- 1Jiangsu Key Laboratory of Medical Science and Laboratory Medicine, Institute of Stem Cell, School of Medicine, Jiangsu University, Zhenjiang, China
- 2Department of Clinical Laboratory, The Affiliated Yixing Hospital of Jiangsu University, Yixing, China
- 3Directorate of University Health Services, University of Cape Coast, Cape Coast, Ghana
Dysfunctional skin barrier and impaired skin homeostasis may lead to or aggravate a series of dermatologic diseases. A large variety of biological events and bioactive molecules are involved in the process of skin wound healing and functional recovery. Calcium ions (Ca2+) released from intracellular stores as well as influx through plasma membrane are essential to skin function. Growing evidence suggests that calcium influx is mainly regulated by calcium-sensing receptors and channels, including voltage-gated, transient potential receptor, store-operated, and receptor-operated calcium channels, which not only maintain cellular Ca2+ homeostasis, but also participate in cell proliferation and skin cell homeostasis through Ca2+-sensitive proteins such as calmodulin (CaM). Furthermore, distinct types of Ca2+ channels not merely work separately, they may work concertedly to regulate cell function. In this review, we discussed different calcium-sensing receptors and channels, including voltage-gated, transient receptor potential, store-operated, and receptor-operated calcium channels, particularly focusing on their regulatory functions and inherent interactions as well as calcium channels-related reagents and drugs, which is expected to bridge basic research and clinical applications in dermatologic diseases.
Introduction
The skin is the largest and heaviest organ of the body and is subjected to a wide spectrum of traumatic injury. During the process of wound healing, a large variety of bioactive molecules are involved. Calcium ions (Ca2+) are one of the most diverse signaling mediators and intracellular second messengers, and contribute to establish and maintain the skin architecture and homeostasis (Pillai et al., 1990). Calcium is normally sequestered outside the cell or stored in organelles such as the endoplasmic reticulum (ER) and sarcoplasmic reticulum (SR); in contrast, calcium levels are low in the cytoplasm, leading to the generation of a calcium concentration gradient. The calcium gradient with peaking calcium concentrations in the stratum granulosum and a steep drop-off in the stratum corneum in epidermis is important to maintain skin barrier function (Elsholz et al., 2014). When the skin is impaired, Ca2+-sensing receptor (CaSR) conveys the signaling of extracellular Ca2+ to interior of the cell through different calcium channels, causing skin cells adhesion, differentiation, and survival.
There are generally two classes of calcium channels, calcium entry channels, which allow extracellular Ca2+ to enter intracelluar cells, and calcium release channels, which transfer Ca2+ from intracellular stores to the cytoplasm. Calcium entry channels include voltage-gated calcium channels (VGCCs), ligand-gated calcium channels, store operated calcium channels (SOCCs) and transient receptor potential (TRP) channels Calcium release channels include ryanodine receptors (RyRs) and inositol 1,4,5-trisphosphate receptors (IP3Rs) (Greenberg, 1997). It is gradually realized that calcium channels are involved in skin function maintenance under physiological and pathological conditions. The purpose of this review is to summarize the current state of calcium-sensing receptors and channels research, their roles in diverse pathological conditions, and the current contribution of calcium pathway-related drugs, which could help to develop new research and therapeutic strategy in intractable skin diseases.
Calcium-Sensing Receptors and Channels Mediated Ca2+ Signaling in Skin Function Maintenance
Ca2+ signaling is strictly regulated while both CaSR and calcium channels are essential components that allow Ca2+ to enter the cell in response to various stimuli. Signal transduction via CaSRs occurs mainly through the Gɑq pathway. G-protein activation further stimulates phospholipase C (PLC), which hydrolyzes phosphatidylinositol-4,5-bisphosphate (PIP2), generating inositol 1,4,5 triphosphate (IP3) and diacylglycerol (DAG). IP3 diffuses through the cytoplasm and binds to IP3 receptors (IP3Rs) on the surface of the ER/SR, thereby promoting Ca2+ transportation from these compartments to the cytoplasm. In addition, released Ca2+ and DAG stimulate protein kinase C (PKC), which phosphorylates other molecules and further generate productions (Dhyani et al., 2020). Elevated [Ca2+]i regulate cell cycle via Ca2+/CaM pathway. The main downstream targets-Ca2+/CaM-kinases (CaMK) and calcineurin (CaN), could regulate transcription factors such as NFAT and NFκB, to influence cell function (Figure 1). During the process, various calcium channels may act independently or in concert to assist the Ca2+ signaling transduction and mediate diverse biological functions.
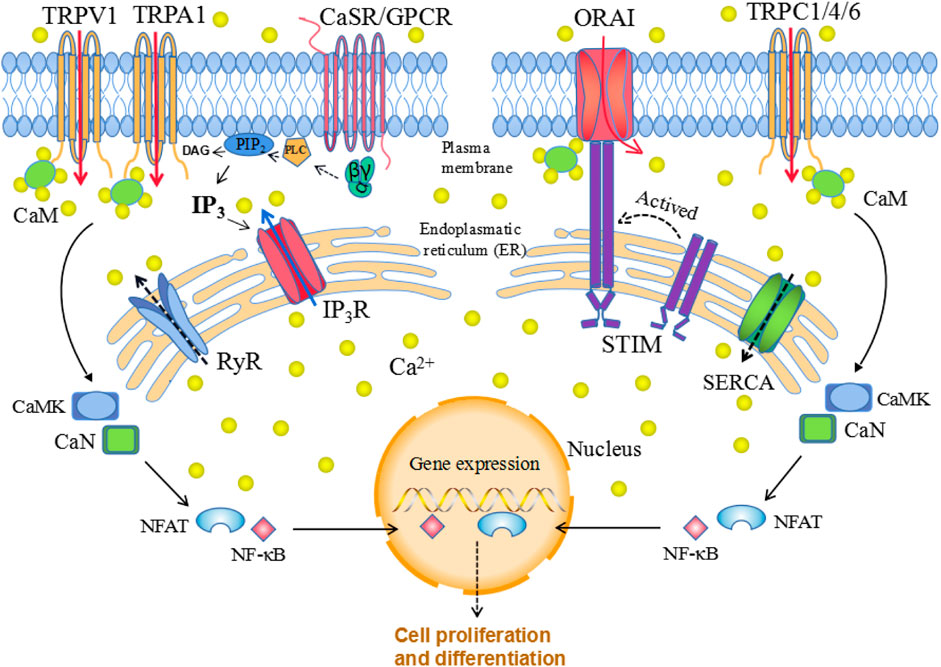
FIGURE 1. Cellular calcium homeostasis. The calcium-sensing receptor (CaSR), which is a G protein-coupled receptor (GPCR), senses changes in extracellular calcium concentrations, leading to phospholipase C (PLC) activation; activated PLC then hydrolyzes phosphatidylinositol-4,5-bisphosphate (PIP2), generating inositol 1,4,5-trisphosphate (IP3) and diacylglycerol (DAG). The binding of IP3 to the IP3 receptor (IP3R) located in the ER allows Ca2+ release from the ER. When the ER Ca2+ stores are depleted, this is sensed by STIM family proteins localized in the ER membrane, which translocate to the plasma membrane and interact with ORAI, thereby triggering selective store-operated Ca2+ entry (SOCE). Similarly, release of Ca2+ through ryanodine receptors (RyRs) is pumped back into the endoplasmic reticulum calcium through ATPase (SERCA). Elevated [Ca2+]i regulate gene expression via Ca2+/CaM pathway. The main downstream targets: Ca2+/CaM-kinases (CaMK) and calcineurin (CaN), could regulate transcription factors such as NFAT and NFκB, to influence cell function (Figure 1).
Voltage-Gated Calcium (Cav) Channels
Electrical activities in the body are mostly transmitted via the plasma membrane. Voltage-gated calcium (CaV) channels are transmembrane protein activated by depolarization of membrane potential (Andrade et al., 2019) and responsible for converting membrane electrical signals to intracellular Ca2+ transients (Wu et al., 2017), leading to the activation of multiple physiological events. CaVs consist of a complex of α1 subunit associated with β, γ, and α2δ subunits (Zamponi et al., 2015). The α1 subunits contain four homologous domains with six transmembrane segments (Mikami et al., 1989), where transmembrane segments S1–S4 form the voltage-sensing module, and S5 and S6 form the pore1 (Tang et al., 2016). When stimulated, cell membranes depolarize and action potentials occur. Voltage-sensing module senses the changes of potential and mediate Ca2+ influx (Catterall et al., 2011). Most physiological phenomena, including muscle contraction, synaptic transmission, hormone secretion, gene expression, and cell death, are stimulated by Ca2+, which functions as a universal second messenger. At the same time, VGCCs was demonstrated to provide Ca2+ with access to the intracellular environment (Catterall, 2011; Wu et al., 2017).
There are 10 subtypes of VGCCs, which can be classified into three subfamilies: Cav1, Cav2, and Cav3. The Cav1 channels, designated as L-type calcium channels, are activated at high voltage to conduct large and long-lasting ion currents (Tsien et al., 1988). Cav2 channels can be classified as P/Q-type, N-type, and R-type based on their current properties and inhibition (Nowycky et al., 1985; Randall and Tsien, 1995). Meanwhile, Cav3 channels, designated as T-type calcium channels, are activated at low voltage and conduct transient currents (Carbone and Lux, 1984; Nowycky et al., 1985). Das et al. (2012) identified that normal melanocytes and melanoma cells both express Cav1, Cav2 channels, while only melanoma cells express Cav3 (T-type) channels. Das et al. (2012) also found that T-type channel blocker kurtoxin could reduce viability and proliferation of the melanoma cells, encouraging T-type channels as potential pharmacological therapy targets. VGCCs are also found to exist on epidermal keratinocytes. Denda et al. (2006) demonstrated that the influx of Ca2+ ions into epidermal keratinocytes delay the barrier recovery, while the administration of nifedipine, verapamil, or R-(+)-BAY K8644, inhibitors of VGCC, accelerate the barrier recovery. When skin barrier is impaired, its electric potential is changed, generating negative electric potentials to promote skin barrier repair (Denda and Kumazawa, 2002). These findings indicate that VGCCs are associated with skin homeostasis and play a negative role in skin barrier recovery.
Transient Receptor Potential Channels
Transient receptor potential (TRP) genes were firstly described in Drosophila melanogaster. The study identified that a visually impaired mutant fly showed a transient instead of sustained response to steady light (Cosens and Manning, 1969). The trp gene as well as the structure and localization of the trp protein was identified 2 decades later by Montell and Rubin (Montell and Rubin, 1989). In humans the first TRP-encoding gene was only reported in 1995 (Wes et al., 1995). Since then, approximately 30 TRP-related genes and 27 different TRP channels in human have been identified (Li, 2017). TRP channels act as communication stations for cells and their functions are cell type-dependent. In neurons, for instance, activated TRP channels may cause depolarization and stimulate electric potential generation. However, in non-excitable cells, TRP channels regulate intracellular calcium concentrations, which are related to keratinocytes proliferation and differentiation to influence skin barrier (Moran et al., 2018). TRP channels were originally described as “polymodal cellular sensors” (Clapham, 2003; Damann et al., 2008; Vay et al., 2012); but they are now considered to be “promiscuous pleiotropic molecules” that can also be triggered by multiple physical, chemical, and other relevant factors (Ramsey et al., 2006; Vriens et al., 2008; Vriens et al., 2009).
TRP channels can be classified into several types depending on their structure. There are seven TRP subfamilies: TRPC (canonical), TRPV (vanilloid), TRPM (melastatin), TRPA (ankyrin), TRPP (polycystin), TRPML (mucolipin), and TRPN (Drosophila NompC) (Goel et al., 2002; Montell et al., 2002; Atoyan et al., 2009). An eighth TRP family was recently identified in yeast and named as TRPY (Li et al., 2017). Based on their homology to Drosophila TRP, TRP subfamilies are classified into group 1 TRP channels, which shows the greatest similarity with Drosophila TRP channels and includes TRPC, TRPV, TRPM, and TRPA; and group 2 TRP channels, - TRPP and TRPM - that are more distantly related to the TRP channels of Drosophila (Li et al., 2017). Emerging evidence suggests that several TRP channels (Alexander et al., 2013) are involved in cutaneous disorders, such as atopic dermatitis (AD), psoriasis, acne vulgaris, various forms of dermatitis, hair growth disorders, and cutaneous malignancies (Toth et al., 2014) (Figure 2).
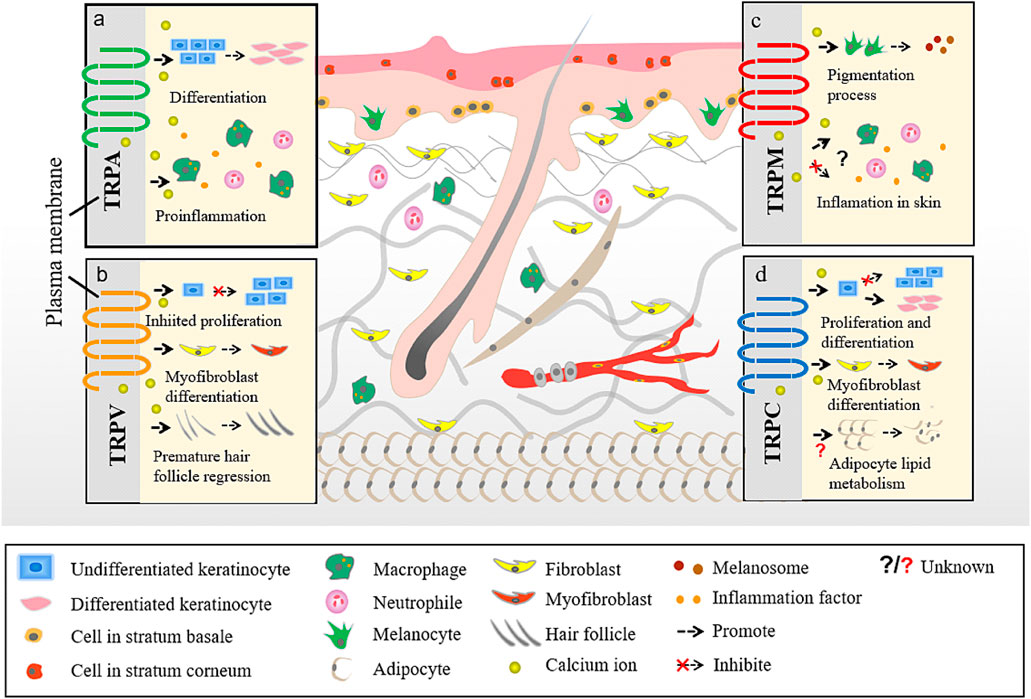
FIGURE 2. Different TRP channels participate in different skin homoeostasis and barrier function. a. The interaction between TRPA and skin. b. The interaction between TRPV and skin. c. The interaction between TRPM and skin. d. The interaction between TRPC and skin.
TRPC
The TRPC family consists of seven members (TRPC1-7), which are highly related to Drosophila TRP channels. TRPCs are non-selective cation channels that are expressed in both excitable and non-excitable cells and regulate intracellular Ca2+ influx in response to numerous physiological or pathological stimuli (Feng, 2017). Increased Ca2+ influx causes membrane depolarization and cytosolic Ca2+ concentration ([Ca2+]c) elevation, both causing critical effect on cell function (Wang et al., 2020). Studies have reported the role of TRPCs in diabetic kidney disease and myocardial injury (He et al., 2017; Ilatovskaya et al., 2018).Blocking of TRPC activity protects the function of podocytes and cardiac cells, indicating TRPC as a promising target for pharmacologic intervention. Moreover, TRPCs are also expressed in human epithelial cells in the epidermis (Tu et al., 2005) and are shown to play a role in Darier’s disease by regulating keratinocyte proliferation and differentiation (Beck et al., 2008).
During fetal development, TRPC1 expression is higher in the brain than in the liver and kidney, while in the adult it is primarily active in the heart, testes, ovary, and numerous regions of the brain (Strubing et al., 2001). In humans, TRPC2 is classified as a pseudogene (Montell et al., 2002). In the epidermis, TRPC1 contributes to keratinocytes differentiation by regulating Ca2+ influx (Cai et al., 2006). TRPC1 on the surface of endothelial cells was demonstrated to interact with both soluble α-Klotho, which is a pleiotropic molecule with multiple effects (e.g., antioxidant and vascular-protective), and vascular endothelial growth factor (VEGF)/VEGF receptor 2, which regulates Ca2+ influx and plasma membrane permeability, thereby contributing to the maintenance of endothelial cell integrity (Mazzotta et al., 2017). Dysregulated TRPC1/3 mRNA in adipocytes cultured from human subcutaneous white adipose tissue has been linked to obesity, diabetes, and cardiovascular disease (Ondrusova et al., 2017). In addition, the expression of TRPC3 has been associated with other skin diseases, such as melanoma, which exhibits an extremely poor prognosis owing to its rapidly progressive and highly metastatic nature. The pharmacological inhibition of TRPC3 by Pyr3, a pyrazol compound, was shown to decrease melanoma cell proliferation and migration, and knockdown of TRPC3 reduced melanoma cell proliferation (Oda et al., 2017).
The trans-differentiation of fibroblasts into myofibroblasts induced by damaged conditions can secrete extracellular matrix to promote wound healing. TRPC6 expression, mediated by noncanonical TGF-β signaling through p38/SRF, activates the Ca2+-responsive protein phosphatase calcineurin, leading to the induction of myofibroblast trans-differentiation (Davis et al., 2012). In the epidermis, TRPC6 is primarily expressed in keratinocytes of the stratum spinosum and stratum granulosum, where keratinocytes undergo differentiation, but not in the basal layer, which suggests that TRPC6 may regulate Ca2+-induced keratinocyte differentiation (Muller et al., 2008). Furthermore, hyperforin, a major component of St. John’s wort, could also activate TRPC6 to enhance Ca2+ entry and ATP-Ca2+ signaling in keratinocytes, thereby contributing to cutaneous wound healing (Takada et al., 2017). Similarly, there is also in vivo and in vitro evidence that the TRPC4 protein is expressed in the cell membrane and cytoplasm of gingival keratinocytes and regulate CaSR-induced increase in intracellular [Ca2+] (Fatherazi et al., 2007).
Additionally, some members of the TRPC family can also interact with each other and may even function as a complex; for instance, TRPC3 can act as a STIM1-dependent SOCC only, by assembling with TRPC1, while TRPC6 functions as a STIM1-dependent channel only, in the presence of TRPC4 (Yuan et al., 2007). STIM1 regulates TRPC1 and TRPC4, which also mediate store-operated Ca2+ entry, and influence keratinocytes differentiation and formation of epidermis barrier via changing Ca2+ concentration (Lee and Lee, 2018).
TRPV
TRPVs are sensitive to various tissue-damaging signals and their activation is generally perceived as pain. It is also demonstrated that thermo-TRP channels such as TRPV1, TRPV2, TRPV3, and TRPV4 can be activated by heat. The TRPV subfamily consists of six members (TRPV1-6) (Li et al., 2017). The immunoreactivity of TRPV1-4 has been differentially identified on basal and supra-basal cells of healthy human skin, and these channels have been proposed to function as thermo-sensory receptors (Chung et al., 2004; Radtke et al., 2011). TRPV5 and TRPV6 proves to be epithelial calcium ion channels, while TRPV1-4 which are referred to as nociceptor that sense the damaging signals (Satheesh et al., 2016).
TRPV1 is a sensor of noxious heat, capsaicin, and protons (low pH) (Caterina et al., 1997), and could also respond to UV stimulation. Hair shaft elongation and matrix keratinocyte proliferation can be inhibited by TRPV1 stimulation and lead to premature hair follicle regression and cell apoptosis (Toth et al., 2009). The application of AEA, an endocannabinoid anandamide, can suppress epidermal cell proliferation and induce cell death due to Ca2+ entry through TRPV1 and the concomitant elevation of the intracellular Ca2+ concentration (Toth et al., 2011). A recent study reported that the inhibition of TRPV1 in differentiated human primary keratinocytes abrogated proteinase-activated receptor-2 (PAR-2) activating peptide SLIGKV, which evoked Ca2+ store depletion and the production of inflammatory mediators (Gouin et al., 2018). These findings indicate that hyperactive TRPV1 may inhibit keratinocytes proliferation and promote inflammation.
TRPV2 is regulated by temperature, ligands such as probenecid and cannabinoids, and lipids. TRPV2 shares a high sequence identity with TRPV1 (>50%), but exhibits a higher temperature threshold and sensitivity for activation than TRPV1 (Zubcevic et al., 2016). Unlike TRPV1, there is little evidence for the presence of TRPV2 in human epithelial cells, except for one study that demonstrated the presence of TRPV2 in human skin by immunostaining. Moreover, there is no existing data regarding the physiological role of TRPV2 in epithelial cells (Radtke et al., 2011). However, in a vitro wound healing model of rats, in a culture model of wound healing, compounds targeting TRPV2 channels were suggested to ameliorate excessive wound contraction through the inhibition of TGF-β1 release and the differentiation of dermal fibroblasts (Ishii et al., 2018).
TRPV3 is responsive to warm temperatures (<33°C) and is predominantly expressed in skin keratinocytes, where it mediates warm and pain sensation (Peier et al., 2002; Smith et al., 2002). A study showed that activated TRPV3 increases thymic stromal lymphopoietin (TSLP), nerve growth factor, prostaglandin E2, and interleukin (IL)-33 production in human keratinocytes and induces scratching behavior in mice (Seo et al., 2020). The stimulation of TRPV3 can also trigger a strong proinflammatory response via the NF-κ B pathway (Szollosi et al., 2018). Studies have also reported that the expression of TRPV3 and TSLP is increased in the tissues of pruritic burn scars (Park et al., 2017), and that TRPV3 induces myofibroblast differentiation, collagen production, and TSLP expression through the TRPV3-Smad2/3 signaling pathway (Um et al., 2020). These results suggest a direct interaction between TRPV3 and pruritus diseases, hence the inhibition of TRPV3 may be a promising therapy target.
Similar to TRPV3, TRPV4 can also be activated by warm temperature (Guler et al., 2002; Watanabe et al., 2002) and was initially reported to be an osmo- or mechano-sensor (Liedtke et al., 2000). TRPV4 is widely expressed throughout the body (Sokabe et al., 2010). In TRPV4-deficient mice, the epidermal barrier is impaired, and displays characteristics such as leaky cell-cell junctions, non-physiological actin rearrangements, and insufficient stratification (Kida et al., 2012). Ammar et al. (Boudaka et al., 2020) found that both cell migration and proliferation were slower in wild-type esophageal keratinocytes than in those with TRPV4 knockout cells. In addition, Adapala et al. (2013) found TRPV4 as a requirement for the TGF-β1-induced differentiation of cardiac fibroblasts into myofibroblasts. The application of AB159908, a TRPV4-specific antagonist, or siRNA knockdown of TRPV4 significantly inhibited TGFβ1-induced differentiation. The role of TRPV4 in cardiac fibroblasts has been demonstrated, but whether TRPV4 functions in dermal fibroblasts of skin, or participates in wound healing and skin barrier homeostasis remain questions that require further studies.
TRPV5 and TRPV6, both calcium-selective channels, are expressed at the apical membrane of Ca2+-transporting epithelia, and serve as entry channels in transepithelial Ca2+ transport (Flores-Aldama et al., 2020). TRPV6, which is also involved in skin barrier formation and function, was shown to play a crucial role in the terminal differentiation process induced by elevated extracellular Ca2+ concentrations (Lehen'Kyi et al., 2007). Furthermore, keratinocytes lacking TRPV6 exhibited a loss of close contacts between adjacent cells and the ability to flatten (Elsholz et al., 2014).
TRPA
TRPA1, an exclusive member of the TRPA family, is widely expressed in sensory neurons and in non-neuronal cells such as epithelial cells and hair cells, and can be activated by noxious external stimuli and low temperature (Talavera et al., 2020). As the members of the TRPV subfamily, TRPA1 is also involved in regulating epidermal cell biological function (Toth et al., 2014). For instance, it has been demonstrated that the topical application of TRPA1 agonists could accelerate barrier recovery from skin permeability (Denda et al., 2010). A recent study also showed that inhibitors of TRPA1 and a neurogenic inflammatory peptide released following TRPA1 activation- CGRP, reduced skin edema and the levels of proinflammatory cytokines (Achanta et al., 2018). In addition, activated TRPA1 has been associated with some of the symptoms of irritant contact dermatitis, such as pain, neurogenic inflammation, and, possibly, itching (Noroes et al., 2019). All these findings suggest that TRPA1 contributes to skin injury and inflammation.
TRPM
As intrinsic membrane proteins, the TRPM subfamily is divided into eight variable types, i.e., TRPM1-8 (Clapham et al., 2001). TRPMs are Ca2+-permeable cation channels except for TRPM4 and 5 (Ullrich et al., 2005; Hof et al., 2016). Human epidermal melanocytes express TRPM1, which is shown to be essential for the pigmentation process (Devi et al., 2009; Oancea et al., 2009). Just as TRPV1 is a key heat detector, TRPM8 is mainly sensitive to environmental cold (Moran, 2018). In contrast to TRPA1, TRPM8 does not seem to contribute to phthalate-induced skin hypersensitivity, as it is not activated by dibutyl phthalate (Kurohane et al., 2013). To date, TRPM8 has not been linked to skin homeostasis or dermatitis (Caterina, 2014). However, its activation can suppress chemically evoked irritation and inhibit TRPV1-mediated CGRP release in colon tissue (Ramachandran et al., 2013), and can also induce an increase in the level of some proinflammatory cytokines in the blood of normotensive rats (Kozyreva et al., 2016).
Store-Operated Ca2+ Channels
As the major store of intracellular Ca2+, ER Ca2+ release could be triggered by IP3,a critical second messenger, causing ER Ca2+ release into the cytosol. (Putney, 2015). proposed a ‘capacitative Ca2+ entry’ hypothesis that the emptying of Ca2+ stores itself activates Ca2+ channels in the plasma membrane to help refill the stores. The hypothesis later renamed store-operated Ca2+ entry, or SOCE (Lewis, 2007). Study also identified that SOCE not only provides Ca2+ for refilling stores, but can itself generate sustained Ca2+ signals that control such essential functions as gene expression, cell metabolism and exocytosis (Parekh and Putney., 2005). Stromal interaction molecule (STIM) proteins (STIM1 and STIM2), are identified as Ca2+ sensors for SOCE. Once Ca2+ store depletion is sensed, STIM will translocate to plasma membrane and activate ORAI Ca2+ channels, the store-operated Ca2+ channel (Umemura et al., 2014; Prakriya and Lewis, 2015). Moreover, Hooper and Soboloff, 2015 found SOCE can be promoted by STIM-activating enhancers, such as STIMATE, which is located in the ER membrane, can translocate to ER-PM junctions where it can facilitate SOCE.
SOCE participates in many biological events, including gene expression, cell metabolism, tumor progression. Umemura et al. (2014) found SOCE to contribute to melanoma progression via the CaMKII/Raf-1/ERK signaling pathway. Additionally, STIM2-gated ORAI1 Ca2+ channels may regulate melanoma, because Stanisz et al. (2014) demonstrated that ORAI1 and STIM2 are highly expressed and control store-operated Ca2+ entry in human melanoma, with silencing of ORAI1 and/or STIM2 inhibiting the ability of proliferation, invasion, and migration of melanoma cells. - These findings indicate that ORAI1 and STIM1/STIM2 are potentially useful therapeutic targets for preventing tumor metastasis. STIM1, rather than ORAI channels also mediates Ca2+-cAMP crosstalk and pigmentation via oligomerization (Motiani et al., 2018).
The ORAI1 protein was shown to be mainly confined to the basal epidermal layer where it plays a critical role in controlling epithelial cell proliferation and polarized motility (Vandenberghe et al., 2013). Numaga-Tomita and Putney, 2013 also found the knockdown of either STIM1 or ORAI1 to strongly suppress SOCE, causing impaired expression of keratin1, an early keratinocytes differentiation marker, and the inhibition of normal growth of HaCaT cells in low Ca2+. Additionally, decreased SOCE in ORAI1-, ORAI2-, and ORAI1/2-deficient immune cells, such as neutrophils, impairs multiple cellular functions, including phagocytosis, degranulation, leukotriene expression, and reactive oxygen species (ROS) production (Grimes et al., 2020). Similarly, ORAI1 and ORAI2 can form a heteromorphic channel complex, in which ORAI2 attenuates the function of ORAI1 and limits SOCE, while ORAI2 fine-tunes the magnitude of SOCE to modulate immune responses (Vaeth et al., 2017). These observations suggest that ORAI proteins may be associated with the skin barrier and immune functions.
ORAI is also reported to interfere with TRP channels. ORAI1 and TRPV1 were found to associate and move in close proximity to each other at the plasma membrane, and Ca2+ entering the cell through TRPV1 channels induced strong calcium-dependent ORAI1 inactivation, which influenced cell migration and wound healing (Bastian-Eugenio et al., 2019). Moreover, Woo et al. (2021) synthesized a chemical derivative of valencene, nootkatol, which could inhibit secretion of collagen-degrading enzymes and matrix metalloproteinase-1 (MMP-1) in keratinocytes via TRPV1 and hyperpigmentation in melanocytes via ORAI1, and as well prevents ultraviolet radiation-induced photoaging. TRPV3/4 and ORAI1 work together in keratinocytes could mediated skin barrier formation, (Saul et al., 2014). This finding also provides a promising strategy in targeting ion channels for preventing photoaging.
Inositol 1,4,5-Trisphosphate Receptor and Ryanodine Receptor
Ca2+ release from intracellular stores, mainly ER/SR, is mediated by intracellular ligand-gated Ca2+ release channels. Two closely related families of intracellular Ca2+ release channels have been identified: the inositol 1,4,5-trisphosphate receptor (IP3R) and the ryanodine receptor (RyR). IP3R channels are located in all cell types with the highest densities in the Purkinje cells of cerebellum while the RyR represents primary Ca2+ release channel in striated muscle. Due to structural homology and similar physiology, there are many functional similarities between IP3R and RyR channels (Serysheva., 2014). In resting cells, cytoplasmic Ca2+ concentration is maintained at ∼ 100 nM, which is lower than extracellular Ca2+ concentration and Ca2+ concentration in ER Ca2+ store. The activation of IP3R and RyR could increase transitorily cytoplasmic Ca2+ concentration.
The activity of IP3R channels is regulated by coupled interplay between the binding of its primary ligands, IP3 and Ca2+. IP3 is also a second messenger produced through phosphoinositide turnover in response to many extracellular stimuli such as hormones, growth factors, neurotransmitters, neurotrophins, odorants, and light (Fedorenko et al., 2014). Tu et al. (2005) indicated TRPC1 interacts with PLCgamma1 and IP3R, causing the activation of SOC in human keratinocytes. Schmitt et al. (2021) recently demonstrated that the inhibition of phospholipase C (PLC), blocks IP3R Ca2+ release, ameliorating alterations of autoantibodies targeting Dsg1 and Dsg3 localization and improving blistering of human epidermis in pemphigus. IP3 binds to IP3R causing the release of Ca2+ from intracellular stores and elevation of cytoplasmic free Ca2+ concentration, which triggers diverse cellular actions, ranging from contraction to secretion, from proliferation to cell death (Baker et al., 2021). Furthermore, IP3R interacts with Ca2+ in a biphasic manner, i.e. activation at low concentrations (up to 0.3 μM) and inhibition at higher concentrations (0.5–1 μM) (Bezprozvanny et al., 1991) and IP3R activity is also regulated by Ca2+-independent accessory proteins, Mg2+, redox potential and ATP (Thrower et al., 2001).
RyRs play an important role in the regulation of intracellular calcium levels in the nervous system and muscle. Sumiko et al. (Denda et al., 2012) reported that RyR1 is strongly expressed in differentiated layers of the epidermis; RyR2 is also expressed in the differentiated layers, especially in the border layer between the stratum corneum and the granular layer; and RyR3 is expressed throughout the epidermis, although its expression is stronger in differentiated layers than in basal layers. A different study showed that the inhibition of RyRs can accelerate wound closure in vivo, while in HaCaT cells (keratinocytes), wound closure is accelerated by treatment with dantrolene, a RyR antagonist (Degovics et al., 2019). These results suggest that in the epidermis, RyRs are associated with both the differentiation of epithelial cells and epidermal barrier homeostasis. Moreover, the application of RyR antagonists may have therapeutic potential for wound treatment. RyRs and other Ca2+ channels, their effectors, and impacts on wound healing are summarized in Figure3.
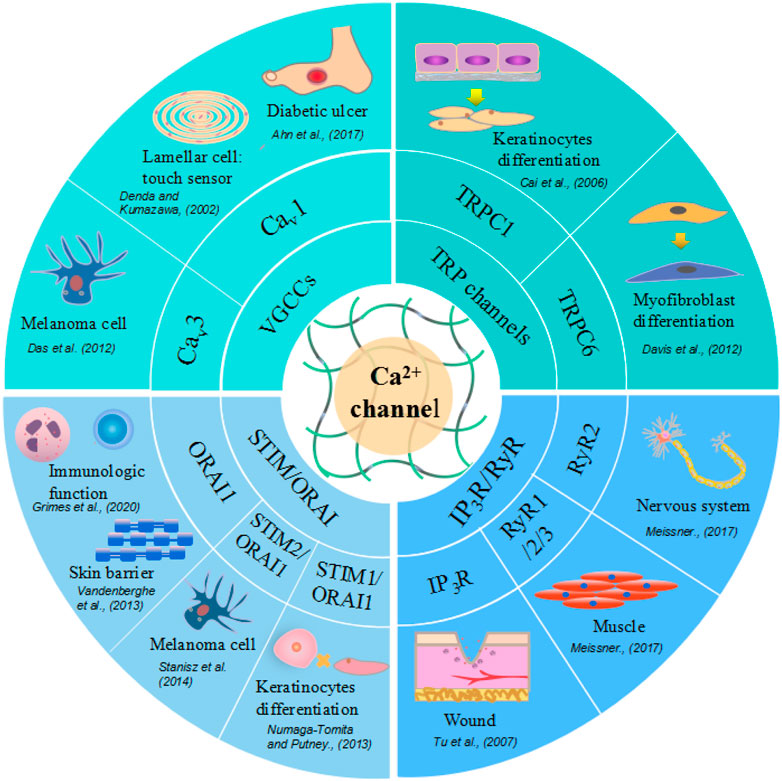
FIGURE 3. Different calcium ion channels and related skin cells and physiological function. Voltage-Gated Calcium (Cav) Channels are lamellar cell touch sensors of skin, with increased expression in melanoma cells and decreased in diabetic ulcer. TRP channels facilitate keratinocytes and myofibroblast differentiation. STIM or ORAI promote keratinocytes proliferation, skin barrier formation and regulate immunologic function. Ryanodine receptors express in nervous system, muscle, brain tissue and also function in wound healing.
Dermatological Diseases
Given calcium channels and their connection with the skin, it is highly likely that calcium channels, especially TRP channels, are involved in certain skin disorders. There is mounting evidence that aberrant TRP channel expression and function contributes to several skin diseases associated with altered cell differentiation and proliferation (Table 1).
Atopic Dermatitis
AD is a common chronic inflammatory skin disorder that results from complex interactions between genetic and environmental factors (Liang et al., 2016). The symptoms of this disease include intense itching, frequent exacerbation (Furue and Kadono, 2015), dry skin in characteristic locations, and pruritus (Bos et al., 2010; Wolf and Wolf, 2012). AD is considered to be a biphasic, T cell-mediated disease (Furue and Kadono, 2017). The T helper (Th) 2 signal predominates in the acute phase, whereas a Th2-Th1 switch promotes disease chronicity (Gittler et al., 2012; Guttman-Yassky et al., 2018).
TRPC6 dysfunction in epithelial cells is found to trigger AD pathogenesis through inhibiting Ca2+ influx, perturbing cell differentiation, and impairing epidermal barrier functions (Sun et al., 2012). Hyperforin, a TRPC6 activator, could partially restore the impaired differentiation of psoriatic keratinocytes (Leuner et al., 2011). Interestingly, the topical application of hyperforin-containing cream can improve the symptoms of AD (Schempp et al., 2003) (Table 2). TRPC6 has potential as a target for AD treatment; however, further preclinical and clinical studies are required to confirm this. Similarly, TRPM8 was reported to be a pharmacological target for tacrolimus (FK506), a macrolide immunosuppressant, for the treatment of AD (Arcas et al., 2019). Lee et al. (2019) reported a novel, topical, nonsteroidal antagonist of TRPV1, PAC-14028 cream, which could moderate atopic dermatitis with promising efficacy and safety. Moreover, treatment with TRPV1 antagonist also ameliorates barrier integrity, as measured by the levels of trans-epidermal water loss and differentiation markers, filaggrin and loricrin (Yun et al., 2011; Yun et al., 2011). Mutations have been identified in the TRPV3 gene, thereby linking TRPV3 to pruritus and AD (Asakawa et al., 2006; Yoshioka et al., 2009). TRPV3 is upregulated in the skin of MC903-induced AD mouse model and the pharmacologic inhibition of TRPV3, attenuates production of inflammation factors and scratching behavior induced by AD (Seo et al., 2020), suggesting that TRPV3 may be a potential therapeutic target for AD. TRPV3 knockout mice exhibit curly whiskers and body fur abnormalities (Cheng et al., 2010), while hairless DS-Nh mice also exhibit signs of spontaneous dermatitis, resembling human AD (Asakawa et al., 2006). Nattkemper et al. (2018) also reported increased expression of genes for TRPV2 and TRPA1 in pruritic atopic skin, and elevated expression of genes for TRPM8 and TRPV3 in pruritic psoriatic skin (Nattkemper et al., 2018). Impaired keratinocyte differentiation is a key feature of AD, whereas inflammatory reaction and TRPC6 activation is sufficient for keratinocyte differentiation. TRPV1, TRPV3, or TRPM8 are responsible for secretion of proinflammation factors and aggravate clinical symptom. This suggests TRPC6, TRPV1, TRPV3 or TRPM8 play a role in development of AD, they may play the parts through different mechanisms and the possibility of existing interaction between them remains unclear. This may account for the different effect of TRPC6 and TRPV1, TRPM8 or TRPV3 on AD.
Psoriasis
Psoriasis, a debilitating skin condition, is considered to be an autoimmunity-mediated disease involving several components of the immune system, including neutrophils (Toichi et al., 2000). In a mouse model of psoriasis, targeted knockout of STIM1 in myeloid lineage cells (including neutrophils) hastened the reversal of psoriatic plaques following the removal of a chemical activator of psoriasis (Steinckwich et al., 2015).
TRPM8 activated by thymol could attenuate the enhanced infiltration of dermal immune cells and downregulate expression of pro-inflammatory cytokines (Wang et al., 2020). Another study reported that TRPCs, including TRPC1, TRPC4, TRPC5, TRPC6, and TRPC7, are inhibited in the epidermis in situ and in vitro-cultured epithelial cells derived from psoriasis patients (Karvonen et al., 2000). Compared with healthy cells, psoriatic epithelial cells show a diminished response after thapsigargin-mediated calcium store depletion, suggestive of impaired SOCE. Furthermore, exposing cultured psoriatic epithelial cells to high levels of extracellular Ca2+ leads to only a minor Ca2+ influx, which is most likely due to the impaired function of TRPCs on the cell surface membrane of epithelial cells (Leuner et al., 2011).
Darier’s Disease
Darier’s disease (DD) (Darier White’s disease, keratosis follicularis), a genetic skin disorder first described in 1889 by Darier and White, is characterized by the loss of intracellular adhesion and disordered keratinization leading to warty plaques and papules in the seborrheic areas (Cooper and Burge, 2003). The causal mutation is located in the ATP2A2 gene that codes for the type 2 SERCA, a protein that regulates intracellular calcium homeostasis by pumping cytosolic calcium back into the ER (Sakuntabhai et al., 1999; Celli et al., 2012). The expression of the SOCCs TRPC1 and TRPC4 increases in response to raised extracellular Ca2+ concentrations (Hovnanian et al., 2004). Importantly, epidermal keratinocytes from Darier’s patients and HaCaT keratinocytes in which SERCA2 expression is knocked down through siRNA treatment, display increased TRPC1 expression (Elsholz et al., 2014), which is speculated to be a compensatory mechanism (Pani and Singh, 2008).
Nonmelanoma Cancers
Skin cancers, the most frequently diagnosed malignancies in humans (Simoes et al., 2015), are broadly divided into melanoma and nonmelanoma types. Basal cell cancers (BCCs) account for 65–70% of all nonmelanoma skin cancers (Wieckiewicz et al., 2013). Additionally, the premalignant form of squamous cell carcinoma (SCC; actinic keratosis), another nonmelanoma skin cancer, accounts for more than 250,000 new cases in the United States annually (Ratushny et al., 2012). Both cancer subtypes originate in the basal layer of the epidermis (Fusi et al., 2014). In BCC, the lack of TRPC1 and TRPC4 protein in vitro can lead to diminished calcium entry after calcium-induced differentiation and subsequently to the failed differentiation of BCC cells (Beck et al., 2008). The induction of TRPC6-mediated Ca2+ influx by triterpenes in epithelial cells isolated from patients with actinic keratosis (in situ SCC) suppresses cell growth and promotes differentiation (Woelfle et al., 2010). Fusi et al., 2014 showed that TRPA1 protein and mRNA expression levels are significantly increased in skin biopsies from patients with solar keratosis, a premalignant form of nonmelanoma skin cancer. Other studies have shown that TRPV4 stimulation leads to the release of IL-8, which in turn, downregulates TRPV4 expression in a human keratinocyte cell line (HaCaT), and the selective reduction of TRPV4 expression may represent an early biomarker of skin carcinogenesis (Fusi et al., 2014).
Olmsted Syndrome
Olmsted syndrome is a rare genodermatosis belonging to the heterogeneous group of palmoplantar keratodermas (PPKs) (Duchatelet and Hovnanian, 2015). The clinical symptoms are variable, but typically severe and disabling (Kariminejad et al., 2014). Lin et al. identified that genetic mutations that alter the same (Gly573Ser, Gly573Cys) or a different residue (Trp692Gly) in TRPV3 are responsible for Olmsted syndrome in humans. Increased intracellular calcium concentrations can cause apoptosis and consequently, the characteristic hyperkeratosis seen in Olmsted syndrome patients (Lin et al., 2012). However, it has been speculated that the pathophysiology of Olmsted syndrome might not be explained solely by abnormal TRPV3 function in keratinocytes, but may also involve immune dysfunction arising in other cells such as cutaneous Langerhans cells (Danso-Abeam et al., 2013).
Prurigo Nodularis
Prurigo nodularis (PN) is a chronic disorder of the skin that is commonly characterized by the presence of multiple, firm, flesh-to-pink colored nodules on the extensor surfaces of the extremities (Stander et al., 2020). Continuous scratching is a major symptom, while itching and scratching of the lesions contribute to the cycle that makes this disease difficult to treat, thus reducing the quality of life of affected patients (Tsianakas et al., 2016). Although TRPV1 was identified as being highly expressed in pathological skin lesions of PN patients (Stander et al., 2004), as well as in UV-irradiated photo-aged and intrinsically aged skin (Lee et al., 2009). The exact mechanism underlying the role of TRPV1 in PN remains unclear.
Skin Aging and UV-Induced Diseases
Although the skin is incredibly durable and has an enormous regenerative capacity, it cannot escape aging due to the turnover rate of epidermal cells (Rinnerthaler et al., 2015). Skin aging leads to, among other effects, deleterious alterations in the structure and function of dermal collagen (Quan et al., 2021). With aging, the structure and function of the skin barrier changes. Some of the changes include, significant increase in the number of SC layers, impairment of SC integrity, delayed recovery after acute perturbation with tape stripping and the epidermis becomes thinner (Choi, 2019). The epidermal calcium gradient which acts as a regulatory signal for skin barrier homeostasis, also become disrupted. Many factors, both internal and external, are responsible for skin aging-associated changes.
Glycolic acid (GA) pretreatment, along with UVB irradiation, can synergistically induce TRPV1 expression in human keratinocytes (Tang et al., 2019), leading to Ca2+ entry and consequently, an enhanced release of cytoplasmic calcium and ER stress (Lai et al., 2011). The regulation of the performance of TRPV1 is still not clear. Lee, Y.M., et al. (2009) reported that TRPV1 expression in human skin epithelial cells can be increased in vivo by UV, concomitant with an upregulation of MMP-1 expression; the latter might be mediated at least in part, by PKC-dependent activation of TRPV1 and subsequent Ca2+ influx (Lee et al., 2009). Moreover, a novel TRPV1-inhibiting peptide was demonstrated to attenuate UV-induced erythema and the expression of MMP-1, MMP-2, IL-6, and IL-8 in human skin in vivo (Kang et al., 2017). Recently, a study found TRPC7 mediated UVB-induced Ca2+ influx, UVB-induced ROS production, and UVB-induced epidermal aging in mice (Hsu et al., 2020). These findings suggest that inhibition of specific TRP channels may delay skin aging. Whether other calcium ion channels are related to aging is yet to be discovered in further studies.
Burn Injury
Burn injuries are associated with substantial morbidity and mortality. Although burn injuries can be caused by friction, cold, heat, radiation, and chemical or electric sources, most are caused by heat from hot liquids, solids, or fire. Generally, burn injuries, particularly severe burns, trigger numerous physiological and pathophysiological responses such as metabolic changes, distributive shock, and inflammatory responses (Jeschke et al., 2020). Following burn injury, calcium will be released from ER calcium stores mediated by increased IP3R activity (Jeschke et al., 2009). TRPV1 is a noxious heat sensor and is associated with mechanical and thermal hyperalgesia after burn injury. The overexpression of fibulin-5, which can promote dermal wound healing, is shown to attenuate burn injury-induced inflammatory responses via the suppression of the TRPV1/CGRP pathway (Hou et al., 2017). Similarly, the expression of TRPV1 and TRPA1 is reported to be upregulated in epithelial cells of an alkali-burned cornea, and the absence of the TRPV1 and TRPA1 genes suppresses post-alkali burn inflammation and facilitates the final healing (Okada et al., 2011; Okada et al., 2014). These results suggest that TRPV1 and TRPA1 play a negative role in burn wound healing, although TRPV1/TRPM3/TRPA1 triple knockout mice lack the acute withdrawal response to noxious heat that is necessary to avoid burn injury (Vandewauw et al., 2018).
Diabetic Ulcer
Diabetes mellitus (DM) is fast becoming a lifestyle-related pandemic. Leg or foot ulcers are the most commonly occurring wounds in diabetic patients (Patel et al., 2019), and can lead to amputation in the most extreme cases. In a model of type-1 DM, the expression of Cav1.2 acting as a cellular calcium channel and plasma membrane Ca2+-ATPase (PMCA), one of the calcium transport-related factors decreases, indicating that the concentration of intracellular calcium gradually depletes (Ahn et al., 2017). Another study showed that azelnidipine (AZL), a new calcium channel blocker with selectivity for L-type voltage-operated calcium channels, facilitates diabetic wound healing via stimulating nitric oxide (NO) production and enhancing processes central to normal wound healing (Bagheri et al., 2011). Although several studies have highlighted the role of TRP channels in pancreatic β cells and in the regulation of insulin secretion (Philippaert and Vennekens, 2017), the potential influence of TRP channels in diabetic ulcers remains unclear and merits further investigation.
Concluding Remarks
As a signal of survival and death, Ca2+ regulate almost all physiological activities and impact nearly every aspect of cellular life. Calcium channels are participants and regulators in the maintenance of calcium dynamic balance and epidermal barrier. The roles of calcium channels in skin homeostasis and different dermatologic diseases have been gradually realized. Different types of calcium channel mediate Ca2+ influx and have similar or distinct function. Various calcium channels are connected with dermatologic diseases and several targeting drugs. The up- or down-regulation of Ca2+ channels may favor recovery of several dermatologic diseases. However, the exact mechanisms mediating the complex roles of relevant Ca2+ channels in dermatologic diseases have not yet been fully elucidated. Ca2+ channels would be effective molecules for targeted therapy and ideal biomarkers for skin cancer diagnosis. Although the role and potential of Ca2+ channels have been investigated in various skin injury and diseases, there are still a number of unknowns surrounding their interactions and skin repair mechanisms, providing focus for further future studies.
Here we highlight only few of them:
•It is not quite clear how the calcium current is changed during the wound healing process?
•How are TRP channels initially opened following wounding and whether the altered expression or function is a cause or a consequence?
•Does Ca2+ channels associate with triggering inflammation and/or switching off the wound healing response?
Ca2+ channels function both in excitable cells, such as neurons, smooth muscle cells via sensing potentials change potentially and the non-excitable cells, like skin cells. How do Ca2+ channels regulate differently on the distinct two types of cells?
There also exists a class of drugs, called calcium channel blockers (or calcium antagonists) that decrease the flow of Ca2+ through calcium channels and agonists which have the opposite effect. Whether it could be a therapy strategy?
In addition to the Ca2+channels mediating the influx of extracellular Ca2+into the cytoplasm, some transporters such as Ca2+pumps, cell membrane sodium-calcium exchangers, and endoplasmic reticulum Ca2+pumps, regulate the release of Ca2+from cell or influx into organelles. Calcium sensing receptors are critical to maintenance of organismal Ca2+ homeostasis. However, they sense not only Ca2+, but also the metabolic environment. They can be regulated by a variety of metabolic signals, including amino acids, polyamines, pH, cAMP, polyvalent cations, ionic strength, making CaSR capable of generating cell- and tissue-specific responses (Breitwieser et al., 2004). There exist other specific ion channels and transporters involved in the regulation of cellular calcium signaling in different cells. Various calcium channels, calcium transporters, and calcium receptors, interact with each other to maintain calcium homeostasis, and the dysfunction of any link may lead to the occurrence of disease. Due to different location, distribution, permeability, selectivity, dynamics and regulation factors of ion channels, the calcium signals generated have different temporal and spatial characteristics and different physiological functions.
Future studies of Ca2+ channels will not only shed lights on their roles in dermatologic diseases but will open new avenues for possible mechanism and further candidate drugs for therapeutics.
Author Contributions
MW and HS conceived the idea and designed the review; MW and YS are responsible for manuscript writing; LL, PW, and OD participated in review inspection.
Funding
This work was supported by the National Natural Science Youth Foundation of China (Grant 82001975), the Natural Science Youth Foundation of the Jiangsu Province (Grant BK20190841), Zhenjiang Major Project of Social Development in Research and Development (Grant SH2018029), Project funded by China Postdoctoral Science Foundation (2020M671371), Jiangsu Province “Entrepreneurship and Innovation Program”-Entrepreneurship and innovation Doctoral category. Top Talent Support Program for young and middle-aged people of Wuxi Health Committee (HB2020108), the Foundation of Clinical Science and Technology of Wuxi (No.Q202059), the Innovation Project for Graduate Student Research of Jiangsu Province (Grant No. KYCX21_3406).
Conflict of Interest
The authors declare that the research was conducted in the absence of any commercial or financial relationships that could be construed as a potential conflict of interest.
Publisher’s Note
All claims expressed in this article are solely those of the authors and do not necessarily represent those of their affiliated organizations, or those of the publisher, the editors and the reviewers. Any product that may be evaluated in this article, or claim that may be made by its manufacturer, is not guaranteed or endorsed by the publisher.
Acknowledgments
We thank the members of Xu Wenrong/Qian Hui Team for excellent assistance and helpful suggestions.
References
Achanta, S., Chintagari, N. R., Brackmann, M., Balakrishna, S., and Jordt, S.-E. (2018). TRPA1 and CGRP Antagonists Counteract Vesicant-Induced Skin Injury and Inflammation. Toxicol. Lett. 293, 140–148. doi:10.1016/j.toxlet.2018.03.007
Adapala, R. K., Thoppil, R. J., Luther, D. J., Paruchuri, S., Meszaros, J. G., Chilian, W. M., et al. (2013). TRPV4 Channels Mediate Cardiac Fibroblast Differentiation by Integrating Mechanical and Soluble Signals. J. Mol. Cell Cardiol. 54, 45–52. doi:10.1016/j.yjmcc.2012.10.016
Ahn, C., Kang, J.-H., and Jeung, E.-B. (2017). Calcium Homeostasis in Diabetes Mellitus. J. Vet. Sci. 18 (3), 261–266. doi:10.4142/jvs.2017.18.3.261
Alexander, S. P. H., Benson, H. E., Faccenda, E., Pawson, A. J., Sharman, J. L., Catterall, W. A., et al. (2013). The Concise Guide to PHARMACOLOGY 2013/14: Ion Channels. Br. J. Pharmacol. 170 (8), 1607–1651. doi:10.1111/bph.12447
Andrade, A., Brennecke, A., Mallat, S., Brown, J., Gomez-Rivadeneira, J., Czepiel, N., et al. (2019). Genetic Associations between Voltage-Gated Calcium Channels and Psychiatric Disorders. Ijms 20 (14), 3537. doi:10.3390/ijms20143537
Arcas, J. M., González, A., Gers-Barlag, K., González-González, O., Bech, F., Demirkhanyan, L., et al. (2019). The Immunosuppressant Macrolide Tacrolimus Activates Cold-Sensing TRPM8 Channels. J. Neurosci. 39 (6), 949–969. doi:10.1523/JNEUROSCI.1726-18.2018
Asakawa, M., Yoshioka, T., Matsutani, T., Hikita, I., Suzuki, M., Oshima, I., et al. (2006). Association of a Mutation in TRPV3 with Defective Hair Growth in Rodents. J. Invest. Dermatol. 126 (12), 2664–2672. doi:10.1038/sj.jid.5700468
Atoyan, R., Shander, D., and Botchkareva, N. V. (2009). Non-neuronal Expression of Transient Receptor Potential Type A1 (TRPA1) in Human Skin. J. Invest. Dermatol. 129 (9), 2312–2315. doi:10.1038/jid.2009.58
Bagheri, M., Jahromi, B. M., Mirkhani, H., Solhjou, Z., Noorafshan, A., Zamani, A., et al. (2011). Azelnidipine, a New Calcium Channel Blocker, Promotes Skin Wound Healing in Diabetic Rats. J. Surg. Res. 169 (1), e101–e107. doi:10.1016/j.jss.2011.02.039
Baker, M. R., Fan, G., Seryshev, A. B., Agosto, M. A., Baker, M. L., and Serysheva, I. I. (2021). Cryo-EM Structure of Type 1 IP3R Channel in a Lipid Bilayer. Commun. Biol. 4, 625. doi:10.1038/s42003-021-02156-4
Barceló, C., Sisó, P., Maiques, O., García-Mulero, S., Sanz-Pamplona, R., Navaridas, R., et al. (2020). T-type Calcium Channels as Potential Therapeutic Targets in Vemurafenib-Resistant BRAFV600E Melanoma. J. Invest. Dermatol. 140 (6), 1253–1265. doi:10.1016/j.jid.2019.11.014
Bastián-Eugenio, C. E., Bohórquez-Hernández, A., Pacheco, J., Sampieri, A., Asanov, A., Ocelotl-Oviedo, J. P., et al. (2019). Heterologous Calcium-dependent Inactivation of Orai1 by Neighboring TRPV1 Channels Modulates Cell Migration and Wound Healing. Commun. Biol. 2, 88. doi:10.1038/s42003-019-0338-1
Beck, B., Lehen’kyi, V. y., Roudbaraki, M., Flourakis, M., Charveron, M., Bordat, P., et al. (2008). TRPC Channels Determine Human Keratinocyte Differentiation: New Insight into Basal Cell Carcinoma. Cell Calcium 43 (5), 492–505. doi:10.1016/j.ceca.2007.08.005
Bezprozvanny, l., Watras, J., and Ehrlich, B. E. (1991). Bell-shaped Calcium-Response Curves of lns(l,4,5)P3- and Calcium-Gated Channels from Endoplasmic Reticulum of Cerebellum. Nature 351, 751–754. doi:10.1038/351751a0
Bos, J. D., Brenninkmeijer, E. E. A., Schram, M. E., Middelkamp-Hup, M. A., Spuls, P. I., and Smitt, J. H. S. (2010). Atopic Eczema or Atopiform Dermatitis. Exp. Dermatol. 19 (4), 325–331. doi:10.1111/j.1600-0625.2009.01024.x
Boudaka, A., Saito, C. T., and Tominaga, M. (2020). Deletion of TRPV4 Enhances In Vitro Wound Healing of Murine Esophageal Keratinocytes. Sci. Rep. 10 (1), 11349. doi:10.1038/s41598-020-68269-8
Breitwieser, G. E., Miedlich, S. U., and Zhang, M. (2004). Calcium Sensing Receptors as Integrators of Multiple Metabolic Signals. Cell Calcium 35, 209–216. doi:10.1016/j.ceca.2003.10.013
Cai, S., Fatherazi, S., Presland, R. B., Belton, C. M., Roberts, F. A., Goodwin, P. C., et al. (2006). Evidence that TRPC1 Contributes to Calcium-Induced Differentiation of Human Keratinocytes. Pflugers Arch. - Eur. J. Physiol. 452 (1), 43–52. doi:10.1007/s00424-005-0001-1
Carbone, E., and Lux, H. D. (1984). A Low Voltage-Activated, Fully Inactivating Ca Channel in Vertebrate Sensory Neurones. Nature 310 (5977), 501–502. doi:10.103b8/310501a010.1038/310501a0
Caterina, M. J., Schumacher, M. A., Tominaga, M., Rosen, T. A., Levine, J. D., and Julius, D. (1997). The Capsaicin Receptor: a Heat-Activated Ion Channel in the Pain Pathway. Nature 389 (6653), 816–824. doi:10.1038/39807
Caterina, M. J. (2014). TRP Channel Cannabinoid Receptors in Skin Sensation, Homeostasis, and Inflammation. ACS Chem. Neurosci. 5 (11), 1107–1116. doi:10.1021/cn5000919
Catterall, W. A. (2011). Voltage-gated Calcium Channels. Cold Spring Harbor Perspect. Biol. 3 (8), a003947. doi:10.1101/cshperspect.a00394710.1101/cshperspect.a003947
Celli, A., Mackenzie, D. S., Zhai, Y., Tu, C.-L., Bikle, D. D., Holleran, W. M., et al. (2012). SERCA2-Controlled Ca2+-dependent Keratinocyte Adhesion and Differentiation Is Mediated via the Sphingolipid Pathway: A Therapeutic Target for Darier's Disease. J. Invest. Dermatol. 132 (4), 1188–1195. doi:10.1038/jid.2011.447
Cheng, X., Jin, J., Hu, L., Shen, D., Dong, X.-p., Samie, M. A., et al. (2010). TRP Channel Regulates EGFR Signaling in Hair Morphogenesis and Skin Barrier Formation. Cell 141 (2), 331–343. doi:10.1016/j.cell.2010.03.013
Choi, E. H. (2019). Aging of the Skin Barrier. Clin. Dermatol. 37 (4), 336–345. doi:10.1016/j.clindermatol.2019.04.009
Chung, M.-K., Lee, H., Mizuno, A., Suzuki, M., and Caterina, M. J. (2004). TRPV3 and TRPV4 Mediate Warmth-Evoked Currents in Primary Mouse Keratinocytes. J. Biol. Chem. 279 (20), 21569–21575. doi:10.1074/jbc.M401872200
Clapham, D. E., Runnels, L. W., and Strübing, C. (2001). The TRP Ion Channel Family. Nat. Rev. Neurosci. 2 (6), 387–396. doi:10.1038/35077544
Clapham, D. E. (2003). TRP Channels as Cellular Sensors. Nature 426 (6966), 517–524. doi:10.1038/nature02196
Cooper, S. M., and Burge, S. M. (2003). Darier??s Disease. Am. J. Clin. Dermatol. 4 (2), 97–105. doi:10.2165/00128071-200304020-00003
Cosens, D. J., and Manning, A. (1969). Abnormal Electroretinogram from a Drosophila Mutant. Nature 224, 285–287. doi:10.1038/224285a0
Damann, N., Voets, T., and Nilius, B. (2008). TRPs in Our Senses. Curr. Biol. 18 (18), R880–R889. doi:10.1016/j.cub.2008.07.063
Danso-Abeam, D., Zhang, J., Dooley, J., Staats, K. A., Van Eyck, L., Van Brussel, T., et al. (2013). Olmsted Syndrome: Exploration of the Immunological Phenotype. Orphanet J. Rare Dis. 8, 79. doi:10.1186/1750-1172-8-79
Das, A., Pushparaj, C., Bahí, N., Sorolla, A., Herreros, J., Pamplona, R., et al. (2012). Functional Expression of Voltage-Gated Calcium Channels in Human Melanoma. Pigment Cel Melanoma Res 25 (2), 200–212. doi:10.1111/j.1755-148X.2012.00978.x10.1111/j.1755-148x.2012.00978.x
Davis, J., Burr, A. R., Davis, G. F., Birnbaumer, L., and Molkentin, J. D. (2012). A TRPC6-dependent Pathway for Myofibroblast Transdifferentiation and Wound Healing In Vivo. Dev. Cel 23 (4), 705–715. doi:10.1016/j.devcel.2012.08.017
Degovics, D., Hartmann, P., Németh, I. B., Árva-Nagy, N., Kaszonyi, E., Szél, E., et al. (2019). A Novel Target for the Promotion of Dermal Wound Healing: Ryanodine Receptors. Toxicol. Appl. Pharmacol. 366, 17–24. doi:10.1016/j.taap.2019.01.021
Denda, M., Fujiwara, S., and Hibino, T. (2006). Expression of Voltage-Gated Calcium Channel Subunit alpha1C in Epidermal Keratinocytes and Effects of Agonist and Antagonists of the Channel on Skin Barrier Homeostasis. Exp. Dermatol. 15 (6), 455–460. doi:10.1111/j.0906-6705.2006.00430.x10.1111/j.0906-6705.2006.00430.x
Denda, M., and Kumazawa, N. (2002). Negative Electric Potential Induces Alteration of Ion Gradient and Lamellar Body Secretion in the Epidermis, and Accelerates Skin Barrier Recovery after Barrier Disruption. J. Invest. Dermatol. 118 (1), 65–72. doi:10.1046/j.0022-202x.2001.01500.x10.1046/j.0022-202x.2001.01500.x
Denda, M., Tsutsumi, M., Goto, M., Ikeyama, K., and Denda, S. (2010). Topical Application of TRPA1 Agonists and Brief Cold Exposure Accelerate Skin Permeability Barrier Recovery. J. Invest. Dermatol. 130 (7), 1942–1945. doi:10.1038/jid.2010.32
Denda, S., Kumamoto, J., Takei, K., Tsutsumi, M., Aoki, H., and Denda, M. (2012). Ryanodine Receptors Are Expressed in Epidermal Keratinocytes and Associated with Keratinocyte Differentiation and Epidermal Permeability Barrier Homeostasis. J. Invest. Dermatol. 132 (1), 69–75. doi:10.1038/jid.2011.256
Devi, S., Kedlaya, R., Maddodi, N., Bhat, K. M. R., Weber, C. S., Valdivia, H., et al. (2009). Calcium Homeostasis in Human Melanocytes: Role of Transient Receptor Potential Melastatin 1 (TRPM1) and its Regulation by Ultraviolet Light. Am. J. Physiology-Cell Physiol. 297 (3), C679–C687. doi:10.1152/ajpcell.00092.2009
Dhyani, V., Gare, S., Gupta, R. K., Swain, S., Venkatesh, K. V., and Giri, L. (2020). GPCR Mediated Control of Calcium Dynamics: A Systems Perspective. Cell Signal. 74, 109717. doi:10.1016/j.cellsig.2020.109717
Duchatelet, S., and Hovnanian, A. (2015). Olmsted Syndrome: Clinical, Molecular and Therapeutic Aspects. Orphanet J. Rare Dis. 10, 33. doi:10.1186/s13023-015-0246-5
Fatherazi, S., Presland, R. B., Belton, C. M., Goodwin, P., Al-Qutub, M., Trbic, Z., et al. (2007). Evidence that TRPC4 Supports the Calcium Selective ICRAC-like Current in Human Gingival Keratinocytes. Pflugers Arch. - Eur. J. Physiol. 453 (6), 879–889. doi:10.1007/s00424-006-0156-4
Fedorenko, O. A., Popugaeva, E., Enomoto, M., Stathopulos, P. B., Ikura, M., and Bezprozvanny, I. (2014). Intracellular Calcium Channels: Inositol-1,4,5-Trisphosphate Receptors. Eur. J. Pharmacol. 739, 39–48. doi:10.1016/j.ejphar.2013.10.074
Feng, S. (2017). TRPC Channel Structure and Properties. Adv. Exp. Med. Biol. 976, 9–23. doi:10.1007/978-94-024-1088-4_2
Flores-Aldama, L., Vandewege, M. W., Zavala, K., Colenso, C. K., Gonzalez, W., Brauchi, S. E., et al. (2020). Evolutionary Analyses Reveal Independent Origins of Gene Repertoires and Structural Motifs Associated to Fast Inactivation in Calcium-Selective TRPV Channels. Sci. Rep. 10 (1), 8684. doi:10.1038/s41598-020-65679-6
Furue, M., and Kadono, T. (2017). "Inflammatory Skin March" in Atopic Dermatitis and Psoriasis. Inflamm. Res. 66 (10), 833–842. doi:10.1007/s00011-017-1065-z
Furue, M., and Kadono, T. (2015). New Therapies for Controlling Atopic Itch. J. Dermatol. 42 (9), 847–850. doi:10.1111/1346-8138.13060
Fusi, C., Materazzi, S., Minocci, D., Maio, V., Oranges, T., Massi, D., et al. (2014). Transient Receptor Potential Vanilloid 4 (TRPV4) Is Downregulated in Keratinocytes in Human Non-melanoma Skin Cancer. J. Invest. Dermatol. 134 (9), 2408–2417. doi:10.1038/jid.2014.145
Gittler, J. K., Shemer, A., Suárez-Fariñas, M., Fuentes-Duculan, J., Gulewicz, K. J., Wang, C. Q. F., et al. (2012). Progressive Activation of TH2/TH22 Cytokines and Selective Epidermal Proteins Characterizes Acute and Chronic Atopic Dermatitis. J. Allergy Clin. Immunol. 130 (6), 1344–1354. doi:10.1016/j.jaci.2012.07.012
Goel, M., Sinkins, W. G., and Schilling, W. P. (2002). Selective Association of TRPC Channel Subunits in Rat Brain Synaptosomes. J. Biol. Chem. 277 (50), 48303–48310. doi:10.1074/jbc.M207882200
Gouin, O., L'Herondelle, K., Buscaglia, P., Le Gall-Ianotto, C., Philippe, R., Legoux, N., et al. (2018). Major Role for TRPV1 and InsP3R in PAR2-Elicited Inflammatory Mediator Production in Differentiated Human Keratinocytes. J. Invest. Dermatol. 138 (7), 1564–1572. doi:10.1016/j.jid.2018.01.034
Greenberg, D. A. (1997). Calcium Channels in Neurological Disease. Ann. Neurol. 42, 275–282. doi:10.1002/ana.410420302
Grimes, D., Johnson, R., Pashos, M., Cummings, C., Kang, C., Sampedro, G. R., et al. (2020). ORAI1 and ORAI2 Modulate Murine Neutrophil Calcium Signaling, Cellular Activation, and Host Defense. Proc. Natl. Acad. Sci. USA 117 (39), 24403–24414. doi:10.1073/pnas.2008032117
Güler, A. D., Lee, H., Iida, T., Shimizu, I., Tominaga, M., and Caterina, M. (2002). Heat-evoked Activation of the Ion Channel, TRPV4. J. Neurosci. 22 (15), 6408–6414. doi:10.1523/jneurosci.22-15-06408.2002
Guttman-Yassky, E., Krueger, J. G., and Lebwohl, M. G. (2018). Systemic Immune Mechanisms in Atopic Dermatitis and Psoriasis with Implications for Treatment. Exp. Dermatol. 27 (4), 409–417. doi:10.1111/exd.13336
Hauser, A. S., Attwood, M. M., Rask-Andersen, M., Schiöth, H. B., and Gloriam, D. E. (2017). Trends in GPCR Drug Discovery: New Agents, Targets and Indications. Nat. Rev. Drug Discov. 16 (12), 829–842. doi:10.1038/nrd.2017.178
He, X., Li, S., Liu, B., Susperreguy, S., Formoso, K., Yao, J., et al. (2017). Major Contribution of the 3/6/7 Class of TRPC Channels to Myocardial Ischemia/reperfusion and Cellular Hypoxia/reoxygenation Injuries. Proc. Natl. Acad. Sci. USA 114 (23), E4582–E4591. doi:10.1073/pnas.1621384114
Hof, T., Sallé, L., Coulbault, L., Richer, R., Alexandre, J., Rouet, R., et al. (2016). TRPM4 Non-selective Cation Channels Influence Action Potentials in Rabbit Purkinje Fibres. J. Physiol. 594 (2), 295–306. doi:10.1113/JP271347
Holland, C., van Drunen, C., Denyer, J., Smart, K., Segboer, C., Terreehorst, I., et al. (2014). Inhibition of Capsaicin-Driven Nasal Hyper-Reactivity by SB-705498, a TRPV1 Antagonist. Br. J. Clin. Pharmacol. 77 (5), 777–788. doi:10.1111/bcp.12219
Hooper, R., and Soboloff, J. (2015). STIMATE Reveals a STIM1 Transitional State. Nat. Cel Biol 17 (10), 1232–1234. doi:10.1038/ncb3245
Hou, X., Li, H., Zhang, C., Wang, J., Li, X., and Li, X. (2017). Overexpression of Fibulin-5 Attenuates Burn-Induced Inflammation via TRPV1/CGRP Pathway. Exp. Cel Res. 357 (2), 320–327. doi:10.1016/j.yexcr.2017.05.029
Hovnanian, A. (2004). Darier's Disease: from Dyskeratosis to Endoplasmic Reticulum Calcium ATPase Deficiency. Biochem. Biophysical Res. Commun. 322 (4), 1237–1244. doi:10.1016/j.bbrc.2004.08.067
Hsu, W. L., Tsai, M. H., Wu, C. Y., Liang, J. L., Lu, J. H., Kahle, J. S., et al. (2020). Nociceptive Transient Receptor Potential Canonical 7 (TRPC7) Mediates Aging‐associated Tumorigenesis Induced by Ultraviolet B. Aging Cell 19 (1), e13075. doi:10.1111/acel.13075
Ilatovskaya, D. V., Blass, G., Palygin, O., Levchenko, V., Pavlov, T. S., Grzybowski, M. N., et al. (2018). A NOX4/TRPC6 Pathway in Podocyte Calcium Regulation and Renal Damage in Diabetic Kidney Disease. Jasn 29 (7), 1917–1927. doi:10.1681/ASN.2018030280
Ishii, T., Uchida, K., Hata, S., Hatta, M., Kita, T., Miyake, Y., et al. (2018). TRPV2 Channel Inhibitors Attenuate Fibroblast Differentiation and Contraction Mediated by Keratinocyte-Derived TGF-Β1 in an In Vitro Wound Healing Model of Rats. J. Dermatol. Sci. 90 (3), 332–342. doi:10.1016/j.jdermsci.2018.03.003
Jeschke, M. G., Gauglitz, G. G., Song, J., Kulp, G. A., Finnerty, C. C., Cox, R. A., et al. (2009). Calcium and ER Stress Mediate Hepatic Apoptosis after Burn Injury. J. Cell Mol. Med. 13 (8B), 1857–1865. doi:10.1111/j.1582-4934.2009.00644.x10.1111/j.1582-4934.2008.00644.x
Jeschke, M. G., van Baar, M. E., Choudhry, M. A., Chung, K. K., Gibran, N. S., and Logsetty, S. (2020). Burn Injury. Nat. Rev. Dis. Primers 6 (1), 11. doi:10.1038/s41572-020-0145-5
Kang, S. M., Han, S., Oh, J.-H., Lee, Y. M., Park, C.-H., Shin, C.-Y., et al. (2017). A Synthetic Peptide Blocking TRPV1 Activation Inhibits UV-Induced Skin Responses. J. Dermatol. Sci. 88 (1), 126–133. doi:10.1016/j.jdermsci.2017.05.009
Kariminejad, A., Barzegar, M., Abdollahimajd, F., Pramanik, R., and McGrath, J. A. (2014). Olmsted Syndrome in an Iranian Boy with a Newde Novomutation inTRPV3. Clin. Exp. Dermatol. 39 (4), 492–495. doi:10.1111/ced.12318
Karvonen, S.-L., Korkiamäki, T., Ylä-Outinen, H., Nissinen, M., Teerikangas, H., Pummi, K., et al. (2000). Psoriasis and Altered Calcium Metabolism: Downregulated Capacitative Calcium Influx and Defective Calcium-Mediated Cell Signaling in Cultured Psoriatic Keratinocytes1. J. Invest. Dermatol. 114 (4), 693–700. doi:10.1046/j.1523-1747.2000.00926.x10.1046/j.1523-1747.2000.00926.x
Kida, N., Sokabe, T., Kashio, M., Haruna, K., Mizuno, Y., Suga, Y., et al. (2012). Importance of Transient Receptor Potential Vanilloid 4 (TRPV4) in Epidermal Barrier Function in Human Skin Keratinocytes. Pflugers Arch. - Eur. J. Physiol. 463 (5), 715–725. doi:10.1007/s00424-012-1081-3
Kozyreva, T. V., Khramova, G. M., Voronova, I. P., and Evtushenko, A. A. (2016). The Influence of Cooling and TRPM8 Ion Channel Activation on the Level of Pro-inflammatory Cytokines in Normotensive and Hypertensive Rats. J. Therm. Biol. 61, 119–124. doi:10.1016/j.jtherbio.2016.09.004
Kurohane, K., Sahara, Y., Kimura, A., Narukawa, M., Watanabe, T., Daimon, T., et al. (2013). Lack of Transient Receptor Potential Melastatin 8 Activation by Phthalate Esters that Enhance Contact Hypersensitivity in Mice. Toxicol. Lett. 217 (3), 192–196. doi:10.1016/j.toxlet.2012.12.025
Lai, W.-W., Hsiao, Y.-P., Chung, J.-G., Wei, Y.-H., Cheng, Y.-W., and Yang, J.-H. (2011). Synergistic Phototoxic Effects of Glycolic Acid in a Human Keratinocyte Cell Line (HaCaT). J. Dermatol. Sci. 64 (3), 191–198. doi:10.1016/j.jdermsci.2011.09.001
Lee, S. E., and Lee, S. H. (2018). Skin Barrier and Calcium. Ann. Dermatol. 30 (3), 265–275. doi:10.5021/ad.2018.30.3.265
Lee, Y. M., Kim, Y. K., Kim, K. H., Park, S. J., Kim, S. J., and Chung, J. H. (2009). A Novel Role for the TRPV1 Channel in UV-Induced Matrix Metalloproteinase (MMP)-1 Expression in HaCaT Cells. J. Cel. Physiol. 219 (3), 766–775. doi:10.1002/jcp.21729
Lee, Y. W., Won, C. H., Jung, K., Nam, H. J., Choi, G., Park, Y. H., et al. (2019). Efficacy and Safety of PAC ‐14028 Cream - a Novel, Topical, Nonsteroidal, Selective TRPV 1 Antagonist in Patients with Mild‐to‐moderate Atopic Dermatitis: a Phase II B Randomized Trial. Br. J. Dermatol. 180 (5), 1030–1038. doi:10.1111/bjd.17455
Lehen'Kyi, V., Beck, B., Polakowska, R., Charveron, M., Bordat, P., Skryma, R., et al. (2007). TRPV6 Is a Ca2+ Entry Channel Essential for Ca2+-Induced Differentiation of Human Keratinocytes. J. Biol. Chem. 282 (31), 22582–22591. doi:10.1074/jbc.M611398200
Leuner, K., Kraus, M., Woelfle, U., Beschmann, H., Harteneck, C., Boehncke, W.-H., et al. (2011). Reduced TRPC Channel Expression in Psoriatic Keratinocytes Is Associated with Impaired Differentiation and Enhanced Proliferation. PLoS One 6 (2), e14716. doi:10.1371/journal.pone.0014716
Lewis, R. S. (2007). The Molecular Choreography of a Store-Operated Calcium Channel. Nature 446 (7133), 284–287. doi:10.1038/nature05637
Li, H. (2017). TRP Channel Classification. Adv. Exp. Med. Biol. 976, 1–8. doi:10.1007/978-94-024-1088-4_1
Liang, Y., Chang, C., and Lu, Q. (2016). The Genetics and Epigenetics of Atopic Dermatitis-Filaggrin and Other Polymorphisms. Clinic Rev. Allerg Immunol. 51 (3), 315–328. doi:10.1007/s12016-015-8508-5
Liedtke, W., Choe, Y., Martí-Renom, M. A., Bell, A. M., Denis, C. S., AndrejŠali, A., et al. (2000). Vanilloid Receptor-Related Osmotically Activated Channel (VR-OAC), a Candidate Vertebrate Osmoreceptor. Cell 103 (3), 525–535. doi:10.1016/s0092-8674(00)00143-4
Lin, Z., Chen, Q., Lee, M., Cao, X., Zhang, J., Ma, D., et al. (2012). Exome Sequencing Reveals Mutations in TRPV3 as a Cause of Olmsted Syndrome. Am. J. Hum. Genet. 90 (3), 558–564. doi:10.1016/j.ajhg.2012.02.006
Mazzotta, C., Manetti, M., Rosa, I., Romano, E., Blagojevic, J., Bellando-Randone, S., et al. (2017). Proangiogenic Effects of Soluble α-Klotho on Systemic Sclerosis Dermal Microvascular Endothelial Cells. Arthritis Res. Ther. 19 (1), 27. doi:10.1186/s13075-017-1233-0
Mikami, A., Imoto, K., Tanabe, T., Niidome, T., Mori, Y., Takeshima, H., et al. (1989). Primary Structure and Functional Expression of the Cardiac Dihydropyridine-Sensitive Calcium Channel. Nature 340 (6230), 230–233. doi:10.1038/340230a0
Montell, C., and Rubin, G. M. (1989). Molecular Characterization of the Drosophila Trp Locus: a Putative Integral Membrane Protein Required for Phototransduction. Neuron 2, 1313–1323. doi:10.1016/0896-6273(89)90069-x
Moran, M. M. (2018). TRP Channels as Potential Drug Targets. Annu. Rev. Pharmacol. Toxicol. 58, 309–330. doi:10.1146/annurev-pharmtox-010617-052832
Motiani, R. K., Tanwar, J., Raja, D. A., Vashisht, A., Khanna, S., Sharma, S., et al. (2018). STIM 1 Activation of Adenylyl Cyclase 6 Connects Ca 2+ and cAMP Signaling during Melanogenesis. EMBO J. 37 (5). doi:10.15252/embj.201797597
Müller, M., Essin, K., Hill, K., Beschmann, H., Rubant, S., Schempp, C. M., et al. (2008). Specific TRPC6 Channel Activation, a Novel Approach to Stimulate Keratinocyte Differentiation. J. Biol. Chem. 283 (49), 33942–33954. doi:10.1074/jbc.M801844200
Nam, J. H., Jung, H. W., Chin, Y.-W., Yang, W.-M., Bae, H. S., and Kim, W. K. (2017). Spirodela Polyrhiza Extract Modulates the Activation of Atopic Dermatitis-Related Ion Channels, Orai1 and TRPV3, and Inhibits Mast Cell Degranulation. Pharm. Biol. 55 (1), 1324–1329. doi:10.1080/13880209.2017.1300819
Nattkemper, L. A., Tey, H. L., Valdes-Rodriguez, R., Lee, H., Mollanazar, N. K., Albornoz, C., et al. (2018). The Genetics of Chronic Itch: Gene Expression in the Skin of Patients with Atopic Dermatitis and Psoriasis with Severe Itch. J. Invest. Dermatol. 138 (6), 1311–1317. doi:10.1016/j.jid.2017.12.029
Nikolaeva-Koleva, M., Butron, L., González-Rodríguez, S., Devesa, I., Valente, P., Serafini, M., et al. (2021). A Capsaicinoid-Based Soft Drug, AG1529, for Attenuating TRPV1-Mediated Histaminergic and Inflammatory Sensory Neuron Excitability. Sci. Rep. 11 (1), 246. doi:10.1038/s41598-020-80725-z
Norões, M. M., Santos, L. G., Gavioli, E. C., de Paula Soares Rachetti, V., Otuki, M. F., de Almeida Cabrini, D., et al. (2019). Role of TRPA1 Receptors in Skin Inflammation Induced by Volatile Chemical Irritants in Mice. Eur. J. Pharmacol. 858, 172460. doi:10.1016/j.ejphar.2019.172460
Nowycky, M. C., Fox, A. P., and Tsien, R. W. (1985). Three Types of Neuronal Calcium Channel with Different Calcium Agonist Sensitivity. Nature 316 (6027), 440–443. doi:10.1038/316440a0
Numaga-Tomita, T., and Putney, J. W. (2013). Role of STIM1 and Orai1-Mediated Calcium Entry in Ca2+-Induced Epidermal Keratinocyte Differentiation. J. Cel Sci 126 (Pt 2), 605–612. doi:10.1242/jcs.115980
Oancea, E., Vriens, J., Brauchi, S., Jun, J., Splawski, I., and Clapham, D. E. (2009). TRPM1 Forms Ion Channels Associated with Melanin Content in Melanocytes. Sci. Signaling 2 (70), ra21. doi:10.1126/scisignal.2000146
Oda, K., Umemura, M., Nakakaji, R., Tanaka, R., Sato, I., Nagasako, A., et al. (2017). Transient Receptor Potential Cation 3 Channel Regulates Melanoma Proliferation and Migration. J. Physiol. Sci. 67 (4), 497–505. doi:10.1007/s12576-016-0480-1
Oh, M.-H., Oh, S. Y., Lu, J., Lou, H., Myers, A. C., Zhu, Z., et al. (2013). TRPA1-dependent Pruritus in IL-13-induced Chronic Atopic Dermatitis. J.I. 191 (11), 5371–5382. doi:10.4049/jimmunol.1300300
Okada, Y., Reinach, P. S., Shirai, K., Kitano, A., Kao, W. W.-Y., Flanders, K. C., et al. (2011). TRPV1 Involvement in Inflammatory Tissue Fibrosis in Mice. Am. J. Pathol. 178 (6), 2654–2664. doi:10.1016/j.ajpath.2011.02.043
Okada, Y., Shirai, K., Reinach, P. S., Kitano-Izutani, A., Miyajima, M., Flanders, K. C., et al. (2014). TRPA1 Is Required for TGF-β Signaling and its Loss Blocks Inflammatory Fibrosis in Mouse Corneal Stroma. Lab. Invest. 94 (9), 1030–1041. doi:10.1038/labinvest.2014.85
Ondrusova, K., Fatehi, M., Barr, A., Czarnecka, Z., Long, W., Suzuki, K., et al. (2017). Subcutaneous white Adipocytes Express a Light Sensitive Signaling Pathway Mediated via a Melanopsin/TRPC Channel axis. Sci. Rep. 7 (1), 16332. doi:10.1038/s41598-017-16689-4
Pani, B., and Singh, B. B. (2008). Darier's Disease: a Calcium-Signaling Perspective. Cell. Mol. Life Sci. 65 (2), 205–211. doi:10.1007/s00018-007-7397-z
Parekh, A. B., and Putney, J. W. (2005). Store-operated Calcium Channels. Physiol. Rev. 85 (2), 757–810. doi:10.1152/physrev.00057.2003
Park, C., Kim, H., Choi, Y., Chung, B., Woo, S.-Y., Song, D.-K., et al. (2017). TRPV3 Channel in Keratinocytes in Scars with Post-Burn Pruritus. Ijms 18 (11), 2425. doi:10.3390/ijms18112425
Patel, S., Srivastava, S., Singh, M. R., and Singh, D. (2019). Mechanistic Insight into Diabetic Wounds: Pathogenesis, Molecular Targets and Treatment Strategies to Pace Wound Healing. Biomed. Pharmacother. 112, 108615. doi:10.1016/j.biopha.2019.108615
Peier, A. M., Reeve, A. J., Andersson, D. A., Moqrich, A., Earley, T. J., Hergarden, A. C., et al. (2002). A Heat-Sensitive TRP Channel Expressed in Keratinocytes. Science 296 (5575), 2046–2049. doi:10.1126/science.1073140
Philippaert, K., and Vennekens, R. (2017). The Role of TRP Channels in the Pancreatic Beta-Cell, 229–250. doi:10.4324/9781315152837-12The Role of TRP Channels in the Pancreatic Beta-Cell
Prakriya, M., and Lewis, R. S. (2015). Store-Operated Calcium Channels. Physiol. Rev. 95 (4), 1383–1436. doi:10.1152/physrev.00020.2014
Putney, J. W. (2005). Capacitative Calcium Entry. J. Cel Biol 169 (3), 381–382. doi:10.1083/jcb.200503161
Quan, T., Xiang, Y., Liu, Y., Qin, Z., Yang, Y., Bou-Gharios, G., et al. (2021). Dermal Fibroblast CCN1 Expression in Mice Recapitulates Human Skin Dermal Aging. J. Invest. Dermatol. 141 (4S), 1007–1016. doi:10.1016/j.jid.2020.07.019
Radtke, C., Sinis, N., Sauter, M., Jahn, S., Kraushaar, U., Guenther, E., et al. (2011). TRPV Channel Expression in Human Skin and Possible Role in Thermally Induced Cell Death. J. Burn Care Res. 32 (1), 150–159. doi:10.1097/BCR.0b013e318203350c
Ramachandran, R., Hyun, E., Zhao, L., Lapointe, T. K., Chapman, K., Hirota, C. L., et al. (2013). TRPM8 Activation Attenuates Inflammatory Responses in Mouse Models of Colitis. Proc. Natl. Acad. Sci. 110 (18), 7476–7481. doi:10.1073/pnas.1217431110
Ramsey, I. S., Delling, M., and Clapham, D. E. (2006). An Introduction to TRP Channels. Annu. Rev. Physiol. 68, 619–647. doi:10.1146/annurev.physiol.68.040204.100431
Randall, A., and Tsien, R. (1995). Pharmacological Dissection of Multiple Types of Ca2+ Channel Currents in Rat Cerebellar Granule Neurons. J. Neurosci. 15 (4), 2995–3012. doi:10.1523/jneurosci.15-04-02995.1995
Ratushny, V., Gober, M. D., Hick, R., Ridky, T. W., and Seykora, J. T. (2012). From Keratinocyte to Cancer: the Pathogenesis and Modeling of Cutaneous Squamous Cell Carcinoma. J. Clin. Invest. 122 (2), 464–472. doi:10.1172/JCI57415
Rinnerthaler, M., Streubel, M. K., Bischof, J., and Richter, K. (2015). Skin Aging, Gene Expression and Calcium. Exp. Gerontol. 68, 59–65. doi:10.1016/j.exger.2014.09.015
Sakuntabhai, A., Ruiz-Perez, V., Carter, S., Jacobsen, N., Burge, S., Monk, S., et al. (1999). Mutations in ATP2A2, Encoding a Ca2+ Pump, Cause Darier Disease. Nat. Genet. 21 (3), 271–277. doi:10.1038/6784
Satheesh, N. J., Uehara, Y., Fedotova, J., Pohanka, M., Büsselberg, D., and Kruzliak, P. (2016). TRPV Currents and Their Role in the Nociception and Neuroplasticity. Neuropeptides 57, 1–8. doi:10.1016/j.npep.2016.01.003
Saul, S., Stanisz, H., Backes, C. S., Schwarz, E. C., and Hoth, M. (2014). How ORAI and TRP Channels Interfere with Each Other: Interaction Models and Examples from the Immune System and the Skin. Eur. J. Pharmacol. 739, 49–59. doi:10.1016/j.ejphar.2013.10.071
Schempp, C. M., Windeck, T., Hezel, S., and Simon, J. C. (2003). Topical Treatment of Atopic Dermatitis with St. John's Wort Cream - a Randomized, Placebo Controlled, Double Blind Half-Side Comparison. Phytomedicine 10 (Suppl. 4), 31–37. doi:10.1078/1433-187x-00306
Schmitt, T., Egu, D. T., Walter, E., Sigmund, A. M., Eichkorn, R., Yazdi, A., et al. (2021). Ca 2+ Signalling Is Critical for Autoantibody‐induced Blistering of Human Epidermis in Pemphigus. Br. J. Dermatol. 1. doi:10.1111/bjd.20091
Seo, S. H., Kim, S., Kim, S.-E., Chung, S., and Lee, S. E. (2020). Enhanced Thermal Sensitivity of TRPV3 in Keratinocytes Underlies Heat-Induced Pruritogen Release and Pruritus in Atopic Dermatitis. J. Invest. Dermatol. 140 (11), 2199–2209. doi:10.1016/j.jid.2020.02.028
Serysheva, I. I. (2014). Toward a High-Resolution Structure of IP3R Channel. Cell Calcium 56 (3), 125–132. doi:10.1016/j.ceca.2014.08.002
Simões, M. C. F., Sousa, J. J. S., and Pais, A. A. C. C. (2015). Skin Cancer and New Treatment Perspectives: a Review. Cancer Lett. 357 (1), 8–42. doi:10.1016/j.canlet.2014.11.001
Smith, G. D., Gunthorpe, M. J., Kelsell, R. E., Hayes, P. D., Reilly, P., Facer, P., et al. (2002). TRPV3 Is a Temperature-Sensitive Vanilloid Receptor-like Protein. Nature 418 (6894), 186–190. doi:10.1038/nature00894
Sokabe, T., Fukumi-Tominaga, T., Yonemura, S., Mizuno, A., and Tominaga, M. (2010). The TRPV4 Channel Contributes to Intercellular junction Formation in Keratinocytes. J. Biol. Chem. 285 (24), 18749–18758. doi:10.1074/jbc.M110.103606
Stander, S., Moormann, C., Schumacher, M., Buddenkotte, J., Artuc, M., Shpacovitch, V., et al. (2004). Expression of Vanilloid Receptor Subtype 1 in Cutaneous Sensory Nerve Fibers, Mast Cells, and Epithelial Cells of Appendage Structures. Exp. Dermatol. 13 (3), 129–139. doi:10.1111/j.0906-6705.2004.0178.x
Ständer, S., Yosipovitch, G., Legat, F. J., Lacour, J.-P., Paul, C., Narbutt, J., et al. (2020). Trial of Nemolizumab in Moderate-To-Severe Prurigo Nodularis. N. Engl. J. Med. 382 (8), 706–716. doi:10.1056/NEJMoa1908316
Stanisz, H., Saul, S., Müller, C. S. L., Kappl, R., Niemeyer, B. A., Vogt, T., et al. (2014). Inverse Regulation of Melanoma Growth and Migration by Orai1/STIM2-dependent Calcium Entry. Pigment Cel Melanoma Res. 27 (3), 442–453. doi:10.1111/pcmr.12222
Steinckwich, N., Myers, P., Janardhan, K. S., Flagler, N. D., King, D., Petranka, J. G., et al. (2015). Role of the Store‐operated Calcium Entry Protein, STIM1, in Neutrophil Chemotaxis and Infiltration into a Murine Model of Psoriasis‐inflamed Skin. FASEB j. 29 (7), 3003–3013. doi:10.1096/fj.14-265215
Strübing, C., Krapivinsky, G., Krapivinsky, L., and Clapham, D. E. (2001). TRPC1 and TRPC5 Form a Novel Cation Channel in Mammalian Brain. Neuron 29 (3), 645–655. doi:10.1016/s0896-6273(01)00240-9
Sun, X.-D., You, Y., Zhang, L., Zheng, S., Hong, Y., Li, J., et al. (2012). The Possible Role of TRPC6 in Atopic Dermatitis. Med. Hypotheses 78 (1), 42–44. doi:10.1016/j.mehy.2011.09.038
Szöllősi, A. G., Vasas, N., Angyal, Á., Kistamás, K., Nánási, P. P., Mihály, J., et al. (2018). Activation of TRPV3 Regulates Inflammatory Actions of Human Epidermal Keratinocytes. J. Invest. Dermatol. 138 (2), 365–374. doi:10.1016/j.jid.2017.07.852
Takada, H., Yonekawa, J., Matsumoto, M., Furuya, K., and Sokabe, M. (2017). Hyperforin/HP-β-Cyclodextrin Enhances Mechanosensitive Ca2+ Signaling in HaCaT Keratinocytes and in Atopic Skin Ex Vivo Which Accelerates Wound Healing. Biomed. Res. Int. 2017, 1–9. doi:10.1155/2017/8701801
Talavera, K., Startek, J. B., Alvarez-Collazo, J., Boonen, B., Alpizar, Y. A., Sanchez, A., et al. (2020). Mammalian Transient Receptor Potential TRPA1 Channels: From Structure to Disease. Physiol. Rev. 100 (2), 725–803. doi:10.1152/physrev.00005.2019
Tang, L., Gamal El-Din, T. M., Swanson, T. M., Pryde, D. C., Scheuer, T., Zheng, N., et al. (2016). Structural Basis for Inhibition of a Voltage-Gated Ca2+ Channel by Ca2+ Antagonist Drugs. Nature 537 (7618), 117–121. doi:10.1038/nature19102
Tang, S.-C., Tang, L.-C., Liu, C.-H., Liao, P.-Y., Lai, J.-C., and Yang, J.-H. (2019). Glycolic Acid Attenuates UVB-Induced Aquaporin-3, Matrix Metalloproteinase-9 Expression, and Collagen Degradation in Keratinocytes and Mouse Skin. Biochem. J. 476 (10), 1387–1400. doi:10.1042/BCJ20180974
Thrower, E. C., Hagar, R. E., and Ehrlich, B. E. (2001). Regulation of Ins(1,4,5)P3 Receptor Isoforms by Endogenous Modulators. Trends Pharmacol. Sci. 22, 580–586. doi:10.1016/s0165-6147(00)01809-5
Toichi, E., Tachibana, T., and Furukawa, F. (2000). Rapid Improvement of Psoriasis Vulgaris during Drug-Induced Agranulocytosis. J. Am. Acad. Dermatol. 43 (2 Pt 2), 391–395. doi:10.1067/mjd.2000.103264
Tóth, B. I., Dobrosi, N., Dajnoki, A., Czifra, G., Oláh, A., Szöllősi, A. G., et al. (2011). Endocannabinoids Modulate Human Epidermal Keratinocyte Proliferation and Survival via the Sequential Engagement of Cannabinoid Receptor-1 and Transient Receptor Potential Vanilloid-1. J. Invest. Dermatol. 131 (5), 1095–1104. doi:10.1038/jid.2010.421
Tóth, B. I., Géczy, T., Griger, Z., Dózsa, A., Seltmann, H., Kovács, L., et al. (2009). Transient Receptor Potential Vanilloid-1 Signaling as a Regulator of Human Sebocyte Biology. J. Invest. Dermatol. 129 (2), 329–339. doi:10.1038/jid.2008.258
Tóth, B. I., Oláh, A., Szöllősi, A. G., and Bíró, T. (2014). TRP Channels in the Skin. Br. J. Pharmacol. 171 (10), 2568–2581. doi:10.1111/bph.12569
Tsianakas, A., Zeidler, C., and Ständer, S. (2016). Prurigo Nodularis Management. Curr. Probl. Dermatol. 50, 94–101. doi:10.1159/000446049
Tsien, R. W., Lipscombe, D., Madison, D. V., Bley, K. R., and Fox, A. P. (1988). Multiple Types of Neuronal Calcium Channels and Their Selective Modulation. Trends Neurosciences 11 (10), 431–438. doi:10.1016/0166-2236(88)90194-4
Tu, C.-L., Chang, W., and Bikle, D. D. (2005). Phospholipase Cγ1 Is Required for Activation of Store-Operated Channels in Human Keratinocytes. J. Invest. Dermatol. 124 (1), 187–197. doi:10.1111/j.0022-202X.2004.23544.x10.1111/j.0022-202x.2004.23544.x
Ullrich, N. D., Voets, T., Prenen, J., Vennekens, R., Talavera, K., Droogmans, G., et al. (2005). Comparison of Functional Properties of the Ca2+-Activated Cation Channels TRPM4 and TRPM5 from Mice. Cell Calcium 37 (3), 267–278. doi:10.1016/j.ceca.2004.11.001
Um, J.-y., Kang, S. Y., Kim, H. J., Chung, B. Y., Park, C. W., and Kim, H. O. (2020). Transient Receptor Potential Vanilloid-3 (TRPV3) Channel Induces Dermal Fibrosis via the TRPV3/TSLP/Smad2/3 Pathways in Dermal Fibroblasts. J. Dermatol. Sci. 97 (2), 117–124. doi:10.1016/j.jdermsci.2019.12.011
Umemura, M., Baljinnyam, E., Feske, S., De Lorenzo, M. S., Xie, L.-H., Feng, X., et al. (2014). Store-operated Ca2+ Entry (SOCE) Regulates Melanoma Proliferation and Cell Migration. PLoS One 9 (2), e89292. doi:10.1371/journal.pone.0089292
Vaeth, M., Yang, J., Yamashita, M., Zee, I., Eckstein, M., Knosp, C., et al. (2017). ORAI2 Modulates Store-Operated Calcium Entry and T Cell-Mediated Immunity. Nat. Commun. 8, 14714. doi:10.1038/ncomms14714
Vandenberghe, M., Raphael, M., Lehen'Kyi, V., Gordienko, D., Hastie, R., Oddos, T., et al. (2013). ORAI1 Calcium Channel Orchestrates Skin Homeostasis. Proc. Natl. Acad. Sci. 110 (50), E4839–E4848. doi:10.1073/pnas.1310394110
Vandewauw, I., De Clercq, K., Mulier, M., Held, K., Pinto, S., Van Ranst, N., et al. (2018). A TRP Channel Trio Mediates Acute Noxious Heat Sensing. Nature 555 (7698), 662–666. doi:10.1038/nature26137
Vay, L., Gu, C., and McNaughton, P. A. (2012). The Thermo-TRP Ion Channel Family: Properties and Therapeutic Implications. Br. J. Pharmacol. 165 (4), 787–801. doi:10.1111/j.1476-5381.2011.01601.x10.1111/j.1476-5381.2011.01601.x
Vriens, J., Nilius, B., and Vennekens, R. (2008). Herbal Compounds and Toxins Modulating TRP Channels. Curr. Neuropharmacol 6 (1), 79–96. doi:10.2174/157015908783769644
Vriens, J., Appendino, G., and Nilius, B. (2009). Pharmacology of Vanilloid Transient Receptor Potential Cation Channels. Mol. Pharmacol. 75 (6), 1262–1279. doi:10.1124/mol.109.055624
Wang, H., Cheng, X., Tian, J., Xiao, Y., Tian, T., Xu, F., et al. (2020). TRPC Channels: Structure, Function, Regulation and Recent Advances in Small Molecular Probes. Pharmacol. Ther. 209, 107497. doi:10.1016/j.pharmthera.2020.107497
Wang, W., Wang, H., Zhao, Z., Huang, X., Xiong, H., and Mei, Z. (2020). Thymol Activates TRPM8-Mediated Ca2+ Influx for its Antipruritic Effects and Alleviates Inflammatory Response in Imiquimod-Induced Mice. Toxicol. Appl. Pharmacol. 407, 115247. doi:10.1016/j.taap.2020.115247
Watanabe, H., Vriens, J., Suh, S. H., Benham, C. D., Droogmans, G., and Nilius, B. (2002). Heat-evoked Activation of TRPV4 Channels in a HEK293 Cell Expression System and in Native Mouse Aorta Endothelial Cells. J. Biol. Chem. 277 (49), 47044–47051. doi:10.1074/jbc.M208277200
Wes, P. D., Chevesich, J., Jeromin, A., Rosenberg, C., Stetten, G., and Montell, C. (1995). TRPC1, a Human Homolog of a Drosophila Store-Operated Channel. Proc. Natl. Acad. Sci. 92 (21), 9652–9656. doi:10.1073/pnas.92.21.9652
Wieckiewicz, W., Bieniek, A., Wieckiewicz, M., and Sroczyk, L. (2013). Interdisciplinary Treatment of BCC Located on the Nose - Review of Literature. Adv. Clin. Exp. Med. 22 (2), 289–293.
Woelfle, U., Laszczyk, M. N., Kraus, M., Leuner, K., Kersten, A., Simon-Haarhaus, B., et al. (2010). Triterpenes Promote Keratinocyte Differentiation In Vitro, Ex Vivo and In Vivo: a Role for the Transient Receptor Potential Canonical (Subtype) 6. J. Invest. Dermatol. 130 (1), 113–123. doi:10.1038/jid.2009.248
Wolf, R., and Wolf, D. (2012). Abnormal Epidermal Barrier in the Pathogenesis of Atopic Dermatitis. Clin. Dermatol. 30 (3), 329–334. doi:10.1016/j.clindermatol.2011.08.023
Woo, J. H., Nam, D. Y., Kim, H. J., Hong, P. T. L., Kim, W. K., and Nam, J. H. (2021). Nootkatol Prevents Ultraviolet Radiation-Induced Photoaging via ORAI1 and TRPV1 Inhibition in Melanocytes and Keratinocytes. Korean J. Physiol. Pharmacol. 25 (1), 87–94. doi:10.4196/kjpp.2021.25.1.87
Wu, J., Yan, N., and Yan, Z. (2017). Structure-Function Relationship of the Voltage-Gated Calcium Channel Cav1.1 Complex. Adv. Exp. Med. Biol. 981, 23–39. doi:10.1007/978-3-319-55858-5_2
Yoshioka, T., Imura, K., Asakawa, M., Suzuki, M., Oshima, I., Hirasawa, T., et al. (2009). Impact of the Gly573Ser Substitution in TRPV3 on the Development of Allergic and Pruritic Dermatitis in Mice. J. Invest. Dermatol. 129 (3), 714–722. doi:10.1038/jid.2008.245
Yuan, J. P., Zeng, W., Huang, G. N., Worley, P. F., and Muallem, S. (2007). STIM1 Heteromultimerizes TRPC Channels to Determine Their Function as Store-Operated Channels. Nat. Cel Biol 9 (6), 636–645. doi:10.1038/ncb1590
Yun, J.-W., Seo, J. A., Jang, W.-H., Koh, H. J., Bae, I.-H., Park, Y.-H., et al. (2011). Antipruritic Effects of TRPV1 Antagonist in Murine Atopic Dermatitis and Itching Models. J. Invest. Dermatol. 131 (7), 1576–1579. doi:10.1038/jid.2011.87
Yun, J.-W., Seo, J. A., Jeong, Y. S., Bae, I.-H., Jang, W.-H., Lee, J., et al. (2010). TRPV1 Antagonist Can Suppress the Atopic Dermatitis-like Symptoms by Accelerating Skin Barrier Recovery. J. Dermatol. Sci. 62 (1), 8–15. doi:10.1016/j.jdermsci.2010.10.014
Zamponi, G. W., Striessnig, J., Koschak, A., and Dolphin, A. C. (2015). The Physiology, Pathology, and Pharmacology of Voltage-Gated Calcium Channels and Their Future Therapeutic Potential. Pharmacol. Rev. 67 (4), 821–870. doi:10.1124/pr.114.009654
Keywords: calcium channels, dermatologic disease, transient receptor potential, therapeutic target, second messenger
Citation: Wang M, Sun Y, Li L, Wu P, DKW O and Shi H (2021) Calcium Channels: Noteworthy Regulators and Therapeutic Targets in Dermatological Diseases. Front. Pharmacol. 12:702264. doi: 10.3389/fphar.2021.702264
Received: 30 April 2021; Accepted: 02 August 2021;
Published: 19 August 2021.
Edited by:
Klaus Groschner, Medical University of Graz, AustriaReviewed by:
Christine Peinelt, University of Bern, SwitzerlandMaud Frieden, Université de Genève, Switzerland
Indu Ambudkar, National Institute of Dental and Craniofacial Research (NIDCR), United States
Copyright © 2021 Wang, Sun, Li, Wu, DKW and Shi. This is an open-access article distributed under the terms of the Creative Commons Attribution License (CC BY). The use, distribution or reproduction in other forums is permitted, provided the original author(s) and the copyright owner(s) are credited and that the original publication in this journal is cited, in accordance with accepted academic practice. No use, distribution or reproduction is permitted which does not comply with these terms.
*Correspondence: Hui Shi, NzM1NTk0OTMyQHFxLmNvbQ==
†These authors have contributed equally to this work.