- 1Department of Physiology, Faculty of Medicine, Universiti Kebangsaan Malaysia, Cheras, Malaysia
- 2Department of Pharmacology, Faculty of Medicine, Universiti Kebangsaan Malaysia, Cheras, Malaysia
- 3Department of Biological Sciences and Biotechnology, Faculty of Science and Technology, Universiti Kebangsaan Malaysia, Bangi, Malaysia
Edible bird’s nest (BN) is a Chinese traditional medicine with innumerable health benefits, including anti-viral, anti-inflammatory, neuroprotective, and immunomodulatory effects. A small number of studies have reported the anti-viral effects of EBN against influenza infections using in vitro and in vivo models, highlighting the importance of sialic acid and thymol derivatives in their therapeutic effects. At present, studies have reported that EBN suppresses the replicated virus from exiting the host cells, reduces the viral replication, endosomal trafficking of the virus, intracellular viral autophagy process, secretion of pro-inflammatory cytokines, reorient the actin cytoskeleton of the infected cells, and increase the lysosomal degradation of viral materials. In other models of disease, EBN attenuates oxidative stress-induced cellular apoptosis, enhances proliferation and activation of B-cells and their antibody secretion. Given the sum of its therapeutic actions, EBN appears to be a candidate that is worth further exploring for its protective effects against diseases transmitted through air droplets. At present, anti-viral drugs are employed as the first-line defense against respiratory viral infections, unless vaccines are available for the specific pathogens. In patients with severe symptoms due to exacerbated cytokine secretion, anti-inflammatory agents are applied. Treatment efficacy varies across the patients, and in times of a pandemic like COVID-19, many of the drugs are still at the experimental stage. In this review, we present a comprehensive overview of anti-viral and anti-inflammatory effects of EBN, chemical constituents from various EBN preparation techniques, and drugs currently used to treat influenza and novel coronavirus infections. We also aim to review the pathogenesis of influenza A and coronavirus, and the potential of EBN in their clinical application. We also describe the current literature in human consumption of EBN, known allergenic or contaminant presence, and the focus of future direction on how these can be addressed to further improve EBN for potential clinical application.
Introduction
Edible bird’s nest (EBN) is a nest produced from the salivary secretion of swiftlets such as Aerodramus sp. and Callocalia sp., which are commonly found in the South-East Asian regions. To date, a myriad of studies reported various therapeutic potentials of EBN, including anti-aging (Hwang et al., 2020), anti-inflammatory (Vimala et al., 2012), anti-viral (Guo et al., 2006), immunomodulatory (Haghani et al., 2016), anti-oxidant (Yew et al., 2014), and so forth. Given its broad health benefits, EBN is a highly sought-after medicinal food in Asia (Abidin et al., 2011; Looi and Omar, 2016).
Recent reports on global mortality caused by seasonal influenza indicate up to 290,000 to 650,000 deaths associated with respiratory illnesses alone (Iuliano et al., 2018), and the Global Burden of Disease Study (GBD) attributed 99,000 to 200,000 annual deaths from lower respiratory tract infections directly to influenza (GBD 2017 Influenza Collaborators, 2019). As of October 2020, a novel coronavirus, otherwise known as severe acute respiratory syndrome coronavirus 2 (SARS-CoV-2), has infected more than 50 million and killed no less than one million worldwide (Worldometer, 2020).
Through antigenic shift and drift, influenza viruses learn to evade inherent immune responses (Kim et al., 2018), hence continuous reformulation of immunization and vaccine persists (Principi et al., 2019). Moreover, suboptimal vaccine-induced immune responses in vulnerable populations like the elderly and young children also present a major strain on the management of influenza infections (Sano et al., 2017). The primary drug of choice for influenza, anti-viral medications are dogged by treatment-resistant viral strains (Hayden et al., 2018; Omoto et al., 2018), expensive treatment cost (Yang, 2019), and adverse effects (Witcher et al., 2019), despite their proven benefits. Whereas for SARS-CoV-2, at present, the efficacy of vaccines or anti-viral medications is plagued by fast mutating nature of the virus.
Over the past decades, there has been a growing interest in the use of natural products for their medicinal values (Kamil et al., 2018; Hamid et al., 2020; Kamil et al., 2020; Prom-In et al., 2020). EBN, is an emerging food product with known anti-viral effects, especially against Influenza A virus (IAV) (Guo et al., 2006; Haghani et al., 2016; Haghani et al., 2017), the most common cause of the global pandemic. EBN significantly reduced viral titer, viral-induced hemagglutination (Haghani et al., 2017), virus-binding activity (Guo et al., 2006), and virus-induced endocytosis and autophagosomes (Haghani et al., 2017). In addition to anti-viral, EBN also demonstrated anti-inflammatory (Vimala et al., 2012), anti-oxidant (Yew et al., 2014), and immunomodulatory properties (Haghani et al., 2016), indicating its potential as an all-around prophylactic or therapeutic option for influenza, SARS-CoV-2 and its associated complications, such as cytokine storm. In this review, we described the pathogenesis of IAV and SARS-CoV-2 infections, current anti-viral medications in clinical practice for both infections, chemical compositions, and potential benefits of EBN as an anti-viral, anti-inflammatory, anti-oxidant, and immunomodulatory agent in the treatment of IAV and SARS-CoV-2 infections.
Influenza A
The IAV is an enveloped virus with segmented negative sense, single-stranded RNA materials. The primary glycoprotein antigen on the virion surface, hemagglutinin (HA), and neuraminidase (NA) are the target of inherent immune response and many anti-viral medications (McAuley et al., 2019). To date, 18 types of H antigen, and 11 N antigens have been identified (Tong et al., 2013). Throughout history, IAV is known to cause various pandemics, such as the H1N1 virus pandemic in 1918 (Saunders-Hastings and Krewski, 2016), H2N2 in 1957, H3N2 in 1968, and another H1N1 virus pandemic in 2009 (Guan et al., 2010).
IAV, through HA on the surface of the virion, binds to the terminal sialic acid residues on mucins (Ma et al., 2017), which is released by the actions of NA cleaving off the terminal sialic acid residues (Yang et al., 2014), leading to endocytosis of IAV into the host cell past the mucosal layer. Once in, HA undergoes conformational changes to expose fusion peptide to promote viral endosomal membrane fusion, and IAV core undergoes acidification via protein entry through the M2 ion channels, allowing vRNPs to be released into the cytoplasm (Padilla-Quirarte et al., 2019). The IAV genome is transcribed and translated to synthesize HA, NA, M2 ion channel, matrix protein (M1), nuclear export protein (NEP), polymerases (PB1, PB2, PA), nucleoprotein (NP), PB1-F2, PA-X, and non-structural protein 1 (NS1). The synthesized viral particles attach to the host cell membrane due to the interaction between HA and sialic acids and released by the catalytic actions of NA on terminal sialic acid residues (Krammer, 2019).
The major types of sialic acid present in the terminal side of the glycans of mammalian and avian glycoproteins and glycolipids are N-acetylneuraminic acid (Neu5Ac; mostly humans) and N-glycolylneuraminic acid (Neu5Gc) (For review Long et al., 2019). HA from human-adapted viruses is known to bind to α2-6-linked sialic acid, whereas HA from avian influenza viruses binds to α2-3-linked sialic acid (Rogers and Paulson, 1983). The X-ray crystallographic and glycan microarray binding studies revealed a receptor binding site of HA from human-adapted viruses contain a bulkier cis conformation adopted by α2-6-linked sialic acid, compared to the HA of avian influenza viruses with thin and straight trans conformation by the α2-3-linked sialic acid (Shi et al., 2014; Lipsitch et al., 2016). Studies also have reported both α2-3 and α2-6 sialic acid linkages in the human lung and bronchus (Walther et al., 2013), α2-6 linkages in the respiratory tracts of ferrets and pigs (Nelli et al., 2010; Jia et al., 2014), and higher expression of α2-3 sialic acid linkages in non-human primates and mice (Gagneux et al., 2003; Ning et al., 2009). Other features of glycans also determine the interaction between virus and host, such as the presence of other sugar moieties or functional groups, length of sialic acid presenting glycans (Long et al., 2019), and second binding site in addition to a usual catalytic sialic acid binding site of NA, such as the hemadsorption (Hd) site (Uhlendorff et al., 2009). More recent findings suggest the binding to the secondary site may occur prior to the binding to the primary site where the enzymatic cleavage occurs (Durrant et al., 2020).
Anti-Viral Medications Against IAV
Vaccination is the primary mode of prevention against influenza. Though, most of the vaccines are not 100% effective as the influenza viruses are constantly evolving (Hurt, 2014). Hence, anti-viral medications are in continuous development given their importance in the management of influenza infections, particularly during the initial phases of a pandemic when vaccines are still in the making. Table 1 shows a comprehensive overview of various anti-virals used to treat IAV infection.
The anti-viral baloxavir marboxil (XofluzaTM) is a cap-dependent endonuclease inhibitor that was developed to treat IAV or B infection (Shionogi and Co. Ltd, 2018). Following Japan in 2018, baloxavir is approved in many other countries, including the United States in 2019 (Takashita et al., 2019). The mechanism of action of baloxavir is dissimilar to neuraminidase inhibitors, where it suppresses the proliferation of virus through inhibition of mRNA synthesis initiation (Koszalka et al., 2017; Noshi et al., 2018). Administration of a single dose of baloxavir within the 48 h of symptom onset rapidly improved the symptoms, with fewer influenza-related complications. Moreover, a single dose of baloxavir also reduced viral replication in high-risk adult and adolescent outpatients with uncomplicated influenza (Portsmouth et al., 2017; Ison et al., 2020). Children older than 12 years of age and adults suffering from IAV or B generally well-tolerated a single dose of baloxavir (Heo, 2018). Otherwise, the most commonly associated adverse effects with baloxavir are diarrhea, nausea, bronchitis, and sinusitis (incidence of ≤3%) (Shirley, 2020), and increase in AST, ALT, and headache (incidence <1%) (Shionogi and Co. Ltd, 2018).
Oseltamivir (TamifluTM) is a specific inhibitor of IAV that has proven clinically effective in adults and children (as young as 1 year) for the chemoprophylaxis and treatment of IAV and B infections (Ward et al., 2005). Oseltamivir is available in oral form (75 mg twice daily used by a large population of patients) (McNicholl and McNicholl, 2001) and intravenously for those unable to tolerate oral dosing (Kamali and Holodniy, 2013). Oral administration of Oseltamivir (75 mg twice daily) significantly reduced the severity and duration of symptoms (McNicholl and McNicholl, 2001). Oseltamivir is relatively well tolerated, with the most commonly associated side effects such as abdominal pain, vomiting, and transient nausea in 5–10 percent of patients studied (Moscona, 2005). Others have also reported Oseltamivir-related serious adverse effects, such as sudden deaths and accidental deaths due to abnormal behaviors (Hama, 2016). Resistance to the drug has also been reported in the past, among patients who have been exposed to the drug previously, and also without any prior exposure (Kamali and Holodniy, 2013).
Zanamivir (Relenza™) is the first neuraminidase inhibitor to be developed, with a high affinity for the neuraminidase binding site (McKimm-Breschkin, 2013). Zanamivir has poor oral bioavailability, hence administered with an inhaler device (Diskhaler) (Diggory et al., 2001). Therefore, the inspiration flow determines the amount of drugs reaching the respiratory tract, which is a limitation, especially for intubated patients (Ison, 2010). For patients older than seven years of age, Zanamivir (10 mg) is inhaled twice daily for 5 days, whereas prophylaxis is given to patients older than 5 years of age once daily for 10–28 days (Samson et al., 2013). Zanamivir reduces the period of symptom alleviation by 10–24% (0.5–1.25 days) (McNicholl and McNicholl, 2001).
Peramivir (Rapivab®) is a novel cyclopentane neuraminidase inhibitor with potent and selective inhibitory action against IAV and B virus’ NA, with similar or more potent in vitro inhibitory effects against IAV and B, than zanamivir or oseltamivir (Fage et al., 2017). In 2014, the Food and Drug Administration approved the use of peramivir for the treatment of acute uncomplicated influenza in patients 18 years and older (Alame et al., 2016). Due to its poor oral bioavailability, peramivir is offered only as an intravenous formulation, and its inhibitory activity against influenza is slightly lower than oseltamivir and zanamivir (Fage et al., 2017). Pediatric and adult patients with uncomplicated influenza generally well tolerate a single dose of peramivir. The common adverse reactions reported are nausea (2.4% of patients) and reduced neutrophil counts (3.2%) (BioCryst, 2018). In children (frequency 1 to <10%), the most frequently associated adverse reactions are rashes at injection sites, tympanic membrane hyperemia, pyrexia, pruritus, and psychomotor hyperactivity (BioCryst, 2018).
Severe Acute Respiratory Syndrome-Related Coronavirus
Coronavirus Disease 2019 (COVID-19) caused by the severe acute respiratory syndrome coronavirus (SARS-CoV-2) has infected more than 50 million, and killed at least 1.2 million worldwide, as of November 2020 (Worldometer, 2020). The Timeline Outbreak by SARs-COV-2 viruses began when severe cases of pneumonia were reported in Wuhan City of Hubei Province in China. Investigations into etiological agents led to the Hunan seafood wet market in Wuhan, China where the sample tested from this wet market was found to be positive for SARS-CoV-2, which was initially known as 2019-nCoV before being renamed as SARS-CoV-2 (Ralph et al., 2020; Wu et al., 2020).
Coronavirus is made of the Membrane (M), Envelope (E), Nucleocapsid (N), and spike protein (S), and two large polyproteins (Yoshimoto, 2020). Among them, the ones that enabled the coronavirus to infect and replicate in humans are S and N proteins. The S protein of SARS-CoV-2 has a receptor-binding domain (RBD) similar to SARS-CoV, which binds to the same receptor Angiotensin-converting Enzyme 2 (ACE2) in humans, but with 10-20-fold higher affinity (Wrapp et al., 2020; Zhang and Holmes, 2020), though MERS-CoV expresses a different receptor, dipeptidyl peptidase 4 or DPP4 (Wang et al., 2013). The binding of the S protein to ACE2 enables the entry of SARS-CoVs into the human cell (Letko et al., 2020), whereas N protein is vital for replication and assembly (V’kovski et al., 2020).
Anti-Viral Agents Against SARS-CoV-2
Remdesivir (RDV) (sold under the trade name Veklury) gained prominence as it is the first anti-viral drug to be approved by the US FDA to be used as a treatment option for SARS-CoV-2. Initially, RDV was developed as a general anti-viral drug for hepatitis C and Ebola, but results were not encouraging. RDV acts by inhibiting the coronavirus’s RNA synthesis through delayed chain termination. RDV was touted as a potential anti-viral therapy option for SARS-CoV-2 early in the pandemic. Trials were quickly initiated. Intravenous RDV was found to have been numerically better but not statistically significant in mortality, clinical improvement, and time taken to clear off the virus (Wang X. et al., 2020). Grein et al. in another trial reported that 68% (36 of 53 patients) of patients showed improvement with regards to oxygen support on day 18, and 84% had significant clinical improvement by day 28 in severe Covid-19 cases (Grein et al., 2020). In both trials, adverse effects were mostly well tolerated with common adverse events reported as constipation, increased total bilirubin, anemia, diarrhea, rash, and hepatic enzymes. Severe adverse events include septic shock, acute kidney injury, and multiple organ dysfunction.
The anti-viral favipiravir (FPV) (sold under the trade name Avigan and Fabiflu) is developed by Toyama Chemical (Japan), has been sold in Japan for the treatment of influenza since 2014. It was one of the early candidates recognized for the pharmacotherapy of SARS-CoV-2 and was used in Wuhan as a treatment option. Since then, a few trials have been initiated in various countries with varying degrees of success. FPV competitively inhibits RNA-dependent RNA polymerase in the virus (Furuta et al., 2013). FPV profoundly improved the latency to relief for cough and pyrexia in patients with COVID-19 (Chen C. et al., 2020). FPV also improved clinical recovery in Day 7 in moderately severe COVID-19 patients as well as decreased auxiliary oxygen therapy and decreased the incidence of dyspnea (Chen L. et al., 2020). Another trial by Cai et al. (2020) reported a significant decrease in the time SARS-CoV-2 viral clearance and improvement in chest imaging in comparison to ritonavir or lopinavir (Cai et al., 2020). FPV is a well-tolerated drug with the most commonly reported adverse event of elevated serum level of uric acid and had a better safety profile compared to lopinavir or ritonavir (Cai et al., 2020; Chen C. et al., 2020).
Ritonavir (LPV/r) and Lopinavir is a fixed-dose anti-viral combination used for the pharmacotherapy and prevention of HIV/AIDS. LPV/r acts by inhibiting the protease activity of viruses. LPV/r was also touted early in the epidemic as an anti-viral treatment for SARS-CoV-2. A preclinical in vitro study by Kang et al. reported lower viral load in LPV/r treated group and concluded profound inhibition of SARS-CoV-2 at plasma concentration (Kang et al., 2020). Many trials have since concluded on its efficacy. Wang et al. reported significant alleviation of pneumonia-associated symptoms (Wang Y. et al., 2020). Cao et al. reported overall lower 28-days mortality (19 vs 25%) on patients receiving LPV/r but it was not statistically significant (Cao et al., 2020). Similarly, Chen C. et al., 2020, in a retrospective study reported no profound difference between LPV/r treated and control groups in symptom improvement or reduction in viral loads (Chen L. et al., 2020). Most studies reported that LPV/r anti-viral is a well-tolerated drug with gastrointestinal adverse effects as the most commonly reported adverse effects. Table 2 shows a comprehensive overview of various anti-viral medications for SARS-CoV-2.
Anti-Viral Effects of EBN
The anti-viral properties of EBN were tested using in vitro and in vivo models and compared across the efficacy of standard anti-viral medications such as oseltamivir and amantadine (Haghani et al., 2017) (Table 3). In anti-viral cell culture studies, EBN did not produce any cytotoxic effects up to 50 mg/ml (Nuradji et al., 2018), whereas some reported CC50 of EBN extracts at a lower concentration range 27.2–32 mg/ml (Haghani et al., 2017). For anti-viral in vivo studies, concentration in the range of 5–2000 mg/kg was tested in 8–10 weeks old female mice via oral gavage and was shown to be free of toxicity and mortality at the end of 14 days of the treatment period (Haghani et al., 2016). The EBN concentration range of 12.5 mg/ml (Haghani et al., 2017), 0.5–4,000 μg/ml (Guo et al., 2006), and 15–35 mg/ml (Nuradji et al., 2018) was employed in most anti-viral investigations against IAV strains of H1N1, H3N2, and H5N1, respectively.
Most of the in vitro anti-viral studies of EBN used the water extraction method to prepare the EBN test sample. Usually, EBN is dried at a temperature higher than ambient for few hours to one day before grinding and filtering EBN with wire mesh to separate the feather and impurities. The ground EBN was then soaked in water or water-based buffer before heated to release the water-soluble parts of the EBN. Pancreatin enzyme was commonly used to simulate the gastrointestinal digestion process to break down the larger protein components of EBN. For in vivo model, EBN extract without the pancreatin enzyme is preferred in the experiment since the animal gut could do the job (Haghani et al., 2016). As the anti-viral effect of the EBN depends on the amount, concentration, and bioavailability of the functional groups; the location of EBN harvest, seasoning effect, and extraction methods can affect the study findings. Table 4 shows an overview of the analysis of EBN composition in various studies.
For its anti-viral effect, EBN water extracts significantly reduced hemagglutination activity of IAV (H1N1, H3N2, and H5N1) in a dose-dependent manner (Guo et al., 2006; Haghani et al., 2017; Nuradji et al., 2018), with increasing efficacy when the treatment duration is prolonged (Haghani et al., 2017). The efficacy of EBN with enzymatic treatment (pancreatin F), and without enzymatic treatment was the focus of many in vitro investigations. EBN without enzymatic treatment reduced extracellular NA copy number, NS1 expression, and at the same time also increased intracellular NS1 expression. EBN with enzymatic treatment increased intracellular NA copy number, increased extracellular NS1 expression, and increased intracellular M2 expression, suggesting the effects of EBN with enzymatic treatment (Pancreatic F) are more inclined to inhibiting the release of the viral materials (Haghani et al., 2016). EBN (from house nest) with and without enzymatic treatment reduced virus titer, with a percentage of protection 42.47 ± 8 and 45.42 ± 8.4, respectively (Haghani et al., 2017). In line with these findings, Guo et al. (2006) reported significant inhibitory effects of EBN with pancreatic enzyme digestion on hemagglutination activity of IAV, whereas little effects when treated with EBN without the pancreatic enzymes. The findings indicate that EBN extracts with smaller proteins (10–25 kDa) when treated with the pancreatic enzyme produced stronger inhibitory effects compared to the larger proteins (more than 50 kDa) expressed in the EBN without enzymatic treatment. The same researchers also reported that EBN extracts (4 mg/ml) did not inhibit the neuraminidase activity of influenza, and therefore, the EBN extracts act on viral hemagglutinin but not viral neuraminidase (Guo et al., 2006). In addition to reducing viral load, EBN also normalized the cellular shapes and reoriented the actin filaments, and reduced the densities of actin filaments caused by the IAV infection. EBN also increased the density and the number of lysosomes in both infected and non-infected cells (Haghani et al., 2017) (Figure 1).
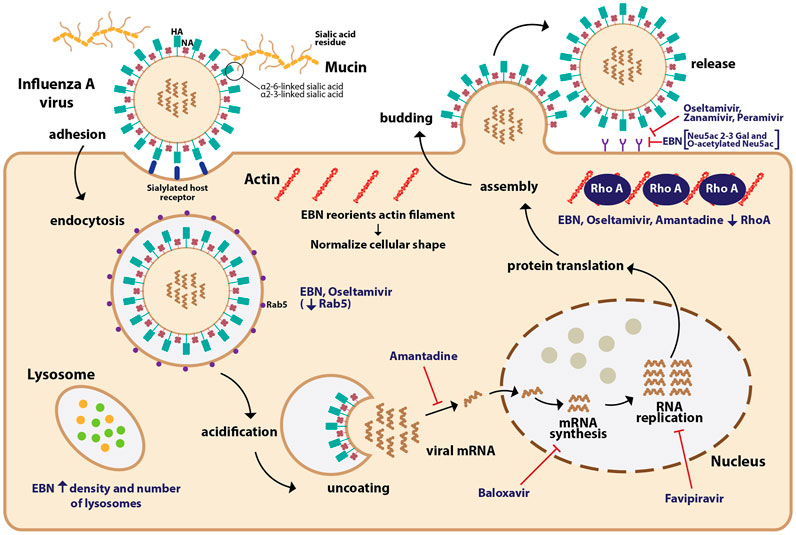
FIGURE 1. The mechanism of action of EBN and anti-viral drugs on influenza A virus (IAV). The IAV binds to the terminal sialic acid residues on mucins, particularly through α2-6 and α2-3 linkages leading to endocytosis of IAV into the host cell. The IAV core undergoes acidification allowing viral RNPs to be released into the cytoplasm. The IAV genetic material is transcribed and translated to produce viral materials, which once assembled, bud out from the infected cell. Anti-viral drugs such as baloxavir inhibit viral mRNA synthesis, favipravir inhibits RNA replication, amantadine inhibits the release of viral mRNA, and neuraminidase inhibitors (oseltamivir, zanamivir, and peramivir) inhibit the release of the IAV. EBN increases the density and number of lysosomes in infected cells. Similar to oseltamivir, EBN reduces the expression of Rab5, which facilitates the endocytosis of IAV. EBN reorients the actin filament and normalizes the cellular shape of infected cells. Similar to oseltamivir and amantadine, EBN also reduces the expression of RhoA, a protein involved in actin filament polymerization. EBN, through its sialic linkages such as Neu5Ac 2-3 Gal, and O-acetylated Neu5Ac may prevent the release of IAV.
Treatment with another enzyme, neuraminidase significantly affected the inhibitory effects of EBN (Guo et al., 2006), suggesting the important role of glycosidic linkages in the anti-viral properties of EBN. Lectin blotting assay revealed Neu5Ac2-3Gal linkage as the major molecular species of sialic acid in the EBN extract along with O-acetylated Neu5Ac (Guo et al., 2006). Based on the location of collection (house vs cave), EBN extracts differ chemically, where EBN collected from the cave (Gua Madai) was reported to contain Neu2, 4,7,8,9 Ac6, which has more O-acetylated branches (Haghani et al., 2016). O-acetylation of N-acetyl residues confers higher resistance of sialic acids to the actions of sialidases from various infectious agents (Corfield et al., 1981; Haverkamp et al., 1982). EBN from Gua Madai recorded the highest percentage of protection against IAV compared to EBN collected from House Nest. Fluorometric High-Performance Liquid Chromatography revealed EBN from Gua Madai contained 6.7 mg/g of Neu5Ac, whereas EBN from House Nest contained 3.2 mg/g of Neu5Ac (Haghani et al., 2017). Both research groups suggest that the higher amount of sialic acid and the diversity of its derivatives increase the anti-viral effects of EBN.
Cytokine Storm in Influenza and COVID-19 Infections
Cytokine storm (CS) or aberrant pro-inflammatory responses is a known complication in severe influenza (Woo et al., 2010; Ishikawa, 2012; Kalaiyarasu et al., 2016) and COVID-19 cases (Chan et al., 2020; Wang Z. et al., 2020; Zhao et al., 2020). Infected cells undergoing apoptosis and necrosis trigger acute inflammatory response recruiting leukocytes and plasma cells to the site of infection (Knipe and Howley, 2013). Hence, increasing the production of pro-inflammatory cytokines and chemokines (La Gruta et al., 2007; Shinya et al., 2012) via transcription factors such as Nf-κB, AP-1, interferon response factors 2 and 7 (Thompson et al., 2011).
In severe influenza cases, increase in transcription activities of NF-κB and IRF 3/7 promote anti-viral pro-inflammatory responses in the lungs, including excessive levels of interferon, tumor necrosis factor (TNF-α), interleukin (Beigel et al., 2005) (IL-1 and IL-6) (Tisoncik et al., 2012), IL-8 (de Jong et al., 2006), and chemokines such as MCP-1, RANTES, causing severe influenza-induced immunopathy (Berri et al., 2014; Chiang et al., 2014) Increased activities of NF-κB also escalate the expression of IκB (an inhibitor of NF-κB) and anti-inflammatory cytokines to attenuate the NF-κB-induced pro-inflammatory responses. Early responses to IAV infections indicate a rise in the levels of RANTES, MCP-1, IL-8, IFN-α, IFN-β, and IFN-κ (Le Goffic et al., 2007; Xagorari and Chlichlia, 2008), whereas late responses have recorded a surge in IL-1α/β, IL-6, TNF-α, IL18, IFN type I, MCP-1, MIP-1α, MIP-1β, RANTES, MCP-3, MIP-3α, and IP-10, especially secreted by macrophages residing in the lower respiratory tract (Jewell et al., 2010). Such changes in cytokine waves tip the balance between pro-inflammatory and anti-inflammatory responses, along with viral virulence causes respiratory epithelial injuries such as acute lung injury or even ARDS (Shimizu, 2019) reducing oxygen saturation level leading to mortality (For review: Gu et al., 2019).
In COVID-19 cases, an increase in IL-6 level was most frequently associated with the severity of the disease (Chen C. et al., 2020; Gao et al., 2020; Ruan et al., 2020) and other cytokines and chemokines, including IL-1B, IL-7, IL-8, IL-9, IL-10, FGF, G-CSF, GM-CSF, IFN-γ, IP-10, MCP-1, MIP-1A, MIP1-β, PDGF, TNF-α, and VEGF (Huang et al., 2020) were also documented. At the early stage of SARS-CoV infection, there is a delay in the release of chemokines, cytokines, hence a low level of IFN. At the same time, the abundant release of pro-inflammatory cytokines such as TNF, IL-1β, IL-6, and chemokines such as CCL-2, CCL03, CCL-5 causes excessive infiltration of inflammatory cells into the lungs (Law et al., 2005; Lau et al., 2013). Pre-clinical findings revealed an early delay in the release of IFN-α/β induced generation of more monocyte chemoattractants such as CCL-2, CCL-7, and CCL-12 by macrophages causing their further accumulation, and production of pro-inflammatory cytokines, including IL-1β, IL-6, TNF-α, and inducible nitric oxide synthase. Cumulatively, IFN-α/β and IFN-γ cause apoptosis of T cells (Channappanavar et al., 2016), airway epithelial cells, alveolar epithelial cells, and endothelial cells (Shimizu, 2019) damaging pulmonary vasculature, leading to vascular leakage and alveolar edema, causing hypoxia (Channappanavar et al., 2016; For review: Ye Q. et al., 2020). Endothelial injuries also cause spillover of cytokines and chemokines into the blood circulation causing multi-organ failure (Tisoncik et al., 2012). In line with this, findings from non-human primates revealed that SARS-CoV-infection-related death is more likely due to excessive immune response rather than virus titer (Smits et al., 2010).
The use of Corticosteroids in Influenza and COVID-19 Infections
The severity of- and mortality due to influenza and COVID-19 infections are determined by the host resistance and virulent factors, where severe infections usually trigger hyperactive host resistance, hence causing adverse symptoms (Liu et al., 2020), such as acute respiratory distress syndrome (ARDS), eventually leading to mortality. Influenza patients with ARDS are managed through corticosteroid therapy for their anti-inflammatory actions. Over the years, a myriad of systematic and meta-analyses have consistently reported that routine corticosteroid treatment in influenza patients may not be appropriate. Many reported a significant association between corticosteroid treatment and higher mortality (Zhang et al., 2015; Ni et al., 2019; Lansbury et al., 2020; Zhou et al., 2020), whereas some have reported that low-to-moderate dose of corticosteroids may reduce mortality (Li et al., 2017). Some have claimed that early and high-dose corticosteroid use is associated with hospital mortality (Lansbury et al., 2020; Sheu et al., 2020; Tsai et al., 2020). In addition to high mortality, corticosteroid treatment also correlated to secondary infections (Yang et al., 2015; Lansbury et al., 2019; Ni et al., 2019; Zhou et al., 2020) due to prolonged hospital stay (Yang et al., 2015; Ni et al., 2019).
Systematic review and meta-analyses of randomized clinical trials revealed that corticosteroids reduced the mortality in critically ill COVID-19 patients (WHO Rapid Evidence Appraisal for COVID-19 Therapies (REACT) Working Group et al., 2020; Ma et al., 2021; van Paassen et al., 2020), especially patients with compromised lung capacity (Bartoletti et al., 2021), however with delayed viral clearance and increased secondary infections (van Paassen et al., 2020). Some have also reported increased short-term mortality following corticosteroid use in COVID-19 patients with ARDS (Liu et al., 2020), whereas some found no significant association between corticosteroid use and mortality (Shuto et al., 2020; Albani et al., 2021). In line with these, some have warned against the use of corticosteroids in non-critically ill COVID-19 patients due to longer hospitalization, longer duration of viral shedding, and progression of non-severe to severe cases (Shuto et al., 2020; Yuan et al., 2020). Due to many limitations such as study designs, sample size, age factors, viral strain, and pharmacogenomics discrepancy, at present, it is not empirically possible to conclude the efficacy of corticosteroid use in COVID-19 or influenza patients.
Anti-Inflammatory Effects of EBN
EBN (House nest from Teluk Intan), when tested for its anti-viral effects using an in vivo model, significantly increased the expression of IFN-γ, IL-1β, IL-2, IL-6, and TNF-α on day 1 following infection, significantly decreased the level of IFN-γ, and upregulated IL-4, IL-6, IL-12, and TNF-α on day 3 post-infection, and reduced IL-10 and IL-12 on day 7. The profound increase in the pro-inflammatory cytokines seen during the early stage of infection is similar to numerous anti-viral medications, including the anti-viral drug oseltamivir used in the same study. On post-infection day 5 and 6, the level of IL-6 remained high in the oseltamivir treated groups, unlike the EBN treated groups where the levels of pro-inflammatory cytokines were well regulated. In the same study, EBN (House nest from Teluk Intan) treated with pancreatic F enzyme significantly decreased the levels of IL-6 and chemokine (C-C motif) ligand 2 (CCL2) in vitro (Haghani et al., 2016). In line with these findings, studies using other disease models also reported EBN-induced inhibition of nitric oxide and TNF-α production in LPS-stimulated macrophages (Aswir and Wan Nazaimoon, 2011; Vimala et al., 2012), improvement in the proliferation of lymphocytes and splenocytes (Zhao et al., 2016), free radical scavenging activities and reduced pro-inflammatory markers such as CRP, TNF-α, CCL2, NF-κB (Yida et al., 2015) (Figure 2). Table 5 shows the anti-inflammatory effects of EBN on various models.
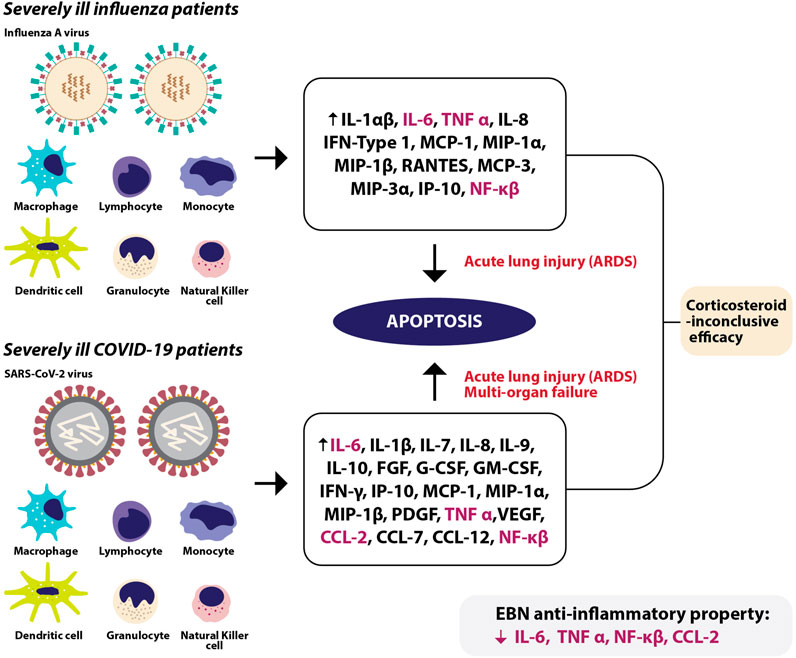
FIGURE 2. In severely ill influenza patients, immune cells release various cytokines and chemokines leading to acute lung injury, or also known as acute respiratory distress syndrome (ARDS) due to apoptosis. In severely ill COVID-19 patients, various immune cells release a myriad of chemokines and cytokines causing apoptosis, leading to ARDS and spillover of cytokines and chemokines into the blood circulation causing multi-organ failure. Among the escalated cytokines and chemokines in severe influenza and COVID-19 infections, EBN was shown to reduce IL-6, TNF-α, CCL-2, and NF-κβ at the pre-clinical level.
The discrepancy in the therapeutic potential of EBN of various sources and preparation techniques should be taken into consideration. For an instance, some researchers have reported increased viability of cells following incubation with EBN (Zhao et al., 2016; Haghani et al., 2017) with increasing concentration of sialic acid (Aswir and Wan Nazaimoon, 2011). Whereas, some reported no improvement in viability of cells following co-incubation of EBN (water extract) with 6-hydroxydopamine (Yew et al., 2014). However, when incubated with crude EBN, 20% of viability was seen (Yew et al., 2014). Moreover, treatment with high-dose crude EBN significantly promoted intracellular production of ROS (twice than 6OH treated level). However, high-dose water extracts of EBN significantly reduced the ROS level (Yew et al., 2014). In mice, all EBN dosages employed in a study induced six division cycles of CD-19 stained (B cells). EBN (0.19, 0.38, and 0.75 mg/ml) only completed one division of CD-3 stained (T cells) cells, indicating that the immunomodulatory property of EBN could be more directly inclined to B cell responses (Zhao et al., 2016).
Bioactive Ingredients in EBN
EBN mainly consists of protein (almost 60%), carbohydrate (25%) (Daud et al., 2019), moisture, fat, and ash (Amiza et al., 2019; Hun Lee et al., 2020; Hui Yan et al., 2021). At present, the potential bioactive ingredients in EBN with anti-viral and immunomodulatory properties are glycoprotein or glycopeptides. Sialylated N-glycan was identified as the main component of EBN contributing to its anti-viral property (Yagi et al., 2008). Fluorometric analysis revealed Neu5Ac 2-3 Gal linkages in the cave and O-acetylated Neu5Ac in house-cultured EBN (Guo et al., 2006). Further analysis identified derivatives of sialic acid such as N-glycol neuraminic acid, N-acetyl neuraminic acid, 5-N-acetyl-8,9-O-acetylneuraminic acid, and others such as thymol-β-glycopyranoside in EBN (Haghani et al., 2016). The importance of sialic acid in human nutrition is well known (Ghosh, 2020). Hydrolysis of EBN produced the highest amount of soluble protein compared to other forms of EBN. The hydrolysates of EBN contained high level of N-acetylneuraminic acid with profound anti-oxidant effects (Gan et al., 2020). The degree of hydrolysis was the highest when EBN was enzymatically treated with alcalase (Amiza et al., 2019; Zulkifli et al., 2019; Hui Yan et al., 2021), recovering a high level of bioactive Siamuc-glycopeptide (Hui Yan et al., 2021), and amino acids including valine, serine, aspartic acid, phenylalanine, tyrosine, histidine, threonine, and leucine (Babji et al., 2018; Noor et al., 2018; Amiza et al., 2019; Hun Lee et al., 2020). In line with this, the anti-oxidant activity of enzymatically treated EBN was significantly higher, especially alcalase compared to just boiled EBN (Babji et al., 2018; Zulkifli et al., 2019). Other glycoprotein and protein such as ovotransferrin, and lactoferrin also identified in EBN, especially higher in house and cave white EBN compared to cave red and black EBN (Hou et al., 2015a). Ovotransferrin is known for its anti-bacterial, anti-viral, and anti-inflammatory properties (Giansanti et al., 2016). Similarly, lactoferrin from milk also been well explored for its anti-viral and anti-bacterial activities (Giansanti et al., 2015).
Most of the studies investigating the potential medicinal values of EBN used water extract (Haghani et al., 2016; Haghani et al., 2017), as bioactive compounds in EBN are water-soluble. Water extraction of EBN results in a mixture of glycopeptides (Guo et al., 2006; Yagi et al., 2008; Ling et al., 2020; Hui Yan et al., 2021) which should be isolated or purified through a binding affinity technique utilizing specifically targeted ligands anchored to the solid phase such as a polyester membrane or cellulose-based surface. The purified or isolated bioactive ingredients can then be released by elution buffer with an appropriate pH. In a large-scale setting, it would be more practical to carry out molecular size range separation (Babji et al., 2018) prior to the purification or isolation process, to reduce the complexity of the extract and slow down the purification step. By fractioning the EBN extract based on their molecular size, the purification step can be further simplified. This should be followed by the identification of bioactive compounds in the fractions. House harvesting of EBN results in various grades of EBN (Grade A-D) (Noor et al., 2018). The fragments and crushed pieces of EBN are of low value for conventional EBN processing. Nonetheless, this EBN might be more applicable as raw material for EBN extract preparation, which involves grinding the EBN into powder, and followed by soaking in water to increase their surface area for reaction with water or enzymes. Subsequently, the crude extract should be subjected to fragmentation based on molecular size and followed by purification. Purified peptides (3 < kDa) showed the strongest anti-oxidant activity compared to extract and crude hydrolysate (Ghassem et al., 2017). Techniques such as freeze-drying removes water while preserving the functional groups of the bioactive compounds. In line with this, freeze-drying has been reported to result in a high yield of EBN compared to oven and spray drying (Gan et al., 2020). The glycopeptides in EBN are easily denatured by the heat drying method, hence the water content should be evaporated under low temperature to preserve the bioactive compounds. Upon usage, the compounds can be reconstituted easily with water to regain their functional property.
EBN Studies in Humans: What we Know so far
EBN is an expensive delicacy for the Chinese and sometimes is considered as the “Caviar of the East” (Marcone, 2005). EBN is exclusively popular as part of the traditional Chinese medicine to treat respiratory ailments, moistening the lungs, heart tonics, and stomach nourishments (Zhao et al., 2016). Owing to its documented effects in the old Chinese medical textbooks, the Chinese frequently consumed EBN as a supplement for health benefits (Zhao et al., 2016). Numerous authentic EBN products, particularly in a form of extract or essence, were sold over the counter where most of them were imported exclusively from the Southeast Asian regions (Dai et al., 2020). To date, no findings have directly related the health benefits of EBN in humans. The closest to human testing were the in vitro setups involving cultured human cell line such as keratinocytes (Kim et al., 2012; Hwang et al., 2020), SH-SY5Y cells (Hou et al., 2015b), lung carcinoma cells (Guo et al., 2006), colonic adenocarcinoma cells (Aswir and Wan Nazaimoon, 2011), chondrocytes (Chua et al., 2013), and hepatocarcinoma cells (Ghassem et al., 2017). In vivo reports were derived from pre-clinical animal models (Yida et al., 2015; Zhao et al., 2016). Hence, the potential benefits of EBN in humans are yet to be proven empirically. In line with human consumption, EBN was reported to cause allergic reactions in pediatric patients due to avian allergen homologous to ovoinhibitor precursor in chicken (66-kd protein) and potentially by other additives or contaminants (Goh et al., 2000; Goh et al., 2001). Compared to fresh EBN extract, commercially prepared EBN showed an undetectable amount of allergen in immunoblot probably due to boiling of the EBN, however still able to trigger an allergic reaction, indicating that the allergen is not heat sensitive. Periodate treatment relinquished the Ig-E binding ability of the allergen suggesting that allergenic epitope could be due to carbohydrate moiety which is known to be resistant to cooking and boiling (Goh et al., 2001). Contaminants such as fungus also been reported to present in EBN, especially plant and soil fungi in raw EBNs and environmental fungi in commercial and boiled EBNs (Chen et al., 2015).
EBN as a Potential Anti-Viral and Anti-Inflammatory Therapies: Future Direction
The anti-viral efficacy of EBN was compared across standard anti-viral medications such as oseltamivir and amantadine, and similar to those drugs EBN also reduced protein involved in viral trafficking such as Rab5 (not amantadine) and protein involved in actin filament polymerization, RhoA. EBN also inhibited the HA activity of the virus (Guo et al., 2006; Haghani et al., 2017; Nuradji et al., 2018). The anti-viral drugs currently being used to treat IAV infection are baloxavir marboxil (inhibits viral mRNA synthesis) (Shionogi and Co. Ltd, 2018), oseltamivir, zanamivir, and peramivir (selective neuraminidase inhibitors) (Gubareva et al., 2000; Ohuchi et al., 2006; Hama, 2015), and favipravir (inhibits RNA polymerase activity) (Furuta et al., 2013). Apart from the neuraminidase inhibitors, it is yet to be explored if a combined therapy of EBN and other classes of anti-viral medications could achieve a synergistic outcome. EBN also attenuated the surge in pro-inflammatory cytokines and chemokines such as TNF-α, CCL-2, NF-κβ, NO, IL-6, and increased IFN-γ (Aswir and Wan Nazaimoon, 2011; Vimala et al., 2012; Haghani et al., 2016), which are dysregulated in severe forms of IAV (Xagorari and Chlichlia, 2008; Tisoncik et al., 2012), and SARS-CoV infections (Gao et al., 2020; Huang et al., 2020). Moreover, EBN also ameliorated apoptosis (Yew et al., 2014), and normalized the cellular shape of IAV-infected cells (Haghani et al., 2017), which may reduce the collateral damage to the host cells in severe infections. Furthermore, EBN also exhibited anti-bacterial property (Hun et al., 2015), and improved B cell activity (Zhao et al., 2016), which may provide additional protection against opportunistic bacterial infections in cases of immunosuppressive use in severely ill patients. Nonetheless, to date, the efficacy of EBN as anti-inflammatory agent is yet to be compared empirically with clinically used anti-inflammatory medications. The allergenic effect of EBN also should be taken into account (Goh et al., 2001), considering the already known high risk of cytokine surge in severe IAV and COVID-19 patients. Techniques such as ultrafiltration tend to isolate larger glycoproteins, with higher allergenic potential, and periodate treatment relinquished the allergenic activity of EBN (Goh et al., 2001). It is imperative that future investigations should further explore how these techniques affect the bioactive yield of EBN, and their health-promoting effects in living organisms. The presence of contaminants such as fungus (Chen et al., 2015), and residual contaminants (Yeo et al., 2021) should be reflected in pre-clinical settings prior to clinical application. Gamma irradiation reduced the microbial activity of EBN to an undetectable level (Babji et al., 2018), whether this equally affects the efficacy of EBN in various in vivo disease models should be investigated further.
Conclusion
The anti-viral and anti-inflammatory property of EBN is promising at the pre-clinical level. Nevertheless, future studies should consider the EBN’s potential allergenic and contaminant factors in their study designs prior to its clinical application.
Author Contributions
KC, NS, MM, IM, and JK performed the literature search and drafted the manuscript. AU, KK, KC, and JK reviewed and finalized the manuscript.
Funding
This study was supported by the GUP-2018-055. Fund provided by the National University of Malaysia (UKM).
Conflict of Interest
The authors declare that the research was conducted in the absence of any commercial or financial relationships that could be construed as a potential conflict of interest.
References
Abidin, F. Z., Hui, C. K., Luan, N. S., Ramli, E. S. M., Hun, L. T., and Abd Ghafar, N. (2011). Effects of Edible Bird's Nest (EBN) on Cultured Rabbit Corneal Keratocytes. BMC Complement. Altern. Med. 11 (1), 1–10. doi:10.1186/1472-6882-11-94
Alame, M. M., Massaad, E., and Zaraket, H. (2016). Peramivir: A Novel Intravenous Neuraminidase Inhibitor for Treatment of Acute Influenza Infections. Front. Microbiol. 7, 450. doi:10.3389/fmicb.2016.00450
Albani, F., Fusina, F., Granato, E., Capotosto, C., Ceracchi, C., Gargaruti, R., et al. (2021). Corticosteroid Treatment Has No Effect on Hospital Mortality in COVID-19 Patients. Sci. Rep. 11, 1015. doi:10.1038/s41598-020-80654-x
Amiza, M. A., Oon, X. X., and Norizah, M. S. (2019). Optimization of Enzymatic Hydrolysis Conditions on the Degree of Hydrolysis of Edible Bird’s Nest Using Alcalase® and Nutritional Composition of the Hydrolysate. Food Res. 3, 570–580. doi:10.26656/fr.2017.3(5).120
Aswir, A. R., and Wan Nazaimoon, W. M. (2011). Effect of Edible Bird's Nest on Cell Proliferation and Tumor Necrosis Factor-Alpha (TNF-α) Release In Vitro. Int. Food Res. J. 18 (3), 1123–1127.
Babji, A. S., Syarmila, E. I., D'Aliah, N., Nadia, N. M., Akbar, H. D., Norrakiah, A. S., et al. (2018). Assessment on Bioactive Components of Hydrolysed Edible Bird Nest. Int. Food Res. J. 25, 1936–1941.
Bartoletti, M., Marconi, L., Scudeller, L., Pancaldi, L., Tedeschi, S., Giannella, M., et al. (2021). Efficacy of Corticosteroid Treatment for Hospitalized Patients with Severe COVID-19: a Multicentre Study. Clin. Microbiol. Infect. 27, 105–111. doi:10.1016/j.cmi.2020.09.014
Beigel, J. H., Farrar, J., Han, A. M., Hayden, F. G., Hyer, R., de Jong, M. D., et al. (2005). Avian Influenza A (H5N1) Infection in Humans. N. Engl. J. Med. 353, 1374–1385. doi:10.1056/NEJMra052211
Berri, F., Lê, V. B., Jandrot-Perrus, M., Lina, B., and Riteau, B. (2014). Switch from Protective to Adverse Inflammation during Influenza: Viral Determinants and Hemostasis Are Caught as Culprits. Cell. Mol. Life Sci. 71, 885–898. doi:10.1007/s00018-013-1479-x
BioCryst (2018). Alpivab (Peramivir): Summary of Product Characteristics. Available at: http://www.ema.europa.eu/(Accessed Aug 2, 2018).
Cai, Q., Yang, M., Liu, D., Chen, J., Shu, D., Xia, J., et al. (2020). Experimental Treatment with Favipiravir for COVID-19: An Open-Label Control Study. Engineering 6, 1192–1198. doi:10.1016/j.eng.2020.03.007
Cao, B., Wang, Y., Wen, D., Liu, W., Wang, J., Fan, G., et al. (2020). A Trial of Lopinavir-Ritonavir in Adults Hospitalized with Severe Covid-19. N. Engl. J. Med. 382, 1787–1799. doi:10.1056/NEJMoa2001282
Chan, J. F.-W., Yuan, S., Kok, K.-H., To, K. K.-W., Chu, H., Yang, J., et al. (2020). A Familial Cluster of Pneumonia Associated with the 2019 Novel Coronavirus Indicating Person-To-Person Transmission: a Study of a Family Cluster. The Lancet 395, 514–523. doi:10.1016/S0140-67362030154-9
Chandwani, A., and Shuter, J. (2008). Lopinavir/ritonavir in the Treatment of HIV-1 Infection: a Review. Ther. Clin. Risk Manag. 4, 1023–1033. doi:10.2147/tcrm.s3285
Channappanavar, R., Fehr, A. R., Vijay, R., Mack, M., Zhao, J., Meyerholz, D. K., et al. (2016). Dysregulated Type I Interferon and Inflammatory Monocyte-Macrophage Responses Cause Lethal Pneumonia in SARS-CoV-Infected Mice. Cell Host & Microbe 19 (2), 181–193. doi:10.1016/j.chom.2016.01.007
Chen, C., Zhang, Y., Huang, J., Yin, P., Cheng, Z., Wu, J., et al. (2020). Favipiravir versus Arbidol for COVID-19: A Randomized Clinical Trial. [medRxiv. Preprint]. doi:10.1101/2020.03.17.20037432
Chen, J. X. J., Wong, S. F., Lim, P. K. C., and Mak, J. W. (2015). Culture and Molecular Identification of Fungal Contaminants in Edible Bird Nests. Food Additives & Contaminants: A 32 (12), 1–10. doi:10.1080/19440049.2015.1101494
Chen, L., Liu, H. G., Liu, W., Liu, J., Liu, K., Shang, J., et al. (2020). Zhonghua Jie He He Hu Xi Za Zhi = Chinese. J. tuberculosis Respir. Dis. 43, 203–208. doi:10.3760/cma.j.issn.1001-0939.2020.03.013
Cheung, P. P. H., Watson, S. J., Choy, K.-T., Fun Sia, S., Wong, D. D. Y., Poon, L. L. M., et al. (2014). Generation and Characterization of Influenza A Viruses with Altered Polymerase Fidelity. Nat. Commun. 5 (1), 1–13. doi:10.1038/ncomms5794
Chiang, J. J., Davis, M. E., and Gack, M. U. (2014). Regulation of RIG-I-like Receptor Signaling by Host and Viral Proteins. Cytokine Growth Factor. Rev. 25, 491–505. doi:10.1016/j.cytogfr.2014.06.005
Chua, K.-H., Lee, T.-H., Nagandran, K., Md Yahaya, N. H., Lee, C.-T., Tjih, E. T. T., et al. (2013). Edible Bird's Nest Extract as a Chondro-Protective Agent for Human Chondrocytes Isolated from Osteoarthritic Knee: In Vitro Study. BMC Complement. Altern. Med. 13 (1), 1–9. doi:10.1186/1472-6882-13-19
Corfield, A. P., Veh, R. W., Wember, M., Michalski, J. C., and Schauer, R. (1981). The Release of N-Acetyl- and N-Glycolloyl-Neuraminic Acid from Soluble Complex Carbohydrates and Erythrocytes by Bacterial, Viral and Mammalian Sialidases. Biochem. J. 197, 293–299. doi:10.1042/bj1970293
Dai, Y., Cao, J., Wang, Y., Chen, Y., and Jiang, L. (2021). A Comprehensive Review of Edible Bird's Nest. Food Res. Int. 140, 109875. doi:10.1016/j.foodres.2020.109875
Daud, N. A., Yusop, S. M., Joe, L. S., and Babji, A. S. (2019). Physicochemical Properties of Glycan Extracted from Swiftlet’s Edible Bird Nest (Genus Collocalia) and its Potential as Prebiotic Component. AIP Conf. Proc. 2111, 050010. doi:10.1063/1.5111258
de Jong, M. D., Simmons, C. P., Thanh, T. T., Hien, V. M., Smith, G. J. D., Chau, T. N. B., et al. (2006). Fatal Outcome of Human Influenza A (H5N1) Is Associated with High Viral Load and Hypercytokinemia. Nat. Med. 12, 1203–1207. doi:10.1038/nm1477
Diggory, P., Fernandez, C., Humphrey, A., Jones, V., and Murphy, M. (2001). Comparison of Elderly People's Technique in Using Two Dry Powder Inhalers to Deliver Zanamivir: Randomised Controlled. BMJ 322, 577. doi:10.1136/bmj.322.7286.577
Durrant, J. D., Kochanek, S. E., Casalino, L., Ieong, P. U., Dommer, A. C., and Amaro, R. E. (2020). Mesoscale All-Atom Influenza Virus Simulations Suggest New Substrate Binding Mechanism. ACS Cent. Sci. 6, 189–196. doi:10.1021/acscentsci.9b01071
Fage, C., Tu, V., Carbonneau, J., Abed, Y., and Boivin, G. (2017). Peramivir Susceptibilities of Recombinant Influenza A and B Variants Selected with Various Neuraminidase Inhibitors. Antivir. Ther. 22 (8), 711–716. doi:10.3851/IMP3158
Furuta, Y., Gowen, B. B., Takahashi, K., Shiraki, K., Smee, D. F., and Barnard, D. L. (2013). Favipiravir (T-705), a Novel Viral RNA Polymerase Inhibitor. Antiviral Res. 100, 446–454. doi:10.1016/j.antiviral.2013.09.015
Gagneux, P., Cheriyan, M., Hurtado-Ziola, N., van der Linden, E. C. M. B., Anderson, D., McClure, H., et al. (2003). Human-specific Regulation of α2-6-linked Sialic Acids. J. Biol. Chem. 278, 48245–48250. doi:10.1074/jbc.M309813200
Gan, J. Y., Chang, L. S., Mat Nasir, N. A., Babji, A. S., and Lim, S. J. (2020). Evaluation of Physicochemical Properties, Amino Acid Profile and Bioactivities of Edible Bird's Nest Hydrolysate as Affected by Drying Methods. LWT 131, 109777. doi:10.1016/j.lwt.2020.109777
Gao, Y., Li, T., Han, M., Li, X., Wu, D., Xu, Y., et al. (2020). Diagnostic Utility of Clinical Laboratory Data Determinations for Patients with the Severe COVID‐19. J. Med. Virol. 92, 791–796. doi:10.1002/jmv.25770
GBD 2017 Influenza Collaborators (2019). Mortality, Morbidity, and Hospitalisations Due to Influenza Lower Respiratory Tract Infections, 2017: an Analysis for the Global Burden of Disease Study 2017. Lancet Respir. Med. 7, 69–89. doi:10.1016/S2213-2600(18)30496-X
Ghassem, M., Arihara, K., Mohammadi, S., Sani, N. A., and Babji, A. S. (2017). Identification of Two Novel Antioxidant Peptides from Edible Bird's Nest (Aerodramus Fuciphagus) Protein Hydrolysates. Food Funct. 8, 2046–2052. doi:10.1039/c6fo01615d
Ghosh, S. (2020). Sialic Acid and Biology of Life: An Introduction. Sialic Acids Sialoglycoconjugates Biol. Life Health Dis., 1–61. doi:10.1016/B978-0-12-816126-5.00001-9
Giansanti, F., Leboffe, L., Angelucci, F., and Antonini, G. (2015). The Nutraceutical Properties of Ovotransferrin and its Potential Utilization as a Functional Food. Nutrients 7 (11), 9105–9115. doi:10.3390/nu7115453
Giansanti, F., Panella, G., Leboffe, L., and Antonini, G. (2016). Lactoferrin from Milk: Nutraceutical and Pharmacological Properties. Pharmaceuticals 9, 61. doi:10.3390/ph9040061
Goh, D. L. M., Chew, F.-T., Chua, K.-Y., Chay, O.-M., and Lee, B.-W. (2000). Edible "bird's Nest"-Induced Anaphylaxis: An Under-recognized Entity? J. Pediatr. 137, 277–279. doi:10.1067/mpd.2000.107108
Goh, D. L. M., Chua, K. Y., Chew, F. T., Liang, R. C. M. Y., Seow, T. K., Ou, K. L., et al. (2001). Immunochemical Characterization of Edible Bird's Nest Allergens. J. Allergy Clin. Immunol. 107, 1082–1088. doi:10.1067/mai.2001.114342
Goldhill, D. H., Te Velthuis, A. J. W., Fletcher, R. A., Langat, P., Zambon, M., Lackenby, A., et al. (2018). The Mechanism of Resistance to Favipiravir in Influenza. Proc. Natl. Acad. Sci. USA 115 (45), 11613–11618. doi:10.1073/pnas.1811345115
Grein, J., Ohmagari, N., Shin, D., Diaz, G., Asperges, E., Castagna, A., et al. (2020). Compassionate Use of Remdesivir for Patients with Severe Covid-19. N. Engl. J. Med. 382, 2327–2336. doi:10.1056/NEJMoa2007016
Gu, Y., Hsu, A. C.-Y., Pang, Z., Pan, H., Zuo, X., Wang, G., et al. (2019). Role of the Innate Cytokine Storm Induced by the Influenza A Virus. Viral Immunol. 32, 244–251. doi:10.1089/vim.2019.0032
Guan, Y., Vijaykrishna, D., Bahl, J., Zhu, H., Wang, J., and Smith, G. J. D. (2010). The Emergence of Pandemic Influenza Viruses. Protein Cell 1, 9–13. doi:10.1007/s13238-010-0008-z
Gubareva, L. V., Kaiser, L., and Hayden, F. G. (2000). Influenza Virus Neuraminidase Inhibitors. The Lancet 355, 827–835. doi:10.1016/S0140-6736(99)11433-8
Gubareva, L. V., Mishin, V. P., Patel, M. C., Chesnokov, A., Nguyen, H. T., De La Cruz, J., et al. (2019). Assessing Baloxavir Susceptibility of Influenza Viruses Circulating in the United States during the 2016/17 and 2017/18 Seasons. Euro Surveill. 24, 1800666. doi:10.2807/1560-7917.ES.2019.24.3.1800666
Guo, C.-T., Takahashi, T., Bukawa, W., Takahashi, N., Yagi, H., Kato, K., et al. (2006). Edible Bird's Nest Extract Inhibits Influenza Virus Infection. Antiviral Res. 70, 140–146. doi:10.1016/j.antiviral.2006.02.005
Haghani, A., Mehrbod, P., Safi, N., Aminuddin, N. A., Bahadoran, A., Omar, A. R., et al. (2016). In vitro and In Vivo Mechanism of Immunomodulatory and Antiviral Activity of Edible Bird's Nest (EBN) against Influenza a Virus (IAV) Infection. J. Ethnopharmacology 185, 327–340. doi:10.1016/j.jep.2016.03.020
Haghani, A., Mehrbod, P., Safi, N., Kadir, F. A. i. A., Omar, A. R., and Ideris, A. (2017). Edible Bird's Nest Modulate Intracellular Molecular Pathways of Influenza A Virus Infected Cells. BMC Complement. Altern. Med. 17, 1–13. doi:10.1186/s12906-016-1498-x
Halimi, N. M., Kasim, Z. M., and Babji, A. S. (2014). Nutritional Composition and Solubility of Edible Bird Nest (Aerodramus Fuchiphagus). AIP Conf. Proc. 1614, 476–481. doi:10.1063/1.4895243
Hama, R. (2015). The Mechanisms of Adverse Reactions to Oseltamivir: Part II. Delayed Type Reactions. Clin. Microbiol. 04, 224. doi:10.4172/2327-5073.1000224
Hama, R. (2016). The Mechanisms of Delayed Onset Type Adverse Reactions to Oseltamivir. Infect. Dis. 48, 651–660. doi:10.1080/23744235.2016.1189592
Hamid, A. A., Aminuddin, A., Yunus, M. H. M., Murthy, J. K., Hui, C. K., and Ugusman, A. (2020). Antioxidative and Anti-inflammatory Activities of Polygonum Minus: a Review of Literature. Rev. Cardiovasc. Med. 21 (2), 275–287. doi:10.31083/j.rcm.2020.02.50
Haverkamp, J., Halbeek, H., Dorland, L., Vliegenthart, J. F. G., Pfeil, R., and Schauer, R. (1982). High-Resolution 1H-NMR Spectroscopy of Free and Glycosidically Linked O- Acetylated Sialic Acids. Eur. J. Biochem. 122, 305–311. doi:10.1111/j.1432-1033.1982.tb05881.x
Hayden, F. G., Sugaya, N., Hirotsu, N., Lee, N., de Jong, M. D., Hurt, A. C., et al. (2018). Baloxavir Marboxil for Uncomplicated Influenza in Adults and Adolescents. N. Engl. J. Med. 379, 913–923. doi:10.1056/NEJMoa1716197
Heo, Y.-A. (2018). Baloxavir: First Global Approval. Drugs 78, 693–697. doi:10.1007/s40265-018-0899-1
Hou, Z., Imam, M. U., Ismail, M., Mahmud, R., Ideris, A., and Ooi, D. J. (2015a). Nutrigenomic Effects of Edible Bird’s Nest On insulin Signaling in Ovariectomized Rats. Drug Des. Devel Ther. 9, 4115–4125. doi:10.2147/DDDT.S80743
Hou, Z., Imam, M. U., Ismail, M., Azmi, N. H., Ismail, N., Ideris, A., et al. (2015b). Lactoferrin and Ovotransferrin Contribute toward Antioxidative Effects of Edible Bird's Nest against Hydrogen Peroxide-Induced Oxidative Stress in Human SH-Sy5y Cells. Biosci. Biotechnol. Biochem. 79, 1570–1578. doi:10.1080/09168451.2015.1050989
Huang, C., Wang, Y., Li, X., Ren, L., Zhao, J., Hu, Y., et al. (2020). Clinical Features of Patients Infected with 2019 Novel Coronavirus in Wuhan, China. The Lancet 395, 497–506. doi:10.1016/S0140-6736(20)30183-5
Hui Yan, T., Lim, S. J., Babji, A. S., Rawi, M. H., and Sarbini, S. R. (2021). Enzymatic Hydrolysis: Sialylated Mucin (SiaMuc) Glycoprotein of Edible Swiftlet's Nest (ESN) and its Molecular Weight Distribution as Bioactive ESN SiaMuc-Glycopeptide Hydrolysate. Int. J. Biol. Macromolecules 175, 422–431. doi:10.1016/j.ijbiomac.2021.02.007
Hun, L. T., Wani, W. A., Tjih, E. T. T., Adnan, N. A., Le Ling, Y., and Aziz, R. A. (2015). Investigations into the Physicochemical, Biochemical and Antibacterial Properties of Edible Bird’s Nest. J. Chem. Pharm. Res. 7, 228–247.
Hun Lee, T., Hau Lee, C., Alia Azmi, N., Kavita, S., Wong, S., Znati, M., et al. (2020). Characterization of Polar and Non‐Polar Compounds of House Edible Bird's Nest (EBN) from Johor, Malaysia. Chem. Biodivers 17, e1900419. doi:10.1002/cbdv.201900419
Hurt, A. C. (2014). The Epidemiology and Spread of Drug Resistant Human Influenza Viruses. Curr. Opin. Virol. 8, 22–29. doi:10.1016/j.coviro.2014.04.009
Hwang, E., Park, S. W., and Yang, J. E. (2020). Anti-aging, Anti-inflammatory, and Wound-Healing Activities of Edible Bird's Nest in Human Skin Keratinocytes and Fibroblasts. Pharmacogn. Mag. 16, 336. doi:10.4103/pm.pm_326_19
Ishikawa, T. (2012). Clinical Preparedness for Cytokine Storm Induced by the Highly Pathogenic H5N1 Influenza Virus. J. Pharmacogenom. Pharmacoproteomics. 03, e131. doi:10.4172/2153-0645.1000e131
Ison, M. G., Portsmouth, S., Yoshida, Y., Shishido, T., Mitchener, M., Tsuchiya, K., et al. (2020). Early Treatment with Baloxavir Marboxil in High-Risk Adolescent and Adult Outpatients with Uncomplicated Influenza (CAPSTONE-2): a Randomised, Placebo-Controlled, Phase 3 Trial. Lancet Infect. Dis. 20, 1204–1214. doi:10.1016/s1473-3099(20)30004-9
Ison, M. G. (2010). Influenza, Including the Novel H1N1, in Organ Transplant Patients. Curr. Opin. Infect. Dis. 23, 365–373. doi:10.1097/QCO.0b013e32833bc1c3
Iuliano, A. D., Roguski, K. M., Chang, H. H., Muscatello, D. J., Palekar, R., Tempia, S., et al. (2018). Estimates of Global Seasonal Influenza-Associated Respiratory Mortality: a Modelling Study. Lancet 391, 1285–1300. doi:10.1016/S0140-6736(17)33293-2
Jackson, D., Barclay, W., and Zürcher, T. (2005). Characterization of Recombinant Influenza B Viruses with Key Neuraminidase Inhibitor Resistance Mutations. J. Antimicrob. Chemother. 55, 162–169. doi:10.1093/jac/dkh528
Jewell, N. A., Cline, T., Mertz, S. E., Smirnov, S. V., Flaño, E., Schindler, C., et al. (2010). Lambda Interferon Is the Predominant Interferon Induced by Influenza A Virus Infection In Vivo. J. Virol. 84, 11515–11522. doi:10.1128/JVI.01703-09
Jia, N., Barclay, W. S., Roberts, K., Yen, H.-L., Chan, R. W. Y., Lam, A. K. Y., et al. (2014). Glycomic Characterization of Respiratory Tract Tissues of Ferrets. J. Biol. Chem. 289, 28489–28504. doi:10.1074/jbc.M114.588541
Kalaiyarasu, S., Kumar, M., Senthil Kumar, D., Bhatia, S., Dash, S. K., Bhat, S., et al. (2016). Highly Pathogenic Avian Influenza H5N1 Virus Induces Cytokine Dysregulation with Suppressed Maturation of Chicken Monocyte-Derived Dendritic Cells. Microbiol. Immunol. 60, 687–693. doi:10.1111/1348-0421.12443
Kamali, A., and Holodniy, M. (2013). Influenza Treatment and Prophylaxis with Neuraminidase Inhibitors: a Review. Infect. Drug Resist. 6, 187–198. doi:10.2147/IDR.S36601
Kamil, K., Kumar, J., Yazid, M. D., and Hj Idrus, R. (2018). Olive and its Phenolic Compound as the Promising Neuroprotective Agent. Jsm 47, 2811–2820. doi:10.17576/jsm-2018-4711-24
Kamil, K., Yazid, M. D., Idrus, R. B. H., and Kumar, J. (2020). Hydroxytyrosol Promotes Proliferation of Human Schwann Cells: An In Vitro Study. Int. J. Environ. Res. Public Health 17 (12), 4404. doi:10.3390/ijerph17124404
Kang, C. K., Seong, M.-W., Choi, S.-J., Kim, T. S., Choe, P. G., Song, S. H., et al. (2020). In vitro activity of Lopinavir/ritonavir and Hydroxychloroquine against Severe Acute Respiratory Syndrome Coronavirus 2 at Concentrations Achievable by Usual Doses. Korean J. Intern. Med. 35 (4), 782–787. doi:10.3904/kjim.2020.157
Khamis, F., Al Naabi, H., Al Lawati, A., Ambusaidi, Z., Al Sharji, M., Al Barwani, U., et al. (2021). Randomized controlled open label trial on the use of favipiravir combined with inhaled interferon beta-1b in hospitalized patients with moderate to severe COVID-19 pneumonia. Int. J. Infect. Dis. 102, 538–543. doi:10.1016/j.ijid.2020.11.008
Kim, K. C., Kang, K. A., Lim, C. M., Park, J. H., Jung, K. S., and Hyun, J. W. (2012). Water Extract of Edible Bird's Nest Attenuated the Oxidative Stress-Induced Matrix Metalloproteinase-1 by Regulating the Mitogen-Activated Protein Kinase and Activator Protein-1 Pathway in Human Keratinocytes. J. Korean Soc. Appl. Biol. Chem. 55, 347–354. doi:10.1007/s13765-012-2030-8
Kim, H., Webster, R. G., and Webby, R. J. (2018). Influenza Virus: Dealing with a Drifting and Shifting Pathogen. Viral Immunol. 31, 174–183. doi:10.1089/vim.2017.0141
Knipe, D. M., and Howley, P. M. (2013). Fields Virology. 6th ed.. Philadelphia, PA: Wolters Kluwer/Lippincott Williams & Wilkins Health.
Ko, W.-C., Rolain, J.-M., Lee, N.-Y., Chen, P.-L., Huang, C.-T., Lee, P.-I., et al. (2020). Arguments in Favour of Remdesivir for Treating SARS-CoV-2 Infections. Int. J. Antimicrob. Agents 55, 105933. doi:10.1016/j.ijantimicag.2020.105933
Komeda, T., Ishii, S., Itoh, Y., Ariyasu, Y., Sanekata, M., Yoshikawa, T., et al. (2015). Post-marketing Safety and Effectiveness Evaluation of the Intravenous Anti-influenza Neuraminidase Inhibitor Peramivir (II): a Pediatric Drug Use Investigation. J. Infect. Chemother. 21, 194–201. doi:10.1016/j.jiac.2014.11.009
Koszalka, P., Tilmanis, D., and Hurt, A. C. (2017). Influenza Antivirals Currently in Late-phase Clinical Trial. Influenza Other Respi Viruses 11, 240–246. doi:10.1111/irv.12446
Krammer, F. (2019). The Human Antibody Response to Influenza A Virus Infection and Vaccination. Nat. Rev. Immunol. 19, 383–397. doi:10.1038/s41577-019-0143-6
La Gruta, N. L., Kedzierska, K., Stambas, J., and Doherty, P. C. (2007). A Question of Self‐preservation: Immunopathology in Influenza Virus Infection. Immunol. Cel Biol. 85, 85–92. doi:10.1038/sj.icb.7100026
Lansbury, L., Rodrigo, C., Leonardi-Bee, J., Nguyen-Van-Tam, J., and Lim, W. S. (2019). Corticosteroids as Adjunctive Therapy in the Treatment of Influenza. Cochrane Database Syst. Rev. 2, CD010406. doi:10.1002/14651858.CD010406.pub3
Lansbury, L. E., Rodrigo, C., Leonardi-Bee, J., Nguyen-Van-Tam, J., and Shen Lim, W. (2020). Corticosteroids as Adjunctive Therapy in the Treatment of Influenza. Crit. Care Med. 48, e98–e106. doi:10.1097/CCM.0000000000004093
Lau, S. K. P., Lau, C. C. Y., Chan, K.-H., Li, C. P. Y., Chen, H., Jin, D.-Y., et al. (2013). Delayed Induction of Proinflammatory Cytokines and Suppression of Innate Antiviral Response by the Novel Middle East Respiratory Syndrome Coronavirus: Implications for Pathogenesis and Treatment. J. Gen. Virol. 94, 2679–2690. doi:10.1099/vir.0.055533-0
Law, H. K. W., Cheung, C. Y., Ng, H. Y., Sia, S. F., Chan, Y. O., Luk, W., et al. (2005). Chemokine Up-Regulation in SARS-Coronavirus-Infected, Monocyte-Derived Human Dendritic Cells. Blood 106, 2366–2374. doi:10.1182/blood-2004-10-4166
Le Goffic, R., Pothlichet, J., Vitour, D., Fujita, T., Meurs, E., Chignard, M., et al. (2007). Cutting Edge: Influenza A Virus Activates TLR3-dependent Inflammatory and RIG-I-dependent Antiviral Responses in Human Lung Epithelial Cells. J. Immunol. 178, 3368–3372. doi:10.4049/jimmunol.178.6.3368
Letko, M., Marzi, A., and Munster, V. (2020). Functional Assessment of Cell Entry and Receptor Usage for SARS-CoV-2 and Other Lineage B Betacoronaviruses. Nat. Microbiol. 5, 562–569. doi:10.1038/s41564-020-0688-y
Li, H., Yang, S.-g., Gu, L., Zhang, Y., Yan, X.-x., Liang, Z.-a., et al. (2017). Effect of Low-To-Moderate-Dose Corticosteroids on Mortality of Hospitalized Adolescents and Adults with Influenza A(H1N1)pdm09 Viral Pneumonia. Influenza Other Respi Viruses 11, 345–354. doi:10.1111/irv.12456
Lim, J., Jeon, S., Shin, H. Y., Kim, M. J., Seong, Y. M., Lee, W. J., et al. (2020). Case of the index patient who caused tertiary transmission of COVID-19 infection in Korea: the application of Lopinavir/Ritonavir for the treatment of COVID-19 infected pneumonia monitored by quantitative RT-PCR. J. Korean Med. Sci. 35 (6), e79. doi:10.3346/jkms.2020.35.e79
Ling, J. W. A., Chang, L. S., Babji, A. S., and Lim, S. J. (2020). Recovery of Value‐added Glycopeptides from Edible Bird's Nest ( EBN ) Co‐products: Enzymatic Hydrolysis, Physicochemical Characteristics and Bioactivity. J. Sci. Food Agric. 100, 4714–4722. doi:10.1002/jsfa.10530
Lipsitch, M., Barclay, W., Raman, R., Russell, C. J., Belser, J. A., Cobey, S., et al. (2016). Viral Factors in Influenza Pandemic Risk Assessment. eLife 5, e18491. doi:10.7554/eLife.18491
Liu, J., Zhang, S., Dong, X., Li, Z., Xu, Q., Feng, H., et al. (2020). Corticosteroid Treatment in Severe COVID-19 Patients with Acute Respiratory Distress Syndrome. J. Clin. Invest. 130, 6417–6428. doi:10.1172/JCI140617
Long, J. S., Mistry, B., Haslam, S. M., and Barclay, W. S. (2019). Host and Viral Determinants of Influenza A Virus Species Specificity. Nat. Rev. Microbiol. 17, 67–81. doi:10.1038/s41579-018-0115-z
Looi, T., and Omar, A. R. (2016). Interface between Engineering and Medicine. Pertanika J. Scholarly Res. Rev. 2, 1–16. doi:10.1016/b978-0-08-100123-3.00001-4
Ma, J., Rubin, B. K., and Voynow, J. A. (2018). Mucins, Mucus, and Goblet Cells. Chest 154, 169–176. doi:10.1016/j.chest.2017.11.008
Ma, S., Xu, C., Liu, S., Sun, X., Li, R., Mao, M., et al. (2021). Efficacy and Safety of Systematic Corticosteroids Among Severe COVID-19 Patients: a Systematic Review and Meta-Analysis of Randomized Controlled Trials. Sig Transduct Target. Ther. 6, 83. doi:10.1038/s41392-021-00521-7
Marcone, M. F. (2005). Characterization of the Edible Bird's Nest the "Caviar of the East". Food Res. Int. 38, 1125–1134. doi:10.1016/j.foodres.2005.02.008
McAuley, J. L., Gilbertson, B. P., Trifkovic, S., Brown, L. E., and McKimm-Breschkin, J. L. (2019). Influenza Virus Neuraminidase Structure and Functions. Front. Microbiol. 10, 39. doi:10.3389/fmicb.2019.00039
McKimm-Breschkin, J. L. (2013). Influenza Neuraminidase Inhibitors: Antiviral Action and Mechanisms of Resistance. Influenza Other Respi Viruses 7, 25–36. doi:10.1111/irv.12047
McNicholl, I. R., and McNicholl, J. J. (2001). Neuraminidase Inhibitors: Zanamivir and Oseltamivir. Ann. Pharmacother. 35, 57–70. doi:10.1345/aph.10118
Moscona, A. (2005). Neuraminidase Inhibitors for Influenza. N. Engl. J. Med. 353, 1363–1373. doi:10.1056/NEJMra050740
Nelli, R. K., Kuchipudi, S. V., White, G. A., Perez, B., Dunham, S. P., and Chang, K.-C. (2010). Comparative Distribution of Human and Avian Type Sialic Acid Influenza Receptors in the Pig. BMC Vet. Res. 6, 4. doi:10.1186/1746-6148-6-4
Ni, Y.-N., Chen, G., Sun, J., Liang, B.-M., and Liang, Z.-A. (2019). The Effect of Corticosteroids on Mortality of Patients with Influenza Pneumonia: a Systematic Review and Meta-Analysis. Crit. Care 23, 99. doi:10.1186/s13054-019-2395-8
Ning, Z.-Y., Luo, M.-Y., Qi, W.-B., Yu, B., Jiao, P.-R., and Liao, M. (2009). Detection of Expression of Influenza Virus Receptors in Tissues of BALB/c Mice by Histochemistry. Vet. Res. Commun. 33, 895–903. doi:10.1007/s11259-009-9307-3
Noor, H. S. M., Babji, A. S., and Lim, S. J. (2018). Nutritional Composition of Different Grades of Edible Bird’s Nest and its Enzymatic Hydrolysis. AIP Conf. Proc. 1940, 020088. doi:10.1063/1.5028003
Noshi, T., Kitano, M., Taniguchi, K., Yamamoto, A., Omoto, S., Baba, K., et al. (2018). In vitro characterization of Baloxavir Acid, a First-In-Class Cap-dependent Endonuclease Inhibitor of the Influenza Virus Polymerase PA Subunit. Antiviral Res. 160, 109–117. doi:10.1016/j.antiviral.2018.10.008
Nuradji, H., Dharmayanti, N. I., Mranata, B., Sudarnika, E., Lukman, D. W., and Wibawan, I. W. T. (2018). Antiviral Activity of Edible Bird's Nest Extract on Highly Pathogenic Avian Influenza H5N1 Viral Infection In Vitro. Hum.Vet. Med. 10, 62–68.
Ohuchi, M., Asaoka, N., Sakai, T., and Ohuchi, R. (2006). Roles of Neuraminidase in the Initial Stage of Influenza Virus Infection. Microbes Infect. 8, 1287–1293. doi:10.1016/j.micinf.2005.12.008
Omoto, S., Speranzini, V., Hashimoto, T., Noshi, T., Yamaguchi, H., Kawai, M., et al. (2018). Characterization of Influenza Virus Variants Induced by Treatment with the Endonuclease Inhibitor Baloxavir Marboxil. Sci. Rep. 8, 9633. doi:10.1038/s41598-018-27890-4
Padilla-Quirarte, H. O., Lopez-Guerrero, D. V., Gutierrez-Xicotencatl, L., and Esquivel-Guadarrama, F. (2019). Protective Antibodies against Influenza Proteins. Front. Immunol. 10, 1677. doi:10.3389/fimmu.2019.01677
Portsmouth, S., Kawaguchi, K., Arai, M., Tsuchiya, K., and Uehara, T. (2017). Cap-dependent Endonuclease Inhibitor S-033188 for the Treatment of Influenza: Results from a Phase 3, Randomized, Double-Blind, Placebo- and Active-Controlled Study in Otherwise Healthy Adolescents and Adults with Seasonal Influenza. Open Forum Infect. Dis. 4, S734. doi:10.1093/ofid/ofx180.001
Principi, N., Camilloni, B., Alunno, A., Polinori, I., Argentiero, A., and Esposito, S. (2019). Drugs for Influenza Treatment: Is There Significant News?. Front. Med. 6, 109. doi:10.3389/fmed.2019.00109
Prom-In, S., Kaewsrichan, J., Wangpradit, N., Kien Hui, C., Yahaya, M. F., Kamisah, Y., et al. (2020). Abelmoschus Esculentus (L.) Moench's Peel Powder Improves High-Fat-Diet-Induced Cognitive Impairment in C57BL/6J Mice. Int. J. Environ. Res. Public Health 17 (15), 5513. doi:10.3390/ijerph17155513
Ralph, R., Lew, J., Zeng, T., Francis, M., Xue, B., Roux, M., et al. (2020). 2019-nCoV (Wuhan Virus), a Novel Coronavirus: Human-To-Human Transmission, Travel-Related Cases, and Vaccine Readiness. J. Infect. Dev. Ctries. 14, 3–17. doi:10.3855/jidc.12425
Renaud, C., Pergam, S. A., Polyak, C., Jain, R., Kuypers, J., Englund, J. A., et al. (2010). Early Emergence of an H275Y Mutation in a Hematopoietic Cell Transplant Recipient Treated with Intravenous Peramivir. Transpl. Infect. Dis. 12, 513–517. doi:10.1111/j.1399-3062.2010.00582.x
Rogers, G. N., and Paulson, J. C. (1983). Receptor Determinants of Human and Animal Influenza Virus Isolates: Differences in Receptor Specificity of the H3 Hemagglutinin Based on Species of Origin. Virology 127, 361–373. doi:10.1016/0042-6822(83)90150-2
Ruan, Q., Yang, K., Wang, W., Jiang, L., and Song, J. (2020). Clinical Predictors of Mortality Due to COVID-19 Based on an Analysis of Data of 150 Patients from Wuhan, China. Intensive Care Med. 46, 846–848. doi:10.1007/s00134-020-05991-x
Samson, M., Pizzorno, A., Abed, Y., and Boivin, G. (2013). Influenza Virus Resistance to Neuraminidase Inhibitors. Antiviral Res. 98, 174–185. doi:10.1016/j.antiviral.2013.03.014
Sano, K., Ainai, A., Suzuki, T., and Hasegawa, H. (2017). The Road to a More Effective Influenza Vaccine: up to Date Studies and Future Prospects. Vaccine 35, 5388–5395. doi:10.1016/j.vaccine.2017.08.034
Saunders-Hastings, P., and Krewski, D. (2016). Reviewing the History of Pandemic Influenza: Understanding Patterns of Emergence and Transmission. Pathogens 5, 66. doi:10.3390/pathogens5040066
Scott, L. J. (2018). Peramivir: A Review in Uncomplicated Influenza. Drugs 78, 1363–1370. doi:10.1007/s40265-018-0981-8
Sheu, T. G., Deyde, V. M., Okomo-Adhiambo, M., Garten, R. J., Xu, X., Bright, R. A., et al. (2008). Surveillance for Neuraminidase Inhibitor Resistance Among Human Influenza A and B Viruses Circulating Worldwide from 2004 to 2008. Antimicrob. Agents Chemother. 52, 3284–3292. doi:10.1128/AAC.00555-08
Sheu, C. C., Chang, W. A., Tsai, M. J., Tsai, J. R., and Chong, I. W. (2020). Early Corticosteroid Treatment in Patients with Influenza-Associated Acute Respiratory Distress Syndrome. Am. J. Respir. Crit. Care Med. 201, A2769.
Shi, Y., Wu, Y., Zhang, W., Qi, J., and Gao, G. F. (2014). Enabling the 'host Jump': Structural Determinants of Receptor-Binding Specificity in Influenza A Viruses. Nat. Rev. Microbiol. 12, 822–831. doi:10.1038/nrmicro3362
Shimizu, M. (2019). “Clinical Features of Cytokine Storm Syndrome,” in Cytokine Storm Syndrome. Editors R. Cron, and E. Behrens (Cham: Springer), 31–41. doi:10.1007/978-3-030-22094-5_3
Shinya, K., Gao, Y., Cilloniz, C., Suzuki, Y., Fujie, M., Deng, G., et al. (2012). Integrated Clinical, Pathologic, Virologic, and Transcriptomic Analysis of H5N1 Influenza Virus-Induced Viral Pneumonia in the Rhesus Macaque. J. Virol. 86, 6055–6066. doi:10.1128/JVI.00365-12
Shionogi and Co. Ltd (2018). XofluxaTM (Baloxavir): Japanese Prescribing Information. Available at: http://www.pmda.go.jp/(Accessed Feb 26, 2018).
Shirley, M. (2020). Baloxavir Marboxil: A Review in Acute Uncomplicated Influenza. Drugs 80, 1109–1118. doi:10.1007/s40265-020-01350-8
Shuto, H., Komiya, K., Yamasue, M., Uchida, S., Ogura, T., Mukae, H., et al. (2020). A Systematic Review of Corticosteroid Treatment for Noncritically Ill Patients with COVID-19. Sci. Rep. 10, 20935. doi:10.1038/s41598-020-78054-2
Smits, S. L., de Lang, A., van den Brand, J. M. A., Leijten, L. M., van IJcken, W. F., Eijkemans, M. J. C., et al. (2010). Exacerbated Innate Host Response to SARS-CoV in Aged Non-human Primates. Plos Pathog. 6, e1000756. doi:10.1371/journal.ppat.1000756
Takashita, E., Kawakami, C., Morita, H., Ogawa, R., Fujisaki, S., Shirakura, M., et al. (2019). Detection of Influenza A(H3N2) Viruses Exhibiting Reduced Susceptibility to the Novel Cap-dependent Endonuclease Inhibitor Baloxavir in Japan, December 2018. Euro Surveill. 24, 1800698. doi:10.2807/1560-7917.ES.2019.24.3.1800698
Thompson, M. R., Kaminski, J. J., Kurt-Jones, E. A., and Fitzgerald, K. A. (2011). Pattern Recognition Receptors and the Innate Immune Response to Viral Infection. Viruses 3, 920–940. doi:10.3390/v3060920
Tisoncik, J. R., Korth, M. J., Simmons, C. P., Farrar, J., Martin, T. R., and Katze, M. G. (2012). Into the Eye of the Cytokine Storm. Microbiol. Mol. Biol. Rev. 76, 16–32. doi:10.1128/MMBR.05015-11
Tong, S., Zhu, X., Li, Y., Shi, M., Zhang, J., Bourgeois, M., et al. (2013). New World Bats Harbor Diverse Influenza A Viruses. Plos Pathog. 9, e1003657. doi:10.1371/journal.ppat.1003657
Tsai, M.-J., Yang, K. Y., Yang, K.-Y., Chan, M.-C., Kao, K.-C., Wang, H.-C., et al. (2020). Impact of Corticosteroid Treatment on Clinical Outcomes of Influenza-Associated ARDS: a Nationwide Multicenter Study. Ann. Intensive Care 10, 26. doi:10.1186/s13613-020-0642-4
Uhlendorff, J., Matrosovich, T., Klenk, H.-D., and Matrosovich, M. (2009). Functional Significance of the Hemadsorption Activity of Influenza Virus Neuraminidase and its Alteration in Pandemic Viruses. Arch. Virol. 154, 945–957. doi:10.1007/s00705-009-0393-x
V'kovski, P., Kratzel, A., Steiner, S., Stalder, H., and Thiel, V. (2020). Coronavirus Biology and Replication: Implications for SARS-CoV-2. Nat. Rev. Microbiol. 19, 1–16. doi:10.1038/s41579-020-00468-6
van Paassen, J., Vos, J. S., Hoekstra, E. M., Neumann, K. M. I., Boot, P. C., and Arbous, S. M. (2020). Corticosteroid Use in COVID-19 Patients: a Systematic Review and Meta-Analysis on Clinical Outcomes. Crit. Care 24, 696. doi:10.1186/s13054-020-03400-9
Vimala, B., Hussain, H., and Nazaimoon, W. M. W. (2012). Effects of Edible Bird's Nest on Tumour Necrosis Factor-Alpha Secretion, Nitric Oxide Production and Cell Viability of Lipopolysaccharide-Stimulated RAW 264.7 Macrophages. Food Agric. Immunol. 23, 303–314. doi:10.1080/09540105.2011.625494
Walther, T., Karamanska, R., Chan, R. W. Y., Chan, M. C. W., Jia, N., Air, G., et al. (2013). Glycomic Analysis of Human Respiratory Tract Tissues and Correlation with Influenza Virus Infection. Plos Pathog. 9, e1003223. doi:10.1371/journal.ppat.1003223
Wang, M. Z., Tai, C. Y., and Mendel, D. B. (2002). Mechanism by Which Mutations at His274 Alter Sensitivity of Influenza a Virus N1 Neuraminidase to Oseltamivir Carboxylate and Zanamivir. Antimicrob Agents Chemother. 46, 3809–3816. doi:10.1128/aac.46.12.3809-3816.2002
Wang, N., Shi, X., Jiang, L., Zhang, S., Wang, D., Tong, P., et al. (2013). Structure of MERS-CoV Spike Receptor-Binding Domain Complexed with Human Receptor DPP4. Cell Res 23, 986–993. doi:10.1038/cr.2013.92
Wang., X., Pan, Z., and Cheng, Z. (2020). Association between 2019-nCoV Transmission and N95 Respirator Use. J. Hosp. Infect. 105, 104–105. doi:10.1101/2020.02.18.20021881
Wang, Y., Zhang, D., Du, G., Du, R., Zhao, J., Jin, Y., et al. (2020). Remdesivir in Adults with Severe COVID-19: a Randomised, Double-Blind, Placebo-Controlled, Multicentre Trial. The Lancet 395, 1569–1578. doi:10.1016/S0140-6736(20)31022-9
Wang, Z., Chen, X., Lu, Y., Chen, F., and Zhang, W. (2020). Clinical Characteristics and Therapeutic Procedure for Four Cases with 2019 Novel Coronavirus Pneumonia Receiving Combined Chinese and Western Medicine Treatment. Biosci. Trends. 14, 64–68. doi:10.5582/bst.2020.01030
Ward, P., Small, I., Smith, J., Suter, P., and Dutkowski, R. (2005). Oseltamivir (Tamiflu) and its Potential for Use in the Event of an Influenza Pandemic. J. Antimicrob. Chemother. 55, i5–i21. doi:10.1093/jac/dki018
WHO Rapid Evidence Appraisal for COVID-19 Therapies (REACT) Working Group, Sterne, J., Sterne, J. A. C., Murthy, S., Diaz, J. V., Slutsky, A. S., Villar, J., et al. (2020). Association between Administration of Systemic Corticosteroids and Mortality Among Critically Ill Patients with COVID-19: A Meta-Analysis. JAMA 324, 1330–1341. doi:10.1001/jama.2020.17023
Witcher, R., Tracy, J., Santos, L., and Chopra, A. (2019). Outcomes and Adverse Effects with Peramivir for the Treatment of Influenza H1N1 in Critically Ill Pediatric Patients. J. Pediatr. Pharmacol. Ther. 24, 497–503. doi:10.5863/1551-6776-24.6.497
Woo, P. C. Y., Tung, E. T. K., Chan, K. H., Lau, C. C. Y., Lau, S. K. P., and Yuen, K. Y. (2010). Cytokine Profiles Induced by the Novel Swine‐Origin Influenza A/H1N1 Virus: Implications for Treatment Strategies. J. Infect. Dis. 201, 346–353. doi:10.1086/649785
Worldometer (2020). Worldometer. Available at: https://www.worldometers.info/coronavirus/(Accessed on 10 20, 2020).
Wrapp, D., Wang, N., Corbett, K. S., Goldsmith, J. A., Hsieh, C.-L., Abiona, O., et al. (2020). Cryo-EM Structure of the 2019-nCoV Spike in the Prefusion Conformation. Science 367, 1260–1263. doi:10.1126/science.abb2507
Wu, F., Zhao, S., Yu, B., Chen, Y.-M., Wang, W., Song, Z.-G., et al. (2020). A New Coronavirus Associated with Human Respiratory Disease in China. Nature 579, 265–269. doi:10.1038/s41586-020-2008-3
Xagorari, A., and Chlichlia, K. (2008). Toll-like Receptors and Viruses: Induction of Innate Antiviral Immune Responses. Tomicroj 2, 49–59. doi:10.2174/1874285800802010049
Yagi, H., Yasukawa, N., Yu, S.-Y., Guo, C.-T., Takahashi, N., Takahashi, T., et al. (2008). The Expression of Sialylated High-Antennary N-Glycans in Edible Bird's Nest. Carbohydr. Res. 343 (8), 1373–1377. doi:10.1016/j.carres.2008.03.031
Yang, X., Steukers, L., Forier, K., Xiong, R., Braeckmans, K., Van Reeth, K., et al. (2014). A Beneficiary Role for Neuraminidase in Influenza Virus Penetration through the Respiratory Mucus. PloS one 9, e110026. doi:10.1371/journal.pone.0110026
Yang, J.-W., Fan, L.-C., Miao, X.-Y., Mao, B., Li, M.-H., Lu, H.-W., et al. (2015). Corticosteroids for the Treatment of Human Infection with Influenza Virus: a Systematic Review and Meta-Analysis. Clin. Microbiol. Infect. 21, 956–963. doi:10.1016/j.cmi.2015.06.022
Yang, T. (2019). Baloxavir Marboxil: the First Cap-dependent Endonuclease Inhibitor for the Treatment of Influenza. Ann. Pharmacother. 53, 754–759. doi:10.1177/1060028019826565
Ye, Q., Wang, B., and Mao, J. (2020). The Pathogenesis and Treatment of the `Cytokine Storm' in COVID-19. J. Infect. 80, 607–613. doi:10.1016/j.jinf.2020.03.037
Ye, X. T., Luo, Y. L., Xia, S. C., Sun, Q. F., Ding, J. G., Zhou, Y., et al. (2020). Clinical Efficacy of Lopinavir/ritonavir in the Treatment of Coronavirus Disease 2019. Eur. Rev. Med. Pharmacol. Sci. 24, 3390–3396. doi:10.26355/eurrev_202003_20706
Yeo, B.-H., Tang, T.-K., Wong, S.-F., Tan, C.-P., Wang, Y., Cheong, L.-Z., et al. (2021). Potential Residual Contaminants in Edible Bird's Nest. Front. Pharmacol. 12, 631136. doi:10.3389/fphar.2021.631136
Yew, M. Y., Koh, R. Y., Chye, S. M., Othman, I., and Ng, K. Y. (2014). Edible Bird's Nest Ameliorates Oxidative Stress-Induced Apoptosis in SH-Sy5y Human Neuroblastoma Cells. BMC Complement. Altern. Med. 14, 1–12. doi:10.1186/1472-6882-14-391
Yida, Z., Imam, M. U., Ismail, M., Hou, Z., Abdullah, M. A., Ideris, A., et al. (2015). Edible Bird's Nest Attenuates High Fat Diet-Induced Oxidative Stress and Inflammation via Regulation of Hepatic Antioxidant and Inflammatory Genes. BMC Complement. Altern. Med. 15, 310. doi:10.1186/s12906-015-0843-9
Yoshida, R., Kohno, S., Kida, H., and Sugaya, N. (2011). Neuraminidase Sequence Analysis and Susceptibilities to Neuraminidase (NA) Inhibitors of Influenza Virus Isolated from Peramivir Clinical Studies. Sapporo, Japan: International Union of Microbiological Societies Congress.
Yoshimoto, F. K. (2020). The Proteins of Severe Acute Respiratory Syndrome Coronavirus-2 (SARS CoV-2 or N-COV19), the Cause of COVID-19. Protein J. 39, 198–216. doi:10.1007/s10930-020-09901-4
Yuan, M., Xu, X., Xia, D., Tao, Z., Yin, W., Tan, W., et al. (2020). Effects of Corticosteroid Treatment for Non-severe COVID-19 Pneumonia: A Propensity Score-Based Analysis. Shock 54, 638–643. doi:10.1097/SHK.0000000000001574
Zhang, Y.-Z., and Holmes, E. C. (2020). A Genomic Perspective on the Origin and Emergence of SARS-CoV-2. Cell 181, 223–227. doi:10.1016/j.cell.2020.03.035
Zhang, Y., Sun, W., Svendsen, E. R., Tang, S., MacIntyre, R. C., Yang, P., et al. (2015). Do corticosteroids Reduce the Mortality of Influenza A (H1N1) Infection? A Meta-Analysis. Crit. Care 19, 46. doi:10.1186/s13054-015-0764-5
Zhao, R., Li, G., Kong, X. J., Huang, X. Y., Li, W., Zeng, Y. Y., et al. (2016). The Improvement Effects of Edible Bird's Nest on Proliferation and Activation of B Lymphocyte and its Antagonistic Effects on Immunosuppression Induced by Cyclophosphamide. Drug Des. Devel. Ther. 10, 371–381. doi:10.2147/DDDT.S88193
Zhao, S., Zhuang, Z., Ran, J., Lin, J., Yang, G., Yang, L., et al. (2020). The Association between Domestic Train Transportation and Novel Coronavirus (2019-nCoV) Outbreak in China from 2019 to 2020: a Data-Driven Correlational Report. Trav. Med. Infect. Dis. 33, 101568. doi:10.1016/j.tmaid.2020.101568
Zhou, Y., Fu, X., Liu, X., Huang, C., Tian, G., Ding, C., et al. (2020). Use of Corticosteroids in Influenza-Associated Acute Respiratory Distress Syndrome and Severe Pneumonia: a Systemic Review and Meta-Analysis. Sci. Rep. 10, 3044. doi:10.1038/s41598-020-59732-7
Keywords: edible bird’s nest, anti-viral, anti-infammatory, influenza, coronavirus, COVID-19, cytokine, bird nest
Citation: Chua KH, Mohamed IN, Mohd Yunus MH, Shafinaz Md Nor N, Kamil K, Ugusman A and Kumar J (2021) The Anti-Viral and Anti-Inflammatory Properties of Edible Bird’s Nest in Influenza and Coronavirus Infections: From Pre-Clinical to Potential Clinical Application. Front. Pharmacol. 12:633292. doi: 10.3389/fphar.2021.633292
Received: 25 November 2020; Accepted: 26 April 2021;
Published: 07 May 2021.
Edited by:
Karl Tsim, Hong Kong University of Science and Technology, ChinaReviewed by:
Hou Hou, Chengde Medical College, ChinaYang-Mooi Lim, Universiti Tunku Abdul Rahman, Malaysia
Jianping Chen, The Fourth Clinical Medical College of Guangzhou University of Chinese Medicine, China
Copyright © 2021 Chua, Mohamed, Mohd Yunus, Shafinaz Md Nor, Kamil, Ugusman and Kumar. This is an open-access article distributed under the terms of the Creative Commons Attribution License (CC BY). The use, distribution or reproduction in other forums is permitted, provided the original author(s) and the copyright owner(s) are credited and that the original publication in this journal is cited, in accordance with accepted academic practice. No use, distribution or reproduction is permitted which does not comply with these terms.
*Correspondence: Jaya Kumar, amF5YWt1bWFyQHVrbS5lZHUubXk=