- 1Department of Pharmacy, Banasthali Vidyapith, Banasthali, India
- 2Department of Chemistry, Banasthali Vidyapith, Banasthali, India
The multifaceted nature of the renin-angiotensin system (RAS) makes it versatile due to its involvement in pathogenesis of the cardiovascular disease. Angiotensin II (Ang II), a multifaceted member of RAS family is known to have various potential effects. The knowledge of this peptide has immensely ameliorated after meticulous research for decades. Several studies have evidenced angiotensin I receptor (AT1 R) to mediate the majority Ang II-regulated functions in the system. Functional crosstalk between AT1 R mediated signal transduction cascades and other signaling pathways has been recognized. The review will provide an up-to-date information and recent discoveries involved in Ang II receptor signal transduction and their functional significance in the cardiovascular system for potential translation in therapeutics. Moreover, the review also focuses on the role of stem cell-based therapies in the cardiovascular system.
Introduction
In the last decades, researchers have successfully unraveled key functions and mediators of the renin-angiotensin system (RAS). Ubiquitously available RAS plays numerous physiological roles including regulation of blood pressure, fluid volume, vascular wall integrity, cell growth, cardiac output, and vascular tone in the body (Forrester et al., 2018). RAS is also involved in maintaining cardiovascular homeostasis, a network of intracellular signaling pathways, and various processes through endocrine, paracrine, and autocrine mechanisms (Bussard and Buss, 2018). Regardless of complexities associated with its movement from the local system occurring virtually in each organ to the hormonal system existing in circulation, the active end product is still Angiotensin II (Ang II) (Colafella and Danser, 2017).
Historically, in 1898, renin was discovered as a pressor compound within the extracts of the renal cortex of rabbits by Robert Tigerstedt. Their work was renewed in 1934, when Henry Goldblatt demonstrated induction of chronic hypertension by constriction of renal arteries in a dog with silver clamps. In continuation to this, Page and Helmer and Braun-Menéndez et al., discovered angiotensin as another compound from renal secretion bearing quick pressor response. These studies focused on the involvement of Ang II in physiological and pathophysiological functions. Besides, RAS inhibiting agents have shown promising benefits in the management of end-organ damage, ischemia, atherosclerosis, and cardiovascular-related disease (Nehme, 2019). A timeline of key historical findings associated with the study and discovery of Ang II associated with RAS is shown in Table 1 (Burton et al., 1985; Gibbons, 1998; Basso and Terragno, 2001; Andrea et al., 2006; Atlas, 2007; Skrbic and Igic, 2009; Benigni et al., 2010).
In view of traditional applications, investigators are making a consistent effort to explore the associated pharmacological effects of Ang II. Unfortunately, it is hoped that the next 100 years of research into RAS will uncover hitherto unimaginable therapeutic opportunities (Ferrario, 2006). The review will provide recent findings on Ang II receptor signal transduction and its functional significance in the cardiovascular system. In addition to this, the review also focuses on the applications of stem cell-based therapies in the cardiovascular system. The majority of pathophysiological conditions including hypertension and cardiac remodeling of Ang II are mediated by AT1 R, which makes specific signaling pathways much clearer. In light of these facts the purpose of the present review is to provide newer insights in future research with an instinct that it will help emerging novel strategies to establish Ang II as a promising therapeutic candidate in translational research in the near future.
Method: Exclusion and Inclusion Criteria
The articles, written in English, published from 1985 to 2020, were exploited for gathering all relevant information of Ang II related articles from search databases namely, Science Direct, Medline/PubMed, Google Scholar, and other sources. Various databases were used to identify peer-reviewed papers dealing with the review theme of angiotensin-induced cardiovascular issues. A pilot review of literature assisted in identifying search terms that were used to categorize articles through a standardized and systematic process. The strings/words used for search purposes were as follows: “angiotensin”, “induced”, “receptor”, “signaling”, “disease”, “mediators”, “animal model”, “biomarkers”, “hypertrophic markers”, “cardiac genes”, “stem cells and others”.
Angiotensin II Receptors and Signaling Pathways
RAS involves different peptides with opposing biological effects. To sum up, the pro-inflammatory, pro-proliferative, and vasoconstrictive molecules are Ang II, AT1 R, and angiotensin-converting enzyme (ACE). Contrarily, AT2 R, ACE2, Ang (1–7), MrgD and MasR, exerts cardio-protective effects. In brief, angiotensinogen produced from the liver is converted into Ang I and Ang II via renin, esterase-2, cathepsin G, kallikrein, chymase, and angiotensin-converting enzyme. Ubiquitous actions of Ang II can be attributed to activation of several signal transduction pathways modulated by receptors including AT1 R and AT2 R to initiate RAS or further get cleaved into peptides namely, Ang IV, Ang (1–7), and alamandine, which express their effects via AT4 R, Mas R and MrgD, respectively (Adamcova et al., 2021; Matsubara, 1998). Interestingly, administration of Ang (1–7) was evidenced to provide a protective effect during chronic infusion of Ang II in rats (Grobe et al., 2007). However, the pharmacology of AT3 R and AT4 R has not been categorized fully and hence they are not definitively classified under mammalian Ang receptors (Figure 1) (Touyz and Berry, 2002). Based on several investigations, AT1 R and AT2 R have been evidenced to be associated with the majority of Ang II mediated signaling pathways (Kawai et al., 2017). AT1 R is clearly different from AT2 R in signalling mechanisms, tissue-specific expressions, and molecular weight. AT2 R may also counter-regulate functions mediated via AT1 R. However, the signaling mechanisms of AT2 R are still speculative compared with those of AT1 R. Moreover, most of the classic cardiovascular effects of Ang II are conveyed by AT1 R, including, vasoconstriction, hyperplasia, sodium retention, vascular cell hypertrophy, myocardial fibrosis, arterial wall thickening, aggravation of inflammatory responses, and stimulation of ROS.
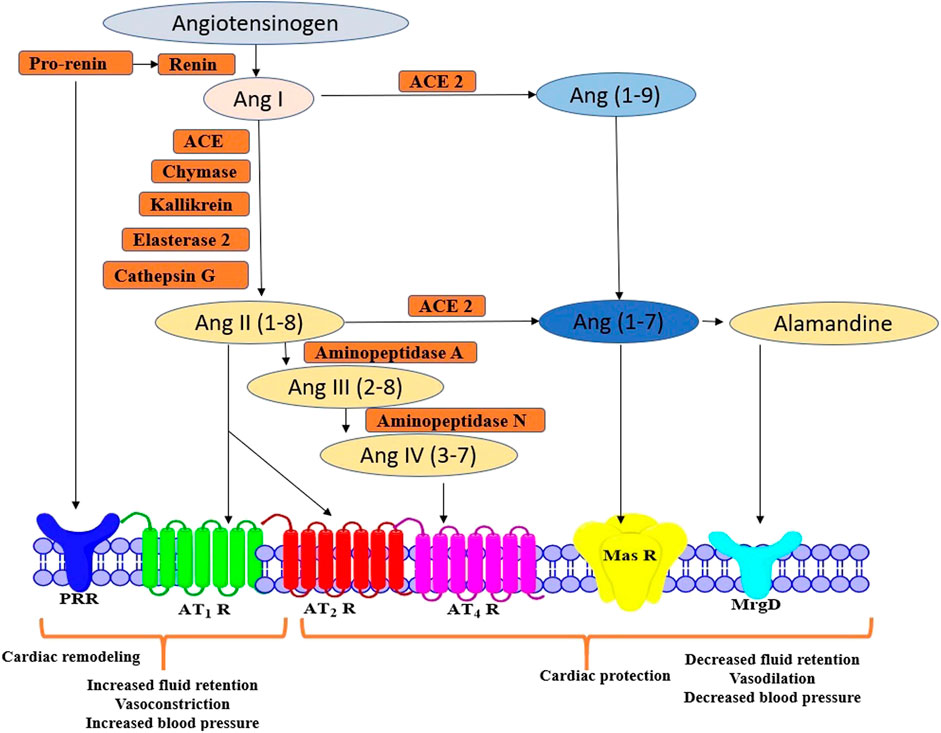
FIGURE 1. Schematic representation of Ang II peptides and receptors in RAS signalling pathway AT1 R, Angiotensin II Type 1 Receptor; AT2 R, Angiotensin II Type 2 Receptor; ANG II, angiotensin II; Ang-(1–7), Angiotensin-(1–7); Ang-(1–9), Angiotensin-(1–9); ACE2, Angiotensin-converting Enzyme 2; Mas R, Mitochondrial assembly protein Receptor.
AT1 R is a G protein-coupled receptor and is widely expressed in the heart, endothelium, smooth muscle, kidney (mainly in glomerulosa cells), brain, adipose tissue and adrenal glands (Li et al., 2012). AT1 R encourages intracellular pathways via activation of subunits of nicotinamide adenine dinucleotide phosphate (NADPH) oxidase, several protein kinases, transactivation of growth factor receptor, or direct interaction with AT1 R interacting proteins like Guanine nucleotide exchange factor (GEF)-like protein (GLP), AT1 R associated protein (AT1 R AP), phospholipase C (PLC γ1) and Janus activated kinase (JAK 2) (Figure 2). In addition, it also turns on several downstream signals, like mitogen-activated protein kinase/extracellular signal-regulated kinases (MAPK/ERK), Ras/Rho, and translocation of MAPK in the nucleus (Ahmadian et al., 2015). AT1 R of mouse and rat exists as Ang II type I subtype A receptor (AT1A R) and Ang II type I subtype B receptor (AT1B R), which have similar activation, ligand binding properties, and identical amino acid sequences, but differ in tissue transcriptional and distributive regulation. AT1A R is widely expressed and regulate blood pressure. Thus, it is anticipated to be the closest homolog to the human AT1 R (Touyz and Berry, 2002).
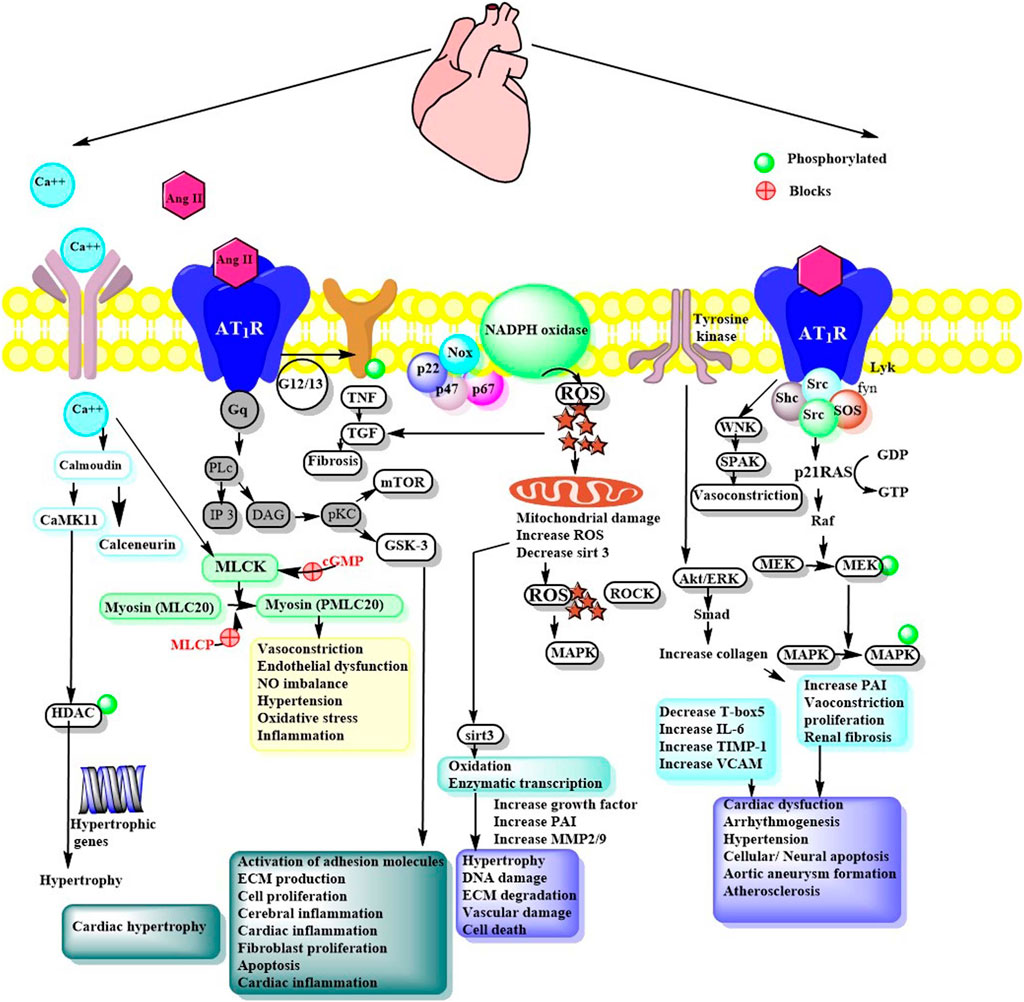
FIGURE 2. Ang II signaling via AT1R mediated pathways. Ang II, Angiotensin II; Ca++, Calcium; CaMKII, Ca2+/calmodulin-dependent protein kinase II; NFAT, Nuclear factor of activated T-cells; HDAC, Histone deacetylases; PLc, phospholipase C; IP3, Ionositol triphosphate; DAG, Diacyl glycerol; pKc, Protein kinase; CaMKII, Calcium/calmodulin- dependent protein kinase-II; NFAT, Nuclear factor of activated T-cells; MLCK, Myosin light chain kinase; MAPK, Mitogen activated protein kinase; PAI, Plasminogen activator inhibitor; NFKB, Nuclear factor-kB; MMP, Matrix metalloproteinase; TIMP, Tissue inhibitor metalloproteinase; VCAM, Vascular cell adhesion molecule; PVN, Paraventricular nucleus; ECM, Extracellular matrix; HDAC, Histone deacetylase; GTP, GDP, Nucleotides; Akt, Protein kinase B; cGMP, Guanosine 3’,5’ cyclic monophosphate; ROS, Reactive oxygen species; Grb2, Growth factor receptor bound protein 2; TGF, Tissue growth factor; PLC, phospholipase C; mTOR, mammalian target of rapamycin; GSK, Glycogen synthase kinase; TNF, tumor necrosis factor; Sirt: Sirtuin; MMP, Matrix metalloprotease; ERK, extracellular-signal-regulated kinase; WNK, lysine deficient protein kinase 1; SPAK, SPS1-related proline/alanine-rich serine-threonine kinase.
G-Protein, Protein Kinases, Nicotinamide Adenine Dinucleotide Phosphate , and Growth Factor-Mediated Signaling
Ang II activation of AT1 R promotes variously convoluted, convergent, and diverse signaling pathways. However, research has established specific components essential for Ang II dependent signaling pathways. In brief, AT1 R binds with heteromeric G-protein (Gq/11, Gi, G12, and G13) and allows activation of secondary messengers such as Rho GEFs, PLCβ, inositol 1,4,5-trisphosphate (IP3), diacyl glycerol (DAG), and reactive oxygen species (ROS). This further regulates downstream effectors like phospholipases. The response may differ depending on effector tissue, such as in vascular smooth muscle cells (VSMCs) contraction is regulated through G12/13 Rho/Rho kinase-mediated myosin light chain phosphatase (MLCP) inhibition or Gq/11 Ca2+sensitive myosin light chain kinase (MLCK) activation. Similarly, Src family kinase also regulates vascular contraction through MLCP inhibition and Rho kinase/RhoA signaling. Ang II mediated AT1 R potentiates various serine/threonine kinases such as PKC, Akt, and mitogen-activated protein kinase (MAPK) family kinases and other intracellular protein kinases like, non-receptor and receptor tyrosine kinases (Figure 2 and Table 2).
Ang II stimulates nicotinamide adenine dinucleotide phosphate (NADPH) oxidases to produce ROS causing renal deterioration, cardiac hypertrophy, and VSMC migration. In general, Ang II increases the production of ROS via activation of the catalytic subunit of NADPH, the Nox family proteins. The catalytic subunits of NADPH include dual oxidase (Duox1 and Duox2) and the Nox family (Nox1-Nox5). Subsequent stimulation of Nox family proteins increases its interactions with associated specific regulatory subunits p67 phox, p47 phox, p22 phox, and Nox1 (Figure 2) (Kawai et al., 2017). Ang II stimulated Nox4 generation in vascular cells and renal tissues via AT1 R is a source of oxidative stress, hypertension and organ failure. Ang II upregulates JNK and Nox4 in BubR1 (budding uninhibited by benzimidazole-related 1) low-expression mice (Aoyagi et al., 2019). Moreover, it stimulates the expression of AT1 R and receptors for advanced glycation end products (RAGE) by stimulating the PKC-ERK-NF-κB signaling pathway. In addition, it can increase intracellular ROS and critical mediators of cardiomyocyte hypertrophy by regulating expression levels of NADPH oxidase 2/4 in H9c2 cells (Lee et al., 2020).
Ang II mediated transactivation of nonreceptor tyrosine kinase (Janus kinase, c-Src, and focal adhesion kinase) and tyrosine kinase (EGF, PDGF, IR, etc.) resulted in modulation of various downstream targets like MAPK (Vukelic and Griendling, 2014). It can act as a growth factor that can regulate cell hypertrophy in VSMCs. Investigations have established dynamic phenomenon for AT1 R dependent transactivation of growth factor receptor-like EGF receptor (EGFR), followed by subsequent activation of Akt/p70S6, mechanistic target of rapamycin (mTOR), and Ras/ERK signaling resulting in cardiac hypertrophy and fibrosis (Eguchi et al., 1999; Eguchi et al., 2001; Ohtsu et al., 2006). Ang II stimulates selective mTOR2-dependent phosphorylation of SGK1 but not Akt (Gleason et al., 2019). The transactivation is mediated by secondary messengers like ROS, PKC, Src kinase, and metalloproteinase-dependent release of EGFR ligands such heparin-binding EGF, TGF- α, and EGF. Peng et al., 2016 evidenced that c-Src dependent EGFR transactivation in ERK/Akt pathway may a play crucial role in Ang II induced cardiac remodeling in H9c2 cells (Peng et al., 2016). AT1 R mediated A Disintegrin And Metalloproteinase 17 (ADAM17) dependent EGFR activation results in VSMC migration and hypertrophy via PI3K/Akt/mTOR/p70S6K pathway and Ras/ERK pathway. Ang II mediated ADAM17 requires ROS and p38 MAPK phosphorylation. Zhang Y. et al. (2019) demonstrated that Ang II promotes Mer tyrosine kinase shedding via AT1R/ROS/p38 MAPK/ADAM17 pathway in macrophages of ApoE−/− mice (Zhang Y. et al., 2019). Furthermore, BMX (bone marrow kinase), a non-receptor tyrosine kinase has been identified as an upstream signalling molecule for Ang II-mediated EGFR activation. Thus, systemic inhibition of EGFR or ADAM17 decreases Ang II-induced cell migration and aortic aneurysm.
Ang II-dependent connective tissue growth factors (CTGF) and transforming growth factor-β (TGF-β) are initial pro-fibrotic mediators involved in cardiac fibrosis (Wong et al., 2018; van Beusekom and Zimmering, 2019). The expression of CTGF and TGF-β are interlinked. Ang II increases mRNA expression of TGF-β and NF-κβ, an important mediator of the hypertrophic growth of the heart, in H9c2 cells (Prathapan et al., 2013). Further, myocardial CTGF expression after Ang II exposure is likely dependent on latent activation of TGF-β via canonical Smad-pathway in NIH/3T3 fibroblasts (Wong et al., 2018). Overexpression of fibroblast growth factor 23 (FGF23) augmented cardiac fibrosis and hypertrophy in Ang II administered mice via PPARα/PLCγ-NFAT1/TGF-β signaling (Liu et al., 2020). Contrarily, FGF21 enhances cardiac function and reduces Ang II induced cardiac hypertrophy through in silent information regulator 1 (SIRT1)/adenosine monophosphate-activated protein kinase (AMPK) pathway (Li S et al., 2019). In addition, Ang II is well known to transactivate insulin-like growth factor I (IGF-I) receptor (IGF-IR) and platelet-derived growth factor (PDGF) receptor (PDGFR) in VSMC (Du et al., 1996). However, unlike EGFR, research on the role of Ang II-mediated IGF-IR, TGF, and PDGFR in cardiovascular pathophysiology is still limited.
Ang II-induced signaling via AT1 R is correlated with MAPK activation and enhanced phosphorylation of protein tyrosine. This fact highlights that besides vasoconstriction, Ang II also possesses the inflammatory and mitogenic properties. Similar to AT1 R, the existence of AT2 R is also opting for increased attraction due to its opposite effect than the former. AT2 R also belongs to the GPCR family and stimulates the SH2 domain-containing phosphatase (SHP-1) and MAPK phophatase1 (MKP-1) resulting in attenuation of tyrosine phosphorylation. In addition, AT2 R accelerates vasorelaxation through PKA-dependent eNOS activation and paracrine signaling through bradykinin/cGMP/NO production. Considering AT2 R, some AT2 R interacting proteins have shown physiological roles in the suppression of tumors, inflammation, ROS production and hypertrophy.
G-Protein Independent Signaling via β-arrestin
Ang II stimulated AT1 R can activate various signaling cascades such as G-protein independent and G-protein dependent signaling. Unlike G-protein dependent signaling, the G-protein independent signal transduction cascade includes G-protein and β-arrestin (Forrester et al., 2018). Isoforms of β-arrestin i. e., β-arrestin 1 and 2 are recruited to AT1 R and stabilize them with high-affinity conformations (Sanni et al., 2010). Mechanistically, β-arrestin were described as a protein that uncouples GPCR from G-protein for mediating receptor internalization and G-protein independent signaling. β-arrestin mediated signaling includes activation of p38 and Akt/MAPK, JUNK, ERK1/2, and Src tyrosine kinases. A study involving human embryonic kidney (HEK)-293 cells biased agonist Sar1, Ire4, Ile8-ANG II (SII) or a mutant AT1 R-DRY/AAY suggested various active conformation of AT1 R. The SII or mutant AT1 R induced G protein independent, but β-arrestin 2-dependent ERK activation (Wei et al., 2003).
AT1 R, both β-arrestin and Gq/11 proteins mediate biased signaling (Ferraino et al., 2021). β-arrestin-biased AT1 R signaling promotes vascular remodeling with the activation of MAPK and Src-based signaling. Interestingly, mechanical activation of AT1 R caused increased affinity toward β-arrestin biased ligand TRV 120023, suggesting stabilization of a biased active receptor conformation (Ma et al., 2021). Apart from its involvement in β-arrestin-dependent signaling, AT1 R was also reported to be involved in stretch-induced pathways in different cells. Interestingly, GRK2 and PKC, the kinase responsible for β-arrestin binding of many GPCRs have also been found to be activated up on a stretch in rat ventricular myocytes (Turu et al., 2019). In a study, the vasoconstrictor responses were increased by Gq/11 AT1 R biased agonists TRV120055 and TRV20056. Here, Gq/11 AT1 R was an essential component of dynamic mechanochemical signaling in VSMC causing myogenic tone (Cui et al., 2020). Alongwith AT1 R, AT2 R is also suggested to be primarily stimulated via G-protein independent signal transduction cascade including β-arrestin and GPCR kinase.
Angiotensin II in Cardiovascular System
As a vital bioactive peptide of RAS, Ang II is associated with diverse mechanistic insights into understanding how Ang II contributes to multiple cardiovascular physiology and pathophysiology functions. Century-old research on RAS has uncovered Ang II and its involvement in the pathophysiology of cardiovascular diseases. Ang II is involved in the regulation of cell communication, impulse propagation, cardiac contractility, apoptosis, growth, and remodeling (Kawai et al., 2017). Summary of in vivo and in vitro pharmacological investigations are presented in Figure 2 and Table 3. In most of the in-vivo studies, the approach used for induction was a subcutaneous infusion of Ang II.
Angiotensin II in Hypertension
Hypertension is one of the most critical predisposing factors for the development of cardiovascular disease. Several factors contributing to the pathogenesis of hypertension include salt intake, stress, and Ang II (Kulkarni et al., 1998; Meneton et al., 2005). Ang II as a powerful vasoconstricting agent can induce aldosterone secretion and thereby retention of salt and water, which regulates blood pressure. In addition to this, Ang II has also been shown to have important oxidative, inflammatory, and immune-mediated actions. Ang II even at doses that do not alter blood pressure, allows the migration of inflammatory cells and induces the expression of inflammatory markers in the aorta of normotensive mice (Lima et al., 2019)
Nair et al. in 2015 found toll-like receptor 4 (TLR4), high-mobility group box 1 (HMGB1), and proinflammatory cytokines mediated immune response contributed to Ang II-induced hypertension in rat tubular epithelial cell line NRK52E (Nair et al., 2015). In support of this concept, they designed another study to determine the involvement of HMGB1 signaling in Ang II-induced hypertension in the para ventricular nucleus (PVN). The interaction between the inflammatory cytokine protein, HMGB1, and TLR4, resulted in the up-regulation of NF-kB which in turn resulted in hypertension in Neuro-2a cells of mice treated with Ang II (Nair and Philips, 2015). These findings were consistent with reports by Li et al. (2016) and Yu et al., 2015 that showed Ang II increases hypertension and hypothalamic infiltration via TLR4/MyD88/NF-κB signaling pathway and peroxisome proliferator-activated receptor-γ (PPAR-γ) in the PVN in hypertensive rats (Yu et al., 2015; Li et al., 2016).
In addition to, TLR4 and PPAR-γ, Ang II is known to induce ROS via activation of NADPH oxidase mediated Nox-1 and p22phox in Ang II-induced DNA damage in-vivo and in-vitro models (Wattanapitayakul et al., 2000; Sarr et al., 2006; Zimnol et al., 2020). Hyperactivation of Nox acts as a major source of ROS production in cardiac tissue, promotes apoptosis and increases oxidative stress via the MAPK pathway (Wen et al., 2019). Ogola et al., 2019 evidenced that pretreatment with G protein-coupled estrogen receptor (GPER) agonist G1 inhibits Ang II-induced ROS, NADPH, Nox4 mRNA expression via cAMP and phosphodiesterase inhibition (Ogola et al., 2019). Moreover, Ang II also induces pressor response and vasoconstriction by reduction of an active form of Ras-related C3 botulinum toxin substrate 1 (Rac1) and Nrf2 nuclear translocation (Pepe et al., 2019). Rac, a small G protein is an essential molecule for the function of NADPH oxidase components along with, phosphorylated Smad 2/3, atrial TGF-β1, and atrial superoxide in Ang II hypertensive rats (Yagi et al., 2010).
Another enzyme source of ROS includes uncoupled nitric oxide synthase (NOS), endoplasmic reticulum oxidase, xanthine oxidase, and mitochondrial oxidase. ROS affects the function of a cell by modifying proteins through post-translation modifications such as phosphorylation and oxidation (carbamylation, glutathionylation, nitrosylation, and sulfenylation). Proteins that are affected include matric metalloproteinases, cytoskeletal structural protein, transcriptional factors, signaling molecules, and ion transporter receptors (Griendling et al., 2016). ROS activate all 3 members of the MAPK family, including JNK, p38MAPK, and ERK1/2, essential for regulating vascular and cardiac cells (Jennings et al., 2010). In a study, catechins were reported to inhibit Ang II-induced VSMC proliferation by inhibiting Ang II activated MAPK and activator protein-1 signaling pathways (Won et al., 2006) (Figure 2). MEK-ERK are phosphorylated in arteries of hypertensive individuals and in a mouse model. Activation of this pathway results in the promotion of human arterial SMC (HASMCs). A known cysteine protease, Cathepsin L/V is interdependent on extracellular matrix accumulation and tissue inflammatory responses, allowing regulation of arterial remodeling. Lu et al., 2020 showed that Z-FF-FMK, a cathepsin inhibitor significantly reduces MEK-ERK phosphorylation (Lu et al., 2020).
Also, Ang II is responsible for causing atherosclerosis through VCAM 1 activation via protease-dependent NF-κB-like transcriptional mechanisms (Tummala et al., 1999). Acute doses of Ang II act primarily on VSMC to reduce blood pressure whereas chronic infusion of Ang II is neutrally mediated (∼10 h) (Li et al., 1996). It has been reported that statins reduce the incidence of cardiovascular remodeling. It is well known that apart from cholesterol-lowering, it also provides pleiotropic effects on the cardiovascular system, including anti-oxidant, anti-inflammatory, and improvement of endothelial function (Yagi et al., 2010). Candesartan, an AT1 R blocker and apocynin, NADPH oxidase inhibitor evidenced reduced pressor effect by AT1R-dependent ROS-SAPK/JNK, ERK1/2, and p38MAPK signaling (Jiang et al., 2019). Likewise, Pitavastatin exerts eNOS based protective action in Ang II-induced cardiovascular remodeling through suppression of (TGF)-β1–Smad 2/3 signaling pathway and oxidative stress (Yagi et al., 2010). Ang II inhibition extensively improved hypertension, hyperfiltration and control renal damage. Inhibition of Ang II enhanced the NF-KB activity which may additionally result in inhibition of its downstream gene expression, particularly NADPH-oxidase.
Angiotensin II in Cardiac Remodeling
Cardiac remodeling can be described as a pathologic or physiologic condition that may occur after volume overload or idiopathic dilated cardiomyopathy, inflammatory heart muscle disease, pressure overload, or myocardial infarction (Cohn et al., 2000). Investigations have shown that Ang II promotes excessive accumulation of collagen leading to cardiac dysfunction as well as cardiac remodeling (Du et al., 2019). Several mechanisms have been implicated in the pathogenesis of Ang II-induced cardiac remodeling including dysfunction, hypertrophy, apoptosis and fibrosis. Ang II has been closely related to remodeling, which acts mainly via AT1 R in the animal and human cardiovascular systems. PKCs-EKR1/2-NFκB-NLRP3-IL1β pathway signaling cascades have been shown to promote Ang II-induced cardiomyocyte hypertrophy in H9c2 cells through AT1 R, RAGE, and NADPH oxidase inhibition (Lee et al., 2020). Soluble RAGE (sRAGE) was demonstrated as a decoy receptor for RAGE in Ang II-induced cardiomyocyte hypertrophy using in vivo and real-time 9.4T MR imaging (Heo et al., 2019). In addition to RAGE, it has been noted that Toll-like receptor 2 (TLR2)- and TLR4-dependent pathways are stimulated by Ang II in cardiac dysfunction, fibrosis and hypertrophy (Lee et al., 2020). TLR4 is involved in the upregulation of monocyte chemoattractant protein (MCP-1), IL-6, and ROS (Matsuda et al., 2015). Ang II stimulated direct binding of STAT3 with TLR4 activates STAT3 via IL-6/glycoprotein 130/JAK 2pathway, resulting in altered gene regulation for cardiac remodeling (Han et al., 2018).
Similarly, TGF-β has been proposed to act in a paracrine/autocrine manner between fibroblast and cardiomyocytes to stimulate cardiac remodeling (Leask, 2010). Valsartan, angiotensin receptor blocker, or Stachydrine mediated inhibition of Ang II/AT1 R/TGF-β signaling is a pivotal mechanism of anti-hypertrophic and anti-fibrotic effect (Teekakirikul et al., 2010; Liu et al., 2019; Tashiro et al., 2020). Ang II-induced fibrosis is associated with altered expression of inflammation-related genes such as TGF-β, TNF-α, MCP-1, IL-6, and type 3 collagen (Azushima et al., 2019).
miRNA including miR-154, miR-155, miR-132, miR-21, miR-503, miR-214, miR-19a, and miR-410 are involved in promoting hypertrophy, fibrosis, apoptosis, and inflammation. On other hand, miR-16, miR-98, miR-30a, miR-133, miR-433 possess cardio-protective effects (Wang Q. et al., 2019; Song et al., 2019; Adamcova et al., 2021). Luciferase assay evidenced that miR-214 acts as a target for Long non-coding RNA (lncRNA) Plscr4 and ameliorated levels of miR-214 oppose the anti-hypertrophic effect of Plscr4 in Ang II treated cardiomyocytes via lncRNA Plscr4-miR-214-mitofusin (Mfn2) axis (Lv et al., 2018). Ang II down-regulated expression of Neuregulin-1 (NRG-1) as a member of the epidermal growth factor family via the circNRG-1/miR-193b-5p-mediated post-transcriptional mechanism in mouse aortic smooth muscle cells (MASMCs) (Sun et al., 2019). Interestingly, the antiaging gene klotho modifies Ang II-induced cardiac remodeling via altering the expression of TGF-β and miR-132, a downstream mediator of TGF-β. LY364947, a TGF-β and klotho gene inhibitor, inhibited fibrosis, hypertrophy, expression of fibrotic marker genes (α-SMA, collagen I), pro-hypertrophic genes (atrial natriuretic peptide (ANP), brain natriuretic peptide (BNP), β-myosin heavy chain (β-MHC)) and Smad2/3 phosphorylation in cultured cardiomyocytes, fibroblasts and heart tissue (Ding et al., 2019). Zheng et al. evidenced similar results that liraglutide, a glucagon-like peptide-1 (GLP-1) receptor agonist, reduced protein levels of Smad2/3/4, AT1 R, TGF-β, collagen I and III and upregulated Smad7 in Ang II-induced fibrosis in rats (Zheng et al., 2019). Transient receptor potential subfamily M member 7 (TRPM7) is also implicated in cardiac fibrosis. Ang II-induced expression of p-Smad and collagen synthesis is inhibited by depletion of TRPM7 in cultured cardiac fibroblasts in rat sick sinus syndrome model (Zhong H et al., 2018).
p38 and MAPK pathways also play a crucial role in extracellular matrix deposition and cell proliferation during hypertensive cardiovascular remodeling (Jing et al., 2011; Jiajing et al., 2020). ERK represents MAPK and is involved in the growth and proliferation of VSMC. However, p38 MAPK inhibitor, PI3K inhibitor, and the c-JNK inhibitor didnot affect arterial blood pressure or renal sympathetic nerve activity in heart failure (Shinohara et al., 2015) (Figure 2). LCZ696, an AT1 R blocker, attenuated the hypertrophic expression of ANP, βMHC, and TIMP2 in Ang II-induced remodeling in cardiomyocyte and collagen I, collagen III, and TGF-β in cardiac fibroblasts via ERK inhibition (Wang Y. et al., 2019). A potent angiogenesis inhibitor, endostatin reduced the levels of ANP and BNP in primary neonatal rat cardiomyocytes and Ang II-treated rats via the cAMP/PKA pathway (Dai et al., 2019). Similar results were observed by ghrelin, a gut peptide that decreased Ang II-induced cardiac fibrosis by inhibiting TGF-β1 and upregulating PPAR-γ in rats. Also, it inhibits smooth muscle cell proliferation stimulated by angiotensin II by preventing cAMP/PKA signaling (Wang et al., 2018).
Astonishingly, a recent study suggested that PUO4F2/Brn-3b transcription factor acts as a novel regulator of adaptive hypertrophic responses in the adult heart along with other markers such as, ANP/βMHC. Levels of Brn-3b mRNA and related proteins are increased in Ang II treated mouse hearts and foetal heart-derived H9c2 cells or primary cultures of neonatal rat ventricular myocytes with varied hypertrophic alterations. Alternate signaling pathways involved in activation of Brn-3b promoter include p42/p44 MAPK/ERK1/2 or calcineurin pathways (Mele et al., 2019; Cheng et al., 2020). Cardiac fibrosis is induced by the pro (renin) receptor, which could be more worsened by the involvement PRR-ERK1/2-NOX4 pathway and ROS during the development of alcoholic cardiomyopathy (Cao et al., 2019). Ang II-induced collagen accumulation in cardiac fibroblast stimulates oxidative stress, ROS and inflammation via NF-κB/MAPK and nuclear factor erythroid 2-related factor (Nrf2)/AMPK pathway (Su et al., 2018; Du et al., 2019). Fibrotic proteins including, matrix metalloproteinases, collagen, and TGF are overexpressed in presence of Ang II through Akt/PI3K and NF-κB/MAPK pathway (Wang L. et al., 2019).
Despite the growing interest in big data approaches, with the aim of studying the genetics of cardiovascular disease, fishes are becoming an increasingly popular choice to study associated genetic alterations. To clarify the putative actions of Ang II in cardiac remodeling, Anguilla Anguilla was selected. Immunoblotting and immunolocalization results suggested that Ang II downregulates both localization and expression of molecules affecting apoptosis and cell growth such as eNOS, heat shock protein-90, and c-kit (Imbrogno et al., 2010; Imbrogno et al., 2013; Filice et al., 2017). Another recent study demonstrated the effect of Ang II on morpho-functional remodeling in heart of Danio rerio. The findings were paralleled by the upregulation of AT1 R and AT2 R expressions. Moreover, a significant change in expression of cytochrome b-245β polypeptide protein, superoxide dismutase 1 soluble mRNAs, NFk-light polypeptide gene enhancer in B cell, and GATA binding protein, indicated cardiac remodeling (Filice et al., 2021).
Angiotensin II in Stem Cell Therapy in Cardiovascular System
Recent investigations have endeavored to improve stem cell functionality, provide stem cells as a promising therapeutic candidate for tissue transplantations in the cardiovascular system. Evolving research has evidenced the influence of RAS on stem cell growth, function, and proliferation (Becher et al., 2011). Several studies have demonstrated the role of Ang II in the differentiation of progenitor cell/stem cell (Matsushita et al., 2006). The presence of AT1 R in differentiated, but not in undifferentiated cells, suggests the concept that Ang II can regulate differentiation of stem cells (Huang et al., 2007). In addition to influencing a different kind of stem cells, the RAS effect on cardiovascular-related stem cell transplantation has largely been evaluated regarding the intracellular pathways of Ang II.
Mesenchymal stem cells (MSCs) are of great significance, along with their various autocrine-paracrine effects on the cardiovascular system and immune system (Zhang et al., 2020). Local RAS component Ang II has been reported to be expressed in rat MSCs. Vascular endothelial growth factor (VEGF) has been recognized in an invasion of extracellular matrix, migration, proliferation and survival of MSCs. Pre-treatment with Ang II increases mRNA expression of VEGF in MSCs through Akt/ERK1/2 signaling pathway via AT1 R. Considering this, pre-treatment of MSCs with LY292002, an Akt inhibitor attenuates Ang II-induced expression of VEGF. Notably, the involvement of Ang II increases the expression/production of VEGF in MSC grafts and improves transplantation efficiency (Liu et al., 2014). Thus, an angiogenic function of Ang II stimulates cells in ischemic regions through VEGF-induced endothelial nitric oxide synthase (eNOS). Ang II induces cardiomyogenic differentiation of rat bone marrow MSCs more efficiently than TGF-β1. The autocrine TGF-β/Smad pathway makes the differentiation of adipose tissue-derived MSCs to SMCs. Also, it acts synergistically with VEGF to ameliorate the differentiation of bone marrow-derived MSCs into endothelial cells (Ikhapoh et al., 2015).
Cardiac hypertrophy is a phenotypic response of the heart associated with various disorders. The genetic factor is an important determinant of phenotypic expression in hypertrophy (Marian, 2008). Neuron-derived orphan receptor-1 (NOR-1) transgenesis upregulates key genes involved in cardiac hypertrophy (Myh7, encoding for β-myosin heavy chain (β-MHC)) and fibrosis (Loxl2, encoding for the ECM modifying enzyme, Loxl2) in Ang II-induced cardiomyocytes (Cañes et al., 2020). In another study, Ang II and TGF-β1 upregulated the expression of the structured cardiomyocytes genes such as DES, TNNT2 and MYH6 as well as main cardiac genes-markers like GATA4, TBX5, and NKX2-5. Also, an increased expression of cardiac ion channel genes is evidenced with Ang II and TGF-β1 in human amniotic fluid-derived MSCs (AF-MSCs). Ang II and TGF-β1 treated AF-MSCs showed an increase in connexin43 protein and Nkx2.5 protein in AF-MSCs (Gasiūnienė et al., 2019). MSC can be an effective route for refining cell-based therapy of angiogenesis, vascular stabilization, and endothelial cell survival (Yang et al., 2019). Besides, TGF-β secretion is associated with the MAPK/ERK pathway; and Ang II in this pathway interferes with TGF-β production.
Bone marrow is one of the major Ang II producing organs and participant in the regulation of immunity and hematopoiesis (Yamashita et al., 2020). Being an inducer of differentiation of MSC, Ang II at doses ranged 0.1 to 10 μM can regulate apoptosis. Ang II could increase mitochondrial ROS through the activation of Nox2. In a study, Ang II at the dose of 1 and 10 μM leads to apoptosis in bone marrow-derived MSCs due to mitochondrial ROS production and mitochondrial DNA leakage mediated via AT1 R. Treatment with AT1 R inhibitor, losartan, markedly inhibited the Ang II-induced apoptosis and mitochondrial ROS (Zhang F. et al., 2019). Ang II production by cleaving enzyme chymase is several times higher in bone marrow than in other tissues. In a study, flow cytometry results showed Ang II generated via a chymase-dependent pathway in bone marrow was 280 -fold higher than in the heart. CD68+ myeloid progenitor possesses higher chymase expression than CD68− progenitor cell in bone marrow (Yamashita et al., 2020). Another study showed that AT1a R was widely expressed by human bone marrow CD34+, CD38− cells, and lymphocytes (Rodgers et al., 2000). Ang II, but not Ang (1–7), increased adhesion of MNCs or CD34+ cells to fibronectin via ACE2/Ang-(1–7)/Mas pathway (Singh et al., 2015). The reported pathway stimulates vasoprotective functions of CD34+ cells.
Ang II inhibits colony growth by myeloid progenitors in a dose-dependent manner via AT1 R (Jokubaitis et al., 2008). Depletion of myeloid cells reduced vascular expression of AT1 R, adhesion molecule and vascular accumulation of oxidative stress, endothelial dysfunction, and Nox2+CD45+ cells (Molitor et al., 2021). Bone marrow-derived fibroblast precursors express certain chemokine receptors, such as CCR2, CCR5, CCR7, and CXCR4. Treatment of wild- type mice with Ang II (1,500 ng/kg/min) caused accumulation of bone marrow-derived fibroblast precursor expressing CD45, CD34, hematopoietic markers, collagen I, and mesenchymal markers. Whereas, the produced effects were abolished in CCR2 deficient mice depicting its role in the pathogenesis of Ang II-induced cardiac fibrosis (Xu et al., 2011). Everolimus, a rapamycin blocker, inhibited Ang II-induced aneurysm in ApoE−/− mice through diminished M1 polarization and suppressed the development of bone marrow CCR2 monocytes (Moore et al., 2015). Ishibashi et al. evidenced that, MCP-1 regulates monocyte-mediated inflammation through leukocyte derived CCR2 receptor (C-C chemokine receptor) and its deficiency can reduce the pathogenic effect in Ang II (1.9 mg/kg per day, s. .c, 4 weeks) induced atherosclerosis and aneurysm in ApoE−/− CCR2−/− and ApoE−/− CCR2+/+ mice (Ishibashi et al., 2004).
Autologous bone marrow MSCs are effective for regression of aneurysms in Ang II-induced ApoE−/− mice (Akita et al., 2019). Bone marrow MSCs derived conditioned medium could prevent aneurysm growth through macrophage polarization regulation (Zhou et al., 2019). In addition, precursor fibronectin type III domain-containing protein 5 or irisin, a novel myokine has the potential to improve bone marrow MSC mediated paracrine effect and engraftments in infarcted hearts (Deng et al., 2020). Previous studies have shown that MSC-derived exosomes have distinct properties, including immunomodulation, angiogenesis, and paracrine effect that protect organ functions in animal studies. Additionally, recent investigations have evidenced that adipose-derived MSCs (ADMSCs) derived exosomes also possess a capacity of immunomodulatory, cardioprotective, and anti-inflammatory effects. Interestingly, irbesartan, an AT1 R blocker, was shown to abolish the effects of ADMSC-derived cell sheets in a rat model (Yamamoto et al., 2018). Administration of miR-19a/19b (exo/miR-19a/19b) using bone marrow-derived MSCs to cardiac HL-1 cells significantly suppressed the apoptosis and fibrosis in infarcted hearts (Wang S. et al., 2020).
Human-induced pluripotent stem cells (iPSCs) possess unique features to differentiate and self-renew into different types of cells in the body (Johansson et al., 2020). They are artificially derived from adult differentiated non-pluripotent somatic cells. In particular, these human cells-based models are anticipated to become an alternative for animal models. iPSCs can express Ang II receptors. As evidenced, Ang II induces the proliferation of PSC and allows their differentiation into MSCs. Treatment of PSC with Tempol, a ROS inhibitor, and Ang II reduces the cell proliferation and DNA synthesis, indicating the involvement of ROS signaling (Ahmadian et al., 2015). The presence and activation of the JAK/STAT pathway play a crucial role in stem cell renewal. It causes p38 phosphorylation and eventually, results in the differentiation of iPSCs in the target cell. A study showed that Ang II could stimulate hypertrophy in human embryonic stem cells (hESC)- and iPSCs derived cardiomyocytes (Földes et al., 2011). However, no significant effect was observed in cell death after treatment of Ang II (200 nM) with hESC- and iPSCs derived cardiomyocytes (Nunes et al., 2017). Immunofluorescent study and RNA seq revealed the involvement of low AT1 R expression in iPSC cardiomyocytes. Long-term Ang II incubation up-regulates AT2 R expression to induce downstream apoptosis signaling in iPSC-derived cardiomyocytes (Gao J. et al., 2020). Whereas, short-term Ang II treatment reduces Young modulus in rat cardiomyocytes via AT1 R. Inhibition using Rho-kinase or TGF-β1 abolished this effect (Swiatlowska et al., 2020).
Erythropoiesis is a tightly regulated process reinforced by systematic hematopoietic progenitor cells and some cohort of multipotent hematopoietic stem cells (HSCs) at its apex. HSCs form the basis of widely practiced therapies, including bone marrow transplantation and stem cell therapies (Jokubaitis et al., 2008). Chronic Ang II infusion imparts important regulatory roles on HSC proliferation, differentiation, and engraftment at the level of bone marrow (Kim et al., 2016) Flow cytometry analysis has revealed the involvement of Ang II in the regulation of hematopoiesis in HSCs and granulocyte/monocyte progenitor cells but, not in megakaryocytes/erythroid progenitors when coculture with stromal S17 cells. (Costa et al., 2021). In addition, protein microsequencing has also reported an increase in Gr-1+/Mac-1+ cells, BB9 protein and decrease expression of Ki67+ in presence of Ang II (Jokubaitis et al., 2008; Costa et al., 2021).
Conclusion and Future Prospects
In the last decades, there has been a noteworthy progression in the number of molecules targeting Ang II signaling pathways. Since the discovery of Ang II, it has been characterized to be involved in various cellular activities such as proliferation, contractility, apoptosis, dysfunctions, remodeling, etc. Dysregulated Ang II signaling is considered to induce various cardiovascular diseases involving hypertension, inflammation, myocardial infarction, atherosclerosis, fibrillation, ventricular dystrophies, etc. While many ARBs are available commercially, the statistics indicate the need to look for novel mechanisms of Ang II causing cardiovascular diseases independently, which would provide a therapeutic target. The involvement of the RAS component in cardiovascular tissue and progenitor cells may regulate development and growth; and thus, allow the preparation of cardiac progenitor cells for clinical transplantation. Being a multi-functional peptide, Ang II stands amidst varied systems integrating multiple cellular signaling events that broadly have counterregulatory or opposing actions. The turmoil in the balance of such processes can lead to diverse pathologies and dysfunctions. Understanding how these processes are integrated with each other in real-time and remain a formidable challenge. Further studies with transgenics or cell-specific knockouts are needed to unravel the role of inflammatory and hypertrophic markers in Ang II-induced remodeling. Another challenge is providing evidence for working with cardiovascular progenitor cells for clinical transplantation. Further, the role of epigenetic/genetic programming impacting the cardiovascular system is an emerging area of study. Many questions still remain as well regarding paracrine, autocrine, and intracrine actions of Ang II and its interaction with receptor-associated proteins. In addition, an approach that integrates elements of metabolomics, proteomics, epigenomics, and genomics is likely to reveal novel, as yet unforeseen facet of Ang II signal transduction. Understanding diverse regulatory signaling mechanisms of Ang II can, therefore, offer better insight into various pathological states which in turn may help in designing ideal drug candidates for their management.
Author Contributions
KV: Writing and drafting review MP: Compilation and design SP: Supervise and editing JD: Manuscript Checking SS: Conceptualization and manuscript Checking.
Conflict of Interest
The authors declare that the research was conducted in the absence of any commercial or financial relationships that could be construed as a potential conflict of interest.
Publisher’s Note
All claims expressed in this article are solely those of the authors and do not necessarily represent those of their affiliated organizations, or those of the publisher, the editors and the reviewers. Any product that may be evaluated in this article, or claim that may be made by its manufacturer, is not guaranteed or endorsed by the publisher.
Acknowledgments
Authors are highly thankful to thankful to Prof. Aditya Shastri, Vice Chancellor, Banasthali Vidyapith, Banasthali, Rajasthan India for providing pivotal support and facilities for successful compilation of work.
References
Adamcova, M., Kawano, I., and Simko, F. (2021). The Impact of microRNAs in Renin-Angiotensin-System-Induced Cardiac Remodelling. Int. J. Mol. Sci. 22 (9), 4762. doi:10.3390/ijms22094762
Ahmadian, E., Jafari, S., and Yari Khosroushahi, A. (2015). Role of Angiotensin II in Stem Cell Therapy of Cardiac Disease. J. Renin Angiotensin Aldosterone Syst. 16 (4), 702–711. doi:10.1177/1470320315621225
Akita, N., Narita, Y., Ogata, A., Usui, A., and Komori, K. (2019). Therapeutic Potential of Allogenic Bone Marrow-Derived Mesenchymal Stem Cells for Aortic Aneurysm. Eur. J. Vasc. Endovascular Surg. 58 (6), e189–e190. doi:10.1016/j.ejvs.2019.06.755
An, S., Cho, S. Y., Kang, J., Lee, S., Kim, H. S., Min, D. J., et al. (2020). Inhibition of 3-phosphoinositide–dependent Protein Kinase 1 (PDK1) Can Revert Cellular Senescence in Human Dermal Fibroblasts. Proc. Natl. Acad. Sci. U S A. 117(49), 31535–31546. doi:10.1073/pnas.1920338117
Andrea, H., Markus, P., Bernd, K., Nada, C., Roland, V., and Karl, F. H. (2006). Angiotensin II Formation in the Kidney and Nephrosclerosis in Ren-2 Hypertensive Rats. Nephrol. Dial. Transpl. 21, 1778–1785. doi:10.1093/ndt/gfl065
Aoyagi, Y., Furuyama, T., Inoue, K., Matsuda, D., Matsubara, Y., Okahara, A., et al. (2019). Attenuation of Angiotensin II–Induced Hypertension in BubR1 Low‐expression Mice via Repression of Angiotensin II Receptor 1 Overexpression. J. Am. Heart Assoc. 8 (23), e011911. doi:10.1161/JAHA.118.011911
Atlas, S. A. (2007). Goodman & Gillman’s the Pharmacological Basics of Therapeutics. New York: Mc Graw Hills, 669–787. doi:10.1007/978-3-540-47210-0_22
Azushima, K., Uneda, K., Wakui, H., Ohki, K., Haruhara, K., Kobayashi, R., et al. (2019). Effects of Rikkunshito on Renal Fibrosis and Inflammation in Angiotensin II-Infused Mice. Sci. Rep. 9 (1), 1–10. doi:10.1038/s41598-019-42657-1
Bai, L., Kee, H. J., Choi, S. Y., Seok, Y. M., Kim, G. R., Kee, S. J., et al. (2021). HDAC5 Inhibition Reduces Angiotensin II-Induced Vascular Contraction, Hypertrophy, and Oxidative Stress in a Mouse Model. Biomed. Pharmacother. 134, 111162. doi:10.1016/j.biopha.2020.111162
Basso, N., and Terragno, N. A. (2001). History about the Discovery of the Renin-Angiotensin System. Hypertension 38, 1246–1249. doi:10.1161/hy1201.101214
Batchu, S. N., Hughson, A., Wadosky, K. M., Morrell, C. N., Fowell, D. J., and Korshunov, V. A. (2016). Role of Axl in T-Lymphocyte Survival in Salt-dependent Hypertension. Arterioscler. Thromb. Vasc. Biol. 36 (8), 1638–1646. doi:10.1161/ATVBAHA.116.307848
Becher, U. M., Endtmann, C., Tiyerili, V., Nickenig, G., and Werner, N. (2011). Endothelial Damage and Regeneration: the Role of the Renin-Angiotensin-Aldosterone System. Curr. Hyper. Rep. 13 (1), 86–92. doi:10.1007/s11906-010-0171-x
Benigni, P., Cassis, P., and Remuzzi, G. (2010). Angiotensin II Revisited: New Roles in Inflammation, Immunology and Aging. EMBO. Mol. Med. 2, 247–257. doi:10.1002/emmm.201000080
Berk, B. C., and Corson, M. A. (1997). Angiotensin II Signal Transduction in Vascular Smooth Muscle: Role of Tyrosine Kinases. Cir. Res. 80 (5), 607–616. doi:10.1161/01.res.80.5.607
Brinks, H. L., and Eckhart, A. D. (2010). Regulation of GPCR Signaling in Hypertension. Biochim. Biophys. Acta Mol. Basis Dis. 1802 (12), 1268–1275. doi:10.1016/j.bbadis.2010.01.005
Brown, A., Azlan, N. F. M., Wu, Z., and Zhang, J. (2021). WNK-SPAK/OSR1-NCC Kinase Signaling Pathway as a Novel Target for the Treatment of Salt-Sensitive Hypertension. Acta Pharmacol. Sinica. 42 (4), 508–517. doi:10.1038/s41401-020-0474-7
Burton, M. A., Gray, B. N., Self, G. W., Heggie, J. C., and Townsend, P. S. (1985). Manipulation of Experimental Rat and Rabbit Liver Tumor Blood Flow with Angiotensin II. Cancer Res. 45, 5390–5393. doi:10.1007/BF00177353
Bussard, R. L., and Buss, L. W. (2018). Angiotensin II: a New Therapeutic Option for Vasodilatory Shock. Ther. Clin. Risk Manag. 14, 287–1298. doi:10.2147/TCRM.S150434
Callera, G. E., Antunes, T. T., He, Y., Montezano, A. C., Yogi, A., Savoia, C., et al. (2016). c-Src Inhibition Improves Cardiovascular Function but Not Remodeling or Fibrosis in Angiotensin II–Induced Hypertension. Hyperten 68 (5), 1179–1190. doi:10.1161/HYPERTENSIONAHA.116.07699
Cañes, L., Martí-Pàmies, I., Ballester-Servera, C., Herraiz-Martínez, A., Alonso, J., Galán, M., et al. (2020). Neuron-derived Orphan Receptor-1 Modulates Cardiac Gene Expression and Exacerbates Angiotensin II-Induced Cardiac Hypertrophy. Clin. Sci. 134 (3), 359–377. doi:10.1042/CS20191014
Cao, X., Yu, S., Wang, Y., Yang, M., Xiong, J., Yuan, H., et al. (2019). Effects of the (Pro)renin Receptor on Cardiac Remodeling and Function in a Rat Alcoholic Cardiomyopathy Model via the PRR-Erk1/2-NOX4 Pathway. Oxid. Med. Cel. Longev. 2019, 4546975. doi:10.1155/2019/4546975
Cao, Y., Li, L., Liu, Y., Chen, G., Tao, Z., Wang, R., et al. (2021). I-κB Kinase-ε Deficiency Attenuates the Development of Angiotensin II-Induced Myocardial Hypertrophy in Mice. Oxid. Med. Cel. Longev. 2021, 6429197. doi:10.1155/2021/6429197
Chen, Y., Ge, Z., Huang, S., Zhou, L., Zhai, C., Chen, Y., et al. (2020). Delphinidin Attenuates Pathological Cardiac Hypertrophy via the AMPK/NOX/MAPK Signaling Pathway. Aging 12 (6), 5362–5383. doi:10.18632/aging.102956
Cheng, H., Li, J., Wu, Q., Zheng, X., Gao, Y., Yang, Q., et al. (2020). Effect of SKF-96365 on Cardiomyocyte Hypertrophy Induced by Angiotensin II. Mol. Med. Rep. 21 (2), 806–814. doi:10.3892/mmr.2019.10877
Cheng, Y., Shen, A., Wu, X., Shen, Z., Chen, X., Li, J., et al. (2021). Qingda Granule Attenuates Angiotensin II-Induced Cardiac Hypertrophy and Apoptosis and Modulates the PI3K/AKT Pathway. Biomed. Pharmacother. 133, 111022. doi:10.1016/j.biopha.2020.111022
Cohn, J. N., Ferrari, R., and Sharpe, N. (2000). Cardiac Remodeling—Concepts and Clinical Implications: a Consensus Paper from an International Forum on Cardiac Remodeling. J. Am. Coll. Cardiol. 35 (3), 569–582. doi:10.1016/s0735-1097(99)00630-0
Colafella, K. M. M., and Danser, A. H. J. (2017). Recent Advances in Angiotensin Research. Hypertension 69, 994–999. doi:10.1161/HYPERTENSIONAHA.117.08931
Costa, M. M., Stilhano, R. S., Oliveira, C. R., Barbosa, C. M., Pereira, G. J., Paredes‐Gamero, E. J., et al. (2021). Angiotensin II Modulates the Murine Hematopoietic Stem Cell and Progenitors Cocultured with Stromal S17 Cells. Cell Biol. Int. 45 (7), 1459–1467. doi:10.1002/cbin.11584
Cui, Y., Kassmann, M., Nickel, S., Zhang, C., Alenina, N., Anistan, Y. M., et al. (2020). Myogenic Vasoconstriction Requires Canonical Gq/11 Signaling of the Angiotensin II Type 1a Receptor in the Murine Vasculature. bioRxiv. doi:10.1101/2020.09.09.289280
Dai, Y. J., Gong, J. X., and Bian, R. (2019). Effect of Endostatin Overexpression on Angiotensin II-Induced Cardiac Hypertrophy in Rats. Chin. Med. J. 132 (22), 2716. doi:10.1097/CM9.0000000000000513
Deng, J., Zhang, N., Wang, Y., Yang, C., Wang, Y., Xin, C., et al. (2020). FNDC5/irisin Improves the Therapeutic Efficacy of Bone Marrow-Derived Mesenchymal Stem Cells for Myocardial Infarction. Stem Cel Res. Ther. 11 (1), 1–15. doi:10.21203/rs.3.rs-16784/v310.1186/s13287-020-01746-z
Ding, J., Tang, Q., Luo, B., Zhang, L., Lin, L., Han, L., et al. (2019). Klotho Inhibits Angiotensin II-Induced Cardiac Hypertrophy, Fibrosis, and Dysfunction in Mice through Suppression of Transforming Growth Factor-β1 Signaling Pathway. Eur. J. Pharmacol. 859, 172549. doi:10.1016/j.ejphar.2019.172549
Du, J., Sperling, L. S., Marrero, M. B., Phillips, L., and Delafontaine, P. (1996). G-protein and Tyrosine Kinase Receptor Cross-Talk in Rat Aortic Smooth Muscle Cells: Thrombin-And Angiotensin II-Induced Tyrosine Phosphorylation of Insulin Receptor Substrate-1 and Insulin-like Growth Factor 1 Receptor. Biochem. Biophys. Res. Commun. 218 (3), 934–939. doi:10.1006/bbrc.1996.0165
Du, Y., Han, J., Zhang, H., Xu, J., Jiang, L., and Ge, W. (2019). Kaempferol Prevents against Ang II-Induced Cardiac Remodeling through Attenuating Ang II-Induced Inflammation and Oxidative Stress. J. Cardiovas. Pharmacol. 74 (4), 326. doi:10.1097/FJC.0000000000000713
Eguchi, S., Iwasaki, H., Ueno, H., Frank, G. D., Motley, E. D, Eguchi, K., et al. (1999). Intracellular Signaling of Angiotensin II-Induced P70 S6 Kinase Phosphorylation at Ser411 in Vascular Smooth Muscle Cells: Possible Requirement of Epidermal Growth Factor Receptor, Ras, Extracellular Signal-Regulated Kinase and AKT. J. Bio. Chem. 274 (52), 36843–36851. doi:10.1074/jbc.274.52.36843
Eguchi, S., Dempsey, P. J., Frank, G. D., Motley, E. D., and Inagami, T. (2001). Activation of MAPKs by Angiotensin II in Vascular Smooth Muscle Cells: Metalloprotease-dependent EGF Receptor Activation Is Required for Activation of ERK and P38 MAPK but Not for JNK. J. Bio. Chem. 276 (11), 7957–7962. doi:10.1074/jbc.M008570200
Fang, P. P., Pan, C. W., Lin, W., Li, J., Huang, S. S., Zhou, G. Y., et al. (2020). ASK1 Enhances Angiotensin II-Induced Liver Fibrosis In Vitro by Mediating Endoplasmic Reticulum Stress-dependent Exosomes. Mediators Inflamm. 2020, 8183713. doi:10.1155/2020/8183713
Ferraino, K. E., Cora, N., Pollard, C. M., Sizova, A., Maning, J., and Lymperopoulos, A. (2021). Adrenal Angiotensin II Type 1 Receptor Biased Signaling: The Case for “Biased” Inverse Agonism for Effective Aldosterone Suppression. Cell. Signal. 82, 109967. doi:10.1016/j.cellsig.2021.109967
Ferrario, C. M. (2006). Role of Angiotensin II in Cardiovascular Disease—Therapeutic Implications of More Than a century of Research. J. Renin Angio. Aldo. S. 7, 3–14. doi:10.3317/jraas.2006.003
Filice, M., Amelio, D., Garofalo, F., David, S., Fucarino, A., and Jensen, F. B. (2017). Angiotensin II Dependent Cardiac Remodeling in the Eel Anguilla anguilla Involves the NOS/NO System. Nitric Oxide 65, 50–59. doi:10.1016/j.niox.2017.02.007
Filice, M., Barca, A., Amelio, D., Leo, S., Mazzei, A., Del Vecchio, G., et al. (2021). Morpho-functional Remodelling of the Adult Zebrafish (Danio rerio) Heart in Response to Waterborne Angiotensin II Exposure. Gen. Comp. Endocrinol. 301, 113663. doi:10.1016/j.ygcen.2020.113663
Földes, G., Mioulane, M., Wright, J. S., Liu, A. Q., Novak, P., Merkely, B., et al. (2011). Modulation of Human Embryonic Stem Cell-Derived Cardiomyocyte Growth: a Testbed for Studying Human Cardiac Hypertrophy? J. Mol. Cel. Cardiol. 50 (2), 367–376. doi:10.1016/j.yjmcc.2010.10.029
Forrester, S. J., Steven, W. B., Curt, D. S., Thomas, M. C., Tatsuo, K., Victor, R., et al. (2018). Angiotensin II Signal Transduction: an Update on Mechanisms of Physiology and Pathophysiology. Physiol. Rev. 98, 1627–1738. doi:10.1152/physrev.00038.2017
Gao, J., Wei, T., Huang, C., Sun, M., and Shen, W. (2020). Sirtuin 3 Governs Autophagy‐dependent Glycolysis during Angiotensin II‐induced Endothelial‐to‐mesenchymal Transition. FASEB J. 34 (12), 16645–16661. doi:10.1096/fj.202001494R
Gao, Q., Wang, P., Wu, Z., Qiu, H., Lin, B., Chen, J., et al. (2020). Angiotensin II Induces Apoptosis in Human Induced Pluripotent Stem Cell-Derived Cardiomyocytes. bioRxiv. doi:10.1101/2020.03.19.998344
Gao, Y., Zhao, D., Xie, W. Z., Meng, T., Xu, C., Liu, Y., et al. (2021). Rap1GAP Mediates Angiotensin II-Induced Cardiomyocyte Hypertrophy by Inhibiting Autophagy and Increasing Oxidative Stress. Oxid. Med. Cel. Longev. 2021. 7848027. doi:10.1155/2021/7848027
Gasiūnienė, M., Petkus, G., Matuzevičius, D., Navakauskas, D., and Navakauskienė, R. (2019). Angiotensin II and TGF-β1 Induce Alterations in Human Amniotic Fluid-Derived Mesenchymal Stem Cells Leading to Cardiomyogenic Differentiation Initiation. Int. J. Stem Cell 12 (2), 251. doi:10.15283/ijsc18126
Ge, W., Hou, C., Zhang, W., Guo, X., Gao, P., Song, X., et al. (2021). Mep1a Contributes to Ang II-Induced Cardiac Remodeling by Promoting Cardiac Hypertrophy, Fibrosis and Inflammation. J. Mol. Cel. Cardiol. 152, 52–68. doi:10.1016/j.yjmcc.2020.11.015
Gibbons, G. H. (1998). The Pathophysiology of Hypertension: the Importance of Angiotensin II in Cardiovascular Remodelling. Am. J. Hypertens. 11, 177S–181S. doi:10.1016/s0895-7061(98)00198-8
Gleason, C. E., Oses-Prieto, J. A., Li, K. H., Saha, B., Situ, G., Burlingame, A. L., et al. (2019). Phosphorylation at Distinct Subcellular Locations Underlies Specificity in mTORC2-Mediated Activation of SGK1 and Akt. J. Cel Sci. 132 (7), jcs224931. doi:10.1242/jcs.224931
González-Núñez, M., Riolobos, A. S., Castellano, O., Fuentes-Calvo, I., de los Ángeles Sevilla, M., Oujo, B., et al. (2015). Heterozygous Disruption of Activin Receptor-like Kinase 1 Is Associated with Increased Arterial Pressure in Mice. Dis. Model. Mech. 8 (11), 1427–1439. doi:10.1242/dmm.019695
Griendling, K. K., Touyz, R. M., Zweier, J. L., Dikalov, S, Chilian, W, Chen, Y. R., et al. (2016). Measurement of Reactive Oxygen Species, Reactive Nitrogen Species, and Redox-dependent Signaling in the Cardiovascular System: a Scientific Statement from the American Heart Association. Circ. Res. 1, 39–75. doi:10.1161/RES.0000000000000110
Grobe, J. L., Mecca, A. P., Lingis, M., Shenoy, V., Bolton, T. A., Machado, J. M., et al. (2007). Prevention of Angiotensin II-Induced Cardiac Remodeling by Angiotensin-(1–7). Am. J. Physiol. Heart Circ. Physiol. 292 (2), 736–742. doi:10.1152/ajpheart.00937.2006
Han, J., Ye, S., Zou, C., Chen, T., Wang, J., Li, J., et al. (2018). Angiotensin II Causes Biphasic STAT3 Activation through TLR4 to Initiate Cardiac Remodeling. Hypertension 72 (6), 1301–1311. doi:10.1161/HYPERTENSIONAHA.118.11860
Heo, D., Lim, S., Lee, J., Lee, M. E., Cho, S., Jeong, J., et al. (2019). Radiological Assessment of Effectiveness of Soluble RAGE in Attenuating Angiotensin II-Induced LVH Mouse Model Using In Vivo 9.4 T MRI. Scientific Rep. 9 (1), 1–10. doi:10.1038/s41598-019-44933-6
Holopainen, T., Räsänen, M., Anisimov, A., Tuomainen, T., Zheng, W., Tvorogov, D., et al. (2015). Endothelial Bmx Tyrosine Kinase Activity Is Essential for Myocardial Hypertrophy and Remodeling. Proc. Natl. Acad. Sci. U S A. 112 (42), 13063–13068. doi:10.1073/pnas.1517810112
Huang, Z., Yu, J., Toselli, P., Bhawan, J., Sudireddy, V., Taylor, L., et al. (2007). Angiotensin II Type 1 and Bradykinin B2 Receptors Expressed in Early-Stage Epithelial Cells Derived from Human Embryonic Stem Cells. J. Cell. Physiol. 211 (3), 816–825. doi:10.1002/jcp.20985
Ikhapoh, I. A., Pelham, C. J., and Agrawal, D. K. (2015). Synergistic Effect of Angiotensin II on Vascular Endothelial Growth Factor-A-Mediated Differentiation of Bone Marrow-Derived Mesenchymal Stem Cells into Endothelial Cells. Stem Cel. Res. Ther. 6 (1), 1–13. doi:10.1186/scrt538
Imbrogno, S., Garofalo, F., Cerra, M. C., Mahata, S. K., and Tota, B. (2010). The Catecholamine Release-Inhibitory Peptide Catestatin (Chromogranin A344-364) Modulates Myocardial Function in Fish. J. Exp. Bio. 213 (21), 3636–3643. doi:10.1242/jeb.045567
Imbrogno, S., Garofalo, F., Amelio, D., Capria, C., and Cerra, M. C. (2013). Humoral Control of Cardiac Remodeling in Fish: Role of Angiotensin II. Gen. Comp. Endocrinol. 194, 189–197. doi:10.1016/j.ygcen.2013.09.009
Ishibashi, M., Egashira, K., Zhao, Q., Hiasa, K. I., Ohtani, K, Ihara, Y., et al. (2004). Bone Marrow–Derived Monocyte Chemoattractantprotein-1 Receptor CCR2 Is Critical in Angiotensin II–Induced Acceleration of Atherosclerosis and Aneurysm Formation in Hypercholesterolemic Mice. Arterioscler. Thromb. Vasc. Biol. 24, 174–178. doi:10.1161/01.ATV.0000143384.69170.2d
Jain, M., Dhanesha, N., Doddapattar, P., Nayak, M. K., Guo, L., Cornelissen, A., et al. (2021). Smooth Muscle Cell–specific PKM2 (Pyruvate Kinase Muscle 2) Promotes Smooth Muscle Cell Phenotypic Switching and Neointimal Hyperplasia. Arterioscler. Thromb. Vasc. Biol. 41 (5), 1724–1737. doi:10.1161/ATVBAHA.121.316021
Jennings, B. L., Sahan-Firat, S., Estes, A. M., Das, K., Farjana, N., Fang, X. R., et al. (2010). Cytochrome P450 1B1 Contributes to Angiotensin II–Induced Hypertension and Associated Pathophysiology. Hypertension 56 (4), 667–674. doi:10.1161/HYPERTENSIONAHA.110.154518
Jiajing, Z., Bi, Y., Jiang, W., Cao, F., Yue, Y., and Wang, Y. (2020). Effects of Cyathula Officinalis Kuan Extracts on Hypertension-Induced Renal Vascular Remodeling by Inhibiting the Expression of Ald, Renin, Ang II and the Activation of Erk1/2 and P38 Signaling Pathways: a Mechanistic Study. Indian J. Pharm. Sci. 82, 1–7. doi:10.36468/pharmaceutical-sciences.spl.1
Jiang, L., Zhou, X., Yang, H., Guan, R., Xin, Y., Wang, J., et al. (2019). Upregulation of AT1 Receptor Mediates a Pressor Effect through ROS-SAPK/JNK Signaling in Glutamatergic Neurons of Rostral Ventrolateral Medulla in Rats with Stress-Induced Hypertension. Front. Physiol. 9, 1860. doi:10.3389/fphys.2018.01860
Jing, L., Zhang, J., Sun, J., Guo, F., An, X., Yang, K., et al. (2011). Inhibition of Extracellular Signal-Regulated Kinases Ameliorates Hypertension-Induced Renal Vascular Remodeling in Rat Models. Int. J. Mol. Sci. 12, 8333–8346. doi:10.3390/ijms12128333
Johansson, M., Ulfenborg, B., Andersson, C. X., Heydarkhan-Hagvall, S., Jeppsson, A., Sartipy, P., et al. (2020). Cardiac Hypertrophy in a Dish: a Human Stem Cell-Based Model. Biol. open 9 (9), bio052381. doi:10.1242/bio.052381
Jokubaitis, V. J., Sinka, L., Driessen, R., Whitty, G., Haylock, D. N., Bertoncello, I., et al. (2008). Angiotensin-converting Enzyme (CD143) marks Hematopoietic Stem Cells in Human Embryonic, Fetal, and Adult Hematopoietic Tissues. Blood. The J. Am. Soc. Hematol. 111 (8), 4055–4063. doi:10.1182/blood-2007-05-091710
Kasuya, Y., Kim, J. D., Hatano, M., Tatsumi, K., and Matsuda, S. (2021). Pathophysiological Roles of Stress-Activated Protein Kinases in Pulmonary Fibrosis. Int. J. Molecul. Sci. 22 (11), 6041. doi:10.3390/ijms22116041
Kawai, T., Forrester, S. J., O’Brien, S., Baggett, A., Rizzo, V., and Eguchi, S. (2017). AT1 Receptor Signaling Pathways in the Cardiovascular System. Pharmacol. Res. 125, 4–13. doi:10.1016/j.phrs.2017.05.008
Kim, J. A., Jang, H. J., Martinez-Lemus, L. A., and Sowers, J. R. (2012). Activation of mTOR/p70S6 Kinase by ANG II Inhibits Insulin-Stimulated Endothelial Nitric Oxide Synthase and Vasodilation. Am. J. Physiol. Endocrinol. 302 (2), E201–E208. doi:10.1152/ajpendo.00497.2011
Kim, S., Zingler, M., Harrison, J. K., Scott, E. W., Cogle, C. R., Luo, D., et al. (2016). Angiotensin II Regulation of Proliferation, Differentiation, and Engraftment of Hematopoietic Stem Cells. Hypertension 67 (3), 574–584. doi:10.1161/HYPERTENSIONAHA.115.06474
Kulkarni, S., O'Farrell, I., Erasi, M., and Kochar, M. S. (1998). Stress and Hypertension. WMJ, 97 (11), 34–38.
Leask, A. (2010). Potential Therapeutic Targets for Cardiac Fibrosis: TGFbeta, Angiotensin, Endothelin, CCN2, and PDGF, Partners in Fibroblast Activation. Circ. Res. 106 (11), 1675–1680. doi:10.1161/CIRCRESAHA.110.217737
Lee, C. Y., Park, H. K., Lee, B. S., Jeong, S., Hyun, S., Choi, J. W., et al. (2020). Novel Therapeutic Effects of Pterosin B on Ang II-Induced Cardiomyocyte Hypertrophy. Molecules 25 (22), 5279. doi:10.3390/molecules25225279
Li, Q., Dale, W. E., Hasser, E. M., and Blaine, E. H. (1996). Acute and Chronic Angiotensin Hypertension: Neural and Nonneural Components, Time Course, and Dose Dependency. Am. J. Physiol. Regul. Integr. Comp. Physiol. 271, 200–207. doi:10.1152/ajpregu.1996.271.1.R200
Li, H., Li, W., Gupta, A. K., Mohler, P. J., Anderson, M. E., and Grumbach, I. M. (2010). Calmodulin Kinase II Is Required for Angiotensin II-Mediated Vascular Smooth Muscle Hypertrophy. Am. J. Physiol. Heart Circ. 298 (2), H688–H698. doi:10.1152/ajpheart.01014.2009
Li, Y., Li, X. H., and Yuan, H. (2012). Angiotensin II Type-2 Receptor-specific Effects on the Cardiovascular System. Cardiovasc. Diagn. Ther. 2 (1), 56. doi:10.3978/j.issn.2223-3652.2012.02.02
Li, H. B., Li, X., Huo, C. J., Su, Q., Guo, J., and Yuan, Z. Y. (2016). TLR4/MyD88/NF-κB Signaling and PPAR-γ within the Paraventricular Nucleus Are Involved in the Effects of Telmisartan in Hypertension. Toxicol. Appl. Pharmacol. 305, 93–102. doi:10.1016/j.taap.2016.06.014
Li, C., Han, R., Kang, L., Wang, J., Gao, Y., Li, Y., et al. (2017). Pirfenidone Controls the Feedback Loop of the AT1R/p38 MAPK/renin-angiotensin System axis by Regulating Liver X Receptor-α in Myocardial Infarction-Induced Cardiac Fibrosis. Sci. Rep. 7 (1), 1–11. doi:10.1038/srep40523
Li, S., Zhu, Z., Xue, M., Yi, X., Liang, J., Niu, C., et al. (2019). Fibroblast Growth Factor 21 Protects the Heart from Angiotensin II-Induced Cardiac Hypertrophy and Dysfunction via SIRT1. Biochim. Biophys. Acta Mol. Basis Dis. 1865 (6), 1241–1252. doi:10.1016/j.bbadis.2019.01.019
Lima, R. S. D., Silva, J. C. D. S., Lima, C. T., Souza, L. E. D., Silva, M. B. D., Baladi, M. G., et al. (2019). Proinflammatory Role of Angiotensin II in the Aorta of Normotensive Mice. Biomed. Res. Int. 106 (11), 1675–1680. doi:10.1155/2019/9326896
Lins, B. B., Casare, F. A. M., Fontenele, F. F., Gonçalves, G. L., and Oliveira-Souza, M. (2021). Long-term Angiotensin II Infusion Induces Oxidative and Endoplasmic Reticulum Stress and Modulates Na+ Transporters through the Nephron. Front. Physiol. 12, 386. doi:10.3389/fphys.2021.642752
Liu, C., Zhang, J. W., Hu, L., Song, Y. C., Zhou, L., Fan, Y., et al. (2014). Activation of the AT1R/HIF-1α/ACE axis Mediates Angiotensin II-Induced VEGF Synthesis in Mesenchymal Stem Cells. Biomed. Res. Int. 2014, 627380. doi:10.1155/2014/627380
Liu, L. J., Yao, F. J., Lu, G. H., Xu, C. G., Xu, Z., Tang, K., et al. (2016). The Role of the Rho/ROCK Pathway in Ang II and TGF-β1-Induced Atrial Remodeling. PLoS One 11 (9), e0161625. doi:10.1371/journal.pone.0161625
Liu, X., Shan, X., Chen, H., Li, Z., Zhao, P., Zhang, C., et al. (2019). Stachydrine Ameliorates Cardiac Fibrosis through Inhibition of Angiotensin II/transformation Growth Factor β1 Fibrogenic axis. Front. Pharmacol. 10, 538. doi:10.3389/fphar.2019.00538
Liu, T., Wen, H., Li, H., Xu, H., Xiao, N., Liu, R., et al. (2020). Oleic Acid Attenuates Ang II (Angiotensin II)-induced Cardiac Remodeling by Inhibiting FGF23 (Fibroblast Growth Factor 23) Expression in Mice. Hypertension 75 (3), 680–692. doi:10.1161/HYPERTENSIONAHA.119.14167
Lu, Y., Sun, X., Peng, L., Jiang, W., Li, W., Yuan, H., et al. (2020). Angiotensin II-Induced Vascular Remodeling and Hypertension Involves Cathepsin L/V-MEK/ERK Mediated Mechanism. Int. J. Cardiol. 298, 98–106. doi:10.1016/j.ijcard.2019.09.070
Luo, H. M., Wu, X., Xian, X., Wang, L. Y., Zhu, L. Y., Sun, H. Y., et al. (2020). Calcitonin Gene‐related Peptide Inhibits Angiotensin II‐induced NADPH Oxidase‐dependent ROS via the Src/STAT3 Signalling Pathway. J. Cel Molecul. Med. 24 (11), 6426–6437. doi:10.1111/jcmm.15288
Lv, L., Li, T., Li, X., Xu, C., Liu, Q., Jiang, H., et al. (2018). The lncRNA Plscr4 Controls Cardiac Hypertrophy by Regulating miR-214. Mol. Ther. Nucleic Acids 10, 387–397. doi:10.1016/j.omtn.2017.12.018
Ma, Z., Viswanathan, G., Sellig, M. T., Jassal, C. S., Choi, I., Xiong, X., et al. (2021). Beta-arrestin-mediated Angiotensin II Type 1 Receptor Activation Promotes Pulmonary Vascular Remodeling in Pulmonary Hypertension. bioRxiv. doi:10.1101/2021.03.23.436676
Marian, A. J. (2008). Genetic Determinants of Cardiac Hypertrophy. Curr. Opin. Cardiol. 23 (3), 199. doi:10.1097/HCO.0b013e3282fc27d9
Matsubara, H. (1998). Pathophysiological Role of Angiotensin II Type 2 Receptor in Cardiovascular and Renal Diseases. Circ. Res. 83, 1182–1191. doi:10.1161/01.RES.83.12.1182
Matsuda, S., Umemoto, S., Yoshimura, K., Itoh, S., Murata, T., Fukai, T., et al. (2015). Angiotensin Ⅱ Activates MCP-1 and Induces Cardiac Hypertrophy and Dysfunction via Toll-like Receptor 4. J. Atheroscler. Thromb. 22, 833–844. doi:10.5551/jat.27292
Matsushita, K., Wu, Y., Okamoto, Y., Pratt, R. E., and Dzau, V. J. (2006). Local Renin Angiotensin Expression Regulates Human Mesenchymal Stem Cell Differentiation to Adipocytes. Hypertension 48 (6), 1095–1102. doi:10.1161/01.HYP.0000248211.82232.a7
Mele, L., Maskell, L. J., Stuckey, D. J., Clark, J. E., Heads, R. J., and Budhram-Mahadeo, V. S. (2019). The POU4F2/Brn-3b Transcription Factor Is Required for the Hypertrophic Response to Angiotensin II in the Heart. Cell Death Dis 10 (8), 1–18. doi:10.1038/s41419-019-1848-y
Meneton, P., Jeunemaitre, X., de Wardener, H. E., and Macgregor, G. A. (2005). Links between Dietary Salt Intake, Renal Salt Handling, Blood Pressure, and Cardiovascular Diseases. Physiolog. Rev. 85 (2), 679–715. doi:10.1152/physrev.00056.2003
Mitchell-Jordan, S. A., Holopainen, T., Ren, S., Wang, S., Warburton, S., Zhang, M. J., et al. (2008). Loss of Bmx Nonreceptor Tyrosine Kinase Prevents Pressure Overload–Induced Cardiac Hypertrophy. Circ. Res. 103 (12), 1359–1362. doi:10.1161/CIRCRESAHA.108.186577
Molitor, M., Rudi, W. S., Garlapati, V., Finger, S., Schüler, R., Kossmann, S., et al. (2021). Nox2+ Myeloid Cells Drive Vascular Inflammation and Endothelial Dysfunction in Heart Failure after Myocardial Infarction via Angiotensin II Receptor Type 1. Cardiovasc. Res. 117 (1), 162–177. doi:10.1093/cvr/cvaa042
Moore, J. P., Vinh, A., Tuck, K. L., Sakkal, S., Krishnan, S. M., Chan, C. T., et al. (2015). M2 Macrophage Accumulation in the Aortic wall during Angiotensin II Infusion in Mice Is Associated with Fibrosis, Elastin Loss, and Elevated Blood Pressure. Am. J. Physiol. Heart Circ. Physiol. 309, 906–917. doi:10.1152/ajpheart.00821.2014
Moraes, J. A., Rodrigues, G., Nascimento-Silva, V., Renovato-Martins, M., Berger, M., Guimarães, J. A., et al. (2017). Effects of Lonomia Obliqua Venom on Vascular Smooth Muscle Cells: Contribution of NADPH Oxidase-Derived Reactive Oxygen Species. Toxins 9 (11), 360. doi:10.3390/toxins9110360
Murga, C., Arcones, A. C., Cruces-Sande, M., Briones, A. M., Salaices, M., and Mayor, F. (2019). G Protein-Coupled Receptor Kinase 2 (GRK2) as a Potential Therapeutic Target in Cardiovascular and Metabolic Diseases. Front. Pharmacol. 10, 112. doi:10.3389/fphar.2019.00112
Nair, J. E., and Philips, F. J. (2015). High-Mobility Group Box 1 (HMGB1) in Angiotensin II Induced Hypertension. FASEB. J. 29, 1059–1067. doi:10.1096/fasebj.29.1_supplement.1059.7
Nair, A. R., Ebenezer, P. J., Saini, Y., and Francis, J. (2015). Angiotensin II-Induced Hypertensive Renal Inflammation Is Mediated through HMGB1-TLR4 Signaling in Rat Tubulo-Epithelial Cells. Exp. Cel. Res. 335 (2), 238–247. doi:10.1016/j.yexcr.2015.05.011
Nehme, A. (2019). An Update on the Tissue Renin Angiotensin System and its Role in Physiology and Pathology. J. Cardiovasc. Dev. Dis. 6, 14. doi:10.3390/jcdd6020014
Nunes, S. S., Feric, N., Pahnke, A., Miklas, J. W., Li, M., Coles, J., et al. (2017). Human Stem Cell-Derived Cardiac Model of Chronic Drug Exposure. ACS Biomater. Sci. Eng. 3, 1911–1921. doi:10.1021/acsbiomaterials.5b00496
Ock, S., Ham, W., Kang, C. W., Kang, H., Lee, W. S., and Kim, J. (2021). IGF-1 Protects against Angiotensin II-Induced Cardiac Fibrosis by Targeting αSMA. Cell Death Discov. 12 (7), 1–10. doi:10.1038/s41419-021-03965-5
Ogola, B. O., Zimmerman, M. A., Sure, V. N., Gentry, K. M., Duong, J. L., Clark, G. L., et al. (2019). G Protein-Coupled Estrogen Receptor Protects from Angiotensin II-Induced Increases in Pulse Pressure and Oxidative Stress. Front. Endocrinol. 10, 586. doi:10.3389/fendo.2019.00586
Ohtsu, H., Dempsey, P. J., Frank, G. D., Brailoiu, E., Higuchi, S., Suzuki, H., et al. (2006). ADAM17 Mediates Epidermal Growth Factor Receptor Transactivation and Vascular Smooth Muscle Cell Hypertrophy Induced by Angiotensin II. Arterioscler. Thromb. Vasc. Biol. 26 (9), 133–137. doi:10.1161/01.ATV.0000236203.90331.d0
Peng, K., Tian, X., Qian, Y., Skibba, M., Zou, C., Liu, Z., et al. (2016). Novel EGFR Inhibitors Attenuate Cardiac Hypertrophy Induced by Angiotensin II. J. Cel. Mol. Med. 20 (3), 482–494. doi:10.1111/jcmm.12763
Pepe, G., Basilicata, M. G., Carrizzo, A., Adesso, S., Ostacolo, C., Sala, M., et al. (2019). β-Lactoglobulin Heptapeptide Reduces Oxidative Stress in Intestinal Epithelial Cells and Angiotensin II-Induced Vasoconstriction on Mouse Mesenteric Arteries by Induction of Nuclear Factor Erythroid 2-related Factor 2 (Nrf2) Translocation. Oxid. Med. Cel 2019, 1616239. doi:10.1155/2019/1616239
Prathapan, A., Vineetha, V. P., Abhilash, P. A., and Raghu, K. G. (2013). Boerhaavia Diffusa L. Attenuates Angiotensin II-Induced Hypertrophy in H9c2 Cardiac Myoblast Cells via Modulating Oxidative Stress and Down-Regulating NF-Κβ and Transforming Growth Factor β1. Br. J. Nutr. 110 (7), 1201–1210. doi:10.1017/S0007114513000561
Rodgers, K. E., Xiong, S., Steer, R., and DiZerega, G. S. (2000). Effect of Angiotensin II on Hematopoietic Progenitor Cell Proliferation. Stem cells 18 (4), 287–294. doi:10.1634/stemcells.18-4-287
Rukavina Mikusic, N. L., Silva, M. G., Pineda, A. M., and Gironacci, M. M. (2020). Angiotensin Receptors Heterodimerization and Trafficking: How Much Do They Influence Their Biological Function? Front. Pharmacol. 11, 1179. doi:10.3389/fphar.2020.01179
Sanni, S. J., Hansen, J. T., Bonde, M. M., Speerschneider, T., Christensen, G. L., Munk, S., et al. (2010). β‐Arrestin 1 and 2 Stabilize the Angiotensin II Type I Receptor in Distinct High‐affinity Conformations. Br. J. Pharmacol. 161 (1), 150–161. doi:10.1111/j.1476-5381.2010.00875.x
Sarr, M., Chataigneau, M., Martins, S., Schott, C., El Bedoui, J., Oak, M. H., et al. (2006). Red Wine Polyphenols Prevent Angiotensin II-Induced Hypertension and Endothelial Dysfunction in Rats: Role of NADPH Oxidase. Cardiovasc. Res. 71, 794–802. doi:10.1016/j.cardiores.2006.05.022
Shao, S., Li, X. D., Lu, Y. Y., Li, S. J., Chen, X. H., Zhou, H. D., et al. (2021). Renal Natriuretic Peptide Receptor-C Deficiency Attenuates NaCl Cotransporter Activity in Angiotensin II–Induced Hypertension. Hypertension 77 (3), 868–881. doi:10.1161/HYPERTENSIONAHA.120.15636
Shinohara, K., Kishi, T., Hirooka, Y., and Sunagawa, K. (2015). Circulating Angiotensin II Deteriorates Left Ventricular Function with Sympathoexcitation via Brain Angiotensin II Receptor. Physiol. Rep. 3 (8), e12514. doi:10.14814/phy2.12514
Simoes, S. C., Balico-Silva, A. L., Parreiras-e-Silva, L. T., Bitencourt, A. L., Bouvier, M., and Costa-Neto, C. M. (2020). Signal Transduction Profiling of Angiotensin II Type 1 Receptor with Mutations Associated to Atrial Fibrillation in Humans. Front. Pharmacol. 11, 2125. doi:10.3389/fphar.2020.600132
Singh, N., Joshi, S., Guo, L., Baker, M. B., Li, Y., Castellano, R. K., et al. (2015). ACE2/Ang-(1–7)/Mas axis Stimulates Vascular Repair-Relevant Functions of CD34+ Cells. Am. J. Physiol. Heart Circ. 309 (10), 1697–1707. doi:10.1152/ajpheart.00854.2014
Skrbic, R., and Igic, R. (2009). Seven Decades of Angiotensin (1939–2009). Peptides 30, 1945–1950. doi:10.1016/j.peptides.2009.07.003
Song, J., Xie, Q., Wang, L., Lu, Y., Liu, P., Yang, P., et al. (2019). The TIR/BB-loop Mimetic AS-1 Prevents Ang II-Induced Hypertensive Cardiac Hypertrophy via NF-κB Dependent Downregulation of miRNA-143. Sci. Rep. 9, 6354. doi:10.1038/s41598-019-42936-x
Su, X., Wang, S., Zhang, H., Yang, G., Bai, Y., Liu, P., et al. (2018) Sulforaphane Prevents Angiotensin II-Induced Cardiomyopathy by Activation of Nrf2 through Epigenetic Modification. 15, 405–417. doi:10.1111/jcmm.16504
Sun, Y., Zhang, S., Yue, M., Li, Y., Bi, J., and Liu, H. (2019). Angiotensin II Inhibits Apoptosis of Mouse Aortic Smooth Muscle Cells through Regulating the circNRG-1/miR-193b-5p/NRG-1 axis. Cell Death Dis 10 (5), 1–12. doi:10.1038/s41419-019-1590-5
Swiatlowska, P., Sanchez-Alonso, J. L., Mansfield, C., Scaini, D., Korchev, Y., Novak, P., et al. (2020). Short-term Angiotensin II Treatment Regulates Cardiac Nanomechanics via Microtubule Modifications. Nanoscale 12 (30), 16315–16329. doi:10.1039/D0NR02474K
Tashiro, K., Kuwano, T., Ideishi, A., Morita, H., Idemoto, Y., Goto, M., et al. (2020). Sacubitril/valsartan Inhibits Cardiomyocyte Hypertrophy in Angiotensin II-Induced Hypertensive Mice Independent of a Blood Pressure-Lowering Effect. Cardiol. Res. 11 (6), 376. doi:10.14740/cr1137
Teekakirikul, P., Eminaga, S., Toka, O., Alcalai, R., Wang, L., Wakimoto, H., et al. (2010). Cardiac Fibrosis in Mice with Hypertrophic Cardiomyopathy Is Mediated by Non-myocyte Proliferation and Requires Tgf-Beta. J. Clin. Invest. 120 (10), 3520–3529. doi:10.1172/JCI42028
Touyz, R. M., and Berry, C. (2002). Recent Advances in Angiotensin II Signaling. Braz. J. Med. Biol. Res. 35, 1001–1015. doi:10.1590/s0100-879x2002000900001
Touyz, R. M., Wu, X. H., He, G., Salomon, S., and Schiffrin, E. L. (2002). Increased Angiotensin II-Mediated Src Signaling via Epidermal Growth Factor Receptor Transactivation Is Associated with Decreased C-Terminal Src Kinase Activity in Vascular Smooth Muscle Cells from Spontaneously Hypertensive Rats. Hypertension 39 (2), 479–485. doi:10.1161/hy02t2.102909
Tummala, P. E., Chen, X. L., Sundell, C. L., Laursen, J. B., Hammes, C. P., and Alexander, R. W. (1999). Angiotensin II Induces Vascular Cell Adhesion Molecule-1 Expression in Rat Vasculature: a Potential Link between the Renin-Angiotensin System and Atherosclerosis. Circulation 100, 1223–1229. doi:10.1161/01.cir.100.11.1223
Turu, G., Balla, A., and Hunyady, L. (2019). The Role of β-arrestin Proteins in Organization of Signaling and Regulation of the AT1 Angiotensin Receptor. Front. Endocrinol. 10, 519. doi:10.3389/fendo.2019.00519
Usui, T., Okada, M., Hara, Y., and Yamawaki, H. (2012). Death-associated Protein Kinase 3 Mediates Vascular Inflammation and Development of Hypertension in Spontaneously Hypertensive Rats. Hypertension 60 (4), 1031–1039. doi:10.1161/HYPERTENSIONAHA.112.200337
van Beusekom, C. D., and Zimmering, T. M. (2019). Profibrotic Effects of Angiotensin II and Transforming Growth Factor Beta on Feline Kidney Epithelial Cells. J. Feline Med. Surg. 21 (8), 780–787. doi:10.1177/1098612X18805862
Vukelic, S., and Griendling, K. K. (2014). Angiotensin II, from Vasoconstrictor to Growth Factor: a Paradigm Shift. Circ. Res. 114 (5), 754–757. doi:10.1161/CIRCRESAHA.114.303045
Wang, S., Cheng, M., Hu, Z., Hu, S., Zou, Q., Lai, X., et al. (2017). Angiotensin II Facilitates Matrix Metalloproteinase-9-Mediated Myosin Light Chain Kinase Degradation in Pressure Overload-Induced Cardiac Hypertrophy. Cell. Physiol. Biochem. 44 (6), 2281–2295. doi:10.1159/000486066
Wang, Q., Sui, X., Chen, R., Ma, P. Y., Teng, Y. L., Ding, T., et al. (2018). Ghrelin Ameliorates Angiotensin II-Induced Myocardial Fibrosis by Upregulating Peroxisome Proliferator-Activated Receptor Gamma in Young Male Rats. Biomed. Res. Int. 2018, 9897581. doi:10.1155/2018/9897581
Wang, Q., Yu, X., Dou, L., Huang, X., Zhu, K., Guo, J., et al. (2019). miR-154-5p Functions as an Important Regulator of Angiotensin II-Mediated Heart Remodeling. Oxid. Med. Cel Longev. 2019, 8768164. doi:10.1155/2019/8768164
Wang, Y., Guo, Z., Gao, Y., Liang, P., Shan, Y., and He, J. (2019). Angiotensin II Receptor Blocker LCZ696 Attenuates Cardiac Remodeling through the Inhibition of the ERK Signaling Pathway in Mice with Pregnancy-Associated Cardiomyopathy. Cell Biosci. 9 (1), 1–14. doi:10.1186/s13578-019-0348-1
Wang, L., Liu, C., Chen, X., and Li, P. (2019). Alamandine Attenuates Long-Term Hypertension-Induced Cardiac Fibrosis Independent of Blood Pressure. Mol. Med. Rep. 19 (6), 4553–4560. doi:10.3892/mmr.2019.10167
Wang, J., Sun, L., Nie, Y., Duan, S., Zhang, T., Wang, W., et al. (2020). Protein Kinase C δ (PKCδ) Attenuates Bleomycin Induced Pulmonary Fibrosis via Inhibiting NF-κB Signaling Pathway. Front. Physiol. 11, 367. doi:10.3389/fphys.2020.00367
Wang, S., Li, L., Liu, T., Jiang, W., and Hu, X. (2020). miR-19a/19b-loaded Exosomes in Combination with Mesenchymal Stem Cell Transplantation in a Preclinical Model of Myocardial Infarction. Regen. Med. 15 (6), 1749–1759. doi:10.2217/rme-2019-0136
Wattanapitayakul, S. K., Weinstein, D. M., Holycross, B. J., and Bauer, J. A. (2000). Endothelial Dysfunction and Peroxynitrite Formation Are Early Events in Angiotensin-Induced Cardiovascular Disorders. FASEB. J. 14, 271–278. doi:10.1096/fasebj.14.2.271
Weber, D. S., Taniyama, Y., Rocic, P., Seshiah, P. N., Dechert, M. A., Gerthoffer, W. T., et al. (2004). Phosphoinositide-dependent Kinase 1 and P21-Activated Protein Kinase Mediate Reactive Oxygen Species–dependent Regulation of Platelet-Derived Growth Factor–Induced Smooth Muscle Cell Migration. Circ. Res. 94 (9), 1219–1226. doi:10.1161/01.RES.0000126848.54740.4A
Wei, H., Ahn, S., Shenoy, S. K., Karnik, S. S., Hunyady, L., Luttrell, L. M., et al. (2003). Independent β-arrestin 2 and G Protein-Mediated Pathways for Angiotensin II Activation of Extracellular Signal-Regulated Kinases 1 and 2. Proc. Natl. Acad. Sci. 100 (19), 10782–10787. doi:10.1073/pnas.1834556100
Wen, Y., Liu, R., Lin, N., Luo, H., Tang, J., Huang, Q., et al. (2019). NADPH Oxidase Hyperactivity Contributes to Cardiac Dysfunction and Apoptosis in Rats with Severe Experimental Pancreatitis through ROS-Mediated MAPK Signaling Pathway. Oxid. Med. Cel Longev. 2019, 4578175. doi:10.1155/2019/4578175
Won, S. M., Park, Y. H., Kim, H. J., Park, K. M., and Lee, W. J. (2006). Catechins Inhibit Angiotensin II-Induced Vascular Smooth Muscle Cell Proliferation via Mitogen-Activated Protein Kinase Pathway. Ex. Mol. Med. 38, 525–534. doi:10.1038/emm.2006.62
Wong, C. K. S., Falkenham, A., Myers, T., and Légaré, J. F. (2018). Connective Tissue Growth Factor Expression after Angiotensin II Exposure Is Dependent on Transforming Growth Factor-β Signaling via the Canonical Smad-dependent Pathway in Hypertensive Induced Myocardial Fibrosis. J. Renin Angiotensin Aldosterone Syst. 19 (1), 1470320318759358. doi:10.1177/1470320318759358
Xu, J., Lin, S. C., Chen, J., Miao, Y., Taffet, G. E., Entman, M. L., et al. (2011). CCR2 Mediates the Uptake of Bone Marrow-Derived Fibroblast Precursors in Angiotensin II-Induced Cardiac Fibrosis. Am. J. Physiol. Heart Circ. 301 (2), 538–547. doi:10.1152/ajpheart.01114.2010
Xu, J. J., Li, R. J., Zhang, Z. H., Yang, C., Liu, S. X., Li, Y. L., et al. (2021). Loganin Inhibits Angiotensin Ii–Induced Cardiac Hypertrophy through the JAK2/STAT3 and NF-κB Signaling Pathways. Front. Pharmacol. 12, 1395. doi:10.3389/fphar.2021.678886
Yagi, S., Akaike, M., Aihara, K. I., Ishikawa, K., Iwase, T., Ikeda, Y., et al. (2010). Endothelial Nitric Oxide Synthase–independent Protective Action of Statin against Angiotensin II–Induced Atrial Remodeling via Reduced Oxidant Injury. Hypertension 55, 918–923. doi:10.1161/HYPERTENSIONAHA.109.146076
Yamamoto, K., Kurata, Y., Inoue, Y., Adachi, M., Tsuneto, M., Miake, J., et al. (2018). Pretreatment with an Angiotensin II Receptor Blocker Abolished Ameliorating Actions of Adipose-Derived Stem Cell Sheet on Cardiac Dysfunction and Remodeling after Myocardial Infarction. Regen. Ther. 9, 79–88. doi:10.1016/j.reth.2018.08.005
Yamashita, T., Ahmad, S., Wright, K. N., Roberts, D. J., VonCannon, J. L., Wang, H., et al. (2020). Noncanonical Mechanisms for Direct Bone Marrow Generating Ang II (Angiotensin II) Predominate in CD68 Positive Myeloid Lineage Cells. Hypertension 75 (2), 500–509. doi:10.1161/HYPERTENSIONAHA.119.13754
Yang, J., Su, J., Xi, S. S., Ke, X. F., Zhu, Y., Lin, H. P., et al. (2019). Human Umbilical Cord Mesenchymal Stem Cells Pretreated with Angiotensin-II Attenuate Pancreas Injury of Rats with Severe Acute Pancreatitis. Biomed. Pharmacother. 117, 109052. doi:10.1016/j.biopha.2019.109052
Yu, Y., Xue, B. J., Wei, S. G., Zhang, Z. H., Beltz, T. G., Guo, F., et al. (2015). Activation of central PPAR-γ Attenuates Angiotensin II–Induced Hypertension. Hypertension 66, 403–411. doi:10.1161/HYPERTENSIONAHA.115.05726
Yuan, Y., Yan, L., Wu, Q. Q., Zhou, H., Jin, Y. G., Bian, Z. Y., et al. (2016). Mnk1 (Mitogen-activated Protein Kinase–Interacting Kinase 1) Deficiency Aggravates Cardiac Remodeling in Mice. Hypertension 68 (6), 1393–1399. doi:10.1161/HYPERTENSIONAHA.116.07906
Zhang, F., Dong, Z., Gao, S., Chen, G., and Liu, D. (2019). AT1R-mediated Apoptosis of Bone Marrow Mesenchymal Stem Cells Is Associated with mtROS Production and mtDNA Reduction. Oxid. Med. Cel. Longev. 2019, 4608165. doi:10.1155/2019/4608165
Zhang, Y., Wang, Y., Zhou, D., Zhang, L. S., Deng, F. X., Shu, S., et al. (2019). Angiotensin II Deteriorates Advanced Atherosclerosis by Promoting MerTK Cleavage and Impairing Efferocytosis through the AT1R/ROS/p38 MAPK/ADAM17 Pathway. Am. J. Physiol. Cel Physiol. 317 (4), 776–787. doi:10.1152/ajpcell.00145.2019
Zhang, Z., Tian, H., Yang, C., Liu, J., Zhang, H., Wang, J., et al. (2020). Mesenchymal Stem Cells Promote the Resolution of Cardiac Inflammation after Ischemia Reperfusion via Enhancing Efferocytosis of Neutrophils. J. Am. Heart Assoc. 9 (5), e014397. doi:10.1161/JAHA.119.014397
Zheng, R. H., Bai, X. J., Zhang, W. W., Wang, J., Bai, F., Yan, C. P., et al. (2019). Liraglutide Attenuates Cardiac Remodeling and Improves Heart Function after Abdominal Aortic Constriction through Blocking Angiotensin II Type 1 Receptor in Rats. Drug Des. Devel. Ther. 13, 2745. doi:10.2147/DDDT.S213910
Zhong, H., Wang, T., Lian, G., Xu, C., Wang, H., and Xie, L. (2018). TRPM7 Regulates Angiotensin II-Induced Sinoatrial Node Fibrosis in Sick Sinus Syndrome Rats by Mediating Smad Signaling. Heart Vessels. 33, 1094–1105. doi:10.1007/s00380-018-1146-0
Zhong, T., Wang, Z., Niloy, S. I., Shen, Y., O’Rourke, S. T., and Sun, C. (2021). Role of PI3-Kinase in Angiotensin II-Induced Cardiac Hypertrophy: Class I versus Class III. Front. Pharmacol. 12, 103. doi:10.3389/fphar.2021.608523
Zhou, Y. Z., Cheng, Z., Wu, Y., Wu, Q. Y., Liao, X. B., Zhao, Y., et al. (2019). Mesenchymal Stem Cell–Derived Conditioned Medium Attenuate Angiotensin II‐induced Aortic Aneurysm Growth by Modulating Macrophage Polarization. J. Cel. Mol. Med. 23 (12), 8233–8245. doi:10.1111/jcmm.14694
Zhou, Y., Xie, Y., Li, T., Zhang, P., Chen, T., Fan, Z., et al. (2021). P21-activated Kinase 1 Mediates Angiotensin II-Induced Differentiation of Human Atrial Fibroblasts via the JNK/c-Jun Pathway. Mol. Med. Rep. 23 (3), 1–11. doi:10.3892/mmr.2021.11846
Zimnol, A., Spicker, N., Balhorn, R., Schröder, K., and Schupp, N. (2020). The NADPH Oxidase Isoform 1 Contributes to Angiotensin II-Mediated DNA Damage in the Kidney. Antioxidants 9 (7), 586. doi:10.3390/antiox9070586
Glossary
AA Aortic aneurysm
ACE angiotensin-converting enzyme
ApoE apolipoprotein E
AR angiotensin receptor
Ang II Angiotensin II
AT1 R Angiotensin type I receptor
AT1 R AP AT I receptor-associated protein
AT1A R Ang II type I subtype A receptor
AT1B R Ang II type I subtype A receptor
AT2 R Angiotensin type II receptor
AT1 R-(PI3K)/Akt Ang II-mediated AT1 R- phosphoinositide 3-kinase/protein kinase B
CaN calcineurin
CTGF connective tissue growth factor
DAG diacylglycerol
ECM extracellular matrix
EGF Endothelial growth factor
EGFR Endothelial growth factor receptor
ERK extracellular-regulated kinase
GLP-1 Glucagon-like peptide-1
HSC hepatic stellate cells
HMGB1 high-mobility group box 1
ICAM Intercellular Adhesion Molecule
IL interleukin
iNOS inducible nitric oxide synthase
IP3 inositol triphosphate
IRAK-4 Interleukin-1 receptor-associated kinase 4
JAK Janus activated kinase
LVH Left ventricular hypertrophy
miR microRNA
MLCK myosin light chain kinase
MLCP myosin light chain phosphatase
MKP-1 MAPK phophatase1
MMP mitochondrial membrane potential
MyD88 Myeloid differentiation factor 88
NADPH nicotinamide adenine dinucleotide phosphate
NO nitric oxide
PI3K phosphatidylinositol 3-kinase
PLC γ1 phospholipase C
PLD phospholipase D
PVN para ventricular nucleus
RAS renin angiotensin system
RASMC rat aortic smooth muscle cells
ROS reactive oxygen species
SFK Src family kinases
SIRT1 sirtuin 1
SOD superoxide dismutase
SPAK Ste20/SPS1-related proline/alanine-rich kinase
SRA suprarenal aorta
STAT signal transducer and activators of transcription
TGF-β1 Transforming growth factor-β1
TLR4 Toll-like receptor 4
TRAF-6 TNF receptor-associated factor 6
TRPC6 transient receptor potential cation channel, subfamily C
VCAM vascular cell adhesion molecule
VEGF vascular endothelial growth factor
WNK with-no-lysine
Keywords: angiotensin II, stem cell, biomarkers, hypertrophic markers, cardiac gene regulation, signaling
Citation: Verma K, Pant M, Paliwal S, Dwivedi J and Sharma S (2021) An Insight on Multicentric Signaling of Angiotensin II in Cardiovascular system: A Recent Update. Front. Pharmacol. 12:734917. doi: 10.3389/fphar.2021.734917
Received: 01 July 2021; Accepted: 09 August 2021;
Published: 20 August 2021.
Edited by:
Chrishan S. Samuel, Monash University, AustraliaReviewed by:
Lucia Morbidelli, University of Siena, ItalyBin-Nan Wu, Kaohsiung Medical University, Taiwan
Copyright © 2021 Verma, Pant, Paliwal, Dwivedi and Sharma. This is an open-access article distributed under the terms of the Creative Commons Attribution License (CC BY). The use, distribution or reproduction in other forums is permitted, provided the original author(s) and the copyright owner(s) are credited and that the original publication in this journal is cited, in accordance with accepted academic practice. No use, distribution or reproduction is permitted which does not comply with these terms.
*Correspondence: Swapnil Sharma, c2tzcGhhcm1hY29sb2d5QGdtYWlsLmNvbQ==
†ORCID: Swapnil Sharma, orcid.org/0000-0003-2639-7096