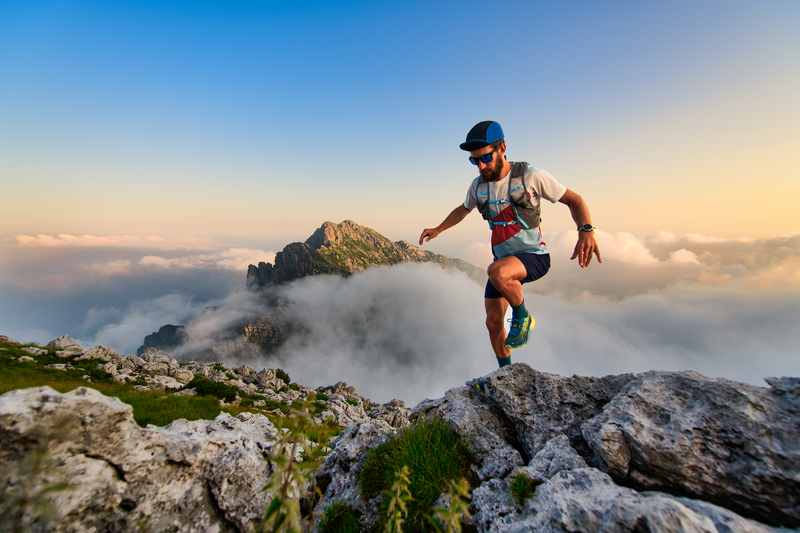
94% of researchers rate our articles as excellent or good
Learn more about the work of our research integrity team to safeguard the quality of each article we publish.
Find out more
ORIGINAL RESEARCH article
Front. Pharmacol. , 16 August 2021
Sec. Experimental Pharmacology and Drug Discovery
Volume 12 - 2021 | https://doi.org/10.3389/fphar.2021.707909
This article is part of the Research Topic Ocular Pharmacology: Recent Breakthroughs and Unmet Needs View all 14 articles
To investigate the role of vascular endothelial growth factor (VEGF) at different phases of diabetic retinopathy (DR), we assessed the retinal protein expression of VEGF-A164 (corresponding to the VEGF165 isoform present in humans, which is the predominant member implicated in vascular hyperpermeability and proliferation), HIF-1α and PKCβ/HuR pathway in Ins2Akita (diabetic) mice at different ages. We used C57BL6J mice (WT) at different ages as control. Retina status, in terms of tissue morphology and neovascularization, was monitored in vivo at different time points by optical coherence tomography (OCT) and fluorescein angiography (FA), respectively. The results showed that VEGF-A164 protein expression increased along time to become significantly elevated (p < 0.05) at 9 and 46 weeks of age compared to WT mice. The HIF-1α protein level was significantly (p < 0.05) increased at 9 weeks of age, while PKCβII and HuR protein levels were increased at 46 weeks of age compared to WT mice. The thickness of retinal nerve fiber layer as measured by OCT was decreased in Ins2Akita mice at 9 and 46 weeks of age, while no difference in the retinal vasculature were observed by FA. The present findings show that the retina of the diabetic Ins2Akita mice, as expected for mice, does not develop proliferative retinopathy even after 46 weeks. However, diabetic Ins2Akita mice recapitulate the same evolution of patients with DR in terms of both retinal neurodegeneration and pro-angiogenic shift, this latter indicated by the progressive protein expression of the pro-angiogenic isoform VEGF-A164, which can be sustained by the PKCβII/HuR pathway acting at post-transcriptional level. In agreement with this last concept, this rise in VEGF-A164 protein is not paralleled by an increment of the corresponding transcript. Nevertheless, the observed increase in HIF-1α at 9 weeks indicates that this transcription factor may favor, in the early phase of the disease, the transcription of other isoforms, possibly neuroprotective, in the attempt to counteract the neurodegenerative effects of VEGF-A164. The time-dependent VEGF-A164 expression in the retina of diabetic Ins2Akita mice suggests that pharmacological intervention in DR might be chosen, among other reasons, on the basis of the specific stages of the pathology in order to pursue the best clinical outcome.
Diabetic retinopathy (DR) is the major eye complication of diabetes mellitus and represents the leading cause of preventable blindness in the working age population, where roughly 90% of patients with type 1 diabetes and approximately 80% with type 2 diabetes for over 10 years will face the disease (Campbell and Doyle, 2019). Among common causes for blindness or severe vision impairment, DR takes the fifth place, and a recent meta-analysis underscores that, due to population growth together with a rise in the corresponding average age, the age-standardized prevalence of DR-related blindness will increase in the future (Leasher et al., 2016; Hammes 2018). The mechanisms involved in the progression of DR, characterized by an initial and prolonged ischemic phase followed by an aggressive vascular proliferation, are still argument of investigation. In particular, the role of VEGF (a factor involved in angiogenesis and cell permeability) on DR progression is still poorly understood. According to the level of microvascular- and ischemic-related damage, DR can be classified into two stages: an early non-proliferative stage (NPDR) and an advanced proliferative stage (PDR). The earliest sign of DR is the loss of pericytes contributing to inner blood-retinal barrier breakdown (Arboleda-Velasquez et al., 2015). Hallmarks of DR are also the increasing thickness of the basement membrane, the hyper-permeability, and the formation of microaneurysms. These functional alterations are followed by microvascular occlusions leading to a progressive retinal ischemia that induces the expression of the Vascular Endothelial Growth Factor-A (VEGF-A). VEGF-A, and especially the main pro-angiogenic isoform VEGF-A165, has a primary role in promoting vascular hyperpermeability; indeed, via phosphorylation of endothelial tight junction proteins it modulates their degradation, finally leading to blood-retinal barrier disruption (Moran et al., 2016). Capillary leakage and subsequent retinal exudation and edema are typical features of the disease. Further, VEGF-A165 is a potent mitogen for endothelial cells triggering their proliferation, migration and tube formation resulting in the growth of new blood vessels along the inside surface of the retina and in the vitreous that, however, in the diabetic retina are fragile and may break (Antonetti et al., 2012). In turn, these events may entail vitreous hemorrhage, subsequent fibrosis, and tractional retinal detachment with risk of permanent vision loss in the affected eye (Dulull et al., 2019). Hence, due to this dual capability to promote both vascular permeability and pathologic angiogenic proliferation, VEGF-A constitutes a key player in DR and therefore a compelling druggable target (Amadio et al., 2016a; Zhao and Singh, 2018). Incidentally, analysis of VEGF-A interaction with binding domains of anti-angiogenic agents used in clinical practice is crucial in order to improve the design of new drugs (Platania et al., 2015).
VEGF-A expression can be regulated at both transcriptional, via hypoxia inducible factor-1α (HIF-1α), and post-transcriptional level through the PKCβ/HuR cascade (Amadio et al., 2008; Amadio et al., 2010; Amadio et al., 2012). Inside the retina the cells that may show an increased expression of VEGF in case of DR are retinal pigmented epithelial cells, pericytes, astrocytes, müller cells, glial cells, and endothelial cells (Antonetti et al., 2012).
The aim of the present study was to investigate the role of the PKCβII/HuR/VEGF-A pathway in the development of DR using the Ins2Akita mouse animal model, which is characterized by a dominant mutation that induces the development of a spontaneous insulin-dependent diabetes with a rapid onset (Barber et al., 2005). Moreover, besides assessing the post-transcriptional involvement of the PKCβII/HuR cascade in regulating VEGF-A164 (corresponding to the VEGF165 isoform present in humans) content, we also examined, in Ins2Akita mice at different ages, the expression of HIF-1α to figure out the contribution of this specific transcriptional factor in the regulation of VEGF-A164 expression.
The recent identification of Ins2Akita mouse where diabetes, followed along time by the appearance of early signs of DR, develops as a consequence of a spontaneous mutation of the Insulin 2 gene makes it a unique model of “human diabetic complications” suitable for testing novel preventive approaches (Barber et al., 2005; Han et al., 2013).
Two groups of animals (5 per group), diabetic Ins2Akita and normoglycemic (C57BL6) mice were followed for 46 weeks. Only males have been included in this study because disease progression in females is slower and less uniform (Han et al., 2013). Ins2Akita female mice are resistant to develop diabetes and glycemia is often slightly, but not significantly increased when compared to controls (Al-Awar et al., 2016). Male C57BL6 and Ins2Akita mice were housed in cages in a temperature-controlled room with a 12:12 light–dark cycle and free access to food and tap water. Body weight (g) and blood glucose concentrations (mg/dl) were measured weekly during the experimental period. The experimental study was approved by the Institutional Animal Care and Use Committee (IACUC) of the San Raffaele Scientific Institute in Milan, according to the National Legislation (D.L. 116/1992) and the European Directive (2010/63/EU) about the use of laboratory animals, and with the license of the Italian Board of Health.
Retinae were homogenized, using a Teflon/glass homogenizer, in the following buffer: 20 mM Tris (pH 7.4), 2 mM EDTA, 0.5 mM EGTA, 50 mM β-mercaptoethanol, 0.32 mM sucrose and a protease inhibitor cocktail (Roche Molecular Biochemicals, Mannheim, Germany) at the dilution suggested by the manufacturer. Proteins were measured according to Bradford’s method, using bovine albumin as internal standard. Then, the proteins were diluted in Sodium Dodecyl Sulphate (SDS) protein gel loading solution, boiled for 5 min, separated on SDS-PolyAcrylamide Gel Electrophoresis, and processed following standard procedures. The mouse monoclonal anti-ELAV/HuR antibody (Santa Cruz Biotech. Inc., Dallas, TX, United States) was diluted at 1:1,000. The rabbit monoclonal antibodies anti-VEGF-A (Abcam, United Kingdom) and anti-HIF1α (Cell Signalling Technology, Netherlands) were diluted at 1:750 and 1:1,000, respectively. The rabbit polyclonal PKC βII (Santa Cruz Biotech. Inc.) was diluted at 1: 500, while the rat monoclonal antibody anti-α-tubulin (Thermo Fisher Scientific, Waltham, MA, United States) was diluted at 1:1,000. The specific antibodies were diluted in TBST buffer [10 mM Tris-HCl, 100 mM NaCl, 0.1% (v/v) Tween 20, pH 7.5] containing 6% milk. The nitrocellulose membrane signals were detected by chemiluminescence. Experiments were performed at least three times for each tissue preparation; the same membranes were reprobed with α-tubulin antibody to normalize the data. Statistical analysis of western blot data was performed on the densitometric values obtained with the ImageJ 1.50i software (downloadable at http://imagej.nih.image/ij).
RNA was extracted from total homogenates by using RNeasy Mini Kit (Qiagen, Germany). The reverse transcription was performed following standard procedures. PCR amplifications were carried out using the Rotor-Gene Q instrument (Qiagen) in the presence of QuantiTect SYBR Green PCR mix (Qiagen) with the specific primers for VEGF164 (provided by SIGMA). The GAPDH mRNA was chosen as the reference gene to normalize the data (the specific primers were provided by Qiagen).
Optical coherence tomography (OCT) and fluorescein angiography (FA) were performed as previously described (Buccarello et al., 2017), taking advantage of the Micron IV instrument (Phoenix Research Laboratories, Pleasanton, CA, United States). Briefly, after anesthesia, mydriasis was induced by administering a drop of tropicamide 0.5% (Visumidriatic, Tibilux Pharma, Milan, Italy) in each eye. OCT images were acquired by performing a circular scan of 550 μm of diameter around the optic nerve head. Both eyes were examined, and the results were averaged. The segmentation of retinal layers was performed using Insight software (Phoenix Research Laboratories, Pleasanton, CA, United States), OCT was followed by the FA study. A solution of 1% fluorescein (5 ml/kg Monico S.p.A., Venezia, Italy) was administered by a single intraperitoneal injection (100 μL). For each animal, the images of central and peripheral retinal vasculature were acquired.
For statistical analysis, the GraphPad Instat statistical package (version 3.05 GraphPad software, San Diego, CA, United States) was used. The data were analyzed by analysis of variance (ANOVA) followed, when significant, by an appropriate post hoc comparison test, as detailed in the legends. Differences were considered statistically significant when p values <0.05.
Blood glucose levels, measured at 5, 7, 9, and 46 weeks of age, were significantly increased in Ins2Akita mice in comparison with their relative wild-type littermates (Figure 1A). Conversely, body weight measured at 9 and 46 weeks of age was significantly reduced in Ins2Akita mice, as a consequence of glycosuria, in comparison with their respective wild-type controls, reaching a statistical significance in 46 weeks old animals (Figure 1B).
FIGURE 1. Blood glucose levels and body weight in wild-type and Ins2Akita mice animals at different ages. Blood glucose levels (A) are expressed in mg/dL, while body weight (B) is expressed in grams. The values are shown as filled dots for wild-type mice and open dots for Ins2Akita animals *p < 0.05 student t-test, vs wild-type.
VEGF protein is increased in Ins2Akita mice: a time-dependent contribution of transcriptional and post-transcriptional mechanisms.
Given the key role of VEGF-A165 in DR development, we assessed its protein expression in wild-type and Ins2Akita mice at different ages. As shown in Figure 2, in Ins2Akita mice we found an increase in VEGF-A164 content (as previously mentioned, this isoform corresponds to the VEGF-A165 present in humans) already at 7 weeks (+38%), which reaches a statistical significance in 9 (+67%) and 46 (+71%) weeks old animals with respect to their relative wild-type littermates. No age-dependent changes in VEGF-A164 protein basal levels, measured in an independent set of experiments, were observed among wild-type mice (Figure 3).
FIGURE 2. VEGF-A protein levels in Ins2Akita mice at different ages. (A): Representative western blottings of VEGF-A164 and the respective α-tubulin, measured in the same samples, of total homogenates of retinae from Ins2Akita (AK) mice at different ages (w = weeks). (B): Densitometric analysis of VEGF-A164 immunoreactivities in the total homogenates of retinae from wild-type and AK mice at different ages. Alpha-tubulin was used as a loading control. Results are expressed as % (±S.E.M.) vs control wild-type (100%, dashed line). *p < 0.05, **p < 0.01 vs wild-type; Tukey’s Multiple Comparison post-hoc test, n = 8–10.
FIGURE 3. VEGF-A, HIF-1α, PKCβII and HuR protein levels in wild-type mice at different ages. Densitometric analysis of VEGF-A164(A), HIF-1α (B), PKCβII (C), and HuR (D) immunoreactivities in the total homogenates of retinae from wild-type (WT) mice at different ages (w = weeks). Alpha-tubulin was used as a loading control. Results are expressed as mean grey levels ratios x 103 (mean ± S.E.M.) of VEGF-A164/α-tubulin (A), HIF-1α/α-tubulin (B), PKCβII/α-tubulin (C) and HuR/α-tubulin (D) immunoreactivities measured by Western blotting, n = 5.
We also measured VEGF-A164 mRNA levels via Real-time PCR. Preliminary results indicate a significant decrease in VEGF-A164 transcript content at 9 and 46 weeks (−78%, p < 0.005 and −63%, p < 0.05, respectively) compared to 3 weeks Ins2Akita mice.
With the purpose of dissecting the implication of both transcriptional and post-transcriptional mechanisms in the regulation of VEGF-A164 protein expression, we measured, respectively, HIF-1α and PKCβII/HuR protein levels in the retina from wild-type and Ins2Akita mice at different ages.
Concerning HIF-1α, as depicted in Figure 4, in Ins2Akita mice we observed a progressive increase in its protein content starting from 3 weeks (+25%) to 7 weeks old animals (5 weeks: +41%; 7 weeks: +49%), reaching a statistically significant rise in 9 weeks old mice (+87%). Instead, a decrease was found in 46 weeks old Ins2Akita mice (−33%), whose levels were significantly lower with respect to 9 weeks old Ins2Akitaanimals. No age-dependent changes in HIF-1α protein basal levels, measured in an independent set of experiments, were observed among wild-type mice (Figure 3).
FIGURE 4. HIF-1α protein levels in Ins2Akita mice at different ages. (A): Representative western blottings of HIF-1α and the respective α-tubulin, measured in the same samples, of total homogenates of retinae from Ins2Akita (AK) mice at different ages (w = weeks). (B): Densitometric analysis of HIF-1α immunoreactivities in the total homogenates of retinae from wild-type and AK mice at different ages. Alpha-tubulin was used as a loading control. Results are expressed as % (±S.E.M.) vs control wild-type (100%, dashed line). *p < 0.05 vs wild-type and §p < 0.05 vs AK-9w; Tukey’s Multiple Comparison post-hoc test, n = 8–10.
We then investigated the PKCβII/HuR pathway, since we previously demonstrated, in another animal model of DR, its key involvement in the post-transcriptional control of VEGF-A protein expression (Amadio et al., 2010). As shown in Figure 5, we observed a significant increase in both PKCβII (+29%) and HuR (+48%) protein levels only in 46 weeks old Ins2Akita mice. No age-dependent changes in PKCβII and HuR protein basal levels, measured in an independent set of experiments, were observed among wild-type mice (Figure 3).
FIGURE 5. PKCβII and HuR protein levels in Ins2Akita mice at different ages. Upper panels: Representative western blottings of PKCβII (A), HuR (B) and the respective α-tubulin, measured in the same samples, of total homogenates of retinae from Ins2Akita (AK) mice at different ages (w = weeks). Lower panels: Densitometric analysis of PKCβII (A) and HuR (B) immunoreactivities in the total homogenates of retinae from wild-type and AK mice at different ages. Alpha-tubulin was used as a loading control. Results are expressed as % (±S.E.M.) vs control wild-type (100%, dashed line). **p < 0.01 vs wild-type; unpaired t-test, n = 8–10.
OCT and fluorescein angiography were performed during the entire study in wild-type and Ins2Akita diabetic mice (Figure 6). The collected images did not highlight any retinal signs of vascular dysfunction in both groups of animals. Thickness of Retinal Nerve Fiber Layer (RNFL) was also examined. The results show a significant decrease in RNFL thickness in 9 weeks of age Ins2Akita mice compared to wild-type littermates, which becomes even more pronounced in 46 weeks old animals (Figure 7).
FIGURE 6. Fundus oculi (left), optical coherence tomography (OCT; center) and fluorescein angiography (right) performed during the study in wild-type and Ins2Akita animals. The shown images were taken in the left eye of a representative wild-type (A) or Ins2Akita(B) mice at 3 and 46 weeks of age.
FIGURE 7. Retinal nerve fiber layer thickness in wild-type and Ins2Akita animals. Retinal nerve fiber layer (RNFL) thickness is expressed in µm and the values are shown as filled dots for wild-type mice and open dots for Ins2Akita animals. *p < 0.05, student t-test vs control wild-type.
Diabetic retinopathy is the primary cause of blindness in adults living in industrialized countries, being the global prevalence around 30–35% within the diabetic population. The loss of visual function is mainly associated with macular edema and the proliferative stage of the disease, with a dramatic impact in terms of countries health system costs. DR is triggered by the chronic hyperglycemia linked to the diabetic condition and by the following metabolic stress. This altered milieu induces changes at microvascular level that result in the inability of capillaries to guarantee to the retina the proper blood supply, thus entailing the formation of non-perfused areas and the development of a hypoxic environment that promotes the production of VEGF-A, a pivotal player in DR pathophysiology (Rigo et al., 2020).
VEGF-A belongs to a family that also includes VEGF-B, -C, -D, and placental growth factor (Amadio et al., 2016a; Ferrara and Adamis, 2016). The human VEGF-A gene consists of eight exons separated by seven introns determining the generation of different isoforms, being the VEGF-A165 the predominant member and the main isoform implicated in vascular hyperpermeability and proliferation (Amadio et al., 2016a). In the diabetic retina, the expression of VEGF-A can be regulated by different pathways, including Protein Kinase C (PKC) (Clarke and Dodson, 2007; Yokota et al., 2007; Ye et al., 2010). PKC consists of at least 10 serine-threonine kinases ubiquitously expressed and involved in several cellular functions (Battaini and Mochly-Rosen, 2007; Govoni et al., 2010). It is worth of note, that the diabetes-related hyperglycemia induces a rise in the amount of diacylglycerol, the physiological activator of PKC. Among the various isoforms, the PKCβ seems to be the isoenzyme primarily activated in the retina, although other PKCs can be also implicated (Aiello, 2002; Kim et al., 2010).
In the present study, we used the Ins2Akita mouse as an animal model of type 1 diabetes, which is endowed with a dominant mutation that induces the development of a spontaneous insulin-dependent diabetes with a rapid onset (Barber et al., 2005). Notably, the mutation in the Insulin 2 gene elicits a conformational change in the insulin protein and its consequent accumulation in pancreatic β cells, leading to β-cells death (Olivares et al., 2017). As supported by literature data, the Ins2Akita mouse is an excellent model to explore the molecular mechanisms implicated in the initiation and early progression of DR. The development, in the Ins2Akita mouse of the late, neovascular stages of DR remains presently unclear (Han et al., 2013; McLenachan et al., 2013). Indeed, we showed that by 5 weeks of age the animals present already significantly elevated levels of blood glucose compared to wild-type littermates, while a decrease in the body weight, due to glycosuria, is evident starting from 9 weeks of age. We also observed a time-dependent increase in VEGF-A164 (this isoform corresponds to the VEGF-A165 present in humans) content at retinal level, starting from 7 weeks of age and becoming gradually more pronounced. This rise in VEGF-A164 protein is not paralleled by an increment of the corresponding transcript, strongly suggesting that it can be sustained by the PKCβII/HuR pathway acting at post-transcriptional level. Nevertheless, the observed increase in HIF-1α at 9 weeks indicates that this transcription factor may favor, in the early phase of the disease, the transcription of other isoforms, possibly neuroprotective, in the attempt to counteract the neurodegenerative effects of VEGF-A164. It is worth of note, that our data show a distinct temporal regulation of VEGF-A expression, implicating two different molecular processes: transcriptional and post-transcriptional. In fact, the transcription factor HIF-1α seems to contribute to the earlier increase in VEGF-A protein content, possibly trying to counteract the neurodegenerative effects of DR through the promotion of neuroprotective VEGF-A isoforms, such as VEGF120/121. The late rise in VEGF-A seems, instead, to rely upon the PKCβII/HuR cascade acting at post-transcriptional level, which favors the expression of VEGF164, the member primary implicated in vascular hyperpermeability and proliferation. To this last regard, these results confirm our previous findings showing, both in vitro (Amadio et al., 2008; Amadio et al., 2012; Marchesi et al., 2020; Platania et al., 2020) and in vivo (Amadio et al., 2010), a post-transcriptional control of VEGF-A expression mediated by the RNA binding protein (RBP) ELAV/HuR.
In mammals, ELAV proteins are a small family of evolutionarily conserved RBPs, orthologues of the elav gene discovered in the fruit fly Drosophila melanogaster. The family includes the ubiquitously expressed HuR and three neuron-specific members (nELAV), namely HuB, HuC, and HuD. The four ELAV proteins can virtually influence any aspect of the post-synthesis fate of the targeted mRNAs, being stability and translation the most relevant and studied mechanisms. Indeed, following intra- and extracellular inputs, ELAV mainly determine an increase in the cytoplasmic stability and/or rate of translation of the target transcripts, by preferentially binding to ARE (adenine-uracil-rich elements) cis-acting elements present within their sequence, although other consensus elements may be implicated (Pascale and Govoni, 2012).
Within this context, in another model of experimental diabetes induced in rodents, namely rats exposed to a single intravenous injection of streptozotocin (STZ), we previously demonstrated that, following a PKC β-mediated phosphorylation, the ELAV/HuR binds to VEGF-A mRNA and positively affects its expression in the retina, thus contributing to abnormally enhanced VEGF-A content in the retinal tissue (Amadio et al., 2010). Notably, STZ-induced diabetic rats show the same features of the NPDR observed in humans, including blood vessels dilation and increased vascular permeability. Further, we also reported that nano-systems loaded with a commercially available siRNA, which specifically switches off the ELAV/HuR expression when injected into the eye of diabetic rats, was able to attenuate the increase in VEGF-A content without suppressing its basal levels (Amadio et al., 2016b). These findings, together with the present data underline the key role of the PKCβ/HuR cascade in regulating the pathologic overexpression of VEGF. Incidentally, the use of nano- or micro-systems could be useful to ameliorate the intra-ocular delivery of pharmacological agents (Conti et al., 1997).
The results obtained by OCT, a non-invasive imaging technique that allows collecting information on the retina morphology, indicate that the retinal nerve fiber layer (RNFL) thickness is dramatically reduced in 46 weeks of age Ins2Akita mice, and strongly suggest that this neurodegenerative event may be sustained by the increased VEGF-A164 levels. Within this general context, it should be taken into consideration that the VEGF-A gene is alternatively spliced to generate VEGF-Axxxa and VEGF-Axxxb isoforms, being the last ones potentially endowed with anti-angiogenic and anti-permeability properties (Qiu et al., 2009). Of interest, it has been reported that DR is associated with a switch in splicing from anti-towards pro-angiogenic isoforms (Perrin et al., 2005). Although the antibody used in the study allowed us to primarily detect VEGF-A164a, it is tempting to speculate that the observed increase in this isoform goes to the detriment of the corresponding VEGF-A164b, which has a critical role in cell protection and survival (Peiris-Pagès 2012). Therefore, this switch in isoforms production might promote a degenerative process in the retina leading to a decrease in RNFL thickness, as we detected in the present work. Nevertheless, it should be also underlined that the literature data regarding VEGF-Axxxb isoforms are conflicting, and some authors even question the existence of VEGF-Axxxb isoforms themselves (Harris et al., 2012; Bridgett et al., 2017).
In conclusion, these data seem to suggest that pharmacological intervention in clinical practice might be chosen, among other reasons, based on the specific stages of the diabetic retinopathy to pursue the best clinical outcome. Clinical studies to evaluate this possibility may be warranted.
The raw data supporting the conclusions of this article will be made available by the authors, without undue reservation.
The animal study was reviewed and approved by Institutional Animal Care and Use Committee (IACUC) of the San Raffaele Scientific Institute in Milan.
CB, GZ, and AP made substantial contributions to conception, design, and interpretation of data. AB, NM, GZ, AP, and IV carried out experiments. AB, NM, GZ, AP, and IV carried out formal analysis of data. CB, GZ, and AP wrote initial draft of the manuscript. CB, AB, GZ, AP, FD, SG, and FB reviewed the manuscript critically for important intellectual content and gave final approval of the version to be submitted.
This work was supported by National Grant PRIN 2015JXE7E8 from the Italian Ministry of Education, University and Research (MIUR).
The authors declare that the research was conducted in the absence of any commercial or financial relationships that could be construed as a potential conflict of interest.
All claims expressed in this article are solely those of the authors and do not necessarily represent those of their affiliated organizations, or those of the publisher, the editors and the reviewers. Any product that may be evaluated in this article, or claim that may be made by its manufacturer, is not guaranteed or endorsed by the publisher.
The Supplementary Material for this article can be found online at: https://www.frontiersin.org/articles/10.3389/fphar.2021.707909/full#supplementary-material
Aiello, L. P. (2002). The Potential Role of PKC Beta in Diabetic Retinopathy and Macular Edema. Surv. Ophthalmol. 47, S263–S269. doi:10.1016/s0039-6257(02)00391-0
Al-Awar, A., Kupai, K., Veszelka, M., Szűcs, G., Attieh, Z., Murlasits, Z., et al. (2016). Experimental Diabetes Mellitus in Different Animal Models. J. Diabetes Res. 2016, 9051426. doi:10.1155/2016/9051426
Amadio, M., Bucolo, C., Leggio, G. M., Drago, F., Govoni, S., and Pascale, A. (2010). The PKCbeta/HuR/VEGF Pathway in Diabetic Retinopathy. Biochem. Pharmacol. 80 (8), 1230–1237. doi:10.1016/j.bcp.2010.06.033
Amadio, M., Govoni, S., and Pascale, A. (2016a). Targeting VEGF in Eye Neovascularization: What's New?: A Comprehensive Review on Current Therapies and Oligonucleotide-Based Interventions under Development. Pharmacol. Res. 103, 253–269. doi:10.1016/j.phrs.2015.11.027
Amadio, M., Osera, C., Lupo, G., Motta, C., Drago, F., Govoni, S., et al. (2012). Protein Kinase C Activation Affects, via the mRNA-Binding Hu-Antigen R/ELAV Protein, Vascular Endothelial Growth Factor Expression in a Pericytic/endothelial Coculture Model. Mol. Vis. 18, 2153–2164.
Amadio, M., Pascale, A., Cupri, S., Pignatello, R., Osera, C., D Agata, V. V., et al. (2016b). Nanosystems Based on siRNA Silencing HuR Expression Counteract Diabetic Retinopathy in Rat. Pharmacol. Res. 111, 713–720. doi:10.1016/j.phrs.2016.07.042
Amadio, M., Scapagnini, G., Lupo, G., Drago, F., Govoni, S., and Pascale, A. (2008). PKCbetaII/HuR/VEGF: A New Molecular cascade in Retinal Pericytes for the Regulation of VEGF Gene Expression. Pharmacol. Res. 57 (1), 60–66. doi:10.1016/j.phrs.2007.11.006
Antonetti, D. A., Klein, R., and Gardner, T. W. (2012). Diabetic Retinopathy. N. Engl. J. Med. 366 (13), 1227–1239. doi:10.1056/NEJMra1005073
Arboleda-Velasquez, J. F., Valdez, C. N., Marko, C. K., and D'Amore, P. A. (2015). From Pathobiology to the Targeting of Pericytes for the Treatment of Diabetic Retinopathy. Curr. Diab. Rep. 15 (2), 573–592. doi:10.1007/s11892-014-0573-2
Barber, A. J., Antonetti, D. A., Kern, T. S., Reiter, C. E., Soans, R. S., Krady, J. K., et al. (2005). The Ins2Akita Mouse as a Model of Early Retinal Complications in Diabetes. Invest. Ophthalmol. Vis. Sci. 46 (6), 2210–2218. doi:10.1167/iovs.04-1340
Battaini, F., and Mochly-Rosen, D. (2007). Happy Birthday Protein Kinase C: Past, Present and Future of a Superfamily. Pharmacol. Res. 55 (6), 461–466. doi:10.1016/j.phrs.2007.05.005
Bridgett, S., Dellett, M., and Simpson, D. A. (2017). RNA-sequencing Data Supports the Existence of Novel VEGFA Splicing Events but Not of VEGFAxxxb Isoforms. Sci. Rep. 7 (1), 58. doi:10.1038/s41598-017-00100-3
Buccarello, L., Sclip, A., Sacchi, M., Castaldo, A. M., Bertani, I., ReCecconi, A., et al. (2017). The C-Jun N-Terminal Kinase Plays a Key Role in Ocular Degenerative Changes in a Mouse Model of Alzheimer Disease Suggesting a Correlation between Ocular and Brain Pathologies. Oncotarget 8 (47), 83038–83051. doi:10.18632/oncotarget.19886
Campbell, M., and Doyle, S. L. (2019). Current Perspectives on Established and Novel Therapies for Pathological Neovascularization in Retinal Disease. Biochem. Pharmacol. 164, 321–325. doi:10.1016/j.bcp.2019.04.029
Clarke, M., and Dodson, P. M. (2007). PKC Inhibition and Diabetic Microvascular Complications. Best Pract. Res. Clin. Endocrinol. Metab. 21, 573–586. doi:10.1016/j.beem.2007.09.007
Conti, B., Bucolo, C., Giannavola, C., Puglisi, G., Giunchedi, P., and Conte, U. (1997). Biodegradable Microspheres for the Intravitreal Administration of Acyclovir: In Vitro/In Vivo Evaluation. Eur. J. Pharm. Sci. 5 (5), 287–293. doi:10.1016/S0928-0987(97)00023-7
Dulull, N., Kwa, F., Osman, N., Rai, U., Shaikh, B., and Thrimawithana, T. R. (2019). Recent Advances in the Management of Diabetic Retinopathy. Drug Discov. Today 24 (8), 1499–1509. doi:10.1016/j.drudis.2019.03.028
Ferrara, N., and Adamis, A. P. (2016). Ten Years of Anti-vascular Endothelial Growth Factor Therapy. Nat. Rev. Drug Discov. 15 (6), 385–403. doi:10.1038/nrd.2015.17
Govoni, S., Amadio, M., Battaini, F., and Pascale, A. (2010). Senescence of the Brain: Focus on Cognitive Kinases. Curr. Pharm. Des. 16 (6), 660–671. doi:10.2174/138161210790883732
Hammes, H. P. (2018). Diabetic Retinopathy: Hyperglycaemia, Oxidative Stress and beyond. Diabetologia 61 (1), 29–38. doi:10.1007/s00125-017-4435-8
Han, Z., Guo, J., Conley, S. M., and Naash, M. I. (2013). Retinal Angiogenesis in the Ins2(Akita) Mouse Model of Diabetic Retinopathy. Invest. Ophthalmol. Vis. Sci. 54, 574–584. doi:10.1167/iovs.12-10959
Harris, S., Craze, M., Newton, J., Fisher, M., Shima, D. T., Tozer, G. M., et al. (2012). Do anti-angiogenic VEGF (VEGFxxxb) Isoforms Exist? A Cautionary Tale. PLoS One 7 (5), e35231. doi:10.1371/journal.pone.0035231
Kim, J. H., Kim, J. H., Jun, H. O., Yu, Y. S., and Kim, K. W. (2010). Inhibition of Protein Kinase C delta Attenuates Blood-Retinal Barrier Breakdown in Diabetic Retinopathy. Am. J. Pathol. 176, 1517–1524. doi:10.2353/ajpath.2010.090398
Leasher, J. L., Bourne, R. R. A., Flaxman, S. R., Jonas, J. B., Keeffe, J., Naidoo, K., et al. (2016). Global Estimates on the Number of People Blind or Visually Impaired by Diabetic Retinopathy: A Meta-Analysis from 1990 to 2010. Dia Care 39 (9), 1643–1649. doi:10.2337/dc15-2171
Marchesi, N., Barbieri, A., Fahmideh, F., Govoni, S., Ghidoni, A., Parati, G., et al. (2020). Use of Dual-Flow Bioreactor to Develop a Simplified Model of Nervous-Cardiovascular Systems Crosstalk: A Preliminary Assessment. PLoS One 15 (11), e0242627. doi:10.1371/journal.pone.0242627
McLenachan, S., Chen, X., McMenamin, P. G., and Rakoczy, E. P. (2013). Absence of Clinical Correlates of Diabetic Retinopathy in the Ins2Akita Retina. Clin. Exp. Ophthalmol. 41 (6), 582–592. doi:10.1111/ceo.12084
Moran, E. P., Wang, Z., Chen, J., Sapieha, P., Smith, L. E., and Ma, J. X. (2016). Neurovascular Cross Talk in Diabetic Retinopathy: Pathophysiological Roles and Therapeutic Implications. Am. J. Physiol. Heart Circ. Physiol. 311 (3), H738–H749. doi:10.1152/ajpheart.00005.2016
Olivares, A. M., Althoff, K., Chen, G. F., Wu, S., Morrisson, M. A., DeAngelis, M. M., et al. (2017). Animal Models of Diabetic Retinopathy. Curr. Diab. Rep. 17 (10), 93–100. doi:10.1007/s11892-017-0913-0
Pascale, A., and Govoni, S. (2012). The Complex World of post-transcriptional Mechanisms: Is Their Deregulation a Common Link for Diseases? Focus on ELAV-like RNA-Binding Proteins. Cell. Mol. Life Sci. 69 (4), 501–517. doi:10.1007/s00018-011-0810-7
Peiris-Pagès, M. (2012). The Role of VEGF 165b in Pathophysiology. Cell. Adh. Migr. 6 (6), 561–568. doi:10.4161/cam.22439
Perrin, R. M., Konopatskaya, O., Qiu, Y., Harper, S., Bates, D. O., and Churchill, A. J. (2005). Diabetic Retinopathy Is Associated with a Switch in Splicing from Anti- to Pro-angiogenic Isoforms of Vascular Endothelial Growth Factor. Diabetologia 48 (11), 2422–2427. doi:10.1007/s00125-005-1951-8
Platania, C. B., Di Paola, L., Leggio, G. M., Romano, G. L., Drago, F., Salomone, S., et al. (2015). Molecular Features of Interaction between VEGFA and Anti-angiogenic Drugs Used in Retinal Diseases: a Computational Approach. Front. Pharmacol. 6, 248–261. doi:10.3389/fphar.2015.00248
Platania, C. B. M., Pittalà, V., Pascale, A., Marchesi, N., Anfuso, C. D., Lupo, G., et al. (2020). Novel Indole Derivatives Targeting HuR-mRNA Complex to Counteract High Glucose Damage in Retinal Endothelial Cells. Biochem. Pharmacol. 175, 113908. doi:10.1016/j.bcp.2020.113908
Qiu, Y., Hoareau-Aveilla, C., Oltean, S., Harper, S. J., and Bates, D. O. (2009). The Anti-angiogenic Isoforms of VEGF in Health and Disease. Biochem. Soc. Trans. 37 (Pt 6), 1207–1213. doi:10.1042/BST0371207
Rigo, S., Duchâteau, E., and Rakic, J. M. (2020). [What's New in Diabetic Retinopathy ?]. Rev. Med. Liege. 75 (5-6), 432–439.
Ye, X., Xu, G., Chang, Q., Fan, J., Sun, Z., Qin, Y., et al. (2010). ERK1/2 Signaling Pathways Involved in VEGF Release in Diabetic Rat Retina. Inves.t Ophthalmol. Vis. Sci. 51 (10), 5226–5233. doi:10.1167/iovs.09-4899
Yokota, T., Utsunomiya, K., Taniguchi, K., Gojo, A., Kurata, H., and Tajima, N. (2007). Involvement of the Rho/Rho Kinase Signaling Pathway in Platelet-Derived Growth Factor BB-Induced Vascular Endothelial Growth Factor Expression in Diabetic Rat Retina. Jpn. J. Ophthalmol. 51, 424–430. doi:10.1007/s10384-007-0471-0
Keywords: VEGF, ELAV/HuR, retinopathy, diabetes, hyperglycemia, akita mouse
Citation: Bucolo C, Barbieri A, Viganò I, Marchesi N, Bandello F, Drago F, Govoni S, Zerbini G and Pascale A (2021) Short-and Long-Term Expression of Vegf: A Temporal Regulation of a Key Factor in Diabetic Retinopathy. Front. Pharmacol. 12:707909. doi: 10.3389/fphar.2021.707909
Received: 10 May 2021; Accepted: 06 August 2021;
Published: 16 August 2021.
Edited by:
Valentina Vellecco, University of Naples Federico II, ItalyReviewed by:
Shusheng Wang, Tulane University, United StatesCopyright © 2021 Bucolo, Barbieri, Viganò, Marchesi, Bandello, Drago, Govoni, Zerbini and Pascale. This is an open-access article distributed under the terms of the Creative Commons Attribution License (CC BY). The use, distribution or reproduction in other forums is permitted, provided the original author(s) and the copyright owner(s) are credited and that the original publication in this journal is cited, in accordance with accepted academic practice. No use, distribution or reproduction is permitted which does not comply with these terms.
*Correspondence: Gianpaolo Zerbini, emVyYmluaS5naWFucGFvbG9AaHNyLml0
†These authors have contributed equally to this work
Disclaimer: All claims expressed in this article are solely those of the authors and do not necessarily represent those of their affiliated organizations, or those of the publisher, the editors and the reviewers. Any product that may be evaluated in this article or claim that may be made by its manufacturer is not guaranteed or endorsed by the publisher.
Research integrity at Frontiers
Learn more about the work of our research integrity team to safeguard the quality of each article we publish.