- 1Department of Pharmacology, Yong Loo Lin School of Medicine, National University of Singapore, Singapore, Singapore
- 2Department of Endocrinology, The First Affiliated Hospital of Shenzhen University (Shenzhen Second People’s Hospital), Shenzhen, China
- 3Department of Pharmacology, School of Medicine, Southern University of Science and Technology, Shenzhen, China
- 4National University of Singapore (Suzhou) Research Institute, Suzhou, China
Cardiovascular diseases are the most common complications of diabetes, and diabetic cardiomyopathy is a major cause of people death in diabetes. Molecular, transcriptional, animal, and clinical studies have discovered numerous therapeutic targets or drugs for diabetic cardiomyopathy. Within this, hydrogen sulfide (H2S), an endogenous gasotransmitter alongside with nitric oxide (NO) and carbon monoxide (CO), is found to play a critical role in diabetic cardiomyopathy. Recently, the protective roles of H2S in diabetic cardiomyopathy have attracted enormous attention. In addition, H2S donors confer favorable effects in myocardial infarction, ischaemia-reperfusion injury, and heart failure under diabetic conditions. Further studies have disclosed that multiplex molecular mechanisms are responsible for the protective effects of H2S against diabetes-elicited cardiac injury, such as anti-oxidative, anti-apoptotic, anti-inflammatory, and anti-necrotic properties. In this review, we will summarize the current findings on H2S biology and pharmacology, especially focusing on the novel mechanisms of H2S-based protection against diabetic cardiomyopathy. Also, the potential roles of H2S in diabetes-aggravated ischaemia-reperfusion injury are discussed.
Introduction
The International Diabetes Federation has estimated that global diabetic prevalence might rise to 10.2% by 2030 and 10.9% by 2045, respectively (Saeedi et al., 2019). On a global scale, increasing epidemic of diabetes exerts serious economic and social implications in many countries, and 77% of diabetic individuals live in low and middle income countries (Standl et al., 2019). Apart from overt diabetic patients, 352.1 million people are estimated to be at risk of diabetes globally (also known as pre-diabetes) (Standl et al., 2019). Diabetic pandemic seems to be one of global public health problems that need to be solved urgently, especially considering the increasing number of deaths caused by diabetic complications (Ayensa-Vazquez et al., 2020; Magliano et al., 2020). Cardiovascular disorders are the most complications of diabetes with a serious impact on healthcare resources, and they are also the main causes of death in diabetic patients (International Hypoglycaemia Study Group, 2019). Diabetes-induced cardiovascular diseases encompass peripheral artery disease, cerebrovascular disease, coronary artery disease, heart failure, and cardiomyopathy (International Hypoglycaemia Study Group, 2019). A host of clinical studies have demonstrated that cardiovascular disease risks are 3-to-4-fold higher in diabetic patients (Xie et al., 2017). Diabetic cardiomyopathy, a unique type of cardiac damage, exhibits abnormal myocardial structures independent of hypertension, coronary artery disease, valvular heart disease or other known precipitating factors for heart failure (Rubler et al., 1972; Adeghate and Singh, 2014; Zamora and Villena, 2019). A population-based study has reported that diabetic patients are at a higher risk to develop diastolic or systolic dysfunctions when compared to normal individuals (Devereux et al., 2000; Bertoni et al., 2006). Diabetic cardiomyopathy has gained increased attention due to its high morbidity and poor prognosis (Oktay et al., 2000; Pikkemaat et al., 2017). Studies have confirmed that the pathogenesis of diabetic cardiomyopathy is multifactorial and mainly include, but not limited to, oxidative/nitrosative stress, impaired calcium homeostasis, mitochondrial dysfunction, and accumulation of advanced glycation end products (AGEs), as well as increased inflammation (Borghetti et al., 2018; Al Hroob et al., 2019). Although several anti-hyperglycemic drugs are commonly used for the treatment of diabetes, they can hardly improve diabetic cardiomyopathy (Borghetti et al., 2018). As a result, there is an urgent unmet medical need to discovery novel and effective therapies directly against diabetic cardiomyopathy.
Historically, hydrogen sulfide (H2S) was viewed as a toxic gas with foul smelling, but more and more studies have identified H2S as a member of the gasotransmitter family, along with nitric oxide (NO) and carbon monoxide (CO) (Beck and Pfeilschifter, 2020). In the cardiovascular system, H2S is endogenously generated by three endogenous enzymes, including cystathionine β-synthase (CBS), cystathionine γ-lyase (CSE), and 3-mercaptopyruvate sulfur transferase (3-MST)/cysteine aminotransferase (CAT) (Chen et al., 2020). Besides, H2S is also generated from d-cysteine by d-amino acid oxidase (DAO) (Wen et al., 2018). In the last several decades, the biological and pharmacological effects of H2S on various systems are gradually revealed after the measurement of brain H2S content in 1989 (Goodwin et al., 1989; Wang, 2002; Wang, 2012). Subsequently, H2S is emerging as an essential regulator in a diversity of physiological functions in the biological systems (Xie et al., 2016). Similar to NO and CO, H2S is critically involved in cardiovascular regulation (Testai et al., 2020). As the third gaseous signaling molecule, H2S is brought into the spotlight of cardiovascular field as H2S exhibits multiple cardiovascular protective effects, including vascular relaxation, pro-angiogenesis, anti-hypertension, anti-atherosclerosis, attenuation of oxidative stress and inflammation, as well as amelioration of myocardial ischemia-reperfusion injury (Gorini et al., 2020; Teoh et al., 2020). Coincidentally, accumulating evidence has suggested that H2S could modulate numerous molecular cascade events to ameliorate diabetes-induced cardiac damage (Kar et al., 2019a). Thereafter, we will highlight recent advances in the current understanding of the ability of H2S to relieve diabetic cardiomyopathy and diabetes-deteriorated ischaemia-reperfusion injury. Interactions of H2S with NO in diabetic cardiomyopathy are also described. Eventually, the possible challenges and directions of H2S-based pharmacological therapy in diabetic cardiomyopathy are overviewed and proposed.
Pathophysiology of Diabetic Cardiomyopathy
In 1954, Lundbaek reported a distinct clinical entity with cardiac dysfunction in type 2 diabetic individuals (termed as diabetic cardiomyopathy), independent of hypertension and coronary artery disease (Lundbaek, 1954). Rubler et al. (1972) had observed glomerulosclerosis and heart failure in diabetic individuals, although these diabetic patients had no hypertension, coronary artery disease, valvular or congenital heart disease. A large population study found that the risk of heart failure hospitalization in diabetic patients was still higher even though all cardiovascular risk factors were well controlled (Rawshani et al., 2018). With rapid increases in diabetic incidence and prevalence, diabetic cardiomyopathy, an important cardiovascular complication of diabetes, is becoming one of the main causes of disability and death in diabetic individuals (Zhang et al., 2021). Of all diabetic complications, diabetic cardiomyopathy accounts for nearly 80% of death in diabetic individuals (Wang and Hill, 2015).
Pathologically, diabetic cardiomyopathy is reflected by cardiac hypertrophy, myocardial apoptosis and fibrosis, and cardiac dysfunction (Ernande et al., 2011; Zhang and Sun, 2020). Numerous molecular mechanisms have been suggested to be involved in the development and progression of diabetic cardiomyopathy, such as oxidative stress, nitrosative/nitrative stress, inflammatory response, mitochondrial dysfunction, endoplasmic reticulum stress, impaired autophagy, cardiomyocyte apoptosis and death, and diabetic microangiopathy (Bugger and Abel, 2014; Varga et al., 2015; Kaludercic and Di Lisa, 2020). Additionally, experimental studies have shown that cardiac glycolipid toxicity, impaired insulin signaling, cardiac energetic impairment, activation of the renin–angiotensin system (RAS) and increased formation of angiotensin II (Ang II), cardiac autonomic neuropathy, reduced NO bioavailability, elevations in AGEs, and dysregulated cardiomyocyte calcium handling, play central roles in diabetic cardiomyopathy pathologies (Figure 1) (Pechánová et al., 2015; Jia et al., 2018a; Jia et al., 2018b; Dhalla and Shah, 2020; Komici et al., 2019; Zamora and Villena, 2019). Although our current understanding of the pathogenesis of diabetic cardiomyopathy is increasing, the etiology of diabetic cardiomyopathy is multifactorial and extremely complicated. There are still gaps in our current knowledge of the precise mechanisms involved in diabetic cardiomyopathy. As a result, more studies are required to deepen the comprehensive mechanisms that underlie the initiation and progression of diabetes-induced cardiac dysfunction, thereby leading to the development of newly effective targets or approaches to reduce the risk of diabetic patients developing life-changing complications, such as heart failure.
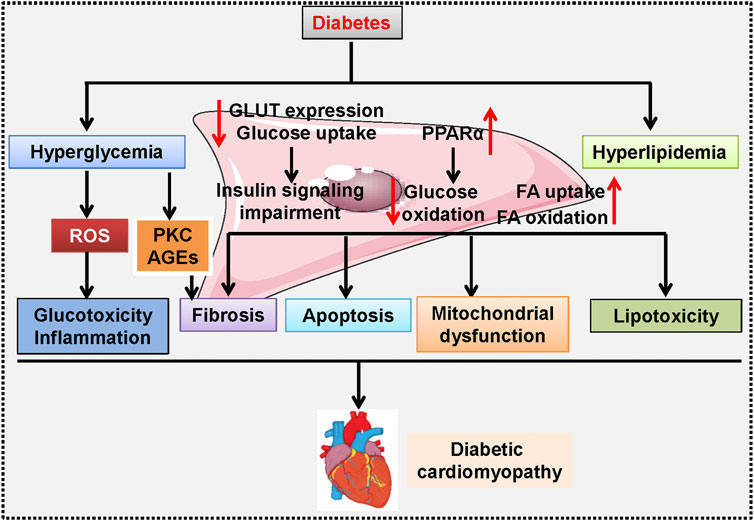
FIGURE 1. A proposed schematic diagram showing the signaling pathways involved in diabetic cardiomyopathy-related cardiac dysfunction. Hyperglycemia induces ROS overproduction and causes glucotoxicity and inflammation in the heart, and activation of PKC promotes AGEs formation, a critical event involved in diabetes-induced cardiac fibrosis. In cardiomyocytes, high glucose inhibits GLUT expression and glucose uptake, but upregulates PPARα to reduce glucose oxidation, thus resulting in insulin signaling impairment. Moreover, hyperlipidemia stimulates FA uptake and oxidation, and renders cardiomyocytes more sensitive to lipotoxicity. All these above changes synergistically contribute to fibrosis, apoptosis, mitochondrial dysfunction, and hypertrophy during the development and progression of diabetic cardiomyopathy. AGE: advanced glycation end products; FA: fatty acids; GLUT: Glucose transporters; PKC: Protein kinase C; PPARα: Peroxisome proliferator activated receptor α; ROS: reactive oxygen species.
Chemistry and Biochemistry of H2S
Previously, H2S is taken as a poisonous and occasionally lethal gas, and it might represent an industrial safety hazard since this colorless gas could be formed from the decomposition of various organic materials (Perna et al., 2011). Because of a special characteristic of rotten smell, H2S is believed to be a toxic byproduct of microbial metabolism in the atmosphere (Pan et al., 2017). H2S could be detected by the human nose at a level of 0.1 ppm, a dose of 400-fold lower than its toxic concentrations (Wang, 2002), while long-term exposure can render desensitization of the olfactory nerve to H2S (Li et al., 2009). H2S is readily dissolved in water due to its weak acid properties (pKa at 37°C, 6.76), and it will split to generate two dissociation states; the hydrosulphide anion (HS−, pKa 7.04), and sulphide anion (S2−, pKa 11.96) (Li et al., 2009). As a consequence, approximately 18.5% of the total sulphide exists as the undissociated acid and 81.5% as the HS− and S2− at physiological pH of 7.4 in aqueous solution (Dombkowski et al., 2004), which are in dynamic equilibrium in the following sequential reactions: H2S⇌HS− +H+⇌S2− + 2H+. Actually, the three equilibria in this formula represent the real dynamics of H2S in solution. It can be easily predicted that this equilibria will continuously shift to the left in an open system according to Le Chatelier’s Principle, thereby causing H2S to escape from the solution (Li and Lancaster, 2013). It has been shown that the half of H2S could be lost from solutions within 5 minutes in cell culture wells, 3 minutes in a bubbled tissue bath and an even shorter time in the Langendorff heart apparatus (DeLeon et al., 2012). Under aerobic conditions, the half-life time of H2S is 2.0, 2.8, and 10.0 min in murine hepatic, renal, and brain homogenates, respectively (Table 1) (Vitvitsky et al., 2012). One should also be aware that the actual concentration of H2S might vary in experimental systems containing headspace, and this may also depict to some extent the remarkable variations in H2S concentrations in cells, tissues or plasma (Furne et al., 2008; Whitfield et al., 2008; Shen et al., 2011; Kolluru et al., 2013). Whist it remains elusive whether the biological functions of H2S are mediated by H2S itself or its derived species, including S2− or HS−, it is being accepted that HS− is a potential nucleophile that could react with different electrophilic cellular targets, such as nitrogen species (RSONS) (Hartle and Pluth, 2016). H2S is a well-known reducing agent that exhibits various chemical properties, such as its redox activity, acidity, and high nucleophilicity, allowing for reactions with multiple cellular targets (Hartle and Pluth, 2016). Although it is quite conceivable that the biochemical effects of H2S may be dependent on its chemical capacities (especially the reductive and nucleophilic properties), disentangling this chemistry seems to be more complicated (Li and Lancaster, 2013; Nagy et al., 2014).
H2S is biologically active since its highly lipophilic characteristics allow it freely to penetrate into all types of the cell membranes without facilitation of membrane channels (Mathai et al., 2009). A famous pioneer in H2S research, Hideo Kimura, had demonstrated that H2S facilitated long-term potentiation in hippocampal tissues at the physiological concentration, pointing this gasotransmitter as an important neuromodulator (Abe and Kimura, 1996). Later, H2S is found to regulate both physiological and pathophysiological processes through a wide spectrum of signaling molecules (Kimura, 2020), such as reacting with superoxide anions (Chang et al., 2008), hypochlorite (Whiteman et al., 2005), hydrogen peroxide (Geng et al., 2004), peroxynitrite (Whiteman et al., 2004), metals (Li and Lancaster, 2013), thiol derivatives (Li and Lancaster, 2013), and NO (Ali et al., 2006; Whiteman et al., 2006) (Table 2). It is highly probable that the list of biomolecules affected by H2S will grow rapidly in the near future.
Since the discovery of H2S production in mammalian cells, many scientists have been working on the biological functions of H2S in this emerging field. The critical roles of H2S at its physiologically relevant concentrations on cardiovascular homeostasis have been well documented (Pan et al., 2017). Endogenous H2S levels are regulated preciously by its generation and elimination in health (Liu et al., 2012). It is important to determine the concentrations of H2S in cells, tissues, and blood samples before its prosperity in the regulation of physiological/pathophysiological functions. To date, several analytical methods are achieved to quantify H2S concentrations in organ tissues and blood, such as fluorescent probes (Zhang et al., 2020b), colorimetry (Sugahara et al., 2016), spectrophotometric analysis (Antoniou et al., 2018), headspace gas determination (Fonseca et al., 2013), polarographic sensor (Doeller et al., 2005), and liquid chromatography-mass spectrometry (Tan et al., 2017). However, different analysis methods have obtained inconsistent range of H2S concentrations (Ibrahim et al., 2021). According to the current literatures, the physiological concentrations of H2S in human/animal blood or tissues range from 15 nM to 300 μM in vivo (Huang et al., 2015; Hackfort and Mishra, 2016; Pan et al., 2017). Likewise, the physiological concentrations of H2S in plasma, serum, and cardiac tissues are considerably inconsistent (Table 3). The low concentration, short half-life time with fast catabolism, and high reactivity of H2S might be challenging for the determination of endogenous H2S in vivo (Ibrahim et al., 2021). Additionally, the wide range of H2S concentrations might result from variable detection methods as the different analysis technologies have distinct disadvantages, such as low sensitivity and specificity, complex preparation processes, and time-consuming procedures (Liu et al., 2012; Wu and Hu, 2018). The striking inconsistency in H2S levels under physiological conditions may lead to uncertainty for the exact mechanistic roles of H2S in physiological processes. Thence, it is essential to improve detection specificity and lower threshold limitations of these techniques, which may be helpful to obtain the actual physiological compartmentalization of H2S in cells, blood, and tissue.
Enzymatic Production and Metabolism of H2S
Endogenous H2S in mammalian systems is primarily produced by enzymatic or non-enzymatic pathways (Yu et al., 2014). The enzymatic pathways that generate H2S are mediated by CBS, CSE, and the coupling of 3-MST with CAT. The enzymatic pathways of H2S formation are illustrated in Figure 2. The intracellular distributions of H2S-generating enzymes are different in which CBS and CSE are strictly localized in the cytoplasm, whereas 3-MST and CAT are expressed in both the mitochondrial and the cytosol (but much higher in mitochondria) (Pan et al., 2017). Regarding tissue specificity localization, CSE is abundantly expressed in the cardiovascular system, whereas CBS expression predominates in the brain, liver, and kidneys under normal physiological conditions (Pan et al., 2017; Sun et al., 2019b). 3-MST and CAT are responsible for H2S formation in the brain and vascular endothelium (Shibuya et al., 2009; Chen et al., 2015). In spite of these observations, the relative contribution of each of these three enzymes to the tissue or circulating H2S levels remains elusive (Donnarumma et al., 2017). In addition to enzymatic pathways, H2S is also produced from inorganic polysulfides, organic polysulfides, and elemental sulfur, which are enriched in garlic (Benavides et al., 2007). Therefore, the healthy benefits of garlic may be associated with H2S production (Rose et al., 2018; Rodrigues and Percival, 2019). Actually, garlic extracts are effectively slowing the development and progression of atherosclerosis through the generation of H2S from S-allylcysteine and S-allylmercaptocysteine (Benavides et al., 2007; Budoff et al., 2009).
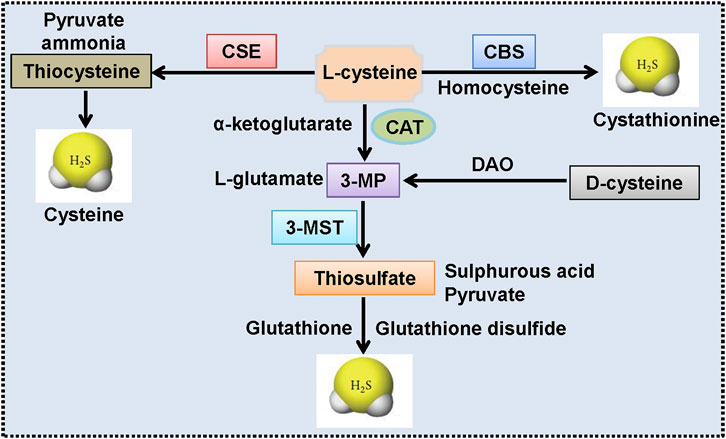
FIGURE 2. Endogenous synthesis pathways of H2S. CSE produces H2S by using l-cysteine as the main substrate, accompanied by the generation of ammonia, pyruvate, and thiocysteine. The latter would be catalyzed into H2S and cysteine. CBS-mediated H2S production is dependent on the transformation of homocysteine and l-cysteine to H2S and cystathionine. CAT catalyzes the conversion of l-cysteine and α-ketoglutarate to 3-MP and l-glutamate, and 3-MP is then catalyzed to sulfurous acid, pyruvate and thiosulfate by 3-MST. Thiosulfate is then reduced to H2S and glutathione disulfide by using reduced glutathione. Moreover, d-cysteine could be converted into 3-MP by DAO, and this reaction is also responsible for the formation of H2S in the mitochondria. 3-MP: 3-mercaptopyruvate; 3-MST, 3-mercaptopyruvate sulfurtransferase; CAT, cysteineamino transferase; CBS, cystathionine β-synthase; CSE, cystathionine γ-lyase; DAO, d-amino acid oxidase; H2S: hydrogen sulfide.
The balance of synthesis and metabolism is essential for maintaining an appropriate concentration of H2S under physiological conditions. Endogenous H2S could be inactivated by several routes (Figure 3). First of all, H2S can be converted into thiosulfate through mitochondrial oxidative modification, thiosulfate is further transformed into sulfite and sulfate (Kimura, 2012). Cytosolic methylation is the second pathway to convert H2S to dimethylsulfide through thiol S-methyltransferase (Wang et al., 2020). Also, binding of hemoglobin to H2S contributes to the generation of sulfhemoglobin (Olson et al., 2014). The excessive H2S could be scavenged by metallo- or disulfide-containing molecules or glutathione disulfide, and could also be released from the lungs (Mani et al., 2014; Donnarumma et al., 2017; Rose et al., 2017; Cao et al., 2019). Although these biosynthesis and degradation pathways of H2S have only recently been identified, these findings will undoubtedly promote the clinical translational research of this gaseous transmitter in future studies. Recently, the physiological significance of H2S metabolites, such as persulfides, polysulfides, and other reactive sulfur species is emerging (Koning et al., 2015). Moreover, H2S and reactive sulfur species could coexist in biological system as they are normally interchangeable (Bolton et al., 2019). It is believed that reactive sulfur species might be responsible for at least some biological activities of H2S (Shimamoto and Hanaoka, 2015). However, the comparisons of the protective effects of H2S and its metabolites on cells or tissues remain unclear, which merits further research.
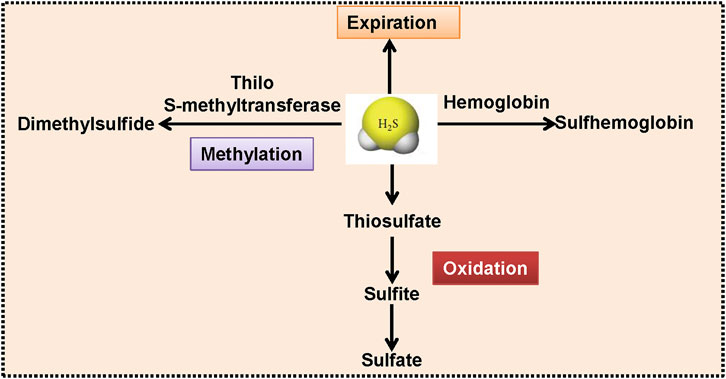
FIGURE 3. The proposed rotes for H2S degradation. With the aid of thiol S-methyltransferase, H2S is methylated into dimethylsulfide. H2S could be oxidized to thiosulfate, coupled with the production of sulfite and sulfate. Hemoglobin interacts with H2S, resulting in the generation of sulfhemoglobin. H2S can be released from the mammalian lungs. H2S, hydrogen sulfide.
The potential of H2S metabolites as biomarkers is being appreciated since the levels of H2S metabolites in serum and urine may reflect renal disease severity, such as chronic kidney disease (Nakanishi et al., 2002; Koning et al., 2015). In chronic heart failure patients, plasma sulfate concentrations tend to be higher, and sulfate clearance is associated with favorable disease outcome (Koning et al., 2017). Although these observations are promising, it is clear that the recognition of H2S metabolites as disease biomarkers is not flawless. Meanwhile, the quantification of H2S metabolites in the ischemia-reperfusion conditions or in other cardiovascular debacles has yet to be fully elucidated. Therefore, large-population studies investigating the potential value of H2S metabolites as disease biomarkers are underway. Overall, our current understanding of H2S metabolites and how their dysfunction contributes to cardiovascular pathogenesis remains largely unclear, thereby requiring more studies.
Pharmacology and Toxicology of H2S
It has been well documented that H2S is a crucial signaling molecule in the cardiovascular system because of its physiological and pathological significances in cardiovascular homeostasis (Liu et al., 2011; Pan et al., 2011; Sun et al., 2011; Liu et al., 2012; Pan et al., 2012; Wang et al., 2015b; Nagpure and Bian, 2016). Plasma and tissue levels of H2S are sophisticatedly controlled by its formation and catabolism in health (Elsey et al., 2010; Huang et al., 2015). Under pathological conditions, endogenous H2S levels and H2S-prodocuing enzyme activities/expressions are significantly altered (Pan et al., 2017; Sun et al., 2019a; Sun et al., 2019b). Both experimental and clinical evidence has revealed that perturbation of endogenous H2S system is obviously linked with the pathologies of cardiovascular disorders, including atherosclerosis, hypertension, diabetic cardiomyopathy, myocardial ischemia/reperfusion injury, endothelial dysfunction, and heart failure (Jiang et al., 2005; Wang et al., 2015c; Gao et al., 2015; Meng et al., 2015; Yang and Wang, 2015; Donnarumma et al., 2017; Kanagy et al., 2017; Li et al., 2017; Kar et al., 2019a). In renal physiology, H2S induces vasodilation, and increases renal blood flow and glomerular filtration rate, leading to an indirect increase of the urinary excretion of Na+ and K+ (Roy et al., 2012). H2S exhibits an inhibitory effect on specific Na+ and K+ transporters in the kidney, thus further increasing the excretion of such electrolyte into the urine (Xia et al., 2009). H2S acts as an oxygen sensor in the renal system, especially in medulla (Bełtowski, 2010). Also, H2S is found to inhibit renin release in rat models of renovascular hypertension (Lu et al., 2010). Hypertension-related nephropathy, a result of long-term hypertension, is the second leading cause of chronic kidney disease world (Hart and Bakris, 2010). The blood pressure lowering effects of exogenous H2S donors have been demonstrated in spontaneous hypertensive rats (Ahmad et al., 2014; Li et al., 2019a), angiotensin II-induced hypertension (Al-Magableh et al., 2015; Hsu and Tain, 2018; Chi et al., 2019), Nw-nitro-l-argininemethyl ester (l-NAME)-induced hypertension (Ji et al., 2014; Jin et al., 2017), and renovascular hypertension (Guo et al., 2017a; Feng et al., 2020). It is reasonable that renal protective effects of H2S are observed in these hypertensive models. Subsequent studies have suggested that suppression of ROS formation and epithelial sodium channel, upregulation of vascular endothelial growth factor might mediate renal protective effects of H2S in hypertension (Zhang et al., 2013; Ahmad et al., 2014; Holwerda et al., 2014; Wang et al., 2015a). Taken together, H2S serves as an ideal candidate for the prevention and treatment of hypertension-related renal damage.
The dose of H2S donor, sodium hydrosulfide (NaHS), ranging from 1.4 mg/kg/d to 56 mg/kg/d was used in animals, but 1 μM to 10 mM in cells (Wang et al., 2020). Similarly, the concentration of other H2S donors varies greatly in both animal and cell experiments (Wang et al., 2020). Here, it is thought-provoking to determine the exact pharmacological doses of H2S that are biologically effective or detrimental. Solving this problem may help to answer whether it is appropriate to use a dissolved exogenous H2S solution as a tool to determine the potential physiological function of endogenous H2S and its putative therapeutic applications. One should keep in mind that high micro-moles or even milli-moles solutions of dissolved H2S in organs or milieubathing cells have been repeatedly used in the literatures (Szabó, 2007; Nicholson and Calvert, 2010; Szabó et al., 2011). However, the solutions of dissolved H2S with high micro-moles or even milli-moles in vitro are above the concentrations of dissolved H2S found in blood and tissues during lethal H2S exposure in vivo. In addition, the level of toxicity varies greatly between different cell types, thus the physiological relevance of the data obtained after local or in vitro administration of H2S at concentrations of micro-moles or milli-moles is far from uncertain. Also, H2S is one of the most toxic mitochondrial poisons, even more toxic than cyanide on a mole-to-mole basis (Cooper and Brown, 2008). The activity of mitochondrial cytochrome c oxidase is diminished by a solution of dissolved H2S at a concentration ranging from 10 to 30 μM (Yong and Searcy, 2001; Leschelle et al., 2005). In vivo studies have shown that a severe depression in respiratory medullary neurons and/or cardiac contractility is observed in rodents and large mammals by infusing or inhaling H2S at levels yielding blood levels of gaseous H2S between 2 and 5 μM (Klingerman et al., 2013; Haouzi et al., 2014; Sonobe and Haouzi, 2016). A depression in cardiac contractility is produced by a solutions of H2S at concentrations above 50 μM on an isolated heart or cardiomyocytess (Geng et al., 2004; Sun et al., 2008; Yong et al., 2008). It is likely that this decrease in cardiac contractility is pathological and toxic at both the level of individual cardiac cells and the whole heart when exogenous H2S concentration reaches higher than the phyiological levels (Judenherc-Haouzi et al., 2016). In all, few micro-moles concentrations of dissolved H2S is toxic to most tissues in vivo, impacting vital respiratory and cardio-vascular functions (Haouzi, 2016). However, it seems that high levels of H2S are still administrated in the past, present and future research at the animal and cellular levels.
To date, it is merited to answer why the toxic levels of H2S are used in physiological studies. The rationality of using high levels (i.e., micro-moles or even milli-moles) of H2S to study and predict its physiological effects depends on the initial reports, where the concentration of H2S in blood or tissue is at high micromolar in the blood or tissues (Furne et al., 2008). Philippe Haouzi has reviewed that two main reasons could explain why high micro-moles of “endogenous” H2S are found in the blood and tissues (Haouzi, 2016). The first reason is related to the nature of the pools of sulfide present in the blood and in tissues, and the second reason for this error is linked with the methodology of measurement of the pool of diffusible/dissolved H2S in a biological milieu (Van de Louw and Haouzi, 2012). The subsequent studies have found that low levels of H2S donors in vivo are unlikely to increase the concentrations of H2S in tissues because of a fact that the majority of H2S in the blood after intravenous or intraperitoneal injections in vivo is immediately oxidized or combined with metallo-proteins (hemoglobin), dropping the level of free H2S to almost zero, unless the lethal levels are applied (Insko et al., 2009; Wintner et al., 2010; Haouzi and Klingerman, 2013; Klingerman et al., 2013). There is a very small margin between the concentration that does not produce an effect and the concentration that can kill. As reviewed by Szabo et al. (2011), the variable concentrations of H2S donors might result in the toxic levels or without chaging H2S concentrations in blood and tissues (Szabó, 2007). A growing number of studies support the “physiological” and protective roles of H2S by using solution of free H2S in the high micro-moles levels that are only found during a lethal in toxication in vivo (Haouzi and Klingerman, 2013). As such, high micro-moles or milli-moles levels of H2S donors are still used to elucidate the properties of endogenous H2S in those ongoing studies. In all, H2S donors regulate a multitude of biological processes at the typically used doses (so-called physiological effect), which should belong to the field of toxicology in many cases (Haouzi and Klingerman, 2013). Future studies are necessary to quantify the actual H2S concentration of each exogenous H2S donor used in the tissues and determine what effects are expected to be produced in keeping with sulfide toxicity and its rapid disappearance in the body or solutions.
H2S: A Potential Cardioprotective Agent Against Diabetic Cardiomyopathy
Plasma H2S levels are remarkably decreased in diabetic patients and animals (King and Lefer, 2011; Guo et al., 2017b; Kesherwani et al., 2017; Suzuki et al., 2017). The levels of H2S in plasma, heart tissues, and urine tend to be lower in aging diabetic mice when compared to controls (Jin et al., 2015). Likewise, the myocardium expressions of H2S-generating enzymes, CSE and 3-MST, are significantly decreased in the heart samples of diabetic mice, whereas CBS levels are significantly elevated (Jin et al., 2015). Both circulating and myocardium levels of H2S are also reduced in high fat diet (HFD)-induced obese diabetic mice (Barr et al., 2015). In streptozotocin (STZ)-induced type 1 diabetic rats, a significant decrease in myocardial H2S concentration is also observed (Palamarchuk et al., 2020). These findings characterize a critical role for endogenous H2S dysfunction in the etiology of diabetic cardiomyopathy.
Recently, both in vivo and in vitro evidence has demonstrated the protective roles of H2S donors in diabetic cardiomyopathy (Table 4 and Table 5) (Wang, 2010; Patel et al., 2015). For instance, administration of exogenous H2S donors ameliorates cardiac dysfunction in diabetic rats and mice (Zhou et al., 2015; Sun et al., 2020d), which may be mediated by multiple signaling pathways or target proteins that are involved in myocardial hypertrophy (Cheng et al., 2016), cardiac fibrosis (Zhou et al., 2015), endoplasmic reticulum stress (Li et al., 2016), S-sulfhydration modification (Sun et al., 2020d), cardiomyocyte apoptosis, oxidative stress, and inflammation (Ye et al., 2018), NO production (Huang et al., 2016a), and autophagy (Wu et al., 2017).
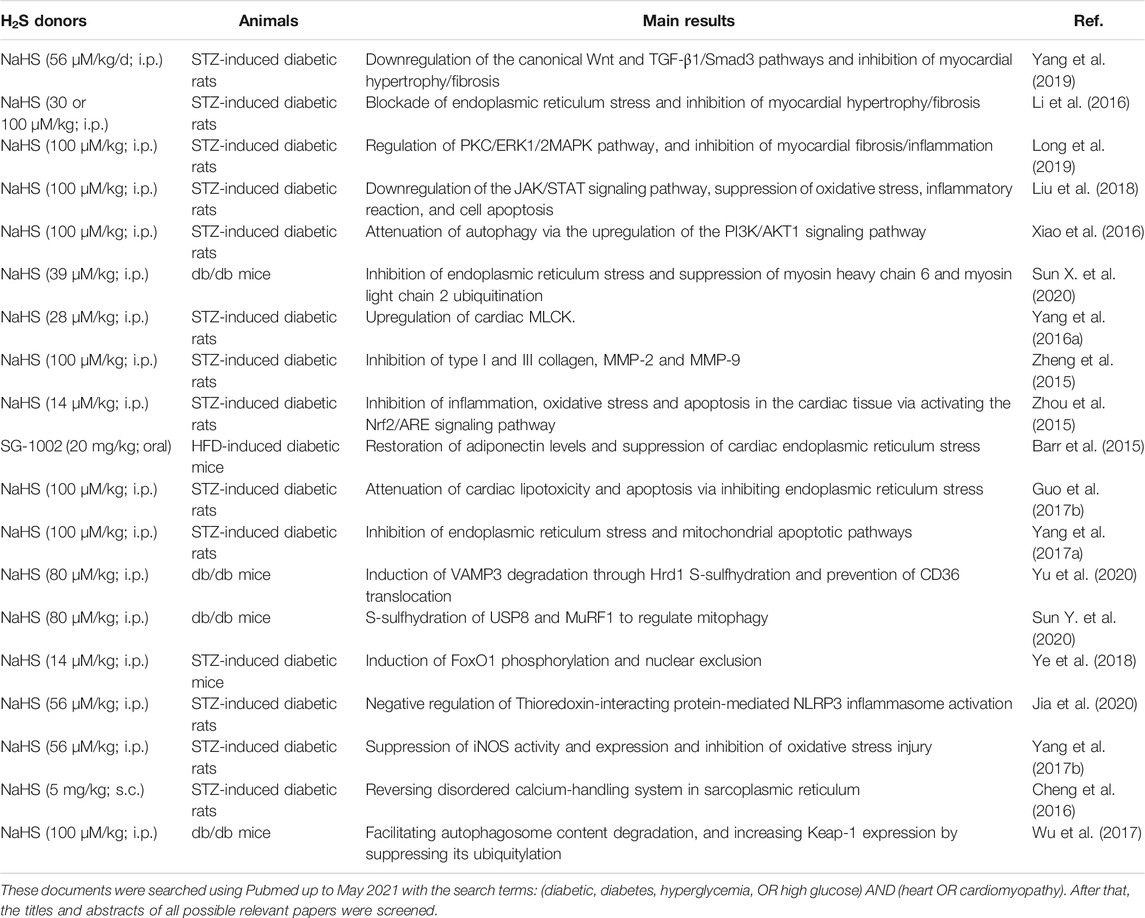
TABLE 4. Molecular mechanisms of H2S donors in the treatment of diabetic cardiomyopathy at the animal level.
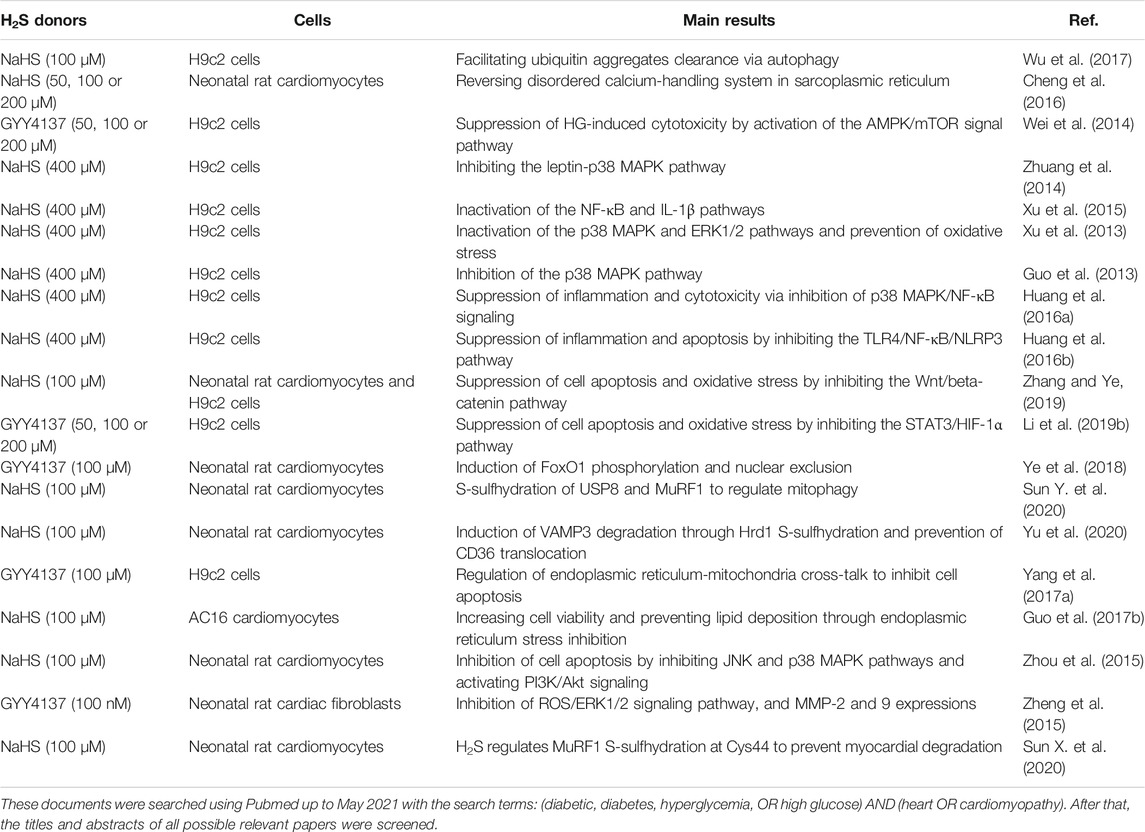
TABLE 5. Molecular mechanisms of H2S donors in the treatment of diabetic cardiomyopathy at the cellular level.
Induction of endogenous H2S production is also beneficial for diabetic cardiomyopathy. Diallyl trisulfide is reported to afford a protection against high glucose-provoked cardiomyocyte apoptosis by stimulating the formation of CSE-derived H2S (Tsai et al., 2015). Metformin, one of the most widely prescribed insulin sensitizer, might benefit diabetic cardiomyopathy by enhancing H2S production in the heart (Wiliński et al., 2013). Phosphodiesterase 5 (PDE5) inhibitors have powerful protective effects against diabetic cardiomyopathy, and this may be dependent on H2S generation (Das et al., 2015). Exercise training mitigates HFD-induced diabetic cardiomyopathy via promoting cardiac H2S biosynthesis and subsequent prevention of cardiomyocyte pyroptosis (Kar et al., 2019b). Pretreatment with curcumin alleviates pathological morphological damage in myocardium tissues from diabetic rats, and the beneficial effects of curcumin might be related with increased myocardium CSE and H2S levels (Tong et al., 2018). These exciting findings suggest the cardioprotective actions of H2S-based therapeutics in preclinical models of diabetic cardiomyopathy. Modulation of endogenous H2S production or application of exogenous H2S donors might serve as promising therapies for the management of diabetic cardiomyopathy. Later, we will provide a comprehensive overview on the critical molecular/cellular mechanisms that mediate H2S-induced favorable effects on diabetic cardiomyopathy. Emerging evidence suggests that H2S-mediated signaling transduction is correlated with production of NO (Paul and Snyder, 2012; Polhemus and Lefer, 2014; Li et al., 2018; Sun et al., 2020b). Thus, the current roles of interactive functions of H2S and NO in diabetic cardiomyopathies are also elaborated in this review.
Inhibition of Myocardial Fibrosis and Hypertrophy
A previous study has found that administration of GYY4137 diminishes rat neonatal cardiac fibroblast migration exposed to high glucose (Zheng et al., 2015). The underlying mechanisms may involve inhibition of reactive oxygen species (ROS)/ERK1/2 signaling, indicating that H2S antagonizes cardiac fibrosis in diabetic cardiomyopathy by regulating redox homeostasis (Zheng et al., 2015). Consistent with this finding, NaHS prevents cardiac hypertrophy/fibrosis and ameliorates left ventricular dysfunction in STZ-induced diabetic rats by activating the nuclear factor erythroid 2-related factor 2 (Nrf2)/antioxidant response element (ARE) signaling pathway, accompanied by upregulations of antioxidant proteins haem oxygenase-1 (HO-1) and Nad(p)h: quinone oxidoreductase 1 (NQO1) in myocardium tissues from diabetic rats (Zhou et al., 2015). Exogenous H2S reverses myocardial hypertrophy and fibrosis through suppressing cardiac levels of myosin heavy chain 6 and myosin light chain 2 ubiquitination in db/db mice (Sun et al., 2020d). Compared with diabetic rats, the left ventricular hemodynamic parameters and myocardial ultrastructure changes (myocardial hypertrophy and fibrosis) are obviously normalized in diabetic animals treated with NaHS, which may be associated with upregulation of cardiac myosin light chain kinase (MLCK) and downregulation of serum creatine kinase MB isozyme (CK-MB) (Yang et al., 2016a).
The myocardial expressions of pro-fibrotic factors, including matrix metalloprotease 2 (MMP-2), tissue inhibitor of metalloproteinase 2 (TIMP-2), collagens, transforming growth factor (TGF)-β1/SMAD family member 3 (Smad3) signaling pathway, are strikingly changed in diabetic rats (Yang et al., 2019). However, an exogenous H2S donor, NaHS, ameliorates diabetes-induced myocardial fibrosis by inhibition of the Wnt and TGF-β1/Smad3 pathway and downregulation of myocardial collagen overproduction (Yang et al., 2019). Administration of NaHS for 8 weeks prevents myocardial hypertrophy and collagen deposition in STZ-induced diabetic rats by suppression of endoplasmic reticulum stress (Li et al., 2016). Inhibition of the protein kinase C (PKC)/extracellular regulated protein kinase 1/2 (ERK1/2) signaling pathway is required for H2S to ameliorate myocardial fibrosis in diabetic rats induced by STZ (Long et al., 2019). Liu et al. (2018) have found that the effects of NaHS on reversing diabetic myocardial fibrosis may be associated with blockade of the Janus kinase/signal transducer and activator of transcription (JAK/STAT) signaling pathway. Hematoxylin and eosin staining and Masson staining results have shown that NaHS is able to improve myocardial hypertrophy and fibrosis in diabetic rats induced by STZ, and the underlying mechanism may be dependent on activation of the phosphatidylinositol 3-kinase (PI3K)/Akt signaling pathway and following autophagy inhibition (Xiao et al., 2016). These above observations evidently suggest that H2S donors function as an attractive alternative for the prevention and treatment of diabetes-provoked myocardial hypertrophy and fibrosis (Kang et al., 2020).
Suppression of Endoplasmic Reticulum Stress
Nowadays, the involvement of endoplasmic reticulum stress in the development and progression of diabetic cardiomyopathy is well accepted (Figure 4) (Xu et al., 2012). Given the important roles of endoplasmic reticulum stress in the pathologies of diabetic cardiomyopathy, inhibition of endoplasmic reticulum stress would be a therapeutic strategy for intervention of diabetic cardiomyopathy. Interestingly, multiple lines of evidence have proved that the protective effects of H2S against diabetic cardiomyopathy are related to inhibition of endoplasmic reticulum stress. For instance, pretreatment of AC16 cardiomyocytes with NaHS could retard palmitic acid-induced cardiac lipotoxicity and apoptosis, which is similar to the effects of an endoplasmic reticulum stress inhibitor 4-phenylbutyric acid (Guo et al., 2017b). The expressions of endoplasmic reticulum stress-associated proteins, including glucose-regulated protein (GRP78), C/EBP-homologous protein (CHOP), and caspase-12, are incremented in diabetic heart tissues, while H2S suppresses diabetes-induced endoplasmic reticulum stress and abrogates myocardial hypertrophy and myocardial collagen deposition in diabetic rats (Li et al., 2016). In line with this, intraperitoneal injection of NaHS improvesc cardiac dysfunction and myocardial ultrastructure damage by suppressing the mRNA expressions of endoplasmic reticulum stress markers, such as CHOP, GRP78 and caspase 12 (Yang et al., 2016b). Furthermore, the upregulated expressions of endoplasmic reticulum stress-related proteins and mitochondrial apoptotic proteins in diabetic cardiac tissues are reduced to normal levels in the presence of NaHS (Yang et al., 2017a).
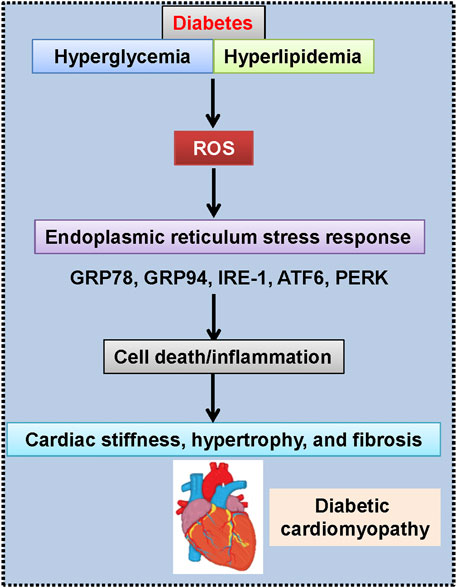
FIGURE 4. Engagement of endoplasmic reticulum stress in diabetic cardiomyopathy. ATF6, activating transcription factor 6; GRP78, glucose-regulated protein-78; GRP94, glucose-regulated protein-94; IRE-1, inositol-requiring kinase-1; PERK, protein kinase R-like ER kinase; ROS, reactive oxygen species.
Deletion of endogenous H2S is critically involved in diabetes-induced cardiac damage, whereas exogenous H2S donor NaHS improves diabetic cardiomyopathy though inhibition of endoplasmic reticulum stress (Guo et al., 2017b). Molecular experiments have revealed that H2S therapy (SG-1002) is capable of relieving HFD-induced cardiomyopathy via suppressing cardiac endoplasmic reticulum stress and restoring cardiac adiponectin levels (Barr et al., 2015). These above findings indicate that H2S donors are postulated to serve as novel cardioprotective agents for diabetic cardiomyopathy due to its strong suppression of endoplasmic reticulum stress-dependent apoptosis pathway in the heart.
Protein S-Sulfhydration
S-sulfhydration (also called S-persulfidation or Persulfidation), a newly post-translational modification by generating a hydropersulfide moiety (–SSH) or polysulfide in specific cysteine residues of target proteins, is discovered to mediate most of the cellular functions induced by H2S (Paul and Snyder, 2012; Paul and Snyder, 2015; Zhang et al., 2017; Sun et al., 2021). Accordingly, several S-sulfhydrated proteins are involved in H2S-mediated cardiovascular benefits in diabetes. HMG-CoA reductase degradation protein (Hrd1), an endoplasmic reticulum transmembrane E3 ubiquitin ligase, is vitally involved in ubiquitylation of various substrate proteins and subsequent protein trafficking (Doroudgar et al., 2015). Vesicle-associated membrane protein 3 (VAMP3) is predominantly expressed in the heart and plays a key role in controlling intracellular membrane trafficking and exocytosis (Haglund and Dikic, 2012). Emerging evidence has shown that triacylglycerol accumulation within the myocardium may be responsible for the development of diabetes-induced heart failure, and this process is mainly modulated by transport protein fatty acid translocase CD36 (Chandler et al., 2003; Coort et al., 2004). VAMP3 contributes to CD36 exocytosis in cardiomyocytes, thus leading to increased myocardial long-chain fatty acid uptake and triacylglycerol accumulation (Pulido et al., 2011). On these grounds, Zhang and colleagues hypothesized that H2S induced VAMP3 degradation through Hrd1 S-sulfhydration and prevented CD36 translocation, thereby alleviating lipid toxicity in the heart tissues of db/db diabetic mice (Yu et al., 2020). As expected, the authors have demonstrated that exogenous H2S restores Hrd1 expression by inducing its S-sulfhydration at Cys115 (Yu et al., 2020). VAMP3 ubiquitylation and degradation induced by H2S is able to delay CD36 exocytosis in cardiomyocytes and reduce droplet formation in the heart tissues from db/db mice (Yu et al., 2020). This study leads to a novel finding that H2S upregulates Hrd1 expression through its S-sulfhydration at Cys115 and results in VAMP3 ubiquitylation and the stability of membrane CD36 expression in cardiomyocytes, which is necessary to prevent myocardial long-chain fatty acid uptake and lipid droplet formation in diabetic heart tissues (Yu et al., 2020). Afterwards, the same group has identified that H2S promotes the S-sulfhydration of ubiquitin specific peptidase 8 (USP8) and muscle RING finger-1 (MuRF1), thereby preventing cardiac structural injury in diabetes (Sun et al., 2020d; Sun et al., 2020e). These observations suggest that protein S-sulfhydration is believed to participate in the protective roles of H2S donors in diabetes-induced cardiomyopathy. In this regard, more S-sulfhydrated proteins will be identified to be responsible for the therapeutic effects of H2S in the context of diabetic cardiomyopathy.
Inhibition of Cardiomyocyte Apoptosis, Oxidative Stress, and Inflammation
Evidence from cell cultures, animal models, and clinical studies has confirmed that H2S grants an anti-diabetic cardiomyopathy characteristic through anti-inflammation, anti-apoptosis, anti-oxidant stress pathways (Powell et al., 2018). Pretreatment of H9c2 cells with NaHS obviously counteracts high glucose-induced cardiac cell apoptosis and inflammation, as evidenced by measurement of caspase-3, inducible nitric oxide synthase (iNOS), cyclooxygenase 2 (COX-2), interleukin 1β (IL-1β), and IL-6 (Huang et al., 2016a). Inhibition of p38 MAPK/NF-κB signaling might mediate these cytoprotective effects of H2S in diabetes-induced cardiac apoptosis and inflammation (Huang et al., 2016a). Moreover, NaHS inactivated the toll-like receptor 4 (TLR4)/nuclear factor kappa-B (NF-κB) pathway to attenuate high glucose-induced nucleotide-binding oligomerization domain-like receptor protein 3 (NLRP3) inflammasome activation and cardiotoxicity in H9c2 cells (Huang et al., 2016b).
In addition, inactivation of Wnt/β-catenin signaling pathway and the signal transducer and activator of transcription 3 (STAT3)/hypoxia-inducible factor-1α (HIF-1α) pathway, upregulations of HO-1 and NQO1, induction of forkhead box O1 (FoxO1) phosphorylation and nuclear exclusion, are proposed to be main mechanisms for H2S donors to attenuate hyperglycemia-induced myocardial apoptosis and oxidative stress (Ye et al., 2018; Li et al., 2019b; Zhang and Ye, 2019). Moreover, H2S donor NaHS is reported to lessen hyperglycemia-induced inflammation, apoptosis, and oxidative stress in cardiac tissues by inhibiting c-Jun N-terminal kinase (JNK)/p38 mitogen-activated protein kinase (MAPK) pathways and activating PI3K/Akt signaling (Zhou et al., 2015). In the heart tissues, hyperglycemia significantly induces cardiac dysfunction with concomitant increases in redox perturbation and inflammatory reactions (Jia et al., 2020). However, treatment with NaHS significantly ameliorates these abnormalities, whereas treatment with propargylglycine (a specific inhibitor of H2S) further aggravated such alterations (Jia et al., 2020). These studies highlight the protective roles of H2S in diabetes-induced cardiac damage, and its potential mechanism may involve the negative regulation of cardiomyocyte apoptosis, oxidative stress, and inflammation.
Different from apoptosis, pyroptosis is a special pattern of cell death whereby the canonical pathway is reliant on caspase-1 and the noncanonical pathway is specifically dependent on caspase-11 (Bergsbaken et al., 2009). Initially found in monocytes and macrophages, pyroptosis is recently found to be involved in pathological inflammation-related cell death (Miao et al., 2010). NLRP3 inflammasome activates caspase-1 and results in cell swelling and lysis, and consecutive release of IL-1β and IL-18, characteristics of pyroptosis (Bergsbaken et al., 2009). Pyroptosis and its downstream inflammatory cytokines are closely linked with cardiovascular diseases, including diabetic cardiomyopathy (Kar et al., 2019a). NLRP3-dependent pyroptosis is detected in diabetic heart tissues, and it participates in the pathogenesis of diabetic cardiomyopathy (Luo et al., 2014; Luo et al., 2017). As a cardioprotective gaseous signaling molecule, H2S donor treatment suppresses caspase-1 activity and caspase-1 recruitment via the apoptosis-associated speck-like protein containing A CARD (ASC) adapter protein in a rat model of myocardial ischemic and inflammatory injury (Toldo et al., 2014). Recently, induction of H2S biosynthesis by exercise ameliorates HFD-induced cardiac structural and metabolic by inhibition of pyroptotic signaling (Kar et al., 2019b). However, further evidence is required to extrapolate the benefits of H2S donors-induced inhibition of pyroptosis on diabetic cardiomyopathy.
Inhibition of the Leptin-p38 Mitogen Activated Protein Kinase Pathway
Leptin, a 16-kD hormone primarily secreted by adipocytes, exhibits a wide range of biological activities through binding to the leptin receptors (Friedman and Halaas, 1998). It is found that diabetes-induced cardiomyocyte hypertrophy may be mediated by leptin and its receptors (Majumdar et al., 2009). Aberrant expression of p38 MAPK is detected in the heart tissues from diabetic animals, and inhibition of p38 MAPK markedly delays the progression of diabetic cardiomyopathy (Zuo et al., 2019). In light of its capacity to facilitate diabetic cardiomyopathy development, p38 MAPK serves as an attractive target for the treatment of diabetic cardiomyopathy (Li et al., 2014). Indeed, inhibition of the p38 MAPK pathway is responsible for H2S-mediated protective effects against high glucose- or oxorubicin-induced cardiotoxicity in H9c2 cells (Guo et al., 2013; Xu et al., 2013).
Venkatesh and coworker have confirmed that leptin and its downstream p38 MAPK signaling pathway contribute to endothelin-1- and angiotensin-II-induced cardiomyocyte hypertrophy (Rajapurohitam et al., 2012). However, it remains to be investigated whether the leptin-p38 MAPK pathway contributed to high glucose-caused cardiomyocyte insult, and whether the pharmacological actions of H2S are related to this signaling pathway. To answer this, Liao’ group measured the leptin, leptin receptors, and p38 MAPK expression levels in high glucose-challenged cardiomyoblasts (Zhuang et al., 2014). Results have shown that the expression levels of leptin and its receptors are significantly enhanced in cardiomyoblasts (H9c2 cells) upon exposure to high glucose (35 mM) for 24 h, while these increases are markedly attenuated by pretreatment with NaHS (400 μM), a donor for H2S (Zhuang et al., 2014). Additionally, high glucose-induced increased phosphorylated p38 MAPK expression is reversed by either NaHS or leptin antagonist, suggesting the involvement of the leptin-p38 MAPK axis in high glucose-elicited cardiomyoblast injury (Zhuang et al., 2014). Importantly, H2S-mediated inactivation of the leptin-p38 MAPK pathway mitigates high glucose-evoked apoptosis, ROS generation, and mitochondrial membrane potential loss in H9c2 cells (Zhuang et al., 2014). Altogether, this study indicates that exogenous H₂S donors protect H9c2 cells against high glucose-induced injury at least in part by inactivating the leptin-p38 MAPK signaling pathway.
Crosstalk With NO
A plethora of studies have revealed the physiological and/or pathological roles of H2S and NO in various systems during the last several decades (Lee and Yen, 2009; El-Mas et al., 2012; Nagpure and Bian, 2016; Magierowski et al., 2018; Wu and Hu, 2018; Mukherjee and Corpas, 2020; Xuan et al., 2020). Importantly, studies on the interaction of H2S and NO in diabetic cardiomyopathy are gradually emerging.
Several studies have disclosed that H2S regulates NO activities in the cardiovascular system (Zhang et al., 2020a), suggesting that H2S might exhibit cardioprotective effects via NO signaling. A phase I clinical trial has demonstrated that administration of a novel H2S prodrug, SG1002, increases H2S and NO levels in both healthy controls and heart failure patients (Polhemus et al., 2015). Exposure of endothelial cells with H2S stimulates NO production from endothelial nitric oxide synthase (eNOS) phosphorylation at Ser 1177 through an Akt-dependent mechanism (Predmore et al., 2011). CSE knockout mice are more susceptible to transverse aortic constriction as CSE-deficient mice display greater cardiac dilatation and dysfunction (Kondo et al., 2013). By contrast, cardiac-specific CSE overexpression or H2S therapy with SG-1002 restores cardiac structure and function after transverse aortic constriction through activation of the vascular endothelial growth factor-Akt-eNOS-NO-cGMP pathway (Kondo et al., 2013). Furthermore, CSE-deficient mice exhibit diminished eNOS activities, dysfunctional NO levels, and are more vulnerable to myocardial and hepatic ischemia reperfusion injury (King et al., 2014).
Acute H2S therapy improves myocardial and hepatic ischemia reperfusion injury by restoring eNOS function and NO bioavailability (King et al., 2014). NaHS treatment restores the impaired endothelial-dependent relaxation of the aorta in diabetic rats by a NOS-dependent mechanism as inhibition of NOS activity by l-NAME abolishes NaHS-mediated vasodilatation in diabetic aortas (Dorofeyeva et al., 2020a). These findings suggest that H2S-mediated cytoprotective signaling may be dependent in large part on eNOS-derived NO formation. As a pro-inflammatory factor and NO producer, iNOS express levels are upregulated in high glucose-incubated H9c2 cells, and H2S counteracts high glucose-induced inflammation and cytotoxicity via inhibition of iNOS expression (Huang et al., 2016a). In keeping with this, myocardial iNOS activities and expressions are significantly elevated in diabetic rats, which are suppressed by treatment with NaHS, a donor for H2S (Yang et al., 2017b). In sharp contrast, modulation of H2S synthesis stimulates eNOS activities and NO production in the heart tissues and aortic rings, thus recovering endothelium-dependent relaxation and arterial elastance, as well as cardiac dysfunction in diabetic rats (Dorofeyeva et al., 2021). Injection of STZ elicits reductions in myocardial CSE expression/activity, H2S and NO levels in rats, and moxonidine treatment abrogated cardiovascular dysfunction in diabetic rats through restoring cardiac H2S and NO levels (El-Sayed et al., 2016). These above findings imply that H2S confers a protective effect on the myocardium in diabetic animals, which may be associated with the regulations of iNOS, eNOS, and NO production.
It should be mentioned here that chemical interaction of H2S with NO might yield nitroxyl (HNO) (De Witt et al., 2001; Hrabie and Keefer, 2002; Cortese-Krott et al., 2014), one-electron-reduced product of NO, and HNO is emerging as a potential pharmacological agent for various diseases by targeting numerous signal pathways (Irvine et al., 2008; Switzer et al., 2009; Sun et al., 2020a; Sun et al., 2020b). An interesting study has shown that CSE-produced H2S scavenges vascular NO levels in peripheral arteries and contributes to blood pressure regulation (Szijártó et al., 2018). Pretreatment of vascular rings from CSE-deficient mice with H2S donor ameliorates endothelial vasorelaxant response by decreasing NO levels and restoring HNO levels in arteries (Szijártó et al., 2018). Our group recently found that mixture of H2S donor NaHS and NO donor sodium nitroprusside (SNP) gives rise to HNO formation in cardiomyocytes (Sun et al., 2020c). HNO donor Angeli’s salt significantly ameliorates hygerglycemia-induced myocardial apoptosis, hypertrophy, fibrosis, ROS generation, and cardiac performance in diabetic mice (Sun et al., 2020c). Induction of caveolin-3/eNOS complex may be required for HNO to ameliorate the development of diabetic cardiomyopathy (Sun et al., 2020c). In consistence with this finding, the left ventricular structural remodeling and diastolic dysfunction are limited by chronic administration of HNO donor 1-nitrosocyclohexylacetate (1-NCA) in diabetic FVB/N mice (Cao et al., 2015). It is well known that NO resistance, a state in which the tissue responsiveness to exogenous and endogenous NO is impaired, is frequently occurred in cardiovascular disease states, including diabetic cardiomyopathy (Velagic et al., 2020).
In diabetic rats, acute NO effects induced by diethylamine-NONOate, including vasodilation, myocardial contraction and relaxation, are evidently impaired (Qin et al., 2020). In contrast, isopropylamine-NONOate-induced acute HNO effects are still preserved in the setting of diabetes, and the positive inotropic effects of HNO are obviously elevated in diabetic rat heart (Qin et al., 2020). This intriguing study provides a possible clue that HNO largely circumvents NO resistance in diabetic heart tissues, and supports a possible role for HNO in the management of ischemia and heart failure in diabetes. These above observations suggest that H2S and NO enter a redox reaction with each other, resulting in the formation of HNO, a potential way to antagonize the occurrence and development of diabetic cardiomyopathy. However, we can not be too optimistic on the preclinical studies of HNO in diabetic cardiomyopathy due to our insufficient understanding of HNO biosynthesis, chemistry, biology, and pharmacology. Thus, these aspects warrant further interrogation with the emphasis on HNO detection methods, long-lasting donors with safety and efficiency, pharmacological potentials beyond the cardiovascular system.
Other Mechanisms
Adenosine 5′-monophosphate (AMP)-activated protein kinase (AMPK) is a metabolic master switch that play an indispensable role in energy metabolism and organic lipid homeostasis (Bai et al., 2018). Its activation exerts a beneficial effect in diabetic cardiomyopathy, while its inactivation is involved in cardio-metabolic abnormalities, including diabetes-induced cardiac apoptosis, myocardial fibrosis, and ventricular hypertrophy (Haye et al., 2020). AMPK negatively regulates mammalian target of rapamycin (mTOR), and downregulation of mTOR mediates a host of biological effects induced by AMPK (Liao et al., 2013). A host of studies have highlighted the importance of AMPK inactivation in the pathogenesis of diabetic cardiomyopathy (Haye et al., 2020). On this basis, it is reasonable to hypothesize that H2S might protect cardiomyocytes from hyperglycemia stress through AMPK-mediated inhibition of mTOR. Indeed, Wei et al. (2014) have ascertained that GYY4137, a novel H2S-releasing molecule, reverses high glucose-induced cell apoptosis, lactate dehydrogenase release, and mitochondrial membrane potential collapse. An AMPK activator, 5-amino-4-imidazole-carboxamide riboside (AICAR), has similar effects to GYY4137 treatment, whereas adenine 9-β-d-arabinofuranoside (an AMPK inhibitor) abolishes GYY4137-mediated cardiac benefits under diabetic conditions (Wei et al., 2014). Most importantly, both GYY4137 and AICAR stimulated AMPK phosphorylation and diminished mTOR phosphorylation in high glucose-challenged H9c2 cells, suggesting that H2S ameliorates diabetes-induced cardiac damage in an AMPK/mTOR pathway-dependent manner (Wei et al., 2014).
The presence of cardiac dysfunction in diabetes is accompanied by overload of intracellular calcium in cardiomyocytes (Chen et al., 2020). When cardiomyocytes are excited, increased calcium ion will flow into the cells through L-type Ca2+ channels, followed by the release of Ca2+ from the sarcoplasmic reticulum (SR) via the ryanodine receptor (RyR2), leading to actin–myosin interaction. Cellular calcium overload is closely related with SR dysfunction, such as RyR2-FK506-binding proteins (FKBP12.6) disassociation, downregulation of sarcoplasmic reticulum Ca2+ ATPase (SERCA2a) and calsequestrin 2 (CASQ2) (Cheng et al., 2012; Carneiro-Júnior et al., 2014; Roussel et al., 2015; Tomasova et al., 2015). In STZ-induced diabetic rats, the expressions of calcium-handling proteins in the SR, such as FKBP12.6, SERCA2a, and CASQ2 are downregulated, while the concentration of diastolic free calcium was elevated, indicating a calcium leak in diabetic heart tissues (Cheng et al., 2016). However, these abnormalities are attenuated in diabetic rats when treated with NaHS, indicating that H2S donors ameliorate diabetic cardiomyopathy-related imbalance in calcium homoeostasis by reversing abnormal expressions of disordered calcium-handling system in the SR (Cheng et al., 2016).
Autophagy plays a critical role in cellular homeostasis by clearing damaged or unnecessary organelles or macronutrients dependent on the lysosomal degradation pathway (Xie et al., 2011). Dysfunction of autophagy is shown to be involved in diabetic cardiomyopathy (Lavandero et al., 2015). It has been revealed that H2S is a regulator in autophagy during the process of diabetic cardiomyopathy. In type 2 db/db mouse heart tissues, NaHS facilitates autophagosome content degradation and promotes ubiquitin aggregate clearance via autophagy, which might exert its cardiovascular effect in diabetic cardiomyopathy (Wu et al., 2017). It is likely that H2S increases autophagic flux to ameliorate diabetic cardiomyopathy.
H2S Protects Against Myocardial Ischemia/Reperfusion Injury in Diabetes
Ischemia/reperfusion cardiac injury is recognized as one of the leading causes of people morbidity and mortality around the world (Yan et al., 2020). Primarily, ischemia/reperfusion leads to the death of cardiomyocytes and increased cardiac infarct size, which can be responsible for up to 50% of the final infarct size (Yellon and Hausenloy, 2007). It has been revealed that H2S donors could reduce myocardial ischemia/reperfusion injury by preserving mitochondrial functions and modulation of the signaling pathways of H2S by pharmacological agents is likely to prevent ischemia/reperfusion cardiac injury (Andreadou et al., 2020). In addition, the protective roles of H2S in myocardial ischaemia/reperfusion injury and cardioprotection by preconditioning or postconditioning have been reviewed (Andreadou et al., 2015). These published findings demonstrated that modulation of endogenous H2S production may be of clinical benefit in cardiac ischaemia/reperfusion injury.
Mounting evidence has found that diabetic hearts are more susceptible to ischemic injury under ischemia, hypoxia or anoxia conditions (Hearse et al., 1975; Feuvray et al., 1979; Feuvray and Lopaschuk, 1997). This notion is further supported by clinical studies that diabetic patients complicated with coronary heart disease and post-myocardial infarction might have a worse prognosis with higher incidence of heart failure or sudden death (Jaffe et al., 1984; Gwilt et al., 1985; Stone et al., 1989; Saeid et al., 2018). Studies have shown that hyperglycemia-induced decrease in coronary collateral blood flow and impaired responses of coronary microcirculation to ischemia, might cooperatively lead to exacerbated cardiac dysfunction during myocardial ischemic states (Gu et al., 2003; Badalzadeh et al., 2015). It is likely that hyperglycemia might aggravate myocardial damage in the heart when combined other heart diseases, such as myocardial ischemia-reperfusion injury (Lejay et al., 2016). Interestingly, evidence is emerging that H2S is a strong protector against myocardial ischemia-reperfusion injury in diabetes (Jeddi et al., 2020). Therefore, in the sections below, we will provide a comprehensive overview of the therapeutic roles of H2S signaling in diabetes-aggravated myocardial infarction or myocardial ischemia-reperfusion injury.
Diabetes not only directly induces cardiac cardiomyopathy (a cardiac disease independent of coronary artery disease, hypertension, and hyperlipidemia), but also worsens cardiac damage in response to myocardial infarction or ischemia/reperfusion (Schramm et al., 2008). To date, various pharmacological interventions are documented to attenuate myocardial ischemia/reperfusion injury in the setting of diabetes (Chin et al., 2017). Among them, H2S-based therapies have attracted tremendous attention because of its great potential in limiting cardiac injury during myocardial ischemia-reperfusion under diabetic state (Donnarumma et al., 2017).
Previously, Bridgette et al. had sought to examine whether H2S granted a cardioprotection against ischemia-reperfusion injury in the setting of type 2 diabetes (Peake et al., 2013). In this work, they found that H2S donor sodium sulfide (Na2S) precondition for 24 h or 7 days all improved myocardial injury in db/db diabetic mice (Peake et al., 2013). In an effort to evaluate the signaling mechanism responsible for the observed cardioprotection, they demonstrated that exogenous H2S ameliorated myocardial ischemia-reperfusion injury in db/db diabetic mice by activating Nrf2 signaling in an ERK1/2-dependent manner (Peake et al., 2013). Later, the same group further validated that H2S provides cardioprotection against myocardial-ischemia reperfusion injury in db/db diabetic mice by activating the ERK1/2 arm of the Reperfusion Injury Salvage Kinase (RISK) pathway (Lambert et al., 2014). Subsequently, several groups have verified that H2S-associated protection against ischemia-reperfusion injury in diabetic heart may also involve eNOS-derived NO production (Jeddi et al., 2020), PI3K/GSK3β pathway (Ansari and Kurian, 2020), and preservation of mitochondria (Ansari and Kurian, 2019; Mahalakshmi and Kurian, 2020). These findings provide ample evidence that H2S renders cardioprotection against ischemia/reperfusion injury in diabetes. However, more studies are needed to assess whether pre- and post-conditioning the heart with different H2S donors could behave differently in terms of ischemia-reperfusion injury limitation.
Clinical Use of H2S Donors in Diabetes
The preclinical studies have provided a robust indication that delivery of H2S donors might represent an effective approach for the prevention and treatment of cardiomyopathy and myocardial ischemia/reperfusion injury under diabetes. These studies await further clinical translation. Until May 2021, a total of 15 clinical studies on H2S are found at clinicaltrials.gov after typing H2S in the search box of “Condition or disease” (https://clinicaltrials.gov/). These studies are performed to test the clinical utilities of H2S in various diseases, including chronic kidney disease (ClinicalTrials.gov Identifier: NCT01232257), hypertension (ClinicalTrials.gov Identifier: NCT03179163), heart failure (ClinicalTrials.gov Identifier: NCT01989208), myocardial Infarction (ClinicalTrials.gov Identifier: NCT03829605, NCT02899364), carotid stenosis (ClinicalTrials.gov Identifier: NCT03303534), peripheral arterial disease (ClinicalTrials.gov Identifier: NCT01407172), cardiovascular disease (ClinicalTrials.gov Identifier: NCT02180074). In a clinical trial (ClinicalTrials.gov Identifier: NCT01989208), the authors investigated the effects of H2S donor SG1002 on heart failure, and they found that administration of SG1002 increased H2S levels and circulating NO bioavailability, but attenuated BNP levels in heart failure patients (Polhemus et al., 2015). However, no results were posted regarding the effects of H2S on myocardial infarction, carotid stenosis, peripheral arterial disease, and hypertension. Likewise, the clinical value of H2S in diabetic cardiomyopathy and diabetes-aggravated cardiac ischemia/reperfusion injury might attract increased interest in the scientific community. Nevertheless, to the best of our knowledge, no clinical studies have been implemented to test the therapeutic potential of H2S in patients with diabetic cardiomyopathy and diabetes-aggravated cardiac ischemia/reperfusion injury. We anticipate that more retrospective and comparative clinical trials will be performed to investigate the therapeutic value of H2S in diabetic cardiomyopathy and its progressed heart failure. Of note, more well-designed preclinical or clinical studies are required to assess the safety, effectiveness, pharmacodynamics of H2S-based therapeutics on the broader population with diabetic cardiomyopathy and diabetes-aggravated cardiac ischemia/reperfusion injury. Tremendous efforts aiming to translate those basic research results into the clinical arena are underway by using H2S-releasing compounds.
Conclusion and Perspectives
In this review, we provided the recent advances in our knowledge on the roles of endogenous H2S or pharmacologically administered H2S donors in diabetes-related cardiomyopathy and myocardial ischemia/reperfusion injury. The present review suggests that H2S acts as a cardioprotective mediator in diabetic heart by regulating various signaling pathways (Figure 5). Both endogenously generated H2S and exogenously supplied H2S donors confer cardioprotection in a variety of settings. Thus, H2S might be recommended as a therapeutic agent against diabetic cardiomyopathy and diabetes-aggravated cardiac ischemia/reperfusion injury in pre-clinical studies.
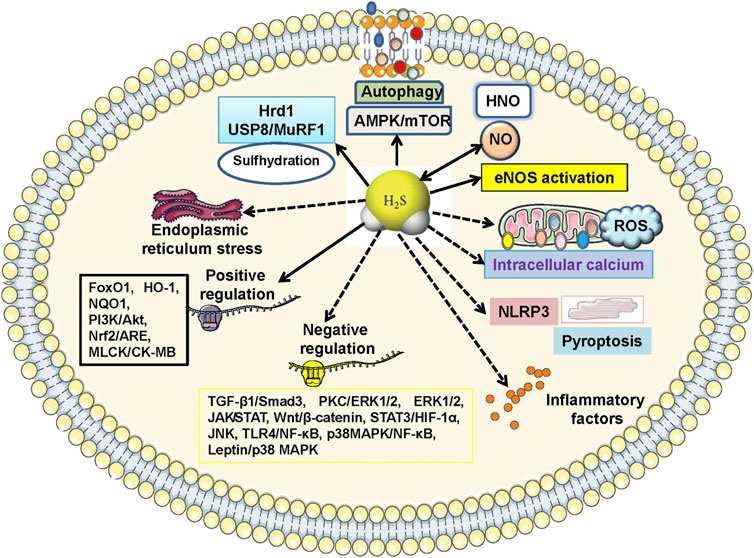
FIGURE 5. Molecular mechanisms of H2S-mediated cardiac benefits in diabetic cardiomyopathy. The cardioprotective actions of H2S on diabetic cardiomyopathy are regulated by multiple signaling cascades. Specifically, 1) inactivation of TGF-β1/Smad3, PKC/ERK1/2, ROS/ERK1/2, JAK/STAT, Wnt pathways, and activation of MLCK/CK-MB, PI3K/AKT, Nrf2/ARE, are responsible for H2S-mediabted suppression on myocardial fibrosis and hypertrophy; 2) blockade of endoplasmic reticulum stress, Wnt/β-catenin, STAT3/HIF-1α, JNK/p38 MAPK, NLRP3, TLR4/NF-κB, p38 MAPK/NF-κB, while stimulation of PI3K/Akt, FoxO1, HO-1, NQO1, contribute to H2S-induced effects against cardiomyocyte apoptosis, oxidative stress and inflammation; 3) regulations of intracellular calcium, leptin/p38 MAPK, NLRP3-mediated pyroptosis, AMPK/mTOR, autophagy, eNOS activation, formation of HNO, are also proposed to H2S-mediated cardiac benefits in diabetic cardiomyopathy. AMPK, adenosine 5′-monophosphate (AMP)-activated protein kinase; ARE, antioxidant response element; CK-MB, creatine kinase MB isozyme; eNOS, endothelial nitric oxide synthase; ERK1/2, extracellular regulated protein kinase 1/2; FoxO1, forkhead box O1; HIF-1α, hypoxia-inducible factor-1α; HNO, nitroxyl; HO-1, haem oxygenase-1; JAK, Janus kinase; JNK, c-Jun N-terminal kinase; MAPK, mitogen-activated protein kinase; MLCK, myosin light chain kinase; mTOR, mammalian target of rapamycin; NLRP3, nucleotide-binding oligomerization domain-like receptor protein 3; NF-κB, nuclear factor kappa-B; NQO1, NAD(P)H:quinone oxidoreductase 1; Nrf2, nuclear factor erythroid 2-related factor 2; PI3K, phosphatidylinositol 3-kinase; PKC, protein kinase C; ROS, reactive oxygen species; Smad3, SMAD family member 3; STAT, signal transducer and activator of transcription; TGF-β1, transforming growth factor β1; TLR4, toll-like receptor 4.
Given that the therapeutic effect of H2S on diabetic cardiomyopathy were mediated by multiple signaling pathways, deciphering into the pros and cons of these cellular events induced by H2S might facilitate the knowledge of pharmacological activities and drug development of H2S. Also, the stable and controllable H2S donors should be designed for clinical management of diabetic cardiomyopathy. Eventually, a combination therapy of H2S donors with other standard medications might be considered as novel strategies to treat diabetic cardiomyopathy or reduce the risk of heart failure in diabetic subjects.
Author Contributions
H-JS, Z-YW, X-WN, and J-SB designed the contents, and conducted initial search of literature, prepared for the figures. H-JS and J-SB drafted the manuscript. J-SB and X-YW critically helped to revise the manuscript. All authors have approved the final manuscript.
Funding
This work was supported by Ministry of Education of Singapore Tier 2 Research grant (No. MOE2017-T2-2-029), the International Cooperative Research Project of Shenzhen Municipal Science and Technology Innovation Council (GJHZ20180416164814621), and China Jiangsu Nature Science Foundation (No. BK20181185).
Conflict of Interest
The authors declare that the research was conducted in the absence of any commercial or financial relationships that could be construed as a potential conflict of interest.
Publisher’s Note
All claims expressed in this article are solely those of the authors and do not necessarily represent those of their affiliated organizations, or those of the publisher, the editors and the reviewers. Any product that may be evaluated in this article, or claim that may be made by its manufacturer, is not guaranteed or endorsed by the publisher.
References
Abe, K., and Kimura, H. (1996). The Possible Role of Hydrogen Sulfide as an Endogenous Neuromodulator. J. Neurosci. 16, 1066–1071. doi:10.1523/jneurosci.16-03-01066.1996
Adeghate, E., and Singh, J. (2014). Structural Changes in the Myocardium during Diabetes-Induced Cardiomyopathy. Heart Fail. Rev. 19, 15–23. doi:10.1007/s10741-013-9388-5
Ahmad, F. U., Sattar, M. A., Rathore, H. A., Tan, Y. C., Akhtar, S., Jin, O. H., et al. (2014). Hydrogen Sulphide and Tempol Treatments Improve the Blood Pressure and Renal Excretory Responses in Spontaneously Hypertensive Rats. Ren. Fail. 36, 598–605. doi:10.3109/0886022X.2014.882218
Al Hroob, A. M., Abukhalil, M. H., Hussein, O. E., and Mahmoud, A. M. (2019). Pathophysiological Mechanisms of Diabetic Cardiomyopathy and the Therapeutic Potential of Epigallocatechin-3-Gallate. Biomed. Pharmacother. 109, 2155–2172. doi:10.1016/j.biopha.2018.11.086
Ali, M. Y., Ping, C. Y., Mok, Y. Y., Ling, L., Whiteman, M., Bhatia, M., et al. (2006). Regulation of Vascular Nitric Oxide In Vitro and In Vivo; a New Role for Endogenous Hydrogen Sulphide?. Br. J. Pharmacol. 149, 625–634. doi:10.1038/sj.bjp.0706906
Al-Magableh, M. R., Kemp-Harper, B. K., and Hart, J. L. (2015). Hydrogen Sulfide Treatment Reduces Blood Pressure and Oxidative Stress in Angiotensin II-Induced Hypertensive Mice. Hypertens. Res. 38, 13–20. doi:10.1038/hr.2014.125
Alyan, A. K., Hanafi, R. S., and Gad, M. Z. (2021). Point-of-care Testing and Optimization of Sample Treatment for Fluorometric Determination of Hydrogen Sulphide in Plasma of Cardiovascular Patients. J. Adv. Res. 27, 1–10. doi:10.1016/j.jare.2019.11.010
Andreadou, I., Iliodromitis, E. K., Rassaf, T., Schulz, R., Papapetropoulos, A., and Ferdinandy, P. (2015). The Role of Gasotransmitters NO, H2S and CO in Myocardial Ischaemia/reperfusion Injury and Cardioprotection by Preconditioning, Postconditioning and Remote Conditioning. Br. J. Pharmacol. 172, 1587–1606. doi:10.1111/bph.12811
Andreadou, I., Schulz, R., Papapetropoulos, A., Turan, B., Ytrehus, K., Ferdinandy, P., et al. (2020). The Role of Mitochondrial Reactive Oxygen Species, NO and H2 S in Ischaemia/reperfusion Injury and Cardioprotection. J. Cel Mol Med 24, 6510–6522. doi:10.1111/jcmm.15279
Ansari, M., and Kurian, G. A. (2019). Hydrogen Sulfide Preconditioning Could Ameliorate Reperfusion Associated Injury in Diabetic Cardiomyopathy Rat Heart through Preservation of Mitochondria. Biochimie 158, 208–216. doi:10.1016/j.biochi.2019.01.011
Ansari, M., and Kurian, G. A. (2020). Mechanism of Hydrogen Sulfide Preconditioning-Associated Protection against Ischemia-Reperfusion Injury Differs in Diabetic Heart that Develops Myopathy. Cardiovasc. Toxicol. 20, 155–167. doi:10.1007/s12012-019-09542-9
Antoniou, C., Savvides, A., Georgiadou, E. C., and Fotopoulos, V. (2018). Spectrophotometric Quantification of Reactive Oxygen, Nitrogen and Sulfur Species in Plant Samples. Methods Mol. Biol. 1694, 155–161. doi:10.1007/978-1-4939-7398-9_16
Ayensa-Vazquez, J. A., Leiva, A., Tauler, P., López-González, A. A., Aguiló, A., Tomás-Salvá, M., et al. (2020). Agreement between Type 2 Diabetes Risk Scales in a Caucasian Population: A Systematic Review and Report. J. Clin. Med. 9, 1546. doi:10.3390/jcm9051546
Badalzadeh, R., Mokhtari, B., and Yavari, R. (2015). Contribution of Apoptosis in Myocardial Reperfusion Injury and Loss of Cardioprotection in Diabetes Mellitus. J. Physiol. Sci. 65, 201–215. doi:10.1007/s12576-015-0365-8
Bai, L., Gao, J., Wei, F., Zhao, J., Wang, D., and Wei, J. (2018). Therapeutic Potential of Ginsenosides as an Adjuvant Treatment for Diabetes. Front. Pharmacol. 9, 423. doi:10.3389/fphar.2018.00423
Barr, L. A., Shimizu, Y., Lambert, J. P., Nicholson, C. K., and Calvert, J. W. (2015). Hydrogen Sulfide Attenuates High Fat Diet-Induced Cardiac Dysfunction via the Suppression of Endoplasmic Reticulum Stress. Nitric Oxide 46, 145–156. doi:10.1016/j.niox.2014.12.013
Beck, K. F., and Pfeilschifter, J. (2020). Gasotransmitter Synthesis and Signalling in the Renal Glomerulus. Implications for Glomerular Diseases. Cell Signal 77, 109823. doi:10.1016/j.cellsig.2020.109823
Behera, J., George, A. K., Voor, M. J., Tyagi, S. C., and Tyagi, N. (2018). Hydrogen Sulfide Epigenetically Mitigates Bone Loss through OPG/RANKL Regulation during Hyperhomocysteinemia in Mice. Bone 114, 90–108. doi:10.1016/j.bone.2018.06.009
Bełtowski, J. (2010). Hypoxia in the Renal Medulla: Implications for Hydrogen Sulfide Signaling. J. Pharmacol. Exp. Ther. 334, 358–363. doi:10.1124/jpet.110.166637
Benavides, G. A., Squadrito, G. L., Mills, R. W., Patel, H. D., Isbell, T. S., Patel, R. P., et al. (2007). Hydrogen Sulfide Mediates the Vasoactivity of Garlic. Proc. Natl. Acad. Sci. U S A. 104, 17977–17982. doi:10.1073/pnas.0705710104
Bergsbaken, T., Fink, S. L., and Cookson, B. T. (2009). Pyroptosis: Host Cell Death and Inflammation. Nat. Rev. Microbiol. 7, 99–109. doi:10.1038/nrmicro2070
Bertoni, A. G., Goff, D. C., D'agostino, R. B., Liu, K., Hundley, W. G., Lima, J. A., et al. (2006). Diabetic Cardiomyopathy and Subclinical Cardiovascular Disease: the Multi-Ethnic Study of Atherosclerosis (MESA). Diabetes Care 29, 588–594. doi:10.2337/diacare.29.03.06.dc05-1501
Bhatia, M., Wong, F. L., Fu, D., Lau, H. Y., Moochhala, S. M., and Moore, P. K. (2005). Role of Hydrogen Sulfide in Acute Pancreatitis and Associated Lung Injury. Faseb j 19, 623–625. doi:10.1096/fj.04-3023fje
Bolton, S. G., Cerda, M. M., Gilbert, A. K., and Pluth, M. D. (2019). Effects of Sulfane Sulfur Content in Benzyl Polysulfides on Thiol-Triggered H2S Release and Cell Proliferation. Free Radic. Biol. Med. 131, 393–398. doi:10.1016/j.freeradbiomed.2018.12.025
Borghetti, G., Von Lewinski, D., Eaton, D. M., Sourij, H., Houser, S. R., and Wallner, M. (2018). Diabetic Cardiomyopathy: Current and Future Therapies. Beyond Glycemic Control. Front. Physiol. 9, 1514. doi:10.3389/fphys.2018.01514
Budoff, M. J., Ahmadi, N., Gul, K. M., Liu, S. T., Flores, F. R., Tiano, J., et al. (2009). Aged Garlic Extract Supplemented with B Vitamins, Folic Acid and L-Arginine Retards the Progression of Subclinical Atherosclerosis: a Randomized Clinical Trial. Prev. Med. 49, 101–107. doi:10.1016/j.ypmed.2009.06.018
Bugger, H., and Abel, E. D. (2014). Molecular Mechanisms of Diabetic Cardiomyopathy. Diabetologia 57, 660–671. doi:10.1007/s00125-014-3171-6
Burguera, E. F., Vela-Anero, Á., Gato-Calvo, L., Vaamonde-García, C., Meijide-Faílde, R., and Blanco, F. J. (2020). Hydrogen Sulfide Biosynthesis Is Impaired in the Osteoarthritic Joint. Int. J. Biometeorol. 64, 997–1010. doi:10.1007/s00484-019-01823-w
Cao, N., Wong, Y. G., Rosli, S., Kiriazis, H., Huynh, K., Qin, C., et al. (2015). Chronic Administration of the Nitroxyl Donor 1-nitrosocyclo Hexyl Acetate Limits Left Ventricular Diastolic Dysfunction in a Mouse Model of Diabetes Mellitus In Vivo. Circ. Heart Fail. 8, 572–581. doi:10.1161/CIRCHEARTFAILURE.114.001699
Cao, X., Ding, L., Xie, Z. Z., Yang, Y., Whiteman, M., Moore, P. K., et al. (2019). A Review of Hydrogen Sulfide Synthesis, Metabolism, and Measurement: Is Modulation of Hydrogen Sulfide a Novel Therapeutic for Cancer?. Antioxid. Redox Signal. 31, 1–38. doi:10.1089/ars.2017.7058
Carneiro-Júnior, M. A., Quintão-Júnior, J. F., Drummond, L. R., Lavorato, V. N., Drummond, F. R., Amadeu, M. A., et al. (2014). Effect of Exercise Training on Ca²⁺ Release Units of Left Ventricular Myocytes of Spontaneously Hypertensive Rats. Braz. J. Med. Biol. Res. 47, 960–965. doi:10.1590/1414-431x20144063
Chandler, M. P., Chavez, P. N., Mcelfresh, T. A., Huang, H., Harmon, C. S., and Stanley, W. C. (2003). Partial Inhibition of Fatty Acid Oxidation Increases Regional Contractile Power and Efficiency during Demand-Induced Ischemia. Cardiovasc. Res. 59, 143–151. doi:10.1016/s0008-6363(03)00327-4
Chang, L., Geng, B., Yu, F., Zhao, J., Jiang, H., Du, J., et al. (2008). Hydrogen Sulfide Inhibits Myocardial Injury Induced by Homocysteine in Rats. Amino Acids 34, 573–585. doi:10.1007/s00726-007-0011-8
Chen, W. L., Niu, Y. Y., Jiang, W. Z., Tang, H. L., Zhang, C., Xia, Q. M., et al. (2015). Neuroprotective Effects of Hydrogen Sulfide and the Underlying Signaling Pathways. Rev. Neurosci. 26, 129–142. doi:10.1515/revneuro-2014-0051
Chen, Y., Jin, S., Teng, X., Hu, Z., Zhang, Z., Qiu, X., et al. (2018). Hydrogen Sulfide Attenuates LPS-Induced Acute Kidney Injury by Inhibiting Inflammation and Oxidative Stress. Oxid Med. Cel Longev. 2018, 6717212. doi:10.1155/2018/6717212
Chen, Y., Zhang, F., Yin, J., Wu, S., and Zhou, X. (2020). Protective Mechanisms of Hydrogen Sulfide in Myocardial Ischemia. J. Cel Physiol 235, 9059–9070. doi:10.1002/jcp.29761
Cheng, Y. S., Tang, Y. Q., Dai, D. Z., and Dai, Y. (2012). AQP4 Knockout Mice Manifest Abnormal Expressions of Calcium Handling Proteins Possibly Due to Exacerbating Pro-inflammatory Factors in the Heart. Biochem. Pharmacol. 83, 97–105. doi:10.1016/j.bcp.2011.10.006
Cheng, Y. S., Dai, D. Z., Dai, Y., Zhu, D. D., and Liu, B. C. (2016). Exogenous Hydrogen Sulphide Ameliorates Diabetic Cardiomyopathy in Rats by Reversing Disordered Calcium-Handling System in Sarcoplasmic Reticulum. J. Pharm. Pharmacol. 68, 379–388. doi:10.1111/jphp.12517
Chi, Z., Byeon, H. E., Seo, E., Nguyen, Q. T., Lee, W., Jeong, Y., et al. (2019). Histone Deacetylase 6 Inhibitor Tubastatin A Attenuates Angiotensin II-Induced Hypertension by Preventing Cystathionine γ-lyase Protein Degradation. Pharmacol. Res. 146, 104281. doi:10.1016/j.phrs.2019.104281
Chin, K. Y., Qin, C., May, L., Ritchie, R. H., and Woodman, O. L. (2017). New Pharmacological Approaches to the Prevention of Myocardial Ischemia- Reperfusion Injury. Curr. Drug Targets 18, 1689–1711. doi:10.2174/1389450116666151001112020
Cooper, C. E., and Brown, G. C. (2008). The Inhibition of Mitochondrial Cytochrome Oxidase by the Gases Carbon Monoxide, Nitric Oxide, Hydrogen Cyanide and Hydrogen Sulfide: Chemical Mechanism and Physiological Significance. J. Bioenerg. Biomembr. 40, 533–539. doi:10.1007/s10863-008-9166-6
Coort, S. L., Hasselbaink, D. M., Koonen, D. P., Willems, J., Coumans, W. A., Chabowski, A., et al. (2004). Enhanced Sarcolemmal FAT/CD36 Content and Triacylglycerol Storage in Cardiac Myocytes from Obese Zucker Rats. Diabetes 53, 1655–1663. doi:10.2337/diabetes.53.7.1655
Cortese-Krott, M. M., Fernandez, B. O., Santos, J. L., Mergia, E., Grman, M., Nagy, P., et al. (2014). Nitrosopersulfide (SSNO(-)) Accounts for Sustained NO Bioactivity of S-Nitrosothiols Following Reaction with Sulfide. Redox Biol. 2, 234–244. doi:10.1016/j.redox.2013.12.031
Das, A., Durrant, D., Salloum, F. N., Xi, L., and Kukreja, R. C. (2015). PDE5 Inhibitors as Therapeutics for Heart Disease, Diabetes and Cancer. Pharmacol. Ther. 147, 12–21. doi:10.1016/j.pharmthera.2014.10.003
De Witt, B. J., Marrone, J. R., Kaye, A. D., Keefer, L. K., and Kadowitz, P. J. (2001). Comparison of Responses to Novel Nitric Oxide Donors in the Feline Pulmonary Vascular Bed. Eur. J. Pharmacol. 430, 311–315. doi:10.1016/s0014-2999(01)01289-4
DeLeon, E. R., Stoy, G. F., and Olson, K. R. (2012). Passive Loss of Hydrogen Sulfide in Biological Experiments. Anal. Biochem. 421, 203–207. doi:10.1016/j.ab.2011.10.016
Devereux, R. B., Roman, M. J., Paranicas, M., O'grady, M. J., Lee, E. T., Welty, T. K., et al. (2000). Impact of Diabetes on Cardiac Structure and Function: the strong Heart Study. Circulation 101, 2271–2276. doi:10.1161/01.cir.101.19.2271
Dhalla, N. S., Shah, A. K., and Tappia, P. S. (2020). Role of Oxidative Stress in Metabolic and Subcellular Abnormalities in Diabetic Cardiomyopathy. Int. J. Mol. Sci. 21, 2413. doi:10.3390/ijms21072413
Doeller, J. E., Isbell, T. S., Benavides, G., Koenitzer, J., Patel, H., Patel, R. P., et al. (2005). Polarographic Measurement of Hydrogen Sulfide Production and Consumption by Mammalian Tissues. Anal. Biochem. 341, 40–51. doi:10.1016/j.ab.2005.03.024
Dombkowski, R. A., Russell, M. J., and Olson, K. R. (2004). Hydrogen Sulfide as an Endogenous Regulator of Vascular Smooth Muscle Tone in trout. Am. J. Physiol. Regul. Integr. Comp. Physiol. 286, R678–R685. doi:10.1152/ajpregu.00419.2003
Donnarumma, E., Trivedi, R. K., and Lefer, D. J. (2017). Protective Actions of H2S in Acute Myocardial Infarction and Heart Failure. Compr. Physiol. 7, 583–602. doi:10.1002/cphy.c160023
Dorofeyeva, N., Drachuk, K., Rajkumar, R., Sabnis, O., and Sagach, V. (2020a). H(2) S Donor Improves Heart Function and Vascular Relaxation in Diabetes. Eur. J. Clin. Invest. 51, e13354. doi:10.1111/eci.13354
Dorofeyeva, N. A., Korkach, I. P., Kutsyk, O. E., and Sagach, V. F. (2021). Modulation of Hydrogen Sulfide Synthesis Improves Heart Function and Endothelium-dependent Vasorelaxation in Diabetes. Can. J. Physiol. Pharmacol. 99, 549–555. doi:10.1139/cjpp-2020-0302
Doroudgar, S., Völkers, M., Thuerauf, D. J., Khan, M., Mohsin, S., Respress, J. L., et al. (2015). Hrd1 and ER-Associated Protein Degradation, ERAD, Are Critical Elements of the Adaptive ER Stress Response in Cardiac Myocytes. Circ. Res. 117, 536–546. doi:10.1161/CIRCRESAHA.115.306993
El-Mas, M. M., Omar, A. G., Helmy, M. M., and Mohy El-Din, M. M. (2012). Crosstalk between central Pathways of Nitric Oxide and Carbon Monoxide in the Hypertensive Action of Cyclosporine. Neuropharmacology 62, 1890–1896. doi:10.1016/j.neuropharm.2011.12.017
El-Sayed, S. S., Zakaria, M. N., Abdel-Ghany, R. H., and Abdel-Rahman, A. A. (2016). Cystathionine-γ Lyase-Derived Hydrogen Sulfide Mediates the Cardiovascular Protective Effects of Moxonidine in Diabetic Rats. Eur. J. Pharmacol. 783, 73–84. doi:10.1016/j.ejphar.2016.04.054
Elsey, D. J., Fowkes, R. C., and Baxter, G. F. (2010). Regulation of Cardiovascular Cell Function by Hydrogen Sulfide (H(2)S). Cell Biochem Funct 28, 95–106. doi:10.1002/cbf.1618
Ernande, L., Bergerot, C., Rietzschel, E. R., De Buyzere, M. L., Thibault, H., Pignonblanc, P. G., et al. (2011). Diastolic Dysfunction in Patients with Type 2 Diabetes Mellitus: Is it Really the First Marker of Diabetic Cardiomyopathy?. J. Am. Soc. Echocardiogr 24, 1268–1275.e1. doi:10.1016/j.echo.2011.07.017
Feng, X., Guo, Q., Xue, H., Duan, X., Jin, S., and Wu, Y. (2020). Hydrogen Sulfide Attenuated Angiotensin II-Induced Sympathetic Excitation in Offspring of Renovascular Hypertensive Rats. Front. Pharmacol. 11, 565726. doi:10.3389/fphar.2020.565726
Feuvray, D., and Lopaschuk, G. D. (1997). Controversies on the Sensitivity of the Diabetic Heart to Ischemic Injury: the Sensitivity of the Diabetic Heart to Ischemic Injury Is Decreased. Cardiovasc. Res. 34, 113–120. doi:10.1016/s0008-6363(97)00037-0
Feuvray, D., Idell-Wenger, J. A., and Neely, J. R. (1979). Effects of Ischemia on Rat Myocardial Function and Metabolism in Diabetes. Circ. Res. 44, 322–329. doi:10.1161/01.res.44.3.322
Fonseca, A. J., Cabrita, A. R., Pinho, L. A., Kim, E. J., and Dewhurst, R. J. (2013). Effects of Protein Sources on Concentrations of Hydrogen Sulphide in the Rumen Headspace Gas of Dairy Cows. Animal 7, 75–81. doi:10.1017/S1751731112000973
Friedman, J. M., and Halaas, J. L. (1998). Leptin and the Regulation of Body Weight in Mammals. Nature 395, 763–770. doi:10.1038/27376
Furne, J., Saeed, A., and Levitt, M. D. (2008). Whole Tissue Hydrogen Sulfide Concentrations Are Orders of Magnitude Lower Than Presently Accepted Values. Am. J. Physiol. Regul. Integr. Comp. Physiol. 295, R1479–R1485. doi:10.1152/ajpregu.90566.2008
Gao, L., Xu, Z., Yin, Z., Chen, K., Wang, C., and Zhang, H. (2015). Association of Hydrogen Sulfide with Alterations of Monocyte Chemokine Receptors, CCR2 and CX3CR1 in Patients with Coronary Artery Disease. Inflamm. Res. 64, 627–635. doi:10.1007/s00011-015-0844-7
Geng, B., Yang, J., Qi, Y., Zhao, J., Pang, Y., Du, J., et al. (2004). H2S Generated by Heart in Rat and its Effects on Cardiac Function. Biochem. Biophys. Res. Commun. 313, 362–368. doi:10.1016/j.bbrc.2003.11.130
Goodwin, L. R., Francom, D., Dieken, F. P., Taylor, J. D., Warenycia, M. W., Reiffenstein, R. J., et al. (1989). Determination of Sulfide in Brain Tissue by Gas Dialysis/ion Chromatography: Postmortem Studies and Two Case Reports. J. Anal. Toxicol. 13, 105–109. doi:10.1093/jat/13.2.105
Gorini, F., Bustaffa, E., Chatzianagnostou, K., Bianchi, F., and Vassalle, C. (2020). Hydrogen Sulfide and Cardiovascular Disease: Doubts, Clues, and Interpretation Difficulties from Studies in Geothermal Areas. Sci. Total Environ. 743, 140818. doi:10.1016/j.scitotenv.2020.140818
Gu, W., Pagel, P. S., Warltier, D. C., and Kersten, J. R. (2003). Modifying Cardiovascular Risk in Diabetes Mellitus. Anesthesiology 98, 774–779. doi:10.1097/00000542-200303000-00029
Guo, R., Lin, J., Xu, W., Shen, N., Mo, L., Zhang, C., et al. (2013). Hydrogen Sulfide Attenuates Doxorubicin-Induced Cardiotoxicity by Inhibition of the P38 MAPK Pathway in H9c2 Cells. Int. J. Mol. Med. 31, 644–650. doi:10.3892/ijmm.2013.1246
Guo, Q., Feng, X., Xue, H., Teng, X., Jin, S., Duan, X., et al. (2017a). Maternal Renovascular Hypertensive Rats Treatment with Hydrogen Sulfide Increased the Methylation of AT1b Gene in Offspring. Am. J. Hypertens. 30, 1220–1227. doi:10.1093/ajh/hpx124
Guo, R., Wu, Z., Jiang, J., Liu, C., Wu, B., Li, X., et al. (2017b). New Mechanism of Lipotoxicity in Diabetic Cardiomyopathy: Deficiency of Endogenous H2S Production and ER Stress. Mech. Ageing Dev. 162, 46–52. doi:10.1016/j.mad.2016.11.005
Gwilt, D. J., Petri, M., Lewis, P. W., Nattrass, M., and Pentecost, B. L. (1985). Myocardial Infarct Size and Mortality in Diabetic Patients. Br. Heart J. 54, 466–472. doi:10.1136/hrt.54.5.466
Hackfort, B. T., and Mishra, P. K. (2016). Emerging Role of Hydrogen Sulfide-microRNA Crosstalk in Cardiovascular Diseases. Am. J. Physiol. Heart Circ. Physiol. 310, H802–H812. doi:10.1152/ajpheart.00660.2015
Haglund, K., and Dikic, I. (2012). The Role of Ubiquitylation in Receptor Endocytosis and Endosomal Sorting. J. Cel Sci 125, 265–275. doi:10.1242/jcs.091280
Han, L., Wu, G., Feng, C., Yang, Y., Li, B., Ge, Y., et al. (2020). Dietary Methionine Restriction Improves the Impairment of Cardiac Function in Middle-Aged Obese Mice. Food Funct. 11, 1764–1778. doi:10.1039/c9fo02819f
Haouzi, P., and Klingerman, C. M. (2013). Fate of Intracellular H2S/HS- and Metallo-Proteins. Respir. Physiol. Neurobiol. 188, 229–230. doi:10.1016/j.resp.2013.05.029
Haouzi, P., Sonobe, T., Torsell-Tubbs, N., Prokopczyk, B., Chenuel, B., and Klingerman, C. M. (2014). In Vivo interactions between Cobalt or Ferric Compounds and the Pools of Sulphide in the Blood during and after H2S Poisoning. Toxicol. Sci. 141, 493–504. doi:10.1093/toxsci/kfu140
Haouzi, P. (2016). Is Exogenous Hydrogen Sulfide a Relevant Tool to Address Physiological Questions on Hydrogen Sulfide?. Respir. Physiol. Neurobiol. 229, 5–10. doi:10.1016/j.resp.2016.03.015
Hart, P. D., and Bakris, G. L. (2010). Hypertensive Nephropathy: Prevention and Treatment Recommendations. Expert Opin. Pharmacother. 11, 2675–2686. doi:10.1517/14656566.2010.485612
Hartle, M. D., and Pluth, M. D. (2016). A Practical Guide to Working with H2S at the Interface of Chemistry and Biology. Chem. Soc. Rev. 45, 6108–6117. doi:10.1039/c6cs00212a
Haye, A., Ansari, M. A., Rahman, S. O., Shamsi, Y., Ahmed, D., and Sharma, M. (2020). Role of AMP-Activated Protein Kinase on Cardio-Metabolic Abnormalities in the Development of Diabetic Cardiomyopathy: A Molecular Landscape. Eur. J. Pharmacol. 888, 173376. doi:10.1016/j.ejphar.2020.173376
He, X. L., Yan, N., Zhang, H., Qi, Y. W., Zhu, L. J., Liu, M. J., et al. (2014). Hydrogen Sulfide Improves Spatial Memory Impairment and Decreases Production of Aβ in APP/PS1 Transgenic Mice. Neurochem. Int. 67, 1–8. doi:10.1016/j.neuint.2014.01.004
Hearse, D. J., Stewart, D. A., and Chain, E. B. (1975). Diabetes and the Survival and Recovery of the Anoxic Myocardium. J. Mol. Cel Cardiol 7, 397–415. doi:10.1016/0022-2828(75)90046-2
Holwerda, K. M., Burke, S. D., Faas, M. M., Zsengeller, Z., Stillman, I. E., Kang, P. M., et al. (2014). Hydrogen Sulfide Attenuates sFlt1-Induced Hypertension and Renal Damage by Upregulating Vascular Endothelial Growth Factor. J. Am. Soc. Nephrol. 25, 717–725. doi:10.1681/ASN.2013030291
Hrabie, J. A., and Keefer, L. K. (2002). Chemistry of the Nitric Oxide-Releasing Diazeniumdiolate ("Nitrosohydroxylamine") Functional Group and its Oxygen-Substituted Derivatives. Chem. Rev. 102, 1135–1154. doi:10.1021/cr000028t
Hsu, C. N., and Tain, Y. L. (2018). Hydrogen Sulfide in Hypertension and Kidney Disease of Developmental Origins. Int. J. Mol. Sci. 19, 1438. doi:10.3390/ijms19051438
Huang, S., Li, H., and Ge, J. (2015). A Cardioprotective Insight of the Cystathionine γ-lyase/hydrogen Sulfide Pathway. Int. J. Cardiol. Heart Vasc. 7, 51–57. doi:10.1016/j.ijcha.2015.01.010
Huang, Z., Dong, X., Zhuang, X., Hu, X., Wang, L., and Liao, X. (2016a). Exogenous Hydrogen Sulfide Protects against High Glucose-Induced Inflammation and Cytotoxicity in H9c2 Cardiac Cells. Mol. Med. Rep. 14, 4911–4917. doi:10.3892/mmr.2016.5846
Huang, Z., Zhuang, X., Xie, C., Hu, X., Dong, X., Guo, Y., et al. (2016b). Exogenous Hydrogen Sulfide Attenuates High Glucose-Induced Cardiotoxicity by Inhibiting NLRP3 Inflammasome Activation by Suppressing TLR4/NF-κB Pathway in H9c2 Cells. Cell Physiol Biochem 40, 1578–1590. doi:10.1159/000453208
Ibrahim, H., Serag, A., and Farag, M. A. (2021). Emerging Analytical Tools for the Detection of the Third Gasotransmitter H2S, a Comprehensive Review. J. Adv. Res. 27, 137–153. doi:10.1016/j.jare.2020.05.018
Insko, M. A., Deckwerth, T. L., Hill, P., Toombs, C. F., and Szabo, C. (2009). Detection of Exhaled Hydrogen Sulphide Gas in Rats Exposed to Intravenous Sodium Sulphide. Br. J. Pharmacol. 157, 944–951. doi:10.1111/j.1476-5381.2009.00248.x
International Hypoglycaemia Study Group (2019). Hypoglycaemia, Cardiovascular Disease, and Mortality in Diabetes: Epidemiology, Pathogenesis, and Management. Lancet Diabetes Endocrinol. 7, 385–396. doi:10.1016/S2213-8587(18)30315-2
Irvine, J. C., Ritchie, R. H., Favaloro, J. L., Andrews, K. L., Widdop, R. E., and Kemp-Harper, B. K. (2008). Nitroxyl (HNO): the Cinderella of the Nitric Oxide story. Trends Pharmacol. Sci. 29, 601–608. doi:10.1016/j.tips.2008.08.005
Jaffe, A. S., Spadaro, J. J., Schechtman, K., Roberts, R., Geltman, E. M., and Sobel, B. E. (1984). Increased Congestive Heart Failure after Myocardial Infarction of Modest Extent in Patients with Diabetes Mellitus. Am. Heart J. 108, 31–37. doi:10.1016/0002-8703(84)90541-6
Jeddi, S., Gheibi, S., Kashfi, K., Carlström, M., and Ghasemi, A. (2020). Protective Effect of Intermediate Doses of Hydrogen Sulfide against Myocardial Ischemia-Reperfusion Injury in Obese Type 2 Diabetic Rats. Life Sci. 256, 117855. doi:10.1016/j.lfs.2020.117855
Ji, W., Liu, S., Dai, J., Yang, T., Jiang, X., Duan, X., et al. (2014). Hydrogen Sulfide Defends against the Cardiovascular Risk of Nw-nitro-L-argininemethyl Ester-Induced Hypertension in Rats via the Nitric Oxide/endothelial Nitric Oxide Synthase Pathway. Chin. Med. J. (Engl) 127, 3751–3757.
Jia, G., Hill, M. A., and Sowers, J. R. (2018a). Diabetic Cardiomyopathy: An Update of Mechanisms Contributing to This Clinical Entity. Circ. Res. 122, 624–638. doi:10.1161/CIRCRESAHA.117.311586
Jia, G., Whaley-Connell, A., and Sowers, J. R. (2018b). Diabetic Cardiomyopathy: a Hyperglycaemia- and Insulin-Resistance-Induced Heart Disease. Diabetologia 61, 21–28. doi:10.1007/s00125-017-4390-4
Jia, Q., Mehmood, S., Liu, X., Ma, S., and Yang, R. (2020). Hydrogen Sulfide Mitigates Myocardial Inflammation by Inhibiting Nucleotide-Binding Oligomerization Domain-like Receptor Protein 3 Inflammasome Activation in Diabetic Rats. Exp. Biol. Med. (Maywood) 245, 221–230. doi:10.1177/1535370219899899
Jiang, H. L., Wu, H. C., Li, Z. L., Geng, B., and Tang, C. S. (2005). Changes of the New Gaseous Transmitter H2S in Patients with Coronary Heart Disease. Di Yi Jun Yi Da Xue Xue Bao 25, 951–954. doi:10.3321/j.issn:1673-4254.2005.08.007
Jin, S., Pu, S. X., Hou, C. L., Ma, F. F., Li, N., Li, X. H., et al. (2015). Cardiac H2S Generation Is Reduced in Ageing Diabetic Mice. Oxid Med. Cel Longev 2015, 758358. doi:10.1155/2015/758358
Jin, S., Teng, X., Xiao, L., Xue, H., Guo, Q., Duan, X., et al. (2017). Hydrogen Sulfide Ameliorated L-NAME-Induced Hypertensive Heart Disease by the Akt/eNOS/NO Pathway. Exp. Biol. Med. (Maywood) 242, 1831–1841. doi:10.1177/1535370217732325
Jin, X., Chen, D., Wu, F., Zhang, L., Huang, Y., Lin, Z., et al. (2020). Hydrogen Sulfide Protects against Ammonia-Induced Neurotoxicity through Activation of Nrf2/ARE Signaling in Astrocytic Model of Hepatic Encephalopathy. Front Cel Neurosci. 14, 573422. doi:10.3389/fncel.2020.573422
Judenherc-Haouzi, A., Zhang, X. Q., Sonobe, T., Song, J., Rannals, M. D., Wang, J., et al. (2016). Methylene Blue Counteracts H2S Toxicity-Induced Cardiac Depression by Restoring L-type Ca Channel Activity. Am. J. Physiol. Regul. Integr. Comp. Physiol. 310, R1030–R1044. doi:10.1152/ajpregu.00527.2015
Kaludercic, N., and Di Lisa, F. (2020). Mitochondrial ROS Formation in the Pathogenesis of Diabetic Cardiomyopathy. Front. Cardiovasc. Med. 7, 12. doi:10.3389/fcvm.2020.00012
Kanagy, N. L., Szabo, C., and Papapetropoulos, A. (2017). Vascular Biology of Hydrogen Sulfide. Am. J. Physiol. Cel Physiol 312, C537–c549. doi:10.1152/ajpcell.00329.2016
Kang, S. C., Sohn, E. H., and Lee, S. R. (2020). Hydrogen Sulfide as a Potential Alternative for the Treatment of Myocardial Fibrosis. Oxid Med. Cel Longev 2020, 4105382. doi:10.1155/2020/4105382
Kar, S., Kambis, T. N., and Mishra, P. K. (2019a). Hydrogen Sulfide-Mediated Regulation of Cell Death Signaling Ameliorates Adverse Cardiac Remodeling and Diabetic Cardiomyopathy. Am. J. Physiol. Heart Circ. Physiol. 316, H1237–h1252. doi:10.1152/ajpheart.00004.2019
Kar, S., Shahshahan, H. R., Hackfort, B. T., Yadav, S. K., Yadav, R., Kambis, T. N., et al. (2019b). Exercise Training Promotes Cardiac Hydrogen Sulfide Biosynthesis and Mitigates Pyroptosis to Prevent High-Fat Diet-Induced Diabetic Cardiomyopathy. Antioxidants (Basel) 8, 638. doi:10.3390/antiox8120638
Karunya, R., Jayaprakash, K. S., Gaikwad, R., Sajeesh, P., Ramshad, K., Muraleedharan, K. M., et al. (2019). Rapid Measurement of Hydrogen Sulphide in Human Blood Plasma Using a Microfluidic Method. Sci. Rep. 9, 3258. doi:10.1038/s41598-019-39389-7
Kasamatsu, S., Kakihana, Y., Koga, T., Yoshioka, H., and Ihara, H. (2020). Generation of Rat Monoclonal Antibody to Detect Hydrogen Sulfide and Polysulfides in Biological Samples. Antioxidants (Basel) 9, 1160. doi:10.3390/antiox9111160
Kesherwani, V., Shahshahan, H. R., and Mishra, P. K. (2017). Cardiac Transcriptome Profiling of Diabetic Akita Mice Using Microarray and Next Generation Sequencing. PLoS One 12, e0182828. doi:10.1371/journal.pone.0182828
Kimura, H. (2012). Metabolic Turnover of Hydrogen Sulfide. Front. Physiol. 3, 101. doi:10.3389/fphys.2012.00101
Kimura, H. (2020). Hydrogen Sulfide Signalling in the CNS - Comparison with NO. Br. J. Pharmacol. 177, 5031–5045. doi:10.1111/bph.15246
King, A. L., and Lefer, D. J. (2011). Cytoprotective Actions of Hydrogen Sulfide in Ischaemia-Reperfusion Injury. Exp. Physiol. 96, 840–846. doi:10.1113/expphysiol.2011.059725
King, A. L., Polhemus, D. J., Bhushan, S., Otsuka, H., Kondo, K., Nicholson, C. K., et al. (2014). Hydrogen Sulfide Cytoprotective Signaling Is Endothelial Nitric Oxide Synthase-Nitric Oxide Dependent. Proc. Natl. Acad. Sci. U S A. 111, 3182–3187. doi:10.1073/pnas.1321871111
Klingerman, C. M., Trushin, N., Prokopczyk, B., and Haouzi, P. (2013). H2S Concentrations in the Arterial Blood during H2S Administration in Relation to its Toxicity and Effects on Breathing. Am. J. Physiol. Regul. Integr. Comp. Physiol. 305, R630–R638. doi:10.1152/ajpregu.00218.2013
Kolluru, G. K., Shen, X., Bir, S. C., and Kevil, C. G. (2013). Hydrogen Sulfide Chemical Biology: Pathophysiological Roles and Detection. Nitric Oxide 35, 5–20. doi:10.1016/j.niox.2013.07.002
Komici, K., Femminella, G. D., De Lucia, C., Cannavo, A., Bencivenga, L., Corbi, G., et al. (2019). Predisposing Factors to Heart Failure in Diabetic Nephropathy: a Look at the Sympathetic Nervous System Hyperactivity. Aging Clin. Exp. Res. 31, 321–330. doi:10.1007/s40520-018-0973-2
Kondo, K., Bhushan, S., King, A. L., Prabhu, S. D., Hamid, T., Koenig, S., et al. (2013). H₂S Protects against Pressure Overload-Induced Heart Failure via Upregulation of Endothelial Nitric Oxide Synthase. Circulation 127, 1116–1127. doi:10.1161/CIRCULATIONAHA.112.000855
Koning, A. M., Frenay, A. R., Leuvenink, H. G., and Van Goor, H. (2015). Hydrogen Sulfide in Renal Physiology, Disease and Transplantation-Tthe Smell of Renal protection. Nitric Oxide 46, 37–49. doi:10.1016/j.niox.2015.01.005
Koning, A. M., Meijers, W. C., Minović, I., Post, A., Feelisch, M., Pasch, A., et al. (2017). The Fate of Sulfate in Chronic Heart Failure. Am. J. Physiol. Heart Circ. Physiol. 312, H415–h421. doi:10.1152/ajpheart.00645.2016
Lambert, J. P., Nicholson, C. K., Amin, H., Amin, S., and Calvert, J. W. (2014). Hydrogen Sulfide Provides Cardioprotection against Myocardial/ischemia Reperfusion Injury in the Diabetic State through the Activation of the RISK Pathway. Med. Gas Res. 4, 20. doi:10.1186/s13618-014-0020-0
Lavandero, S., Chiong, M., Rothermel, B. A., and Hill, J. A. (2015). Autophagy in Cardiovascular Biology. J. Clin. Invest. 125, 55–64. doi:10.1172/JCI73943
Lee, C. Y., and Yen, M. H. (2009). Nitric Oxide and Carbon Monoxide, Collaborative and Competitive Regulators of Hypertension. Chang Gung Med. J. 32, 12–21.
Lejay, A., Fang, F., John, R., Van, J. A., Barr, M., Thaveau, F., et al. (2016). Ischemia Reperfusion Injury, Ischemic Conditioning and Diabetes Mellitus. J. Mol. Cel Cardiol 91, 11–22. doi:10.1016/j.yjmcc.2015.12.020
Leschelle, X., Goubern, M., Andriamihaja, M., Blottière, H. M., Couplan, E., Gonzalez-Barroso, M. D., et al. (2005). Adaptative Metabolic Response of Human Colonic Epithelial Cells to the Adverse Effects of the Luminal Compound Sulfide. Biochim. Biophys. Acta 1725, 201–212. doi:10.1016/j.bbagen.2005.06.002
Li, Q., and Lancaster, J. R. (2013). Chemical Foundations of Hydrogen Sulfide Biology. Nitric Oxide 35, 21–34. doi:10.1016/j.niox.2013.07.001
Li, H. W., and Xiao, F. Y. (2020). Effect of Hydrogen Sulfide on Cardiomyocyte Apoptosis in Rats with Myocardial Ischemia-Reperfusion Injury via the JNK Signaling Pathway. Eur. Rev. Med. Pharmacol. Sci. 24, 2054–2061. doi:10.26355/eurrev_202002_20383
Li, L., Bhatia, M., Zhu, Y. Z., Zhu, Y. C., Ramnath, R. D., Wang, Z. J., et al. (2005). Hydrogen Sulfide Is a Novel Mediator of Lipopolysaccharide-Induced Inflammation in the Mouse. Faseb j 19, 1196–1198. doi:10.1096/fj.04-3583fje
Li, L., Hsu, A., and Moore, P. K. (2009). Actions and Interactions of Nitric Oxide, Carbon Monoxide and Hydrogen Sulphide in the Cardiovascular System and in Inflammation-Aa Tale of Three Gases!. Pharmacol. Ther. 123, 386–400. doi:10.1016/j.pharmthera.2009.05.005
Li, J., Peng, L., Du, H., Wang, Y., Lu, B., Xu, Y., et al. (2014). The Protective Effect of Beraprost Sodium on Diabetic Cardiomyopathy through the Inhibition of the P38 MAPK Signaling Pathway in High-Fat-Induced SD Rats. Int. J. Endocrinol. 2014, 901437. doi:10.1155/2014/901437
Li, F., Luo, J., Wu, Z., Xiao, T., Zeng, O., Li, L., et al. (2016). Hydrogen Sulfide Exhibits Cardioprotective Effects by Decreasing Endoplasmic Reticulum Stress in a Diabetic Cardiomyopathy Rat Model. Mol. Med. Rep. 14, 865–873. doi:10.3892/mmr.2016.5289
Li, H., Mani, S., Wu, L., Fu, M., Shuang, T., Xu, C., et al. (2017). The Interaction of Estrogen and CSE/H2S Pathway in the Development of Atherosclerosis. Am. J. Physiol. Heart Circ. Physiol. 312, H406–h414. doi:10.1152/ajpheart.00245.2016
Li, Z., Polhemus, D. J., and Lefer, D. J. (2018). Evolution of Hydrogen Sulfide Therapeutics to Treat Cardiovascular Disease. Circ. Res. 123, 590–600. doi:10.1161/CIRCRESAHA.118.311134
Li, J., Teng, X., Jin, S., Dong, J., Guo, Q., Tian, D., et al. (2019a). Hydrogen Sulfide Improves Endothelial Dysfunction by Inhibiting the Vicious Cycle of NLRP3 Inflammasome and Oxidative Stress in Spontaneously Hypertensive Rats. J. Hypertens. 37, 1633–1643. doi:10.1097/HJH.0000000000002101
Li, J., Yuan, Y. Q., Zhang, L., Zhang, H., Zhang, S. W., Zhang, Y., et al. (2019b). Exogenous Hydrogen Sulfide Protects against High Glucose-Induced Apoptosis and Oxidative Stress by Inhibiting the STAT3/HIF-1α Pathway in H9c2 Cardiomyocytes. Exp. Ther. Med. 18, 3948–3958. doi:10.3892/etm.2019.8036
Liao, L. Z., Chen, Y. L., Lu, L. H., Zhao, Y. H., Guo, H. L., and Wu, W. K. (2013). Polysaccharide from Fuzi Likely Protects against Starvation-Induced Cytotoxicity in H9c2 Cells by Increasing Autophagy through Activation of the AMPK/mTOR Pathway. Am. J. Chin. Med. 41, 353–367. doi:10.1142/S0192415X13500262
Liu, Y. H., Yan, C. D., and Bian, J. S. (2011). Hydrogen Sulfide: a Novel Signaling Molecule in the Vascular System. J. Cardiovasc. Pharmacol. 58, 560–569. doi:10.1097/FJC.0b013e31820eb7a1
Liu, Y. H., Lu, M., Hu, L. F., Wong, P. T., Webb, G. D., and Bian, J. S. (2012). Hydrogen Sulfide in the Mammalian Cardiovascular System. Antioxid. Redox Signal. 17, 141–185. doi:10.1089/ars.2011.4005
Liu, M., Li, Y., Liang, B., Li, Z., Jiang, Z., Chu, C., et al. (2018). Hydrogen Sulfide Attenuates Myocardial Fibrosis in Diabetic Rats through the JAK/STAT Signaling Pathway. Int. J. Mol. Med. 41, 1867–1876. doi:10.3892/ijmm.2018.3419
Long, J., Liu, M., Liu, S., Tang, F., Tan, W., Xiao, T., et al. (2019). H2S Attenuates the Myocardial Fibrosis in Diabetic Rats through Modulating PKC-ERK1/2MAPK Signaling Pathway. Technol. Health Care 27, 307–316. doi:10.3233/THC-199029
Lu, M., Liu, Y. H., Goh, H. S., Wang, J. J., Yong, Q. C., Wang, R., et al. (2010). Hydrogen Sulfide Inhibits Plasma Renin Activity. J. Am. Soc. Nephrol. 21, 993–1002. doi:10.1681/ASN.2009090949
Lundbaek, K. (1954). Diabetic Angiopathy: a Specific Vascular Disease. Lancet 266, 377–379. doi:10.1016/s0140-6736(54)90924-1
Luo, B., Li, B., Wang, W., Liu, X., Xia, Y., Zhang, C., et al. (2014). NLRP3 Gene Silencing Ameliorates Diabetic Cardiomyopathy in a Type 2 Diabetes Rat Model. PLoS One 9, e104771. doi:10.1371/journal.pone.0104771
Luo, B., Huang, F., Liu, Y., Liang, Y., Wei, Z., Ke, H., et al. (2017). NLRP3 Inflammasome as a Molecular Marker in Diabetic Cardiomyopathy. Front. Physiol. 8, 519. doi:10.3389/fphys.2017.00519
Lv, S., Wu, N., Wang, Q., and Yang, L. H. (2020). Endogenous Hydrogen Sulfide Alleviates Methotrexate-Induced Cognitive Impairment by Attenuating Endoplasmic Reticulum Stress-Induced Apoptosis via CHOP and Caspase-12. Fundam. Clin. Pharmacol. 34, 559–570. doi:10.1111/fcp.12543
Ma, N., Liu, H. M., Xia, T., Liu, J. D., and Wang, X. Z. (2018). Chronic Aerobic Exercise Training Alleviates Myocardial Fibrosis in Aged Rats through Restoring Bioavailability of Hydrogen Sulfide. Can. J. Physiol. Pharmacol. 96, 902–908. doi:10.1139/cjpp-2018-0153
Ma, J., Shi, C., Liu, Z., Han, B., Guo, L., Zhu, L., et al. (2019). Hydrogen Sulfide Is a Novel Regulator Implicated in Glucocorticoids-Inhibited Bone Formation. Aging (Albany NY) 11, 7537–7552. doi:10.18632/aging.102269
Magierowski, M., Magierowska, K., Hubalewska-Mazgaj, M., Surmiak, M., Sliwowski, Z., Wierdak, M., et al. (2018). Cross-talk between Hydrogen Sulfide and Carbon Monoxide in the Mechanism of Experimental Gastric Ulcers Healing, Regulation of Gastric Blood Flow and Accompanying Inflammation. Biochem. Pharmacol. 149, 131–142. doi:10.1016/j.bcp.2017.11.020
Magliano, D. J., Sacre, J. W., Harding, J. L., Gregg, E. W., Zimmet, P. Z., and Shaw, J. E. (2020). Young-onset Type 2 Diabetes Mellitus - Implications for Morbidity and Mortality. Nat. Rev. Endocrinol. 16, 321–331. doi:10.1038/s41574-020-0334-z
Mahalakshmi, A., and Kurian, G. A. (2020). Mitochondrial Dysfunction Plays a Key Role in the Abrogation of Cardioprotection by Sodium Hydrosulfide post-conditioning in Diabetic Cardiomyopathy Rat Heart. Naunyn Schmiedebergs Arch. Pharmacol. 393, 339–348. doi:10.1007/s00210-019-01733-z
Majumdar, P., Chen, S., George, B., Sen, S., Karmazyn, M., and Chakrabarti, S. (2009). Leptin and Endothelin-1 Mediated Increased Extracellular Matrix Protein Production and Cardiomyocyte Hypertrophy in Diabetic Heart Disease. Diabetes Metab. Res. Rev. 25, 452–463. doi:10.1002/dmrr.964
Mani, S., Untereiner, A., Wu, L., and Wang, R. (2014). Hydrogen Sulfide and the Pathogenesis of Atherosclerosis. Antioxid. Redox Signal. 20, 805–817. doi:10.1089/ars.2013.5324
Mathai, J. C., Missner, A., Kügler, P., Saparov, S. M., Zeidel, M. L., Lee, J. K., et al. (2009). No Facilitator Required for Membrane Transport of Hydrogen Sulfide. Proc. Natl. Acad. Sci. U S A. 106, 16633–16638. doi:10.1073/pnas.0902952106
Meng, G., Ma, Y., Xie, L., Ferro, A., and Ji, Y. (2015). Emerging Role of Hydrogen Sulfide in Hypertension and Related Cardiovascular Diseases. Br. J. Pharmacol. 172, 5501–5511. doi:10.1111/bph.12900
Miao, E. A., Leaf, I. A., Treuting, P. M., Mao, D. P., Dors, M., Sarkar, A., et al. (2010). Caspase-1-induced Pyroptosis Is an Innate Immune Effector Mechanism against Intracellular Bacteria. Nat. Immunol. 11, 1136–1142. doi:10.1038/ni.1960
Mok, Y. Y., Atan, M. S., Yoke Ping, C., Zhong Jing, W., Bhatia, M., Moochhala, S., et al. (2004). Role of Hydrogen Sulphide in Haemorrhagic Shock in the Rat: Protective Effect of Inhibitors of Hydrogen Sulphide Biosynthesis. Br. J. Pharmacol. 143, 881–889. doi:10.1038/sj.bjp.0706014
Mukherjee, S., and Corpas, F. J. (2020). Crosstalk Among Hydrogen Sulfide (H2S), Nitric Oxide (NO) and Carbon Monoxide (CO) in Root-System Development and its Rhizosphere Interactions: A Gaseous Interactome. Plant Physiol. Biochem. 155, 800–814. doi:10.1016/j.plaphy.2020.08.020
Nagpure, B. V., and Bian, J. S. (2016). Interaction of Hydrogen Sulfide with Nitric Oxide in the Cardiovascular System. Oxid Med. Cel Longev 2016, 6904327. doi:10.1155/2016/6904327
Nagy, P., Pálinkás, Z., Nagy, A., Budai, B., Tóth, I., and Vasas, A. (2014). Chemical Aspects of Hydrogen Sulfide Measurements in Physiological Samples. Biochim. Biophys. Acta 1840, 876–891. doi:10.1016/j.bbagen.2013.05.037
Nakanishi, T., Otaki, Y., Hasuike, Y., Nanami, M., Itahana, R., Miyagawa, K., et al. (2002). Association of Hyperhomocysteinemia with Plasma Sulfate and Urine Sulfate Excretion in Patients with Progressive Renal Disease. Am. J. Kidney Dis. 40, 909–915. doi:10.1053/ajkd.2002.36320
Nicholson, C. K., and Calvert, J. W. (2010). Hydrogen Sulfide and Ischemia-Reperfusion Injury. Pharmacol. Res. 62, 289–297. doi:10.1016/j.phrs.2010.06.002
Oktay, A. A., Aktürk, H. K., Paul, T. K., O’keefe, J. H., Ventura, H. O., Koch, C. A., et al. (2000). “Diabetes, Cardiomyopathy, and Heart Failure,”. Editors K. R. Feingold, B. Anawalt, A. Boyce, G. Chrousos, W. W. de Herder, K. Dunganet al. (South Dartmouth (MA): MDText.com, Inc). Copyright © 2000-2020.
Olson, K. R., Deleon, E. R., and Liu, F. (2014). Controversies and Conundrums in Hydrogen Sulfide Biology. Nitric Oxide 41, 11–26. doi:10.1016/j.niox.2014.05.012
Palamarchuk, I., Zaichko, N., Melnyk, A., Nechiporuk, V., and Yurchenko, P. (2020). Cardiomyocyte DNA Content and its Link to CSE/H2S System in the Heart of Experimental Diabetic Rats. Georgian Med. News 301, 147–152.
Pan, L. L., Liu, X. H., Gong, Q. H., and Zhu, Y. Z. (2011). S-Propargyl-cysteine (SPRC) Attenuated Lipopolysaccharide-Induced Inflammatory Response in H9c2 Cells Involved in a Hydrogen Sulfide-dependent Mechanism. Amino Acids 41, 205–215. doi:10.1007/s00726-011-0834-1
Pan, L. L., Liu, X. H., Gong, Q. H., Yang, H. B., and Zhu, Y. Z. (2012). Role of Cystathionine γ-lyase/hydrogen Sulfide Pathway in Cardiovascular Disease: a Novel Therapeutic Strategy?. Antioxid. Redox Signal. 17, 106–118. doi:10.1089/ars.2011.4349
Pan, L. L., Qin, M., Liu, X. H., and Zhu, Y. Z. (2017). The Role of Hydrogen Sulfide on Cardiovascular Homeostasis: An Overview with Update on Immunomodulation. Front. Pharmacol. 8, 686. doi:10.3389/fphar.2017.00686
Patel, V. B., Mclean, B. A., Chen, X., and Oudit, G. Y. (2015). Hydrogen Sulfide: an Old Gas with New Cardioprotective Effects. Clin. Sci. (Lond) 128, 321–323. doi:10.1042/CS20140668
Paul, B. D., and Snyder, S. H. (2012). H₂S Signalling through Protein Sulfhydration and beyond. Nat. Rev. Mol. Cel Biol 13, 499–507. doi:10.1038/nrm3391
Paul, B. D., and Snyder, S. H. (2015). H2S: A Novel Gasotransmitter that Signals by Sulfhydration. Trends Biochem. Sci. 40, 687–700. doi:10.1016/j.tibs.2015.08.007
Peake, B. F., Nicholson, C. K., Lambert, J. P., Hood, R. L., Amin, H., Amin, S., et al. (2013). Hydrogen Sulfide Preconditions the Db/db Diabetic Mouse Heart against Ischemia-Reperfusion Injury by Activating Nrf2 Signaling in an Erk-dependent Manner. Am. J. Physiol. Heart Circ. Physiol. 304, H1215–H1224. doi:10.1152/ajpheart.00796.2012
Pechánová, O., Varga, Z. V., Cebová, M., Giricz, Z., Pacher, P., and Ferdinandy, P. (2015). Cardiac NO Signalling in the Metabolic Syndrome. Br. J. Pharmacol. 172, 1415–1433. doi:10.1111/bph.12960
Peleli, M., Bibli, S. I., Li, Z., Chatzianastasiou, A., Varela, A., Katsouda, A., et al. (2020). Cardiovascular Phenotype of Mice Lacking 3-mercaptopyruvate Sulfurtransferase. Biochem. Pharmacol. 176, 113833. doi:10.1016/j.bcp.2020.113833
Perna, A. F., Lanza, D., Sepe, I., Raiola, I., Capasso, R., De Santo, N. G., et al. (2011). Hydrogen Sulfide, a Toxic Gas with Cardiovascular Properties in Uremia: How Harmful Is it?. Blood Purif. 31, 102–106. doi:10.1159/000321838
Pikkemaat, M., Melander, O., Hjerpe, P., and Bengtsson Boström, K. (2017). Prediction of Treatment Response in Patients with Newly Diagnosed Type 2 Diabetes: the Skaraborg Diabetes Register. J. Diabetes Complications 31, 854–858. doi:10.1016/j.jdiacomp.2017.02.013
Polhemus, D. J., and Lefer, D. J. (2014). Emergence of Hydrogen Sulfide as an Endogenous Gaseous Signaling Molecule in Cardiovascular Disease. Circ. Res. 114, 730–737. doi:10.1161/CIRCRESAHA.114.300505
Polhemus, D. J., Li, Z., Pattillo, C. B., Gojon, G., Gojon, , Giordano, T., et al. (2015). A Novel Hydrogen Sulfide Prodrug, SG1002, Promotes Hydrogen Sulfide and Nitric Oxide Bioavailability in Heart Failure Patients. Cardiovasc. Ther. 33, 216–226. doi:10.1111/1755-5922.12128
Possomato-Vieira, J. S., Gonçalves-Rizzi, V. H., do Nascimento, R. A., Wandekin, R. R., Caldeira-Dias, M., Chimini, J. S., et al. (2018). Clinical and Experimental Evidences of Hydrogen Sulfide Involvement in Lead-Induced Hypertension. Biomed. Res. Int. 2018, 4627391. doi:10.1155/2018/4627391
Powell, C. R., Dillon, K. M., and Matson, J. B. (2018). A Review of Hydrogen Sulfide (H2S) Donors: Chemistry and Potential Therapeutic Applications. Biochem. Pharmacol. 149, 110–123. doi:10.1016/j.bcp.2017.11.014
Predmore, B. L., Julian, D., and Cardounel, A. J. (2011). Hydrogen Sulfide Increases Nitric Oxide Production from Endothelial Cells by an Akt-dependent Mechanism. Front. Physiol. 2, 104. doi:10.3389/fphys.2011.00104
Pulido, I. R., Jahn, R., and Gerke, V. (2011). VAMP3 Is Associated with Endothelial Weibel-Palade Bodies and Participates in Their Ca(2+)-dependent Exocytosis. Biochim. Biophys. Acta 1813, 1038–1044. doi:10.1016/j.bbamcr.2010.11.007
Qin, C. X., Anthonisz, J., Leo, C. H., Kahlberg, N., Velagic, A., Li, M., et al. (2020). Nitric Oxide Resistance, Induced in the Myocardium by Diabetes, Is Circumvented by the Nitric Oxide Redox Sibling, Nitroxyl. Antioxid. Redox Signal. 32, 60–77. doi:10.1089/ars.2018.7706
Rajapurohitam, V., Kilic, A., Javadov, S., and Karmazyn, M. (2012). Role of NF-κB and P38 MAPK Activation in Mediating Angiotensin II and Endothelin-1-Induced Stimulation in Leptin Production and Cardiomyocyte Hypertrophy. Mol. Cel Biochem 366, 287–297. doi:10.1007/s11010-012-1307-x
Rawshani, A., Rawshani, A., Franzén, S., Sattar, N., Eliasson, B., Svensson, A. M., et al. (2018). Risk Factors, Mortality, and Cardiovascular Outcomes in Patients with Type 2 Diabetes. N. Engl. J. Med. 379, 633–644. doi:10.1056/NEJMoa1800256
Renieris, G., Katrini, K., Damoulari, C., Akinosoglou, K., Psarrakis, C., Kyriakopoulou, M., et al. (2020). Serum Hydrogen Sulfide and Outcome Association in Pneumonia by the SARS-CoV-2 Coronavirus. Shock 54, 633–637. doi:10.1097/SHK.0000000000001562
Rodrigues, C., and Percival, S. S. (2019). Immunomodulatory Effects of Glutathione, Garlic Derivatives, and Hydrogen Sulfide. Nutrients 11, 295. doi:10.3390/nu11020295
Rose, P., Moore, P. K., and Zhu, Y. Z. (2017). H2S Biosynthesis and Catabolism: New Insights from Molecular Studies. Cell Mol Life Sci 74, 1391–1412. doi:10.1007/s00018-016-2406-8
Rose, P., Moore, P. K., and Zhu, Y. Z. (2018). Garlic and Gaseous Mediators. Trends Pharmacol. Sci. 39, 624–634. doi:10.1016/j.tips.2018.03.009
Roussel, J., Thireau, J., Brenner, C., Saint, N., Scheuermann, V., Lacampagne, A., et al. (2015). Palmitoyl-carnitine Increases RyR2 Oxidation and Sarcoplasmic Reticulum Ca2+ Leak in Cardiomyocytes: Role of Adenine Nucleotide Translocase. Biochim. Biophys. Acta 1852, 749–758. doi:10.1016/j.bbadis.2015.01.011
Roy, A., Khan, A. H., Islam, M. T., Prieto, M. C., and Majid, D. S. (2012). Interdependency of Cystathione γ-lyase and Cystathione β-synthase in Hydrogen Sulfide-Induced Blood Pressure Regulation in Rats. Am. J. Hypertens. 25, 74–81. doi:10.1038/ajh.2011.149
Rubler, S., Dlugash, J., Yuceoglu, Y. Z., Kumral, T., Branwood, A. W., and Grishman, A. (1972). New Type of Cardiomyopathy Associated with Diabetic Glomerulosclerosis. Am. J. Cardiol. 30, 595–602. doi:10.1016/0002-9149(72)90595-4
Saeedi, P., Petersohn, I., Salpea, P., Malanda, B., Karuranga, S., Unwin, N., et al. (2019). Global and Regional Diabetes Prevalence Estimates for 2019 and Projections for 2030 and 2045: Results from the International Diabetes Federation Diabetes Atlas, 9th Edition. Diabetes Res. Clin. Pract. 157, 107843. doi:10.1016/j.diabres.2019.107843
Saeid, F., Aniseh, J., Reza, B., and Manouchehr, V. S. (2018). Signaling Mediators Modulated by Cardioprotective Interventions in Healthy and Diabetic Myocardium with Ischaemia-Reperfusion Injury. Eur. J. Prev. Cardiol. 25, 1463–1481. doi:10.1177/2047487318756420
Schramm, T. K., Gislason, G. H., Køber, L., Rasmussen, S., Rasmussen, J. N., Abildstrøm, S. Z., et al. (2008). Diabetes Patients Requiring Glucose-Lowering Therapy and Nondiabetics with a Prior Myocardial Infarction Carry the Same Cardiovascular Risk: a Population Study of 3.3 Million People. Circulation 117, 1945–1954. doi:10.1161/CIRCULATIONAHA.107.720847
Shen, X., Pattillo, C. B., Pardue, S., Bir, S. C., Wang, R., and Kevil, C. G. (2011). Measurement of Plasma Hydrogen Sulfide In Vivo and In Vitro. Free Radic. Biol. Med. 50, 1021–1031. doi:10.1016/j.freeradbiomed.2011.01.025
Shi, L., Liu, X. Y., Huang, Z. G., Ma, Z. Y., Xi, Y., Wang, L. Y., et al. (2019). Endogenous Hydrogen Sulfide and ERK1/2-STAT3 Signaling Pathway May Participate in the Association between Homocysteine and Hypertension. J. Geriatr. Cardiol. 16, 822–834. doi:10.11909/j.issn.1671-5411.2019.11.007
Shibuya, N., Mikami, Y., Kimura, Y., Nagahara, N., and Kimura, H. (2009). Vascular Endothelium Expresses 3-mercaptopyruvate Sulfurtransferase and Produces Hydrogen Sulfide. J. Biochem. 146, 623–626. doi:10.1093/jb/mvp111
Shimamoto, K., and Hanaoka, K. (2015). Fluorescent Probes for Hydrogen Sulfide (H2S) and Sulfane Sulfur and Their Applications to Biological Studies. Nitric Oxide 46, 72–79. doi:10.1016/j.niox.2014.11.008
Shimizu, Y., Polavarapu, R., Eskla, K. L., Nicholson, C. K., Koczor, C. A., Wang, R., et al. (2018). Hydrogen Sulfide Regulates Cardiac Mitochondrial Biogenesis via the Activation of AMPK. J. Mol. Cel Cardiol 116, 29–40. doi:10.1016/j.yjmcc.2018.01.011
Sonobe, T., and Haouzi, P. (2016). Sulfide Intoxication-Induced Circulatory Failure Is Mediated by a Depression in Cardiac Contractility. Cardiovasc. Toxicol. 16, 67–78. doi:10.1007/s12012-015-9309-z
Standl, E., Khunti, K., Hansen, T. B., and Schnell, O. (2019). The Global Epidemics of Diabetes in the 21st century: Current Situation and Perspectives. Eur. J. Prev. Cardiol. 26, 7–14. doi:10.1177/2047487319881021
Stone, P. H., Muller, J. E., Hartwell, T., York, B. J., Rutherford, J. D., Parker, C. B., et al. (1989). The Effect of Diabetes Mellitus on Prognosis and Serial Left Ventricular Function after Acute Myocardial Infarction: Contribution of Both Coronary Disease and Diastolic Left Ventricular Dysfunction to the Adverse Prognosis. The MILIS Study Group. J. Am. Coll. Cardiol. 14, 49–57. doi:10.1016/0735-1097(89)90053-3
Sugahara, S., Suzuki, M., Kamiya, H., Yamamuro, M., Semura, H., Senga, Y., et al. (2016). Colorimetric Determination of Sulfide in Microsamples. Anal. Sci. 32, 1129–1131. doi:10.2116/analsci.32.1129
Sun, Y. G., Cao, Y. X., Wang, W. W., Ma, S. F., Yao, T., and Zhu, Y. C. (2008). Hydrogen Sulphide Is an Inhibitor of L-type Calcium Channels and Mechanical Contraction in Rat Cardiomyocytes. Cardiovasc. Res. 79, 632–641. doi:10.1093/cvr/cvn140
Sun, Y., Tang, C. S., Du, J. B., and Jin, H. F. (2011). Hydrogen Sulfide and Vascular Relaxation. Chin. Med. J. (Engl) 124, 3816–3819.
Sun, Q., Wang, B., Li, Y., Sun, F., Li, P., Xia, W., et al. (2016). Taurine Supplementation Lowers Blood Pressure and Improves Vascular Function in Prehypertension: Randomized, Double-Blind, Placebo-Controlled Study. Hypertension 67, 541–549. doi:10.1161/HYPERTENSIONAHA.115.06624
Sun, H. J., Wu, Z. Y., Cao, L., Zhu, M. Y., Liu, T. T., Guo, L., et al. (2019a). Hydrogen Sulfide: Recent Progression and Perspectives for the Treatment of Diabetic Nephropathy. Molecules 24, 2857. doi:10.3390/molecules24152857
Sun, H. J., Wu, Z. Y., Nie, X. W., and Bian, J. S. (2019b). Role of Endothelial Dysfunction in Cardiovascular Diseases: The Link between Inflammation and Hydrogen Sulfide. Front. Pharmacol. 10, 1568. doi:10.3389/fphar.2019.01568
Sun, Y., Teng, Z., Sun, X., Zhang, L., Chen, J., Wang, B., et al. (2019c). Exogenous H2S Reduces the Acetylation Levels of Mitochondrial Respiratory Enzymes via Regulating the NAD+-SIRT3 Pathway in Cardiac Tissues of Db/db Mice. Am. J. Physiol. Endocrinol. Metab. 317, E284–e297. doi:10.1152/ajpendo.00326.2018
Sun, H. J., Lee, W. T., Leng, B., Wu, Z. Y., Yang, Y., and Bian, J. S. (2020a). Nitroxyl as a Potential Theranostic in the Cancer Arena. Antioxid. Redox Signal. 32, 331–349. doi:10.1089/ars.2019.7904
Sun, H. J., Wu, Z. Y., Cao, L., Zhu, M. Y., Nie, X. W., Huang, D. J., et al. (2020b). Role of Nitroxyl (HNO) in Cardiovascular System: From Biochemistry to Pharmacology. Pharmacol. Res. 159, 104961. doi:10.1016/j.phrs.2020.104961
Sun, H. J., Xiong, S. P., Wu, Z. Y., Cao, L., Zhu, M. Y., Moore, P. K., et al. (2020c). Induction of caveolin-3/eNOS Complex by Nitroxyl (HNO) Ameliorates Diabetic Cardiomyopathy. Redox Biol. 32, 101493. doi:10.1016/j.redox.2020.101493
Sun, X., Zhao, D., Lu, F., Peng, S., Yu, M., Liu, N., et al. (2020d). Hydrogen Sulfide Regulates Muscle RING finger-1 Protein S-Sulfhydration at Cys44 to Prevent Cardiac Structural Damage in Diabetic Cardiomyopathy. Br. J. Pharmacol. 177, 836–856. doi:10.1111/bph.14601
Sun, Y., Lu, F., Yu, X., Wang, B., Chen, J., Lu, F., et al. (2020e). Exogenous H2S Promoted USP8 Sulfhydration to Regulate Mitophagy in the Hearts of Db/db Mice. Aging Dis. 11, 269–285. doi:10.14336/AD.2019.0524
Sun, H.-J., Wu, Z.-Y., Nie, X.-W., and Bian, J.-S. (2021). Role of Hydrogen Sulfide and Polysulfides in Neurological Diseases: Focus on Protein S-Persulfidation. Cn 19, 868–884. doi:10.2174/1570159x18666200905143550
Suzuki, K., Sagara, M., Aoki, C., Tanaka, S., and Aso, Y. (2017). Clinical Implication of Plasma Hydrogen Sulfide Levels in Japanese Patients with Type 2 Diabetes. Intern. Med. 56, 17–21. doi:10.2169/internalmedicine.56.7403
Switzer, C. H., Flores-Santana, W., Mancardi, D., Donzelli, S., Basudhar, D., Ridnour, L. A., et al. (2009). The Emergence of Nitroxyl (HNO) as a Pharmacological Agent. Biochim. Biophys. Acta 1787, 835–840. doi:10.1016/j.bbabio.2009.04.015
Szabó, C. (2007). Hydrogen Sulphide and its Therapeutic Potential. Nat. Rev. Drug Discov. 6, 917–935. doi:10.1038/nrd2425
Szabó, G., Veres, G., Radovits, T., Gero, D., Módis, K., Miesel-Gröschel, C., et al. (2011). Cardioprotective Effects of Hydrogen Sulfide. Nitric Oxide 25, 201–210. doi:10.1016/j.niox.2010.11.001
Szijártó, I. A., Markó, L., Filipovic, M. R., Miljkovic, J. L., Tabeling, C., Tsvetkov, D., et al. (2018). Cystathionine γ-Lyase-Produced Hydrogen Sulfide Controls Endothelial NO Bioavailability and Blood Pressure. Hypertension 71, 1210–1217. doi:10.1161/HYPERTENSIONAHA.117.10562
Tan, B., Jin, S., Sun, J., Gu, Z., Sun, X., Zhu, Y., et al. (2017). New Method for Quantification of Gasotransmitter Hydrogen Sulfide in Biological Matrices by LC-MS/MS. Sci. Rep. 7, 46278. doi:10.1038/srep46278
Teoh, J. P., Li, X., Simoncini, T., Zhu, D., and Fu, X. (2020). Estrogen-Mediated Gaseous Signaling Molecules in Cardiovascular Disease. Trends Endocrinol. Metab. 31, 773–784. doi:10.1016/j.tem.2020.06.001
Testai, L., Citi, V., Martelli, A., Brogi, S., and Calderone, V. (2020). Role of Hydrogen Sulfide in Cardiovascular Ageing. Pharmacol. Res. 160, 105125. doi:10.1016/j.phrs.2020.105125
Toldo, S., Das, A., Mezzaroma, E., Chau, V. Q., Marchetti, C., Durrant, D., et al. (2014). Induction of microRNA-21 with Exogenous Hydrogen Sulfide Attenuates Myocardial Ischemic and Inflammatory Injury in Mice. Circ. Cardiovasc. Genet. 7, 311–320. doi:10.1161/CIRCGENETICS.113.000381
Tomasova, L., Pavlovicova, M., Malekova, L., Misak, A., Kristek, F., Grman, M., et al. (2015). Effects of AP39, a Novel Triphenylphosphonium Derivatised Anethole Dithiolethione Hydrogen Sulfide Donor, on Rat Haemodynamic Parameters and Chloride and Calcium Cav3 and RyR2 Channels. Nitric Oxide 46, 131–144. doi:10.1016/j.niox.2014.12.012
Tong, F., Chai, R., Jiang, H., and Dong, B. (2018). In Vitro/vivo Drug Release and Anti-diabetic Cardiomyopathy Properties of Curcumin/PBLG-PEG-PBLG Nanoparticles. Int. J. Nanomed. 13, 1945–1962. doi:10.2147/IJN.S153763
Tsai, C. Y., Wen, S. Y., Shibu, M. A., Yang, Y. C., Peng, H., Wang, B., et al. (2015). Diallyl Trisulfide Protects against High Glucose-Induced Cardiac Apoptosis by Stimulating the Production of Cystathionine Gamma-Lyase-Derived Hydrogen Sulfide. Int. J. Cardiol. 195, 300–310. doi:10.1016/j.ijcard.2015.05.111
Van de Louw, A., and Haouzi, P. (2012). Oxygen Deficit and H2S in Hemorrhagic Shock in Rats. Crit. Care 16, R178. doi:10.1186/cc11661
Varga, Z. V., Giricz, Z., Liaudet, L., Haskó, G., Ferdinandy, P., and Pacher, P. (2015). Interplay of Oxidative, Nitrosative/nitrative Stress, Inflammation, Cell Death and Autophagy in Diabetic Cardiomyopathy. Biochim. Biophys. Acta 1852, 232–242. doi:10.1016/j.bbadis.2014.06.030
Velagic, A., Qin, C., Woodman, O. L., Horowitz, J. D., Ritchie, R. H., and Kemp-Harper, B. K. (2020). Nitroxyl: A Novel Strategy to Circumvent Diabetes Associated Impairments in Nitric Oxide Signaling. Front. Pharmacol. 11, 727. doi:10.3389/fphar.2020.00727
Vitvitsky, V., Kabil, O., and Banerjee, R. (2012). High Turnover Rates for Hydrogen Sulfide Allow for Rapid Regulation of its Tissue Concentrations. Antioxid. Redox Signal. 17, 22–31. doi:10.1089/ars.2011.4310
Wang, Z. V., and Hill, J. A. (2015). Diabetic Cardiomyopathy: Catabolism Driving Metabolism. Circulation 131, 771–773. doi:10.1161/CIRCULATIONAHA.115.015357
Wang, Q., Song, B., Jiang, S., Liang, C., Chen, X., Shi, J., et al. (2015a). Hydrogen Sulfide Prevents Advanced Glycation End-Products Induced Activation of the Epithelial Sodium Channel. Oxid Med. Cel Longev 2015, 976848. doi:10.1155/2015/976848
Wang, R., Szabo, C., Ichinose, F., Ahmed, A., Whiteman, M., and Papapetropoulos, A. (2015b). The Role of H2S Bioavailability in Endothelial Dysfunction. Trends Pharmacol. Sci. 36, 568–578. doi:10.1016/j.tips.2015.05.007
Wang, W., Feng, S. J., Li, H., Zhang, X. D., and Wang, S. X. (2015c). Correlation of Lower Concentrations of Hydrogen Sulfide with Activation of Protein Kinase CβII in Uremic Accelerated Atherosclerosis Patients. Chin. Med. J. (Engl) 128, 1465–1470. doi:10.4103/0366-6999.157653
Wang, Y., Yu, R., Wu, L., and Yang, G. (2020). Hydrogen Sulfide Signaling in Regulation of Cell Behaviors. Nitric Oxide 103, 9–19. doi:10.1016/j.niox.2020.07.002
Wang, R. (2002). Two's Company, Three's a Crowd: Can H2S Be the Third Endogenous Gaseous Transmitter?. Faseb j 16, 1792–1798. doi:10.1096/fj.02-0211hyp
Wang, R. (2010). Hydrogen Sulfide: the Third Gasotransmitter in Biology and Medicine. Antioxid. Redox Signal. 12, 1061–1064. doi:10.1089/ars.2009.2938
Wang, R. (2012). Physiological Implications of Hydrogen Sulfide: a Whiff Exploration that Blossomed. Physiol. Rev. 92, 791–896. doi:10.1152/physrev.00017.2011
Wei, W. B., Hu, X., Zhuang, X. D., Liao, L. Z., and Li, W. D. (2014). GYY4137, a Novel Hydrogen Sulfide-Releasing Molecule, Likely Protects against High Glucose-Induced Cytotoxicity by Activation of the AMPK/mTOR Signal Pathway in H9c2 Cells. Mol. Cel Biochem 389, 249–256. doi:10.1007/s11010-013-1946-6
Wen, Y. D., Wang, H., and Zhu, Y. Z. (2018). The Drug Developments of Hydrogen Sulfide on Cardiovascular Disease. Oxid Med. Cel Longev. 2018, 4010395. doi:10.1155/2018/4010395
Whiteman, M., Armstrong, J. S., Chu, S. H., Jia-Ling, S., Wong, B. S., Cheung, N. S., et al. (2004). The Novel Neuromodulator Hydrogen Sulfide: an Endogenous Peroxynitrite 'scavenger'?. J. Neurochem. 90, 765–768. doi:10.1111/j.1471-4159.2004.02617.x
Whiteman, M., Cheung, N. S., Zhu, Y. Z., Chu, S. H., Siau, J. L., Wong, B. S., et al. (2005). Hydrogen Sulphide: a Novel Inhibitor of Hypochlorous Acid-Mediated Oxidative Damage in the Brain?. Biochem. Biophys. Res. Commun. 326, 794–798. doi:10.1016/j.bbrc.2004.11.110
Whiteman, M., Li, L., Kostetski, I., Chu, S. H., Siau, J. L., Bhatia, M., et al. (2006). Evidence for the Formation of a Novel Nitrosothiol from the Gaseous Mediators Nitric Oxide and Hydrogen Sulphide. Biochem. Biophys. Res. Commun. 343, 303–310. doi:10.1016/j.bbrc.2006.02.154
Whitfield, N. L., Kreimier, E. L., Verdial, F. C., Skovgaard, N., and Olson, K. R. (2008). Reappraisal of H2S/sulfide Concentration in Vertebrate Blood and its Potential Significance in Ischemic Preconditioning and Vascular Signaling. Am. J. Physiol. Regul. Integr. Comp. Physiol. 294, R1930–R1937. doi:10.1152/ajpregu.00025.2008
Wiliński, B., Wiliński, J., Somogyi, E., Piotrowska, J., and Opoka, W. (2013). Metformin Raises Hydrogen Sulfide Tissue Concentrations in Various Mouse Organs. Pharmacol. Rep. 65, 737–742. doi:10.1016/s1734-1140(13)71053-3
Wintner, E. A., Deckwerth, T. L., Langston, W., Bengtsson, A., Leviten, D., Hill, P., et al. (2010). A Monobromobimane-Based Assay to Measure the Pharmacokinetic Profile of Reactive Sulphide Species in Blood. Br. J. Pharmacol. 160, 941–957. doi:10.1111/j.1476-5381.2010.00704.x
Wu, D., Hu, Q., and Zhu, D. (2018). An Update on Hydrogen Sulfide and Nitric Oxide Interactions in the Cardiovascular System. Oxid Med. Cel Longev 2018, 4579140. doi:10.1155/2018/4579140
Wu, J., Tian, Z., Sun, Y., Lu, C., Liu, N., Gao, Z., et al. (2017). Exogenous H2S Facilitating Ubiquitin Aggregates Clearance via Autophagy Attenuates Type 2 Diabetes-Induced Cardiomyopathy. Cell Death Dis 8, e2992. doi:10.1038/cddis.2017.380
Xia, M., Chen, L., Muh, R. W., Li, P. L., and Li, N. (2009). Production and Actions of Hydrogen Sulfide, a Novel Gaseous Bioactive Substance, in the Kidneys. J. Pharmacol. Exp. Ther. 329, 1056–1062. doi:10.1124/jpet.108.149963
Xiao, T., Luo, J., Wu, Z., Li, F., Zeng, O., and Yang, J. (2016). Effects of Hydrogen Sulfide on Myocardial Fibrosis and PI3K/AKT1-Regulated Autophagy in Diabetic Rats. Mol. Med. Rep. 13, 1765–1773. doi:10.3892/mmr.2015.4689
Xie, M., Morales, C. R., Lavandero, S., and Hill, J. A. (2011). Tuning Flux: Autophagy as a Target of Heart Disease Therapy. Curr. Opin. Cardiol. 26, 216–222. doi:10.1097/HCO.0b013e328345980a
Xie, Z. Z., Liu, Y., and Bian, J. S. (2016). Hydrogen Sulfide and Cellular Redox Homeostasis. Oxid Med. Cel Longev. 2016, 6043038. doi:10.1155/2016/6043038
Xie, J., Ikram, M. K., Cotch, M. F., Klein, B., Varma, R., Shaw, J. E., et al. (2017). Association of Diabetic Macular Edema and Proliferative Diabetic Retinopathy with Cardiovascular Disease: A Systematic Review and Meta-Analysis. JAMA Ophthalmol. 135, 586–593. doi:10.1001/jamaophthalmol.2017.0988
Xu, J., Zhou, Q., Xu, W., and Cai, L. (2012). Endoplasmic Reticulum Stress and Diabetic Cardiomyopathy. Exp. Diabetes Res. 2012, 827971. doi:10.1155/2012/827971
Xu, W., Wu, W., Chen, J., Guo, R., Lin, J., Liao, X., et al. (2013). Exogenous Hydrogen Sulfide Protects H9c2 Cardiac Cells against High Glucose-Induced Injury by Inhibiting the Activities of the P38 MAPK and ERK1/2 Pathways. Int. J. Mol. Med. 32, 917–925. doi:10.3892/ijmm.2013.1462
Xu, W., Chen, J., Lin, J., Liu, D., Mo, L., Pan, W., et al. (2015). Exogenous H2S Protects H9c2 Cardiac Cells against High Glucose-Induced Injury and Inflammation by Inhibiting the Activation of the NF-κB and IL-1β Pathways. Int. J. Mol. Med. 35, 177–186. doi:10.3892/ijmm.2014.2007
Xuan, L., Li, J., Wang, X., and Wang, C. (2020). Crosstalk between Hydrogen Sulfide and Other Signal Molecules Regulates Plant Growth and Development. Int. J. Mol. Sci. 21, 4593. doi:10.3390/ijms21134593
Yan, H. F., Tuo, Q. Z., Yin, Q. Z., and Lei, P. (2020). The Pathological Role of Ferroptosis in Ischemia/reperfusion-Related Injury. Zool Res. 41, 220–230. doi:10.24272/j.issn.2095-8137.2020.042
Yang, G., and Wang, R. (2015). H2S and Blood Vessels: An Overview. Handb Exp. Pharmacol. 230, 85–110. doi:10.1007/978-3-319-18144-8_4
Yang, R., Jia, Q., Liu, X., Wang, Y., Gao, Q., and Ma, S. (2016a). Effect of Hydrogen Sulfide on Cardiac Myosin Light Chain Kinase Expression in Diabetic Rats. Zhong Nan Da Xue Xue Bao Yi Xue Ban 41, 353–358. doi:10.11817/j.issn.1672-7347.2016.04.003
Yang, R., Jia, Q., Liu, X. F., Gao, Q., Wang, L., and Ma, S. F. (2016b). Effect of Hydrogen Sulfide on Oxidative Stress and Endoplasmic Reticulum Stress in Diabetic Cardiomyopathy. Zhongguo Ying Yong Sheng Li Xue Za Zhi 32, 8–12.
Yang, F., Yu, X., Li, T., Wu, J., Zhao, Y., Liu, J., et al. (2017a). Exogenous H2S Regulates Endoplasmic Reticulum-Mitochondria Cross-Talk to Inhibit Apoptotic Pathways in STZ-Induced Type I Diabetes. Am. J. Physiol. Endocrinol. Metab. 312, E190–e203. doi:10.1152/ajpendo.00196.2016
Yang, R., Jia, Q., Liu, X. F., Wang, Y. Y., and Ma, S. F. (2017b). Effects of Hydrogen Sulfide on Inducible Nitric Oxide Synthase Activity and Expression of Cardiomyocytes in Diabetic Rats. Mol. Med. Rep. 16, 5277–5284. doi:10.3892/mmr.2017.7247
Yang, R., Jia, Q., Ma, S. F., Wang, Y., Mehmood, S., and Chen, Y. (2019). Exogenous H2S Mitigates Myocardial Fibrosis in Diabetic Rats through Suppression of the Canonical Wnt Pathway. Int. J. Mol. Med. 44, 549–558. doi:10.3892/ijmm.2019.4237
Ye, P., Gu, Y., Zhu, Y. R., Chao, Y. L., Kong, X. Q., Luo, J., et al. (2018). Exogenous Hydrogen Sulfide Attenuates the Development of Diabetic Cardiomyopathy via the FoxO1 Pathway. J. Cel Physiol 233, 9786–9798. doi:10.1002/jcp.26946
Yellon, D. M., and Hausenloy, D. J. (2007). Myocardial Reperfusion Injury. N. Engl. J. Med. 357, 1121–1135. doi:10.1056/NEJMra071667
Yong, R., and Searcy, D. G. (2001). Sulfide Oxidation Coupled to ATP Synthesis in Chicken Liver Mitochondria. Comp. Biochem. Physiol. B Biochem. Mol. Biol. 129, 129–137. doi:10.1016/s1096-4959(01)00309-8
Yong, Q. C., Pan, T. T., Hu, L. F., and Bian, J. S. (2008). Negative Regulation of Beta-Adrenergic Function by Hydrogen Sulphide in the Rat Hearts. J. Mol. Cel Cardiol 44, 701–710. doi:10.1016/j.yjmcc.2008.01.007
Yu, X. H., Cui, L. B., Wu, K., Zheng, X. L., Cayabyab, F. S., Chen, Z. W., et al. (2014). Hydrogen Sulfide as a Potent Cardiovascular Protective Agent. Clin. Chim. Acta 437, 78–87. doi:10.1016/j.cca.2014.07.012
Yu, M., Du, H., Wang, B., Chen, J., Lu, F., Peng, S., et al. (2020). Exogenous H2S Induces Hrd1 S-Sulfhydration and Prevents CD36 Translocation via VAMP3 Ubiquitylation in Diabetic Hearts. Aging Dis. 11, 286–300. doi:10.14336/AD.2019.0530
Yusuf, M., Kwong Huat, B. T., Hsu, A., Whiteman, M., Bhatia, M., and Moore, P. K. (2005). Streptozotocin-induced Diabetes in the Rat Is Associated with Enhanced Tissue Hydrogen Sulfide Biosynthesis. Biochem. Biophys. Res. Commun. 333, 1146–1152. doi:10.1016/j.bbrc.2005.06.021
Zamora, M., and Villena, J. A. (2019). Contribution of Impaired Insulin Signaling to the Pathogenesis of Diabetic Cardiomyopathy. Int. J. Mol. Sci. 20, 2833. doi:10.3390/ijms20112833
Zhang, J. R., and Sun, H. J. (2020). Roles of Circular RNAs in Diabetic Complications: From Molecular Mechanisms to Therapeutic Potential. Gene 763, 145066. doi:10.1016/j.gene.2020.145066
Zhang, M., and Ye, M. (2019). Hydrogen Sulfide Attenuates High Glucose-Induced Myocardial Injury in Rat Cardiomyocytes by Suppressing Wnt/beta-Catenin Pathway. Curr. Med. Sci. 39, 938–946. doi:10.1007/s11596-019-2120-5
Zhang, J., Chen, S., Liu, H., Zhang, B., Zhao, Y., Ma, K., et al. (2013). Hydrogen Sulfide Prevents Hydrogen Peroxide-Induced Activation of Epithelial Sodium Channel through a PTEN/PI(3,4,5)P3 Dependent Pathway. PLoS One 8, e64304. doi:10.1371/journal.pone.0064304
Zhang, D., Du, J., Tang, C., Huang, Y., and Jin, H. (2017). H2S-Induced Sulfhydration: Biological Function and Detection Methodology. Front. Pharmacol. 8, 608. doi:10.3389/fphar.2017.00608
Zhang, P., Yu, Y., Wang, P., Shen, H., Ling, X., Xue, X., et al. (2020a). Role of Hydrogen Sulfide in Myocardial Ischemia-Reperfusion Injury. J. Cardiovasc. Pharmacol. 77, 130–141. doi:10.1097/FJC.0000000000000943
Zhang, Y., Chen, Y., Bai, Y., Xue, X., He, W., and Guo, Z. (2020b). FRET-based Fluorescent Ratiometric Probes for the Rapid Detection of Endogenous Hydrogen Sulphide in Living Cells. Analyst 145, 4233–4238. doi:10.1039/d0an00531b
Zhang, Y., Zhuang, S., Jiang, S., Zhang, J., Chen, Y., Canu, I. G., et al. (2021). Preparation of Nanocomposite Peptide and its Inhibitory Effect on Myocardial Injury in Type-II Diabetic Rats. J. Nanosci Nanotechnol 21, 1378–1384. doi:10.1166/jnn.2021.18652
Zheng, D., Dong, S., Li, T., Yang, F., Yu, X., Wu, J., et al. (2015). Exogenous Hydrogen Sulfide Attenuates Cardiac Fibrosis through Reactive Oxygen Species Signal Pathways in Experimental Diabetes Mellitus Models. Cel Physiol Biochem 36, 917–929. doi:10.1159/000430266
Zhou, X., An, G., and Lu, X. (2015). Hydrogen Sulfide Attenuates the Development of Diabetic Cardiomyopathy. Clin. Sci. (Lond) 128, 325–335. doi:10.1042/CS20140460
Zhuang, X. D., Hu, X., Long, M., Dong, X. B., Liu, D. H., and Liao, X. X. (2014). Exogenous Hydrogen Sulfide Alleviates High Glucose-Induced Cardiotoxicity via Inhibition of Leptin Signaling in H9c2 Cells. Mol. Cel Biochem 391, 147–155. doi:10.1007/s11010-014-1997-3
Zuo, G., Ren, X., Qian, X., Ye, P., Luo, J., Gao, X., et al. (2019). Inhibition of JNK and P38 MAPK-Mediated Inflammation and Apoptosis by Ivabradine Improves Cardiac Function in Streptozotocin-Induced Diabetic Cardiomyopathy. J. Cel Physiol 234, 1925–1936. doi:10.1002/jcp.27070
Glossary
1-NCA 1-nitrosocyclohexylacetate
3-MP 3-mercaptopyruvate
3-MST 3-mercaptopyruvate sulfurtransferase
AGEs advanced glycation end products
AICAR 5-amino-4-imidazole-carboxamide riboside
AMPK adenosine 5′-monophosphate (AMP)-activated protein kinase
Ang II angiotensin II
ARE antioxidant response element
ASC apoptosis-associated speck-like protein containing A CARD
CASQ2 calsequestrin 2
CAT cysteine aminotransferase
CBS cystathionine β-synthase
CHOP C/EBP-homologous protein
CK-MB creatine kinase MB isozyme
CO carbon monoxide
COX-2 cyclooxygenase-2
CSE cystathionine γ-lyase
DAO d-amino acid oxidase
eIF2 eukaryotic initiation factor 2
ERK1/2 extracellular regulated protein kinase 1/2
GAPDH glyceraldehyde-3-phosphate dehydrogenase
GRP78 glucose-regulated protein
H2S hydrogen sulfide
HFD high fat diet
HNO nitroxyl
HO-1 heme oxygenase 1
Hrd1 HMG-CoA reductase degradation protein
IL-1β interleukin 1β
iNOS inducible nitric oxide synthase
JAK Janus kinase
JNK c-Jun N-terminal kinase
MAPK mitogen activated protein kinase
MLCK myosin light chain kinase
MMP-2 matrix metalloprotease 2
mTOR mammalian target of rapamycin
MuRF1 muscle RING finger-1
NaHS sodium hydrosulfide
NF-κB nuclear transcription factor-κB
NLRP3 nucleotide-binding oligomerization domain-like receptor protein 3
NO nitric oxide
NQO1 Nad(p)h: quinone oxidoreductase 1
Nrf2 nuclear factor erythroid 2-related factor 2
PDE5 phosphodiesterase 5
PERK pancreatic endoplasmic reticulum kinase
PI3K phosphatidylinositol 3-kinase
PKC protein kinase C
RAS renin–angiotensin system
ROS reactive oxygen species
RyR2 ryanodine receptor
SERCA2a sarcoplasmic reticulum Ca2+ ATPase
Smad3 SMAD family member 3
SNP sodium nitroprusside
STAT3 signal transducer and activator of transcription 3
STZ streptozotocin
TGF-β1 transforming growth factor β1
TIMP-2 tissue inhibitor of metalloproteinase 2
TLR4 toll-like receptor 4
USP8 ubiquitin specific peptidase 8
VAMP3 vesicle-associated membrane protein 3
Keywords: diabetes, diabetic cardiomyopathy, nitric oxide, hydrogen sulfide, ischaemia-reperfusion injury
Citation: Sun H-J, Wu Z-Y, Nie X-W, Wang X-Y and Bian J-S (2021) An Updated Insight Into Molecular Mechanism of Hydrogen Sulfide in Cardiomyopathy and Myocardial Ischemia/Reperfusion Injury Under Diabetes. Front. Pharmacol. 12:651884. doi: 10.3389/fphar.2021.651884
Received: 11 January 2021; Accepted: 23 September 2021;
Published: 26 October 2021.
Edited by:
Xinhua Qu, Shanghai JiaoTong University, ChinaReviewed by:
Narasimham L. Parinandi, The Ohio State University, United StatesAysegul Kucuk, KSBU, Turkey
Copyright © 2021 Sun, Wu, Nie, Wang and Bian. This is an open-access article distributed under the terms of the Creative Commons Attribution License (CC BY). The use, distribution or reproduction in other forums is permitted, provided the original author(s) and the copyright owner(s) are credited and that the original publication in this journal is cited, in accordance with accepted academic practice. No use, distribution or reproduction is permitted which does not comply with these terms.
*Correspondence: Jin-Song Bian, cGhjYmpzQG51cy5lZHUuc2c=