- 1Department of Anatomy and Neurobiology, University of Tennessee Health Science Center, Memphis, TN, United States
- 2Department of Biological Sciences, University of Toledo, Toledo, OH, United States
- 3Program of Neuroscience, University of Maryland School of Medicine, Baltimore, MD, United States
- 4Department of Physiological Sciences, University of Florida, Gainesville, FL, United States
- 5Neuroscience Institute, University of Tennessee Health Science Center, Memphis, TN, United States
Transient receptor potential melastatin 3 channel (TRPM3) is a calcium-permeable nonselective cation channel that plays an important role in modulating glucose homeostasis in the pancreatic beta cells. However, how TRPM3 is regulated under physiological and pathological conditions is poorly understood. In this study, we found that both intracellular and extracellular protons block TRPM3 through its binding sites in the pore region. We demonstrated that external protons block TRPM3 with an inhibitory pH50 of 5.5. whereas internal protons inhibit TRPM3 with an inhibitory pH50 of 6.9. We identified three titratable residues, D1059, D1062, and D1073, at the vestibule of the channel pore that contributes to pH sensitivity. The mutation of D1073Q reduced TRPM3 current by low external pH 5.5 from 62 ± 3% in wildtype to 25 ± 6.0% in D1073Q mutant. These results indicate that D1073 is essential for pH sensitivity. In addition, we found that a single mutation of D1059 or D1062 enhanced pH sensitivity. In summary, our findings identify molecular determinants respionsible for the pH regulation of TRPM3. The inhibition of TRPM3 by protons may indicate an endogenous mechanism governing TRPM3 gating and its physiological/pathological functions.
Introduction
Transient receptor potential channels (TRP channels) are membrane proteins that allow organisms to readily sense the environment (Clapham, 2003). They detect stimuli including temperature (Brauchi and Orio, 2011; Vandewauw et al., 2018), voltage (Montell et al., 1985; Clapham, 2003; Brauchi and Orio, 2011), mechanical force (osmolarity (Strotmann et al., 2000; Liedtke and Friedman, 2003; Quallo et al., 2015), pressure (Suzuki et al., 2003), stretch (Strotmann et al., 2000; Maroto et al., 2005; Hardie and Franze, 2012), gravity (Sun et al., 2009)), light (Minke, 1977; Montell et al., 1985; Hardie, 2014), proton concentrations (Chandrashekar et al., 2006; Gerdes et al., 2007; Semtner et al., 2007), and various chemical signals (Caterina et al., 1997; McKemy et al., 2002; Everaerts et al., 2011). TRP channels form homomeric or heteromeric cation channels that can be selective or non-selective to cations; most TRP members are permeable to Ca2+ (Pan et al., 2011). Upon activation, TRP channels change membrane potential or intracellular calcium concentration ([Ca2+]i) to promote downstream signal transductions. Depending on sequence homology and channel architecture, TRP channels are divided into seven subfamilies, namely, TRPA (Ankyrin), TRPC (Canonical), TRPM (Melastatin), TRPML (Mucolipin), TRPP (Polycystin), TRPV (Vanilloid), and TRPN (NompC, no vertebrate member). 27 vertebrate members of these subfamilies are expressed in humans (Venkatachalam and Montell, 2007; Uchida et al., 2019).
TRPM3 belongs to the subfamily of TRPM (Melastatin). TRPM3 is a non-selective cation channel that is permeable to Ca2+, Na+, Mn2+, and Mg2+ ions with a permeability ratio of PCa/PNa of 1.57 ± 0.31 (Grimm et al., 2003). RT-qPCR analyses have shown expression of human (hTRPM3), mouse (mTRPM3), and rat (rTRPM3) TRPM3 in a variety of tissues, with the most abundant expression in the brain, kidney, pituitary gland, and adipose tissues (Zamudio-Bulcock et al., 2011; Oberwinkler and Philipp, 2014). In sensory neurons, TRPM3 functions as a noxious heat sensor. For example, TRPM3 deficient mice lack the normal response to noxious heat and do not develop inflammatory heat hyperalgesia (Vriens et al., 2011; Vandewauw et al., 2018). Vangeel et al., also recently demonstrated functional expression of TRPM3 in a large subset of nociceptor neurons in humans, Thus, TRPM3 has been suggested as a potential drug target for novel analgesics (Moran and Szallasi, 2018; Vangeel et al., 2020). Although the role of TRPM3 in the central nervous system has not been explored in detail yet, a recent study showed high-level expression of TRPM3 in mouse CA2 and CA3 hippocampal neurons and in the dentate gyrus. Field potential recordings suggested that TRPM3 agonists inhibit synaptic transmission, and reduce long-term potentiation (LTP) in the mouse hippocampus (Held et al., 2020). In humans, genetic analyses have linked TRPM3 mutations with intellectual disability, epilepsy, inherited cataracts, and glaucoma (Bennett et al., 2014; Dyment et al., 2019). In the cardiovascular system, TRPM3 localizes in the perivascular nerves of mouse mesenteric arteries and induces vasodilation by stimulating CGRP (calcitonin gene-related peptide) receptors (Alonso-Carbajo et al., 2019). TRPM3 forms homomultimeric channels, which are constitutively active (Grimm et al., 2003). However, TRPM3 can also be activated by the endogenous neurosteroid pregnenolone sulfate (PS), as well as nifedipineand clotrimazole. Mefenamic acid, diclofenac, progesterone, and flavanones have been reported to inhibit TRPM3 (Wagner et al., 2008; Uchida et al., 2019). PS has been shown to increase neurotransmitter release, strengthen synaptic transmission, and modulate synaptic plasticity (Smith et al., 2014). However, whether TRPM3 contributes to any of these neuronal functions of PS or not, is not clear (Zamudio-Bulcock et al., 2011). One study has shown that the PS-induced potentiation of spontaneous glutamate release in Purkinje neurons of developing rats is mediated by TRPM3 (Zamudio-Bulcock et al., 2011). In non-neuronal cells, PS upregulates activator protein 1 (AP-1) and early growth response protein 1 (Egr-1) transcriptional activity, which can be blocked by TRPM3 antagonists (Lesch et al., 2014). TRPM3 also upregulates c-Jun and c-Fos promoter activity and stimulates CRE-controlled reporter gene transcription in insulinoma and pancreatic β-cells, in a TRPM3-dependent manner (Muller et al., 2011). In vascular smooth muscle cells, PS increases [Ca2+]i and modulates contractile responses, which can be inhibited by TRPM3 inhibitors (Naylor et al., 2010). PS also increases [Ca2+]i in fibroblast-like synoviocytes and suppresses the secretion of hyaluronan via TRPM3 (Ciurtin et al., 2010).
Extracellular and intracellular protons modulate ion channel activity. Indeed, intracellular acidosis activates inward rectifier K+ channel family protein Kir6.1, K2p channel TREK-1, and TREK-2. However, the activity of Kir1.1, Kir4.1, and Kir5.1 is inhibited by both intracellular and extracellular protons. Gap junction channels, connexins, are also inhibited by intracellular acidosis. In addition, both intracellular and extracellular acidification block the K2p channel TRESK, as well as depress or inhibit TWIK-1 and TWIK-2. Some of the ion channels, such as, TASK-2, TRESK, TALK-1, and TALK-2, which are inhibited at low pH can be activated by alkalization. Protons cannot activate or inhibit P2X2 and P2X5 homomultimers but decrease the potency and efficacy of ATP gating of P2X5 and sensitize P2X2 receptors to ATP. TRP channels are no exception regarding variable activity in response to pH. Specifically, TRPV1, TRPV4, and TRPC4 are activated by a reduction of pH. In contrast, TRPC5 currents are increased by an acidic pH until 6.0, at which point further decreases in pH reduce current (Holzer, 2009). PKD2L1 (TRPP2) expressing neurons show action potentials in response to citric acid (Huang et al., 2006), whereas intracellular and extracellular pH inhibits TRPM2 (Du et al., 2009; Starkus et al., 2010; Yang et al., 2010). Yet very little is known about how pH regulates TRPM3. In the current study, we examined how TRPM3 activation by PS is modulated by different extracellular and intracellular pH conditions. In all experiments, we activated TRPM3 by external application of PS. Thus, all subsequent mentions of TRPM3 activity in this manuscript must be considered as TRPM3 activity in response to PS, and not TRPM3 constitutive activity. As PS-induced TRPM3 currents are almost two orders of magnitude higher than TRPM3 constitutive currents (Grimm et al., 2003; Lee et al., 2003; Wagner et al., 2008), we concluded that for our experiments, it is reasonable to exclude the effects of constitutive TRPM3 activity.
Materials and Methods
Plasmid and Molecular Biology
The cDNA of the human TRPM3 channel (accession number AJ505026) with C-terminal GFP tag was provided by Christian Harteneck (University of Tübingen, Tübingen, Germany) (Grimm et al., 2003). Alternative splicing patterns of TRPM3 is highly conserved across human and rodents (Oberwinkler et al., 2005). To date, 25 isoforms of mTRPM3 protein have been identified, including a recently discovered variant - TRPM3γ3. Splicing events affect exons 8, 13, 15, 17, 20, 24, and 28 of TRPM3. α variants lack exon 2; β variants lack exon 1; and γ variants lack a large part of exon 28 (Oberwinkler and Philipp, 2014; Uchida et al., 2019). Our hTRPM3 cDNA contains all 30 exons, where 389 amino acids in exon 28 have been replaced with the alternative carboxy terminus of seven residues.This truncation does not affect functional activity of the ion channel (Grimm et al., 2003; Oberwinkler and Philipp, 2014). Mutations of hTRPM3-GFP were generated by site-directed mutagenesis (performed by GENEWIZ Inc.). The predicted mutations were verified by sequencing analysis.
Cell Culture and Overexpression of hTransient Receptor Potential Melastatin 3 Channel-GFP and the Mutants in HEK-293 Cells
Human embryonic kidney (HEK)-293 cells were used to transiently overexpress wild-type hTRPM3-GFP and its mutants. The cells were grown in DMEM/F12 medium (Fisher Scientific, Waltham, MA, United States. Catalog no. MT10090CV) supplemented with 10% bovine growth serum (HyClone, Logan, UT, United States. Catalog no. SH30541.03), 100 U/ml penicillin/100 mg/ml streptomycin (Fisher Scientific, catalog no. SV30010) at 37°C in a 5% CO2, humidity-controlled incubator. Lipofectamine 2000 (Thermo Fisher Scientific, Waltham, MA, United States. Catalog no. 18324012) was used for the transfection of TRPM3 into the cells in a 35 mm culture dish according to the manufacturer’s instructions. Successfully transfected cells were identified by their fused GFP when illuminated at 480 nm excitation wavelength. Electrophysiological recordings were conducted between 36- and 48-h post-transfection.
Electrophysiology
All patch-clamp experiments were performed at room temperature (20–22°C). TRPM3 whole-cell currents were recorded using an Axopatch 200B amplifier. Data were digitized at 10 kHz and digitally filtered offline at 5 kHz. Patch electrodes were pulled by Sutter P-97 micropipette puller and fire-polished to the resistance of 3–5 MΩ when filled with internal solutions. Series resistance (Rs) was compensated up to 90% to reduce series resistance errors to <5 mV. Cells in which Rs was >8 MΩ were discarded (Du et al., 2009).
For whole-cell current recording, ramp voltage stimuli (250 ms duration) were delivered at 1 s intervals and ranging from −100 to +100 mV. The internal pipette solution for whole-cell current recordings contained (in mM): 115 Cs-methanesulfonate (CsSO3CH3), 8 NaCl, 10 Cs-EGTA, 5 Na2-ATP and 10 HEPES, with pH adjusted to 7.2 with CsOH. In high intracellular Ca2+ experiments, 0.93 mM CaCl2 was added to the above-mentioned intracellular solution and EGTA was reduced to 1 mM, resulting in 1 µM free intracellular Ca2+. MaxChelator (https://somapp.ucdmc.ucdavis.edu/pharmacology/bers/maxchelator/downloads.htm) software from the University of California, Davis was used to calculate free [Ca2+]i. To avoid proton activated Cl− currents conducted by endogenous anion channels of HEK-293 cells (Lambert and Oberwinkler, 2005), NaCl in standard Tyrode solution was replaced with Na-glutamate for all whole-cell current recordings. This external solution contained (in mM): 145 Na-glutamate, 5 KCl, 2 CaCl2, 1 MgCl2, 10 HEPES, and 10 glucose, with pH adjusted to 7.4 with glutamic acid. Internal and external acidic pH solutions were prepared as described previously with slight modifications (Du et al., 2009). In brief, 10 mM HEPES was replaced by 10 mM MES for the solutions at pH ≤ 6.0. Bath solutions containing 1 mM–60 mM NH4Cl were prepared by decreasing Na+ concentrations to 85 mM to keep the osmolarity constant Osmolarity was adjusted to 300 ± 10 mOsm with mannitol. In experiments designed to test protons permeability of TRPM3, pipette solutions contained (in mM): 120 NMDG, 108 glutamic acid, 10 HEPES, 10 EGTA, with pH adjusted to 7.2 with NMDG. External solutions for testing proton permeability contained (in mM): 145 NMDG, 10 HEPES, and 10 Glucose; and pH was adjusted with glutamic acid. To prepare the pH 5.5 external solution for proton permeability, 10 mM HEPES was replaced with 10 mM MES. PS was dissolved in DMSO to prepare 100 mM stock solution, and an adequate volume of stock PS solution was added to the external solution to achieve the required concentration. All the chemicals used in electrophysiological experiments were from Sigma-Aldrich, St. Louis, MO, United States.
Data Analysis
Statistical data were analyzed using GraphPad Prism 8. Pooled data are presented as mean ± SEM. Concentration-response curves were fitted by an equation of the form: E = Emax{1/[1+(EC50/C)n]} where E is the effect at concentration C, Emax is the maximal effect, IC50 is the concentration for half-maximal effect, and n is the Hill coefficient (Du et al., 2009). The concentration of proton required for half-maximal inhibition is denoted by IC50 (when proton concentration is expressed as molar concentration) and pH50 (when proton concentration is expressed by pH value). Statistical comparison of two groups was performed by unpaired Student’s t-test, with p < 0.05 considered statistically significant. Statistical comparison of three or more groups was performed by one-way ANOVA with Tukey’s post hoc multiple comparisons.
Results
Extracellular and Intracellular Acidic pH Inhibits Transient Receptor Potential Melastatin 3 Channel
We studied the effects of low pH on TRPM3 by overexpressing hTRPM3-GFP in HEK-293 cells and recording whole-cell currents in response to PS. We found that low extracellular pH inhibited TRPM3 in a reversible manner (Figures 1A–C). To avoid proton activated endogenous anion channel conducted Cl− currents, external Cl− was replaced by glutamate (See Methods). The inhibitory effect of low extracellular pH (pHo) was only observed below pH 6.0. Specifically, at pHo 7.0 and 6.0, recorded TRPM3 currents were equivalent to pHo 7.4 (p > 0.05 in both groups). At a pHo below 6.0, acidic conditions exhibited significant inhibition of TRPM3. pHo 5.5, caused ∼60% reduction in TRPM3 whole-cell current induced by PS (Figure 1). Although the concentration-dependent effect of extracellular pH on TRPM3 activity was weak, fitting these data in a non-linear regression curve resulted in a concentration-dependent relationship with a pH50 value of 5.5 ± 0.13, and, Hill coefficient of nH = 2.5 (Figure 1D). When PS was applied continuously while reducing extracellular pH (Figures 1E–G), a concentration-dependent relationship was observed with a pH50 value of 5.5 ± 0.17, and a Hill coefficient of nH = 2.6 (Figure 1H). To further measure the inhibitory effect of low extracellular pH on TRPM3, we held the membrane potential at +60 mV continuesly during the recordings. We found that low pH significantly blocked PS-induced TRPM3 currents (Supplementary Figure S1). Interstingly, as shown in Figure 1A, application of low extracellular pH (pH ≤ 5.5) with PS produced an initial activation of TRPM3 before blocking it. This suggested that the onset of low pHo inhibition is slower than the PS activation. Because acidic pH has been shown to block TRPM channels intracellularly (Du et al., 2009; Starkus et al., 2010; Yang et al., 2010), we next asked if intracellular low pH could inhibit TRPM3. To answer this question, we first investigated the effects of intracellular low pH (pHi) on TRPM3. Whole-cell TRPM3 currents were recorded using the low-pH pipette solutions (see experimental procedures) while keeping extracellular pH constant at 7.4 (Figure 2A). To investigate the extent of modulation of TRPM3 by protons, we also introduced higher pHi than the physiological pHi of 7.2. we found that the TRPM3 current plateaued at about pHi 7.6. Acidic pH had similar inhibitory effects on both the outward and inward TRPM3 currents (Figure 2A). There was no significant difference in the steady-state inhibition between inward and outward currents, suggesting that there were no voltage-dependent effects of acidic pHi on inward and outward TRPM3 currents (Figure 2B). Low pHi markedly reduced TRPM3 current in a concentration-dependent manner with a pH50 value of 6.90 ± 0.11 (outward current at +100 mV) (Figure 2C) and pH50 value of 6.90 ± 0.15 (inward current at −100 mV) (Figure 2D). The Hill coefficient was nH = 1.6. Combined, our findings suggesting that extracellular acidic pH 6.0 did not affect TRPM3 current but low intracellular low pH had an inhibitory pH50 value of 6.9. Thus, these data suggest that TRPM3 sensitivity to pH was stronger on the cytoplasmic side.
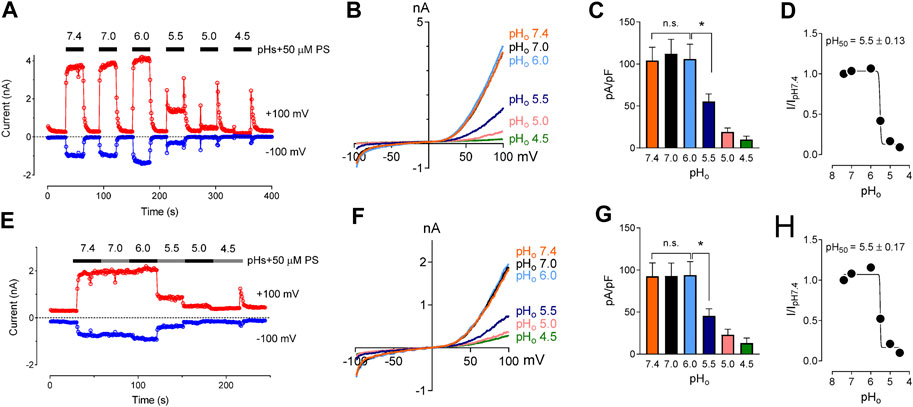
FIGURE 1. Inhibitory effect of extracellular acidic pH on TRPM3 activation by PS. (A) Time course of TRPM3 currents elicited by voltage ramps ranging −100 to +100 mV. Both inward and outward currents were completely and reversibly inhibited by pHo 4.5 50 µM PS was applied extracellularly and was washed with PS-free extracellular solution between subsequent PS application. Inward and outward currents were measured at −100 and +100 mV, respectively. (B) Representative recording of TRPM3 current in (A) by ramp protocols ranging from −100 to +100 mV at the indicated pHo. (C) Mean current amplitude of TRPM3 at the indicated pHo in (A) (mean ± SEM; n = 11, * indicates p < 0.05 by unpaired Student’s t-test; “n.s.” indicates not statistically significant). (D) pHo concentration-dependence of TRPM3 activation by PS. TRPM3 currents exerted at +100 mV were utilized for the plot. The current amplitude at the indicated pHo normalized to the current amplitude at pHo 7.4 in (A). Background electrical activity before application of PS is subtracted in all quantitative analysis. (E) Time course of TRPM3 currents elicited by voltage ramps ranging −100 to +100 mV. PS was applied continuously while reducing extracellular pH without allowing any washing period between subsequent extracellular solution applications. (F) Representative recording of TRPM3 current in (E) by ramp protocols ranging from −100 to +100 mV at the indicated pHo. (G) Mean current amplitude of TRPM3 at the indicated pHo in (E) (mean ± SEM; n = 7, * indicates p < 0.05 by unpaired Student’s t-test; “n.s.” indicates not statistically significant). (H) pHo concentration-dependence of TRPM3 activation by PS. TRPM3 currents exerted at +100 mV were utilized for the plot. The current amplitude at the indicated pHo normalized to the current amplitude at pHo 7.4 in (E).
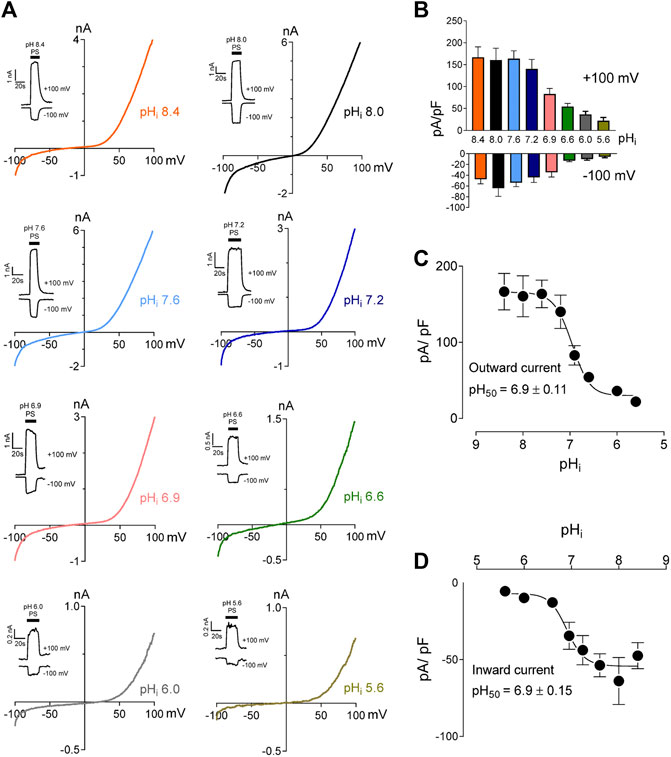
FIGURE 2. Intracellular acidification blocks TRPM3 activation by PS in a concentration-dependent manner. (A) Representative recordings and time courses (insert) of TRPM3 current by ramp protocols ranging from −100 to +100 mV at the indicated pHi. PS was applied through the extracellular solution (pHo 7.4) and different cells were exposed to different pHi while keeping that pHi constant. (B) Mean current amplitude of TRPM3 at the indicated pHi (mean ± SEM; n = 8–14). (C,D) pHi concentration-dependence of TRPM3 activation by PS. c and d show outward and inward current, respectively, elicited by TRPM3 after extracellular PS application, by voltage ramp ranging −100 to +100 mV. TRPM3 currents exerted at −100 and +100 mV were considered as inward and outward currents, respectively, and were utilized for these plots. All currents were normalized to the corresponding capacitance of the cell overexpressing hTRPM3-GFP. Each data point is the mean of 8–14 cells with the error bar showing SEM, at the indicated pHi. Inhibitory pH50 values were measured separately for outward (pH50 = 6.9 ± 0.11) and inward (pH50 = 6.9 ± 0.15) currents.
Effect of Low pHi on Concentration-Dependence of Transient Receptor Potential Melastatin 3 Channel Activation by Pregnenolone Sulfate
PS binds directly to the extracellular side of the TRPM3 channel to activate it. TRPM3 channel stays both functional and unaffected by the presence of intracellular PS (Wagner et al., 2008). Previous studies have shown that PS activates TRPM3 in a concentration-dependent manner with an EC50 value of 12 and 23 µM for outward and inward current respectively (Wagner et al., 2008). To investigate the effect of protons on PS concentration-dependent activation of TRPM3, we perfused the cells with a wide range of PS concentrations (1–500 µM). pHi was held constant at 7.2 or 6.0. TRPM3 whole-cell outward and inward currents showed very similar PS concentration-dependence in both pHi conditions (Figures 3A,B). The EC50 values for the outward currents were 16 µM (pHi 7.2) and 15 µM (pHi 6.0) (Figure 3C). This result suggested that higher proton concentrations inside the cell decreased TRPM3 maximal activation at any given PS concentration. However, protons did not affect the PS concentration-dependent activation of TRPM3 as EC50 values for the inward currents were 21 µM (pHi 7.2) and 26 µM (pHi 6.0) (Figure 3D). This result indicated that the PS concentration-dependent curve of TRPM3 inward currents did not show any change in response to low pHi. In summary, these data suggested that intracellular protons do not compete with PS for binding sites.
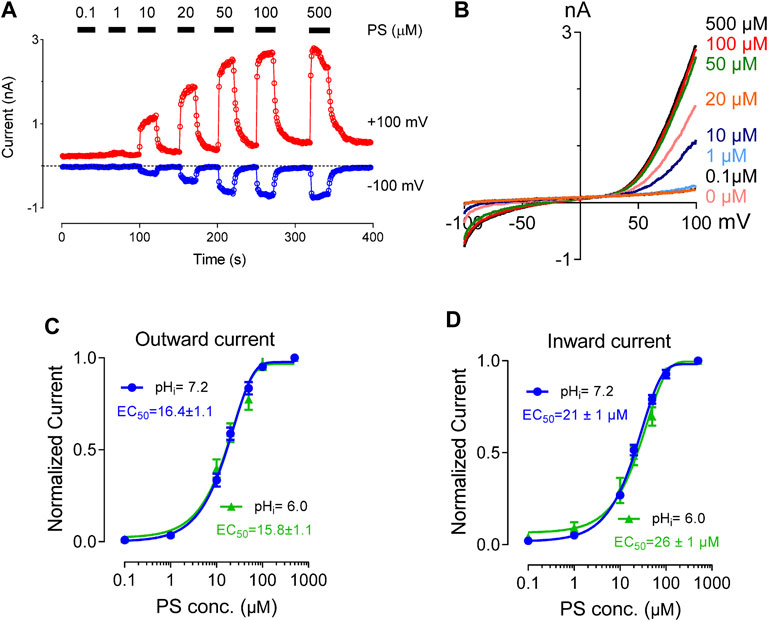
FIGURE 3. Concentration-response curve for PS-induced currents in hTRPM3, at the indicated intracellular pH. (A) Time course of TRPM3 currents elicited by voltage ramps ranging −100 to +100 mV. PS was applied extracellularly in increasing concentration sequence at concentrations of 0, 0.1, 1, 10, 20, 50, 100, and 500 µM, with an adequate washing period between subsequent PS applications. (B) Representative recording of TRPM3 current at the indicated PS concentration in (A). (C) Outward current normalized to maximum concentration-response (500 µM) of the PS, with error bars showing SEM (n = 7 cells). The EC50 values of PS for the outward currents are 16.4 ± 1.1 µM at pH 7.2 and 15.8 ± 1.1 µM at pH 6.0. (D). Inward current normalized to maximum concentration-response (500 µM) of the PS, with error bars showing SEM (n = 7 cells). The EC50 values of PS for the inward currents are 21.0 ± 1.0 µM at pH 7.2 and 26.0 ± 1.0 µM at pH 6.0.
Transient Receptor Potential Melastatin 3 Channel Inhibition by Protons can be Reversed by Increasing pHi
Extracellular application of NH4Cl produces a rise in pHi resulting from an influx of NH3. NH3 binds intracellular protons and causes alkalization inside the cells (Jacobs, 1922; Warburg, 1922). We observed that protons blocked TRPM3 from the cytoplasmic side. Thus, we tested whether raising pHi by applying NH4Cl could rescue the TRPM3 currents. We perfused the cells with solutions where part of NaCl was replaced by an equal amount of NH4Cl (see experimental procedures), to test whether increasing pHi while keeping extracellular pH the same can reverse the blocking effects of protons on TRPM3. As shown in Figure 4A,B, 30 and 60 mM NH4Cl potentiated the PS-induced TRPM3 currents significantly, while potentiation was only slightly different on the outward and inward currents (Figure 4C) We attribute this increase to the influx of NH3 into the cell and removing bound protons from the TRPM3 cytoplasmic side. Overall, this result supported our finding that TRPM3 could be blocked by an acidic intracellularpH Indeed, different concentrations of NH4Cl were applied to the same cell, and all NH4Cl applications changed intracellular pH. To minimize the residual effects of NH3, we allowed adequate washing time between two consecutive NH4Cl + PS applications (Figure 4D–F).
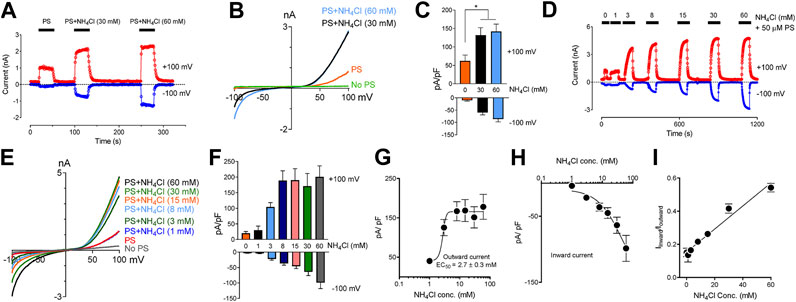
FIGURE 4. Inhibitory effect of low intracellular pH on TRPM3 can be reversed by perfusing cells with extracellular solution containing NH4Cl. (A) Time course showing outward and inward current, recorded at +100 and −100 mV respectively, obtained from HEK cells overexpressing TRPM3, under voltage ramp protocol ranging −100 to +100 mV, (pHi = 6.0, n = 11 cells). Indicated concentrations of NH4Cl was applied extracellularly along with PS. To achieve similar osmolarity, all extracellular Na+ concentration was lowered to 85 mM and osmolarities were adjusted to 300 ± 10 mOsm by mannitol. Adequate washing time (1–3 min) was provided after each application of NH4Cl, to bring the current down to the basal level, which is 6.0 for all the cells recorded. Representative current was plotted vs. time in the presence of extracellular NH4Cl and PS, at the indicated concentrations. To achieve similar osmolarity, all extracellular buffer Na+ concentration was lowered to 85 mM and osmolarities were adjusted to 300 ± 10 mOsm by mannitol. (B) Representative recording of TRPM3 current by ramp protocols ranging from −100 mV to +100 mV at the indicated NH4Cl concentrations. (C) Mean outward and inward TRPM3 current at the indicated NH4Cl concentrations (mean ± SEM, n = 11 cells). * indicates p < 0.05 by unpaired Student’s t-test. (D) Representative recording of TRPM3 current by the dose-dependent effects of NH4Cl. The applications of extracellular NH4Cl at the indicated concentrations. (E) Representative recording of TRPM3 current by ramp protocols ranging from −100 mV to +100 mV at the indicated NH4Cl concentration. (From panel d) (F) Mean outward and inward TRPM3 current at the indicated NH4Cl concentration. (From replicated experiment of (D)) (mean ± SEM, n = 11 cells). (G,H) Concentration-dependence curves of effects of NH4Cl on TRPM3 activations, outward (G) and inward (H) currents, respectively. All currents were normalized to the corresponding capacitance of the cell overexpressing hTRPM3 (mean ± SEM, n = 11 cells) at the indicated NH4Cl concentration. EC50 values were measured separately for outward (NH4Cl50 = 2.7 ± 0.3 µM) currents, whereas the inward currents continue to increase after applying 60 mM NH4Cl. (I) Mean ratio of inward current to outward current plotted against NH4Cl concentrations (mean ± SEM, n = 11 cells).
Subsequently, we tested whether external NH4Cl exhibited a concentration-dependent rescuing effect on TRPM3 activity or not. We perfused the cells with modified Tyrode solutions containing different concentrations of NH4Cl (1–60 mM) (Figure 4D–F). Na+ concentrations in all solutions were kept the same, and osmolarities were adjusted to 300 ± 10 mOSM with mannitol. We observed a five-fold increase in TRPM3 activity at only 3 mM (NH4Cl) concentration (Figure 4D). Even 1 mM NH4Cl rescued some TRPM3 activity (p < 0.05) (Figure 4D). Although, when compared with 3 mM NH4Cl, some of the higher concentrations showed a significant increase in TRPM3 activity, there was no substantial increase in TRPM3 activity by further increasing the NH4Cl concentration. The PS concentration-dependent manner with a EC50 value of 2.7 ± 0.3 µM, the Hill coefficient is nH = 1.1 for the outward currents (Figure 1G) and inward currents (Figure 1H). We also observed a higher increase in inward current than corresponding outward current (Figure 4I), with the ratio of inward current to the outward current increasing from 0.13 at 1 mM NH4Cl to 0.54 at 60 mM NH4Cl. When plotting the correlation between the outward and inward currents, we observed a linear realationship between the outward and inward currents (Figure 1I). It is important to note that because we did not measure the exact pHi changes following external NH4Cl applications, the pHi differences before and after NH4Cl applications, are unknown. However, despite this limitation, these experiments support the claim that TRPM3 activity is highly sensitive to changes in intracellular pH changes in the physiological pHi range (pHi 6.0–8.0). ITo ensure that application of NH4Cl and PS for an extended period of time did not affect TRPM3 activity and thus confound our results, we also examined individual cells following only one exposure to different concentrations of NH4Cl. The results of these experiments are summarized in Supplementary Figure S2A. Perfusion of individual cells with different concentrations of NH4Cl (Supplementary Figure S2B) showed similar response when compared to TRPM3 currents from our earlier experiments (Figure 4D). In summary, these data suggest that the increase of intracellular pH boosts TRPM3 activities.
Transient Receptor Potential Melastatin 3 Channel is Potentially Permeable to Protons
The lipid bilayer (eg., cell membrane) presents a strong barrier for the transport of charged ions through eukaryotic cell membranes. Although the permeability of protons is higher than other monovalent cations (Tepper and Voth, 2005), it is unlikely that these mechanisms can transport sufficient protons across the membrane to have a direct impact unless protons are passing through the overexpressed ion-channel itself. We thus examined whether protons can permeate through TRPM3. To test this, we recorded TRPM3 inward current in HEK-293 cells, overexpressing hTRPM3-GFP, by holding the membrane at −100 mV and applying PS and low pH solutions externally. We maintained pHi at 7.6 to provide a higher concentration gradient for protons. All cations except protons were removed by using NMDG in the external and internal solutions (Figure 5A). PS, along with NMDG pH 5.5 extracellular solution, produced a small transient inward current in TRPM3 transfected HEK-293 cells (Figure 5B). Indeed, the amplitude of this current was significantly lower than the TRPM3 currents observed using theTyrode solution (Figures 5C,D). This result was likely due to removal of all cations besides a limited amount of protons, with the extracellular and intracellular proton concentrations calculated to be 3.2 × 10–3 mM (pH 5.5) and 2.5 ×10–5 mM (pH 7.6), respectively. These cation concentrations are several orders of magnitude lower than the total cation concentrations of the Tyrode solution. However, since there were no other cations in our solution and although low in amplitude, the current we observed suggests the passage of proton through the TRPM3 channel. We spectulate that the transient nature of the current is because the intracellular protons inhibit TRPM3 after their permeation. Mock transfected cells did not produce any inward current in response to PS (Data not shown). This evidence indicates that TRPM3 is potentially permeable to protons.
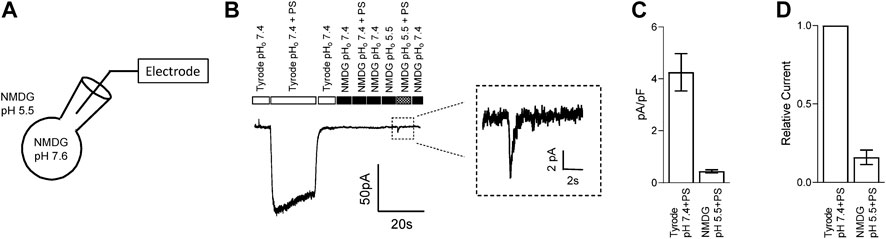
FIGURE 5. TRPM3 is potentially permeable to protons. (A) Schematic showing the protons permeation recording condition, where all intracellular and extracellular ions were replaced by NMDG and glutamic acid, respectively, except for protons. 50 µM PS was applied while holding hTRPM3-GFP transfected HEK cells at −100 mV. (B) Inward currents were elicited by the PS application. No PS activated current under the NMDG solution at pHo 7.40, while lowering down the pHo to 5.50 generated a small and transient inward current. Insert panel shows the inward current recorded during NMDG-PS (pH 5.5) application. (C) Mean current amplitude in response to PS at the indicated conditions (mean ± SEM, n = 8 cells). (D) Relative current amplitude in response to PS, comparing with Tyrode (pHo 7.4) solution response of the same cell (mean ± SEM, n = 8 cells).
The Inhibition of Intracellular Ca2+ on Transient Receptor Potential Melastatin 3 Channel is pH-Independent
TRPM3 displays higher permeability for divalent cations than monovalent cations. For the splice variant TRPM3α2, 24% of total TRPM3 current is expected to result from Ca2+ (Przibilla et al., 2018), suggesting a large increase in [Ca2+]i following TRPM3 activation. Multiple studies verified this effect showing an increase in [Ca2+]i in a micromolar range following TRPM3 activation (Vriens et al., 2011; Straub et al., 2013; Przibilla et al., 2018). A recent study demonstrated that an increase in [Ca2+]i, independent of TRPM3 activity, inhibits TRPM3 in a calmodulin-dependent manner (Przibilla et al., 2018). This suggests a potential negative feedback mechanism that regulates a high increase in [Ca2+]i resulting from TRPM3 activation. Studies conducted by others enlist [Ca2+]i as a regulator of TRPM3 activity. In our study, we found that intracellular protons block TRPM3. Hence, we asked the question, how do these two regulatory mechanisms interact with each other? To find the effect of [Ca2+]i on the concentration-dependent inhibition of TRPM3 by protons, we tested TRPM3 activity in two different [Ca2+]i conditions, while providing a wide range of pHi. In addition to the inhibition of TRPM3 current by [Ca2+]i observed by Przibilla (Przibilla et al., 2018), we observed an additionally delayed inhibition of TRPM3 current by Ca2+. For example, low [Ca2+]i (<10 nM) did not show a delayed inhibition of TRPM3 current (red point, Figure 6A). Under 1 µM [Ca2+]i, the recorded current showed a further inhibition after initial activation and the residual current amplitudes were less than 50% of the initial activation (red point, Figures 6B,C). The inhibition was pH dependent (Figure 6D). However, the pHi concentration-dependence of TRPM3 was not affected by [Ca2+]i, as they showed similar pH50 values (7.0 ± 0.1, nH = 2.1; 7.1 ± 0.1, nH = 1.4; 7.2 ± 0.5, nH = 1.6) for low [Ca2+]i and high Ca2+, (Figure 6E). It is noteworthy that despite having similar pH50 values, TRPM3 outward current densities were significantly decreased in high [Ca2+]i conditions (Figure 6E). We also analyzed the ratio of delayed current intensities compared to the initial current intensities. We did not observe any significant difference between the ratios resulting from different pHi (Figure 6F). Overall, these results indicate that, although [Ca2+]i inhibits TRPM3, it does not affect the regulation of TRPM3 by intracellular protons.
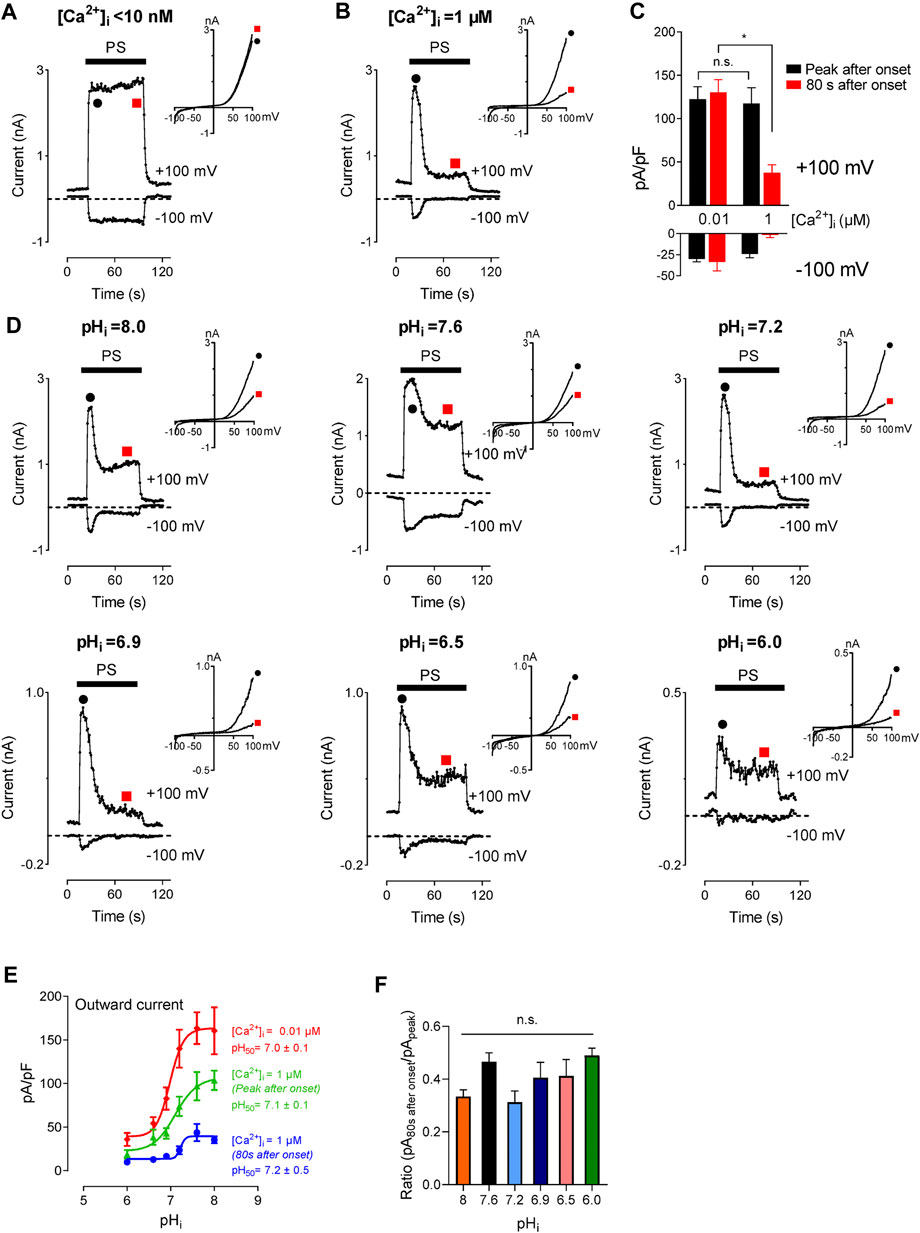
FIGURE 6. Inhibitory effect of intracellular high Ca2+ on TRPM3 activation by PS. (A,B) Time course of TRPM3 currents elicited by voltage ramps ranging −100 to +100 mV at the indicated [Ca2+]i concentration. At high [Ca2+]i (1 µM), TRPM3 is activated by PS initially (Black symbol) but runs down soon afterward (Red symbol). Whereas the TRPM3 current was sustained at the low [Ca2+]i condition. Inserts show the representative recordings from the indicated points. (C) Mean current amplitude of TRPM3 at the indicated [Ca2+]i (mean ± SEM; n = 10). * indicates p < 0.05 by unpaired Student’s t-test; “n.s.” indicates not statistically significant. (D) Time course and representative recordings of TRPM3 current with [Ca2+]i (1 µM) at the indicated pHi from pHi 8.0 to pHi 6.0. (E) pH concentration-dependence of TRPM3 activation by PS at the indicated [Ca2+]i. Green and blue symbols both represent high [Ca2+]i, while green represents currents elicited right after PS application (peak after onset), and blue represent currents remaining after inhibition of by high [Ca2+]i (80 s after onset). All currents were normalized to the corresponding capacitance of the cell overexpressing hTRPM3. Each data point is the mean of 8–14 cells with the error bar showing SEM, at the indicated pHi. (F) Mean ratio of the peak current after onset to the current 80 s after onset (mean ± SEM, n = 8–14 cells). “n.s” indicates not statistically significant among groups by one-way ANOVA with Tukey’s post hoc multiple comparisons.
Molecular Mechanism Underlying Transient Receptor Potential Melastatin 3 Channel Inhibition by Protons
Glutamate, aspartate, histidine, and lysine residues are potential proton acceptors, especially glutamate and aspartate, which present negative charges (Zhou and Pang, 2018). To identify the amino acid residues of TRPM3 accountable for its sensitivity to pHi, we prepared hTRPM3-GFP mutant plasmids with mutations in the pore region. We selected all eight glutamate and aspartate amino acids, in the vestibule of the loop between S5 and S6 transmembrane domains (Figure 7A). These eight residues were either glutamate and aspartate amino acid and mutated to glutamine. We created two double mutants (E1034Q–E1035Q, E1072Q–D1073Q) and six single mutants (E1055Q, D1059Q, D1062Q, E1069Q, E1072Q, and D1073Q). We expressed these mutants in HEK-293 cells and recorded elicited currents in response to PS, while perfusing with physiological and low pH external solutions (Figure 7B). Except for E1055Q, which resulted in a non-functional ion-channel, all other mutants exhibited identical I-V relation to WT-hTRPM3, although most of the mutants showed markedly reduced current amplitudes (Figure 7B). We perfused cells expressing these mutants with external solutions of pH 7.4 and pH 5.5. The pH of 5.5 was selected as representative of low pH external solutions because at this pH, WT-TRPM3 currents were blocked significantly, yet sufficient activity remained for analysis. We compared the percent decrease in TRPM3 current from pHo 7.4 to pHo 5.5 for all the mutants and WT-TRPM3 (Figure 7C). The double mutant (E1034Q–E1035Q), E1069Q, and E1072Q showed similar sensitivity to protons when compared with the WT-TRPM3. Mutants D1059Q and D1062Q were found to be more sensitive to protons, as the reduction of whole-cell current due to low pHo was increased to 93.9 and 84.1% in D1059Q and D1062Q, respectively, compared to 56.5% observed in WT-TRPM3. Mutant D1073Q showed significantly less sensitivity toward protons, as pHo 5.5 reduced its current amplitude only by 25%. As summarized in Figure 7C, these results establish the amino acid residues D1059Q, D1062Q, and D1073Q as key determinants of proton sensitivity in TRPM3. Although it is unclear at this moment why mutating these residues produce variable effects (increased or decreased proton sensitivity), these data suggested that the pore vestibule of TRPM3 is critical for pH sensitivity. Further studies are required to delineate the underlying mechanism responsible for these variable effects.
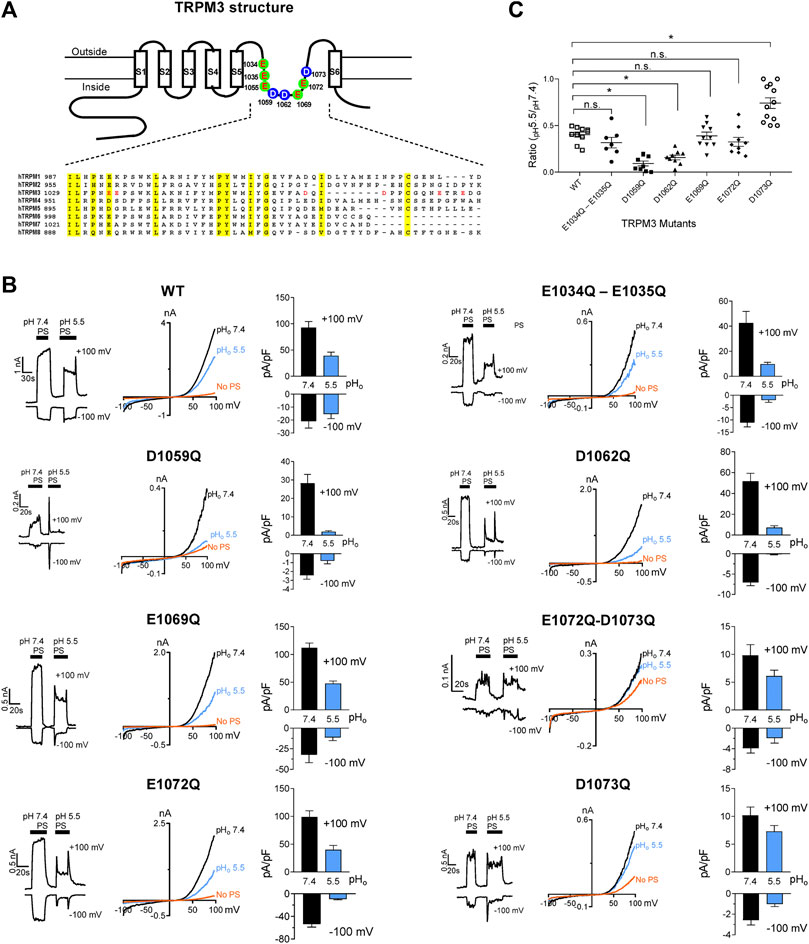
FIGURE 7. Changes in protons sensitivity of TRPM3 mutants. (A) Upper panel: schematic of TRPM3 structure and the substituted amino acid residues in the putative pore region of hTRPM3. Lower panel: sequence alignment of human TRPM channel pore regions. (B) Time course and representative recordings of TRPM3 mutants and wild-type currents elicited by voltage ramps ranging −100 to +100 mV, at the indicated pHo. Bar graphs show mean outward and inward current amplitudes at the indicated pHo (mean ± SEM, n = 7–12 cells) Internal solution had a constant pH of 7.20 for all the recordings. (C) The ratio of outward current amplitudes at pHo 5.5 and pHo 7.4, of TRPM3 mutants and WT control. To obtain these ratios, the current elicited by +100 mV at pHo 5.5 was divided by the current elicited by +100 mV at pHo 7.4 of the same cell (n = 7–12 cells). p values, comparing each group with WT-TRPM3, * indicates p < 0.05 by unpaired Student’s t-test; “n.s.” indicates not statistically significant.
Discussion
Our data suggest that TRPM3 is a proton-permeable channel that is blocked by both extracellular and intracellular protons. We also demonstrated that the blocking effect of the intracellular protons could be reversed by decreasing intracellular protons concentrations, which indicates a reversible binding of protons to the intracellular amino acid residues. We identified several residues localized in the pore region that might be responsible for protons sensitivity of TRPM3. Finally, we demonstrated that low internal pH produces a downward shift of PS concentration-dependent activation of TRPM3 by reducing the amplitude of TRPM3-mediated currents at any given pHo. Overall, we report evidence for the regulatory role of protons on TRPM3 activation and the molecular mechanism responsible for it.
Acidification has modulatory effects on a variety of ion channels (discussed in the introduction) including TRP channels. Extracellular acidic pH modulates TRPV1 channel gating (Jordt et al., 2000; Ryu et al., 2003), stimulates TRPC4 and TRPC5, but inhibits TRPC6 (Semtner et al., 2007), TRPM5 (Liu et al., 2005), and TRPV5 (Yeh et al., 2003). Extracellular pH alsopotentiates TRPM6 and TRPM7 inward currents (Jiang et al., 2005; Li et al., 2006; Li et al., 2007). Intracellular protons, on the other hand, inhibit TRPM7 (Kozak et al., 2005), and block TRPM8 (Andersson et al., 2004). Here we demonstrate that PS-induced TRPM3 outward and inward currents are both inhibited by protons. Although both intracellular and extracellular acidic conditions inhibit TRPM3, there are differences between internal and external proton-induced inhibition. Extracellular protons cannot inhibit TRPM3 until pH reaches less than 6.0, but intracellular pH inhibited TRPM3 with pH50 value of 6.9. We demonstrated a very sharp inhibition by protons at an external pH below 6.0; however, this inhibition did not follow a typical concentration-response relationship. Conversely, internal protons efficiently blocked TRPM3 current and showed a concentration-response relationship. These results suggested that protons do not compete with PS for the same binding site of TRPM3 and that TRPM3 may contain proton-binding sites in the cytoplasmic domains. It is plausible that an increase in extracellular proton concentration causes protons influx through TRPM3 that enables protons to bind to its internal binding site to inhibit TRPM3. To rule out the effects of proton-activated endogenous Cl− currents, we conducted all whole-cell recordings under very low intracellular and extracellular Cl− concentrations. Indeed, our external and internal solutions contained 8 mM Cl−, but this proton concentration was not capable of activating endogenous anions channels in HEK-293 cells (Lambert and Oberwinkler, 2005).
We found that extracellular pH (<6.0) strongly blocked TRPM3 activities, which would indicate TRPM3 is regulated by extracellular acidic pH directly. However, the dose-dependent curve indictaed a low pH50. Reduced intracellular pH appeared to have clear pH concentration-dependent effects on TRPM3, which begs the question then how do external protons affect TRPM3 gating properties? The cell membrane must have a mechanism for the proton permeation and thus change the intracellular pH. Protons may cross the TRPM3 while it is open, although we cannot exclude the possibility that protons may permeate the cell membrane directly.
Our data suggest that protons directly cross TRPM3 when it is activated by PS. Consistently, no proton current was found without activating TRPM3, supporting the conclusion that TRPM3 is permeable to protons. We also studied the regulation of TRPM3 by Ca2+. Previous studies have shown that TRPM3 is a Ca2+ permeable ion channel (Grimm et al., 2003; Lee et al., 2003). TRPM3 channel activity strongly depends on intracellular Ca2+ (Przibilla et al., 2018). Along with the inhibition of TRPM3 current amplitude, we also found that Ca2+ accelerated the decay time of TRPM3. Increased [Ca2+]i from minimum to 1 µM significantly reduced the plateau of the current. Becasue [Ca2+]i is a key regulator of TRPM3 gating, we studied the interaction between [Ca2+]i and protons. High [Ca2+]i did not change the pH50 of the effects of intracellular protons on TRPM3 (Figure 6). Vice versa, decreased protons did not affect the Ca2+ inhibition on TRPM3 (Figure 6). Therefore, it appears unlikely that protons can inhibit TRPM3 channel activities by competing with [Ca2+]i for a binding site on the cytoplasmic side.
Another well-studied TRPM channel that shows similar pH sensitivity is TRPM2. Extracellular acidic pH has been shown to inhibit TRPM2 with a pH50 = 6.5 (Starkus et al., 2010), 5.32 (Du et al., 2009), 4.7 (Yang et al., 2010), depending on the concentration of excellular and intracellular Ca2+ in the recording conditions. For example, Starkus et al. demonstrated that increasing extracellular Ca2+ counteracts the pH-induced inhibition (Starkus et al., 2010) and Du et al. demonstrated that increasing intracellular Ca2+ does not counteract the extracellular pH-induced inhibition (Du et al., 2009). In addition, similar to TRPM3, intracellular low pH also inhibits TRPM2 channels. Two groups have identified binding sites on the TRPM2 near the pore that might be responsible for the pH inhibition. Specifically, Lys952 and Asp1002 and several other residues in the outer vestibule were found to be important in mediating the extracellular pH inhibition whereas Asp933, is important in mediating the intracellular pH inhibition.
To determine the molecular mechanism by which intracellular protons inhibit TRPM3, we mutated all the titratable residues in the pore region between S5 and S6. Among most of the mutations of Asp and Glu residues, three residues-D1059, D1062, and D1073, which are predicted to reside in the pore region, strongly changed the proton sensitivities. We conclude these residues in the pore region could be the proton binding sites. Of course, other intracellular binding sites are also possible. For example, the C terminus of the S4-S5 linker is thought to be critical for changing the TRP channel’s pHi sensitivity (Du et al., 2009). Whether intracellular protons change TRPM3 gating properties through these residues is still unknown, and thus it will be of interest to investigate whether acidic intracellular pH alters intracellular signaling pathways in future studies. It will also be of interest to investigate other potential proton binding sites that might interact with [Ca2+]i near the intracellular mouth and act as the [Ca2+]i-activating site to regulate [Ca2+]i mediated TRPM3 activation. Further investigation is required to test this hypothesis.
Our study might have significant physiological and pathophysiological implications since TRPM3 channels are expressed in adipocytes, pancreatic beta-cells, the kidney, eye, brain and in the pituitary gland. (Fonfria et al., 2006). Stimulation of endogenously expressed TRPM3 channels has been shown to trigger insulin secretion by insulinoma cells. Changes in extracellular pH are also known to affect glucose-stimulated insulin secretion (Hyder et al., 2001). Some reports suggest that during metabolic acidosis, insulin secretion is depressed (Bigner et al., 1996; Mak, 1998). Thus, it is possible that a low pH-mediated dampening of TRPM3 activity might contribute to the decreased insulin secretion observed in metabolic acidosis. If true, then modulating TRPM3 activity might be a potential future clinical application in treating acidosis induced pancreatic disorders. In addition, TRPM3 is required to induce release of calcitonin gene-related peptide (CGRP) (Held et al., 2015). In sensory neurons, the neuropeptide CGRP promotes neurogenic inflammation (Edvinsson et al., 1987). Thus, acidosis generated from infiltrating inflammatory cells might also inhibits TRPM3. Thus, although speculative, such a negative feedback mechanism of protons on regulation of TRPM3 might be beneficial in controlling these important cellular processes.
Collectively, we demonstrated that external and internal acidic pH show strong and state-dependent inhibition of the TRPM3 channels. Asp1073 residue in the vestibule of the channel pore is critical in modulating this inhibition. Given the physiological significance of TRPM3 in numerous cells, including pancreatic beta cells and sensory neurons, understanding TRPM3 gating by protons may generate new physiological and/or pathological insights.
Data Availability Statement
The raw data supporting the conclusions of this article will be made available by the authors, without undue reservation.
Author Contributions
JD conceived and supervised the project. JD, MS, and LX designed the experiments with input from Y-SL and LR, MS, and LX did most of the patch-clamp experiments. Y-SL and LR performed molecular biology experiments and oversaw the mutagenesis of TRPM3. MS and JD drafted the manuscript with input from all authors who contributed to finalizing the manuscript.
Funding
JD is supported by the National Institutes of Mental Health (5R01 MH113986). LR is supported by the National Institutes of Health (R00HL119560, OT2OD023854, OT2OD026582).
Acknowledgments
We thank Lixia Yue for her suggestions and comments. We thank Boren Lin, Erin Koffman, Tyler Ortyl, Farzaneh Naghavi, and Kritika Singh for their comments.
Conflict of Interest
The authors declare that the research was conducted in the absence of any commercial or financial relationships that could be construed as a potential conflict of interest.
Supplementary Material
The Supplementary Material for this article can be found online at: https://www.frontiersin.org/articles/10.3389/fphar.2021.632711/full#supplementary-material.
References
Alonso-Carbajo, L., Alpizar, Y. A., Startek, J. B., Lopez-Lopez, J. R., Perez-Garcia, M. T., and Talavera, K. (2019). Activation of the cation channel TRPM3 in perivascular nerves induces vasodilation of resistance arteries. J. Mol. Cell. Cardiol. 129, 219–230. doi:10.1016/j.yjmcc.2019.03.003
Andersson, D. A., Chase, H. W., and Bevan, S. (2004). TRPM8 activation by menthol, icilin, and cold is differentially modulated by intracellular pH. J. Neurosci. 24, 5364–5369. doi:10.1523/JNEUROSCI.0890-04.2004
Bennett, T. M., Mackay, D. S., Siegfried, C. J., and Shiels, A. (2014). Mutation of the melastatin-related cation channel, TRPM3, underlies inherited cataract and glaucoma. PloS One 9, e104000. doi:10.1371/journal.pone.0104000
Bigner, D. R., Goff, J. P., Faust, M. A., Burton, J. L., Tyler, H. D., and Horst, R. L. (1996). Acidosis effects on insulin response during glucose tolerance tests in Jersey cows. J. Dairy Sci. 79, 2182–2188. doi:10.3168/jds.S0022-0302(96)76594-3
Brauchi, S., and Orio, P. (2011). Voltage sensing in thermo-TRP channels. Adv. Exp. Med. Biol. 704, 517–530. doi:10.1007/978-94-007-0265-3_28
Caterina, M. J., Schumacher, M. A., Tominaga, M., Rosen, T. A., Levine, J. D., and Julius, D. (1997). The capsaicin receptor: a heat-activated ion channel in the pain pathway. Nature 389, 816–824. doi:10.1038/39807
Chandrashekar, J., Hoon, M. A., Ryba, N. J., and Zuker, C. S. (2006). The receptors and cells for mammalian taste. Nature 444, 288–294. doi:10.1038/nature05401
Ciurtin, C., Majeed, Y., Naylor, J., Sukumar, P., English, A. A., Emery, P., et al. (2010). TRPM3 channel stimulated by pregnenolone sulphate in synovial fibroblasts and negatively coupled to hyaluronan. BMC Muscoskel. Disord. 11, 111. doi:10.1186/1471-2474-11-111
Clapham, D. E. (2003). TRP channels as cellular sensors. Nature 426, 517–524. doi:10.1038/nature02196
Du, J., Xie, J., and Yue, L. (2009). Modulation of TRPM2 by acidic pH and the underlying mechanisms for pH sensitivity. J. Gen. Physiol. 134, 471–488. doi:10.1085/jgp.200910254
Dyment, D. A., Terhal, P. A., Rustad, C. F., Tveten, K., Griffith, C., Jayakar, P., et al. (2019). De novo substitutions of TRPM3 cause intellectual disability and epilepsy. Eur. J. Hum. Genet. 27, 1611–1618. doi:10.1038/s41431-019-0462-x
Edvinsson, L., Ekman, R., Jansen, I., Mcculloch, J., and Uddman, R. (1987). Calcitonin gene-related peptide and cerebral blood vessels: distribution and vasomotor effects. J. Cerebr. Blood Flow Metabol. 7, 720–728. doi:10.1038/jcbfm.1987.126
Everaerts, W., Gees, M., Alpizar, Y. A., Farre, R., Leten, C., Apetrei, A., et al. (2011). The capsaicin receptor TRPV1 is a crucial mediator of the noxious effects of mustard oil. Curr. Biol. 21, 316–321. doi:10.1016/j.cub.2011.01.031
Fonfria, E., Murdock, P. R., Cusdin, F. S., Benham, C. D., Kelsell, R. E., and Mcnulty, S. (2006). Tissue distribution profiles of the human TRPM cation channel family. J. Recept. Signal Transduct. Res. 26, 159–178. doi:10.1080/10799890600637506
Gerdes, J. M., Liu, Y., Zaghloul, N. A., Leitch, C. C., Lawson, S. S., Kato, M., et al. (2007). Disruption of the basal body compromises proteasomal function and perturbs intracellular Wnt response. Nat. Genet. 39, 1350–1360. doi:10.1038/ng.2007.12
Grimm, C., Kraft, R., Sauerbruch, S., Schultz, G., and Harteneck, C. (2003). Molecular and functional characterization of the melastatin-related cation channel TRPM3. J. Biol. Chem. 278, 21493–21501. doi:10.1074/jbc.M300945200
Hardie, R. C., and Franze, K. (2012). Photomechanical responses in Drosophila photoreceptors. Science 338, 260–263. doi:10.1126/science.1222376
Hardie, R. C. (2014). Photosensitive TRPs. Handb. Exp. Pharmacol. 223, 795–826. doi:10.1007/978-3-319-05161-1_4
Held, K., Kichko, T., De Clercq, K., Klaassen, H., Van Bree, R., Vanherck, J. C., et al. (2015). Activation of TRPM3 by a potent synthetic ligand reveals a role in peptide release. Proc. Natl. Acad. Sci. U. S. A. 112, E1363–E1372. doi:10.1073/pnas.1419845112
Held, K., Mulier, M., Van Ranst, N., Ge, Y., Voets, T., Wang, Y. T., et al. (2020). TRPM3 inhibits synaptic transmission and plasticity in the Hippocampus. Biophys. J. 118, 21a. doi:10.1016/j.bpj.2019.11.297
Holzer, P. (2009). Acid-sensitive ion channels and receptors. Handb. Exp. Pharmacol. 194, 283–332. doi:10.1007/978-3-540-79090-7_9
Huang, A. L., Chen, X., Hoon, M. A., Chandrashekar, J., Guo, W., Tränkner, D., et al. (2006). The cells and logic for mammalian sour taste detection. Nature 442, 934–938. doi:10.1038/nature05084
Hyder, A., Laue, C., and Schrezenmeir, J. (2001). Effect of extracellular pH on insulin secretion and glucose metabolism in neonatal and adult rat pancreatic islets. Acta Diabetol. 38, 171–178. doi:10.1007/s592-001-8075-9
Jacobs, M. H. (1922). The influence of ammonium salts on cell reaction. J. Gen. Physiol. 5, 181–188. doi:10.1085/jgp.5.2.181
Jiang, J., Li, M., and Yue, L. (2005). Potentiation of TRPM7 inward currents by protons. J. Gen. Physiol. 126, 137–150. doi:10.1085/jgp.200409185
Jordt, S. E., Tominaga, M., and Julius, D. (2000). Acid potentiation of the capsaicin receptor determined by a key extracellular site. Proc. Natl. Acad. Sci. U. S. A. 97, 8134–8139. doi:10.1073/pnas.100129497
Kozak, J. A., Matsushita, M., Nairn, A. C., and Cahalan, M. D. (2005). Charge screening by internal pH and polyvalent cations as a mechanism for activation, inhibition, and rundown of TRPM7/MIC channels. J. Gen. Physiol. 126, 499–514. doi:10.1085/jgp.200509324
Lambert, S., and Oberwinkler, J. (2005). Characterization of a proton-activated, outwardly rectifying anion channel. J. Physiol. 567, 191–213. doi:10.1113/jphysiol.2005.089888
Lee, N., Chen, J., Sun, L., Wu, S., Gray, K. R., Rich, A., et al. (2003). Expression and characterization of human transient receptor potential melastatin 3 (hTRPM3). J. Biol. Chem. 278, 20890–20897. doi:10.1074/jbc.M211232200
Lesch, A., Rubil, S., and Thiel, G. (2014). Activation and inhibition of transient receptor potential TRPM3-induced gene transcription. Br. J. Pharmacol. 171, 2645–2658. doi:10.1111/bph.12524
Li, M., Du, J., Jiang, J., Ratzan, W., Su, L. T., Runnels, L. W., et al. (2007). Molecular determinants of Mg2+ and Ca2+ permeability and pH sensitivity in TRPM6 and TRPM7. J. Biol. Chem. 282, 25817–25830. doi:10.1074/jbc.M608972200
Li, M., Jiang, J., and Yue, L. (2006). Functional characterization of homo- and heteromeric channel kinases TRPM6 and TRPM7. J. Gen. Physiol. 127, 525–537. doi:10.1085/jgp.200609502
Liedtke, W., and Friedman, J. M. (2003). Abnormal osmotic regulation in trpv4-/- mice. Proc. Natl. Acad. Sci. U. S. A. 100, 13698–13703. doi:10.1073/pnas.1735416100
Liu, D., Zhang, Z., and Liman, E. R. (2005). Extracellular acid block and acid-enhanced inactivation of the Ca2+-activated cation channel TRPM5 involve residues in the S3-S4 and S5-S6 extracellular domains. J. Biol. Chem. 280, 20691–20699. doi:10.1074/jbc.M414072200
Mak, R. H. (1998). Effect of metabolic acidosis on insulin action and secretion in uremia. Kidney Int. 54, 603–607. doi:10.1046/j.1523-1755.1998.00023.x
Maroto, R., Raso, A., Wood, T. G., Kurosky, A., Martinac, B., and Hamill, O. P. (2005). TRPC1 forms the stretch-activated cation channel in vertebrate cells. Nat. Cell Biol. 7, 179–185. doi:10.1038/ncb1218
Mckemy, D. D., Neuhausser, W. M., and Julius, D. (2002). Identification of a cold receptor reveals a general role for TRP channels in thermosensation. Nature 416, 52–58. doi:10.1038/nature719
Minke, B. (1977). Drosophila mutant with a transducer defect. Biophys. Struct. Mech. 3, 59–64. doi:10.1007/BF00536455
Montell, C., Jones, K., Hafen, E., and Rubin, G. (1985). Rescue of the Drosophila phototransduction mutation trp by germline transformation. Science 230, 1040–1043. doi:10.1126/science.3933112
Moran, M. M., and Szallasi, A. (2018). Targeting nociceptive transient receptor potential channels to treat chronic pain: current state of the field. Br. J. Pharmacol. 175, 2185–2203. doi:10.1111/bph.14044
Muller, I., Rossler, O. G., and Thiel, G. (2011). Pregnenolone sulfate activates basic region leucine zipper transcription factors in insulinoma cells: role of voltage-gated Ca2+ channels and transient receptor potential melastatin 3 channels. Mol. Pharmacol. 80, 1179–1189. doi:10.1124/mol.111.074781
Naylor, J., Li, J., Milligan, C. J., Zeng, F., Sukumar, P., Hou, B., et al. (2010). Pregnenolone sulphate- and cholesterol-regulated TRPM3 channels coupled to vascular smooth muscle secretion and contraction. Circ. Res. 106, 1507–1515. doi:10.1161/CIRCRESAHA.110.219329
Oberwinkler, J., Lis, A., Giehl, K. M., Flockerzi, V., and Philipp, S. E. (2005). Alternative splicing switches the divalent cation selectivity of TRPM3 channels. J. Biol. Chem. 280, 22540–22548. doi:10.1074/jbc.M503092200
Oberwinkler, J., and Philipp, S. E. (2014). TRPM3. Handb. Exp. Pharmacol. 222, 427–459. doi:10.1007/978-3-642-54215-2_17
Pan, Z., Yang, H., and Reinach, P. S. (2011). Transient receptor potential (TRP) gene superfamily encoding cation channels. Hum. Genom. 5, 108–116. doi:10.1186/1479-7364-5-2-108
Przibilla, J., Dembla, S., Rizun, O., Lis, A., Jung, M., Oberwinkler, J., et al. (2018). Ca(2+)-dependent regulation and binding of calmodulin to multiple sites of Transient Receptor Potential Melastatin 3 (TRPM3) ion channels. Cell Calcium 73, 40–52. doi:10.1016/j.ceca.2018.03.005
Quallo, T., Vastani, N., Horridge, E., Gentry, C., Parra, A., Moss, S., et al. (2015). TRPM8 is a neuronal osmosensor that regulates eye blinking in mice. Nat. Commun. 6, 7150. doi:10.1038/ncomms8150
Ryu, S., Liu, B., and Qin, F. (2003). Low pH potentiates both capsaicin binding and channel gating of VR1 receptors. J. Gen. Physiol. 122, 45–61. doi:10.1085/jgp.200308847
Semtner, M., Schaefer, M., Pinkenburg, O., and Plant, T. D. (2007). Potentiation of TRPC5 by protons. J. Biol. Chem. 282, 33868–33878. doi:10.1074/jbc.M702577200
Smith, C. C., Gibbs, T. T., and Farb, D. H. (2014). Pregnenolone sulfate as a modulator of synaptic plasticity. Psychopharmacology (Berlin) 231, 3537–3556. doi:10.1007/s00213-014-3643-x
Starkus, J. G., Fleig, A., and Penner, R. (2010). The calcium-permeable non-selective cation channel TRPM2 is modulated by cellular acidification. J. Physiol. 588, 1227–1240. doi:10.1113/jphysiol.2010.187476
Straub, I., Krugel, U., Mohr, F., Teichert, J., Rizun, O., Konrad, M., et al. (2013). Flavanones that selectively inhibit TRPM3 attenuate thermal nociception in vivo. Mol. Pharmacol. 84, 736–750. doi:10.1124/mol.113.086843
Strotmann, R., Harteneck, C., Nunnenmacher, K., Schultz, G., and Plant, T. D. (2000). OTRPC4, a nonselective cation channel that confers sensitivity to extracellular osmolarity. Nat. Cell Biol. 2, 695–702. doi:10.1038/35036318
Sun, Y., Liu, L., Ben-Shahar, Y., Jacobs, J. S., Eberl, D. F., and Welsh, M. J. (2009). TRPA channels distinguish gravity sensing from hearing in Johnston's organ. Proc. Natl. Acad. Sci. U. S. A. 106, 13606–13611. doi:10.1073/pnas.0906377106
Suzuki, M., Mizuno, A., Kodaira, K., and Imai, M. (2003). Impaired pressure sensation in mice lacking TRPV4. J. Biol. Chem. 278, 22664–22668. doi:10.1074/jbc.M302561200
Tepper, H. L., and Voth, G. A. (2005). Protons may leak through pure lipid bilayers via a concerted mechanism. Biophys. J. 88, 3095–3108. doi:10.1529/biophysj.104.056184
Uchida, K., Fukuta, N., Yamazaki, J., and Tominaga, M. (2019). Identification and classification of a new TRPM3 variant (gamma subtype). J. Physiol. Sci. 69, 623–634. doi:10.1007/s12576-019-00677-6
Vandewauw, I., De Clercq, K., Mulier, M., Held, K., Pinto, S., Van Ranst, N., et al. (2018). A TRP channel trio mediates acute noxious heat sensing. Nature 555, 662–666. doi:10.1038/nature26137
Vangeel, L., Benoit, M., Miron, Y., Miller, P. E., De Clercq, K., Chaltin, P., et al. (2020). Functional expression and pharmacological modulation of TRPM3 in human sensory neurons. Br. J. Pharmacol. 177, 2683–2695. doi:10.1111/bph.14994
Venkatachalam, K., and Montell, C. (2007). TRP channels. Annu. Rev. Biochem. 76, 387–417. doi:10.1146/annurev.biochem.75.103004.142819
Vriens, J., Owsianik, G., Hofmann, T., Philipp, S. E., Stab, J., Chen, X., et al. (2011). TRPM3 is a nociceptor channel involved in the detection of noxious heat. Neuron 70, 482–494. doi:10.1016/j.neuron.2011.02.051
Wagner, T. F., Loch, S., Lambert, S., Straub, I., Mannebach, S., Mathar, I., et al. (2008). Transient receptor potential M3 channels are ionotropic steroid receptors in pancreatic beta cells. Nat. Cell Biol. 10, 1421–1430. doi:10.1038/ncb1801
Warburg, E. J. (1922). Studies on carbonic acid compounds and hydrogen ion activities in blood and salt solutions. A contribution to the theory of the equation of lawrence J. Henderson and K. A. Hasselbach: introduction. Biochem. J. 16, 153–154. doi:10.1042/bj0160153
Yang, W., Zou, J., Xia, R., Vaal, M. L., Seymour, V. A., Luo, J., et al. (2010). State-dependent inhibition of TRPM2 channel by acidic pH. J. Biol. Chem. 285, 30411–30418. doi:10.1074/jbc.M110.139774
Yeh, B. I., Sun, T. J., Lee, J. Z., Chen, H. H., and Huang, C. L. (2003). Mechanism and molecular determinant for regulation of rabbit transient receptor potential type 5 (TRPV5) channel by extracellular pH. J. Biol. Chem. 278, 51044–51052. doi:10.1074/jbc.M306326200
Zamudio-Bulcock, P. A., Everett, J., Harteneck, C., and Valenzuela, C. F. (2011). Activation of steroid-sensitive TRPM3 channels potentiates glutamatergic transmission at cerebellar Purkinje neurons from developing rats. J. Neurochem. 119, 474–485. doi:10.1111/j.1471-4159.2011.07441.x
Keywords: transient receptor potential channels, TRPM3, protons, Pregnenolone sulfate, site-directed mutagenesis, PH sensitivity
Citation: Hossain Saad MZ, Xiang L, Liao Y-S, Reznikov LR and Du J (2021) The Underlying Mechanism of Modulation of Transient Receptor Potential Melastatin 3 by protons. Front. Pharmacol. 12:632711. doi: 10.3389/fphar.2021.632711
Received: 23 November 2020; Accepted: 04 January 2021;
Published: 02 February 2021.
Edited by:
Tian-Le Xu, Shanghai Jiao Tong University, ChinaCopyright © 2021 Hossain Saad, Xiang, Liao, Reznikov and Du. This is an open-access article distributed under the terms of the Creative Commons Attribution License (CC BY). The use, distribution or reproduction in other forums is permitted, provided the original author(s) and the copyright owner(s) are credited and that the original publication in this journal is cited, in accordance with accepted academic practice. No use, distribution or reproduction is permitted which does not comply with these terms.
*Correspondence: Jianyang Du, amR1MTVAdXRoc2MuZWR1
†These authors have contributed equally to this work