. J. Neurochem. 54, 823–827. doi:10.1111/j.1471-4159.1990.tb02325.x
Schapira, A. H., Cooper, J. M., Dexter, D., Jenner, P., Clark, J. B., and Marsden, C. D. (1989). Mitochondrial Complex I Deficiency in Parkinson's Disease. Lancet 1, 1269. doi:10.1016/s0140-6736(89)92366-0
Schipper, H. M., Song, W., Tavitian, A., and Cressatti, M. (2019). The Sinister Face of Heme Oxygenase-1 in Brain Aging and Disease. Prog. Neurobiol. 172, 40–70. doi:10.1016/j.pneurobio.2018.06.008
Segal, A. W., and Jones, O. T. (1978). Novel Cytochrome B System in Phagocytic Vacuoles of Human Granulocytes. Nature 276, 515–517. doi:10.1038/276515a0
Segal, A. W., Jones, O. T., Webster, D., and Allison, A. C. (1978). Absence of a Newly Described Cytochrome B from Neutrophils of Patients with Chronic Granulomatous Disease. Lancet 2, 446–449. doi:10.1016/s0140-6736(78)91445-9
Segura-Aguilar, J., Muñoz, P., and Paris, I. (2016). Aminochrome as New Preclinical Model to Find New Pharmacological Treatment that Stop the Development of Parkinson's Disease. Curr. Med. Chem. 23, 346–359. doi:10.2174/0929867323666151223094103
Segura-Aguilar, J., Paris, I., Muñoz, P., Ferrari, E., Zecca, L., and Zucca, F. A. (2014). Protective and Toxic Roles of Dopamine in Parkinson's Disease. J. Neurochem. 129, 898–915. doi:10.1111/jnc.12686
Seidi, A., Kaji, H., Annabi, N., Ostrovidov, S., Ramalingam, M., and Khademhosseini, A. (2011). A Microfluidic-Based Neurotoxin Concentration Gradient for the Generation of an In Vitro Model of Parkinson's Disease. Biomicrofluidics 5, 22214. doi:10.1063/1.3580756
Sharma, N., Khurana, N., Muthuraman, A., and Utreja, P. (2021). Pharmacological Evaluation of Vanillic Acid in Rotenone-Induced Parkinson's Disease Rat Model. Eur. J. Pharmacol. 903, 174112. doi:10.1016/j.ejphar.2021.174112
Sheng, X., Yang, S., Wen, X., Zhang, X., Ye, Y., Zhao, P., et al. (2021). Neuroprotective Effects of Shende'an Tablet in the Parkinson's Disease Model. Chin. Med. 16, 18. doi:10.1186/s13020-021-00429-y
Shih, A. Y., Johnson, D. A., Wong, G., Kraft, A. D., Jiang, L., Erb, H., et al. (2003). Coordinate Regulation of Glutathione Biosynthesis and Release by Nrf2-Expressing Glia Potently Protects Neurons from Oxidative Stress. J. Neurosci. 23, 3394–3406. doi:10.1523/JNEUROSCI.23-08-03394.2003
Shih, Y. T., Chen, I. J., Wu, Y. C., and Lo, Y. C. (2011). San-Huang-Xie-Xin-Tang Protects against Activated Microglia- and 6-OHDA-Induced Toxicity in Neuronal SH-Sy5y Cells. Evid Based. Complement. Altern. Med. 2011, 429384. doi:10.1093/ecam/nep025
Sian, J., Dexter, D. T., Lees, A. J., Daniel, S., Agid, Y., Javoy-Agid, F., et al. (1994). Alterations in Glutathione Levels in Parkinson's Disease and Other Neurodegenerative Disorders Affecting Basal Ganglia. Ann. Neurol. 36, 348–355. doi:10.1002/ana.410360305
Siebert, A., Desai, V., Chandrasekaran, K., Fiskum, G., and Jafri, M. S. (2009). Nrf2 Activators Provide Neuroprotection against 6-hydroxydopamine Toxicity in Rat Organotypic Nigrostriatal Cocultures. J. Neurosci. Res. 87, 1659–1669. doi:10.1002/jnr.21975
Sies, H. (2020a). Findings in Redox Biology: From H(2)O(2) to Oxidative Stress. J. Biol. Chem. 295, 13458–13473. doi:10.1074/jbc.X120.015651
Sies, H. (2020b). Oxidative Stress: Concept and Some Practical Aspects. Antioxidants 9, 852. doi:10.3390/antiox9090852
Smirnova, N. A., Kaidery, N. A., Hushpulian, D. M., Rakhman, I. I., Poloznikov, A. A., Tishkov, V. I., et al. (2016). Bioactive Flavonoids and Catechols as Hif1 and Nrf2 Protein Stabilizers - Implications for Parkinson's Disease. Aging Dis. 7, 745–762. doi:10.14336/AD.2016.0505
Son, H. J., Choi, J. H., Lee, J. A., Kim, D. J., Shin, K. J., and Hwang, O. (2015). Induction of NQO1 and Neuroprotection by a Novel Compound KMS04014 in Parkinson's Disease Models. J. Mol. Neurosci. 56, 263–272. doi:10.1007/s12031-015-0516-7
Son, H. J., Han, S. H., Lee, J. A., Shin, E. J., and Hwang, O. (2017). Potential Repositioning of Exemestane as a Neuroprotective Agent for Parkinson's Disease. Free Radic. Res. 51, 633–645. doi:10.1080/10715762.2017.1353688
Spillantini, M. G., Schmidt, M. L., Lee, V. M., Trojanowski, J. Q., Jakes, R., and Goedert, M. (1997). Alpha-synuclein in Lewy Bodies. Nature 388, 839–840. doi:10.1038/42166
Srivastav, S., Fatima, M., and Mondal, A. C. (2018). Bacopa Monnieri Alleviates Paraquat Induced Toxicity in Drosophila by Inhibiting Jnk Mediated Apoptosis through Improved Mitochondrial Function and Redox Stabilization. Neurochem. Int. 121, 98–107. doi:10.1016/j.neuint.2018.10.001
Staal, R. G., Mosharov, E. V., and Sulzer, D. (2004). Dopamine Neurons Release Transmitter via a Flickering Fusion Pore. Nat. Neurosci. 7, 341–346. doi:10.1038/nn1205
Subramaniam, S. R., and Chesselet, M. F. (2013). Mitochondrial Dysfunction and Oxidative Stress in Parkinson's Disease. Prog. Neurobiol. 106-107, 17–32. doi:10.1016/j.pneurobio.2013.04.004
Sun, W., Zheng, J., Ma, J., Wang, Z., Shi, X., Li, M., et al. (2021). Increased Plasma Heme Oxygenase-1 Levels in Patients with Early-Stage Parkinson's Disease. Front. Aging Neurosci. 13, 621508. doi:10.3389/fnagi.2021.621508
Sun, X., Zhang, H., Xie, L., Qian, C., Ye, Y., Mao, H., et al. (2020). Tristetraprolin Destabilizes NOX2 mRNA and Protects Dopaminergic Neurons from Oxidative Damage in Parkinson's Disease. FASEB J. 34, 15047–15061. doi:10.1096/fj.201902967R
Swanson, C. R., Du, E., Johnson, D. A., Johnson, J. A., and Emborg, M. E. (2013). Neuroprotective Properties of a Novel Non-thiazoledinedione Partial PPAR- γ Agonist against MPTP. PPAR Res. 2013, 582809. doi:10.1155/2013/582809
Talalay, P. (2000). Chemoprotection against Cancer by Induction of Phase 2 Enzymes. BioFactors 12, 5–11. doi:10.1002/biof.5520120102
Tarafdar, A., and Pula, G. (2018). The Role of NADPH Oxidases and Oxidative Stress in Neurodegenerative Disorders. Int. J. Mol. Sci. 19, 3824. doi:10.3390/ijms19123824
Teahan, C., Rowe, P., Parker, P., Totty, N., and Segal, A. W. (1987). The X-Linked Chronic Granulomatous Disease Gene Codes for the Beta-Chain of Cytochrome B-245. Nature 327, 720–721. doi:10.1038/327720a0
Tebay, L. E., Robertson, H., Durant, S. T., Vitale, S. R., Penning, T. M., Dinkova-Kostova, A. T., et al. (2015). Mechanisms of Activation of the Transcription Factor Nrf2 by Redox Stressors, Nutrient Cues, and Energy Status and the Pathways through Which it Attenuates Degenerative Disease. Free Radic. Biol. Med. 88, 108–146. doi:10.1016/j.freeradbiomed.2015.06.021
Terada, K., Murata, A., Toki, E., Goto, S., Yamakawa, H., Setoguchi, S., et al. (2020). Atypical Antipsychotic Drug Ziprasidone Protects against Rotenone-Induced Neurotoxicity: An In Vitro Study. Molecules 25, 4206. doi:10.3390/molecules25184206
Thapa, P., Katila, N., Choi, D. Y., Choi, H., and Nam, J. W. (2021). Suntamide A, a Neuroprotective Cyclic Peptide from Cicadidae Periostracum. Bioorg. Chem. 106, 104493. doi:10.1016/j.bioorg.2020.104493
Tiwari, M. N., Agarwal, S., Bhatnagar, P., Singhal, N. K., Tiwari, S. K., Kumar, P., et al. (2013). Nicotine-encapsulated Poly(lactic-Co-Glycolic) Acid Nanoparticles Improve Neuroprotective Efficacy against MPTP-Induced Parkinsonism. Free Radic. Biol. Med. 65, 704–718. doi:10.1016/j.freeradbiomed.2013.07.042
Todorovic, M., Wood, S. A., and Mellick, G. D. (2016). Nrf2: a Modulator of Parkinson's Disease. J. Neural Transm. 123, 611–619. doi:10.1007/s00702-016-1563-0
Tong, H., Zhang, X., Meng, X., Lu, L., Mai, D., and Qu, S. (2018). Simvastatin Inhibits Activation of NADPH Oxidase/p38 MAPK Pathway and Enhances Expression of Antioxidant Protein in Parkinson Disease Models. Front. Mol. Neurosci. 11, 165. doi:10.3389/fnmol.2018.00165
Trachootham, D., Lu, W., Ogasawara, M. A., Nilsa, R. D., and Huang, P. (2008). Redox Regulation of Cell Survival. Antioxid. Redox signaling 10, 1343–1374. doi:10.1089/ars.2007.1957
Tran, N., Nguyen, A. N., Takabe, K., Yamagata, Z., and Miyake, K. (2017). Pre-treatment with Amitriptyline Causes Epigenetic Up-Regulation of Neuroprotection-Associated Genes and Has Anti-apoptotic Effects in Mouse Neuronal Cells. Neurotoxicology and teratology 62, 1–12. doi:10.1016/j.ntt.2017.05.002
Tseng, W. T., Hsu, Y. W., and Pan, T. M. (2016). Dimerumic Acid and Deferricoprogen Activate Ak Mouse Strain Thymoma/Heme Oxygenase-1 Pathways and Prevent Apoptotic Cell Death in 6-Hydroxydopamine-Induced SH-Sy5y Cells. J. Agric. Food Chem. 64, 5995–6002. doi:10.1021/acs.jafc.6b01551
Tseng, Y. T., Hsu, Y. Y., Shih, Y. T., and Lo, Y. C. (2012). Paeonol Attenuates Microglia-Mediated Inflammation and Oxidative Stress-Induced Neurotoxicity in Rat Primary Microglia and Cortical Neurons. Shock 37, 312–318. doi:10.1097/SHK.0b013e31823fe939
Turrens, J. F. (2003). Mitochondrial Formation of Reactive Oxygen Species. J. Physiol. 552, 335–344. doi:10.1113/jphysiol.2003.049478
Valente, E. M., Abou-Sleiman, P. M., Caputo, V., Muqit, M. M., Harvey, K., Gispert, S., et al. (2004). Hereditary Early-Onset Parkinson's Disease Caused by Mutations in PINK1. Science 304, 1158–1160. doi:10.1126/science.1096284
Valente, E. M., Bentivoglio, A. R., Dixon, P. H., Ferraris, A., Ialongo, T., Frontali, M., et al. (2001). Localization of a Novel Locus for Autosomal Recessive Early-Onset Parkinsonism, PARK6, on Human Chromosome 1p35-P36. Am. J. Hum. Genet. 68, 895–900. doi:10.1086/319522
van Duijn, C. M., Dekker, M. C., Bonifati, V., Galjaard, R. J., Houwing-Duistermaat, J. J., Snijders, P. J., et al. (2001). Park7, a Novel Locus for Autosomal Recessive Early-Onset Parkinsonism, on Chromosome 1p36. Am. J. Hum. Genet. 69, 629–634. doi:10.1086/322996
van Muiswinkel, F. L., and Kuiperij, H. B. (2005). The Nrf2-ARE Signalling Pathway: Promising Drug Target to Combat Oxidative Stress in Neurodegenerative Disorders. Curr. Drug Targets CNS Neurol. Disord. 4, 267–281. doi:10.2174/1568007054038238
Vermot, A., Petit-Härtlein, I., Smith, S., and Fieschi, F. (2021). NADPH Oxidases (NOX): An Overview from Discovery, Molecular Mechanisms to Physiology and Pathology. Antioxidants 10, 890. doi:10.3390/antiox10060890
Vilariño-Güell, C., Wider, C., Ross, O. A., Dachsel, J. C., Kachergus, J. M., Lincoln, S. J., et al. (2011). VPS35 Mutations in Parkinson Disease. Am. J. Hum. Genet. 89, 162–167. doi:10.1016/j.ajhg.2011.06.001
Vile, G. F., Basu-Modak, S., Waltner, C., and Tyrrell, R. M. (1994). Heme Oxygenase 1 Mediates an Adaptive Response to Oxidative Stress in Human Skin Fibroblasts. Proc. Natl. Acad. Sci. United States America 91, 2607–2610. doi:10.1073/pnas.91.7.2607
Vinish, M., Anand, A., and Prabhakar, S. (2011). Altered Oxidative Stress Levels in Indian Parkinson's Disease Patients with PARK2 Mutations. Acta Biochim. Pol. 58, 165–169. doi:10.18388/abp.2011_2260
Wang, G., Ma, W., and Du, J. (2018). β-Caryophyllene (BCP) Ameliorates MPP+ Induced Cytotoxicity. Biomed. Pharmacother. = Biomédecine pharmacothérapie 103, 1086–1091. doi:10.1016/j.biopha.2018.03.168
Wang, H., Dou, S., Zhu, J., Shao, Z., Wang, C., and Cheng, B. (2020). Ghrelin Mitigates MPP(+)-induced Cytotoxicity: Involvement of ERK1/2-Mediated Nrf2/HO-1 and Endoplasmic Reticulum Stress PERK Signaling Pathway. Peptides 133, 170374. doi:10.1016/j.peptides.2020.170374
Wang, H., Wang, Y., Zhao, L., Cui, Q., Wang, Y., and Du, G. (2016). Pinocembrin Attenuates MPP(+)-induced Neurotoxicity by the Induction of Heme Oxygenase-1 through ERK1/2 Pathway. Neurosci. Lett. 612, 104–109. doi:10.1016/j.neulet.2015.11.048
Wang, L., Cai, X., Shi, M., Xue, L., Kuang, S., Xu, R., et al. (2020). Identification and Optimization of Piperine Analogues as Neuroprotective Agents for the Treatment of Parkinson's Disease via the Activation of Nrf2/keap1 Pathway. Eur. J. Med. Chem. 199, 112385. doi:10.1016/j.ejmech.2020.112385
Wang L., L., Wang, R., Jin, M., Huang, Y., Liu, A., Qin, J., et al. (2014). Carvedilol Attenuates 6-Hydroxydopamine-Induced Cell Death in PC12 Cells: Involvement of Akt and Nrf2/ARE Pathways. Neurochem. Res. 39, 1733–1740. doi:10.1007/s11064-014-1367-2
Wang, T., Li, C., Han, B., Wang, Z., Meng, X., Zhang, L., et al. (2020). Neuroprotective Effects of Danshensu on Rotenone-Induced Parkinson's Disease Models In Vitro and In Vivo. BMC Complement. Med. therapies 20, 20. doi:10.1186/s12906-019-2738-7
Wang, X. L., Xing, G. H., Hong, B., Li, X. M., Zou, Y., Zhang, X. J., et al. (2014). Gastrodin Prevents Motor Deficits and Oxidative Stress in the MPTP Mouse Model of Parkinson's Disease: Involvement of ERK1/2-Nrf2 Signaling Pathway. Life Sci. 114, 77–85. doi:10.1016/j.lfs.2014.08.004
Wang, Y., Yu, X., Zhang, P., Ma, Y., Wang, L., Xu, H., et al. (2018). Neuroprotective Effects of Pramipexole Transdermal Patch in the MPTP-Induced Mouse Model of Parkinson's Disease. J. Pharmacol. Sci. 138, 31–37. doi:10.1016/j.jphs.2018.08.008
Wang, Y., Zhao, W., Li, G., Chen, J., Guan, X., Chen, X., et al. (2017). Neuroprotective Effect and Mechanism of Thiazolidinedione on Dopaminergic Neurons In Vivo and In Vitro in Parkinson's Disease. PPAR Res. 2017, 4089214. doi:10.1155/2017/4089214
Wei, P. C., Lee-Chen, G. J., Chen, C. M., Wu, Y. R., Chen, Y. J., Lin, J. L., et al. (2019). Neuroprotection of Indole-Derivative Compound NC001-8 by the Regulation of the NRF2 Pathway in Parkinson's Disease Cell Models. Oxidative Med. Cell. longevity 2019, 5074367. doi:10.1155/2019/5074367
Wei, Y. Z., Zhu, G. F., Zheng, C. Q., Li, J. J., Sheng, S., Li, D. D., et al. (2020). Ellagic Acid Protects Dopamine Neurons from Rotenone-Induced Neurotoxicity via Activation of Nrf2 Signalling. J. Cell. Mol. Med. 24, 9446–9456. doi:10.1111/jcmm.15616
Weinreb, O., Mandel, S., Youdim, M., and Amit, T. (2013). Targeting Dysregulation of Brain Iron Homeostasis in Parkinson's Disease by Iron Chelators. Free Radic. Biol. Med. 62, 52–64. doi:10.1016/j.freeradbiomed.2013.01.017
Werkman, T. R., Glennon, J. C., Wadman, W. J., and McCreary, A. C. (2006). Dopamine Receptor Pharmacology: Interactions with Serotonin Receptors and Significance for the Aetiology and Treatment of Schizophrenia. CNS Neurol. Disord. Drug Targets 5, 3–23. doi:10.2174/187152706784111614
Williamson, T. P., Johnson, D. A., and Johnson, J. A. (2012). Activation of the Nrf2-ARE Pathway by siRNA Knockdown of Keap1 Reduces Oxidative Stress and Provides Partial protection from MPTP-Mediated Neurotoxicity. Neurotoxicology 33, 272–279. doi:10.1016/j.neuro.2012.01.015
Woo, S. Y., Kim, J. H., Moon, M. K., Han, S. H., Yeon, S. K., Choi, J. W., et al. (2014). Discovery of Vinyl Sulfones as a Novel Class of Neuroprotective Agents toward Parkinson's Disease Therapy. J. Med. Chem. 57, 1473–1487. doi:10.1021/jm401788m
Wruck, C. J., Claussen, M., Fuhrmann, G., Römer, L., Schulz, A., Pufe, T., et al. (2007). Luteolin Protects Rat PC12 and C6 Cells against MPP+ Induced Toxicity via an ERK Dependent Keap1-Nrf2-ARE Pathway. J. Neural Transm. Supplementum 72, 57–67. doi:10.1007/978-3-211-73574-9_9
Wu, C. C., Hsu, M. C., Hsieh, C. W., Lin, J. B., Lai, P. H., and Wung, B. S. (2006). Upregulation of Heme Oxygenase-1 by Epigallocatechin-3-Gallate via the Phosphatidylinositol 3-kinase/Akt and ERK Pathways. Life Sci. 78, 2889–2897. doi:10.1016/j.lfs.2005.11.013
Wu, C. R., Chang, H. C., Cheng, Y. D., Lan, W. C., Yang, S. E., and Ching, H. (2018). Aqueous Extract of Davallia Mariesii Attenuates 6-Hydroxydopamine-Induced Oxidative Damage and Apoptosis in B35 Cells through Inhibition of Caspase Cascade and Activation of PI3K/AKT/GSK-3β Pathway. Nutrients 10, 1449. doi:10.3390/nu10101449
Wu, D. C., Teismann, P., Tieu, K., Vila, M., Jackson-Lewis, V., Ischiropoulos, H., et al. (2003). NADPH Oxidase Mediates Oxidative Stress in the 1-Methyl-4-Phenyl-1,2,3,6-Tetrahydropyridine Model of Parkinson's Disease. Proc. Natl. Acad. Sci. United States America 100, 6145–6150. doi:10.1073/pnas.0937239100
Wu, J., Zhao, Y. M., and Deng, Z. K. (2018). Neuroprotective Action and Mechanistic Evaluation of Protodioscin against Rat Model of Parkinson's Disease. Pharmacol. Rep. 70, 139–145. doi:10.1016/j.pharep.2017.08.013
Wu, L., Xu, H., Cao, L., Li, T., Li, R., Feng, Y., et al. (2017). Salidroside Protects against MPP+-Induced Neuronal Injury through DJ-1-Nrf2 Antioxidant Pathway. Evid Based. Complement. Altern. Med. 2017, 5398542. doi:10.1155/2017/5398542
Wu, W., Han, H., Liu, J., Tang, M., Wu, X., Cao, X., et al. (2021). Fucoxanthin Prevents 6-OHDA-Induced Neurotoxicity by Targeting Keap1. Oxidative Med. Cell. longevity 2021, 6688708. doi:10.1155/2021/6688708
Wu, X. F., Block, M. L., Zhang, W., Qin, L., Wilson, B., Zhang, W. Q., et al. (2005). The Role of Microglia in Paraquat-Induced Dopaminergic Neurotoxicity. Antioxid. Redox signaling 7, 654–661. doi:10.1089/ars.2005.7.654
Xiao, H., Lv, F., Xu, W., Zhang, L., Jing, P., and Cao, X. (2011). Deprenyl Prevents MPP(+)-induced Oxidative Damage in PC12 Cells by the Upregulation of Nrf2-Mediated NQO1 Expression through the Activation of PI3K/Akt and Erk. Toxicology 290, 286–294. doi:10.1016/j.tox.2011.10.007
Xu, H., Wang, Y., Song, N., Wang, J., Jiang, H., and Xie, J. (2017). New Progress on the Role of Glia in Iron Metabolism and Iron-Induced Degeneration of Dopamine Neurons in Parkinson's Disease. Front. Mol. Neurosci. 10, 455. doi:10.3389/fnmol.2017.00455
Xu, L. L., Wu, Y. F., Yan, F., Li, C. C., Dai, Z., You, Q. D., et al. (2019). 5-(3,4-Difluorophenyl)-3-(6-methylpyridin-3-yl)-1,2,4-oxadiazole (DDO-7263), a Novel Nrf2 Activator Targeting Brain Tissue, Protects against MPTP-Induced Subacute Parkinson's Disease in Mice by Inhibiting the NLRP3 Inflammasome and Protects PC12 Cells against Oxidative Stress. Free Radic. Biol. Med. 134, 288–303. doi:10.1016/j.freeradbiomed.2019.01.003
Xu, Y., Tang, D., Wang, J., Wei, H., and Gao, J. (2019). Neuroprotection of Andrographolide against Microglia-Mediated Inflammatory Injury and Oxidative Damage in PC12 Neurons. Neurochem. Res. 44, 2619–2630. doi:10.1007/s11064-019-02883-5
Yamamoto, M., Kensler, T. W., and Motohashi, H. (2018). The KEAP1-NRF2 System: a Thiol-Based Sensor-Effector Apparatus for Maintaining Redox Homeostasis. Physiol. Rev. 98, 1169–1203. doi:10.1152/physrev.00023.2017
Yamamoto, N., Izumi, Y., Matsuo, T., Wakita, S., Kume, T., Takada-Takatori, Y., et al. (2010). Elevation of Heme Oxygenase-1 by Proteasome Inhibition Affords Dopaminergic Neuroprotection. J. Neurosci. Res. 88, 1934–1942. doi:10.1002/jnr.22363
Yamamoto, N., Sawada, H., Izumi, Y., Kume, T., Katsuki, H., Shimohama, S., et al. (2007). Proteasome Inhibition Induces Glutathione Synthesis and Protects Cells from Oxidative Stress: Relevance to Parkinson Disease. J. Biol. Chem. 282, 4364–4372. doi:10.1074/jbc.M603712200
Yan, J., Pang, Y., Zhuang, J., Lin, H., Zhang, Q., Han, L., et al. (2019). Selenepezil, a Selenium-Containing Compound, Exerts Neuroprotective Effect via Modulation of the Keap1-Nrf2-ARE Pathway and Attenuates Aβ-Induced Cognitive Impairment In Vivo. ACS Chem. Neurosci. 10, 2903–2914. doi:10.1021/acschemneuro.9b00106
Yan, J., Qiao, L., Wu, J., Fan, H., Sun, J., and Zhang, Y. (2018). Simvastatin Protects Dopaminergic Neurons against MPP+-Induced Oxidative Stress and Regulates the Endogenous Anti-oxidant System through ERK. Cell Physiol. Biochem. 51, 1957–1968. doi:10.1159/000495720
Yang, C., Mo, Y., Xu, E., Wen, H., Wei, R., Li, S., et al. (2019). Astragaloside IV Ameliorates Motor Deficits and Dopaminergic Neuron Degeneration via Inhibiting Neuroinflammation and Oxidative Stress in a Parkinson's Disease Mouse Model. Int. immunopharmacology 75, 105651. doi:10.1016/j.intimp.2019.05.036
Yang, C., Zhao, J., Cheng, Y., Le, X. C., and Rong, J. (2015). N-propargyl Caffeate Amide (PACA) Potentiates Nerve Growth Factor (NGF)-Induced Neurite Outgrowth and Attenuates 6-Hydroxydopamine (6-Ohda)-Induced Toxicity by Activating the Nrf2/HO-1 Pathway. ACS Chem. Neurosci. 6, 1560–1569. doi:10.1021/acschemneuro.5b00115
Yang, L., Calingasan, N. Y., Thomas, B., Chaturvedi, R. K., Kiaei, M., Wille, E. J., et al. (2009). Neuroprotective Effects of the Triterpenoid, CDDO Methyl Amide, a Potent Inducer of Nrf2-Mediated Transcription. PloS one 4, e5757. doi:10.1371/journal.pone.0005757
Yang, Y., Kong, F., Ding, Q., Cai, Y., Hao, Y., and Tang, B. (2020). Bruceine D Elevates Nrf2 Activation to Restrain Parkinson's Disease in Mice through Suppressing Oxidative Stress and Inflammatory Response. Biochem. biophysical Res. Commun. 526, 1013–1020. doi:10.1016/j.bbrc.2020.03.097
Ye, Q., Huang, B., Zhang, X., Zhu, Y., and Chen, X. (2012). Astaxanthin Protects against MPP(+)-induced Oxidative Stress in PC12 Cells via the HO-1/NOX2 axis. BMC Neurosci. 13, 156. doi:10.1186/1471-2202-13-156
Yoritaka, A., Hattori, N., Uchida, K., Tanaka, M., Stadtman, E. R., and Mizuno, Y. (1996). Immunohistochemical Detection of 4-hydroxynonenal Protein Adducts in Parkinson Disease. Proc. Natl. Acad. Sci. United States America 93, 2696–2701. doi:10.1073/pnas.93.7.2696
Zahra, W., Rai, S. N., Birla, H., Singh, S. S., Rathore, A. S., Dilnashin, H., et al. (2020). Neuroprotection of Rotenone-Induced Parkinsonism by Ursolic Acid in PD Mouse Model. CNS Neurol. Disord. Drug Targets 19, 527–540. doi:10.2174/1871527319666200812224457
Zakharova, E. T., Sokolov, A. V., Pavlichenko, N. N., Kostevich, V. A., Abdurasulova, I. N., Chechushkov, A. V., et al. (2018). Erythropoietin and Nrf2: Key Factors in the Neuroprotection provided by Apo-Lactoferrin. Biometals 31, 425. doi:10.1007/s10534-018-0111-9
Zawada, W. M., Banninger, G. P., Thornton, J., Marriott, B., Cantu, D., Rachubinski, A. L., et al. (2011). Generation of Reactive Oxygen Species in 1-Methyl-4-Phenylpyridinium (MPP+) Treated Dopaminergic Neurons Occurs as an NADPH Oxidase-dependent Two-Wave cascade. J. neuroinflammation 8, 129. doi:10.1186/1742-2094-8-129
Zenkov, N. K., Kozhin, P. M., Chechushkov, A. V., Martinovich, G. G., Kandalintseva, N. V., and Menshchikova, E. B. (2017). Mazes of Nrf2 Regulation. Biochemistry 82, 556–564. doi:10.1134/S0006297917050030
Zgorzynska, E., Dziedzic, B., and Walczewska, A. (2021). An Overview of the Nrf2/ARE Pathway and its Role in Neurodegenerative Diseases. Int. J. Mol. Sci. 22, 9592. doi:10.3390/ijms22179592
Zhang, B., Wang, G., He, J., Yang, Q., Li, D., Li, J., et al. (2019). Icariin Attenuates Neuroinflammation and Exerts Dopamine Neuroprotection via an Nrf2-dependent Manner. J. neuroinflammation 16, 92. doi:10.1186/s12974-019-1472-x
Zhang, C., Li, C., Chen, S., Li, Z., Jia, X., Wang, K., et al. (2017). Berberine Protects against 6-OHDA-Induced Neurotoxicity in PC12 Cells and Zebrafish through Hormetic Mechanisms Involving PI3K/AKT/Bcl-2 and Nrf2/HO-1 Pathways. Redox Biol. 11, 1–11. doi:10.1016/j.redox.2016.10.019
Zhang, D., Hu, X., Wei, S. J., Liu, J., Gao, H., Qian, L., et al. (2008). Squamosamide Derivative FLZ Protects Dopaminergic Neurons against Inflammation-Mediated Neurodegeneration through the Inhibition of NADPH Oxidase Activity. J. neuroinflammation 5, 21. doi:10.1186/1742-2094-5-21
Zhang, J., Perry, G., Smith, M. A., Robertson, D., Olson, S. J., Graham, D. G., et al. (1999). Parkinson's Disease Is Associated with Oxidative Damage to Cytoplasmic DNA and RNA in Substantia Nigra Neurons. Am. J. Pathol. 154, 1423–1429. doi:10.1016/S0002-9440(10)65396-5
Zhang, L., Hao, J., Zheng, Y., Su, R., Liao, Y., Gong, X., et al. (2018). Fucoidan Protects Dopaminergic Neurons by Enhancing the Mitochondrial Function in a Rotenone-Induced Rat Model of Parkinson's Disease. Aging Dis. 9, 590–604. doi:10.14336/AD.2017.0831
Zhang, M., An, C., Gao, Y., Leak, R. K., Chen, J., and Zhang, F. (2013). Emerging Roles of Nrf2 and Phase II Antioxidant Enzymes in Neuroprotection. Prog. Neurobiol. 100, 30–47. doi:10.1016/j.pneurobio.2012.09.003
Zhang, N., Shu, H. Y., Huang, T., Zhang, Q. L., Li, D., Zhang, G. Q., et al. (2014). Nrf2 Signaling Contributes to the Neuroprotective Effects of Urate against 6-OHDA Toxicity. PloS one 9, e100286. doi:10.1371/journal.pone.0100286
Zhang, S., Wang, R., and Wang, G. (2019). Impact of Dopamine Oxidation on Dopaminergic Neurodegeneration. ACS Chem. Neurosci. 10, 945–953. doi:10.1021/acschemneuro.8b00454
Zhang, W., Wang, T., Pei, Z., Miller, D. S., Wu, X., Block, M. L., et al. (2005). Aggregated Alpha-Synuclein Activates Microglia: a Process Leading to Disease Progression in Parkinson's Disease. FASEB J. 19, 533–542. doi:10.1096/fj.04-2751com
Zhang, X. L., Yuan, Y. H., Shao, Q. H., Wang, Z. Z., Zhu, C. G., Shi, J. G., et al. (2017). DJ-1 Regulating PI3K-Nrf2 Signaling Plays a Significant Role in Bibenzyl Compound 20C-Mediated Neuroprotection against Rotenone-Induced Oxidative Insult. Toxicol. Lett. 271, 74–83. doi:10.1016/j.toxlet.2017.02.022
Zhang, X. S., Ha, S., Wang, X. L., Shi, Y. L., Duan, S. S., and Li, Z. A. (2015). Tanshinone IIA Protects Dopaminergic Neurons against 6-Hydroxydopamine-Induced Neurotoxicity through miR-153/nf-E2-Related Factor 2/antioxidant Response Element Signaling Pathway. Neuroscience 303, 489–502. doi:10.1016/j.neuroscience.2015.06.030
Zhang, Z., Cui, W., Li, G., Yuan, S., Xu, D., Hoi, M. P., et al. (2012). Baicalein Protects against 6-OHDA-Induced Neurotoxicity through Activation of Keap1/Nrf2/HO-1 and Involving PKCα and PI3K/AKT Signaling Pathways. J. Agric. Food Chem. 60, 8171–8182. doi:10.1021/jf301511m
Zhang, Z., Li, G., Szeto, S., Chong, C. M., Quan, Q., Huang, C., et al. (2015). Examining the Neuroprotective Effects of Protocatechuic Acid and Chrysin on In Vitro and In Vivo Models of Parkinson Disease. Free Radic. Biol. Med. 84, 331–343. doi:10.1016/j.freeradbiomed.2015.02.030
Zhang, Z., Peng, L., Fu, Y., Wang, W., Wang, P., and Zhou, F. (2021). Ginnalin A Binds to the Subpockets of Keap1 Kelch Domain to Activate the Nrf2-Regulated Antioxidant Defense System in SH-Sy5y Cells. ACS Chem. Neurosci. 12, 872–882. doi:10.1021/acschemneuro.0c00713
Zhao, J., Cheng, Y. Y., Fan, W., Yang, C. B., Ye, S. F., Cui, W., et al. (2015). Botanical Drug Puerarin Coordinates with Nerve Growth Factor in the Regulation of Neuronal Survival and Neuritogenesis via Activating ERK1/2 and PI3K/Akt Signaling Pathways in the Neurite Extension Process. CNS Neurosci. Ther. 21, 61–70. doi:10.1111/cns.12334
Zhao, M., Wang, B., Zhang, C., Su, Z., Guo, B., Zhao, Y., et al. (2021). The DJ1-Nrf2-STING axis Mediates the Neuroprotective Effects of Withaferin A in Parkinson's Disease. Cel Death Differ. 28, 2517. doi:10.1038/s41418-021-00767-2
Zhao, Y. F., Zhang, Q., Xi, J. Y., Li, Y. H., Ma, C. G., and Xiao, B. G. (2015). Multitarget Intervention of Fasudil in the Neuroprotection of Dopaminergic Neurons in MPTP-Mouse Model of Parkinson's Disease. J. Neurol. Sci. 353, 28–37. doi:10.1016/j.jns.2015.03.022
Zhao, Y., Han, Y., Wang, Z., Chen, T., Qian, H., He, J., et al. (2020). Rosmarinic Acid Protects against 1-Methyl-4-Phenyl-1,2,3,6-Tetrahydropyridine-Induced Dopaminergic Neurotoxicity in Zebrafish Embryos. Toxicol. vitro 65, 104823. doi:10.1016/j.tiv.2020.104823
Zheng, J., Zhu, J. L., Zhang, Y., Zhang, H., Yang, Y., Tang, D. R., et al. (2020). PGK1 Inhibitor CBR-470-1 Protects Neuronal Cells from MPP+. Aging 12, 13388–13399. doi:10.18632/aging.103443
Zhou, J., Qu, X. D., Li, Z. Y., Ji, W., Liu, Q., Ma, Y. H., et al. (2014). Salvianolic Acid B Attenuates Toxin-Induced Neuronal Damage via Nrf2-dependent Glial Cells-Mediated Protective Activity in Parkinson's Disease Models. PloS one 9, e101668. doi:10.1371/journal.pone.0101668
Zhou, Q., Chen, B., Wang, X., Wu, L., Yang, Y., Cheng, X., et al. (2016). Sulforaphane Protects against Rotenone-Induced Neurotoxicity In Vivo: Involvement of the mTOR, Nrf2, and Autophagy Pathways. Scientific Rep. 6, 32206. doi:10.1038/srep32206
Zhou, Y., Jiang, Z., Lu, H., Xu, Z., Tong, R., Shi, J., et al. (2019). Recent Advances of Natural Polyphenols Activators for Keap1-Nrf2 Signaling Pathway. Chem. biodiversity 16, e1900400. doi:10.1002/cbdv.201900400
Zhu, J. L., Wu, Y. Y., Wu, D., Luo, W. F., Zhang, Z. Q., and Liu, C. F. (2019). SC79, a Novel Akt Activator, Protects Dopaminergic Neuronal Cells from MPP(+) and Rotenone. Mol. Cell. Biochem. 461, 81–89. doi:10.1007/s11010-019-03592-x
Zhu, L., Li, D., Chen, C., Wang, G., Shi, J., and Zhang, F. (2019). Activation of Nrf2 Signaling by Icariin Protects against 6-OHDA-Induced Neurotoxicity. Biotechnol. Appl. Biochem. 66, 465–471. doi:10.1002/bab.1743
Zou, Y. M., Liu, J., Tian, Z. Y., Lu, D., and Zhou, Y. Y. (2015). Systematic Review of the Prevalence and Incidence of Parkinson's Disease in the People's Republic of China. Neuropsychiatr. Dis. Treat. 11, 1467–1472. doi:10.2147/NDT.S85380
Zou, Y., Wang, R., Guo, H., and Dong, M. (2015). Phytoestrogen β-Ecdysterone Protects PC12 Cells against MPP+-Induced Neurotoxicity In Vitro: Involvement of PI3K-Nrf2-Regulated Pathway. Toxicol. Sci. 147, 28–38. doi:10.1093/toxsci/kfv111
Zou, Z. C., Fu, J. J., Dang, Y. Y., Zhang, Q., Wang, X. F., Chen, H. B., et al. (2021). Pinocembrin-7-Methylether Protects SH-Sy5y Cells against 6-Hydroxydopamine-Induced Neurotoxicity via Modulating Nrf2 Induction through AKT and ERK Pathways. Neurotoxicity Res. 39, 1323–1337. doi:10.1007/s12640-021-00376-4
Keywords: Parkinson’s disease, oxidative stress, Nrf2, heme oxygenase-1, neuroprotection
Citation: Wang Y, Gao L, Chen J, Li Q, Huo L, Wang Y, Wang H and Du J (2021) Pharmacological Modulation of Nrf2/HO-1 Signaling Pathway as a Therapeutic Target of Parkinson’s Disease. Front. Pharmacol. 12:757161. doi: 10.3389/fphar.2021.757161
Received: 11 August 2021; Accepted: 21 October 2021;
Published: 23 November 2021.
Edited by:
Matilde Otero-Losada, Consejo Nacional de Investigaciones Científicas y Técnicas. CAECIHS.UAI-CONICET, ArgentinaReviewed by:
Sachchida Nand Rai, University of Allahabad, IndiaIosif Pediaditakis, Flagship Pioneering, United States
Copyright © 2021 Wang, Gao, Chen, Li, Huo, Wang, Wang and Du. This is an open-access article distributed under the terms of the Creative Commons Attribution License (CC BY). The use, distribution or reproduction in other forums is permitted, provided the original author(s) and the copyright owner(s) are credited and that the original publication in this journal is cited, in accordance with accepted academic practice. No use, distribution or reproduction is permitted which does not comply with these terms.
*Correspondence: Jichen Du, ZGpjMTg5ZkAxMjYuY29t
†These authors have contributed equally to this work