- 1Department of Pharmacology, School of Basic Medicine, Shanxi Medical University, Taiyuan, China
- 2Key Laboratory of Cellular Physiology, Ministry of Education, Shanxi Medical University, Taiyuan, China
- 3Department of General Surgery, Shanxi Bethune Hospital (Third Hospital of Shanxi Medical University), Taiyuan, China
- 4School of Pharmacy, Shanxi Medical University, Taiyuan, China
- 5School of Management, Shanxi Medical University, Taiyuan, China
- 6Department of Endocrinology, First Hospital of Shanxi Medical University, Taiyuan, China
Angiotensin II type 1 (AT1) receptor blockers (ARBs), as antihypertensive drugs, have drawn attention for their benefits to individuals with diabetes and prediabetes. However, the direct effects of ARBs on insulin secretion remain unclear. In this study, we aimed to investigate the insulinotropic effect of ARBs and the underlying electrophysiological mechanism. We found that only telmisartan among the three ARBs (telmisartan, valsartan, and irbesartan) exhibited an insulin secretagogue role in rat islets. Independent of AT1 receptor and peroxisome proliferator-activated receptor γ (PPARγ), telmisartan exerted effects on ion channels including voltage-dependent potassium (Kv) channels and L-type voltage-gated calcium channels (VGCCs) to promote extracellular Ca2+ influx, thereby potentiating insulin secretion in a glucose-dependent manner. Furthermore, we identified that telmisartan directly inhibited Kv2.1 channel on a Chinese hamster ovary cell line with Kv2.1 channel overexpression. Acute exposure of db/db mice to a telmisartan dose equivalent to therapeutic doses in humans resulted in lower blood glucose and increased plasma insulin concentration in OGTT. We further observed the telmisartan-induced insulinotropic and electrophysiological effects on pathological pancreatic islets or β-cells isolated from db/db mice. Collectively, our results establish an important insulinotropic function of telmisartan distinct from other ARBs in the treatment of diabetes.
Introduction
Diabetes and hypertension constitute common clinical conditions that are interlinked through numerous pathophysiological mechanisms (Deedwania, 2004; Ferrannini and Cushman, 2012). In particular, hypertension substantively increases the risk of type 2 diabetes mellitus (T2DM), as revealed by a prospective cohort study wherein subjects with hypertension were almost 2.5 times more likely to develop T2DM than those with normal blood pressure (Gress et al., 2000). In turn, the majority (70–80%) of patients with T2DM also have hypertension (Fox et al., 2015). The coexistence of both conditions significantly increases the risks of developing nephropathy, heart failure, and other cardiovascular disease, leading to high rates of mortality and morbidity (Deedwania, 2004; Ferrannini and Cushman, 2012). Therefore, the identification of drugs that prevent both conditions would be of considerable clinical importance.
Growing evidence indicated that angiotensin II type 1 (AT1) receptor blockers (ARBs), an important drug class in the treatment of hypertension and heart failure, provided beneficial effects for patients with diabetes and prediabetes. Several clinical trials and retrospective-analyses have shown that ARBs reduce the incidence of new-onset diabetes among patients with hypertension and heart failure (Yusuf et al., 2005; Kjeldsen et al., 2006; McMurray et al., 2010). Moreover, it has been repeatedly demonstrated that ARBs improve glycemia in T2DM and its related complications such as atherosclerosis and nephropathy (Parving et al., 2001; Viberti and Wheeldon, 2002; Candido et al., 2004; Makino et al., 2008). In addition, ARBs have been highly recommended in pharmacological therapy regimens for patients with both diabetes and hypertension by the American Diabetes Association (American Diabetes Association, 2015).
T2DM is a metabolic disorder syndrome characterized by insulin resistance and deficiency. The confirmed benefits of ARBs in patients with diabetes and prediabetes have been primarily attributed to blockade of the local renin–angiotensin system (RAS). ARBs suppress oxidative stress and inflammatory responses resulting from overactivity of this system, thereby protecting β-cells against dysfunction and improving insulin sensitivity to maintain euglycemia (Shiuchi et al., 2004; Hunyady and Catt, 2006; Nagel et al., 2006; van der Zijl et al., 2011; Li et al., 2012). However, insufficient insulin secretion constitutes a fundamental process that determines the onset and progression of T2DM (Levy et al., 1998; Weyer et al., 1999), few studies have focused on the effect of ARBs on insulin secretion or its underlying mechanism.
In the present study, we applied three ARBs, namely telmisartan, valsartan, and irbesartan to evaluate the effects of ARBs on insulin secretion and investigate the underlying electrophysiological mechanism. Notably, our data showed that unlike other ARBs, telmisartan glucose-dependently elevated the intracellular [Ca2+] ([Ca2+]i) levels of β-cells through its distinctive action on ion channels, leading to enhanced insulin secretion. Our findings suggest that in addition to the typical beneficial effects of ARBs, telmisartan may serve as an insulin secretagogue in the treatment of patients with both diabetes and hypertension.
Materials and Methods
Animals and Drugs
Adult male Wistar rats, weighing 240–260 g, were purchased from Beijing Weitong Lihua experimental animal center (Beijing, PR China). Five-week-old male diabetic db/db mice (BKS - Leprem2Cd479/Gpt, stock number T002407) were obtained from GemPharmatech Co., Ltd (Nanjing, China). Rats and mice were maintained in specific-pathogen-free surroundings, with a 12 h-light/dark cycles under controlled temperature (22 ± 2°C) and humidity (55–60%) conditions, and with free access to water and food. All animal care and experimental procedures conformed to the ethical guidelines for animal research at Shanxi Medical University and were approved by the Animal Care and Use Committee of Shanxi Medical University (Taiyuan, China). Telmisartan, valsartan, GW9662 were purchased from MedChemExpress (Shanghai, China), irbesartan was purchased from Aladdin (Shanghai, China).
Isolation and Culture of Islets and Cells
The rat pancreas was isolated following injection of 1 mg/ml collagenase P (Roche, Indianapolis, IN, United States) through the common bile duct. After digestion at 37°C for 11 min and density gradient centrifugation with Histopaque-1077 (Sigma-Aldrich, St. Louis, MO, United States) for 23 min, the expanded pancreas was dispersed, and islets remaining in the supernatant separated from the sediment. The islets were hand-collected under a dissection microscope, and single islet cells were obtained from islets using Dispase II (Roche, Indianapolis, United States) digestion. The db/db mouse islets were similarly obtained, although the pancreas was injected with 1 mg/ml collagenase V (Roche, Indianapolis, United States), then digested for 16 min and centrifuged twice with Hanks Balanced Salt Solution. Isolated islets and cells were cultured in RPMI 1640 (Hyclone, Thermo Scientific, Waltham, MA, United States) medium containing 11.1 mM glucose, supplemented with 10% fetal bovine serum, 1% penicillin and streptomycin at 37°C in a humidified atmosphere of 5% CO2, 95% air.
Chinese hamster ovary (CHO) cells were obtained from the National Infrastructure of Cell Line Resource (Beijing, China). Lentivirus vectors overexpressing voltage-dependent potassium (Kv) 2.1 channels were constructed (Shanghai Genechem Co., Ltd., Shanghai, China) to transfect CHO cells, and the CHO-Kv2.1 cell line was established. CHO-Kv2.1 cells were cultured in Dulbecco’s modified Eagle’s medium (Hyclone, Thermo Scientific, Waltham, MA, United States) containing 4500 mg/L glucose in addition to 10% fetal bovine serum, 1% penicillin and streptomycin and 0.5 μg/ml puromycin (Beijing Solarbio Science & Technology Co., Ltd., Beijing, China). CHO cells were cultured under similar conditions except for puromycin selection.
Insulin Secretion Assay
Handpicked separated islets were cultured for 1–2 days before the experiment. A total of five islets per tube were pre-incubated in Krebs Ringer bicarbonate- HEPES (KRBH) buffer under 2.8 mM glucose conditions for 30 min. The KRBH buffer contained 128.8 mM NaCl, 4.8 mM KCl, 1.2 mM KH2PO4, 1.2 mM MgSO4, 2.5 mM CaCl2, 5 mM NaHCO3, and 10 mM HEPES, adjusted to pH 7.4 with NaOH prior to the addition of 2% bovine serum albumin. Islets were then treated with different drugs and glucose conditions as indicated, and supernatant liquid was collected at the end of every 30 min incubation, and stored at −20 C for insulin concentration measurement. Insulin secretion was determined using an Iodine [125I] Insulin Radioimmunoassay Kit (North Biological Technology Research Institute of Beijing).
Calcium Imaging Technology
Calcium imaging was carried out at 28–30°C using the calcium-sensitive dye Fura 2-AM (Dojindo Laboratories, Kumamoto, Japan), using an OLYMPUS IX71 inverted microscope and Meta Fluor software 7.8 (Molecular Devices, Sunnyvale, CA, United States). Islet cells were cultured on coverslips coated with adhesion reagent for 6–10 h, then were loaded with 2 μM Fura 2-AM in KRBH buffer with addition of 2.8 mM glucose for 30 min at 37°C. Ca2+-free KRBH with the same composition as KRBH except NaCl was 132.2 mM, CaCl2 was omitted, and 0.1 mM EGTA was added. Subsequently, the loading buffer was removed, and cells were washed twice with KRBH solution to remove excessive fluorescent dye. Fura-2 was excited at 340 and 380 nm wavelengths in 1 s intervals with fluorescence emission detected at 510–520 nm wavelengths. The ratio of fluorescence intensity (F340/F380) was recorded to measure intracellular Ca2+ concentrations.
Fura 2-AM-loaded islet cells on coverslips were transferred to a glass chamber containing KRBH buffer with appropriate glucose conditions. Between each test, the reagent was dripped onto the coverslip and F340/F380 data points were acquired to monitor the changes of intracellular Ca2+ level. The average value during 30 s F340/F380 spikes (15 s before and after the peak of F340/F380) for each test was used to compare the change of Ca2+ concentrations under different treatments, unless otherwise stated.
Electrophysiology
Whole-cell recording patch-clamp technology was applied to detect voltage-activated currents and record action potentials using an EPC-10 amplifier and PULSE software from HEKA Electronik (Lambrecht, Germany) at room temperature. Islet cells were cultured on glass coverslips coated with cell adherent reagent (Applygen Technologies Inc., Beijing, China).
In voltage-clamp mode, to record Kv currents, patch pipettes (5–8 MΩ) were loaded with intracellular solution containing 10 mM NaCl, 1 mM MgCl2, 0.05 mM EGTA, 140 mM KCl, 0.3 mM Mg-ATP, and 10 mM HEPES, pH 7.25 adjusted with KOH. Cells were transferred to a recording chamber containing extracellular solution consisting of 138 mM NaCl, 5.6 mM KCl, 1.2 mM MgCl2·6H2O, 2.6 mM CaCl2, 11.1 mM glucose, and 5 mM HEPES (pH 7.4 adjusted with NaOH). The β-cells were identified by cell capacitance (>7 pF) (Göpel et al., 1999) and were clamped to a holding potential of −70 mV, then test potentials were elicited by ranging from −70 to 80 mV in 10 mV steps for 400 ms.
For voltage-gated Ca2+channel (VGCC) currents, the intracellular solution contained: 120 mM CsCl, 20 mM TEA (Sigma-Aldrich), 5 mM MgATP, 1 mM MgCl2, 0.05 mM EGTA, and 10 mM HEPES (pH 7.25 adjusted with CsOH). The extracellular solution consisted of: 100 mM NaCl, 20 mM TEA, 20 mMBaCl2, 4 mM CsCl, 1 mM MgCl2, 5 mM HEPES, and 3 mM glucose (pH 7.4 adjusted with NaOH). Ca2+ was replaced with Ba2+ as the charge carrier in the extracellular solution to eliminate Ca2+-dependent inactivation of the VGCCs. β-cells were clamped to a holding potential of −70 mV, and then elicited by test potentials of −50 to 30 mV in 10 mV steps for 50 ms.
In current-clamp mode, β-cells were elicited by 4 ms currents of 150 pA to record action potentials. The time between the initiation and the point where membrane potential returned to within 10 mV of the resting membrane potential, was considered to be the measurement of action potential duration.
In vivo Evaluation of Mice and Drug Administration
At the age of 8 wk, the mice were given fasting glucose teste to ensure that diabetes models were successfully established. Given that a therapeutic doses of telmisartan are 40–80 mg/day in humans, the conversion for mice was approximately 8.2–16.4 mg/kg of body weight (Nair and Jacob, 2016). In our experiment, the mice were administered acute oral telmisartan treatment at 15 mg/kg of body weight. At 2 h following drug intake, when the onset of action of telmisartan reached a maximum, the oral glucose tolerance test (OGTT) was performed (Gohlke et al., 2001).
OGTT
At the age of 8 wk, following overnight fasting (14 h), the mice were randomly divided into groups receiving treatment with telmisartan (in drinking water containing 0.5% carboxymethyl cellulose sodium salt) or vehicle by gavage. For OGTTs, groups of mice were fed with glucose at 1.5 g/kg body weight orally, then a blood sample was collected from the tail vein and glucose levels were assessed using a Sinocare Glucometer (Changsha, China) at baseline (0 min) and after 15, 30, 60, 90, and 120 min. At the age of 11 wk, the mice were treated as described above and additional blood samples (50 μl) were obtained in a heparinized microhematocrit tube at 0, 15, 30, 60, 90, and 120 min. After centrifugation, the plasma was collected for insulin concentration measurement using the Mercodia Mouse Insulin ELISA (stock number 10-1247-01, Uppsala, Sweden).
Statistical Analysis
All experimental data are presented as the means ± SEM. p < 0.05 was considered to indicate statistical significance. Shapiro–Wilk tests were used to analyze the normality of the data. Upon normal distribution, the means of numerical variables were compared using the Student’s t test or one-way analysis of variance (ANOVA), whereas data with non-normal distribution were analyzed using the Mann–Whitney Rank Sum Test or Kruskal–Wallis one-way ANOVA on Ranks. If any statistically significant difference was detected among three or more groups, the Student–Newman–Keuls method or Tukey test was performed for post hoc comparisons, unless otherwise stated.
Results
Telmisartan, but Not Valsartan or Irbesartan, Enhances Glucose-stimulated Insulin Secretion (GSIS)
To examine the effects of ARBs on insulin secretion, isolated rat islets were treated with various doses of telmisartan. As shown in Figure 1A, telmisartan (10 and 50 μM) potentiated insulin secretion under 8.3 mM glucose conditions but had no effect under 2.8 mM glucose. Furthermore, the data in Figure 1B confirmed that telmisartan-induced insulin secretion was glucose-dependent. Next, the functions of other ARBs were evaluated. Notably, no promotion of insulin secretion was observed following treatment with valsartan and irbesartan under 8.3 (data not shown) and 16.7 mM glucose conditions (Figures 1C,D). Telmisartan, valsartan and irbesartan are clinically available ARBs owing to their high specificity for AT1 receptors (Michel et al., 2013), and the antihypertensive bioactivity of valsartan and irbesartan has been confirmed by us, therefore our results suggested that telmisartan-mediated insulinotropic effect was independent of AT1 receptors.
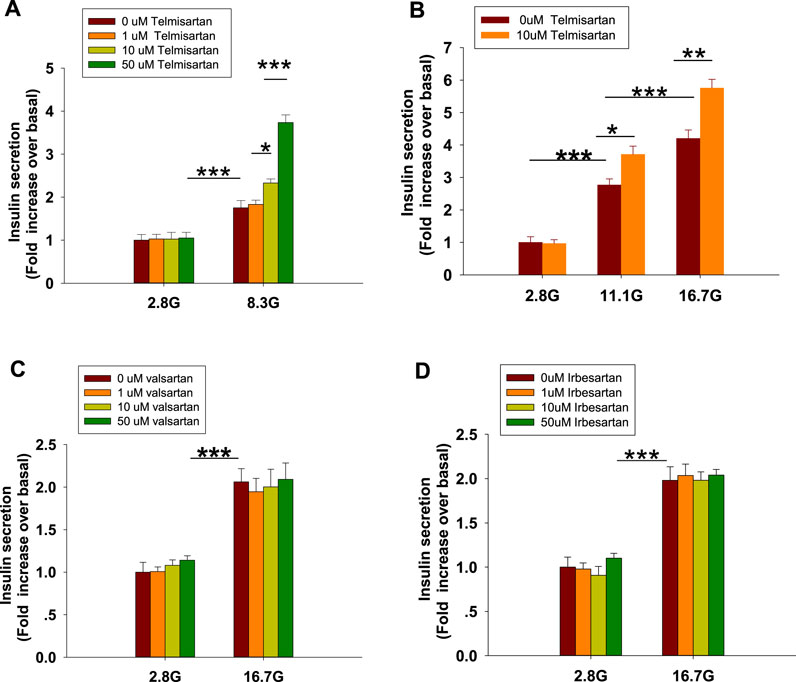
FIGURE 1. Only telmisartan among the three ARBs potentiated insulin secretion in rat islets (n = 7 tubes of islet). (A) Rat islets were treated with various doses (1, 10, and 50 μM) of telmisartan under 2.8 and 8.3 mM glucose (denoted as 2.8 G and 8.3 G) conditions. (B) Islets were treated with 10 μM telmisartan under different glucose concentrations (2.8, 11.1, and 16.7 mM). (C,D) Rat islets were treated with various doses (1, 10, and 50 μM) of valsartan or irbesartan under 2.8 and 16.7 mM glucose conditions. The islets compared between the groups in each graph are collected from the same animal. All results are normalized to basal secretion at 2.8 G, and reported as the means ± SEM. Statistical differences among three or more groups were compared using one-way analysis of variance (ANOVA) and Student–Newman–Keuls method post hoc analysis. Statistical differences between two groups (with or without telmisartan) under the same glucose condition in (B) were determined using an unpaired two-tailed Student’s t test. *p < 0.05, **p < 0.01, ***p < 0.001.
Telmisartan, but Not Valsartan or Irbesartan, Increases ([Ca2+]i) Concentration in β-Cells
Within β-cells, eliciting an increase in [Ca2+]i causes insulin granule exocytosis; therefore, the elevation in [Ca2+]i level is essential to induce insulin secretion (Sabatini et al., 2019). To verify whether the insulinotropic effect of telmisartan was related to the change in [Ca2+]i, we applied the calcium-sensitive dye Fura 2-AM to detect changes in fluorescence intensity. Telmisartan (10 and 50 μM) induced an acute increase in fluorescence intensity dose-dependently. Moreover, the elevation only occurred under high (11.1 and 16.7 mM) (Figures 2C–F) but not low (2.8 mM) (Figures 2A,B) glucose conditions. In addition, in the calcium imaging experiment, neither valsartan (Figures 2G,H) nor irbesartan (Figures 2I,J) increased the [Ca2+]i concentration of β-cells under high glucose conditions (16.7 mM).
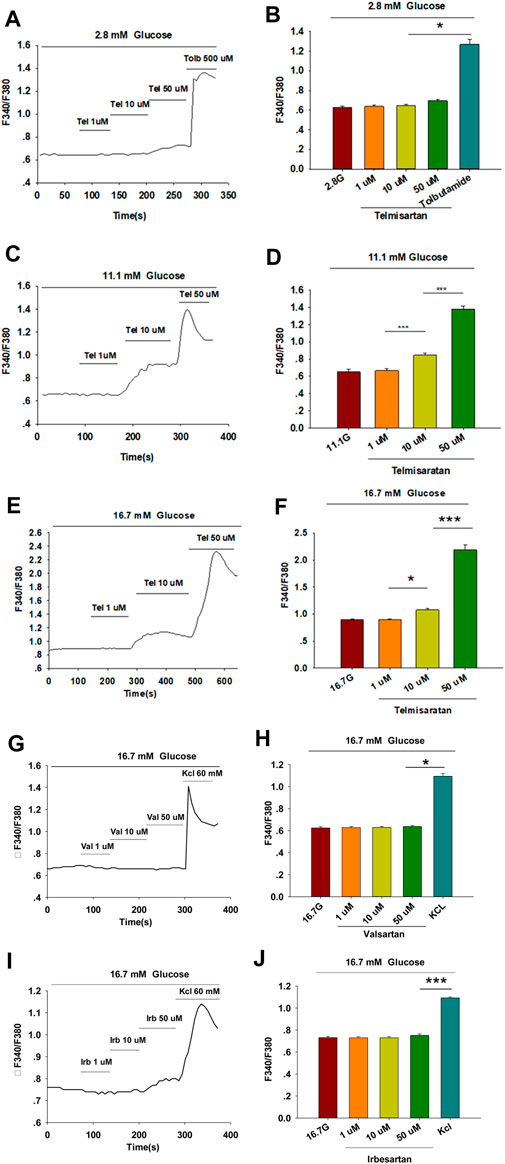
FIGURE 2. Only telmisartan among the three ARBs increased intracellular [Ca2+] ([Ca2+]i) concentration in rat pancreatic β-cells. (A) The trace shows the changes of [Ca2+]i concentration in β-cells treated with 1, 10, and 50 μM telmisartan (Tel) under 2.8 mM glucose conditions; 500 μM tolbutamide (Tolb) was used as a positive control. (B) The average value during 30 s F340/F380 spikes for each test in response to different doses of telmisartan under 2.8 mM glucose conditions as indicated (n = 9 cells). (C,D) The trace shows the changes of [Ca2+]i concentration in β-cells treated with different doses of telmisartan under 11.1 mM glucose conditions, and the average value during 30 s F340/F380 spikes for each test as indicated (n = 9 cells). (E,F) The trace shows the changes of [Ca2+]i concentration in β-cells treated with different doses of telmisartan under 16.7 mM glucose conditions, and the average value during 30 s F340/F380 spikes for each test as indicated (n = 9 cells). (G–J) The trace shows the changes of [Ca2+]i concentration in β-cells treated with 1, 10, and 50 μM of valsartan (Val) or irbesartan (Irb) under 16.7 mM glucose conditions respectively, and the average value during 30 s F340/F380 spikes for each test as indicated. KCl (60 mM) was used as a positive control (n = 9 cells). The cells compared between the groups in each graph are isolated from the same animal. All results are reported as the means ± SEM. Statistical differences among three or more groups were compared using one-way ANOVA, and followed by Student–Newman–Keuls Method post hoc analysis in (D,F,J), or Tukey post hoc analysis in (B,H). *p < 0.05, ***p < 0.001.
Peroxisome Proliferator-activated Receptor γ (PPARγ) Is Not Involved in Telmisartan-Induced Insulin Secretion and Elevation of [Ca2+]i Levels
Telmisartan and irbesartan have also been reported to function as a partial agonist of PPARγ (Schupp et al., 2004, 2005). In view of the absence of changes in insulin secretion and [Ca2+]i levels with irbesartan, we speculated that PPARγ might not be responsible for the effects of telmisartan on these measures. We therefore performed the insulin secretion assay and calcium imaging experiment using GW9662, a selective PPARγ antagonist. As shown in Figure 3, GW9662 alone had no effect on GSIS and [Ca2+]i concentration, and the addition of GW9662 did not influence the effects of telmisartan on insulin secretion or [Ca2+]i level. However, we found GW9662 could inhibit rosiglitazone-induced PPARγ activation (data not shown). Therefore, our results demonstrated that PPARγ was not involved in telmisartan-induced insulin secretion and elevation of [Ca2+]i levels.
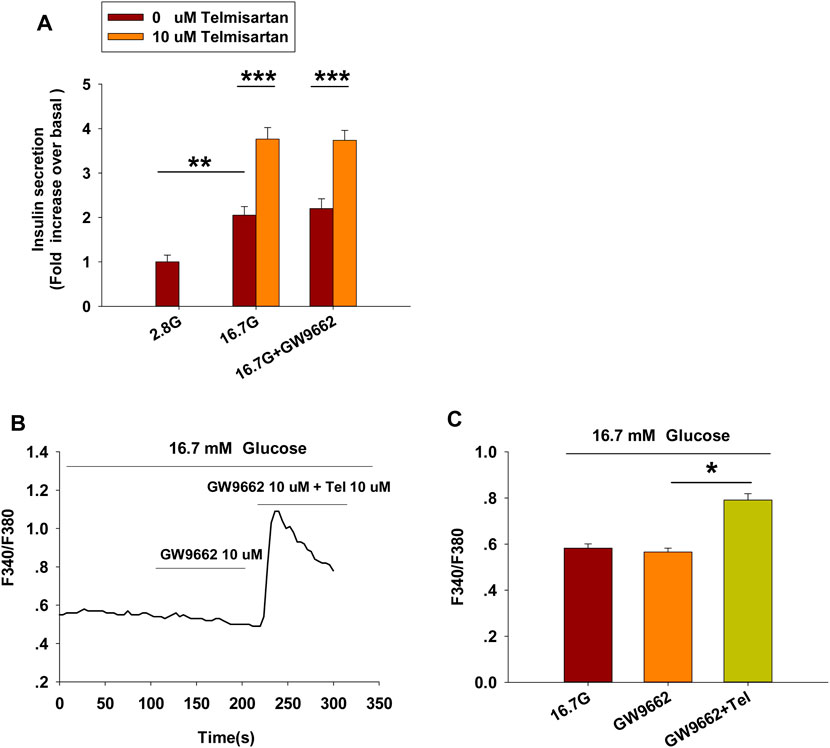
FIGURE 3. PPARγ does not participate in the pathway of telmisartan-induced insulin secretion and elevation of [Ca2+]i levels (A) Telmisartan (10 μM) potentiated glucose-stimulated insulin secretion in the presence or absence of the PPARγ inhibitor GW9662 (10 μM) (n = 7 tubes of islet). All insulin secretion results are normalized to basal secretion at 2.8 Mm glucose concentration. (B) The trace shows the changes of [Ca2+]i concentration in β-cells treated with GW9662 (10 μM) alone or in combination with telmisartan (Tel 10 μM) under 16.7 mM glucose conditions. (C) The average value during 30 s F340/F380 spikes for each test in response to GW9662 alone or in combination with telmisartan under 16.7 mM glucose conditions as indicated (n = 9 cells). The islets or cells compared between the groups in each graph are collected from the same animal. All results are reported as the means ± SEM. In (A), statistical differences between two groups (with or without telmisartan) were compared using an unpaired two-tailed Student’s t test, and difference among three groups without telmisartan were compared using one-way ANOVA and Student-Newman-Keuls method post hoc analysis. In (C), difference among three groups was determined by one-way ANOVA and Tukey Test post hoc analysis. *p < 0.05, **p < 0.01, ***p < 0.001.
Telmisartan Affects [Ca2+]i Concentration Through Extracellular Ca2+ Influx Rather Than Intracellular Ca2+ Stores Release
[Ca2+]i levels of β-cells are tightly maintained through the regulation of extracellular Ca2+ influx and the movement of Ca2+ within intracellular stores (Sabatini et al., 2019). We thus examined the concentration of [Ca2+]i in the absence of extracellular Ca2+ to study the pathway by which telmisartan increases [Ca2+]i concentration. Calcium imaging (Figures 4A,B) showed that telmisartan-induced elevation of [Ca2+]i levels was reversed in Ca2+-free KRBH medium, although the [Ca2+]i level was considerably increased upon intracellular Ca2+ mobilization via thapsigargin.
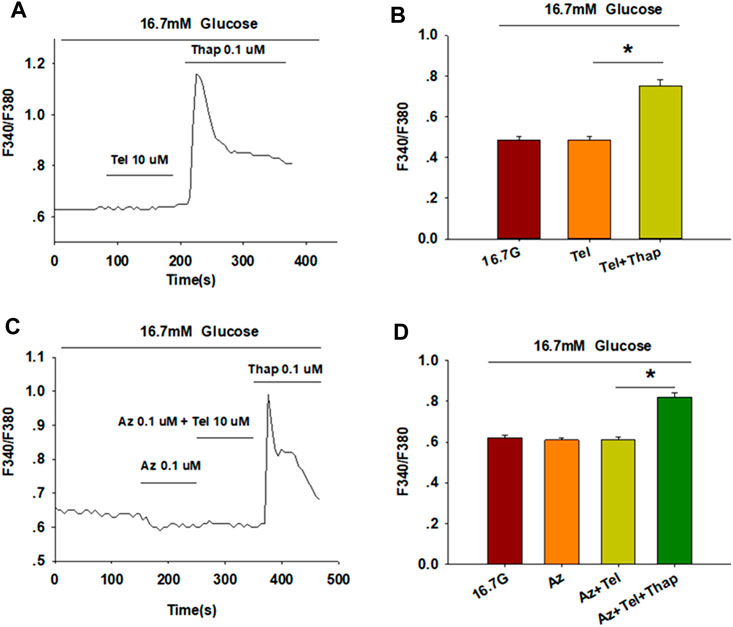
FIGURE 4. Telmisartan enhances [Ca2+]i levels through extracellular Ca2+ influx, rather than intracellular Ca2+ stores release. (A) The trace shows the changes of [Ca2+]i concentration in β-cells treated with telmisartan (Tel, 10 μM) under 16.7 mM glucose conditions in Ca2+-free KRBH medium. (B) The average value of F340/F380 during each test in response to telmisartan (Tel, 10 μM) in Ca2+-free KRB medium (n = 23 cells). (C) The trace shows the changes of [Ca2+]i concentration in β-cells treated with telmisartan (Tel 10 μM) under 16.7 mM glucose conditions with addition of the L-type VGCC blocker azelnidipine (Az, 0.1 μM). (D) The mean value of F340/F380 during each test in response to telmisartan (10 μM) with added azelnidipine (0.1 μM). Thapsigargin (Thap, 0.1 μM) was used as a positive control (n = 12 cells). The cells compared between the groups in each graph are isolated from the same animal. All results are reported as the means ± SEM. Statistical differences among three or more groups were determined by one-way ANOVA, followed by Tukey Test post hoc analysis. *p < 0.05.
Moreover, telmisartan-induced effects on [Ca2+]i levels were monitored following the application of azelnidipine, an L-type voltage-gated Ca2+channel (VGCC) blocker. We observed that the increase in [Ca2+]i levels with telmisartan was completely blocked by azelnidipine, supporting that telmisartan enhances extracellular calcium influx through L-type VGCCs. Conversely, significant elevation remained upon thapsigargin addition (Figures 4C,D), confirming the lack of telmisartan effect on intracellular calcium stores.
Telmisartan Inhibits Voltage-gated Potassium (Kv) Channels, and Prolongs Action Potential Durations (APDs) in β-Cells
Pancreatic β-cells are electrically excitatory. Previous studies have demonstrated that Kv channels play an important role in GSIS and glucose-stimulated increase of [Ca2+]i (Roe et al., 1996; MacDonald and Wheeler, 2003; Herrington et al., 2006); therefore, we applied patch-clamp techniques to explore the effects of telmisartan on the Kv channels of β-cells. Figures 5A,B illustrate that telmisartan decreased the Kv channel currents compared with that of controls.
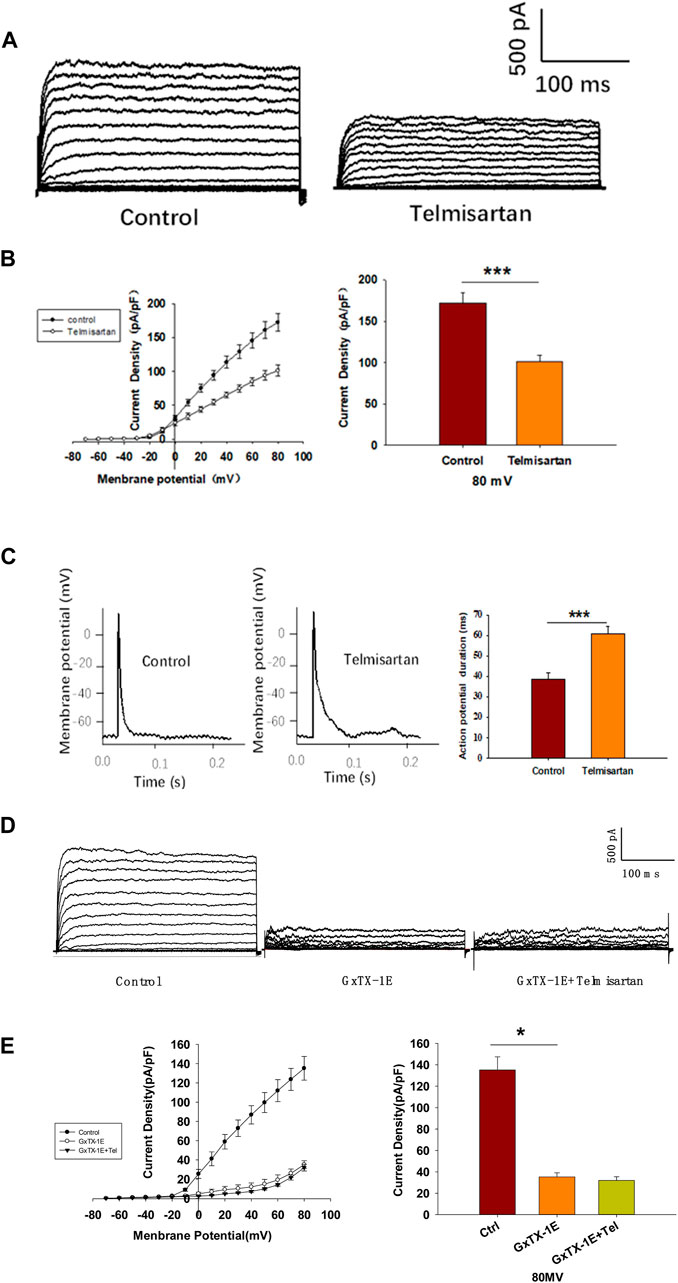
FIGURE 5. Pancreatic β-cells treated with telmisartan exhibit reduced Kv currents and extended APD. (A) Kv currents were recorded in voltage-clamp mode with holding potential from −70 to +80 mV in 10 mV increments. Representative current traces recorded in control and telmisartan-treated (10 μM) β-cells. (B) Current-voltage relationship curves of Kv channels and summary of the mean current density of Kv channels recorded at 80 mV depolarization (control n = 9 cells, telmisartan n = 7 cells). (C) Action potentials were elicited by 4 ms, 150 pA current. Representative action potential waveforms for β-cells treated without or with telmisartan (10 μM) and summary of the mean APDs (n = 7 cells). (D) Representative Kv current traces recorded under treatment of 100 nM Guangxitoxin-1E (GxTX-1E) alone or in combination with 10 μM telmisartan (Tel). (E) Current-voltage relationship curves of Kv channels and summary of the mean current density of Kv channels recorded at 80 mV depolarization (n = 7 cells). The cells compared between the groups in each graph are collected from the same animal. Statistical differences between two groups were determined using an unpaired two-tailed Student’s t test. For comparing the effects of GxTX-1E groups, Tukey Test post hoc analysis was applied. ***p < 0.001, *p < 0.05.
Kv channels participate in the repolarization of action potentials of β-cells, so that inhibition of Kv channels delays the repolarization, thus prolonging the APD, namely extending the duration of extracellular Ca2+ influx (MacDonald and Wheeler, 2003; Herrington et al., 2006; Jacobson and Philipson, 2007). Therefore, we next recorded the action potentials in current-clamp mode to observe the effect of telmisartan on APD. As presented in Figure 5C, comparison of APDs with or without telmisartan indicated that telmisartan extended APD.
As the Kv2.1 channel constitutes the main subtype among Kv families involved in the regulation of insulin release by β-cells (MacDonald et al., 2001; Jacobson et al., 2007; Li et al., 2013), we applied specific Kv2.1 channel blocker Guangxitoxin-1E (GxTX-1E) in patch-clamp experiments. As shown in Figures 5D,E, Kv currents had extremely reduced amplitudes when compared to the controls, moreover, telmisartan did not further significantly inhibit Kv current in the presence of GxTX-1E. Therefore, our results suggested that the inhibition of Kv2.1 was mainly responsible for the effect of telmisartan on Kv channels in β-cell.
Telmisartan Directly Inhibits Kv2.1 Channels, Independent of AT1 Receptor and PPARγ
We evaluated the effects of valsartan and irbesartan in the voltage-clamp experiment. Neither valsartan nor irbesartan exhibited similar effects on Kv channels as those observed with telmisartan treatment (Figures 6A,B). Moreover, GW9662 addition did not influence the telmisartan-induced inhibition of Kv channels (Figures 6C,D). The results indicated that telmisartan inhibited Kv channels independent of the AT1 receptor and PPARγ.
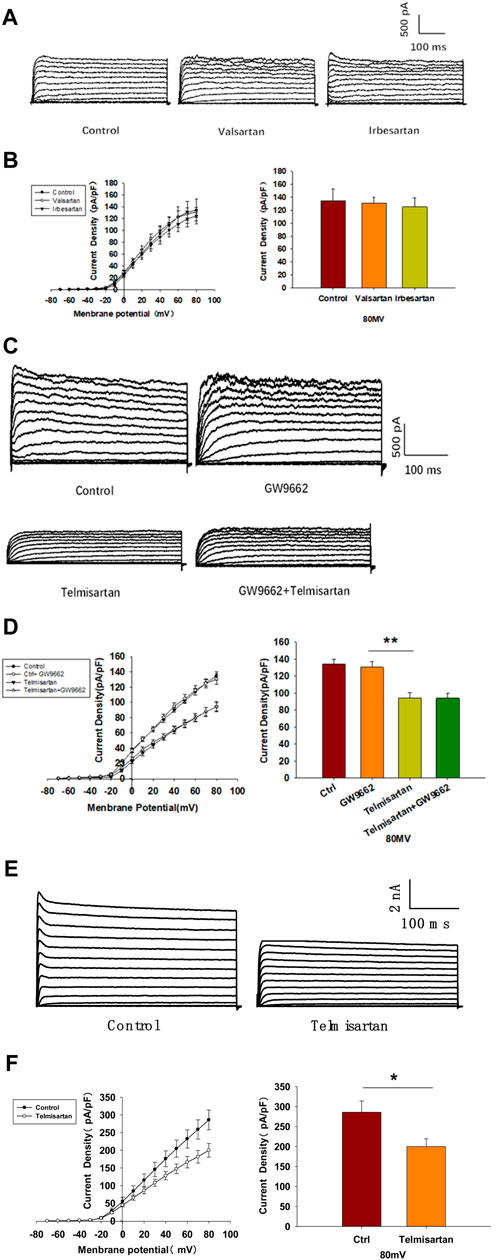
FIGURE 6. The AT-1 receptor and PPARγ are not involved in the telmisartan-induced inhibition of Kv channels, whereas telmisartan exerts a direct effect on Kv2.1 channels. (A) Representative current traces recorded upon treatment with valsartan (10 μM) and irbesartan (10 μM) in β-cells. (B) Current-voltage relationship curves and the summary of the mean current density of Kv channels recorded at 80 mV depolarization (control n = 7 cells, valsartan n = 8 cells, irbesartan n = 6 cells). (C) Representative current traces recorded under treatment of telmisartan (10 μM) alone or in combination with GW9662 (10 μM) in β-cells. (D) Current-voltage relationship curves and the summary of the mean current density of Kv channels recorded at 80 mV depolarization (control n = 8 cells, GW9662 n = 12 cells, telmisartan n = 7 cells, telmisartan+GW9662 n = 10 cells). (E) The CHO-Kv2.1 cell line was constructed using a lentivirus vector overexpressing Kv2.1 channels. Representative current traces recorded without or with telmisartan (10 μM) in CHO-Kv2.1 cells. (F) Current-voltage relationship curves and the summary of the mean current density of Kv channels recorded at 80 mV depolarization (control n = 10 cells, telmisartan n = 8 cells). The cells compared between the groups in each graph are isolated from the same animal except CHO cells. All results are reported as the means ± SEM. Statistical differences between two groups were determined using an unpaired two-tailed Student’s t test. Statistical differences among three or more groups were compared using one-way ANOVA. For comparing the effects of GW9662 groups, Tukey Test post hoc analysis was applied. *p < 0.05, **p < 0.01.
We therefore hypothesized that telmisartan might directly inhibit Kv channels. Considering the importance of Kv2.1 channels in β-cells, we carried out patch-clamp experiments to determine whether telmisartan directly inhibited Kv2.1 channels. Chinese hamster ovary (CHO) cells, which do not express any endogenous Kv channels (Yu and Kerchner, 1998), were utilized to establish the Kv2.1-overexpressing CHO-Kv2.1 cell line. Under whole-cell voltage-clamp mode, Kv2.1 channel currents and their suppression by telmisartan were both detected in CHO-Kv2.1 cells (Figures 6E,F), suggesting that telmisartan exerted direct inhibition on Kv2.1 channels.
Telmisartan Activates L-type VGCCs Independent of the AT1 Receptor and PPARγ
To further confirm whether Kv channels are alone involved in mediating telmisartan-induced insulin secretion and increase of [Ca2+]i levels, tetraethylammonium chloride (TEA), a potent inhibitor of Kv channels, was employed in pancreatic β-cells. Previous studies have shown that 20 mM TEA blocks the majority of Kv channels and causes calcium elevation (Roe et al., 1996; MacDonald et al., 2001). As shown in Figure 7A, TEA stimulated insulin secretion under 11.1 mM glucose conditions and telmisartan still significantly promoted insulin secretion in the presence of TEA, indicating that other factors may participate in telmisartan-stimulated insulin secretion. Consistent with this results, telmisartan also enhanced the [Ca2+]i concentration in the presence of TEA under 11.1 mM glucose conditions (Figure 7B).
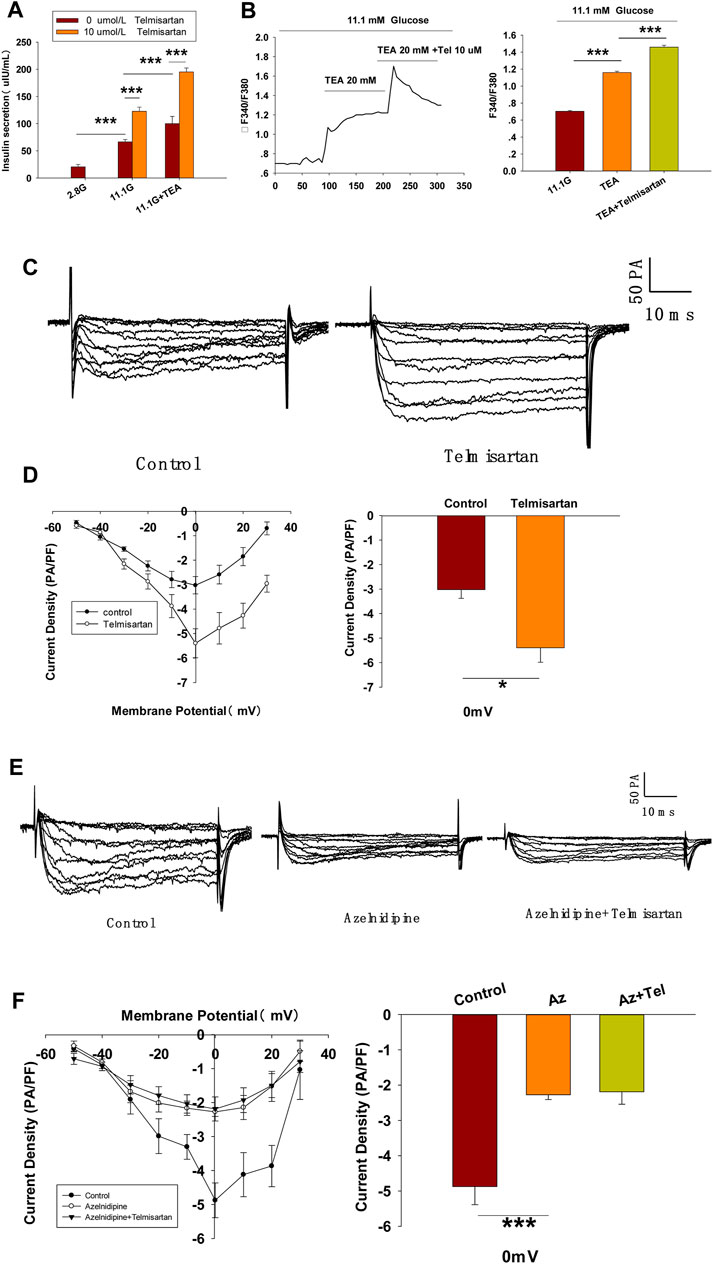
FIGURE 7. L-type VGCCs also mediate telmisartan-induced insulin secretion and increase of [Ca2+]i levels, independent of the AT-1 receptor and PPARγ. (A) Rat islets were treated with telmisartan (10 μM) in the presence or absence of TEA (20 mM) under 2.8 and 11.1 mM glucose conditions and insulin secretion was measured (n = 7 tubes of islet). All insulin secretion results are normalized to basal secretion at 2.8 Mm glucose condition. (B) The changes of ([Ca2+]i) concentration in β-cells treated with 20 mM TEA and in combination with 10 μM telmisartan (Tel) under 11.1 mM glucose conditions, and the average value during 30 s F340/F380 spikes for each test (n = 9 cells). (C) VGCCs were recorded in voltage-clamp mode with test potentials from −50 to 30 mV in 10 mV increments. Representative current traces recorded in control and telmisartan-treated (10 μM) β-cells. (D) Current-voltage relationship curves of VGCCs and summary of the mean Ca2+current density recorded at 0 mV(Continued)
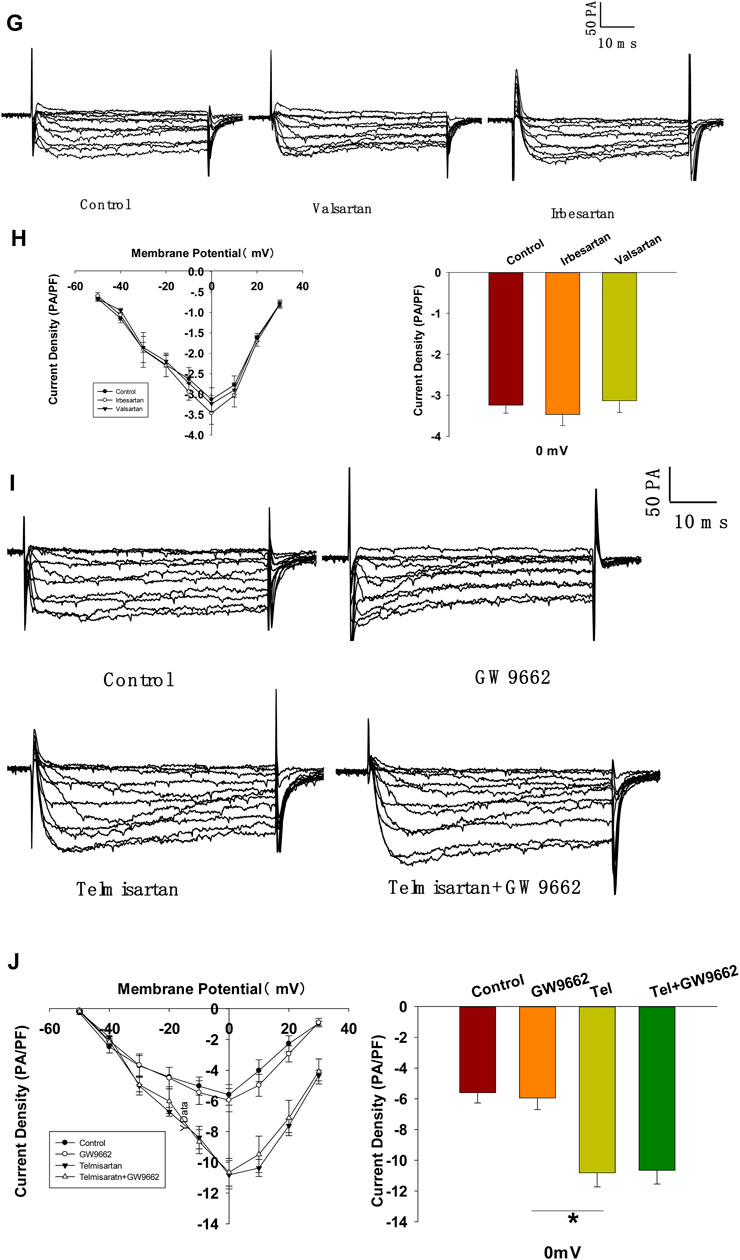
FIGURE 7. depolarization (control, n = 7 cells; telmisartan, n = 8 cells). (E) Representative VGCC current traces recorded under treatment of 0.1 μM azelnidipine (Az) alone or in combination with 10 μM telmisartan (Tel). (F) Current-voltage relationship curves of VGCC and summary of the mean current density of Kv channels recorded at 0 mV depolarization (control, n = 6 cells; Az, n = 7 cells; Az+Tel, n = 6 cells). (G) Representative current traces recorded with treatment of valsartan (10 μM) and irbesartan (10 μM) in β-cells. (H) Current-voltage relationship curves and the summary of the mean Ca2+current density recorded at 0 mV depolarization (n = 7 cells). (I) Representative current trances recorded under treatment of telmisartan (10 μM) alone or in combination with GW9662 (10 μM) in β-cells. (J) Current-voltage relationship curves and the summary of the mean Ca2+ current density recorded at 0 mV depolarization (control, n = 10 cells; GW9662, n = 6; telmisartan, n = 8 cells; telmisartan+GW9662, n = 6 cells). The cells compared between the groups in each graph are isolated from the same animal. All results are reported as the means ± SEM. Statistical differences between two groups were determined using an unpaired two-tailed Student’s t test. Statistical differences among three or more groups were compared using one-way ANOVA and Student–Newman–Keuls method post hoc analysis. Effects on VGCCs between telmisartan and control in (D) were compared using the Mann–Whitney Rank Sum Test. For comparing the effects of GW9662 groups in (J), Dunn’s method post hoc analysis was applied. *p < 0.05, ***p < 0.001.
Since telmisartan-stimulated insulin secretion was Ca2+ dependent, we performed patch-clamp experiments to observe the effects of telmisartan on VGCCs in pancreatic β-cells. As presented in Figures 7C,D, telmisartan increased voltage-dependent inward Ca2+ currents densities compared with those of controls. As telmisartan enhances extracellular calcium influx through L-type VGCCs, we further observed the effect of telmisartan on L-type VGCCs using azelnidipine. The results showed that the activation of VGCCs by telmisartan was blocked in the presence of azelnidipine (Figures 7E,F). In addition, no significant difference was observed when VGCC currents were recorded following treatment with valsartan or irbesartan (Figures 7G,H); telmisartan-induced activation of VGCCs was not inhibited by GW9662 co-administration (Figures 7I,J). The results thus demonstrated that telmisartan activated L-type VGCCs of β-cells; moreover, neither the AT1 receptor nor the PPARγ mediated this effect.
Telmisartan Ameliorates Hyperglycemia by Increasing Insulin Secretion in vivo and Amplifies GSIS in vitro in Db/Db Mice
We applied db/db mice as T2DM model mice to determine whether telmisartan induced hypoglycemic effects in vivo. Male mice were administered with telmisartan (15 mg/kg) or vehicle once by gavage at the age of 8 and 11 wk, then OGTT was performed to observe the effects of telmisartan on glucose response.
In 8-wk-old mice, blood glucose levels monitoring revealed that glucose clearance at 30 min and thereafter was improved significantly in telmisartan-treated mice, and noticeable difference was observed when the glycemic response was measured via the area under the curve (AUC) compared with that of control (Figure 8A). However, although the time of peak blood glucose was similarly advanced to 15 min in 11-wk-old mice, and the AUC results showed significant difference between the groups, it was not until 90 and 120 min (approximately 1 h later than in 8-wk-old mice) that the blood glucose values were markedly lower than those of controls (Figure 8B). Furthermore, the levels of plasma insulin in the telmisartan-treated group were considerably higher than those in the control group at 15, 30, and 60 min with the AUC differing significantly between the groups (Figure 8C). Therefore, the glucose-lowering effect of telmisartan was accompanied by the increase in the levels of plasma insulin, suggesting that the hypoglycemic effects of telmisartan were a result of increased insulin secretion. We speculated that the glucose-lowering effect of telmisartan was delayed and weakened in 11-wk-old mice, possibly owing to the progression of insulin resistance and the deterioration of β-cell function in db/db mice. In addition, our data in vivo were not completely consistent with the previous results of telmisartan in regard to acute regulation of pancreatic islet microcirculation and glycaemia (Olverling et al., 2013). The reasons for this discrepancy might conceivably relate to differences in concentrations of drug administration in experiments, and different impaired β-cell function state caused by species and age of the animal model.
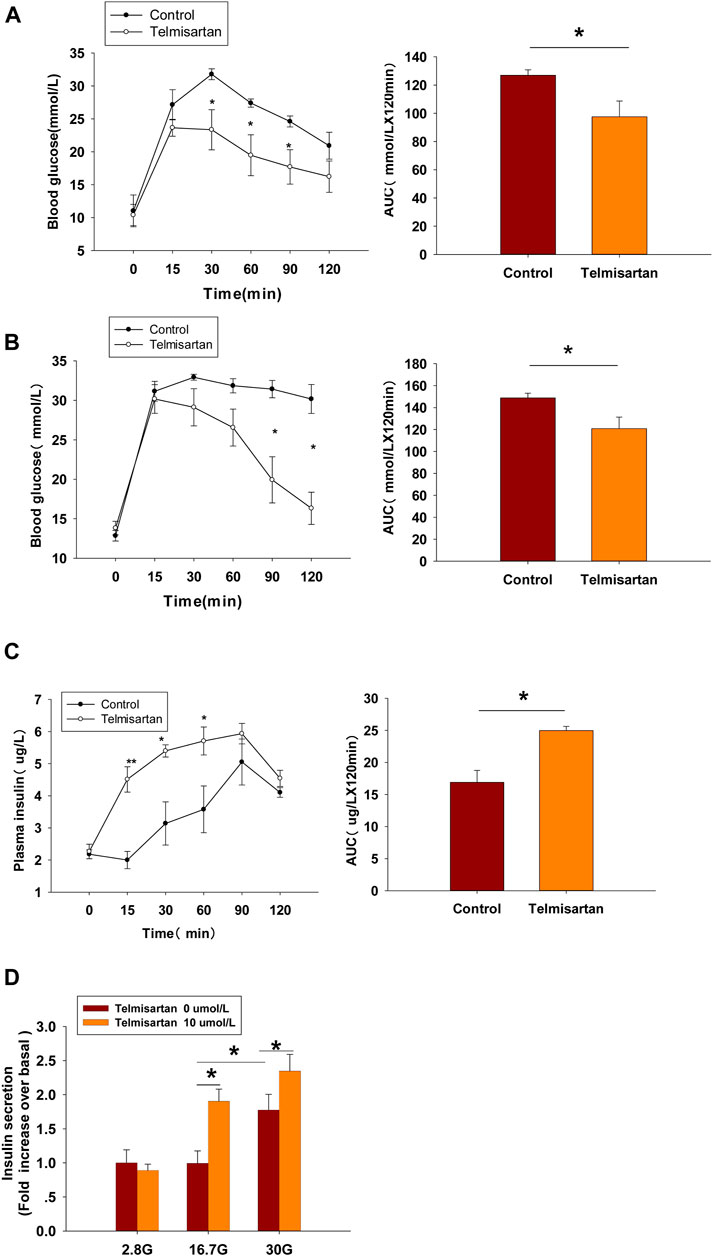
FIGURE 8. Telmisartan improves glucose tolerance in db/db mice, elevates GSIS levels in isolated islets, and exerts similar electrophysiological effects on β-cells of db/db mice. (A) OGTT was performed and AUCs for OGTT were calculated from the data in 8-wk-old mice. (B) OGTT and AUC for OGTT in 11-wk-old mice. (C) Serum insulin levels at corresponding times and AUC in 11-wk-old mice. (D) Db/db mice islets were treated with or without telmisartan (10 μM) under different glucose concentrations (2.8, 16.7, and 30 mM) (n = 6 tubes of islet). (E) Representative Kv channels current trances recorded with treatment of telmisartan (10 μM) in β-cells. (F) Current-voltage relationship curves and the summary of the mean current density of Kv channels recorded at 80 mV depolarization (n = 6 cells). (G) Representative Ca2+ current trances recorded with treatment of telmisartan (10 μM) in β-cells. (H) Current-voltage relationship curves and the summary of the mean Ca2+(Continued)
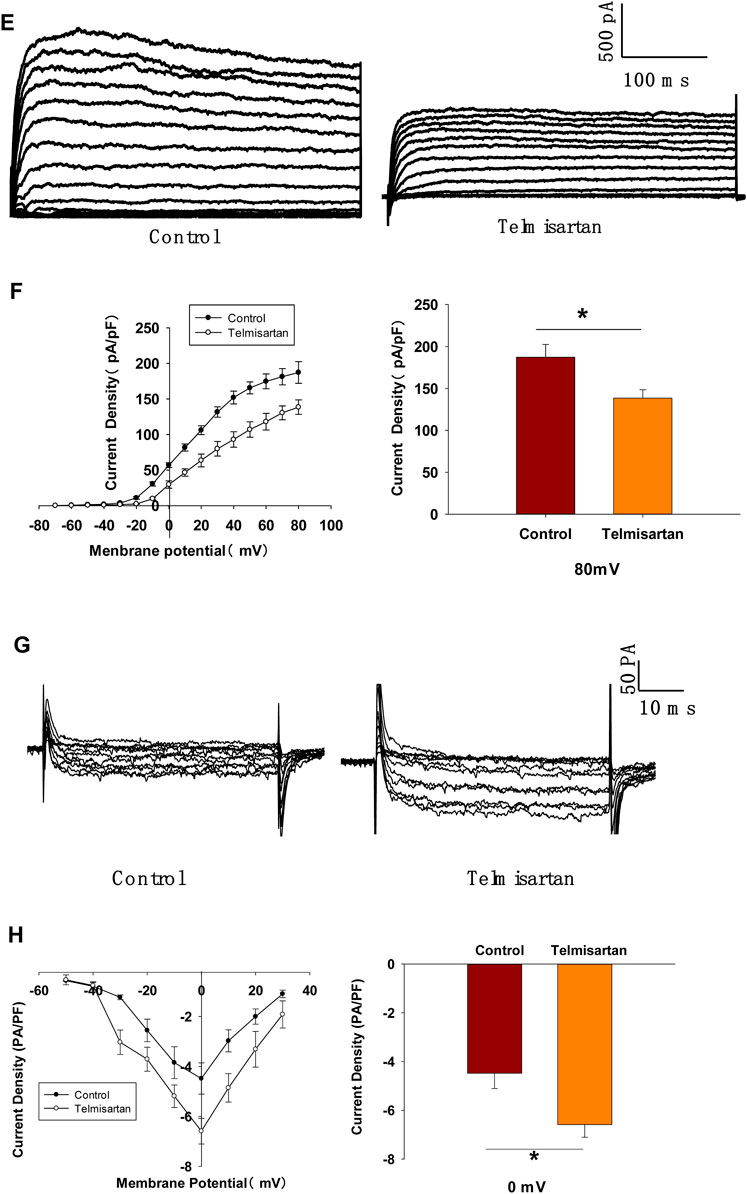
FIGURE 8. current density recorded at 0 mV depolarization (control, n = 6 cells; telmisartan, n = 7 cells). In (D), all the islets were isolated from the same mouse, and the insulin secretion results are normalized to basal secretion at 2.8 Mm glucose concentration. In (E,G), the cells compared between the groups in each graph are collected from the same animal. All results are reported as the means ± SEM. Statistical differences between two groups were determined using the unpaired two-tailed Student’s t test unless otherwise stated. Glucose levels in 11-wk-old mice at indicated time points were compared using the Mann–Whitney Rank Sum Test except at 0 min. AUCs calculated from the data of glucose levels and plasma insulin levels in 11-wk-old mice were compared using the Mann–Whitney Rank Sum Test. As for the insulin assay in (D), statistical differences among three groups (without telmisartan) were compared using one-way ANOVA and followed by Student–Newman–Keuls method post hoc analysis, and differences between two groups under the same glucose conditions were compared using the paired t test. *p < 0.05.
At the end of the experiment, islets were isolated from db/db mice and used for an ex vivo study. The results showed that telmisartan potentiated insulin secretion under 16.7 and 30 mM glucose conditions (Figure 8D). Consistent with the results of in vivo studies, telmisartan treatment similarly enhanced GSIS under the pathological condition of diabetes. However, GSIS in cultured islets only occurred under 30 mM glucose conditions whereas 2.8 and 16.7 mM glucose showed equivalent secretion. The bluntness of GSIS might be related to impaired β-cell function caused by long-term exposure to high glucose and lipids in the development of diabetes in the db/db mice (Olofsson et al., 2007).
In T2DM, high glucose and free fatty acids leads to adverse effects (including blunted GSIS and decreased cell viability) (Olofsson et al., 2007; Tan et al., 2013), with the modulation of Kv and voltage-gated Ca2+ channels by “glucolipotoxicity” also being involved (Hoppa et al., 2011; Lee et al., 2018). We next performed the patch-clamp experiments to ascertain whether telmisartan exerted similar electrophysiological effects on pathological β-cells. We observed that both the decreased Kv channel currents (Figures 8E,F) and increased VGCC currents (Figures 8G,H) remained in telmisartan-treated β-cells of db/db mice. Therefore, under the pathological condition of T2DM, telmisartan still served the function of an insulin secretagogue through its action on ion channels.
Discussion
ARBs are of critical importance to individuals with both diabetes and hypertension. We therefore carried out the study to better understand the beneficial effects of ARBs for diabetes. Notably, we revealed an insulin secretagogue role for telmisartan, which is not present in other ARBs. In the present study, isolated islets were exposed to telmisartan for only 30 min prior to the insulin secretion assay, and glucose-lowering effects were observed in db/db mice following acute telmisartan administration. The evidences both in vitro and in vivo thus demonstrated the rapid insulinotropic effect of telmisartan. By affecting local pancreatic RAS, ARBs have previously been reported to influence pancreatic islet blood flow to regulate rapidly serum insulin levels in gender-specific rats (Huang et al., 2007, 2008). However, to the best of our knowledge, no prior reports of telmisartan exist with respect to this direct effect on insulin secretion.
Moreover, our results showed that telmisartan, enhances insulin secretion in a glucose-dependent manner. Even at high concentrations (50 μM), no insulinotropic effect of telmisartan was observed under low glucose conditions (2.8 mM) (Figure 1A). This indicated that telmisartan might be applied as an insulin secretagogue without the risk of hypoglycemia. Hypoglycemia is a frequent and severe adverse effect. Not only can apparent hypoglycemia cause coma or the disruption of daily life, but unrecognized, recurrent hypoglycemia can also lead to life-threatening cardiac complications such as arrhythmias and myocardial ischemia, and cause permanent cognitive impairment that may accelerate the onset of dementia (Frier, 2014). In addition, emerging evidence suggests that forcing the β-cells to secrete insulin constantly, termed insulin hypersecretion, might have the potential to accelerate the decline in β-cell function and thus may constitute a contributing factor to the progression of T2DM (Aston-Mourney et al., 2008; Rustenbeck et al., 2010). Therefore, glucose-independent insulinotropic agents have exhibited poor durability in maintaining long-term glycemic control (Kahn et al., 2006). In comparison, our study showed that telmisartan increased insulin secretion in a manner proportional to the accumulating glucose concentration, thereby avoiding the risk of overstimulating the β-cells.
By means of its function as both an ARB and a partial agonist for PPAR-γ, telmisartan provides numerous beneficial effects in ameliorating hyperglycemia and related complications in T2DM (Nagel et al., 2006; Goyal et al., 2008; Makino et al., 2008; Yamana et al., 2008; Hasegawa et al., 2009; Saitoh et al., 2009; Perl et al., 2010; Li et al., 2012). However, our results demonstrated that telmisartan also functioned rapidly as an insulin secretagogue, consequent to its unique electrophysiological effects on ion channels, which were independent of the AT1 receptor and PPARγ.
Glucose-induced insulin secretion and increase of [Ca2+]i are tightly controlled by ion channels that regulate cell membrane potential. The closure of KATP channels caused by high glucose results in membrane depolarization and opening of Kv channels and VGCCs (Kalwat and Cobb, 2017; Sabatini et al., 2019). Kv channels mediate repolarization of β-cells, and antagonize the Ca2+ influx induced by VGCC activation. Blockade of Kv channels therefore prolongs action potential duration, leading to an increase of insulin secretion. In support of this notion, here we found that inhibition of Kv channels was linked to telmisartan-induced augmentation of GSIS.
Moreover, we identified that telmisartan directly inhibited Kv2.1 channel. The Kv2.1 channel, as a Kv family member, accounts for the majority of Kv currents on β-cells, serving to not only negatively regulate GSIS but also potentiate β-cell apoptosis (Kim et al., 2012; Tingting et al., 2018). And Kv2.1 null mice exhibit reduced fasting blood glucose levels and elevated serum insulin levels (Jacobson et al., 2007). Previous studies attributed telmisartan-induced protective effects against β-cells apoptosis and dysfunction to its action on the AT1 receptor and PPARγ (Hasegawa et al., 2009; Saitoh et al., 2009; Li et al., 2012; Wang et al., 2019), however, our results indicated that the inhibition of Kv2.1 might also be involved. Moreover, based on its dual effects including regulation of insulin secretion and β-cell apoptosis, Kv2.1 is considered as a promising therapeutic target for T2DM by most researchers in the field. However, despite the occasional reports of small molecule Kv2.1 inhibitor (Zhou et al., 2016; Tingting et al., 2018), no specific drugs have been developed for therapeutic use. Alternatively, as drug repurposing has become a successful approach to accelerate novel anti-diabetic drug development (Turner et al., 2016), our favorable finding provides insight with regard to new options for anti-diabetic drug discovery. Furthermore, as Kv2.1 also serves as the key channel during neuronal apoptosis and its cleavage inhibits neuronal apoptosis (Yao et al., 2009; Liu et al., 2018), the potential neuroprotective role of telmisartan also warrants further investigation.
It should be noted here that there are also other isoforms of Kv channel contributing to the regulation of GSIS in β-cells, such as KCNQ1 (Torekov et al., 2014; Thompson et al., 2020), Kv1 channel family (MacDonald et al., 2001). Accordingly, our data did not exclude the possibility that telmisartan also interacts with other Kv channel isoforms.
Of note, although the potent Kv channel inhibitor TEA blocks the majority of Kv channels, we found that telmisartan showed additional potentiation on insulin secretion and [Ca2+]i concentration in the presence of TEA. Indeed, our findings revealed that in addition to Kv channels, L-type VGCCs mediated the effects of telmisartan, which were also independent of the AT1 receptor and PPARγ. The possible channel isoforms and signaling pathway related to the activation of L-type VGCC by telmisartan require further study. Interestingly, L-type VGCCs play dominant roles in the pathogenesis of hypertension (Sven et al., 2003; Pesic et al., 2004; Barrett et al., 2016; Tamargo and Ruilope, 2016), and our results seemed to contradict the antihypertensive role of telmisartan. We hypothesized that it might be due to the overactivation of RAS on hypertension condition, when the effect of telmisartan in blocking AT1 receptor was dominant. However, it would be worth investigating whether the action of telmisartan on L-type VGCC influenced its antihypertensive effects.
Moreover, we concluded that KATP channels were unlikely to be involved in telmisartan-regulated insulin secretion. Specifically, the insulinotropic effect of inhibition of KATP channels is glucose-independent (Dukes et al., 1994; Henquin, 2011), whereas telmisartan did not enhance insulin secretion under low glucose (2.8 mM) conditions (Figures 1A,D). Conversely, the KATP antagonist tolbutamide increased [Ca2+]i concentrations in β-cells under low glucose conditions (Figures 2A,B), suggesting that telmisartan and tolbutamide act on separate targets.
The present study is not without limitations. Our results have been obtained using rodents such as Wistar rats or db/db mice. In terms of ion channels, including Kv channels and VGCC, human β-cells differ from rodent cells in channel isoforms and electrophysiological activity (Rorsman and Ashcroft, 2018). Some ion channels (large-conductance Ca2+ activated K+ channels, P/Q Ca2+ channels) which are considered less important in rodent β-cells play a more prominent role in human β-cells (Braun et al., 2008; Fridlyand et al., 2013). Therefore, whether the effect of telmisartan may be replicated in human remains to be determined.
In summary, our results showed that beyond AT1 receptor blockade or PPARγ activation, telmisartan also inhibits Kv channels and activates L-type VGCCs to promote extracellular Ca2+ influx, thereby enhancing [Ca2+]i levels and amplifying GSIS. Our findings provide a new understanding of an anti-diabetes mechanism for telmisartan that is distinct from that of other ARBs, and may have important implications for determination of the choice of ARBs for the treatment of patients with both hypertension and diabetes. In addition, our identification of telmisartan also acting as a Kv2.1 inhibitor and glucose-dependent insulinotropic agent, provides a foundation for the development of new anti-diabetic drugs.
Data Availability Statement
The original contributions presented in the study are included in the article/Supplementary Material, further inquiries can be directed to the corresponding authors.
Ethics Statement
The animal study was reviewed and approved by Shanxi Medical University, and were approved by the Animal Care and Use Committee of Shanxi Medical University (Taiyuan, China).
Author Contributions
YZ, YL, and TL conceived and designed the study; TL performed the ex vivo experiments with assistance from LC, HX, XY, ML, ZL, and QG; TL, LC, HY, and LZ carried out the in vivo experiments; TL, MZ, and PH analyzed the data. TL, YZ, and YL wrote the manuscript.
Funding
This work was supported by NSFC (81670710; 81770776; 81973378 and 82073909), 136 project in Shanxi BethuneHospital (2019XY015), Cultivate Scientific Research Excellence Programs of Higher Education Institutions in Shanxi (2019KJ022), FSKSC and 1331KSC, Department of Education Innovation Project in Shanxi Province (2019BY078), Shanxi Youth Science and Technology Research Fund (201901D211323), and Shanxi Scholarship Council of China (2020-172).
Conflict of Interest
The authors declare that the research was conducted in the absence of any commercial or financial relationships that could be construed as a potential conflict of interest.
Publisher’s Note
All claims expressed in this article are solely those of the authors and do not necessarily represent those of their affiliated organizations, or those of the publisher, the editors and the reviewers. Any product that may be evaluated in this article, or claim that may be made by its manufacturer, is not guaranteed or endorsed by the publisher.
Supplementary Material
The Supplementary Material for this article can be found online at: https://www.frontiersin.org/articles/10.3389/fphar.2021.739637/full#supplementary-material
References
American Diabetes Association (2015). Standards of Medical Care in Diabetes-2015 Abridged for Primary Care Providers. Clin. Diabetes 33, 97–111. doi:10.2337/diaclin.33.2.97
Aston-Mourney, K., Proietto, J., Morahan, G., and Andrikopoulos, S. (2008). Too Much of a Good Thing: Why it Is Bad to Stimulate the Beta Cell to Secrete Insulin. Diabetologia 51, 540–545. doi:10.1007/s00125-008-0930-2
Barrett, P. Q., Guagliardo, N. A., Klein, P. M., Hu, C., Breault, D. T., and Beenhakker, M. P. (2016). Role of Voltage-Gated Calcium Channels in the Regulation of Aldosterone Production from Zona Glomerulosa Cells of the Adrenal Cortex. J. Physiol. 594, 5851–5860. doi:10.1113/JP271896
Braun, M., Ramracheya, R., Bengtsson, M., Zhang, Q., Karanauskaite, J., Partridge, C., et al. (2008). Voltage-gated Ion Channels in Human Pancreatic Beta-Cells: Electrophysiological Characterization and Role in Insulin Secretion. Diabetes 57, 1618–1628. doi:10.2337/db07-0991
Candido, R., Allen, T. J., Lassila, M., Cao, Z., Thallas, V., Cooper, M. E., et al. (2004). Irbesartan but Not Amlodipine Suppresses Diabetes-Associated Atherosclerosis. Circulation 109, 1536–1542. doi:10.1161/01.CIR.0000124061.78478.94
Deedwania, P. C. (2004). Diabetes and Hypertension, the Deadly Duet: Importance, Therapeutic Strategy, and Selection of Drug Therapy. Cardiol. Clin. 23, 139–152. doi:10.1016/j.ccl.2004.06.006
Dukes, I. D., McIntyre, M. S., Mertz, R. J., Philipson, L. H., Roe, M. W., Spencer, B., et al. (1994). Dependence on NADH Produced During Glycolysis for Beta-Cell Glucose Signaling. J. Biol. Chem. 269, 10979–10982. doi:10.1016/s0021-9258(19)78078-8
Ferrannini, E., and Cushman, W. C. (2012). Diabetes and Hypertension: The Bad Companions. Lancet 380, 601–610. doi:10.1016/S0140-6736(12)60987-8
Fox, C. S., Golden, S. H., Anderson, C., Bray, G. A., Burke, L. E., de Boer, I. H., et al. (2015). Update on Prevention of Cardiovascular Disease in Adults with Type 2 Diabetes Mellitus in Light of Recent Evidence: A Scientific Statement from the American Heart Association and the American Diabetes Association. Diabetes Care 38, 1777–1803. doi:10.1161/CIR.000000000000023010.2337/dci15-0012
Fridlyand, L. E., Jacobson, D. A., and Philipson, L. H. (2013). Ion Channels and Regulation of Insulin Secretion in Human β-cells: A Computational Systems Analysis. Islets 5, 1–15. doi:10.4161/isl.24166
Frier, B. M. (2014). Hypoglycaemia in Diabetes Mellitus: Epidemiology and Clinical Implications. Nat. Rev. Endocrinol. 10, 711–722. doi:10.1038/nrendo.2014.170
Gohlke, P., Weiss, S., Jansen, A., Wienen, W., Stangier, J., Rascher, W., et al. (2001). AT1 Receptor Antagonist Telmisartan Administered Peripherally Inhibits Central Responses to Angiotensin II in Conscious Rats. J. Pharmacol. Exp. Ther. 298, 62–70.
Göpel, S., Kanno, T., Barg, S., Galvanovskis, J., and Rorsman, P. (1999). Voltage-gated and Resting Membrane Currents Recorded from B-Cells in Intact Mouse Pancreatic Islets. J. Physiol. 521 Pt 3 (Pt 3), 717–728. doi:10.1111/j.1469-7793.1999.00717.x
Goyal, B. R., Mesariya, P., Goyal, R. K., and Mehta, A. A. (2008). Effect of Telmisartan on Cardiovascular Complications Associated with Streptozotocin Diabetic Rats. Mol. Cel. Biochem. 314, 123–131. doi:10.1007/s11010-008-9772-y
Gress, T. W., Nieto, F. J., Shahar, E., Wofford, M. R. R., and Brancati, F. L. L. (2000). Hypertension and Antihypertensive Therapy as Risk Factors for Type 2 Diabetes Mellitus. Atherosclerosis Risk in Communities Study. N. Engl. J. Med. 342, 905–912. doi:10.1056/NEJM200003303421301
Hasegawa, G., Fukui, M., Hosoda, H., Asano, M., Harusato, I., Tanaka, M., et al. (2009). Telmisartan, an Angiotensin II Type 1 Receptor Blocker, Prevents the Development of Diabetes in Male Spontaneously Diabetic Torii Rats. Eur. J. Pharmacol. 605, 164–169. doi:10.1016/j.ejphar.2009.01.001
Henquin, J. C. (2011). The Dual Control of Insulin Secretion by Glucose Involves Triggering and Amplifying Pathways in β-cells. Diabetes Res. Clin. Pract. 93 Suppl 1 (Suppl. 1), S27–S31. doi:10.1016/S0168-8227(11)70010-9
Herrington, J., Zhou, Y. P., Bugianesi, R. M., Dulski, P. M., Feng, Y., Warren, V. A., et al. (2006). Blockers of the Delayed-Rectifier Potassium Current in Pancreatic Beta-Cells Enhance Glucose-dependent Insulin Secretion. Diabetes 55, 1034–1042. doi:10.2337/diabetes.55.04.06.db05-0788
Hoppa, M. B., Collins, S., Ramracheya, R., Hodson, L., Amisten, S., Zhang, Q., et al. (2011). Chronic Palmitate Exposure Inhibits Insulin Secretion by Dissociation of Ca2+ Channels from Secretory Granules. Cell Metab 13, 487. doi:10.1016/j.cmet.2011.03.002
Huang, Z., Jansson, L., and Sjöholm, A. (2008). Gender-specific Regulation of Pancreatic Islet Blood Flow, Insulin Levels and Glycaemia in Spontaneously Diabetic Goto-Kakizaki Rats. Clin. Sci. (Lond) 115, 35–42. doi:10.1042/CS20070386
Huang, Z., Jansson, L., and Sjöholm, A. (2007). Vasoactive Drugs Enhance Pancreatic Islet Blood Flow, Augment Insulin Secretion and Improve Glucose Tolerance in Female Rats. Clin. Sci. (Lond) 112, 69–76. doi:10.1042/CS20060176
Hunyady, L., and Catt, K. J. (2006). Pleiotropic AT1 Receptor Signaling Pathways Mediating Physiological and Pathogenic Actions of Angiotensin II. Mol. Endocrinol. 20, 953–970. doi:10.1210/me.2004-0536
Jacobson, D. A., Kuznetsov, A., Lopez, J. P., Kash, S., Ammälä, C. E., and Philipson, L. H. (2007). Kv2.1 Ablation Alters Glucose-Induced Islet Electrical Activity, Enhancing Insulin Secretion. Cel Metab 6, 229–235. doi:10.1016/j.cmet.2007.07.010
Jacobson, D. A., and Philipson, L. H. (2007). Action Potentials and Insulin Secretion: New Insights into the Role of Kv Channels. Diabetes Obes. Metab. 9 Suppl 2 (Suppl. 2), 89–98. doi:10.1111/j.1463-1326.2007.00784.x
Kahn, S. E., Haffner, S. M., Heise, M. A., Herman, W. H., Holman, R. R., Jones, N. P., et al. (2006). Glycemic Durability of Rosiglitazone, Metformin, or Glyburide Monotherapy. N. Engl. J. Med. 355, 2427–2443. doi:10.1056/NEJMoa066224
Kalwat, M. A., and Cobb, M. H. (2017). Mechanisms of the Amplifying Pathway of Insulin Secretion in the β Cell. Pharmacol. Ther. 179, 17–30. doi:10.1016/j.pharmthera.2017.05.003
Kim, S. J., Widenmaier, S. B., Choi, W. S., Nian, C., Ao, Z., Warnock, G., et al. (2012). Pancreatic β-cell Prosurvival Effects of the Incretin Hormones Involve Post-translational Modification of Kv2.1 Delayed Rectifier Channels. Cell Death Differ 19, 333–344. doi:10.1038/cdd.2011.102
Kjeldsen, S. E., Julius, S., Mancia, G., McInnes, G. T., Hua, T., Weber, M. A., et al. (2006). Effects of Valsartan Compared to Amlodipine on Preventing Type 2 Diabetes in High-Risk Hypertensive Patients: the VALUE Trial. J. Hypertens. 24, 1405–1412. doi:10.1097/01.hjh.0000234122.55895.5b
Lee, C. H., Chu, C. S., Tsai, H. J., Ke, L. Y., Lee, H. C., Yeh, J. L., et al. (2018). Xanthine-derived KMUP-1 Reverses Glucotoxicity-Activated Kv Channels Through the cAMP/PKA Signaling Pathway in Rat Pancreatic β Cells. Chem. Biol. Interact. 279, 171–176. doi:10.1016/j.cbi.2017.11.017
Levy, J., Atkinson, A. B., Bell, P. M., McCance, D. R., and Hadden, D. R. (1998). Beta-cell Deterioration Determines the Onset and Rate of Progression of Secondary Dietary Failure in Type 2 Diabetes Mellitus: The 10-year Follow-Up of the Belfast Diet Study. Diabet. Med. 15, 290–296. doi:10.1002/(SICI)1096-9136(199804)15:4<290::AID-DIA570>3.0.CO;2-M
Li, X., Yuan, L., Li, J., Li, H., and Cheng, S. (2012). Blockade of Renin Angiotensin System Increased Resistance to STZ-Induced Diabetes in Rats with Long-Term High-Fat Diet. Exp. Diabetes Res. 2012, 618923. doi:10.1155/2012/618923
Li, X. N., Herrington, J., Petrov, A., Ge, L., Eiermann, G., Xiong, Y., et al. (2013). The Role of Voltage-Gated Potassium Channels Kv2.1 and Kv2.2 in the Regulation of Insulin and Somatostatin Release from Pancreatic Islets. J. Pharmacol. Exp. Ther. 344, 407–416. doi:10.1124/jpet.112.199083
Liu, F., Zhang, Y., Liang, Z., Sun, Q., Liu, H., Zhao, J., et al. (2018). Cleavage of Potassium Channel Kv2.1 by BACE2 Reduces Neuronal Apoptosis. Mol. Psychiatry 23, 1542–1554. doi:10.1038/s41380-018-0060-2
MacDonald, P. E., Ha, X. F. F., Wang, J., Smukler, S. R., Sun, A. M. M., Gaisano, H. Y., et al. (2001). Members of the Kv1 and Kv2 Voltage-dependent K(+) Channel Families Regulate Insulin Secretion. Mol. Endocrinol. 15, 1423–1435. doi:10.1210/mend.15.8.0685
MacDonald, P. E., and Wheeler, M. B. (2003). Voltage-dependent K(+) Channels in Pancreatic Beta Cells: Role, Regulation and Potential as Therapeutic Targets. Diabetologia 46, 1046–1062. doi:10.1007/s00125-003-1159-8
Makino, H., Haneda, M., Babazono, T., Moriya, T., Ito, S., Iwamoto, Y., et al. (2008). Microalbuminuria Reduction with Telmisartan in Normotensive and Hypertensive Japanese Patients with Type 2 Diabetes: A Post-hoc Analysis of the Incipient to Overt: Angiotensin II Blocker, Telmisartan, Investigation on Type 2 Diabetic Nephropathy (INNOVATION) Study. Hypertens. Res. 31, 657–664. doi:10.1291/hypres.31.657
McMurray, J. J., McMurray, J. J., Holman, R. R., Haffner, S. M., Bethel, M. A., Holzhauer, B., et al. (2010). Effect of Valsartan on the Incidence of Diabetes and Cardiovascular Events. N. Engl. J. Med. 362, 1477–1490. doi:10.1056/NEJMoa1001121
Michel, M. C., Foster, C., Brunner, H. R., and Liu, L. (2013). A Systematic Comparison of the Properties of Clinically Used Angiotensin II Type 1 Receptor Antagonists. Pharmacol. Rev. 65, 809–848. doi:10.1124/pr.112.007278
Nagel, J. M., Tietz, A. B., Göke, B., and Parhofer, K. G. (2006). The Effect of Telmisartan on Glucose and Lipid Metabolism in Nondiabetic, Insulin-Resistant Subjects. Metabolism 55, 1149–1154. doi:10.1016/j.metabol.2006.04.011
Nair, A. B., and Jacob, S. (2016). A Simple Practice Guide for Dose Conversion Between Animals and Human. J. Basic Clin. Pharm. 7, 27–31. doi:10.4103/0976-0105.177703
Olofsson, C. S., Collins, S., Bengtsson, M., Eliasson, L., Salehi, A., Shimomura, K., et al. (2007). Long-term Exposure to Glucose and Lipids Inhibits Glucose-Induced Insulin Secretion Downstream of Granule Fusion with Plasma Membrane. Diabetes 56, 1888–1897. doi:10.2337/db06-1150
Olverling, A., Huang, Z., Nyström, T., and Sjöholm, Å. (2013). Acute Regulation of Pancreatic Islet Microcirculation and Glycaemia by Telmisartan and Ramipril: Discordant Effects Between Normal and Type 2 Diabetic Rats. Clin. Sci. (Lond) 125, 433–438. doi:10.1042/CS20120635
Parving, H. H., Lehnert, H., Bröchner-Mortensen, J., Gomis, R., Andersen, S., Arner, P., et al. (2001). The Effect of Irbesartan on the Development of Diabetic Nephropathy in Patients with Type 2 Diabetes. N. Engl. J. Med. 345, 870–878. doi:10.1056/NEJMoa011489
Perl, S., Schmölzer, I., Sourij, H., Pressl, H., Eder, M., Zweiker, R., et al. (2010). Telmisartan Improves Vascular Function Independently of Metabolic and Antihypertensive Effects in Hypertensive Subjects with Impaired Glucose Tolerance. Int. J. Cardiol. 139, 289–296. doi:10.1016/j.ijcard.2008.10.048
Pesic, A., Madden, J. A., Pesic, M., and Rusch, N. J. (2004). High Blood Pressure Upregulates Arterial L-type Ca2+ Channels: Is Membrane Depolarization the Signal?. Circ. Res. 94, e97–104. doi:10.1161/01.RES.0000131495.93500.3c
Roe, M. W., Worley, J. F., Mittal, A. A., Kuznetsov, A., DasGupta, S., Mertz, R. J., et al. (1996). Expression and Function of Pancreatic Beta-Cell Delayed Rectifier K+ Channels. Role in Stimulus-Secretion Coupling. J. Biol. Chem. 271, 32241–32246. doi:10.1074/jbc.271.50.32241
Rorsman, P., and Ashcroft, F. M. (2018). Pancreatic β-Cell Electrical Activity and Insulin Secretion: Of Mice and Men. Physiol. Rev. 98, 117–214. doi:10.1152/physrev.00008.2017
Rustenbeck, I., Baltrusch, S., and Tiedge, M. (2010). Do insulinotropic Glucose-Lowering Drugs Do More Harm Than Good? The Hypersecretion Hypothesis Revisited. Diabetologia 53, 2105–2111. doi:10.1007/s00125-010-1839-0
Sabatini, P. V., Speckmann, T., and Lynn, F. C. (2019). Friend and Foe: β-cell Ca2+ Signaling and the Development of Diabetes. Mol. Metab. 21, 1–12. doi:10.1016/j.molmet.2018.12.007
Saitoh, Y., Hongwei, W., Ueno, H., Mizuta, M., and Nakazato, M. (2009). Telmisartan Attenuates Fatty-Acid-Induced Oxidative Stress and NAD(P)H Oxidase Activity in Pancreatic Beta-Cells. Diabetes Metab. 35, 392–397. doi:10.1016/j.diabet.2009.04.005
Schupp, M., Clemenz, M., Gineste, R., Witt, H., Janke, J., Helleboid, S., et al. (2005). Molecular Characterization of New Selective Peroxisome Proliferator-Activated Receptor Gamma Modulators with Angiotensin Receptor Blocking Activity. Diabetes 54, 3442–3452. doi:10.2337/diabetes.54.12.3442
Schupp, M., Janke, J., Clasen, R., Unger, T., and Kintscher, U. (2004). Angiotensin Type 1 Receptor Blockers Induce Peroxisome Proliferator-Activated Receptor-Gamma Activity. Circulation 109, 2054–2057. doi:10.1161/01.CIR.0000127955.36250.65
Shiuchi, T., Iwai, M., Li, H. S., Wu, L., Min, L. J., Li, J. M., et al. (2004). Angiotensin II Type-1 Receptor Blocker Valsartan Enhances Insulin Sensitivity in Skeletal Muscles of Diabetic Mice. Hypertension 43, 1003–1010. doi:10.1161/01.HYP.0000125142.41703.64
Sven, M., Verena, S., Andrea, W., Robert, F., Susanne, F., Wegener Jörg, W., et al. (2003). Dominant Role of Smooth Muscle L-type Calcium Channel Cav1.2 for Blood Pressure Regulation. EMBO J. 22, 6027–6034. doi:10.1093/emboj/cdg583
Tamargo, J., and Ruilope, L. M. (2016). Investigational Calcium Channel Blockers for the Treatment of Hypertension. Expert Opin. Investig. Drugs 25, 1295–1309. doi:10.1080/13543784.2016.1241764
Tan, C., Voss, U., Svensson, S., Erlinge, D., and Olde, B. (2013). High Glucose and Free Fatty Acids Induce Beta Cell Apoptosis via Autocrine Effects of ADP Acting on the P2Y(13) Receptor. Purinergic Signal. 9, 67–79. doi:10.1007/s11302-012-9331-6
Thompson, E., Eldstrom, J., and Fedida, D. (2021). Hormonal Signaling Actions on Kv7.1 (KCNQ1) Channels. Annu. Rev. Pharmacol. Toxicol. 61, 381–400. doi:10.1146/annurev-pharmtox-010919-023645
Tingting, Z., Du, M., Tong, Z., Lingling, Q., Zhiyuan, Z., and Jing, C. (2018). ETA as a Novel Kv2.1 Inhibitor Ameliorates β-cell Dysfunction and Hyperglycaemia. Clin. Exp. Pharmacol. Physiol. 45, 1257–1264. doi:10.1111/cep.2018.45.issue-11
Torekov, S. S., Iepsen, E., Christiansen, M., Linneberg, A., Pedersen, O., Holst, J. J., et al. (2014). KCNQ1 Long QT Syndrome Patients Have Hyperinsulinemia and Symptomatic Hypoglycemia. Diabetes 63, 1315–1325. doi:10.2337/db13-1454
Turner, N., Zeng, X. Y., Osborne, B., Rogers, S., and Ye, J. M. (2016). Repurposing Drugs to Target the Diabetes Epidemic. Trends Pharmacol. Sci. 37, 379–389. doi:10.1016/j.tips.2016.01.007
van der Zijl, N. J., Moors, C. C., Goossens, G. H., Hermans, M. M., Blaak, E. E., and Diamant, M. (2011). Valsartan Improves {beta}-Cell Function and Insulin Sensitivity in Subjects with Impaired Glucose Metabolism: A Randomized Controlled Trial. Diabetes Care 34, 845–851. doi:10.2337/dc10-2224
Viberti, G., and Wheeldon, N. M. (2002). Microalbuminuria Reduction with Valsartan in Patients with Type 2 Diabetes MellitusMicroalbuminuria Reduction with Valsartan in Patients with Type 2 Diabetes Mellitus: A Blood Pressure-independent Effect. Circulation 106, 672–678. doi:10.1161/01.cir.0000024416.33113.0a
Wang, Y., Xue, J., Li, Y., Zhou, X., Qiao, S., and Han, D. (2019). Telmisartan Protects Against High Glucose/high Lipid-Induced Apoptosis and Insulin Secretion by Reducing the Oxidative and ER Stress. Cell Biochem. Funct. 37, 161–168. doi:10.1002/cbf.3383
Weyer, C., Bogardus, C., Mott, D. M., and Pratley, R. E. (1999). The Natural History of Insulin Secretory Dysfunction and Insulin Resistance in the Pathogenesis of Type 2 Diabetes Mellitus. J. Clin. Invest. 104, 787–794. doi:10.1172/JCI7231
Yamana, A., Arita, M., Furuta, M., Shimajiri, Y., and Sanke, T. (2008). The Angiotensin II Receptor Blocker Telmisartan Improves Insulin Resistance and Has Beneficial Effects in Hypertensive Patients with Type 2 Diabetes and Poor Glycemic Control. Diabetes Res. Clin. Pract. 82, 127–131. doi:10.1016/j.diabres.2008.07.003
Yao, H., Zhou, K., Yan, D., Li, M., and Wang, Y. (2009). The Kv2.1 Channels Mediate Neuronal Apoptosis Induced by Excitotoxicity. J. Neurochem. 108, 909–919. doi:10.1111/j.1471-4159.2008.05834.x
Yu, S. P., and Kerchner, G. A. (1998). Endogenous Voltage-Gated Potassium Channels in Human Embryonic Kidney (HEK293) Cells. J. Neurosci. Res. 52, 612–617. doi:10.1002/(SICI)1097-4547(19980601)52:5<612::AID-JNR13>3.0.CO;2-3
Yusuf, S., Ostergren, J. B., Gerstein, H. C., Pfeffer, M. A., Swedberg, K., Granger, C. B., et al. (2005). Effects of Candesartan on the Development of a New Diagnosis of Diabetes Mellitus in Patients with Heart Failure. Circulation 112, 48–53. doi:10.1161/CIRCULATIONAHA.104.528166
Keywords: telmisartan, insulin secretion, Kv channel, AT1 receptor, L-type VGCC
Citation: Liu T, Cui L, Xue H, Yang X, Liu M, Zhi L, Yang H, Liu Z, Zhang M, Guo Q, He P, Liu Y and Zhang Y (2021) Telmisartan Potentiates Insulin Secretion via Ion Channels, Independent of the AT1 Receptor and PPARγ. Front. Pharmacol. 12:739637. doi: 10.3389/fphar.2021.739637
Received: 11 July 2021; Accepted: 30 August 2021;
Published: 14 September 2021.
Edited by:
Ewa Krystyna Szczepanska-Sadowska, Medical University of Warsaw, PolandReviewed by:
Åke Sjöholm, Gävle Hospital, SwedenPaola Miranda Sulis, Federal University of Santa Catarina, Brazil
Copyright © 2021 Liu, Cui, Xue, Yang, Liu, Zhi, Yang, Liu, Zhang, Guo, He, Liu and Zhang. This is an open-access article distributed under the terms of the Creative Commons Attribution License (CC BY). The use, distribution or reproduction in other forums is permitted, provided the original author(s) and the copyright owner(s) are credited and that the original publication in this journal is cited, in accordance with accepted academic practice. No use, distribution or reproduction is permitted which does not comply with these terms.
*Correspondence: Yunfeng Liu, bmVjdGFybGl1QDE2My5jb20=; Yi Zhang, eWl6aGFuZzMxM0AxNjMuY29t