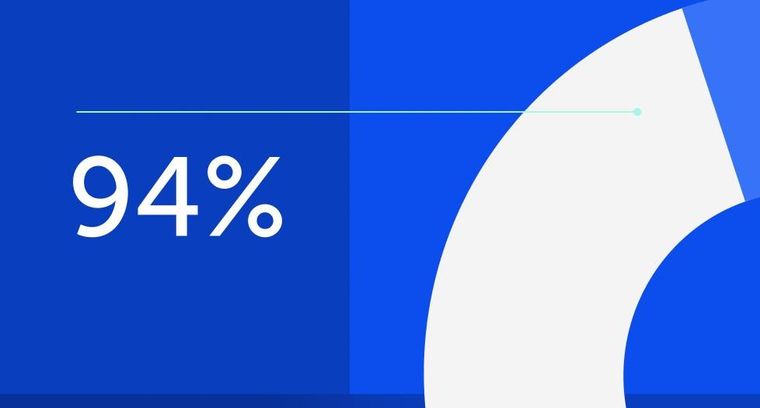
94% of researchers rate our articles as excellent or good
Learn more about the work of our research integrity team to safeguard the quality of each article we publish.
Find out more
ORIGINAL RESEARCH article
Front. Pharmacol., 29 January 2021
Sec. Experimental Pharmacology and Drug Discovery
Volume 12 - 2021 | https://doi.org/10.3389/fphar.2021.632815
This article is part of the Research TopicRecent Advances in G Protein-Coupled Receptor Signalling: Impact of Intracellular Location, Environment and Biased AgonismView all 9 articles
G-protein-coupled-receptor (GPCR) signaling is exquisitely controlled to achieve spatial and temporal specificity. The endogenous protein kinase inhibitor peptide (PKI) confines the spatial and temporal spread of the activity of protein kinase A (PKA), which integrates inputs from three major types of GPCRs. Despite its wide usage as a pharmaceutical inhibitor of PKA, it was unclear whether PKI only inhibits PKA activity. Here, the effects of PKI on 55 mouse kinases were tested in in vitro assays. We found that in addition to inhibiting PKA activity, both PKI (6–22) amide and full-length PKIα facilitated the activation of multiple isoforms of protein kinase C (PKC), albeit at much higher concentrations than necessary to inhibit PKA. Thus, our results call for appropriate interpretation of experimental results using PKI as a pharmaceutical agent. Furthermore, our study lays the foundation to explore the potential functions of PKI in regulating PKC activity and in coordinating PKC and PKA activities.
G-protein-coupled receptor (GPCR) signaling is highly regulated, and its exquisite spatial and temporal specificity has important implications on its function. Spatially, GPCRs and their signaling components are located in specific cell types, subcellular compartments, and microdomains, and their spatial localization specifies their functions (Smith et al., 2006; Chen and Sabatini, 2012; Lur and Higley, 2015; Jong et al., 2018; Thomsen et al., 2018; Lobingier and von Zastrow, 2019; Weinberg et al., 2019). Temporally, GPCR activation can lead to transient, sustained, or oscillatory patterns of intracellular signals, and the timing of GPCR activation relative to synaptic inputs is critical for how synapses are modified in the nervous system (Gu and Yakel, 2011; Muñoz and Rudy, 2014; Yagishita et al., 2014; Grundmann and Kostenis, 2017). Thus, understanding the regulators of the spatial and temporal features of GPCR signaling is important to understand GPCR functions.
One important intracellular integrator that lies downstream of multiple GPCRs is protein kinase A (PKA) (Gilman, 1995; Chen et al., 2017). PKA is activated by cyclic AMP (cAMP), which is produced by adenylate cyclases (ACs) (Krebs et al., 1959; Sutherland et al., 1968; Gilman, 1995). AC activity is stimulated by Gαs-coupled receptors and inhibited by Gαi-coupled receptors (Gilman, 1995). Furthermore, we recently discovered that endogenous Gαq-coupled receptors also activate hippocampal PKA (Chen et al., 2017). Therefore, three out of the four classes of GPCRs converge to regulate PKA activity. Furthermore, PKA phosphorylates diverse substrates to regulate synaptic and cellular functions, both within and outside the nervous system (Brandon et al., 1997; Greengard, 2001). Therefore, PKA is a biochemical integrator of signaling from numerous GPCRs that exert important cellular and physiological functions.
The spatial and temporal specificity of PKA is under exquisite control, notably by the A-kinase-anchoring proteins (AKAPs), the regulatory subunits of PKA, and the endogenously expressed PKA inhibitory peptide, PKI (Walsh et al., 1971; Taylor et al., 1990; Dalton and Dewey, 2006; Smith et al., 2006). PKI binds to the catalytic subunit of PKA, thereby preventing it from being active (Ashby and Walsh, 1972, Ashby and Walsh, 1973) despite release from a PKA regulatory subunit. Synaptic stimulation decreases the expression of one of the isoforms of PKI, PKIα (De Lecea et al., 1998). Furthermore, chronic infusion of antisense pkiα reduces neuronal excitability and eliminates hippocampal plasticity (De Lecea et al., 1998). Therefore, PKI inhibits PKA activity and has important cellular functions.
In addition to the important functions of endogenous PKI, shorter peptides of PKI, for example PKI (6–22) amide, are widely used as pharmaceutical agents to inhibit PKA activity (Cheng et al., 1986; Glass et al., 1989a, Glass et al., 1989b). However, it was unclear if PKI inhibits only PKA or if it inhibits other enzymes as well. Previous studies examined the effect of PKI on a few kinases, including protein kinase G (PKG) and protein kinase C (PKC) (Gill et al., 1976; Cheng et al., 1986; Glass et al., 1986; Day et al., 1989; Smith et al., 1990), but the effects of PKI on a broad panel of kinases were not known.
Here, we analyzed the effect of PKI on 55 kinases, selected based on their similarity to PKA and their expression patterns. We confirmed that both PKI (6–22) amide and full length PKIα inhibited PKA activity with sub-nanomolar IC50. In addition, we found that at high concentrations, PKI (6–22) amide inhibited calcium/calmodulin-dependent protein kinase I (CamK1). Surprisingly, at concentrations often used in pharmacology experiments, PKI (6–22) amide facilitated the activity of multiple PKC isoforms, rho-associated, coiled-coil-containing protein kinase 1 (ROCK1), and p70S6 Kinase (p70S6K). Synthesized full length PKIα also facilitated the activity of ROCK1 and multiple PKC isoforms. These results are important not only for interpretation of experiments using PKI as a pharmacological agent, but also sheds light on potential biological functions of endogenous PKI.
PKA inhibitor (PKI) fragment (6–22) amide was purchased from Tocris (Cat. No. 1904). Full length mouse PKIα was synthesized from L amino acids and then purified with high-performance liquid chromatography (HPLC) by the Koch Institute Biopolymers and Proteomics Facility. Mouse PKIα shares 74 out of the 76 amino acids with human PKIα, and the two differing amino acids are not in the 6–22 region.
Kinase assays were conducted in cell-free, in vitro assays with purified kinases with the Thermo Fisher Scientific SelectScreen Kinase Profiling Service. CamK1, DAPK1, and NUAK1 (ARK5) were screened with the Adapta Universal Kinase Assay, and the rest of the kinases were screened with the Z′-LYTE Peptide Kinase Assay (Rodems et al., 2002) (Figure 1B). All kinases used were human proteins expressed in and purified from Sf9 insect cell culture. Only one lot of kinase was used for each assay. Kinase activity was determined and reported on the lot-specific Certificate of Analysis at Thermo Fisher web site. The kinase concentration used in the assay was adjusted to achieve ∼25% substrate phosphorylation, with 10–50% as the allowable range in the 0% inhibition control. The % inhibition value was calculated with the formula specified in the assay manuals.
FIGURE 1. Kinase selection and screening strategy for the effect of PKI on kinases. (A) Schematic showing how 55 kinases were selected out of ∼510 mouse kinases for screening of the effect of PKI on kinase activity. (B) Schematic illustrating the principle of the Z′-LYTE Kinase Assay from Thermo Fisher (Rodems et al., 2002). With no inhibition, the kinase phosphorylates the kinase substrate that separates between the donor (coumarin) and acceptor (fluorescein). The phosphorylation prevents subsequent cleavage by a site-specific protease. Thus, the uncleaved phosphorylated product will exhibit FÖrster Resonance Energy Transfer (FRET). With effective kinase inhibition, the peptide is not phosphorylated and is subsequently cleaved by the protease, and hence there is no FRET.
All the raw data can be found in Supplementary Table S1. Dose-response curves were fit using variable-slope nonlinear regression (4 parameters) in GraphPad Prism (GraphPad Software). Kruskal-Wallis followed by Dunn’s Multiple Comparison Test was used to evaluate statistical significance in Supplementary Figure S1.
To investigate whether PKI has effects on kinases beyond PKA, we screened the effects of PKI on a panel of kinases. There are about 510 kinases encoded in the mouse genome, and screening all of them would be too costly. Therefore, we limited our selection of kinases to screen via a tiered system (Figure 1A). First, among the 510 kinases, 322 can be screened for activity enhancement or inhibition via either Adapta Universal Kinase Assay or the Z′-LYTE Peptide Kinase Assay. These are in vitro, cell-free assays using purified kinases and therefore suitable for measuring direct kinase action. Second, since PKA is a serine/threonine kinase, we deduced that PKI would most likely target other serine/threonine kinases. Thus, we limited our screen to the 191 serine/threonine kinases among the 322 kinases that can be screened. Third, among the 191 serine/threonine kinases, we reasoned that PKI would mostly act on two kinase groups: the AGC kinases of which PKA is a member, and the CamK kinases since these share substrate similarities with PKA. In total, among the 191 serine/threonine kinases, 81 kinases belong to the AGC and CamK groups. Finally, since PKI plays important roles in neuronal function by regulating synaptic plasticity, and small peptide of PKI has been extensively used as pharmaceutical agent to inhibit PKA activity in the cortex, striatum, and hippocampus, we further narrowed down to 55 kinases that are expressed in these three brain regions based on RNA sequencing data (Cembrowski et al., 2016; Saunders et al., 2018; Zeisel et al., 2018). Therefore, we screened the effects of PKI on 55 kinases, which were selected based on the availability of kinase screen, kinase groups, and expression pattern of these kinases.
Fifty-two kinases were screened with the Z′-LYTE Peptide Kinase Assay (Figure 1B) (Rodems et al., 2002) and three kinases were screened with the Adapta Universal Kinase Assay. In the Z′-LYTE assay, the kinase substrate is flanked by a donor and acceptor fluorophore. Phosphorylation of the substrate prevents subsequent cleavage by a site-specific protease, and thus enables Förster Resonance Energy Transfer (FRET) between the two fluorophores. In contrast, kinase inhibition results in no phosphorylation, and the substrate can therefore be cleaved by the protease, resulting in no FRET. The degree of FRET is therefore correlated with kinase activity. For three of the 55 kinases [CamK1, death-associated protein kinase 1 (DAPK1), and AMPK-related protein kinase 5 (ARK5)], Z′-LYTE assays were not available, and Adapta Universal Kinase Assay was used instead. Adapta is a fluorescence-based immunoassay that detects ADP produced by kinase activity. In summary, two cell-free kinase assays were used to screen for the effect of PKI on fifty-five serine/threonine kinases.
PKI (6–22) amide was used to for the initial screen because this short peptide is a potent inhibitor of PKA and has been widely used to inhibit PKA activity in numerous studies. We first determined with a high concentration of PKI (6–22) amide (5 µM) the kinases whose activities are PKI sensitive at all and therefore merit further studies with dose response curves. As expected, 5 µM PKI (6–22) amide effectively inhibited PKA activity by 85%. PKI (6–22) amide had no effect on the majority of other kinases. However, at 5 μM, PKI (6–22) amide inhibited five other kinases by more than 10%. Surprisingly, PKI (6–22) amide (5 µM) enhanced the activity of 20 kinases by more than 10% (expressed in Figure 2 as negative inhibition). Notably, the eight most facilitated kinases were all isoforms of the PKC family. The amount of facilitation ranged from 59 to 93% (Figure 2).
FIGURE 2. PKI (6–22) amide (5 µM) inhibits some kinases but facilitates others. This bar graph shows the % inhibition of 55 kinases by PKI (6–22) amide (5 µM). The kinases are sorted from the most inhibited (top) to the most facilitated (bottom). Dotted lines show ±10% inhibition. N = 2 for each enzyme. The graph shows individual data points (aligned dots) and median with range (lines).
Since we were surprised by the facilitating effect of PKI (6–22) amide on the PKC family, we set out to disambiguate the potential effect of PKI (6–22) amide on PKC in the kinase reaction, on the protease reaction (development reaction), or on fluorescence itself. PKI (6–22) amide did not induce any significant fluorescence readout change when added in or after the development reaction, but did induce a large change when added in the kinase reaction for PKC epsilon (Supplementary Figure S1). These control experiments indicate that PKI (6–22) amide indeed facilitated PKC activity.
To determine the concentration at which PKI (6–22) amide exerts effects, the dose-response curves of PKI (6–22) amide on activities of 11 selected kinases were measured. These kinases included 1) PKA, 2) all five other kinases whose activity is inhibited by more than 10% at 5 μM, and 3) five selected kinases whose activity is increased by more than 10% at 5 µM – three of these are PKC isoforms. The IC50 of PKI (6–22) amide for PKA was 0.61 nM, comparable to the value determined from previous studies (Glass et al., 1989a) (Figure 3A). PKI (6–22) amide altered the other kinase activities at much higher concentrations (Figures 3A,B). However, at the PKI concentrations often used in pharmacology experiments (1–10 µM), there were clear effects on several kinases. For example, at 5 μM, PKI (6–22) amide facilitated PKCα and PKCζ by more than 50%. Even at 1.7 µM, PKI (6–22) amide inhibited CamK1 activity by 30%, and facilitated PKCα activity by 33%.
FIGURE 3. Dose-response curves of the effects of PKI (6–22) amide on kinase activity. (A) Dose-response curve of PKI (6–22) amide on inhibited kinases. (B) Dose response curve of PKI (6–22) amide on facilitated kinases. The 5 µM PKI(6–22) amide replicates for the relevant enzymes from Figure 2 were also plotted with the 5 µM PKI(6–22) amide data here to show variability of data. N = 2–4 for each PKI concentration and each enzyme. The graph shows individual replicates (staggered dots) and fitted curves for each enzyme.
Full length PKI may display different potency and specificity compared with the short peptide. Therefore, although the screen with PKI (6–22) amide above was useful in determining the specificity of PKI as pharmaceutical agents, it is important to perform dose-response curves with full length PKI to assess whether endogenous PKI may alter activities of kinases other than PKA. Full length mouse PKIα protein was synthesized and purified by High-Performance Liquid Chromatography (HPLC), and was subsequently used to determine the dose response curve of seven kinases (Figure 4).
FIGURE 4. Dose-response curves of the effects of full length PKIα on kinase activity. Dose-response curve of full length PKIα on the activities of seven kinases. Note a negative value in % inhibition means PKI facilitates kinase activity. N = 2 for each concentration. The graph shows individual replicates (staggered symbols) and fitted curves.
PKIα inhibited PKA activity with an IC50 of 0.11 nM, with higher potency than PKI (6–22) amide (Figure 4). PKIα did not have significant effect on CamK1 or p70S6K (Figure 4). In addition, PKIα also facilitated multiple PKC isoforms and ROCK1 at much higher concentrations (1–5 µM) (Figure 4). At 5 μM, full length PKIα facilitated PKCα, PKCβII, PKCζ, and ROCK1 by 25, 36, 42, and 24% respectively.
In order to understand the specificity of PKI on kinases, we screened both PKI (6–22) amide and full length PKIα against a panel of 55 kinases. In addition to inhibiting PKA activity, PKI (6–22) amide also inhibited CaMK1, and facilitated the activities of p70S6K, ROCK1, and multiple PKC isoforms. Full length PKIα, at high concentrations, also facilitated the activity of ROCK1 and multiple PKC isoforms. These results inform the design and interpretation of experiments using PKI as a pharmacological agent, provide a starting point to explore novel functions of endogenous PKI, and raise the interesting possibility that PKI may act as a molecular switch between PKA and PKC activity.
We found that in addition to inhibiting PKA activity, PKI also facilitated the kinase activity of multiple PKC isoforms. This was surprising because previous studies found little influence of PKI on PKC (Day et al., 1989; Smith et al., 1990). In principle, this could be due to PKI interfering with 1) the protease reaction, 2) the fluorescence detection, or 3) the FRET response. Interference with the protease reaction was minimal, because PKI had very little effect on the control assay with unphosphorylated cleavable substrates (PKI + no ATP vs. no ATP conditions in the kinase reaction). In addition, adding PKI in the protease development reaction for PKC did not result in a significant FRET change (Supplementary Figure S1). Similarly, neither full length PKIα nor PKI (6–22) amide is autofluorescent, as evidenced by the test compound fluorescence interference control with no kinase/peptide mixture. Finally, PKI did not have any significant effects on FRET itself, because the FRET readout was not significantly different when PKI was added after the development reaction. These data indicate that PKI facilitated the kinase activity of multiple PKC isoforms.
Several technical differences may explain the differences between our results and previous studies that did not find significant influence of PKI on PKC. First, these studies differed in the form of PKI used: Smith et al. (Smith et al., 1990) used PKI-tide whose sequence is IAAGRTGRRQAIHDILVAA, whereas we used PKI (6–22) amide whose sequence is TYADFIASGRTGRRNAI (bolded amino acids are the shared fragment, and differences in sequences within the shared fragment are underlined). Second, the forms of PKC used are different: we used purified human recombinant PKC isoforms expressed in insect cells, whereas previous studies used partially purified PKC without clear distinction of isoforms (Day et al., 1989; Smith et al., 1990). Finally, although previous results did not reach statistical significance, Day et al. (Day et al., 1989) showed a mild enhancement of PKC activity by full length PKI in a cell-based assay with PKI transfection.
PKI (6–22) amide and other short peptides of PKI are widely used in pharmacological experiments to inhibit PKA activity. They are often used at between 1 and 10 μM, either intracellularly through a whole-cell recording pipette, or applied in the bath in cell-permeant myristoylated forms. Here, we found that PKI (6–22) amide, at these concentrations, also inhibits CamK1, and facilitates the activity of multiple PKC isoforms, possibly acting as a positive allosteric modulator. Future directions will involve testing the effects of PKI (6–22) amide on kinase activity in a cell-based system. Importantly, for any users of PKI to perturb PKA functions, these additional targets of PKI need to be taken into account to design experiments and interpret results.
Despite the additional targets of PKI identified in our study, PKI seems more specific than the other commonly used PKA inhibitors, H89 and KT 5720 (Murray, 2008). This may be due to their different mechanisms of action. PKI binds to the catalytic subunit of PKA with high affinity by serving as a pseudosubstrate, whereas H89 and KT 5720 competitively inhibits ATP binding on the PKA catalytic subunit. The off-target effects of these reagents have partial overlap (Murray, 2008). For example, PKBα is inhibited by all three compounds, whereas PDK1 activity is facilitated by PKI (6–22) amide but inhibited by KT5720. Similarly, H89 inhibits ROCK2 potently but PKI has little effect on ROCK2. Since all three reagents are commonly used as pharmacological inhibitors of PKA, their non-selective actions need to be carefully considered to interpret experimental results.
We found that full length PKIα, one of the endogenously expressed isoforms, enhanced the activity of ROCK1 and multiple PKC isoforms at high concentrations. This raises the interesting possibility that PKI acts as a molecular switch to regulate the balance between PKA and PKC activity. However, the validity of this hypothesis depends on the endogenous concentration of PKIα, which is hard to determine, partially because the local concentrations of PKIα within a subcellular compartment may be higher than what can be determined with traditional biochemical methods. Our study therefore lays the foundation for future explorations on how endogenous PKI impacts cellular signaling and confines it in space and time through non-classical targets.
The original contributions presented in the study are included in the article/Supplementary Material. Further inquiries can be directed to the corresponding authors.
YC designed the experiments, implemented the experiments, analyzed the data, and wrote the manuscript. BS provided feedback on experimental design and data analysis, and edited the manuscript.
This work was supported by grants from the Brain & Behavior Research Foundation (NARSAD Young Investigator Grant 28323 to YC), a Goldenson Fund postdoctoral fellowship (to YC) and NINDS (R37NS046579, to BS).
The authors declare that the research was conducted in the absence of any commercial or financial relationships that could be construed as a potential conflict of interest.
We thank the team at ThermoFisher SelectScreen Kinase Profiling Services for helpful discussions and additional control experiments.
The Supplementary Material for this article can be found online at: https://www.frontiersin.org/articles/10.3389/fphar.2021.632815/full#supplementary-material.
Ashby, C. D., and Walsh, D. A. (1972). Characterization of the interaction of a protein inhibitor with adenosine 3′,5′-monophosphate-dependent protein kinases. I. Interaction with the catalytic subunit of the protein kinase. J. Biol. Chem. 247, 6637–6642. doi:10.1016/s0021-9258(19)44739-x
Ashby, C. D., and Walsh, D. A. (1973). Characterization of the interaction of a protein inhibitor with adenosine 3′,5′-monophosphate-dependent protein kinases. II. Mechanism of action with the holoenzyme. J. Biol. Chem. 248, 1255–1261. doi:10.1016/s0021-9258(19)44290-7
Brandon, E. P., Idzerda, R. L., and McKnight, G. S. (1997). PKA isoforms, neural pathways, and behaviour: making the connection. Curr. Opin. Neurobiol. 7, 397–403. doi:10.1016/s0959-4388(97)80069-4
Cembrowski, M. S., Wang, L., Sugino, K., Shields, B. C., and Spruston, N. (2016). Hipposeq: a comprehensive RNA-seq database of gene expression in hippocampal principal neurons. Elife 5, e14997. doi:10.7554/eLife.14997
Chen, Y., Granger, A., Tran, T., Saulnier, J. L., Kirkwood, A., and Sabatini, B. L. (2017). Endogenous Gαq-coupled neuromodulator receptors activate protein kinase A. Neuron 96, 1070–1083. doi:10.1016/j.neuron.2017.10.023
Chen, Y., and Sabatini, B. L. (2012). Signaling in dendritic spines and spine microdomains. Curr. Opin. Neurobiol. 22, 389–396. doi:10.1016/j.conb.2012.03.003
Cheng, H. C., Kemp, B. E., Pearson, R. B., Smith, A. J., Misconi, L., Van Patten, S. M., et al. (1986). A potent synthetic peptide inhibitor of the cAMP-dependent protein kinase. J. Biol. Chem., 261, 989.
Dalton, G. D., and Dewey, W. L. (2006). Protein kinase inhibitor peptide (PKI): a family of endogenous neuropeptides that modulate neuronal cAMP-dependent protein kinase function. Neuropeptides 40, 23–34. doi:10.1016/j.npep.2005.10.002
Day, R. N., Walder, J. A., and Maurer, R. A. (1989). A protein kinase inhibitor gene reduces both basal and multihormone-stimulated prolactin gene transcription. J. Biol. Chem. 264, 431–436.
De Lecea, L., Criado, J. R., Rivera, S., Wen, W., Soriano, E., Henriksen, S. J., et al. (1998). Endogenous protein kinase A inhibitor (PKIalpha) modulates synaptic activity. J. Neurosci. Res. 53, 269–278. doi:10.1002/(SICI)1097-4547(19980801)53:3<269::AID-JNR1>3.0.CO;2-8
Gill, G. N., Holdy, K. E., Walton, G. M., and Kanstein, C. B. (1976). Purification and characterization of 3′:5′ cyclic GMP dependent protein kinase. Proc. Natl. Acad. Sci. U.S.A., 73, 3918. doi:10.1073/pnas.73.11.3918
Gilman, A. G. (1995). Nobel Lecture. G proteins and regulation of adenylyl cyclase. Biosci. Rep. 15, 65–97. doi:10.1007/BF01200143
Glass, D. B., Cheng, H. C., Kemp, B. E., and Walsh, D. A. (1986). Differential and common recognition of the catalytic sites of the cGMP-dependent and cAMP-dependent protein kinases by inhibitory peptides derived from the heat-stable inhibitor protein. J. Biol. Chem., 261, 12166.
Glass, D. B., Cheng, H. C., Mende-Mueller, L., Reed, J., and Walsh, D. A. (1989a). Primary structural determinants essential for potent inhibition of cAMP-dependent protein kinase by inhibitory peptides corresponding to the active portion of the heat-stable inhibitor protein. J. Biol. Chem. 264, 8802–8810. doi:10.1016/s0021-9258(18)81864-6
Glass, D. B., Lundquist, L. J., Katz, B. M., and Walsh, D. A. (1989b). Protein kinase inhibitor-(6-22)-amide peptide analogs with standard and nonstandard amino acid substitutions for phenylalanine 10. Inhibition of cAMP-dependent protein kinase. J. Biol. Chem., 264, 14579.
Greengard, P. (2001). The neurobiology of slow synaptic transmission. Science 294, 1024–1030. doi:10.1126/science.294.5544.1024294/5544/1024
Grundmann, M., and Kostenis, E. (2017). Temporal bias: time-encoded dynamic GPCR signaling. Trends Pharmacol. Sci. 38, 1110–1124. doi:10.1016/j.tips.2017.09.004
Gu, Z., and Yakel, J. L. (2011). Timing-dependent septal cholinergic induction of dynamic hippocampal synaptic plasticity. Neuron 71, 155–165. doi:10.1016/j.neuron.2011.04.026
Jong, Y. J. I., Harmon, S. K., and O’Malley, K. L. (2018). Intracellular GPCRs play key roles in synaptic plasticity. ACS Chem. Neurosci. 9, 2162–2172. doi:10.1021/acschemneuro.7b00516
Krebs, E. G., Graves, D. J., and Fischer, E. H. (1959). Factors affecting the activity of muscle phosphorylase b kinase. J. Biol. Chem. 234, 2867–2873. doi:10.1016/s0021-9258(18)69685-1
Lobingier, B. T., and von Zastrow, M. (2019). When trafficking and signaling mix: how subcellular location shapes G protein-coupled receptor activation of heterotrimeric G proteins. Traffic 20, 130–136. doi:10.1111/tra.12634
Lur, G., and Higley, M. J. (2015). Glutamate receptor modulation is restricted to synaptic microdomains. Cell Rep. 12, 326–334. doi:10.1016/j.celrep.2015.06.029
Muñoz, W., and Rudy, B. (2014). Spatiotemporal specificity in cholinergic control of neocortical function. Curr. Opin. Neurobiol. 26, 149–160. doi:10.1016/j.conb.2014.02.015
Murray, A. J. (2008). Pharmacological PKA inhibition: all may not be what it seems. Sci. Signal. 1, re4. doi:10.1126/scisignal.122re4
Rodems, S. M., Hamman, B. D., Lin, C., Zhao, J., Shah, S., Heidary, D., et al. (2002). A FRET-based assay platform for ultra-high density drug screening of protein kinases and phosphatases. Assay Drug Dev. Technol., 1, 9. doi:10.1089/154065802761001266
Saunders, A., Macosko, E. Z., Wysoker, A., Goldman, M., Krienen, F. M., de Rivera, H., et al. (2018). Molecular diversity and specializations among the cells of the adult mouse brain. Cell 174, 1015–1030. doi:10.1016/j.cell.2018.07.028
Smith, F. D., Langeberg, L. K., and Scott, J. D. (2006). The where’s and when’s of kinase anchoring. Trends Biochem. Sci. 31, 316–323. doi:10.1016/j.tibs.2006.04.009
Smith, M. K., Colbran, R. J., and Soderling, T. R. (1990). Specificities of autoinhibitory domain peptides for four protein kinases. Implications for intact cell studies of protein kinase function. J. Biol. Chem., 265, 1837.
Sutherland, E. W., Robison, G. A., and Butcher, R. W. (1968). Some aspects of the biological role of adenosine 3′,5′-monophosphate (cyclic AMP). Circulation 37, 279–306. doi:10.1161/01.cir.37.2.279
Taylor, S. S., Buechler, J. A., and Yonemoto, W. (1990). cAMP-dependent protein kinase: framework for a diverse family of regulatory enzymes. Annu. Rev. Biochem. 59, 971–1005. doi:10.1146/annurev.bi.59.070190.004543
Thomsen, A. R. B., Jensen, D. D., Hicks, G. A., and Bunnett, N. W. (2018). Therapeutic targeting of endosomal G-protein-coupled receptors. Trends Pharmacol. Sci. 39, 879–891. doi:10.1016/j.tips.2018.08.003
Walsh, D. A., Ashby, C. D., Gonzalez, C., Calkins, D., and Fischer, E. H. (1971). Krebs EG: purification and characterization of a protein inhibitor of adenosine 3′,5′-monophosphate-dependent protein kinases. J. Biol. Chem. 246, 1977. doi:10.1016/s0021-9258(19)77177-4
Weinberg, Z. Y., Crilly, S. E., and Puthenveedu, M. A. (2019). Spatial encoding of GPCR signaling in the nervous system. Curr. Opin. Cell Biol. 57, 83–89. doi:10.1016/j.ceb.2018.12.006
Keywords: protein kinase A, protein kinase C, protein kinase inhibitor peptide, kinase screen, specificity, endogenous, inhibition, facilitation
Citation: Chen Y and Sabatini BL (2021) The Kinase Specificity of Protein Kinase Inhibitor Peptide. Front. Pharmacol. 12:632815. doi: 10.3389/fphar.2021.632815
Received: 24 November 2020; Accepted: 04 January 2021;
Published: 29 January 2021.
Edited by:
Roger J. Summers, Monash University, AustraliaReviewed by:
Michelle L. Halls, Monash University, AustraliaCopyright © 2021 Chen and Sabatini. This is an open-access article distributed under the terms of the Creative Commons Attribution License (CC BY). The use, distribution or reproduction in other forums is permitted, provided the original author(s) and the copyright owner(s) are credited and that the original publication in this journal is cited, in accordance with accepted academic practice. No use, distribution or reproduction is permitted which does not comply with these terms.
*Correspondence: Yao Chen, eWFvY2hlbkB3dXN0bC5lZHU=; Bernardo L. Sabatini, YmVybmFyZG9fc2FiYXRpbmlAaG1zLmhhcnZhcmQuZWR1
Disclaimer: All claims expressed in this article are solely those of the authors and do not necessarily represent those of their affiliated organizations, or those of the publisher, the editors and the reviewers. Any product that may be evaluated in this article or claim that may be made by its manufacturer is not guaranteed or endorsed by the publisher.
Research integrity at Frontiers
Learn more about the work of our research integrity team to safeguard the quality of each article we publish.