- 1Department of Medical Biology, Amsterdam UMC, University of Amsterdam, Amsterdam, Netherlands
- 2Department of Experimental Cardiology, Amsterdam UMC, University of Amsterdam, Amsterdam, Netherlands
- 3Department of Cardiothoracic Surgery, Amsterdam UMC, University of Amsterdam, Amsterdam, Netherlands
- 4Department of Cardiology, Amsterdam UMC, University of Amsterdam, Amsterdam, Netherlands
Introduction: Atrial fibrillation (AF) is the most common cardiac arrhythmia. Consequently, novel therapies are being developed. Ultimately, the impact of compounds on the action potential (AP) needs to be tested in freshly isolated human atrial myocytes. However, the frequent depolarized state of these cells upon isolation seriously hampers reliable AP recordings.
Purpose: We assessed whether AP recordings from single human atrial myocytes could be improved by providing these cells with a proper inward rectifier K+ current (IK1), and consequently with a regular, non-depolarized resting membrane potential (RMP), through “dynamic clamp”.
Methods: Single myocytes were enzymatically isolated from left atrial appendage tissue obtained from patients with paroxysmal AF undergoing minimally invasive surgical ablation. APs were elicited at 1 Hz and measured using perforated patch-clamp methodology, injecting a synthetic IK1 to generate a regular RMP. The injected IK1 had strong or moderate rectification. For comparison, a regular RMP was forced through injection of a constant outward current. A wide variety of ion channel blockers was tested to assess their modulatory effects on AP characteristics.
Results: Without any current injection, RMPs ranged from −9.6 to −86.2 mV in 58 cells. In depolarized cells (RMP positive to −60 mV), RMP could be set at −80 mV using IK1 or constant current injection and APs could be evoked upon stimulation. AP duration differed significantly between current injection methods (p < 0.05) and was shortest with constant current injection and longest with injection of IK1 with strong rectification. With moderate rectification, AP duration at 90% repolarization (APD90) was similar to myocytes with regular non-depolarized RMP, suggesting that a synthetic IK1 with moderate rectification is the most appropriate for human atrial myocytes. Importantly, APs evoked using each injection method were still sensitive to all drugs tested (lidocaine, nifedipine, E-4031, low dose 4-aminopyridine, barium, and apamin), suggesting that the major ionic currents of the atrial cells remained functional. However, certain drug effects were quantitatively dependent on the current injection approach used.
Conclusion: Injection of a synthetic IK1 with moderate rectification facilitates detailed AP measurements in human atrial myocytes. Therefore, dynamic clamp represents a promising tool for testing novel antiarrhythmic drugs.
Introduction
Atrial fibrillation (AF) is the most common type of sustained cardiac arrhythmia in the elderly, with a substantially increasing prevalence anticipated over the next decades (Wakili et al., 2011; Milnes et al., 2012; Colilla et al., 2013; Krijthe et al., 2013). Data from the Framingham Heart Study revealed that AF is independently associated with a 50–90% increase in the risk of death (Benjamin et al., 1998). Clinical AF therapy focuses on prevention of severe complications secondary to AF, in addition to restoration of normal atrial rhythm (Milnes et al., 2012; Kirchhof et al., 2016; January et al., 2019). However, currently available drugs are associated with significant side effects as most of these compounds also affect ventricular function (Milnes et al., 2012). Consequently, current research focuses on atrial-specific pharmacological targeting of ion channels, such as KV1.5, Kir3.1/3.4, small conductance Ca2+-activated K+ (SK) channels, and NaV1.5, in order to generate new therapeutic strategies for AF (Antzelevitch and Burashnikov, 2010; Dobrev and Nattel, 2010; Wakili et al., 2011; Dobrev et al., 2012; Milnes et al., 2012; Peyronnet and Ravens, 2019).
Various in vitro (Papke and Smith-Maxwell, 2009; Ebert et al., 2012; Devalla et al., 2015; Hu et al., 2018) and in vivo animal models (Olgin and Verheule, 2002; Finet et al., 2009; Nishida et al., 2010), including AF models, are available for atrial drug testing, all with their individual strengths and limitations. While these models provide an excellent starting point for atrial and AF-related drug studies, assessments of action potentials (APs) of native human atrial cardiomyocytes remain essential due to distinct inter-species differences in ion channel expression profiles (Varró et al., 2021), and because drugs frequently act through AP shortening or prolonging effects, which may be frequency dependent (Peyronnet and Ravens, 2019). Samples of human left or right atrial appendage can be readily obtained during cardiac surgery (Hatem et al., 2010; Voigt et al., 2015), and studies employing such samples have provided detailed knowledge of human atrial electrophysiology in healthy and diseased states, as reviewed elsewhere (Nattel et al., 2007; Schotten et al., 2011; Dobrev et al., 2012; Heijman et al., 2014). So far, a variety of methods have been used for the electrophysiological analysis of human atrial tissue and cells, including sharp electrode measurements (Trautwein et al., 1962; Ten Eick and Singer, 1979), patch clamp methodology (Van Wagoner et al., 1997; Hoppe and Beuckelmann, 1998), multi-electrode arrays (MEAs) (Kanagaratnam et al., 2006), and voltage-sensitive fluorescence (Krul et al., 2015). In particular, the patch clamp technique, which is considered the gold standard in electrophysiology due to the ability to measure both membrane currents and APs in single cells (Kornreich, 2007), has demonstrated the functional presence of various types of ion channels in human atrial cells. However, AP measurements from isolated human atrial myocytes using patch clamp methodology are limited by the frequent depolarized state of the resting membrane potential (RMP) of these myocytes (Hoppe and Beuckelmann, 1998; Colman et al., 2018). Upon isolation, generally only a fraction of cells, approximately 5–20%, have an RMP sufficiently negative to elicit APs (Amos et al., 1996; Schreieck et al., 2000), and even within this fraction highly depolarized myocytes are frequently excluded from analysis (Loose et al., 2014).
Various patch clamp studies on human atrial myocytes have addressed this major problem of depolarized state by injection of a hyperpolarizing current of constant amplitude, resulting in an RMP near −80 mV (Wang et al., 1993; Le Grand et al., 1994; Bénardeau et al., 1996; Workman et al., 2001; Pau et al., 2003; Workman et al., 2003; Lagrutta et al., 2006; Limberg et al., 2011; Workman et al., 2012; Skibsbye et al., 2014; Schmidt et al., 2015). However, this hyperpolarizing current of constant amplitude also affects the AP repolarization phase to a considerable extent, thereby potentially hampering the assessment of the impact of drugs on AP duration (APD).
Human induced pluripotent stem cell-derived cardiomyocytes (hiPSC-CMs) frequently display similar depolarized membrane potentials due to their relative immaturity (Veerman et al., 2015). In these cells, however, the dynamic clamp methodology (Wilders, 2006; Berecki et al., 2014; Ortega et al., 2018; Verkerk and Wilders, 2021) has been successfully applied to overcome the depolarized state by injection of an in silico inward rectifier K+ current (IK1) (Bett et al., 2013; Meijer van Putten et al., 2015; Verkerk et al., 2017), which is the main current responsible for setting the RMP close to the potassium equilibrium potential (EK) of approximately −85 mV. Consequently, hiPSC-CMs could be stimulated at fixed rates with fast sodium current (INa) driven upstrokes. Furthermore, this dynamic clamp reduced the variability of various AP parameters (Verkerk et al., 2017), thus allowing easier detection of small changes in these parameters.
We here tested whether the injection of a synthetic IK1, which is computed in real time based on the acquired membrane potential and then injected into the cell through the patch pipette, can also improve AP measurements in human left atrial appendage (LAA) myocytes. We used two different IK1 current-voltage relationships, i.e., one with large and one with moderate rectification, and compared the results to those obtained with the injection of a repolarizing current of constant amplitude. In addition, we tested a broad range of ion channel blockers to see if drugs are still able to modify AP characteristics under these conditions and to test if drug effects are dependent on the current injection approach used.
Materials and Methods
Human Cell Preparation
Human Myocardial Tissue
Written informed consent was obtained from all patients, and the study was approved by the local ethics committee and conducted in accordance with the Declaration of Helsinki. LAAs were obtained from eight patients with paroxysmal AF undergoing minimally invasive surgical ablation (mini-maze procedure). Table 1 shows the patient characteristics. Immediately after surgical removal of the specimens, the LAA tissue was placed in chilled (0°C) Ca2+-free MOPS solution, and carried to the laboratory within 30 min. The Ca2+-free MOPS solution was composed of (in mM): 100 NaCl, 10 KCl, 5 glucose, 5 MgSO4, 50 taurine, 11 creatine, 5 MOPS; pH 7.0 (NaOH).
Isolated Human Atrial Myocytes
Single cells were obtained by an enzymatic isolation method modified from Dobrev et al. (2000). In short, fatty tissue and endocardial connective tissue were removed and the remaining tissue was cut into small cubic pieces (≈1 mm3), and washed three times for 10 min in Ca2+-free MOPS solution (37°C). Then, the LAA tissue pieces were incubated for 60 min in Ca2+-free MOPS solution (37°C) to which collagenase A (250 U/ml; Roche) and proteinase XXIV (3.5–7.0 U/ml; Sigma) were added. In these 60 min, the Ca2+ concentration was gradually increased to 0.15 mM by adding Ca2+ three times (i.e., at 15, 40, and 50 min). Subsequently, the tissue pieces were placed in 0.1 mM Ca2+ MOPS solution (37°C) with collagenase A (310 U/ml; Roche). During this last incubation period, the tissue pieces were gently stirred and at regular intervals of 15 min the solution was microscopically examined for the presence of dissociated rod-shaped myocytes. Once single, rod-shaped myocytes were observed (usually after 75 min), cells were removed, and this was repeated every 10 min for 80 min, resulting in several batches of isolated cells. The isolated cells were transferred into Ca2+-free MOPS solution (37°C) containing 1% BSA. Isolated cells were stored for at least 45 min at room temperature in a modified Tyrode’s solution containing (in mM): 145 NaCl, 5.4 KCl, 0.9 CaCl2, 0.5 MgCl2, 5.5 glucose and 5.0 HEPES; pH 7.4 (NaOH).
Electrophysiological Experiments
Recording Procedures and Data Acquisition
The myocytes were placed in a cell chamber mounted on the stage of an inverted microscope (Nikon Diaphot), and allowed to settle for 5 min before they were superfused with Tyrode’s solution containing 1.8 mM CaCl2 and 1 mM MgCl2. Single myocytes having smooth surfaces with cross striations were selected for electrophysiological measurements. APs were recorded using an Axopatch 200B amplifier (Molecular Devices, Sunnyvale, CA, United States). Data acquisition, voltage control, and analysis were accomplished using custom software. All potentials were corrected for the estimated liquid junction potential (Barry and Lynch, 1991). Pipettes (resistance 2–3 MΩ) were pulled from borosilicate glass capillaries (Harvard Apparatus, United Kingdom) using a custom-made vertical microelectrode puller. Cell membrane capacitance (Cm) was estimated by dividing the time constant of the decay of the capacitive transient in response to 5 mV hyperpolarizing voltage clamp steps from −40 mV by the series resistance. Average Cm was 114 ± 9 pF (n = 58; mean ± SEM). Signals were low-pass filtered with a cut-off frequency of 5 kHz and digitized at 40 kHz.
Action Potentials
APs were measured at 36 ± 0.2°C using the amphotericin-perforated patch-clamp method. These were mostly obtained from the 5–8th isolation batch of myocytes, because the myocytes of the first batches had relatively “weak” membranes and frequently displayed loss of seal, unwanted ruptured patch, and/or large “leak currents”. Pipette solution contained (in mM): 125 K-gluc, 20 KCl, 5 NaCl, 0.44 amphotericin-B, 10 HEPES (pH 7.2; KOH). APs were continuously elicited at 1 Hz with square 3-ms, ≈1.2× threshold current pulses through the patch pipette. APs were measured at steady-state baseline conditions and at 5 min after drug application. We analyzed RMP, maximum AP upstroke velocity (dV/dtmax), AP amplitude (APA), AP plateau amplitude (PlatA; measured 20 ms after initiation of the AP upstroke), and APD at 20, 50, and 90% repolarization (APD20, APD50, and APD90, respectively). Parameters from 10 consecutive APs were averaged.
Constant Current Injection and Dynamic Clamp
In the present study, we used constant and dynamic clamp current injections to set the RMP of single atrial myocytes at −80 mV, i.e., close to the RMP in atrial tissue from patients with AF (Christ et al., 2008). Constant current was injected with our standard patch clamp software and the amount of injected current was adapted in every myocyte to set its RMP at −80 mV. A custom dynamic clamp setup was used to inject an in silico IK1, as illustrated in Figure 1, and previously described in detail (Meijer van Putten et al., 2015). In short, we extended our standard patch clamp setup with a separate Real-Time Linux (RT-Linux) based PC that continuously reads in the membrane potential (Vm) of the atrial myocyte and computes the Vm-dependent synthetic IK1. Within the time step ∆t2 of 40 µs, a command potential is generated that, combined with a command potential for any stimulus current, is sent to the patch clamp amplifier to inject this current into the human atrial myocyte. For our synthetic IK1, we used two different IK1 current-voltage relationships.
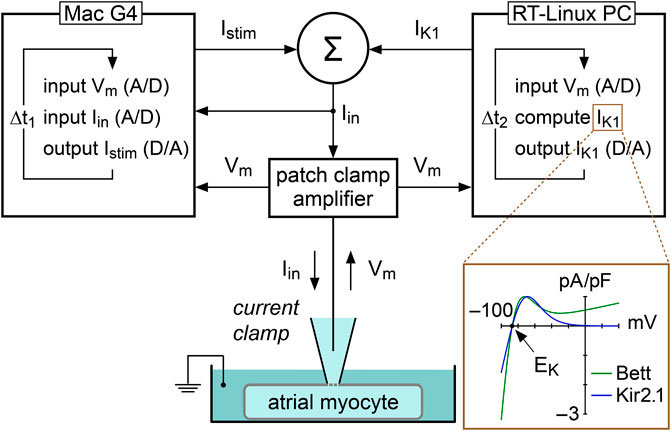
FIGURE 1. Experimental approach to supply a patched atrial myocyte with a synthetic inward rectifier K+ current (IK1). A Real-Time Linux (RT-Linux) based PC computes the synthetic IK1 according to the membrane potential (Vm) that is read into the PC. After addition of any stimulus current (Istim), this IK1 is sent to the patch clamp amplifier, which operates in current clamp mode and injects the net current (Iin) into the patched myocyte. This process is updated with a time step Δt2 of 40 µs. Istim is sent out by the Apple Macintosh (Mac) G4 computer that is used to control the experiment and record both Vm and Iin at a rate of 40 kHz, corresponding with a time step Δt1 of 25 µs. Inset: current–voltage relationship of the synthetic IK1 with moderate rectification (“Bett” current; Bett et al., 2013) and the synthetic IK1 with strong rectification (“Kir2.1” current; Meijer van Putten et al., 2015). EK denotes the potassium equilibrium potential.
One IK1 had a current-voltage relationship based on a fit to data from Kir2.1 channels expressed in HEK-293 cells by Dhamoon et al. (2004), defined “Kir2.1” in the remainder of the study. The Kir2.1 current has a strong rectification, as illustrated in Figure 1 (inset), resulting in absence of a substantial current positive to −20 mV (Dhamoon et al., 2004; Meijer van Putten et al., 2015), and is computed according to:
in which Vm and EK denote the membrane potential and potassium equilibrium potential (in mV), respectively, and EK amounts to −86.9 mV. “A” denotes a scaling factor which is adapted in every experiment to set the RMP at −80 mV. If A is set to 1, the peak outward current density of IK1 amounts to 1 pA/pF.
The other IK1 had a current-voltage relationship showing moderate rectification, defined “Bett” in the rest of the study, referring to the IK1 used in the dynamic clamp study by Bett et al. (2013). Their IK1 has a substantial current at positive potentials, as illustrated in Figure 1 (inset), consistent with IK1 currents observed in human atrial myocytes (Koumi et al., 1995), and is computed according to:
in which Vm and EK denote the membrane potential and potassium equilibrium potential (in mV), respectively, and EK amounts to −86.9 mV. “A” denotes a scaling factor which is adapted in every experiment to set the RMP at −80 mV. If A is set to 1, the peak outward current density of IK1 equals 1 pA/pF.
Drugs
Various general ion channel blockers with well-known effects on human atrial tissue and/or cells were used to test their ability to modify AP characteristics when applying current injection and to validate if potential drug effects depend on the IK1 or constant current injection approach used. Each drug was tested in myocytes isolated from two patients. 4-Aminopyridine (4-AP; Merck) was freshly prepared as 10 mM stock solution in Tyrode’s solution and pH was readjusted to 7.4 (HCl). Nifedipine (Sigma) was prepared as 5 mM stock solution in ethanol, and stored in the dark. E-4031 (Tocris), apamin (Sigma), and BaCl2 (Sigma) were prepared as 5, 0.1, and 1000 mM stock solutions, respectively, in distilled water. All stock solutions and lidocaine (10 mg/ml; Braun) were diluted appropriately before use.
Statistics
All data are presented as mean ± SEM. Potential differences in membrane capacitance between non-depolarized and depolarized myocytes were assessed with an unpaired Student’s t-test. Drug effects were tested with a paired Student’s t-test. Differences between three or more groups were assessed using one-way RM ANOVA followed by pairwise comparison using the Student-Newman-Keuls test. p < 0.05 was considered statistically significant.
Results
Basic Action Potential Characterization of Isolated Human Atrial Myocytes
We patched 58 single human atrial myocytes enzymatically isolated from LAAs that were obtained from eight patients with paroxysmal AF (Table 1). Figure 2A shows the distribution of the RMP of these cells. In only 15 (26%) of these cells, we were able to obtain an RMP sufficiently negative (−70 mV or more negative; Figure 2A, leftmost group of bars) to elicit APs. A typical AP of an intrinsically non-depolarized myocyte is shown in Figure 2B. Cm did not differ significantly between non-depolarized and depolarized myocytes (112 ± 13 vs. 115 ± 12 pF, respectively) and the AP parameters of the 15 intrinsically non-depolarized cells appear in Figure 2D as black bars. The remaining 43 (74%) of these cells were too depolarized to evoke APs with an INa driven upstroke (Gelband et al., 1972; Berecki et al., 2010). Typically, the RMP of these cells was positive to −60 mV (Figure 2A, rightmost group of bars).
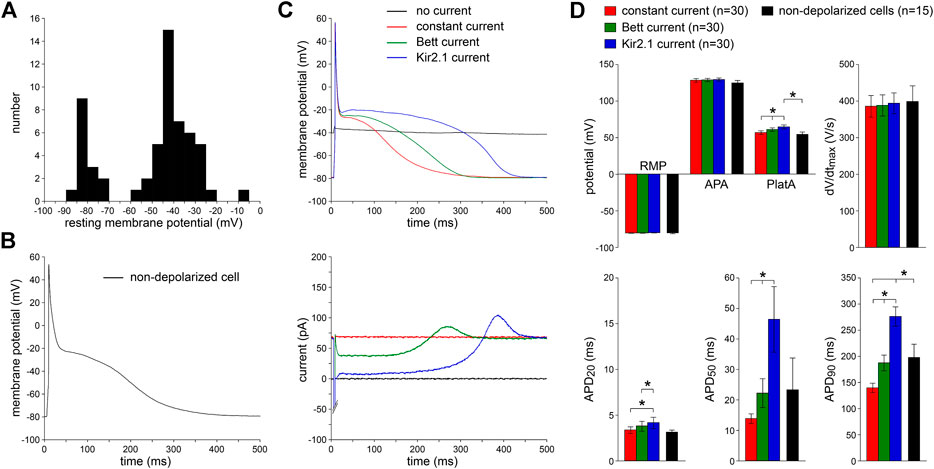
FIGURE 2. Effects of injection of constant repolarizing current or synthetic IK1 (with moderate or strong rectification, supplied through dynamic clamp) on action potentials of enzymatically isolated human atrial myocytes. (A) Resting membrane potential (RMP) of 58 human atrial myocytes in absence of repolarizing current injection. Note the group of 15 non-depolarized myocytes (leftmost group of bars) with an RMP of −70 mV or more negative. (B) Typical action potential (AP), elicited at 1 Hz, of a non-depolarized myocyte in absence of any repolarizing current injection. (C)Top: typical APs elicited at 1 Hz in presence of injected constant or IK1 current within one originally depolarized cell (with an original RMP near −40 mV). Bottom: associated injected currents. Synthetic IK1 supplied as detailed in Figure 1. (D) Average AP parameters of 30 originally depolarized myocytes during constant current and IK1 injections with dynamic clamp. Non-depolarized cells are the 15 cells of panel A with an RMP of −70 mV or more negative in absence of any repolarizing current injection. APA, AP amplitude; PlatA, amplitude of the AP plateau; dV/dtmax, maximum AP upstroke velocity; APD20, APD50, and APD90, AP duration at 20, 50, and 90% repolarization, respectively. *p < 0.05.
Effects of IK1 and Constant Current Injection on Action Potentials
Next, we studied the effects of the two types of dynamic clamp-injected synthetic IK1 currents (Figure 1, inset) as well as constant current injection on the AP morphology of 30 depolarized myocytes. To compare potential differences of these three approaches, all types of current injection were tested in every cell measured. Figure 2C, top panel, shows representative recordings of the membrane potential. The associated traces in Figure 2C, bottom panel, show the injected currents. In the absence of IK1 or constant current injection, the membrane potential was around −40 mV and no APs could be evoked (black lines). Upon switching on the dynamic clamp system with the synthetic “Kir2.1” IK1 [i.e., the IK1 with strong rectification (Figure 1, inset)], the RMP hyperpolarized consistent with the generated outward current (blue lines). We chose an IK1 amplitude that set the RMP at −80 mV, which is close to the RMP in human AF cells previously reported by Christ et al. (2008) and to the RMP that we observed in the 15 intrinsically non-depolarized cells (Figure 2D). At this potential, the synthetic IK1 was still substantial [0.71 ± 0.08 pA/pF (n = 30), consistent with the current-voltage relationship of Kir2.1]. When the synthetic IK1 was added, APs could be elicited, and average AP characteristics with the Kir2.1 injection are summarized Figure 2D (blue bars). The APs had fast upstroke velocities of ≈400 V/s, indicating that they were importantly driven by INa (Berecki et al., 2010). The phase 1 repolarization was fast, resulting in a relatively short APD20 and a plateau potential of around −20 mV, typical for human atrial myocytes with the co-called “Type A” (Bénardeau et al., 1996) and “Type 3” (Dawodu et al., 1996; Workman et al., 2001) AP shape. Thus, the dynamic clamp-injected Kir2.1 IK1 enabled the generation of APs in originally depolarized human atrial myocytes.
We followed the same approach for the injection of the “Bett” IK1, i.e., the IK1 with moderate rectification (Figure 1, inset). The results are shown in Figure 2C,D, as green lines and bars, respectively. Again, the RMP was successfully hyperpolarized to −80 mV [with an IK1 amplitude of 0.73 ± 0.08 pA/pF (n = 30) at this potential], and APs could be evoked. However, the APD was significantly shorter at all repolarization phases and the AP plateau amplitude was significantly lower compared to the APs with Kir2.1 IK1 injection (Figure 2C,D). As expected from the IK1 current-voltage relationships (Figure 1, inset), this was due to the lower amount of rectification resulting in a larger injected outward current during phase 1 and phase 2 of the AP (Figure 2C, bottom). In each of the cells, we also applied the constant current injection (Figure 2C,D, red lines and bars). Again, the RMP was successfully hyperpolarized to −80 mV [with an outward current amplitude of 0.71 ± 0.09 pA/pF (n = 30)]. However, this approach was accompanied by a severe AP shortening compared to APs measured with Kir2.1 IK1 injection (Figure 2C,D), due to the large injected outward current over the complete course of the AP (Figure 2C, bottom).
While all three types of injected current resulted in successful RMP hyperpolarization to −80 mV, and consequently allowed the elicitation of INa driven APs, the APD and AP plateau amplitude differed significantly between the methods used. On average, APD20, APD50, and APD90 were longest using the Kir2.1 current and shortest with the constant current injection (Figure 2D). Neither the APA nor the dV/dtmax differed between the three different current types, but AP plateau amplitude was lowest with constant current injection and highest with injection of Kir2.1 (Figure 2D). To test which approach most closely resembled the physiological conditions, we compared the AP parameters measured with each of the three types of current injection with those of the non-depolarized cells. As shown in Figure 2D, the average AP parameters of the 15 non-depolarized cells (black bars) matched closely with the AP parameters measured with the current injection methods, except that APD90 significantly differed from the Kir2.1 and constant current injection approaches. In addition, the AP plateau amplitude was significantly different between the non-depolarized cells and the cells injected with Kir2.1 IK1.
In summary, both IK1 formulations as well as the constant current injection appeared sufficient to obtain a stable RMP near −80 mV, enabling the generation of INa driven APs. Especially APs obtained with the Bett IK1 were highly similar to those of non-depolarized cells.
Effects of Drugs
Next, we tested a wide range of ion channel blockers to find out whether these still exert their effects when applying current injection to obtain an RMP near −80 mV and whether the effects on AP morphology depend on the injection approach used. We anticipated that drug effects, especially those related to APD, may differ between approaches consequent to the observed APD differences among the latter (Figure 2D).
Lidocaine
First, we tested the effects of 20 µM lidocaine, an antiarrhythmic agent that specifically blocks INa (Bean et al., 1983). Figure 3, shows typical examples (Figure 3A) and the average effects (Figure 3B) of the partial block of INa at this concentration of lidocaine, respectively. Lidocaine significantly reduced dV/dtmax by ≈ 10%, as also illustrated in Figure 3A, inset, and significantly shortened APD at all repolarization phases. AP plateau amplitude was significantly decreased, but RMP and APA were unaltered. The effects of lidocaine did not differ significantly between the IK1 and constant current injections.
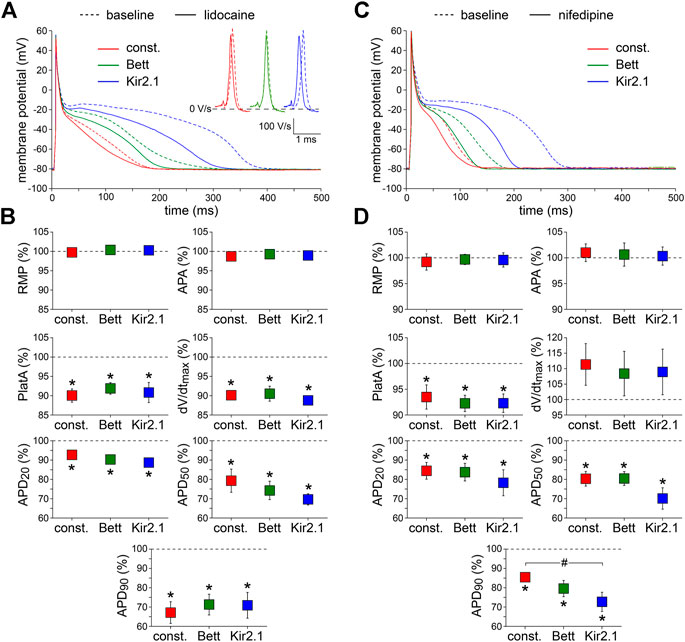
FIGURE 3. Effects of inward current blockade on APs measured with constant and IK1 current injection. (A) Typical APs of one atrial myocyte in absence (baseline) and presence of lidocaine to block the fast Na+ current. Inset: first derivatives of the AP upstrokes, reflecting the amplitude of the fast Na+ current. (B) Average AP parameters measured in five cells in presence of lidocaine. AP parameters are normalized to the AP parameters measured under baseline conditions in the same cell. (C) Typical APs of one atrial myocyte in absence (baseline) and presence of nifedipine to block the L-type Ca2+ current. (D) Average AP parameters measured in six cells in presence of nifedipine. AP parameters are normalized to the AP parameters measured under baseline conditions in the same cell. *p < 0.05, drug vs. baseline (paired t-test); #p < 0.05, drug effects observed with constant current, Bett current, and Kir2.1 current (ANOVA with follow-up test).
Nifedipine
Second, 0.1 µM nifedipine was used to specifically block the L-type Ca2+ current (ICa,L) by ≈ 50% (Eroglu et al., 2020). Figure 3C,D, show representative examples and summarizes the average effects on AP parameters, respectively. Nifedipine did not affect RMP, APA, or dV/dtmax, but significantly reduced APD at all repolarization phases as well as AP plateau amplitude. The nifedipine-induced AP shortening was most prominent with injection of Kir2.1 IK1 (Figure 3C,D), i.e., the approach with the longest baseline APs. In case of APD90, the effects of nifedipine differed significantly between the Kir2.1 IK1 and constant current injection approaches (Figure 3D).
4-Aminopyridine
Third, we assessed the effects of 50 µM 4-AP, which is known to reduce the ultra-rapid component of the delayed rectifier K+ current (IKur) by ≈50% without affecting the transient outward K+ current (Ito1) (Wang et al., 1993). Figure 4A,B, show typical examples of APs and the average effects of the low dose 4-AP, respectively. 4-AP affected all AP parameters except RMP and dV/dtmax. The 4-AP-induced blockade of IKur did not only result in a significant increase in APA and AP plateau amplitude, but also in a significant AP prolongation at most repolarization phases. At 50% repolarization, the increase in APD was significantly larger with the Kir2.1 IK1 compared to the constant current injection method, and at 90% repolarization the increase in APD was significantly larger with the Kir2.1 IK1 compared to both the Bett IK1 and constant current injection methods.
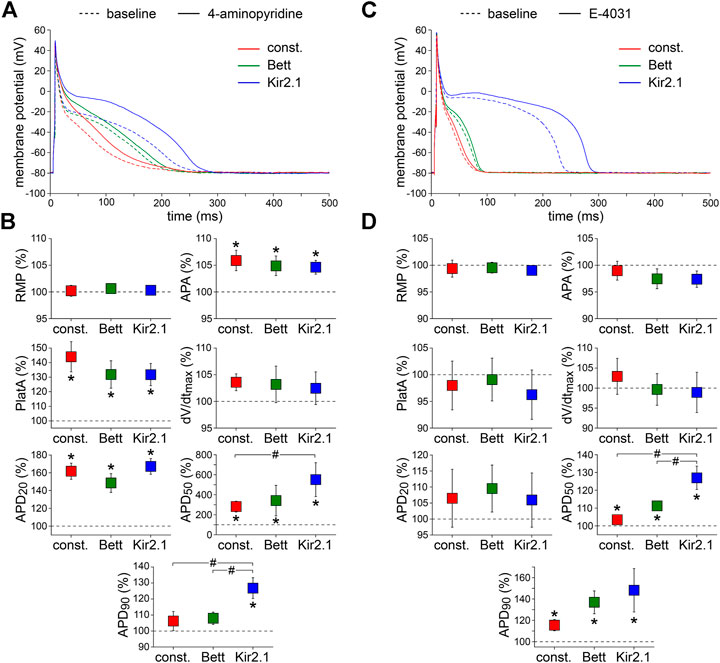
FIGURE 4. Effects of delayed rectifier K+ current blockade on APs measured with constant and IK1 current injection. (A) Typical APs of one atrial myocyte in absence (baseline) and presence of low dose 4-aminopyridine (4-AP) to block the ultra-rapid delayed rectifier K+ current. (B) Average AP parameters measured in five cells in presence of 4-AP. AP parameters are normalized to the AP parameters measured under baseline conditions in the same cell. (C) Typical APs of one atrial myocyte in absence (baseline) and presence of E-4031 to block the rapid delayed rectifier K+ current. (D) Average AP parameters measured in five cells in presence of E-4031. AP parameters are normalized to the AP parameters measured under baseline conditions in the same cell. *p < 0.05, drug vs. baseline (paired t-test); #p < 0.05, drug effects observed with constant current, Bett current, and Kir2.1 current (ANOVA with follow-up test).
E-4031
Fourth, we measured the effects of specific and complete blockade of the rapid component of the delayed rectifier K+ current (IKr), which was induced by 5 µM E-4031 (Sanguinetti and Jurkiewicz, 1990). Figure 4C,D show typical examples and the average effects of E-4031, respectively. E-4031 resulted in a significant increase in both APD50 and APD90, without affecting other AP parameters. The effects of E-4031 on APD50 were most prominent with the Kir2.1 IK1 approach, resulting in a significantly larger increase as compared to the Bett IK1 and constant current injection methods.
Apamin
Fifth, the effects of the bee venom neurotoxin apamin (Banks et al., 1979), which is a highly potent and specific blocker of small conductance Ca2+-activated K+ channels (SK channels) (Xu et al., 2003; Yu et al., 2014), was tested at a concentration of 50 pM. Typical examples and the average effects of SK channel blockade on APs are shown in Figure 5A,B, respectively. Apamin significantly depolarized the RMP, significantly decreased APA and dV/dtmax, and significantly increased APD90 with all three types of current injection. The effects of apamin did not differ significantly between the IK1 and constant current injection approaches.
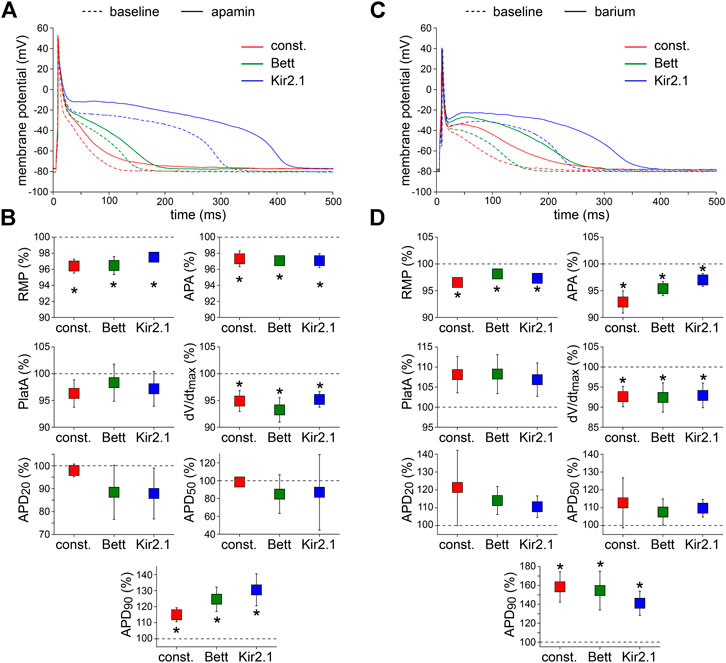
FIGURE 5. Effects of apamin and barium on APs measured with constant and IK1 current injection. (A) Typical APs of one atrial myocyte in absence (baseline) and presence of apamin to block the Ca2+-dependent K+ current. (B) Average AP parameters measured in five cells in presence of apamin. AP parameters are normalized to the AP parameters measured under baseline conditions in the same cell. (C) Typical APs of one atrial myocyte in absence (baseline) and presence of barium to block the native IK1. (D) Average AP parameters measured in four cells in presence of barium. AP parameters are normalized to the AP parameters measured under baseline conditions in the same cell. *p < 0.05, drug vs. baseline (paired t-test).
Barium
Finally, we tested the effects of 1 mM Ba2+, which completely blocks inward rectifier channels (Schram et al., 2003), to find out whether IK1 is still functional in the initially depolarized cells. Figure 5C shows typical AP recordings before and after the addition of Ba2+ to the extracellular solution; Figure 5D summarizes the average AP changes. As illustrated, Ba2+ significantly depolarized the RMP, significantly decreased APA and dV/dtmax, and induced significant prolongation of the APD90. The effects were similar with all three types of current injection.
Discussion
Overview
In accordance with previous studies, we here observed a wide range of RMP values in single human LAA myocytes following isolation. In the depolarized cells (RMP positive to −60 mV), RMP could be set at −80 mV using IK1 or constant current injection, and consequently APs could be evoked upon stimulation. The AP morphology of cells with current injection was quite similar to that of regular non-depolarized cells, especially when using IK1 with “Bett” characteristics, i.e., with moderate rectification. APs evoked using constant current or IK1 injection were still sensitive to all tested drugs (lidocaine, nifedipine, low dose of 4-AP, E-4031, apamin and barium), suggesting that the major ionic currents of the atrial cells were still functional in the initially depolarized myocytes. However, drug effects, especially those related to APD, were in some cases dependent on the current injection approach used.
Current Injection Facilitates Action Potential Recordings From Human Atrial Myocytes
In ≈75% of the freshly isolated human atrial myocytes, we found an RMP positive to −60 mV (Figure 2A). While this is generally observed with patch clamp methodology in this cell type (Amos et al., 1996; Schreieck et al., 2000; Loose et al., 2014), the exact reason for the depolarized state of the RMP still remains to be resolved. It may be intrinsic to human LAA myocytes (Mary-Rabine et al., 1980; Escande et al., 1986), for example due to the presence of the hyperpolarization-activated inward current (If) (Thuringer et al., 1992; Hoppe and Beuckelmann, 1998; Stillitano et al., 2013), the lower density of IK1 compared to ventricular myocytes (Varró et al., 1993), or the presence of an as yet unknown “native” depolarizing conductance. In addition, it may be introduced by the diseased state of the myocytes (Nattel et al., 2007), since the tissue in the present study is obtained from patients suffering from AF. However, depolarized cells are also frequently found in myocytes isolated from tissue obtained from patients undergoing aortocoronary bypass surgery and from donor hearts (Amos et al., 1996; Schreieck et al., 2000), suggesting that the diseased state is a less plausible explanation. In fact, IK1 is upregulated in response to AF (Van Wagoner et al., 1997; Bosch et al., 1999; Workman et al., 2001; Dobrev et al., 2005), suggesting a limited number of depolarized cells, which, however, was not the case, as shown in Figure 2A. It might also result from technical issues related to the patch clamp technique with imperfect seals resulting in “leak currents” that depolarize the RMP (Verkerk and Wilders, 2021). RMP depolarizations would be more pronounced in smaller cells, but in the present study non-depolarized and depolarized myocytes had a similar Cm. Finally, effects from cell isolation procedures cannot be excluded (Varró et al., 2021). We here isolated myocytes enzymatically by the chunk method, and this method results in smaller IK1 densities compared to myocytes isolated using Langendorff perfusion (Hoshino et al., 2012).
The issue of the depolarized state of freshly isolated native human atrial myocytes is similar to that of hiPSC-CMs (Veerman et al., 2015), a relatively new and very promising model to test drugs (Magdy et al., 2018) and genetic disorders (Hoekstra et al., 2012; Brandão et al., 2017) in a human background. Recently, we and others have developed a dynamic clamp method to overcome the depolarized state of hiPSC-CMs [for reviews, see Ortega et al. (2018) and Verkerk and Wilders (2021)]. In the present study, we have extended the applicability of dynamic clamp to native human LAA myocytes of AF patients (Figure 2). We found that a stable RMP of −80 mV in our myocytes was achieved with ≈0.7 pA/pF repolarizing current. This amount of current is largely similar to the 0.3–1.2 pA/pF mentioned in, or calculated from, other studies (Bénardeau et al., 1996; Workman et al., 2001; Limberg et al., 2011; Workman et al., 2012) in human right atrial appendage myocytes. Using IK1 or constant current injection, APs could be evoked upon stimulation. The AP upstroke velocity, dV/dtmax, was high, demonstrating that the AP upstroke is due to activation of INa (Berecki et al., 2010). The AP morphology was largely comparable to the so-called “Type A” and “Type 3” APs, as defined by Bénardeau et al. (1996) and Dawodu et al. (1996), respectively. Thus, the APs had a fast phase 1 repolarization, without a dome, and a plateau at around −20 mV. Other AP types were not observed, consistent with studies of Workman and colleagues (Workman et al., 2001; Workman et al., 2012). The exact reason for the notable finding of Type A/Type 3 APs is unknown. We exclude the dynamic clamp, or the use of the originally depolarized cells, as mechanism because it was also found when injecting Kir2.1 current, which lacks a repolarizing current at positive potentials, as well as in non-depolarized cells, without injection of any repolarizing current. It also may be related to experimental techniques. Here, we have used the perforated patch clamp technique for the AP measurements, while many studies employed the ruptured patch technique, where the pipette solution frequently contains EGTA to buffer intracellular Ca2+. Consequently, Ca2+ modulation of various ion channels and exchangers may be overlooked using the latter approach (Hume and Leblanc, 1988; Koivumäki et al., 2011). This may be of particular relevance for SK channels, the expression of which is increased during the early stages of AF (Ozgen et al., 2007; Qi et al., 2014). Moreover, effects of regional differences and heterogeneity in AP morphology in human atria (Gelband et al., 1972; Gong et al., 2008) may also contribute substantially to our primary finding of Type A/Type 3 APs. Finally, chronic treatment of AF patients with Ca2+ antagonists is known to depress ICa,L, resulting in shorter APs with suppressed plateau amplitudes (Le Grand et al., 1991), but in our study just one out of 8 patients was treated with Ca2+ antagonists (Table 1).
In the present study, we used two different IK1 equations as well as a constant current to set the RMP at a non-depolarized level, which made AP elicitation possible. We found that the APD and AP plateau potential were dependent on the approach of current injection. In the case of the Kir2.1 IK1, which exhibits strong rectification (Figure 1, inset), the outward current at the AP plateau level is negligible (Figure 2C, blue lines). With the Bett IK1, which has a moderate rectification consistent with a more atrial-like IK1 (Bett et al., 2013), the outward current at the plateau level is no longer negligible (Figure 2C, green lines) and consequently shortens the AP and lowers the AP plateau potential substantially (Figure 2D). The constant current injection results in an even larger outward current at the plateau level (Figure 2C, red lines), causing a substantial lowering of the plateau level and most pronounced APD shortening (Figure 2D). These findings indicate the importance of the amount of rectification and agree with previous findings in hiPSC-CMs and computer simulations using mathematical models of hiPSC-CMs and human atrial cells (Dhamoon et al., 2004; Meijer van Putten et al., 2015; Verkerk et al., 2017). With the Bett IK1, AP plateau potential and APD match closely with those of non-depolarized cells (Figure 2D), suggesting that this approach of dynamic clamp current injection is most suitable to overcome the depolarized state of freshly isolated human atrial myocytes.
Drug-Induced Action Potential Changes and Comparison With Previous Studies
We have additionally tested various drugs to find out whether their modulatory effects could still be detected in the APs measured with constant and dynamic clamp injected currents. We have focused on well-established drugs with well-known effects on human atrial tissue and/or cells.
Lidocaine
We found a lidocaine-induced reduction in dV/dtmax in human atrial myocytes, consistent with findings using sharp microelectrode measurements in human atrial fibers (Lauribe et al., 1989) and in agreement with the blockade of peak INa by lidocaine in human atria (Jia et al., 1993). We also observed a decrease of APD in response to lidocaine, which confirms previous findings in human atrial fibers (Lauribe et al., 1989). The AP shortening is likely due to blockade of the slowly inactivating component of INa, in combination with a decrease in INa window current caused by a hyperpolarizing shift in INa voltage dependency (Jia et al., 1993). A lidocaine-induced AP shortening is also found in dog (Burashnikov et al., 2007), but is in contrast with findings in guinea pig, rabbit, and rat, where no effect (Betancourt and Dresel, 1979; Shirayama et al., 1991; Goineau et al., 2012) or even an increase in APD (Goldberg and Roberts, 1981) was observed. These contradictory findings in various species clearly highlight the need to validate drugs in a human myocyte setting.
While the effect on dV/dtmax was relatively mild, the severe APD decrease observed upon lidocaine treatment might be explained by the voltage dependency of lidocaine-induced INa blockade, with a preference of blockade at more positive potentials (Bean et al., 1983; Jia et al., 1993). In our experiments, we did not find a significant difference in lidocaine effects using the various current injections. Previously it was found that the effect of lidocaine on dV/dtmax is more pronounced in the setting of longer APs (Burashnikov et al., 2015), but apparently the APD differences due to the various current injections (Figure 2D) were too small to obtain a detectable effect in upstroke velocities of APs stimulated at 1 Hz.
Nifedipine
In our patch clamp studies, nifedipine shortened the APD, which is in agreement with previous findings in human atrial myocytes (Li and Nattel, 1997; Van Wagoner et al., 1999; Workman et al., 2001). The shortening was smaller compared to these previous studies, but that is likely related to the used concentrations. We used 0.1 µM nifedipine, which is close to the IC50 (Eroglu et al., 2020), while a concentration with almost complete ICa,L blockade was used in the previous studies. One should note that a nifedipine-induced decrease in ICa,L may also influence intracellular Ca2+ dynamics and consequently the sodium-calcium exchange current (INCX), which has a relatively high impact on atrial APs due to its high expression level and the rather negative plateau of the atrial AP (Bénardeau et al., 1996; Carmeliet, 2004). Thus the AP shortening in response to nifedipine is likely not only attributable to reduced ICa,L, but also due to a reduction in INCX (Bénardeau et al., 1996).
The effect of nifedipine on APD was more pronounced using the dynamic clamp-injected Kir2.1 IK1 compared to the Bett and constant current injections. We speculate that this relates to the distinct AP morphologies induced by the different current injection strategies, with a significantly longer APD obtained with Kir2.1 IK1 injection (Figure 2D). Previous studies revealed that drugs modulating APD have an augmented effect in cells with longer APs (Wu et al., 2008; Bárándi et al., 2010; Matsa et al., 2011). This can be explained by the lower net repolarizing current in longer APs, making such APs more sensitive to any change in repolarizing and depolarizing current (Gaur et al., 2020). Indeed, our experiments demonstrate that depending on the current injection approach used, drug effects can be more or less pronounced.
4-Aminopyridine
A low dose of the selective IKur blocker 4-AP (Wang et al., 1993) significantly prolonged APD20, consistent with previous findings in isolated human atrial myocytes of patients undergoing coronary artery bypass surgery (Wang et al., 1993; Li et al., 2008) and in human right atrial trabeculae from patients in sinus rhythm and chronic AF (Wettwer et al., 2004). We also found a significant APD50 prolongation, consistent with the study of Li and colleagues (Li et al., 2008), and this prolongation was even more pronounced with the Kir2.1 IK1 injection. In our experiments, IKur blockade by 4-AP also resulted in a significant APD90 prolongation when the Kir2.1 IK1 injection was used, but not when the Bett or constant current injection was applied. Variable 4-AP effects on APD90 are also reported by others. Li et al. (2008) found no significant change in APD90, but Wang et al. (1993) found a 66% prolongation and Wettwer et al. (2004) reported a 7% increase in tissue of AF patients. In tissue of patients in sinus rhythm, however, the APD90 was shortened in response to 4-AP (Wettwer et al., 2004). Underlying disease with a consequent more positive AP plateau potential in AF tissue is thought to be responsible for these variable findings (Wettwer et al., 2004). Differences in AP plateau potential may also be an explanation for our distinct APD90 findings, with a prolongation only found with Kir2.1 IK1 injection, which typically has the largest AP plateau potential amplitude, PlatA (Figure 2D).
E-4031
In our experiments, we found that E-4031 prolonged the APD50 and APD90. This contrasts with previous findings in human atrial myocytes from patients in sinus rhythm (Wettwer et al., 2004; Skibsbye et al., 2014), although Wettwer and colleagues did mention a tendency to AP prolongation. The reason underlying this apparent discrepancy is unclear, but may be consequent to the diseased state of our cells and/or the use of initially depolarized AF cells in the present study. In addition, it might be related to differences in AP morphology because IKr channel activation during relatively short atrial APs with negative plateau phase will be far from complete (Carmeliet, 2004). Indeed, we found that the AP prolonging effect of E-4031 at 50% repolarization was more pronounced using the Kir2.1 IK1, likely because there is more time for channel activation in the setting of the relatively long APD50 associated with the Kir2.1 IK1 (Figure 2D). This, in combination with the lower net current at the plateau of the Kir2.1 IK1 APs, likely makes the effects of drugs that modulate the plateau current more easily detectable.
Apamin
Apamin did not affect APD50, but prolonged APD90 consistent with previously observed SK blockade effects in human single atrial myocytes and tissue (Xu et al., 2003; Skibsbye et al., 2014). In addition, we found that apamin depolarized the RMP, and decreased APA and dV/dtmax in agreement with NS8593-induced SK current reduction measured with microelectrodes in human atrial tissue (Skibsbye et al., 2014). Since the used apamin concentration does not block INa (Yu et al., 2014), the decreased dV/dtmax observed by us is likely the consequence of the RMP depolarization resulting in more inactivated INa channels (Berecki et al., 2010). The latter could also explain the observed decrease in APA. Apamin-induced AP prolongation has also been observed in mice (Xu et al., 2003), but not in dog and rat (Nagy et al., 2009), further emphasizing the need for experiments on human cells and tissue. In the present study, we have used cells from paroxysmal AF patients and there is growing evidence that SK channels may contribute to AF-induced APD abbreviation. Some laboratories report upregulation of SK channel expression in chronic AF, but this is not a consistent finding [Peyronnet and Ravens (2019) and Shamsaldeen et al. (2019), and primary references cited therein]. Therefore, we cannot exclude that the apamin-induced effects we observed are influenced by the diseased state of the tissue used.
Barium
We here demonstrate a small, but significant RMP depolarization upon application of Ba2+, which is consistent with previous findings in human right atrial appendage (Escande et al., 1986). This indicates that the native IK1 was still functionally present in the initially depolarized cells and that the injected current does not obscure changes in IK1 completely. However, spontaneous activity was not observed in the measured cells, as occurred in a computer model of a guinea pig ventricular myocyte upon an 81% decrease in IK1 (Silva and Rudy, 2003). On the other hand, the application of 0.05–5 mM Ba2+ usually did not induce automatic activity in single ventricular myocytes obtained from adult guinea pig hearts (Imoto et al., 1987). Anyhow, one should keep in mind that drug effects on the native IK1 will be masked by the in silico injection of IK1 to a certain extent, simply because the in silico IK1 is not affected by the drug. The observed RMP depolarization may also lead to inactivation of INa channels and thus underlie the observed decrease in APA and dV/dtmax (Berecki et al., 2010). Additionally, we here observed a substantial Ba2+-induced increase in APD90 (Figure 5C,D), consistent with previous findings in human right atrial appendage and papillary muscle (Escande et al., 1986; Jost et al., 2013). The effects of IK1 blockade on the APs were independent of the used current injections.
Limitations
We demonstrated that the APs of initially depolarized atrial myocytes, with three different types of current injection to arrive at a stable RMP near −80 mV, were sensitive to all drugs tested. This indicates that the major ionic currents were still functional in the initially depolarized cells. Drugs were not tested on intrinsically non-depolarized cells because of the limited number of such cells. Therefore, we cannot exclude that drug effects differ between initially depolarized myocytes on the one hand and intrinsically non-depolarized cells on the other hand. Frequency dependence was not tested in initially depolarized myocytes due to the already lengthy experimental protocol of the three current injection approaches in combination with the drug effect evaluations. Further studies are required to test whether the frequency dependencies of the initially depolarized myocytes and the non-depolarized ones are similar.
In our patch clamp experiments, we injected an in silico IK1 or constant current of ≈0.7 pA/pF to obtain a stable RMP near −80 mV in LAA myocytes of AF patients. It has been consistently demonstrated, in both animal models and humans, that IK1 is upregulated during AF (Van Wagoner et al., 1997; Bosch et al., 1999; Workman et al., 2001; Dobrev et al., 2005), resulting in a 4–6 mV RMP hyperpolarization in AF tissue. Thus, if myocytes of patients in sinus rhythm would be included, the IK1 density must likely be adapted with also consequent effects on the AP repolarization. Nevertheless, this limitation could also be considered as strength of the dynamic clamp to systematically study the effects of remodeled IK1 in human AF, but further studies are needed to address this issue in detail.
Conclusion
Patch-clamp recordings of APs from human atrial myocytes can be optimized through dynamic clamp in order to provide these cells with a substantial IK1 resulting in a close-to-physiological RMP. The APs measured using moderate IK1 rectification (“Bett” IK1) matched closely those of non-depolarized cells, but effects of AP prolonging or shortening drugs are more pronounced using the dynamic clamp-injected IK1 with strong IK1 rectification (“Kir2.1” IK1) for drugs blocking ICa,L, IKur, or IKr. Dynamic clamp therefore constitutes an appropriate tool for studying AP properties of human atrial myocytes, especially for the purpose of testing novel antiarrhythmic drugs.
Data Availability Statement
The raw data supporting the conclusions of this article will be made available by the authors, without undue reservation.
Ethics Statement
The studies involving human participants were reviewed and approved by the Ethics Committee of the Amsterdam University Medical Centers, University of Amsterdam. The patients/participants provided their written informed consent to participate in this study.
Author Contributions
AV and RW conceived, designed, and performed the experiments, and analyzed the data. AV, RW, CR, and GM wrote the paper. GM and MK isolated the human atrial myocytes and performed the patient administration. JZ installed the RT-Linux system and wrote the dynamic clamp software, AD coordinated and performed the surgeries, and JG contributed to scientific discussions.
Funding
This work was supported by two Innovational Research Incentives Scheme Vidi grants from the Netherlands Organisation for Health Research and Development (ZonMw 91714371 to CR and ZonMw 016.146.310 to JG).
Conflict of Interest
The authors declare that the research was conducted in the absence of any commercial or financial relationships that could be construed as a potential conflict of interest.
Glossary
4-AP 4-aminopyridine
ACE angiotensin-converting enzyme
AF atrial fibrillation
AP action potential
APA action potential amplitude
APD action potential duration
APD20 action potential duration at 20% repolarization
APD50 action potential duration at 50% repolarization
APD90 action potential duration at 90% repolarization
Cm cell membrane capacitance
dV/dtmax maximum action potential upstroke velocity
EK potassium equilibrium potential
hiPSC-CMs human induced pluripotent stem cell-derived cardiomyocytes
IC50 half-maximal inhibitory concentration
ICa,L L-type Ca2+ current
If hyperpolarization-activated inward current
Iin injected current
IK1 inward rectifier K+ current
IKr rapid component of the delayed rectifier K+ current
IKur ultra-rapid component of the delayed rectifier K+ current
INa fast sodium current
INCX sodium-calcium exchange current
Istim stimulus current
Ito1 transient outward K+ current
LAA left atrial appendage
MEA multi-electrode array
PlatA amplitude of the action potential plateau
RMP resting membrane potential
RT-Linux Real-Time Linux
SK channels small conductance Ca2+-activated K+ channels
Vm membrane potential
References
Amos, G. J., Wettwer, E., Metzger, F., Li, Q., Himmel, H. M., and Ravens, U. (1996). Differences between outward currents of human atrial and subepicardial ventricular myocytes. J. Physiol. 491, 31–50. doi:10.1113/jphysiol.1996.sp021194
Antzelevitch, C., and Burashnikov, A. (2010). Atrial-selective sodium channel block as a novel strategy for the management of atrial fibrillation. Ann. N. Y. Acad. Sci. 1188, 78–86. doi:10.1111/j.1749-6632.2009.05086.x
Bárándi, L., Virág, L., Jost, N., Horváth, Z., Koncz, I., Papp, R., et al. (2010). Reverse rate-dependent changes are determined by baseline action potential duration in mammalian and human ventricular preparations. Basic Res. Cardiol. 105, 315–323. doi:10.1007/s00395-009-0082-7
Banks, B. E., Brown, C., Burgess, G. M., Burnstock, G., Claret, M., Cocks, T. M., et al. (1979). Apamin blocks certain neurotransmitter-induced increases in potassium permeability. Nature 282, 415–417. doi:10.1038/282415a0
Barry, P. H., and Lynch, J. W. (1991). Liquid junction potentials and small cell effects in patch-clamp analysis. J. Membr. Biol. 121, 101–117. doi:10.1007/BF01870526
Bean, B. P., Cohen, C. J., and Tsien, R. W. (1983). Lidocaine block of cardiac sodium channels. J. Gen. Physiol. 81, 613–642. doi:10.1085/jgp.81.5.613
Bénardeau, A., Hatem, S. N., Rücker-Martin, C., Le Grand, B., Macé, L., Dervanian, P., et al. (1996). Contribution of Na+/Ca2+ exchange to action potential of human atrial myocytes. Am. J. Physiol. 271, H1151–H1161. doi:10.1152/ajpheart.1996.271.3.H1151
Benjamin, E. J., Wolf, P. A., D’Agostino, R. B., Silbershatz, H., Kannel, W. B., and Levy, D. (1998). Impact of atrial fibrillation on the risk of death: the Framingham Heart Study. Circulation 98, 946–952. doi:10.1161/01.cir.98.10.946
Berecki, G., Wilders, R., De Jonge, B., Van Ginneken, A. C. G., and Verkerk, A. O. (2010). Re-evaluation of the action potential upstroke velocity as a measure of the Na+ current in cardiac myocytes at physiological conditions. PLoS One 5, e15772. doi:10.1371/journal.pone.0015772
Berecki, G., Verkerk, A. O., Van Ginneken, A. C. G., and Wilders, R. (2014). Dynamic clamp as a tool to study the functional effects of individual membrane currents. Methods Mol. Biol. 1183, 309–326. doi:10.1007/978-1-4939-1096-0_20
Betancourt, O. J., and Dresel, P. E. (1979). Interaction of lidocaine and calcium on the electrical characteristics of rabbit atria. J. Pharmacol. Exp. Ther. 210, 64–69.
Bett, G. C. L., Kaplan, A. D., Lis, A., Cimato, T. R., Tzanakakis, E. S., Zhou, Q., et al. (2013). Electronic “expression” of the inward rectifier in cardiocytes derived from human-induced pluripotent stem cells. Heart Rhythm 10, 1903–1910. doi:10.1016/j.hrthm.2013.09.061
Bosch, R. F., Zeng, X., Grammer, J. B., Popovic, K., Mewis, C., and Kühlkamp, V. (1999). Ionic mechanisms of electrical remodeling in human atrial fibrillation. Cardiovasc. Res. 44, 121–131. doi:10.1016/s0008-6363(99)00178-9
Brandão, K. O., Tabel, V. A., Atsma, D. E., Mummery, C. L., and Davis, R. P. (2017). Human pluripotent stem cell models of cardiac disease: from mechanisms to therapies. Dis. Model. Mech. 10, 1039–1059. doi:10.1242/dmm.030320
Burashnikov, A., Belardinelli, L., and Antzelevitch, C. (2015). Inhibition of IKr potentiates development of atrial-selective INa block leading to effective suppression of atrial fibrillation. Heart Rhythm 12, 836–844. doi:10.1016/j.hrthm.2014.12.033
Burashnikov, A., Di Diego, J. M., Zygmunt, A. C., Belardinelli, L., and Antzelevitch, C. (2007). Atrium-selective sodium channel block as a strategy for suppression of atrial fibrillation: differences in sodium channel inactivation between atria and ventricles and the role of ranolazine. Circulation 116, 1449–1457. doi:10.1161/CIRCULATIONAHA.107.704890
Carmeliet, E. (2004). Intracellular Ca2+ concentration and rate adaptation of the cardiac action potential. Cell Calcium 35, 557–573. doi:10.1016/j.ceca.2004.01.010
Christ, T., Wettwer, E., Voigt, N., Hála, O., Radicke, S., Matschke, K., et al. (2008). Pathology-specific effects of the IKur/Ito/IK,ACh blocker AVE0118 on ion channels in human chronic atrial fibrillation. Br. J. Pharmacol. 154, 1619–1630. doi:10.1038/bjp.2008.209
Colilla, S., Crow, A., Petkun, W., Singer, D. E., Simon, T., and Liu, X. (2013). Estimates of current and future incidence and prevalence of atrial fibrillation in the U.S. adult population. Am. J. Cardiol. 112, 1142–1147. doi:10.1016/j.amjcard.2013.05.063
Colman, M. A., Saxena, P., Kettlewell, S., and Workman, A. J. (2018). Description of the human atrial action potential derived from a single, congruent data source: novel computational models for integrated experimental-numerical study of atrial arrhythmia mechanisms. Front. Physiol. 9, 1211. doi:10.3389/fphys.2018.01211
Dawodu, A. A., Monti, F., Iwashiro, K., Schiariti, M., Chiavarelli, R., and Puddu, P. E. (1996). The shape of human atrial action potential accounts for different frequency-related changes in vitro. Int. J. Cardiol. 54, 237–249. doi:10.1016/0167-5273(96)02605-8
Devalla, H. D., Schwach, V., Ford, J. W., Milnes, J. T., El-Haou, S., Jackson, C., et al. (2015). Atrial-like cardiomyocytes from human pluripotent stem cells are a robust preclinical model for assessing atrial-selective pharmacology. EMBO Mol. Med. 7, 394–410. doi:10.15252/emmm.201404757
Dhamoon, A. S., Pandit, S. V., Sarmast, F., Parisian, K. R., Guha, P., Li, Y., et al. (2004). Unique Kir2.x properties determine regional and species differences in the cardiac inward rectifier K+ current. Circ. Res. 94, 1332–1339. doi:10.1161/01.RES.0000128408.66946.67
Dobrev, D., Carlsson, L., and Nattel, S. (2012). Novel molecular targets for atrial fibrillation therapy. Nat. Rev. Drug Discov. 11, 275–291. doi:10.1038/nrd3682
Dobrev, D., Friedrich, A., Voigt, N., Jost, N., Wettwer, E., Christ, T., et al. (2005). The G protein-gated potassium current IK,ACh is constitutively active in patients with chronic atrial fibrillation. Circulation 112, 3697–3706. doi:10.1161/CIRCULATIONAHA.105.575332
Dobrev, D., and Nattel, S. (2010). New antiarrhythmic drugs for treatment of atrial fibrillation. Lancet 375, 1212–1223. doi:10.1016/S0140-6736(10)60096-7
Dobrev, D., Wettwer, E., Himmel, H. M., Kortner, A., Kuhlisch, E., Schüler, S., et al. (2000). G-Protein β3-subunit 825T allele is associated with enhanced human atrial inward rectifier potassium currents. Circulation 102, 692–697. doi:10.1161/01.cir.102.6.692
Ebert, A. D., Liang, P., and Wu, J. C. (2012). Induced pluripotent stem cells as a disease modeling and drug screening platform. J. Cardiovasc. Pharmacol. 60, 408–416. doi:10.1097/FJC.0b013e318247f642
Eroglu, T. E., Mohr, G. H., Blom, M. T., Verkerk, A. O., Souverein, P. C., Torp-Pedersen, C., et al. (2020). Differential effects on out-of-hospital cardiac arrest of dihydropyridines: real-world data from population-based cohorts across two European countries. Eur. Heart J. Cardiovasc. Pharmacother. 6, 347–355. doi:10.1093/ehjcvp/pvz038
Escande, D., Coraboeuf, E., Planché, C., and Lacour-Gayet, F. (1986). Effects of potassium conductance inhibitors on spontaneous diastolic depolarization and abnormal automaticity in human atrial fibers. Basic Res. Cardiol. 81, 244–257. doi:10.1007/BF01907407
Finet, J. E., Rosenbaum, D. S., and Donahue, J. K. (2009). Information learned from animal models of atrial fibrillation. Cardiol. Clin. 27, 45–54. doi:10.1016/j.ccl.2008.09.005
Gaur, N., Ortega, F., Verkerk, A. O., Mengarelli, I., Krogh-Madsen, T., Christini, D. J., et al. (2020). Validation of quantitative measure of repolarization reserve as a novel marker of drug induced proarrhythmia. J. Mol. Cell Cardiol. 145, 122–132. doi:10.1016/j.yjmcc.2020.04.019
Gelband, H., Bush, H. L., Rosen, M. R., Myerburg, R. J., and Hoffman, B. F. (1972). Electrophysiologic properties of isolated preparations of human atrial myocardium. Circ. Res. 30, 293–300. doi:10.1161/01.res.30.3.293
Goineau, S., Castagné, V., Guillaume, P., and Froget, G. (2012). The comparative sensitivity of three in vitro safety pharmacology models for the detection of lidocaine-induced cardiac effects. J. Pharmacol. Toxicol. Methods 66, 52–58. doi:10.1016/j.vascn.2012.06.001
Goldberg, P. B., and Roberts, J. (1981). Age-related changes in rat atrial sensitivity to lidocaine. J. Gerontol. 36, 520–528. doi:10.1093/geronj/36.5.520
Gong, D., Zhang, Y., Cai, B., Meng, Q., Jiang, S., Li, X., et al. (2008). Characterization and comparison of Na+, K+ and Ca2+ currents between myocytes from human atrial right appendage and atrial septum. Cell. Physiol. Biochem. 21, 385–394. doi:10.1159/000129631
Hatem, S. N., Coulombe, A., and Balse, E. (2010). Specificities of atrial electrophysiology: clues to a better understanding of cardiac function and the mechanisms of arrhythmias. J. Mol. Cel. Cardiol. 48, 90–95. doi:10.1016/j.yjmcc.2009.08.029
Heijman, J., Voigt, N., Nattel, S., and Dobrev, D. (2014). Cellular and molecular electrophysiology of atrial fibrillation initiation, maintenance, and progression. Circ. Res. 114, 1483–1499. doi:10.1161/CIRCRESAHA.114.302226
Hoekstra, M., Mummery, C. L., Wilde, A. A. M., Bezzina, C. R., and Verkerk, A. O. (2012). Induced pluripotent stem cell derived cardiomyocytes as models for cardiac arrhythmias. Front. Physiol. 3, 346. doi:10.3389/fphys.2012.00346
Hoppe, U. C., and Beuckelmann, D. J. (1998). Characterization of the hyperpolarization-activated inward current in isolated human atrial myocytes. Cardiovasc. Res. 38, 788–801. doi:10.1016/s0008-6363(98)00047-9
Hoshino, S., Omatsu-Kanbe, M., Nakagawa, M., and Matsuura, H. (2012). Postnatal developmental decline in Ik1 in mouse ventricular myocytes isolated by the Langendorff perfusion method: comparison with the chunk method. Pflugers Arch. 463, 649–668. doi:10.1007/s00424-012-1084-0
Hu, J., Han, J., Li, H., Zhang, X., Liu, L. L., Chen, F., et al. (2018). Human embryonic kidney 293 cells: a vehicle for biopharmaceutical manufacturing, structural biology, and electrophysiology. Cells Tissues Organs. 205, 1–8. doi:10.1159/000485501
Hume, J. R., and Leblanc, R. N. (1988). A whole-cell patch clamp technique which minimizes cell dialysis. Mol. Cel. Biochem. 80, 49–57. doi:10.1007/BF00231003
Imoto, Y., Ehara, T., and Matsuura, H. (1987). Voltage- and time-dependent block of ik1 underlying Ba2+-induced ventricular automaticity. Am. J. Physiol. 252, H325–H333. doi:10.1152/ajpheart.1987.252.2.H325
January, C. T., Wann, L. S., Calkins, H., Chen, L. Y., Cigarroa, J. E., Cleveland, J. C., et al. (2019). 2019 AHA/ACC/HRS focused update of the 2014 AHA/ACC/HRS guideline for the management of patients with atrial fibrillation: a report of the American College of Cardiology/American Heart Association task force on clinical practice guidelines and the Heart Rhythm Society. J. Am. Coll. Cardiol. 74, 104–132. doi:10.1016/j.jacc.2019.01.011
Jia, H., Furukawa, T., Singer, D. H., Sakakibara, Y., Eager, S., Backer, C., et al. (1993). Characteristics of lidocaine block of sodium channels in single human atrial cells. J. Pharmacol. Exp. Ther. 264, 1275–1284.
Jost, N., Virág, L., Comtois, P., Ordög, B., Szuts, V., Seprényi, G., et al. (2013). Ionic mechanisms limiting cardiac repolarization reserve in humans compared to dogs. J. Physiol. 591, 4189–4206. doi:10.1113/jphysiol.2013.261198
Kanagaratnam, P., Dupont, E., Rothery, S., Coppen, S., Severs, N. J., and Peters, N. S. (2006). Human atrial conduction and arrhythmogenesis correlates with conformational exposure of specific epitopes on the connexin40 carboxyl tail. J. Mol. Cel. Cardiol. 40, 675–687. doi:10.1016/j.yjmcc.2006.01.002
Kirchhof, P., Benussi, S., Kotecha, D., Ahlsson, A., Atar, D., Casadei, B., et al. (2016). 2016 ESC guidelines for the management of atrial fibrillation developed in collaboration with EACTS. Eur. Heart J. 37, 2893–2962. doi:10.1093/eurheartj/ehw210
Koivumäki, J. T., Korhonen, T., and Tavi, P. (2011). Impact of sarcoplasmic reticulum calcium release on calcium dynamics and action potential morphology in human atrial myocytes: a computational study. PLoS Comput. Biol. 7, e1001067. doi:10.1371/journal.pcbi.1001067
Kornreich, B. G. (2007). The patch clamp technique: principles and technical considerations. J. Vet. Cardiol. 9, 25–37. doi:10.1016/j.jvc.2007.02.001
Koumi, S., Backer, C. L., and Arentzen, C. E. (1995). Characterization of inwardly rectifying K+ channel in human cardiac myocytes. Alterations in channel behavior in myocytes isolated from patients with idiopathic dilated cardiomyopathy. Circulation 92, 164–174. doi:10.1161/01.cir.92.2.164
Krijthe, B. P., Kunst, A., Benjamin, E. J., Lip, G. Y., Franco, O. H., Hofman, A., et al. (2013). Projections on the number of individuals with atrial fibrillation in the European Union, from 2000 to 2060. Eur. Heart J. 34, 2746–2751. doi:10.1093/eurheartj/eht280
Krul, S. P. J., Berger, W. R., Veldkamp, M. W., Driessen, A. H. G., Wilde, A. A. M., Deneke, T., et al. (2015). Treatment of atrial and ventricular arrhythmias through autonomic modulation. JACC Clin. Electrophysiol. 1, 496–508. doi:10.1016/j.jacep.2015.09.013
Lagrutta, A., Wang, J., Fermini, B., and Salata, J. J. (2006). Novel, potent inhibitors of human Kv1.5 K+ channels and ultrarapidly activating delayed rectifier potassium current. J. Pharmacol. Exp. Ther. 317, 1054–1063. doi:10.1124/jpet.106.101162
Lauribe, P., Escande, D., Nottin, R., and Coraboeuf, E. (1989). Electrical activity of human atrial fibres at frequencies corresponding to atrial flutter. Cardiovasc. Res. 23, 159–168. doi:10.1093/cvr/23.2.159
Le Grand, B., Hatem, S., Deroubaix, E., Couetil, J. P., and Coraboeuf, E. (1991). Calcium current depression in isolated human atrial myocytes after cessation of chronic treatment with calcium antagonists. Circ. Res. 69, 292–300. doi:10.1161/01.res.69.2.292
Le Grand, B., Hatem, S., Deroubaix, E., Couétil, J. P., and Coraboeuf, E. (1994). Depressed transient outward and calcium currents in dilated human atria. Cardiovasc. Res. 28, 548–556. doi:10.1093/cvr/28.4.548
Li, G. R., and Nattel, S. (1997). Properties of human atrial ICa at physiological temperatures and relevance to action potential. Am. J. Physiol. 272, H227–H235. doi:10.1152/ajpheart.1997.272.1.H227
Li, G. R., Wang, H. B., Qin, G. W., Jin, M. W., Tang, Q., Sun, H. Y., et al. (2008). Acacetin, a natural flavone, selectively inhibits human atrial repolarization potassium currents and prevents atrial fibrillation in dogs. Circulation 117, 2449–2457. doi:10.1161/CIRCULATIONAHA.108.769554
Limberg, S. H., Netter, M. F., Rolfes, C., Rinné, S., Schlichthörl, G., Zuzarte, M., et al. (2011). TASK-1 channels may modulate action potential duration of human atrial cardiomyocytes. Cel. Physiol. Biochem. 28, 613–624. doi:10.1159/000335757
Loose, S., Mueller, J., Wettwer, E., Knaut, M., Ford, J., Milnes, J., et al. (2014). Effects of IKur blocker MK-0448 on human right atrial action potentials from patients in sinus rhythm and in permanent atrial fibrillation. Front. Pharmacol. 5, 26. doi:10.3389/fphar.2014.00026
Magdy, T., Schuldt, A. J. T., Wu, J. C., Bernstein, D., and Burridge, P. W. (2018). Human induced pluripotent stem cell (hiPSC)-derived cells to assess drug cardiotoxicity: opportunities and problems. Annu. Rev. Pharmacol. Toxicol. 58, 83–103. doi:10.1146/annurev-pharmtox-010617-053110
Mary-Rabine, L., Hordof, A. J., Danilo, P., Malm, J. R., and Rosen, M. R. (1980). Mechanisms for impulse initiation in isolated human atrial fibers. Circ. Res. 47, 267–277. doi:10.1161/01.res.47.2.267
Matsa, E., Rajamohan, D., Dick, E., Young, L., Mellor, I., Staniforth, A., et al. (2011). Drug evaluation in cardiomyocytes derived from human induced pluripotent stem cells carrying a long QT syndrome type 2 mutation. Eur. Heart J. 32, 952–962. doi:10.1093/eurheartj/ehr073
Meijer van Putten, R. M., Mengarelli, I., Guan, K., Zegers, J. G., Van Ginneken, A. C. G., Verkerk, A. O., et al. (2015). Ion channelopathies in human induced pluripotent stem cell derived cardiomyocytes: a dynamic clamp study with virtual Ik1. Front. Physiol. 6, 7. doi:10.3389/fphys.2015.00007
Milnes, J. T., Madge, D. J., and Ford, J. W. (2012). New pharmacological approaches to atrial fibrillation. Drug Discov. Today 17, 654–659. doi:10.1016/j.drudis.2012.02.007
Nagy, N., Szuts, V., Horváth, Z., Seprényi, G., Farkas, A. S., Acsai, K., et al. (2009). Does small-conductance calcium-activated potassium channel contribute to cardiac repolarization? J. Mol. Cel. Cardiol. 47, 656–663. doi:10.1016/j.yjmcc.2009.07.019
Nattel, S., Maguy, A., Le Bouter, S., and Yeh, Y. H. (2007). Arrhythmogenic ion-channel remodeling in the heart: heart failure, myocardial infarction, and atrial fibrillation. Physiol. Rev. 87, 425–456. doi:10.1152/physrev.00014.2006
Nishida, K., Michael, G., Dobrev, D., and Nattel, S. (2010). Animal models for atrial fibrillation: clinical insights and scientific opportunities. Europace 12, 160–172. doi:10.1093/europace/eup328
Olgin, J. E., and Verheule, S. (2002). Transgenic and knockout mouse models of atrial arrhythmias. Cardiovasc. Res. 54, 280–286. doi:10.1016/s0008-6363(02)00225-0
Ortega, F. A., Grandi, E., Krogh-Madsen, T., and Christini, D. J. (2017). Applications of dynamic clamp to cardiac arrhythmia research: role in drug target discovery and safety pharmacology testing. Front. Physiol. 8, 1099. doi:10.3389/fphys.2017.01099
Ozgen, N., Dun, W., Sosunov, E. A., Anyukhovsky, E. P., Hirose, M., Duffy, H. S., et al. (2007). Early electrical remodeling in rabbit pulmonary vein results from trafficking of intracellular SK2 channels to membrane sites. Cardiovasc. Res. 75, 758–769. doi:10.1016/j.cardiores.2007.05.008
Papke, R. L., and Smith-Maxwell, C. (2009). High throughput electrophysiology with Xenopus oocytes. Comb. Chem. High Throughput Screen. 12, 38–50. doi:10.2174/138620709787047975
Pau, D., Workman, A. J., Kane, K. A., and Rankin, A. C. (2003). Electrophysiological effects of 5-hydroxytryptamine on isolated human atrial myocytes, and the influence of chronic β-adrenoceptor blockade. Br. J. Pharmacol. 140, 1434–1441. doi:10.1038/sj.bjp.0705553
Peyronnet, R., and Ravens, U. (2019). Atria-selective antiarrhythmic drugs in need of alliance partners. Pharmacol. Res. 145, 104262. doi:10.1016/j.phrs.2019.104262
Qi, X. Y., Diness, J. G., Brundel, B. J., Zhou, X. B., Naud, P., Wu, C. T., et al. (2014). Role of small-conductance calcium-activated potassium channels in atrial electrophysiology and fibrillation in the dog. Circulation 129, 430–440. doi:10.1161/CIRCULATIONAHA.113.003019
Sanguinetti, M. C., and Jurkiewicz, N. K. (1990). Two components of cardiac delayed rectifier K+ current. Differential sensitivity to block by class III antiarrhythmic agents. J. Gen. Physiol. 96, 195–215. doi:10.1085/jgp.96.1.195
Schmidt, C., Wiedmann, F., Voigt, N., Zhou, X. B., Heijman, J., Lang, S., et al. (2015). Upregulation of K2P3.1 K+ current causes action potential shortening in patients with chronic atrial fibrillation. Circulation 132, 82–92. doi:10.1161/CIRCULATIONAHA.114.012657
Schotten, U., Verheule, S., Kirchhof, P., and Goette, A. (2011). Pathophysiological mechanisms of atrial fibrillation: a translational appraisal. Physiol. Rev. 91, 265–325. doi:10.1152/physrev.00031.2009
Schram, G., Pourrier, M., Wang, Z., White, M., and Nattel, S. (2003). Barium block of Kir2 and human cardiac inward rectifier currents: evidence for subunit-heteromeric contribution to native currents. Cardiovasc. Res. 59, 328–338. doi:10.1016/S0008-6363(03)00366-3
Schreieck, J., Wang, Y., Overbeck, M., Schömig, A., and Schmitt, C. (2000). Altered transient outward current in human atrial myocytes of patients with reduced left ventricular function. J. Cardiovasc. Electrophysiol. 11, 180–192. doi:10.1111/j.1540-8167.2000.tb00318.x
Shamsaldeen, Y. A., Culliford, L., Clout, M., James, A. F., Ascione, R., Hancox, J. C., et al. (2019). Role of SK channel activation in determining the action potential configuration in freshly isolated human atrial myocytes from the SKArF study. Biochem. Biophys. Res. Commun. 512, 684–690. doi:10.1016/j.bbrc.2019.03.074
Shirayama, T., Inoue, D., Inoue, M., Tatsumi, T., Yamahara, Y., Asayama, J., et al. (1991). Electrophysiological effects of sodium channel blockers on guinea pig left atrium. J. Pharmacol. Exp. Ther. 259, 884–893.
Silva, J., and Rudy, Y. (2003). Mechanism of pacemaking in IK1-downregulated myocytes. Circ. Res. 92, 261–263. doi:10.1161/01.res.0000057996.20414.c6
Skibsbye, L., Poulet, C., Diness, J. G., Bentzen, B. H., Yuan, L., Kappert, U., et al. (2014). Small-conductance calcium-activated potassium (SK) channels contribute to action potential repolarization in human atria. Cardiovasc. Res. 103, 156–167. doi:10.1093/cvr/cvu121
Stillitano, F., Lonardo, G., Giunti, G., Del Lungo, M., Coppini, R., Spinelli, V., et al. (2013). Chronic atrial fibrillation alters the functional properties of If in the human atrium. J. Cardiovasc. Electrophysiol. 24, 1391–1400. doi:10.1111/jce.12212
Ten Eick, R. E., and Singer, D. H. (1979). Electrophysiological properties of diseased human atrium. I. Low diastolic potential and altered cellular response to potassium. Circ. Res. 44, 545–557. doi:10.1161/01.res.44.4.545
Thuringer, D., Lauribe, P., and Escande, D. (1992). A hyperpolarization-activated inward current in human myocardial cells. J. Mol. Cel. Cardiol. 24, 451–455. doi:10.1016/0022-2828(92)91833-q
Trautwein, W., Kassebaum, D. G., Nelson, R. M., and Hechthh, H. H. (1962). Electrophysiological study of human heart muscle. Circ. Res. 10, 306–312. doi:10.1161/01.res.10.3.306
Van Wagoner, D. R., Pond, A. L., Lamorgese, M., Rossie, S. S., McCarthy, P. M., and Nerbonne, J. M. (1999). Atrial L-type Ca2+ currents and human atrial fibrillation. Circ. Res. 85, 428–436. doi:10.1161/01.res.85.5.428
Van Wagoner, D. R., Pond, A. L., McCarthy, P. M., Trimmer, J. S., and Nerbonne, J. M. (1997). Outward K+ current densities and Kv1.5 expression are reduced in chronic human atrial fibrillation. Circ. Res. 80, 772–781. doi:10.1161/01.res.80.6.772
Varró, A., Nánási, P. P., and Lathrop, D. A. (1993). Potassium currents in isolated human atrial and ventricular cardiocytes. Acta Physiol. Scand. 149, 133–142. doi:10.1111/j.1748-1716.1993.tb09605.x
Varró, A., Tomek, J., Nagy, N., Virag, L., Passini, E., Rodriguez, B., et al. (2021). Cardiac transmembrane ion channels and action potentials: cellular physiology and arrhythmogenic behavior Physiol. Rev. In press. doi:10.1152/physrev.00024.2019
Veerman, C. C., Kosmidis, G., Mummery, C. L., Casini, S., Verkerk, A. O., and Bellin, M. (2015). Immaturity of human stem-cell-derived cardiomyocytes in culture: fatal flaw or soluble problem? Stem Cell Dev. 24, 1035–1052. doi:10.1089/scd.2014.0533
Verkerk, A., Veerman, C., Zegers, J., Mengarelli, I., Bezzina, C., and Wilders, R. (2017). Patch-clamp recording from human induced pluripotent stem cell-derived cardiomyocytes: improving action potential characteristics through dynamic clamp. Int. J. Mol. Sci. 18, 1873. doi:10.3390/ijms18091873
Verkerk, A. O., and Wilders, R. (2020). Dynamic clamp in electrophysiological studies on stem cell-derived cardiomyocytes—why and how? J. Cardiovasc. Pharmacol. 77, 267-279. doi:10.1097/FJC.0000000000000955
Voigt, N., Pearman, C. M., Dobrev, D., and Dibb, K. M. (2015). Methods for isolating atrial cells from large mammals and humans. J. Mol. Cel. Cardiol. 86, 187–198. doi:10.1016/j.yjmcc.2015.07.006
Wakili, R., Voigt, N., Kääb, S., Dobrev, D., and Nattel, S. (2011). Recent advances in the molecular pathophysiology of atrial fibrillation. J. Clin. Invest. 121, 2955–2968. doi:10.1172/JCI46315
Wang, Z., Fermini, B., and Nattel, S. (1993). Sustained depolarization-induced outward current in human atrial myocytes. Evidence for a novel delayed rectifier K+ current similar to Kv1.5 cloned channel currents. Circ. Res. 73, 1061–1076. doi:10.1161/01.res.73.6.1061
Wettwer, E., Hála, O., Christ, T., Heubach, J. F., Dobrev, D., Knaut, M., et al. (2004). Role of IKur in controlling action potential shape and contractility in the human atrium: influence of chronic atrial fibrillation. Circulation 110, 2299–2306. doi:10.1161/01.CIR.0000145155.60288.71
Wilders, R. (2006). Dynamic clamp: a powerful tool in cardiac electrophysiology. J. Physiol. (Lond) 576, 349–359. doi:10.1113/jphysiol.2006.115840
Workman, A. J., Kane, K. A., and Rankin, A. C. (2001). The contribution of ionic currents to changes in refractoriness of human atrial myocytes associated with chronic atrial fibrillation. Cardiovasc. Res. 52, 226–235. doi:10.1016/s0008-6363(01)00380-7
Workman, A. J., Kane, K. A., and Rankin, A. C. (2003). Characterisation of the Na, K pump current in atrial cells from patients with and without chronic atrial fibrillation. Cardiovasc. Res. 59, 593–602. doi:10.1016/s0008-6363(03)00466-8
Workman, A. J., Marshall, G. E., Rankin, A. C., Smith, G. L., and Dempster, J. (2012). Transient outward K+ current reduction prolongs action potentials and promotes afterdepolarisations: a dynamic-clamp study in human and rabbit cardiac atrial myocytes. J. Physiol. 590, 4289–4305. doi:10.1113/jphysiol.2012.235986
Wu, L., Guo, D., Li, H., Hackett, J., Yan, G. X., Jiao, Z., et al. (2008). Role of late sodium current in modulating the proarrhythmic and antiarrhythmic effects of quinidine. Heart Rhythm 5, 1726–1734. doi:10.1016/j.hrthm.2008.09.008
Xu, Y., Tuteja, D., Zhang, Z., Xu, D., Zhang, Y., Rodriguez, J., et al. (2003). Molecular identification and functional roles of a Ca2+-activated K+ channel in human and mouse hearts. J. Biol. Chem. 278, 49085–49094. doi:10.1074/jbc.M307508200
Keywords: drug testing, patch clamp, human, cardiac myocytes, left atrial appendage, action potential, dynamic clamp, inward rectifier potassium current
Citation: Verkerk AO, Marchal GA, Zegers JG, Kawasaki M, Driessen AHG, Remme CA, de Groot JR and Wilders R (2021) Patch-Clamp Recordings of Action Potentials From Human Atrial Myocytes: Optimization Through Dynamic Clamp. Front. Pharmacol. 12:649414. doi: 10.3389/fphar.2021.649414
Received: 04 January 2021; Accepted: 18 February 2021;
Published: 12 April 2021.
Edited by:
Tamer M. A. Mohamed, University of Louisville, United StatesReviewed by:
Oscar Casis, Universidad del País Vasco UPV/EHU, SpainFelix Wiedmann, Heidelberg University Hospital, Germany
Andrew F. James, University of Bristol, United Kingdom
Copyright © 2021 Verkerk, Marchal, Zegers, Kawasaki, Driessen, Remme, de Groot and Wilders. This is an open-access article distributed under the terms of the Creative Commons Attribution License (CC BY). The use, distribution or reproduction in other forums is permitted, provided the original author(s) and the copyright owner(s) are credited and that the original publication in this journal is cited, in accordance with accepted academic practice. No use, distribution or reproduction is permitted which does not comply with these terms.
*Correspondence: Arie O. Verkerk, YS5vLnZlcmtlcmtAYW1zdGVyZGFtdW1jLm5s
†These authors have contributed equally to this work