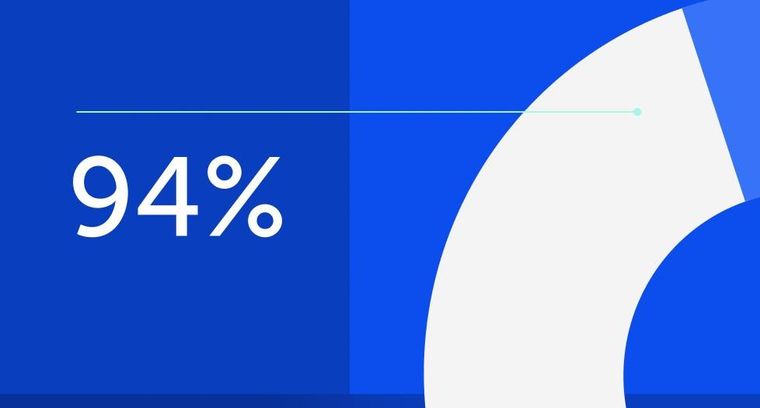
94% of researchers rate our articles as excellent or good
Learn more about the work of our research integrity team to safeguard the quality of each article we publish.
Find out more
REVIEW article
Front. Pharmacol., 09 September 2021
Sec. Inflammation Pharmacology
Volume 12 - 2021 | https://doi.org/10.3389/fphar.2021.735472
This article is part of the Research TopicWomen in Inflammation Pharmacology: 2021View all 5 articles
Sepsis is a continuing problem in modern healthcare, with a relatively high prevalence, and a significant mortality rate worldwide. Currently, no specific anti-sepsis treatment exists despite decades of research on developing potential therapies. Annexins are molecules that show efficacy in preclinical models of sepsis but have not been investigated as a potential therapy in patients with sepsis. Human annexins play important roles in cell membrane dynamics, as well as mediation of systemic effects. Most notably, annexins are highly involved in anti-inflammatory processes, adaptive immunity, modulation of coagulation and fibrinolysis, as well as protective shielding of cells from phagocytosis. These discoveries led to the development of analogous peptides which mimic their physiological function, and investigation into the potential of using the annexins and their analogous peptides as therapeutic agents in conditions where inflammation and coagulation play a large role in the pathophysiology. In numerous studies, treatment with recombinant human annexins and annexin analogue peptides have consistently found positive outcomes in animal models of sepsis, myocardial infarction, and ischemia reperfusion injury. Annexins A1 and A5 improve organ function and reduce mortality in animal sepsis models, inhibit inflammatory processes, reduce inflammatory mediator release, and protect against ischemic injury. The mechanisms of action and demonstrated efficacy of annexins in animal models support development of annexins and their analogues for the treatment of sepsis. The effects of annexin A5 on inflammation and platelet activation may be particularly beneficial in disease caused by SARS-CoV-2 infection. Safety and efficacy of recombinant human annexin A5 are currently being studied in clinical trials in sepsis and severe COVID-19 patients.
Sepsis is defined as life-threatening organ dysfunction caused by a dysregulated host response to infection (Singer et al., 2016). The Global Burden of Disease Study reported there were 11 million sepsis-related deaths globally (1 in 5 of all deaths) in 2017 (Rudd et al., 2020). Subsequently, coronavirus disease 2019 (COVID-19) caused by severe acute respiratory syndrome coronavirus-2 (SARS-CoV-2), a novel coronavirus (Wiersinga et al., 2020), has emerged as a cause of sepsis. While most people infected with this coronavirus are asymptomatic or only show mild symptoms, many have developed severe disease that requires hospitalization, of whom about 20–25% with critical COVID-19 have been admitted to intensive care units (ICU) (CanadaA of (2021) Epid, 2021). As of August 28, 2021, over 4.5 million deaths (2.1% of total cases) have been attributed to COVID-19 although this rate varies widely among countries, ranging from 0.8% in New Zealand to 7.8% in Mexico (www.worldometers.info/coronavirus).
Despite much research, no specific anti-sepsis treatment exists, and management relies on early administration of broad-spectrum antibiotics, resuscitative measures, and corticosteroids to reduce patient mortality (Rice and Bernard, 2005; Ferrer et al., 2009; Cohen et al., 2015). A number of therapeutics have been investigated for their potential as sepsis treatment, including drotrecogin alfa (activated human protein C), which briefly became known as the only drug approved specifically for use in sepsis before it was withdrawn from the market (Angus, 2012). Annexin A5 is a 36-kDa endogenous protein and part of a 12-member family of ubiquitously and constitutively expressed proteins, and possesses anticoagulant, anti-inflammatory and anti-apoptotic properties. The anticoagulant effect of annexin A5 is achieved through binding to phosphatidylserine (PS) on cell membranes of activated platelets and endothelial cells to prevent the assembly of prothrombinase complex and thrombin generation (van Genderen et al., 2008a; Gu et al., 2015a). Unlike activated protein C (Bernard et al., 2001), it does not have thrombolytic action and thus is less likely to cause adverse bleeding events. Additionally, annexin A5 improves nitric oxide signalling and vascular endothelial function through inhibition of inflammation and endothelial activation (Ewing et al., 2011a). These properties make it a promising therapeutic agent to reduce the consequences of endothelial injury in sepsis and COVID-19. In addition, annexin A1 and A2 have also been shown to have protective roles in coagulopathy and inflammation. Although other annexins such as annexin A3, which has been shown to mediate pathogen clearance and its expression is increased in neutrophils in patients with sepsis, their therapeutic potential in sepsis is currently not clear (Toufiq et al. 2020). In this review, we summarize existing knowledge of annexin functions and potential therapeutic applications of annexin A1, A2 and A5 in sepsis and COVID-19.
The annexin superfamily contains over 1,000 proteins found in over 65 different species of plants and animals (Gerke and Moss, 2002; Moss and Morgan, 2004). All annexins share a similar structural core that is highly conserved throughout all members of the superfamily. This core consists of four homologous domains of approximately 70 amino acid residues (except for Annexin A6 which contains eight domains) (Gerke and Moss, 2002), and gives annexins a common 3D structure.
Twelve annexin genes have been found in humans, dispersed among several chromosomes (Moss and Morgan, 2004). Though known primarily as an intracellular protein, several annexins are found extracellularly where they bind to various extracellular and cell membrane ligands and receptors that mediate systemic effects, most notably in coagulation and inflammation (Raynal and Pollard, 1994; Gerke and Moss, 2002; Lizarbe et al., 2013; Schloer et al., 2018). Key to a number of these interactions is the annexin’s conserved ability to bind with negatively charged phospholipids such as PS present on cell membrane surfaces (Schloer et al., 2018).
Annexin A1, also known as lipocortin, is found in a number of tissues, including lung, kidney, bone marrow, intestine, spleen, thymus, brain, and seminal fluid (Fava et al., 1989). Its extracellular activity has been studied extensively, specifically in the realm of inflammation (Fava et al., 1989). Annexin A1 must be externalized to exhibit its anti-inflammatory effects (Blume et al., 2009; Gavins and Hickey, 2012). Its release is regulated by glucocorticoids, and it inhibits the action of phospholipase A2, which subsequently blocks release of arachidonic acid, resulting in anti-inflammatory actions (Sheikh and Solito, 2018).
The key anti-inflammatory effect of extracellular annexin A1 is its interference and modification of the adhesion and migration of leukocytes, which are fundamental steps in the inflammatory response (Gavins and Hickey, 2012). Annexin A1 induces L-selectin shedding by neutrophils, likely by binding to formyl peptide receptors, which results in detachment of adherent leukocytes from endothelium, preventing their trans-endothelial migration (De Coupade et al., 2003; Gavins et al., 2003; Gavins and Hickey, 2012). Annexin A1 also binds and activates cell messengers that activate apoptosis machinery (Solito et al., 2003; Gavins and Hickey, 2012), which prevents necrosis and release of inflammatory factors. It has a role in controlling release of nitric oxide and inhibits cyclooxygenase 2 expression, thereby upregulating potent anti-inflammatory cytokines such as interleukin (IL)-10 (Minghetti et al., 1999). A recent study shows a role for annexin A1 in the regulation of nucleotide-binding oligomerization (NOD-), LRR- and pyrin domain-containing protein (NLRP3) inflammasome activation (Sanches et al., 2020a).
Annexin A1 also has a role in adaptive immunity. Exposing T cells stimulated with CD3 and CD28 to annexin A1 prolongs stimulation of AKT and extracellular signal-regulated kinase pathways, and increases proliferation of T cells (D’Acquisto et al., 2007; Sheikh and Solito, 2018). In tuberculosis, the absence of annexin A1 resulted in impaired CD8+ T cell response, and reduced dendritic cell mediated efferocytosis (Tzelepis et al., 2015). Additionally, annexin A1 promotes wound healing, as annexin A1 supplementation rescued defects in mice in intestinal wound repair caused by NOX1 gene deficiency (Babbin et al., 2008; Leoni et al., 2013).
Annexin A2, also known as chromobindin-8, lipocortin II, and placental anticoagulant protein, is present in many cells, including endothelial cells, monocytes, macrophages, dendritic cells, trophoblast cells, and epithelial cells (Luo and Hajjar, 2013). Though extracellular activity has been observed, it works primarily while bound on the external leaflets of cell membranes, and is not appreciably found in plasma (Hajjar and Krishnan, 1999). Expressed as a tetramer with the S100A10 calcium binding protein, annexin II tetramer (AIIt) translocation to the cell surface is a key step in regulation of fibrinolysis (Hajjar and Krishnan, 1999), and serves as a co-receptor for plasminogen and tissue plasminogen activator (tPA) (Hajjar and Krishnan, 1999).
On the endothelial cell surface, AIIt promotes production of plasmin, resulting in the subsequent breakdown of fibrin (Hajjar and Krishnan, 1999). Annexin A2 deficient mice display deposition of fibrin in the microvasculature and incomplete clearance of injury-induced arterial thrombi (Ling et al., 2004). In humans, an increased annexin A2 expression in acute promyelocytic leukemia (APL) is associated with hyperfibrinolysis and bleeding (Menell et al., 1999; Liu et al. 2011) while reduced annexin A2 expression impairs cell surface fibrinolysis and may constitute a risk factor for venous thromboembolism (Fassel et al., 2021).
AIIt’s action on fibrinolysis regulation is both inhibitory and stimulatory. In the presence of tPA, it stimulates the production of plasmin, enhancing fibrinolysis. However, once plasmin is produced, AIIt rapidly degrades it by stimulating autoproteolysis, thereby inhibiting fibrinolysis (Fitzpatrick et al., 2000). The relationship between annexin A2, AIIt, and fibrinolysis is thus complex.
In sepsis and inflammation, annexin A2 has different roles at different stages of the disease process. In early stages, it limits vascular permeability, preventing edema and extravasation and thereby modulating leukocyte recruitment and inflammatory mediator release. Within hours, it protects internal membranes, preventing inflammasome actions by protecting lysosome membranes and preventing cytokine production and release. By late stages it promotes angiogenesis and wound healing (Dallacasagrande and Hajjar, 2020). However, annexin A2 can serve as a site of adhesion and entry for bacteria such as Pseudomonas aeruginosa, Salmonella typhimurium and rickettsia resulting in pro-inflammatory actions by causing apoptosis and release of inflammatory cytokines (Kirschnek et al., 2005; Miyahara et al., 2009; Jolly et al., 2014; He et al., 2019), may enable viral infection and replication (Taylor et al., 2018), and excessive angiogenesis induced by annexin A2 may induce tissue damage (Liu and Hajjar, 2016). Studies in sepsis models have found that knockout mice that lack annexin A2 have more severe disease and decreased survival in polymicrobial sepsis, with significantly higher bacterial load, worse tissue integrity, and greater distant organ damage (He et al., 2016). In humans, lower levels of annexin A2 correlated with increased sepsis severity (West et al., 2015), and plasmin generation capacity is greatly reduced in septic patients compared to healthy controls (Lanfranco et al., 2013).
Annexin A5 inhibits blood coagulation. Annexin A5 is found in human placenta (syncytiotrophoblasts), blood vessels throughout the body, and membranes in the heart, lung, and liver. Annexin A5 is an accessory protein that helps direct cytosolic phospholipase A2 to the membrane of activated platelets where it controls its activity (Tzima et al., 2000; Tzima and Walker, 2000) and is highly expressed in cell types including vascular endothelial cells that serve a barrier function (Flaherty et al., 1990; Wen et al., 1999; Rand et al., 2012a). The protein self-assembles into trimers that form a protective 2-dimensional crystal lattice when it binds to PS exposed on cell membranes, in the presence of calcium (Reutelingsperger and Van Heerde, 1997; van Genderen et al., 2008b). This lattice acts as a shield, as annexin A5 competes with coagulation factors and prothrombin from binding to PS, and thereby prevents formation of the prothrombin complex and thrombin, resulting in an anticoagulation effect (Andree et al., 1992; Van Heerde et al., 1994; Reutelingsperger and Van Heerde, 1997; van Genderen et al., 2008b).
Annexin A5 binds the apical surface of syncytiotrophoblasts, and maintains normal blood flow through the placenta by preventing coagulation (Krikun et al., 1994). Its shielding ability also promotes membrane resealing in human trophoblasts in pregnancy (Krikun et al., 1994; Rand et al., 1997). Disruption of this shield in pregnant women with antiphospholipid syndrome may cause miscarriage, as the apical surface of the placenta becomes thrombogenic (Rand et al., 1997; Hunt et al., 2011).
Protein kinase C (PKC) is a family of protein kinase enzymes involved in a variety of signal transduction pathways such as apoptosis, cell proliferation, differentiation, and T cell activation through phosphorylation of serine and threonine amino acid residues on the target proteins. PKCs are activated by increases in intracellular diacylglycerol (DAG). There are 15 PKC isozymes in humans. PKC-α, -β, -γ, -δ, and -ε isoforms have important roles in atherosclerosis, myocardial ischemia/reperfusion injury, cardiac hypertrophy, fibrosis and heart failure (Singh et al., 2017). Annexin A5 has been shown to transiently interact with PKC-δ in cells after PKC-δ stimulation, but before PKC-δ translocates to the particulate fraction (Kheifets et al., 2006). In addition, the recruitment of PKC-θ to the membrane, NF-κB signaling and T-cell activation are impaired in ANXA5 knockout T cells, suggesting a critical role of annexin A5 in T-cell activation (Hu et al., 2020).
Annexin A5 protects cells from apoptosis and reduces inflammation. PS on cell membranes is also a key signal molecule in apoptosis, signaling for immune cells to phagocytose apoptotic cells (Balasubramanian and Schroit, 2003). Annexin A5’s shielding ability inhibits this phagocytosis by physically shielding surface expression ligands located near PS residues, as well as down-regulating the expression of those ligands, and may play a role in preventing the engulfment of living cells expressing PS (Kenis et al., 2006). Notably, annexin A5 inhibits proteolytic activation of caspase-3, an executioner caspase critical to apoptosis (Gidon-Jeangirard et al., 1999). Annexin A5 also bind to PS on microparticles released from platelets and leukocytes. The microparticles have pro-coagulant and pro-inflammatory properties, and are implicated in a number of disease conditions, including atherosclerosis, cancer, autoimmune disease, and sepsis (Ardoin et al., 2007). Not only is the annexin A5 binding and shielding capable of acting as a physical constraint to blebbing and microparticle shedding, its binding to microparticle membrane is sufficient to reduce coagulant and inflammatory processes promoted by these microparticles (Thiagarajan and Tait, 1991). Furthermore, annexin A5 coated microparticles or extracellular vesicles (EVs) are more readily taken up by monocytes and macrophages. In mice infected with E. coli, annexin A5 treatment decreases circulating EVs and dose-dependently delays the development of sepsis (Tontanahal et al., 2021). Additionally, annexin A5 reduces inflammation by shifting the macrophages from the classically activated phenotype (M1) to alternatively activated (M2) phenotype via directly interacting with pyruvate kinase M2 (PKM2) in the liver (Xu et al., 2020).
Annexin A5 has additional actions that may be important in health and disease. It binds to glycosaminoglycans such as heparin and heparan sulfate, a major component of cell surface proteoglycans of endothelial cells (Capila et al., 2001), promoting anticoagulation. Annexin A5 interacts with the N-terminal leucine-rich repeats (LRR) of polycystin-1, a transmembrane protein expressed apical membranes, adherens junctions and desmosomes. Annexin A5 inhibits the effects of polycystin-1 on the recruitment of E-cadherin to reform junctions in canine kidney cells (Markoff et al., 2007). Annexin A5 may be involved in regulation of vascular endothelial cell proliferation as a signaling protein for vascular endothelial growth factor receptor-2 (VEGFR-2) by directly interacting with the intracellular domain of the receptor (Wen et al., 1999). Annexin A5 also binds to distinct sites of negatively charged phospholipids present in oxidized low-density lipoprotein (Van Tits et al., 2005), and has been shown to reduce vascular inflammation and improve endothelial function in ApoE knockout mice of atherosclerosis (Ewing et al., 2011b).
Annexin A5 is the only annexin currently used in clinical practice. Based on the observation that PS is expressed on the surface of mammalian apoptotic cells (Fadok et al., 1992), which annexin A5 binds with a high affinity, annexin A5 can be used in flow cytometry to detect apoptotic cells after chemotherapy (Koopman et al., 1994; Belhocine et al., 2002), cord blood to improve prediction of potency for engraftment (Duggleby et al., 2012), and detection of anti-platelet antibodies for the diagnosis of antiphospholipid syndrome (Tomer et al., 2007). It has been evaluated as a marker for lymphocyte apoptosis in sepsis but results changed with storage time prior to the assay (Greineder et al., 2007). Annexin A5 can also be used to quality check stored platelets, as they expose more PS residues over time (Tait et al., 1999).
Various annexin A5 imaging probes have been found to be safe in human imaging studies in vivo (Boersma et al., 2005), and have been used to image apoptosis in various conditions, including cardiovascular disease (Thimister et al., 2003; Korngold et al., 2008), allograft rejection in transplants (Blankenberg et al., 2000; Kown et al., 2001; Narula et al., 2001), evaluation of cancer treatment efficacy by assessing apoptosis of tumor cells after treatment (Belhocine et al., 2002; Mochizuki et al., 2003; Kartachova et al., 2004).
Difficulty in manufacturing large quantities of the annexin A1 protein, and antibody formation against injected protein have limited its therapeutic use (Flower and Rothwell, 1994). Because of this, small peptides derived from the N terminal region of annexin A1 were developed to retain its biological activity. Of the three analogue peptides (Gavins and Hickey, 2012), Ac2-26 has undergone the most extensive evaluation but only in animal models (Table 1).
Annexin A1 and its derivative peptides have been shown to have therapeutic effect in various disease states, including sepsis (Gavins et al., 2012; Zhang et al., 2018), lung inflammation (da Cunha et al., 2012) and chronic obstructive pulmonary disease (COPD) (Possebon et al., 2018), myocardial infarction (D’ Amico et al., 2000; La et al., 2001; Qin et al., 2015), and intestinal wound repair (Babbin et al., 2008). Studies confirmed that endogenous annexin A1 plays a role in promoting phagocytosis (Yona et al., 2006; Scannell et al., 2007), and its derived peptide Ac2-26 increases macrophage phagocytosis of apoptotic polymorphonuclear leukocytes (PMNs) (Maderna et al., 2005). Ac2-26 administration in murine lipopolysaccharide (LPS) models of sepsis has demonstrated inhibition of cardiomyocyte apoptosis and myocardial damage (Zhang et al., 2018), reduced leukocyte adhesion and cerebrovascular inflammatory response in the brain (Gavins et al., 2012), and reduced inflammatory cytokine release and subsequent decreased inflammation in lung (da Cunha et al., 2012; Possebon et al., 2018). Another annexin A1 analogue (CR-Ac2-50) reduces inflammation and attenuates myocardial dysfunction in a polymicrobial sepsis model (Dalli et al., 2013; Gobbetti et al., 2014).
In ischemic insult, Ac2-26 and annexin A1 demonstrate cardioprotective effects in rats and mice, reducing infarct size and myeloperoxidase activity (D’ Amico et al., 2000; La et al., 2001; Qin et al., 2015), although not as completely as corticosteroid treatment (Ritchie et al., 2003). In mice models of ischemia and reperfusion, annexin A1 mimetics prevent white blood cell adhesion and markers of inflammation in cerebral infarct (Gavins et al., 2007) and inhibit macrophage infiltration, maintaining glomerular filtration rate and urine osmolality while preventing acute tubular necrosis in renal ischemia (Facio et al., 2011).
Zhang and co-workers reported that annexin A2 and its tetramer AnxA2-S100A10 directly activated human macrophages through toll-like receptor-4 (TLR4) signaling, facilitating its internalization in the cell and signaling for release of anti-inflammatory cytokines (Zhang et al., 2015). However, annexin A2 has not been explored as a therapeutic. This may be due to anticoagulant and anti-inflammatory properties that are weaker than annexin A1 or A5, mechanisms of action for modulation of fibrinolysis that are not fully understood and variable based on tissue type as shown with its pleiotropic activity (Bharadwaj et al., 2013), and potential pro-inflammatory actions that facilitate bacterial and viral infections (Taylor et al., 2018; He et al., 2019). Additionally, increased annexin A2 expression is a common phenomenon in many cancers, and correlates to adverse clinical outcomes in patients (Christensen et al., 2018). So, while annexin A2 has endogenous anticoagulant activity, it may serve as a potential therapeutic target rather than a treatment.
Because of its high affinity binding to PS exposed on the membrane of apoptotic cells, various annexin A5 imaging probes have been used and found to be safe in human imaging studies (Boersma et al., 2005), including cardiovascular disease (Thimister et al., 2003; Korngold et al., 2008), allograft rejection in transplants (Blankenberg et al., 2000; Kown et al., 2001; Narula et al., 2001), and evaluation of cancer treatment efficacy by assessing apoptosis of tumor cells after treatment (Belhocine et al., 2002; Mochizuki et al., 2003; Kartachova et al., 2004). Annexin A5 and its human recombinant homodimer, diannexin, which was designed to have a longer circulating half time and greater binding affinity (Rand et al., 2012b), has been explored as a therapeutic protein in a wide range of disease conditions and contexts, most notably inflammation, sepsis, hemorrhage, coagulopathy, ischemia, and organ transplant. However, most of these studies are in animal models of endotoxemia (Table 2).
Annexin A5 can bind LPS directly to reduce its activity and macrophage response to LPS, and pretreatment with annexin A5 in mice reduces serum tumor necrosis factor α (TNF-α) concentrations similar to control animals (Rand et al., 2012a). Annexin A5 blocks TLR4 signaling in dendritic cells and cardiomyocytes, thereby reducing LPS induced proinflammatory cytokine release of IL-1β, IL-6 and TNF-α (Arnold et al., 2014; Park et al., 2016). It also inhibits late acting mediators of systemic inflammation such as high mobility group box 1 (HMGB-1), inducing an anti-inflammatory response (Park et al., 2016). Annexin A5 can repair LPS induced damage to cardiomyocyte adherens junctions, preventing cardiac inflammation, and improving hemodynamics in mice (Gu et al., 2015b). Animal survival in endotoxemia was improved, showing significant improvements not only when annexin A5 was injected immediately after LPS injection, but also with delayed annexin A5 treatment 4 h after LPS injection. This therapeutic window could be relevant to clinical sepsis (Arnold et al., 2014). Besides beneficial results shown in heart tissue in endotoxemia, annexin A5 treatment also improved tissue damage and function in the liver (Park et al., 2016), and gut (Beattie et al., 2019) in similar conditions, with anti-inflammatory effects reducing endothelial damage, and protective effects against sepsis induced coagulopathy by preventing fibrin deposition in organ microvasculature (Beattie et al., 2019).
Annexin A5 and diannexin’s ability to bind PS with high affinity make them potent inhibitors of platelet activity by preventing thrombin generation and reducing platelet accumulation. In mice, diannexin treatment inhibited thrombi formation by reducing platelet accumulation, and increased blood loss after tail tip transection (Rand et al., 2012b). In human monocytes, annexin A5 inhibited procoagulant activity induced by LPS, and a relatively low concentration of annexin A5 (0.1 μg/ml) was sufficient for anticoagulant effects to occur with an IC50 of 0.6 μg/ml (or 17.6 nM), demonstrating its potency (Sato et al., 2004). Annexin A5 also reduced thrombin generation induced by cecal ligation and perforation, which is a clinically relevant model of sepsis (Wang et al., 2018).
Annexin A5 has also generated interest in ischemia-reperfusion injury and organ transplant, based on the anticoagulant and anti-inflammatory effects, as well as endogenous annexin A5’s ability to promote membrane repair in certain tissues (Bouter et al., 2011). In murine models of hepatic ischemia reperfusion injury, diannexin treatment preserved sinusoidal endothelial cell integrity, prevented cell swelling and inflammatory cell recruitment, and reduced inflammatory mediator release, thereby reducing hepatic apoptosis and restoring sinusoidal blood flow (Shen et al., 2007; Teoh et al., 2007). In a murine model of lung transplant, diannexin treatment produced protection from inflammation, cell death, and fibrinolysis, as well as improvement of compromised organ function (Hashimoto et al., 2016).
Therapeutic use of diannexin in humans is limited. In a phase 2 trial with 58 renal transplant patients receiving marginal donor kidneys, a single diannexin intravenous bolus of 400 μg/kg was associated with improved renal function and lesser need for dialysis compared to control patients (Cooper et al., 2010). However, a subsequent multicenter phase 2/3 trial evaluating the efficacy, safety, and tolerability of diannexin in kidney transplant recipients was terminated prematurely after recruiting 21 patients out of 591 planned (NCT01442337) based on additional review of pre-clinical toxicology data (Tsapepas et al., 2013).
A randomized and placebo-controlled phase 1 clinical trial was conducted to evaluate safety, tolerance and pharmacokinetics of recombinant human annexin A5 (SY-005) in 94 healthy subjects (NCT04217629). The trial was recently completed. Doses of intravenous injection of SY-005 from 0.75 to 20 mg per person were well tolerated without any major adverse events, suggesting that recombinant human annexin A5 is safe in healthy subjects. Another phase 1 study using annexin A5 as a therapeutic drug is recruiting healthy volunteers (NCT04850339).
The emergence of severe acute respiratory syndrome coronavirus-2 (SARS-CoV-2) on a global scale has driven a large need for treatments of COVID-19 induced sepsis and acute respiratory distress syndrome (ARDS). In COVID-19, SARS-CoV-2 enters the host cells via angiotensin converting enzyme 2 (ACE2) (Figure 1) and several proteases including transmembrane protease serine 2, furin and cathepsin L/B (Gheblawi et al., 2020; Hoffmann et al., 2020). Once inside the cell, it replicates rapidly and causes a systemic proinflammatory response with increased cytokine levels, leukocytosis, lymphopenia (in CD4+ and CD8+ T cells) and decreased interferon-α (IFNα) expression in CD4+ T cells. SARS-CoV-2 infiltration of cells is also capable of activating inflammasomes, such as NOD-, LRR- and pyrin domain-containing protein (NLRP3), which eventually leads to pore formation on the cell surface, IL-1β and IL-18 secretion and eventual pyroptosis, which further promotes inflammation (Yap et al., 2020). Thrombocytopenia, elevated prothrombin time, and high levels of D-dimers seen in COVID-19 infection suggest coagulopathy (Prompetchara et al., 2020), and though the initial target organ is the lung, the thromboinflammatory process subsequently can affect all organs of the body (Oudkerk et al., 2020). The imbalance between pro- and anti-inflammatory responses causes dysfunction of multiple organs, including the lung, liver, kidney, and the cardiovascular system, as well as coagulation/thrombolysis system, leading to septic shock with a high mortality (Blanco-Melo et al., 2020).
FIGURE 1. Effects of SARS-CoV-2 infection on host cells. SARS-CoV-2 exploits ACE2 receptors to gain entry to cells and causes endocytosis of ACE2 receptors. This internalization reduces availability of ACE2 in affected cells, causing systemic renin-angiotensin system deregulation and subsequent up-regulation of inflammatory responses, pyroptosis and release of inflammatory cytokines. Direct invasion of endothelial cells by SARS-CoV-2 and ensuing inflammation results in endotheliitis and increased release of von Willebrand factor from endothelial cells, promoting clotting in vessels.
Annexins may have therapeutic potential in viral sepsis. While the mechanism of sepsis is different between bacterial and viral insults, they share commonalities in the activated receptors and released cytokines (DeMerle et al., 2021). To this end, annexins could be an effective therapy for patients suffering from COVID-19 induced sepsis or coagulopathy (Figure 2). Generally, severe cases of COVID-19 are associated with excessive cytokine release, with large amounts of IL-6, IL-1β, IL-2, IFN-γ, TNF-α, and other cytokines released (Fraser et al., 2020). IL-6 is a key inflammatory cytokine in SARS-CoV-2 infection, and its levels in COVID-19 patients are higher than those usually seen in severe bacterial sepsis (Levi, 2020; Paar et al., 2020). IL-6 levels in COVID-19 patients are a strong predictor of mortality and lung damage, and it has been suggested as a key therapeutic target in COVID-19 associated pathologies (Levi, 2020; Paar et al., 2020). Annexins have been found in various animal models to reduce the levels of IL-6, so there may be potential for its use in treatment of COVID-19 inflammation as an adjunct or alternative approach to anti-IL-6 receptor antibodies (Shen et al., 2007; Wever et al., 2011; Possebon et al., 2018). Additionally, NLRP3 activation promotes IL-1β expression and induces pyroptosis (Gao et al., 2018). A recent study showed that addition of Ac2-26 peptide decreases levels of IL-1β in macrophages, suggesting inhibition of the NLRP3 inflammasome (Sanches et al., 2020b). It is therefore possible that annexin A1, and possibly other annexins such as A5, may also be capable of protecting cells against SARS-CoV-2 induced pyroptosis.
FIGURE 2. Schematic of hypothesis. Multifaceted actions of annexin A5 that are proposed to mitigate ARDS and multi-organ failure in coronavirus 2019 disease. SARS-CoV-2 causes sepsis and subsequent ARDS and multi-organ failure by multiple mechanisms, notably by promoting systemic inflammation and coagulopathy by its ability to internalize ACE2 and cause endotheliitis. Annexin A5 is hypothesized to impede these actions by its ability to reduce inflammation in disease states, inhibit thrombosis and lessen coagulopathy.
COVID-19 systemic inflammation is accompanied by hemostatic abnormalities, especially in severe disease, with elevated coagulation, complement activation, higher incidence of venous thromboembolic events, and disseminated intravascular coagulopathy (DIC) (Levi et al., 2020; Paar et al., 2020). Heparin has been reported to reduce mortality due to SARS-CoV-2, and anticoagulation has been suggested as an important step to managing COVID-19 and mitigating the interplay between inflammation and thrombosis that occurs in the disease (Magro, 2020). Annexin A1 analogue Ac2-26 and recombinant human annexin A5, which have been shown in animal models of sepsis and other inflammatory models to reduce expression of pro-inflammatory cytokines, possess anticoagulant properties via inhibition of thrombin generation and platelet aggregation, and modulate the response to apoptosis, are promising therapeutic agents to reduce the severity of inflammatory processes in COVID-19. However, the exact mechanism of COVID-19 coagulopathy is not fully understood. Some studies of COVID-19 describe a pattern similar to DIC (Levi et al., 2020; Paar et al., 2020), which is seen in bacterial sepsis, while others suggest that COVID-19 associated coagulopathy is different from classic sepsis induced coagulopathy (SIC) and DIC. Direct endothelial infection by SARS-CoV-2 through ACE2, which is abundantly expressed on endothelial cells, induces endothelialitis and releases von Willebrand factor (vWF) and upregulates tissue factor expression, promoting thrombosis (Varga et al., 2020). Additionally, a function of ACE2 is to catalyze angiotensin II conversion to angiotensin-(1–7). SARS-CoV-2 binding to ACE2 and subsequent internalization downregulates surface ACE2 expression, leading to angiotensin II elevation, vasoconstriction, and decreased blood flow, which enhances thrombosis (Liu et al., 2020). Further, endothelial cell activation and glycocalyx degradation can stimulate platelet activation and microthrombi generation. Notably, we recently reported in critically ill COVID-19 patients evidence for greater endothelial cell activation and glycocalyx degradation than non-COVID-19 subjects (Fraser et al., 2020). It is possible that annexin A5 treatment could mitigate these effects and consequences of endothelial injury.
Extracellular HMGB-1 is another potential therapeutic target. Extracellular HMGB-1 is released from dying cells and forms complexes with extracellular DNA, RNA, and other molecules after lytic cell death, which are endocytosed. It has been proposed that SARS-CoV-2 RNA may enter cells via HMGB1-assisted transfer, promoting viral infection (Andersson et al., 2020a). Plasma levels of HMGB-1 in COVID-19 patients admitted to the ICU were significantly elevated in comparison to healthy subjects and non-ICU COVID-19 patients (Chen et al., 2020a). SARS-CoV-2 (Choudhury and Mukherjee, 2020) and other viruses, including RSV, Ebola, and dengue (Olejnik et al., 2018), also activate toll-like receptor-4 (TLR4) to induce the inflammatory response. High mobility group protein-box 1 (HMGB-1) is another ubiquitous protein implicated in the inflammatory response to infections, including SARS-CoV-2 (Sundén-Cullberg et al., 2005; Andersson et al., 2020b; Chen et al., 2020b). Since annexin A5 inhibits HMGB-1 interaction with TLR4 in models of sepsis and inflammation (Park et al., 2016), it could interfere with HMGB1-mediated SARS-CoV-2 infection.
We have started a randomized, double blind and placebo-controlled phase 2 clinical trial to evaluate safety, tolerance and pharmacokinetics of recombinant human annexin A5 in COVID-19 patients with sepsis (NCT04748757). The intervention is SY-005, 50 or 100 μg/kg intravenously every 12 h for 7 days, with a placebo comparator. The primary outcomes are feasibility metrics of enrollment, treatment delivered per protocol, data collection per protocol, and adverse events. At the time of this submission, 12 patients were recruited with no serious adverse events.
Annexins are endogenously produced proteins with anti-inflammatory, anti-apoptotic, and anticoagulant activities. Annexins and their peptide analogues, when administered in various conditions in different animal models of disease, can alleviate and improve inflammation, coagulation, and ischemia-reperfusion injury. Evidence to date suggests that annexins could be used as a treatment, prophylactic therapy, or adjuvant therapy in various contexts, most notably sepsis, coagulopathy, and ischemic-reperfusion injury. Confirmation of these benefits is required in human trials. Annexin therapy could thus be an effective therapy in various disease conditions, especially sepsis, and novel diseases such as SARS-CoV-2 infection.
LM prepared the first draft of the manuscript. All authors reviewed, revised, and provided final approval to the manuscript.
LM was supported by a Summer Research Training Program stipend from Schulich School of Medicine and Dentistry. CMM and QF received a research grant from the Government of Ontario to conduct an investigator-initiated clinical trial of annexin A5 in patients with COVID-19. Work in QF laboratory was supported by grants from the Canadian Institutes of Health Research (CIHR), the Heart and Stroke Foundation of Canada (HSFC), and the Richard and Jean Ivey Fund. QF is a Richard and Jean Ivey Chair in Molecular Toxicology, Schulich School of Medicine and Dentistry, Western University.
QF is an inventor of a patent owned by Lawson Health Research Institute on annexin and its use to treat inflammatory disorders.
The remaining authors declare that the research was conducted in the absence of any commercial or financial relationships that could be construed as a potential conflict of interest.
All claims expressed in this article are solely those of the authors and do not necessarily represent those of their affiliated organizations, or those of the publisher, the editors and the reviewers. Any product that may be evaluated in this article, or claim that may be made by its manufacturer, is not guaranteed or endorsed by the publisher.
ACE2, angiotensin converting enzyme 2; AIIt, annexin II tetramer; APL, acute promyelocytic leukemia; ARDS, acute respiratory distress syndrome; DIC, disseminated intravascular coagulopathy; HMGB-1, high mobility group box 1; IFN, interferon; IL, interleukin; LPS, lipopolysaccharide; LRR, leucine-rich repeat; NLRP-3, NOD-, LRR- and pyrin domain-containing protein; PMN, polymorphonuclear leukocyte; PS, phosphatidylserine; SARS-CoV-2, severe acute respiratory syndrome coronavirus-2; TLR4, toll-like receptor-4; TNF-α, tumor necrosis factor α; tPA, tissue plasminogen activator; VEGFR-2, vascular endothelial growth factor receptor-2; vWF, von Willebrand factor.
Andersson, U., Ottestad, W., and Tracey, K. J. (2020). Extracellular HMGB1: a Therapeutic Target in Severe Pulmonary Inflammation Including COVID-19? Mol. Med. 26 (1), 42–13. doi:10.1186/s10020-020-00172-4
Andersson, U., Ottestad, W., and Tracey, K. J. (2020). Extracellular HMGB1: a Therapeutic Target in Severe Pulmonary Inflammation Including COVID-19? Mol. Med. 26 (1), 42. doi:10.1186/s10020-020-00172-4
Andree, H. A., Stuart, M. C., Hermens, W. T., Reutelingsperger, C. P., Hemker, H. C., Frederik, P. M., et al. (1992). Clustering of Lipid-Bound Annexin V May Explain its Anticoagulant Effect. J. Biol. Chem. 267 (25), 17907–17912. doi:10.1016/s0021-9258(19)37128-5
Angus, D. C. (2012). Drotrecogin Alfa (activated). a Sad Final Fizzle to a Roller-Coaster Party. Crit. Care 16 (1), 107. doi:10.1186/cc11152
Ardoin, S. P., Shanahan, J. C., and Pisetsky, D. S. (2007). The Role of Microparticles in Inflammation and Thrombosis. Scand. J. Immunol. 66 (2-3), 159–165. doi:10.1111/j.1365-3083.2007.01984.x
Arnold, P., Lu, X., Amirahmadi, F., Brandl, K., Arnold, J. M., and Feng, Q. (2014). Recombinant Human Annexin A5 Inhibits Proinflammatory Response and Improves Cardiac Function and Survival in Mice with Endotoxemia. Crit. Care Med. 42 (1), e32–41. doi:10.1097/CCM.0b013e3182a63e01
Babbin, B. A., Laukoetter, M. G., Nava, P., Koch, S., Lee, W. Y., Capaldo, C. T., et al. (2008). Annexin A1 Regulates Intestinal Mucosal Injury, Inflammation, and Repair. J. Immunol. 181 (7), 5035–5044. doi:10.4049/jimmunol.181.7.5035
Balasubramanian, K., and Schroit, A. J. (2003). Aminophospholipid Asymmetry: a Matter of Life and Death. Annu. Rev. Physiol. 65, 701–734. doi:10.1146/annurev.physiol.65.092101.142459
Beattie, G., Cohan, C., Miraflor, E., Brigode, W., and Victorino, G. P. (2019). Protective Effect of Phosphatidylserine Blockade in Sepsis Induced Organ Dysfunction. Surgery 166 (5), 844–848. doi:10.1016/j.surg.2019.05.020
Belhocine, T., Steinmetz, N., Hustinx, R., Bartsch, P., Jerusalem, G., Seidel, L., et al. (2002). Increased Uptake of the Apoptosis-Imaging Agent (99m)Tc Recombinant Human Annexin V in Human Tumors after One Course of Chemotherapy as a Predictor of Tumor Response and Patient Prognosis. Clin. Cancer Res. 8 (9), 2766–2774.
Bernard, G. R., Vincent, J. L., Laterre, P. F., LaRosa, S. P., Dhainaut, J. F., Lopez-Rodriguez, A., et al. (2001). Efficacy and Safety of Recombinant Human Activated Protein C for Severe Sepsis. N. Engl. J. Med. 344 (10), 699–709. doi:10.1056/NEJM200103083441001
Bharadwaj, A., Bydoun, M., Holloway, R., and Waisman, D. (2013). Annexin A2 Heterotetramer: Structure and Function. Int. J. Mol. Sci. 14 (3), 6259–6305. doi:10.3390/ijms14036259
Blanco-Melo, D., Nilsson-Payant, B. E., Liu, W. C., Uhl, S., Hoagland, D., Møller, R., et al. (2020). Imbalanced Host Response to SARS-CoV-2 Drives Development of COVID-19. Cell 181 (5), 1036–e9. doi:10.1016/j.cell.2020.04.026
Blankenberg, F. G., Robbins, R. C., Stoot, J. H., Vriens, P. W., Berry, G. J., Tait, J. F., et al. (2000). Radionuclide Imaging of Acute Lung Transplant Rejection with Annexin V. Chest 117 (3), 834–840. doi:10.1378/chest.117.3.834
Blume, K. E., Soeroes, S., Waibel, M., Keppeler, H., Wesselborg, S., Herrmann, M., et al. (2009). Cell Surface Externalization of Annexin A1 as a Failsafe Mechanism Preventing Inflammatory Responses during Secondary Necrosis. J. Immunol. 183 (12), 8138–8147. doi:10.4049/jimmunol.0902250
Boersma, H. H., Kietselaer, B. L., Stolk, L. M., Bennaghmouch, A., Hofstra, L., Narula, J., et al. (2005). Past, Present, and Future of Annexin A5: from Protein Discovery to Clinical Applications. J. Nucl. Med. 46 (12), 2035–2050.
Bouter, A., Gounou, C., Bérat, R., Tan, S., Gallois, B., Granier, T., et al. (2011). Annexin-A5 Assembled into Two-Dimensional Arrays Promotes Cell Membrane Repair. Nat. Commun. 2 (1), 270–279. doi:10.1038/ncomms1270
Canada PHA of (2021). Epidemiological Summary of COVID-19 Cases in Canada. Aem. Published April 19, 2020. Available at: https://health-infobase.canada.ca/covid-19/epidemiological-summary-covid-19-cases.html (Accessed March 18, 2021).
Capila, I., Hernáiz, M. J., Mo, Y. D., Mealy, T. R., Campos, B., Dedman, J. R., et al. (2001). Annexin V-Hheparin Oligosaccharide Complex Suggests Heparan Sulfate-Mmediated Assembly on Cell Surfaces. Structure 9 (1), 57–64. doi:10.1016/S0969-2126(00)00549-9
Chen, L., Long, X., Xu, Q., Tan, J., Wang, G., Cao, Y., et al. (2020). Elevated Serum Levels of S100A8/A9 and HMGB1 at Hospital Admission Are Correlated with Inferior Clinical Outcomes in COVID-19 Patients. Cell Mol Immunol. 17 (9), 992–994. doi:10.1038/s41423-020-0492-x
Chen, L., Long, X., Xu, Q., Tan, J., Wang, G., Cao, Y., et al. (2020). Elevated Serum Levels of S100A8/A9 and HMGB1 at Hospital Admission Are Correlated with Inferior Clinical Outcomes in COVID-19 Patients. Cel Mol Immunol 17 (9), 992–994. doi:10.1038/s41423-020-0492-x
Choudhury, A., and Mukherjee, S. (2020). In Silico studies on the Comparative Characterization of the Interactions of SARS-CoV-2 Spike Glycoprotein with ACE-2 Receptor Homologs and Human TLRs. J. Med. Virol. 92, 2105–2113. doi:10.1002/jmv.25987
Christensen, M. V., Høgdall, C. K., Jochumsen, K. M., and Høgdall, E. V. S. (2018). Annexin A2 and Cancer: a Systematic Review. Int. J. Oncol. 52 (1), 5–18. doi:10.3892/ijo.2017.4197
Cohen, J., Vincent, J. L., Adhikari, N. K., Machado, F. R., Angus, D. C., Calandra, T., et al. (2015). Sepsis: a Roadmap for Future Research. Lancet Infect. Dis. 15 (5), 581–614. doi:10.1016/S1473-3099(15)70112-X
Cooper, M., Kapur, S., and Stratta, R. (2010). Diannexin, a Novel Ischemia/reperfusion Therapeutic Agent, Reduces Delayed Graft Function (DGF) in Renal Transplant Recipients from Marginal Donors. Vancouver, BC: American Transplant Congress.
D’ Amico, M., Di Filippo, C., La, M., Solito, E., McLean, P. G., JFlower, R. J., et al. (2000). Lipocortin 1 Reduces Myocardial Ischemia‐reperfusion Injury by Affecting Local Leukocyte Recruitment. FASEB j. 14 (13), 1867–1869. doi:10.1096/fj.99-0602fje
D’Acquisto, F., Merghani, A., Lecona, E., Rosignoli, G., Raza, K., Buckley, C. D., et al. (2007). Annexin-1 Modulates T-Cell Activation and Differentiation. Blood 109 (3), 1095–1102. doi:10.1182/blood-2006-05-022798
Da Cunha, E. E., Oliani, S. M., and Damazo, A. S. (2012). Effect of Annexin-A1 Peptide Treatment during Lung Inflammation Induced by Lipopolysaccharide. Pulm. Pharmacol. Ther. 25 (4), 303–311. doi:10.1016/j.pupt.2012.04.002
Dallacasagrande, V., and Hajjar, K. A. (2020). Annexin A2 in Inflammation and Host Defense. Cells 9 (6), 1499. doi:10.3390/cells9061499
Dalli, J., Consalvo, A. P., Ray, V., Di Filippo, C., D'Amico, M., Mehta, N., et al. (2013). Proresolving and Tissue-Protective Actions of Annexin A1-Based Cleavage-Resistant Peptides Are Mediated by Formyl Peptide Receptor 2/lipoxin A4 Receptor. J. Immunol. 190 (12), 6478–6487. doi:10.4049/jimmunol.1203000
De Coupade, C., Solito, E., and Levine, J. D. (2003). Dexamethasone Enhances Interaction of Endogenous Annexin 1 with L-Selectin and Triggers Shedding of L-Selectin in the Monocytic Cell Line U-937. Br. J. Pharmacol. 140 (1), 133–145. doi:10.1038/sj.bjp.0705413
DeMerle, K. M., Angus, D. C., Baillie, J. K., Brant, E., Calfee, C. S., Carcillo, J., et al. (2021). Sepsis Subclasses: A Framework for Development and Interpretation*. Crit. Care Med. 49, 748–759. Online First. doi:10.1097/CCM.0000000000004842
Duggleby, R. C., Querol, S., Davy, R. C., Fry, L. J., Gibson, D. A., Horton, R. B., et al. (2012). Flow Cytometry Assessment of Apoptotic CD34+ Cells by Annexin V Labeling May Improve Prediction of Cord Blood Potency for Engraftment. Transfusion 52 (3), 549–559. doi:10.1111/j.1537-2995.2011.03305.x
Ewing, M. M., de Vries, M. R., Nordzell, M., Pettersson, K., de Boer, H. C., van Zonneveld, A. J., et al. (2011). Annexin A5 Therapy Attenuates Vascular Inflammation and Remodeling and Improves Endothelial Function in Mice. Arterioscler Thromb. Vasc. Biol. 31 (1), 95–101. doi:10.1161/ATVBAHA.110.216747
Ewing, M. M., De Vries, M. R., Nordzell, M., Pettersson, K., de Boer, H. C., van Zonneveld, A. J., et al. (2011). Annexin A5 Therapy Attenuates Vascular Inflammation and Remodeling and Improves Endothelial Function in Mice. Arterioscler Thromb. Vasc. Biol. 31 (1), 95–101. doi:10.1161/ATVBAHA.110.216747
Facio, F. N., Sena, A. A., Araújo, L. P., Mendes, G. E., Castro, I., Luz, M. A., et al. (2011). Annexin 1 Mimetic Peptide Protects against Renal Ischemia/reperfusion Injury in Rats. J. Mol. Med. (Berl) 89 (1), 51–63. doi:10.1007/s00109-010-0684-4
Fadok, V. A., Voelker, D. R., Campbell, P. A., Cohen, J. J., Bratton, D. L., and Henson, P. M. (1992). Exposure of Phosphatidylserine on the Surface of Apoptotic Lymphocytes Triggers Specific Recognition and Removal by Macrophages. J. Immunol. 148 (7), 2207–2216.
Fava, R. A., McKanna, J., and Cohen, S. (1989). Lipocortin I (P35) Is Abundant in a Restricted Number of Differentiated Cell Types in Adult Organs. J. Cel Physiol 141 (2), 284–293. doi:10.1002/jcp.1041410209
Fassel, H., Chen, H., Ruisi, M., Kumar, N., DeSancho, M., and Hajjar, K. A. (2021). Reduced Expression of Annexin A2 Is Associated with Impaired Cell Surface Fibrinolysis and Venous Thromboembolism. Blood 137 (16), 2221–2230. doi:10.1182/blood.2020008123
Ferrer, R., Artigas, A., Suarez, D., Palencia, E., Levy, M. M., Arenzana, A., et al. (2009). Effectiveness of Treatments for Severe Sepsis: a Prospective, Multicenter, Observational Study. Am. J. Respir. Crit. Care Med. 180 (9), 861–866. doi:10.1164/rccm.200812-1912OC
Fitzpatrick, S. L., Kassam, G., Choi, K. S., Kang, H. M., Fogg, D. K., and Waisman, D. M. (2000). Regulation of Plasmin Activity by Annexin II Tetramer. Biochemistry 39 (5), 1021–1028. doi:10.1021/bi991411z
Flaherty, M. J., West, S., Heimark, R. L., Fujikawa, K., and Tait, J. F. (1990). Placental Anticoagulant Protein-I: Measurement in Extracellular Fluids and Cells of the Hemostatic System. J. Lab. Clin. Med. 115 (2), 174–181.
Flower, R. J., and Rothwell, N. J. (1994). Lipocortin-1: Cellular Mechanisms and Clinical Relevance. Trends Pharmacol. Sci. 15 (3), 71–76. doi:10.1016/0165-6147(94)90281-X
Fraser, D. D., Cepinskas, G., Slessarev, M., Martin, C., Daley, M., Miller, M. R., et al. (2020). Inflammation Profiling of Critically Ill Coronavirus Disease 2019 Patients. Crit. Care Explor 2 (6), e0144. doi:10.1097/cce.0000000000000144
Gao, Y. L., Zhai, J. H., and Chai, Y. F. (2018). Recent Advances in the Molecular Mechanisms Underlying Pyroptosis in Sepsis. Mediators Inflamm. 2018, 5823823. doi:10.1155/2018/5823823
Gavins, F. N., Dalli, J., Flower, R. J., Granger, D. N., and Perretti, M. (2007). Activation of the Annexin 1 Counter-regulatory Circuit Affords protection in the Mouse Brain Microcirculation. FASEB J. 21 (8), 1751–1758. doi:10.1096/fj.06-7842com
Gavins, F. N., and Hickey, M. J. (2012). Annexin A1 and the Regulation of Innate and Adaptive Immunity. Front. Immunol. 3 (NOV), 354. doi:10.3389/fimmu.2012.00354
Gavins, F. N., Hughes, E. L., Buss, N. A., Holloway, P. M., Getting, S. J., and Buckingham, J. C. (2012). Leukocyte Recruitment in the Brain in Sepsis: Involvement of the Annexin 1-FPR2/ALX Anti-inflammatory System. FASEB J. 26 (12), 4977–4989. doi:10.1096/fj.12-205971
Gavins, F. N., Yona, S., Kamal, A. M., Flower, R. J., and Perretti, M. (2003). Leukocyte Antiadhesive Actions of Annexin 1: ALXR- and FPR-Related Anti-inflammatory Mechanisms. Blood 101 (10), 4140–4147. doi:10.1182/blood-2002-11-3411
Gerke, V., and Moss, S. E. (2002). Annexins: from Structure to Function. Physiol. Rev. 82 (2), 331–371. doi:10.1152/physrev.00030.2001
Gheblawi, M., Wang, K., Viveiros, A., Nguyen, Q., Zhong, J. C., Turner, A. J., et al. (2020). Angiotensin-converting Enzyme 2: SARS-CoV-2 Receptor and Regulator of the Renin-Angiotensin System: Celebrating the 20th Anniversary of the Discovery of ACE2. Circ. Res. 126, 1456–1474. doi:10.1161/CIRCRESAHA.120.317015
Gidon-Jeangirard, C., Hugel, B., Holl, V., Toti, F., Laplanche, J. L., Meyer, D., et al. (1999). Annexin V Delays Apoptosis while Exerting an External Constraint Preventing the Release of CD4+ and PrPc+ Membrane Particles in a Human T Lymphocyte Model. J. Immunol. 162 (10), 5712–5718.
Gobbetti, T., Coldewey, S. M., Chen, J., McArthur, S., le Faouder, P., Cenac, N., et al. (2014). Nonredundant Protective Properties of FPR2/ALX in Polymicrobial Murine Sepsis. Proc. Natl. Acad. Sci. U S A. 111 (52), 18685–18690. doi:10.1073/pnas.1410938111
Greineder, C. F., Nelson, P. W., Dressel, A. L., Erba, H. P., and Younger, J. G. (2007). In Vitro and In Silico Analysis of Annexin V Binding to Lymphocytes as a Biomarker in Emergency Department Sepsis Studies. Acad. Emerg. Med. 14 (9), 763–771. doi:10.1197/j.aem.2007.01.017
Gu, C., Liu, M., Zhao, T., Zhai, L., and Wang, Y. (2015). Recombinant Human Annexin A5 Can Repair the Disrupted Cardiomyocyte Adherens Junctions in Endotoxemia. Shock 44 (1), 83–89. doi:10.1097/SHK.0000000000000370
Gu, C., Liu, M., Zhao, T., Zhai, L., and Wang, Y. (2015). Recombinant Human Annexin A5 Can Repair the Disrupted Cardiomyocyte Adherens Junctions in Endotoxemia. Shock 44 (1), 83–89. doi:10.1097/SHK.0000000000000370
Hajjar, K. A., and Krishnan, S. (1999). Annexin II: A Mediator of the Plasmin/plasminogen Activator System. Trends Cardiovasc. Med. 9 (5), 128–138. doi:10.1016/S1050-1738(99)00020-1
Hashimoto, K., Kim, H., Oishi, H., Chen, M., Iskender, I., Sakamoto, J., et al. (2016)., 151. Mosby, 861–869. doi:10.1016/j.jtcvs.2015.10.112Annexin V Homodimer Protects against Ischemia Reperfusion-Induced Acute Lung Injury in Lung TransplantationJ. Thorac. Cardiovasc. Surg.
He, S., Li, X., Li, R., Fang, L., Sun, L., Wang, Y., et al. (2016). Annexin A2 Modulates ROS and Impacts Inflammatory Response via IL-17 Signaling in Polymicrobial Sepsis Mice. PLOS Pathog. 12 (7), e1005743. doi:10.1371/journal.ppat.1005743
He, X., Zhang, W., Chang, Q., Su, Z., Gong, D., Zhou, Y., et al. (2019). A New Role for Host Annexin A2 in Establishing Bacterial Adhesion to Vascular Endothelial Cells: Lines of Evidence from Atomic Force Microscopy and an In Vivo Study. Lab. Invest. 99 (11), 1650–1660. doi:10.1038/s41374-019-0284-z
Hoffmann, M., Kleine-Weber, H., Schroeder, S., Krüger, N., Herrler, T., Erichsen, S., et al. (2020). SARS-CoV-2 Cell Entry Depends on ACE2 and TMPRSS2 and Is Blocked by a Clinically Proven Protease Inhibitor. Cell 181 (2), 271–e8. doi:10.1016/j.cell.2020.02.052
Hu, Z., Li, L., Zhu, B., Huang, Y., Wang, X., Lin, X., et al. (2020). Annexin A5 Is Essential for PKCθ Translocation during T-Cell Activation. J. Biol. Chem. 295 (41), 14214–14221. doi:10.1074/jbc.RA120.015143
Hunt, B. J., Wu, X. X., De Laat, B., Arslan, A. A., Stuart-Smith, S., and Rand, J. H. (2011). Resistance to Annexin A5 Anticoagulant Activity in Women with Histories for Obstetric Antiphospholipid Syndrome. Am. J. Obstet. Gynecol. 205 (5), 485–e17-23. doi:10.1016/j.ajog.2011.06.019
Jolly, C., Winfree, S., Hansen, B., and Steele-Mortimer, O. (2014). The Annexin A2/p11 Complex Is Required for Efficient Invasion of Salmonella typhimurium in Epithelial Cells. Cell Microbiol. 16 (1), 64–77. doi:10.1111/cmi.12180
Kartachova, M., Haas, R. L. M., Valdés Olmos, R. A., Hoebers, F. J. P., Van Zandwijk, N., and Verheij, M. (2004). In Vivo imaging of Apoptosis by 99mTc-Annexin V Scintigraphy: Visual Analysis in Relation to Treatment Response. Radiother. Oncol. 72, 333–339. doi:10.1016/j.radonc.2004.07.008
Kenis, H., Van Genderen, H., Deckers, N. M., Lux, P. A., Hofstra, L., Narula, J., et al. (2006). Annexin A5 Inhibits Engulfment through Internalization of PS-Expressing Cell Membrane Patches. Exp. Cel Res. 312 (6), 719–726. doi:10.1016/j.yexcr.2005.11.023
Kheifets, V., Bright, R., Inagaki, K., Schechtman, D., and Mochly-Rosen, D. (2006). Protein Kinase C delta (deltaPKC)-Annexin V Interaction: a Required Step in deltaPKC Translocation and Function. J. Biol. Chem. 281 (32), 23218–23226. doi:10.1074/jbc.M602075200
Kirschnek, S., Adams, C., and Gulbins, E. (2005). Annexin II Is a Novel Receptor for Pseudomonas aeruginosa. Biochem. Biophys. Res. Commun. 327 (3), 900–906. doi:10.1016/j.bbrc.2004.12.089
Koopman, G., Reutelingsperger, C. P., Kuijten, G. A., Keehnen, R. M., Pals, S. T., and van Oers, M. H. (1994). Annexin V for Flow Cytometric Detection of Phosphatidylserine Expression on B Cells Undergoing Apoptosis. Blood 84 (5), 1415–1420. doi:10.1182/blood.v84.5.1415.1415
Korngold, E. C., Jaffer, F. A., Weissleder, R., and Sosnovik, D. E. (2008). Noninvasive Imaging of Apoptosis in Cardiovascular Disease. Heart Fail. Rev. 13 (2), 163–173. doi:10.1007/s10741-007-9068-4
Kown, M. H., Strauss, H. W., Blankenberg, F. G., Berry, G. J., Stafford-Cecil, S., Tait, J. F., et al. (2001). In Vivo imaging of Acute Cardiac Rejection in Human Patients Using (99m)technetium Labeled Annexin V. Am. J. Transpl. 1 (3), 270–277. doi:10.1034/j.1600-6143.2001.001003270.x
Krikun, G., Lockwood, C. J., Wu, X. X., Zhou, X. D., Guller, S., Calandri, C., et al. (1994). The Expression of the Placental Anticoagulant Protein, Annexin V, by Villous Trophoblasts: Immunolocalization and In Vitro Regulation. Placenta 15 (6), 601–612. doi:10.1016/S0143-4004(05)80407-2
La, M., D'Amico, M., Bandiera, S., Di Filippo, C., Oliani, S. M., Gavins, F. N., et al. (2001). Annexin 1 Peptides Protect against Experimental Myocardial Ischemia-Reperfusion: Analysis of Their Mechanism of Action. FASEB J. 15 (12), 2247–2256. doi:10.1096/fj.01-0196com
Lanfranco, J., Chen, H., Harris, K., Almeida, D., Berlin, D., and Hajjar, K. (2013). Decreased Expression of Surface Annexin A2 in Human Sepsis Syndrome. Chest 144 (4), 408A. doi:10.1378/chest.1704251
Leoni, G., Alam, A., Neumann, P. A., Lambeth, J. D., Cheng, G., McCoy, J., et al. (2013). Annexin A1, Formyl Peptide Receptor, and NOX1 Orchestrate Epithelial Repair. J. Clin. Invest. 123 (23), 443–454. doi:10.1172/JCI65831DS1
Levi, M., Thachil, J., Iba, T., and Levy, J. H. (2020). Coagulation Abnormalities and Thrombosis in Patients with COVID-19. Lancet Haematol. 7 (6), e438–e440. doi:10.1016/S2352-3026(20)30145-9
Levi, M. (2020). Tocilizumab for Severe COVID-19: a Promising Intervention Affecting Inflammation and Coagulation. Eur. J. Intern. Med. 76, 21–22. doi:10.1016/j.ejim.2020.05.018
Ling, Q., Jacovina, A. T., Deora, A., Febbraio, M., Simantov, R., Silverstein, R. L., et al. (2004). Annexin II Regulates Fibrin Homeostasis and Neoangiogenesis In Vivo. J. Clin. Invest. 113 (1), 38–48. doi:10.1172/JCI19684
Liu, W., and Hajjar, K. A. (2016). The Annexin A2 System and Angiogenesis. Biol. Chem. 397 (10), 1005–1016. doi:10.1515/hsz-2016-0166
Liu, Y., Wang, Z., Jiang, M., Dai, L., Zhang, W., Wu, D., et al. (2011). Expression of Annexin II and Its Role in the Fibrinolytic Activity in Acute Promyelocytic Leukemia. Leuk. Res. 35 (7), 879–884. doi:10.1016/j.leukres.2010.11.008
Liu, Y., Yang, Y., Zhang, C., Huang, F., Wang, F., Yuan, J., et al. (2020). Clinical and Biochemical Indexes from 2019-nCoV Infected Patients Linked to Viral Loads and Lung Injury. Sci. China Life Sci. 63 (3), 364–374. doi:10.1007/s11427-020-1643-8
Lizarbe, M. A., Barrasa, J. I., Olmo, N., Gavilanes, F., and Turnay, J. (2013). Annexin-phospholipid Interactions. Functional Implications. Int. J. Mol. Sci. 14 (2), 2652–2683. doi:10.3390/ijms14022652
Luo, M., and Hajjar, K. A. (2013). Annexin A2 System in Human Biology: Cell Surface and beyond. Semin. Thromb. Hemost. 39 (4), 338–346. doi:10.1055/s-0033-1334143
Maderna, P., Yona, S., Perretti, M., and Godson, C. (2005). Modulation of Phagocytosis of Apoptotic Neutrophils by Supernatant from Dexamethasone-Treated Macrophages and Annexin-Derived Peptide Ac(2-26). J. Immunol. 174 (6), 3727–3733. doi:10.4049/jimmunol.174.6.3727
Magro, G. (2020). COVID-19: Review on Latest Available Drugs and Therapies against SARS-CoV-2. Coagulation and Inflammation Cross-Talking. Virus. Res. 286, 198070. doi:10.1016/j.virusres.2020.198070
Markoff, A., Bogdanova, N., Knop, M., Rüffer, C., Kenis, H., Lux, P., et al. (2007). Annexin A5 Interacts with Polycystin-1 and Interferes with the Polycystin-1 Stimulated Recruitment of E-Cadherin into Adherens Junctions. J. Mol. Biol. 369 (4), 954–966. doi:10.1016/j.jmb.2007.03.070
Menell, J. S., Cesarman, G. M., Jacovina, A. T., McLaughlin, M. A., Lev, E. A., and Hajjar, K. A. (1999). Annexin II and Bleeding in Acute Promyelocytic Leukemia. N. Engl. J. Med. 340 (13), 994–1004. doi:10.1056/NEJM199904013401303
Minghetti, L., Nicolini, A., Polazzi, E., Greco, A., Perretti, M., Parente, L., et al. (1999). Down-regulation of Microglial Cyclo-Oxygenase-2 and Inducible Nitric Oxide Synthase Expression by Lipocortin 1. Br. J. Pharmacol. 126 (6), 1307–1314. doi:10.1038/sj.bjp.0702423
Miyahara, A., Nakanishi, N., Ooka, T., Hayashi, T., Sugimoto, N., and Tobe, T. (2009). Enterohemorrhagic Escherichia coli Effector EspL2 Induces Actin Microfilament Aggregation through Annexin 2 Activation. Cel Microbiol. 11 (2), 337–350. doi:10.1111/j.1462-5822.2008.01256.x
Mochizuki, T., Kuge, Y., Zhao, S., Tsukamoto, E., Hosokawa, M., Strauss, H. W., et al. (2003). Detection of Apoptotic Tumor Response In Vivo after a Single Dose of Chemotherapy with 99mTc-Annexin V. J. Nucl. Med. 44 (1), 92–97.
Moss, S. E., and Morgan, R. O. (2004). The Annexins. Genome Biol. 5 (4), 219. doi:10.1186/gb-2004-5-4-219
Narula, J., Acio, E. R., Narula, N., Samuels, L. E., Fyfe, B., Wood, D., et al. (2001). Annexin-V Imaging for Noninvasive Detection of Cardiac Allograft Rejection. Nat. Med. 7 (12), 1347–1352. doi:10.1038/nm1201-1347
Olejnik, J., Hume, A. J., and Mühlberger, E. (2018). Toll-like Receptor 4 in Acute Viral Infection: Too Much of a Good Thing. Plos Pathog. 14 (12), e1007390. doi:10.1371/journal.ppat.1007390
Oudkerk, M., Büller, H. R., Kuijpers, D., van Es, N., Oudkerk, S. F., McLoud, T., et al. (2020). Diagnosis, Prevention, and Treatment of Thromboembolic Complications in COVID-19: Report of the National Institute for Public Health of the Netherlands. Radiology 297 (1), E216–E222. doi:10.1148/radiol.2020201629
Paar, V., Wernly, B., Zhou, Z., Motloch, L. J., Hoppe, U. C., Egle, A., et al. (2020). Anti-coagulation for COVID-19 Treatment: Both Anti-thrombotic and Anti-inflammatory? J. Thromb. Thrombolysis, 51 1–6. Published online July 6. doi:10.1007/s11239-020-02212-6
Park, J. H., Jang, J. H., Choi, E. J., Kim, Y. S., Lee, E. J., Jung, I. D., et al. (2016). Annexin A5 Increases Survival in Murine Sepsis Model by Inhibiting HMGB1-Mediated Pro-inflammation and Coagulation. Mol. Med. 22, 424–436. doi:10.2119/molmed.2016.00026
Possebon, L., Costa, S. S., Souza, H. R., Azevedo, L. R., Sant'Ana, M., Iyomasa-Pilon, M. M., et al. (2018). Mimetic Peptide AC2-26 of Annexin A1 as a Potential Therapeutic Agent to Treat COPD. Int. Immunopharmacol 63, 270–281. doi:10.1016/j.intimp.2018.08.011
Prompetchara, E., Ketloy, C., and Palaga, T. (2020). Immune Responses in COVID-19 and Potential Vaccines: Lessons Learned from SARS and MERS Epidemic. Asian Pac. J. Allergy Immunol. 38, 1–9. doi:10.12932/AP-200220-0772
Qin, C., Yang, Y. H., May, L., Gao, X., Stewart, A. G., Tu, Y., et al. (2015). Cardioprotective Potential of Annexin-A1 Mimetics in Myocardial Infarction. Pharmacol. Ther. 148, 47–65. doi:10.1016/j.pharmthera.2014.11.012
Rand, J. H., Wu, X. X., Andree, H. A., Lockwood, C. J., Guller, S., Scher, J., et al. (1997). Pregnancy Loss in the Antiphospholipid-Antibody Syndrome-Aa Possible Thrombogenic Mechanism. N. Engl. J. Med. 337 (3), 154–160. doi:10.1056/NEJM199707173370303
Rand, J. H., Wu, X. X., Lin, E. Y., Griffel, A., Gialanella, P., and McKitrick, J. C. (2012). Annexin A5 Binds to Lipopolysaccharide and Reduces its Endotoxin Activity. mBio 3 (2). doi:10.1128/mBio.00292-11
Rand, M. L., Wang, H., Pluthero, F. G., Stafford, A. R., Ni, R., Vaezzadeh, N., et al. (2012). Diannexin, an Annexin A5 Homodimer, Binds Phosphatidylserine with High Affinity and Is a Potent Inhibitor of Platelet-Mediated Events during Thrombus Formation. J. Thromb. Haemost. 10 (6), 1109–1119. doi:10.1111/j.1538-7836.2012.04716.x
Raynal, P., and Pollard, H. B. (1994). Annexins: the Problem of Assessing the Biological Role for a Gene Family of Multifunctional Calcium- and Phospholipid-Binding Proteins. Biochim. Biophys. Acta 1197 (1), 63–93. doi:10.1016/0304-4157(94)90019-1
Reutelingsperger, C. P. M., and Van Heerde, W. L. Annexin V, the Regulator of Phosphatidylserine-Catalyzed Inflammation and Coagulation during Apoptosis. Cell Mol. Life Sci. (Cmls) 1997, Vol 53(6):527–532. doi:10.1007/s000180050067
Rice, T. W., and Bernard, G. R. (2005). Therapeutic Intervention and Targets for Sepsis. Annu. Rev. Med. 56, 225–248. doi:10.1146/annurev.med.56.082103.104356
Ritchie, R. H., Sun, X., Bilszta, J. L., Gulluyan, L. M., and Dusting, G. J. (2003). Cardioprotective Actions of an N-Terminal Fragment of Annexin-1 in Rat Myocardium In Vitro. Eur. J. Pharmacol. 461 (2-3), 171–179. doi:10.1016/S0014-2999(03)01314-1
Rudd, K. E., Johnson, S. C., Agesa, K. M., Shackelford, K. A., Tsoi, D., Kievlan, D. R., et al. (2020). Global, Regional, and National Sepsis Incidence and Mortality, 1990-2017: Analysis for the Global Burden of Disease Study. Lancet 395 (10219), 200–211. doi:10.1016/S0140-6736(19)32989-7
Sanches, J. M., Branco, L. M., Duarte, G. H. B., Oliani, S. M., Bortoluci, K. R., Moreira, V., et al. (2020). Annexin A1 Regulates NLRP3 Inflammasome Activation and Modifies Lipid Release Profile in Isolated Peritoneal Macrophages. Cells 9 (4). doi:10.3390/cells9040926
Sanches, J. M., Branco, L. M., Duarte, G. H. B., Oliani, S. M., Bortoluci, K. R., Moreira, V., et al. (2020). Annexin A1 Regulates NLRP3 Inflammasome Activation and Modifies Lipid Release Profile in Isolated Peritoneal Macrophages. Cells 9 (4), 926. doi:10.3390/cells9040926
Sato, H., Konishi, Y., Tanaka, H., Takahashi, O., and Tanaka, T. (2004). Annexin V Inhibits Lipopolysaccharide-Induced Procoagulant Activity on Human Monocytes. Thromb. Res. 114 (1), 45–49. doi:10.1016/j.thromres.2004.04.012
Scannell, M., Flanagan, M. B., deStefani, A., Wynne, K. J., Cagney, G., Godson, C., et al. (2007). Annexin-1 and Peptide Derivatives Are Released by Apoptotic Cells and Stimulate Phagocytosis of Apoptotic Neutrophils by Macrophages. J. Immunol. 178 (7), 4595–4605. doi:10.4049/jimmunol.178.7.4595
Schloer, S., Pajonczyk, D., and Rescher, U. (2018). Annexins in Translational Research: Hidden Treasures to Be Found. Int. J. Mol. Sci. 19 (6). doi:10.3390/ijms19061781
Sheikh, M. H., and Solito, E. (2018). Annexin A1: Uncovering the many Talents of an Old Protein. Int. J. Mol. Sci. 19 (4). doi:10.3390/ijms19041045
Shen, X. D., Ke, B., Zhai, Y., Tsuchihashi, S. I., Gao, F., Duarte, S., et al. (2007). Diannexin, a Novel Annexin V Homodimer, Protects Rat Liver Transplants against Cold Ischemia-Reperfusion Injury. Am. J. Transpl. 7 (11), 2463–2471. doi:10.1111/j.1600-6143.2007.01967.x
Singer, M., Deutschman, C. S., Seymour, C. W., Shankar-Hari, M., Annane, D., Bauer, M., et al. (2016). The Third International Consensus Definitions for Sepsis and Septic Shock (Sepsis-3). JAMA 315 (8), 801–810. doi:10.1001/jama.2016.0287
Singh, R. M., Cummings, E., Pantos, C., and Singh, J. (2017). Protein Kinase C and Cardiac Dysfunction: a Review. Heart Fail. Rev. 22 (6), 843–859. doi:10.1007/s10741-017-9634-3
Solito, E., Kamal, A., Russo-Marie, F., Buckingham, J. C., Marullo, S., and Perretti, M. (2003). A Novel Calcium-dependent Proapoptotic Effect of Annexin 1 on Human Neutrophils. FASEB J. 17 (11), 1544–1546. doi:10.1096/fj.02-0941fje
Sundén-Cullberg, J., Norrby-Teglund, A., Rouhiainen, A., Rauvala, H., Herman, G., Tracey, K. J., et al. (2005). Persistent Elevation of High Mobility Group Box-1 Protein (HMGB1) in Patients with Severe Sepsis and Septic Shock*. Crit. Care Med. 33 (3), 564–573. doi:10.1097/01.CCM.0000155991.88802.4D
Tait, J. F., Smith, C., and Wood, B. L. (1999). Measurement of Phosphatidylserine Exposure in Leukocytes and Platelets by Whole-Blood Flow Cytometry with Annexin V. Blood Cell Mol Dis. 25 (5-6), 271–278. doi:10.1006/bcmd.1999.0254
Taylor, J. R., Skeate, J. G., and Kast, W. M. (2018). Annexin A2 in Virus Infection. Front. Microbiol. 9 (DEC), 2954. doi:10.3389/fmicb.2018.02954
Teoh, N. C., Ito, Y., Field, J., Bethea, N. W., Amr, D., McCuskey, M. K., et al. (2007). Diannexin, a Novel Annexin V Homodimer, Provides Prolonged protection against Hepatic Ischemia-Reperfusion Injury in Mice. Gastroenterology 133 (2), 632–646. doi:10.1053/j.gastro.2007.05.027
Thiagarajan, P., and Tait, J. F. (1991). Collagen-induced Exposure of Anionic Phospholipid in Platelets and Platelet-Derived Microparticles. J. Biol. Chem. 266 (36), 24302–24307. doi:10.1016/s0021-9258(18)54228-9
Thimister, P. W. L., Hofstra, L., Han Liem, I., Boersma, H. H., Kemerink, G., Reutelingsperger, C. P. M., et al. In Vivo Detection of Cell Death in the Area at Risk in Acute Myocardial Infarction. J. Nucl. Med. Vol 2003; 44 (3), 391–396.
Tomer, A., Bar-Lev, S., Fleisher, S., Shenkman, B., Friger, M., and Abu-Shakra, M. (2007). Antiphospholipid Antibody Syndrome: the Flow Cytometric Annexin A5 Competition Assay as a Diagnostic Tool. Br. J. Haematol. 139 (1), 113–120. doi:10.1111/j.1365-2141.2007.06751.x
Tontanahal, A., Arvidsson, I., and Karpman, D. (2021). Annexin Induces Cellular Uptake of Extracellular Vesicles and Delays Disease in Escherichia coli O157:H7 Infection. Microorganisms 9 (6), 1143. doi:10.3390/microorganisms9061143
Toufiq, M., Roelands, J., Alfaki, M., Syed Ahamed Kabeer, B., and Saadaou, M. (2020). Annexin A3 in Sepsis: Novel Perspectives from an Exploration of Public Transcriptome Data. Immunology 161 (4), 291–302. doi:10.1111/imm.13239
Tsapepas, D. S., Powell, J. T., Martin, S. T., Hardy, M. A., and Ratner, L. E. (2013). An Update to Managing Renal Transplant Ischemia Reperfusion Injury: Novel Therapies in the Pipeline. Clin. Transpl. 27 (5), n/a. doi:10.1111/ctr.12204
Tzelepis, F., Verway, M., Daoud, J., Gillard, J., Hassani-Ardakani, K., Dunn, J., et al. (2015). Annexin1 Regulates DC Efferocytosis and Cross-Presentation during Mycobacterium tuberculosis Infection. J. Clin. Invest. 125 (2), 752–768. doi:10.1172/JCI77014
Tzima, E., Trotter, P. J., Hastings, A. D., Orchard, M. A., and Walker, J. H. (2000). Investigation of the Relocation of Cytosolic Phospholipase A2 and Annexin V in Activated Platelets. Thromb. Res. 97 (6), 421–429. doi:10.1016/S0049-3848(99)00215-7
Tzima, E., and Walker, J. H. (2000). Platelet Annexin V: the Ins and Outs. Platelets 11 (5), 245–251. doi:10.1080/09537100050129251
van Genderen, H. O., Kenis, H., Hofstra, L., Narula, J., and Reutelingsperger, C. P. (2008). Extracellular Annexin A5: Functions of Phosphatidylserine-Binding and Two-Dimensional Crystallization. Biochim. Biophys. Acta 1783 (6), 953–963. doi:10.1016/j.bbamcr.2008.01.030
van Genderen, H. O., Kenis, H., Hofstra, L., Narula, J., and Reutelingsperger, C. P. (2008). Extracellular Annexin A5: Functions of Phosphatidylserine-Binding and Two-Dimensional Crystallization. Biochim. Biophys. Acta 1783 (6), 953–963. doi:10.1016/j.bbamcr.2008.01.030
Van Heerde, W. L., Poort, S., van 't Veer, C., Reutelingsperger, C. P., and De Groot, P. G. (1994). Binding of Recombinant Annexin V to Endothelial Cells: Effect of Annexin V Binding on Endothelial-Cell-Mediated Thrombin Formation. Biochem. J. 302 ( Pt 1) (1), 305–312. doi:10.1042/bj3020305
Van Tits, L., De Graaf, J., Toenhake, H., Van Heerde, W., and Stalenhoef, A. (2005). C-reactive Protein and Annexin A5 Bind to Distinct Sites of Negatively Charged Phospholipids Present in Oxidized Low-Density Lipoprotein. Arterioscler Thromb. Vasc. Biol. 25 (4), 717–722. doi:10.1161/01.ATV.0000157979.51673.2c
Varga, Z., Flammer, A. J., Steiger, P., Haberecker, M., Andermatt, R., Zinkernagel, A. S., et al. (2020). Endothelial Cell Infection and Endotheliitis in COVID-19. Lancet 395 (10234), 1417–1418. doi:10.1016/S0140-6736(20)30937-5
Wang, Y., Zhang, S., Luo, L., Norström, E., Braun, O. Ö., Mörgelin, M., et al. (2018). Platelet-derived Microparticles Regulates Thrombin Generation via Phophatidylserine in Abdominal Sepsis. J. Cel Physiol 233 (2), 1051–1060. doi:10.1002/jcp.25959
Wen, Y., Edelman, J. L., Kang, T., and Sachs, G. (1999). Lipocortin V May Function as a Signaling Protein for Vascular Endothelial Growth Factor receptor-2/Flk-1. Biochem. Biophys. Res. Commun. 258 (3), 713–721. doi:10.1006/bbrc.1999.0678
West, F., Lanfranco, J., Chen, H., Almeida, D., Berlin, D., and Hajjar, K. (2015). Annexin A2 Is Degraded in Human Mononuclear Cells in Sepsis. Chest 148 (4), 187A. doi:10.1378/chest.2262775
Wever, K. E., Wagener, F. A., Frielink, C., Boerman, O. C., Scheffer, G. J., Allison, A., et al. (2011). Diannexin Protects against Renal Ischemia Reperfusion Injury and Targets Phosphatidylserines in Ischemic Tissue. PLoS ONE 6 (8), e24276. doi:10.1371/journal.pone.0024276
Wiersinga, W. J., Rhodes, A., Cheng, A. C., Peacock, S. J., and Prescott, H. C. (2020). Pathophysiology, Transmission, Diagnosis, and Treatment of Coronavirus Disease 2019 (COVID-19): A Review. JAMA 324, 782–793. doi:10.1001/jama.2020.12839
Xu, F., Guo, M., Huang, W., Feng, L., Zhu, J., Luo, K., et al. (2020). Annexin A5 Regulates Hepatic Macrophage Polarization via Directly Targeting PKM2 and Ameliorates NASH. Redox Biol. 36, 101634. doi:10.1016/j.redox.2020.101634
Yap, J. K. Y., Moriyama, M., and Iwasaki, A. (2020). Inflammasomes and Pyroptosis as Therapeutic Targets for COVID-19. J. Immunol. 205 (2), 307–312. doi:10.4049/jimmunol.2000513
Yona, S., Heinsbroek, S. E., Peiser, L., Gordon, S., Perretti, M., and Flower, R. J. (2006). Impaired Phagocytic Mechanism in Annexin 1 Null Macrophages. Br. J. Pharmacol. 148 (4), 469–477. doi:10.1038/sj.bjp.0706730
Zhang, L., Zheng, Y. L., Hu, R. H., Zhu, L., Hu, C. C., Cheng, F., et al. (2018). Annexin A1 Mimetic Peptide AC2-26 Inhibits Sepsis-Induced Cardiomyocyte Apoptosis through LXA4/PI3K/AKT Signaling Pathway. Curr. Med. Sci. 38 (6), 997–1004. doi:10.1007/s11596-018-1975-1
Keywords: sepsis, COVID-19, annexin (A1, A2 and A5), inflammation, coagulation
Citation: Mui L, Martin CM, Tschirhart BJ and Feng Q (2021) Therapeutic Potential of Annexins in Sepsis and COVID-19. Front. Pharmacol. 12:735472. doi: 10.3389/fphar.2021.735472
Received: 05 July 2021; Accepted: 23 August 2021;
Published: 09 September 2021.
Edited by:
Gerard Bannenberg, Global Organization for EPA and DHA Omega-3s (GOED), United StatesReviewed by:
Ana Rodriguez, New York University, United StatesCopyright © 2021 Mui, Martin, Tschirhart and Feng. This is an open-access article distributed under the terms of the Creative Commons Attribution License (CC BY). The use, distribution or reproduction in other forums is permitted, provided the original author(s) and the copyright owner(s) are credited and that the original publication in this journal is cited, in accordance with accepted academic practice. No use, distribution or reproduction is permitted which does not comply with these terms.
*Correspondence: Claudio M. Martin, Y21hcnRpbjFAdXdvLmNh
Disclaimer: All claims expressed in this article are solely those of the authors and do not necessarily represent those of their affiliated organizations, or those of the publisher, the editors and the reviewers. Any product that may be evaluated in this article or claim that may be made by its manufacturer is not guaranteed or endorsed by the publisher.
Research integrity at Frontiers
Learn more about the work of our research integrity team to safeguard the quality of each article we publish.