- 1College of First Clinical Medicine, Shandong University of Traditional Chinese Medicine, Jinan, China
- 2College of Traditional Chinese Medicine, Shandong University of Traditional Chinese Medicine, Jinan, China
- 3Qingdao Academy of Chinese Medical Sciences, Shandong University of Traditional Chinese Medicine, Qingdao, China
- 4Department of Oncology, Weifang Traditional Chinese Hospital, Weifang, China
The treatment process of tumor is advanced with the development of immunotherapy. In clinical experience, immunotherapy has achieved very significant results. However, the application of immunotherapy is limited by a variety of immune microenvironment. For a long time in the past, polysaccharides such as lentinan and Ganoderma lucidum glycopeptide have been used in clinic as adjuvant drugs to widely improve the immunity of the body. However, their mechanism in tumor immunotherapy has not been deeply discussed. Studies have shown that natural polysaccharides can stimulate innate immunity by activating upstream immune cells so as to regulate adaptive immune pathways such as T cells and improve the effect of immunotherapy, suggesting that polysaccharides also have a promising future in cancer therapy. This review systematically discusses that polysaccharides can directly or indirectly activate macrophages, dendritic cells, natural killer cells etc., binding to their surface receptors, inducing PI3K/Akt, mitogen-activated protein kinase, Notch and other pathways, promote their proliferation and differentiation, increasing the secretion of cytokines, and improve the state of immune suppression. These results provide relevant basis for guiding polysaccharide to be used as adjuvants of cancer immunotherapy.
Introduction
Cancer remains one of the major health threats worldwide despite the continuous development of diagnostic tools and therapeutic drugs. Updated immunotherapy plays a role in the treatment of tumors, especially the immunotherapy represented by immune checkpoint inhibitor (ICI) gradually shows significant efficacy. Despite numerous reports of clinical benefits from immunotherapy, real-world studies have shown a low response rate to immunotherapy, with fewer than 20% people responding (Haslam and Prasad, 2019). This pessimistic response rate may be closely related to the different microenvironmental states of immune inhibition in tumor patients.
The state of immune microenvironment is crucial to immune efficacy, and immune cytokines play a key role in the transformation of immune microenvironment by regulating immune cells. In the tumor immune microenvironment of ineffective population (cold tumor), the immune infiltrating cells are often in a state of lack or inhibition, and the tumor itself will also produce immunosuppressive cytokines (Duan et al., 2020), further exacerbating the immunosuppressive state. On the contrary, the effective population (“hot” tumor) are characterized by the accumulation of pro-inflammatory cytokines and immune cells infiltration. The activation of immune effector cells promotes the production of cytokines and specific antibodies, which can better trigger the immune response (Figure 1). A research team at the University of North Carolina (Hollern et al., 2019) found that in triple-negative breast cancer, T follicular helper cells activated by ICIs regulate the production of antibodies by B cells, which are critical to the efficacy of immunotherapy. In addition, natural killer cells (NKs) can lyse tumor cells by recognizing tumor-derived antigens or cell surface stress molecules (Paul and Lal, 2017). Macrophages can directly participate in tumoricidal activity through antibody-dependent cell-mediated cytotoxicity (ADCC) and antibody-dependent cellular phagocytosis (ADCP) (Ginefra et al., 2020).
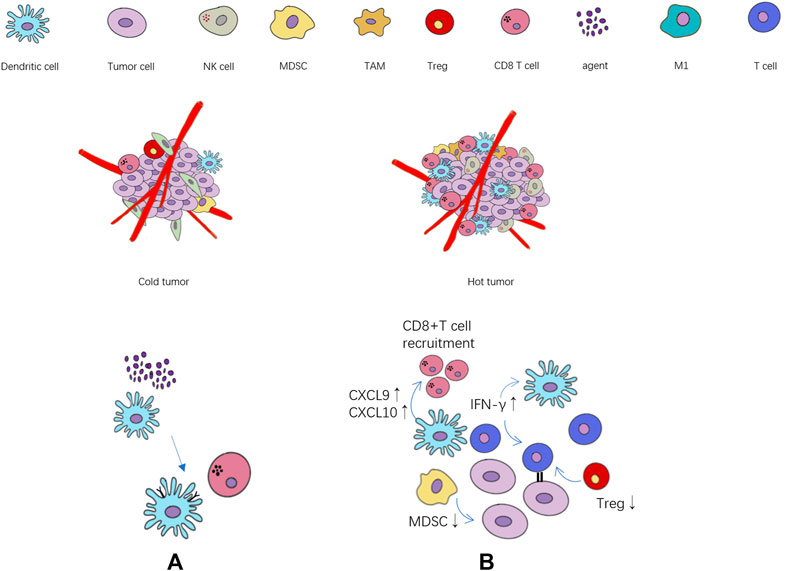
FIGURE 1. The relationship between the state of tumor immune cell infiltration and immunotherapy. (A) The agents activate the maturation of dendritic cells, and further activate CD8+ T cell. (B) Cytokines, chemokines and immune cells associated with immune activation and immunosuppression state. ↑: immune activation ↓: immune suppression.
Immune cells are divided into innate immune cells and acquired immune cells. In the course of tumorigenesis and development, the innate and adaptive immune systems play a role in host protection. Immune cells infiltrate tumors, co-evolve and cooperate with tumor cells, create a microenvironment of inflammation and immunosuppression, and promote tumor growth and spread. The innate immune system can directly inhibit tumor progression by participating in the tumor-killing activity, or it can indirectly participate in the anti-tumor process by releasing various inflammatory cytokines and recruiting adaptive immune cells (Fridman et al., 2017; Ginefra et al., 2020).
When the solid tumor grows to a certain extent, it will be invasive and cause slight damages in the surrounding tissue, inducing inflammatory signals, thus causing innate immune cells to recruit to this site and stimulate immune cells to produce IFN-γ and various chemokines (Tang et al., 2012), which stimulate the innate immunity, causing the death of tumor cells. The tumor cell fragments formed after apoptosis are absorbed by local dendritic cells and migrated to the draining lymph nodes, thus inducing tumor-specific CD4+ and CD8+T cells to migrate to the tumor site, producing the acquired immunity and stimulating the anti-tumor activity.
Innate immune cells (macrophages, neutrophils, DCs, and NKs), in conjunction with adaptive immunity (T and B lymphocytes), provide a strong first line of defense against cancer cells, detecting and eliminating more immunogenic cancer cells and counteracting spontaneous tumor growth (Pasto et al., 2020). Adaptive immunity is not an autonomous process, requiring antigen presentation from specialized cells in a pro-inflammatory environment. It is undeniable that the application of T cell immunotherapy in cancer treatment has achieved unprecedented success. However, its application is still limited to several tumor types. In this context, the regulation of innate immunity seems to have important implications for the intervention of tumor immunotherapeutic effects. Therefore, it is worth exploring whether innate immunity can be used as a potential target for immunotherapy or whether adaptive immunity can be further regulated through the activation of innate immunity.
It has been found that the innate immunity can have an impact on current T cell cancer immunotherapy and can provide potential opportunities for the development of new therapeutic strategies. Therefore, we need to look for drugs that regulate the non-specific immunity. At the same time, natural compounds have attracted people’s attention because of their wide range of non-specific targets and high level of safety. Polysaccharides, polyphenols, flavonoids, terpenes, saponins, and other natural substances have been proved to have anti-tumor and immunomodulatory effects (Deng et al., 2020). However, we believe that compared with other drugs, natural polysaccharides are biological response modulators (BRM), which produce a wide range of immune enhancement mainly by activating the host immune system, including the innate and acquired immunity (Jiang et al., 2010; Hou et al., 2020). It has little cytotoxicity in humans and has great potential for combination therapy. Additionally, their extraction source drugs have better immune regulation effects on the body, and are more suitable for the construction of follow-up derivatives. In the clinical experience, their extracted source drugs have better immune regulation ability and anti-tumor activity on human body, and the diversity of composition and structure is more suitable for the construction of subsequent derivatives. Furthermore, a number of experimental studies have shown that natural polysaccharides can regulate the host immune system and activate the anti-tumor activity of immune cells in a tumor microenvironment (TME). Therefore, the study of natural polysaccharides on tumor immunotherapy is of great significance. This review discusses the regulation effect of innate immune regulation on tumor immunotherapy and natural product polysaccharide on innate immune cells.
The Regulatory Mechanism of the Tumor Immune System
The Immunomodulatory Mechanism of Macrophage Cell
Macrophages, one of the important members of the body’s innate immune defense Frontier, derived from bone marrow progenitor cells (Hume et al., 2002), playing an important role as a bridge between the innate immunity and acquired immunity. They exist in almost all tissues and their main functions are phagocytosis and degradation of dead cells, cell fragments, and foreign pathogens, as well as coordinating inflammatory processes (Hume, 2006; Bonnardel and Guilliams, 2018). Furthermore, macrophages can present antigens, secrete a variety of active substances, as well as regulate the local microenvironment and other physiological functions (Gordon and Plüddemann, 2017).
Macrophages can be activated by different stimuli to polarize M1 and M2 phenotypes, which reflect the characteristics of Th1 and Th2 cells, respectively (Locati et al., 2013). CD86 is considered to be a marker of M1 macrophages, while CD206 is usually used to identify and screen M2 macrophages (Shapouri-Moghaddam et al., 2018; Wang et al., 2019). Under the stimulation of IFN-γ, microbial stimulation (such as LPS), and cytokines (TNF, GM-CSF), macrophages often polarize to the M1 phenotype, with high bactericidal, bacteriostatic, pro-inflammatory and tumor cytotoxic activity, and the potential to kill tumor cells. However, in the TME, differentiated macrophages are typically polarized to the M2 phenotype by anti-inflammatory molecules (such as IL4, IL-13, etc.) (Mantovani et al., 2004; Locati et al., 2013; Tao et al., 2020). Type M2 tumor-associated macrophage (TAM) contributes to tissue repair, tumor angiogenesis, wound healing, and promotes the survival, proliferation, and spread of tumor cells (Wei et al., 2019; Tao et al., 2020). Therefore, in order to balance the level of tumor immunosuppression and immune activation for killing or clearing the tumor, we need to polarize macrophages to M1 or adjust the ratio of M1 to M2.
As a group of cells characterized with plasticity and pluripotency, macrophages show significant functional differences under the influence of different microenvironments. TAMs exist widely in the anoxic region of TME, and most of them are M2 polarized, resulting in immunosuppressive phenotype and inhibition of T cell-mediated adaptive immunity. A series of studies have shown that TAMs affect the therapeutic effect of anti-pd-L1 and anti-CTLA-4 drugs to a great extent, and the curative effect has been observed when traditional immunotherapy were combined with macrophage targeting strategy (Ginefra et al., 2020), but its specific mechanism needs to be further explored.
In general, the recruitment, phagocytosis, survival and functional of macrophages show a high correlation with specific immunity, while the polarization and distribution of M1 and M2 macrophages have been shown to affect the course and treatment response of cancer (Larionova et al., 2020). Therefore, the adjuvant therapy strategy of macrophages as drug targets may improve the efficiency of immunotherapy in an all-round way.
The Immunomodulatory Mechanism of Dendritic Cells
The dendritic cells (DCs) are a subset of innate immune cells that are the key mediators of anti-tumor immunity, while T lymphocytes are the key components of anti-virus and anti-tumor immunity. Further elucidating the biological mechanism of DC infiltration and activation in tumor and controlling T cell immunity will be of great significance for the selection of a reasonable combination therapy in the future. Conceptually, this will promote the transformation of T cell inflammation, and increase the proportion of patients who benefit from cancer immunotherapy (Garris and Luke, 2020).
Dendritic cells are generally divided into plasmacytoid precursor DCs (pDCs) and conventional DCs (cDCs). In previous studies, pDC is mainly by producing I-IFN to participate in antiviral immunity or other dendritic cell activation; In contrast, cDC can further differentiate through the expression of surface immune receptors, or through cross-present antigens and other ways to activate anti-tumor specific immune effects (Chan and Housseau, 2008). Thus, the DC can be used to stimulate immature T lymphocytes, which forms a heterogeneous group of specialized antigens presenting cell (APC), and its function is integrated into innate and adaptive immune responses (Ginefra et al., 2020).
Tumor cells often create an immunosuppressive microenvironment through some mechanisms, affecting the maturation and activation of DC, leading to insufficient T cell activation, and possibly inducing T cell tolerance to tumor-associated antigens. In addition, metabolites in TME (such as lactic acid, etc.) also inhibit the function of DC (Wculek et al., 2020). As the APC in tumor microenvironment (TME), the DC initiates the cancer immune cycle by cross-presenting tumor-associated antigens to naive T cells (Ginefra et al., 2020). VEGF, IL-6 and IL-10, which are usually overexpressed in TME, can activate STAT3 signaling, thereby inducing an immature tolerance phenotype in tumor-associated DCs and promoting tumor progression (Nefedova et al., 2004; Yu et al., 2009). The regulation method mentioned in this article is a general summary of the experiment of plant polysaccharides to activate DCs. In the past, people have tried a variety of polysaccharides to regulate DCs, thereby improving the body’s anti-tumor immunity.
The Immune Regulation Mechanism of Natural Killer Cells
NK cells are important innate immune cells that constitute the first line of defense against microbial infections and cancer development, with a strong killing function against tumor cells, virus-infected cells, and other physiologically stressed cells (Paul and Lal, 2017; Trefny et al., 2020). The NK cell activity is a significant index of the non-specific immune system. They can not only directly kill tumor cells by secreting cytoplasmic particles, releasing various cytokines, participating in death receptor-mediated apoptosis and ADCC, but also indirectly play a role by producing different cytokines and chemokines interacting with other immune cells (Trefny et al., 2020).
NK cells promote the recruitment of DC to solid tumors by releasing a variety of chemokine ligands (such as CCL5, XCL2, etc.), and promote the polarization of Th1 cells by releasing IFN-γ (Chiossone et al., 2018). In TME, DC-NK crosstalk can promote the maturation of DC, which makes DC secreting IL-12 and promoting the expression of CD86 so that to enhance the activation of CD8 T cell. NK cells can also indirectly regulate T cells by regulating APCs to ensure the initiation of T cells (Pierce et al., 2020). Therefore, there is a significant correlation between tumor-related immune function impairment and the biological function of NK cells.
In cancer patients receiving various treatments, the immune function may be reduced or impaired, resulting in decreased cytotoxic T lymphocytes and NK activity. For advanced cancer patients, NK activity is significantly reduced and cytokine production is impaired and related to poor prognosis (Gao et al., 2005a). When some NK cells did not express ligands for MHC-I molecules, or when there was a lack of MHC-I presentation, they could be regarded as hyporesponsiveness of NK cells (Zimmer et al., 1998; Anfossi et al., 2006). Additionally, the expression of PD-1 on NK cells may be related to the low responsiveness of NK cells (Trefny et al., 2020). Therefore, the low reactivity of NK cells may be related to the clinical benefits of ICIs. Regulating the activity of NK cells can synergistically regulate multi-level immune response, and finally achieve protective and lasting immunity against tumors.
In short, tumor immunotherapy needs to activate the immune mechanism again and maintain its dynamic balance. However, according to the heterogeneity of individuals and tumors, the effects of immunotherapy are very different: non-synonymous somatic mutations in individual genes or low genomic mutation load are related to the lack of clinical benefits and immune resistance of ICIs (Law et al., 2020). The expansion of immunosuppressive cells, the expression of inhibitory cytokines and proteins, as well as angiogenesis suppress the immune response, which is the reason for the unsatisfactory clinical results of cancer vaccines (Hollingsworth and Jansen, 2019; Mougel et al., 2019). This prompted us to seek a way to induce the activation of immune cells, assist the way of action of immune preparations, and enhance therapeutic effects.
Natural Polysaccharides in Anti-Tumor Activity
We searched PubMed and Google Scholar using keywords such as “polysaccharide,” “macrophage cell,” “dendritic cell,” “NK cell,” “cancer immunotherapy,” among others. From the retrieved literature, we summarized the regulatory mechanism of plant polysaccharides represented by Chinese herbal and fungal polysaccharides in tumor immunotherapy.
It is reported that more than 300 kinds of bioactive polysaccharides have been isolated from natural products. According to the sources, they can be divided into five categories: fungal, higher plant, lichen and algae, animal, and bacterial polysaccharides (Yin et al., 2019). Polysaccharides are generally composed of glycosidic covalently linked monosaccharides and contained different proportions of mannose, galactose, glucose, xylose, arabinose, rhamnose and other types of monosaccharides (Pang et al., 2018). At present, researches are focused on arabinogalactan, galactomannan, and pectin polysaccharides from higher plants, β-glucan from fungi, and sulfated polysaccharides from seaweed (Yu et al., 2018). Plant polysaccharides contain a variety of skeleton structures and have great potential for structural diversity so that they have the most powerful ability to carry a large amount of biological information (Jiao et al., 2016). In addition, several laboratory studies have shown that fungal polysaccharides have higher anti-tumor functions, while plant polysaccharides may have a better effect of enhancing the immunomodulatory effect (Jiang et al., 2010; Tang et al., 2012; Hou et al., 2020).
Previous studies have shown that polysaccharides are involved in the regulation of various immune cell-mediated biological phenomena, having certain anti-tumor and extensive immunomodulatory effects (Jiang et al., 2010). We briefly summarized the regulation process of polysaccharides on innate immunity in Figure 2, and summarized the respective action pathways of plant and fungal polysaccharides in Supplementary Figure S1. Their anti-tumor activity is usually mediated through two main pathways. One refers to the direct suppression/eradication of malignant cells and activation of the innate and/or adaptive immune system, while the other contributes to the activation of various immune cells and increases the production of a series of important immunomodulatory cytokines (Li W. et al., 2019). The binding of polysaccharides to specific receptors [Toll-like receptors 4 (TLR4), scavenger receptor (SR), etc.] on DCs and macrophages promotes their activation and maturation, enhances the production of pro-inflammatory cytokines, stimulating polarization to Th1, induces the activation of antigen-specific CD8+ CTLs, and improves the immunosuppressive state of the tumor microenvironment (Liu et al., 2016). Several researches have shown that polysaccharides stimulate the release of TNF-α and NO in macrophages, and they contribute to the anti-tumor activity and immune regulation of tumor-bearing hosts. Moreover, they can also enhance the antibacterial activity of neutrophils and promote the cytotoxicity of NK cells (Li W. et al., 2019; Bamodu et al., 2019; Del Corno et al., 2020).
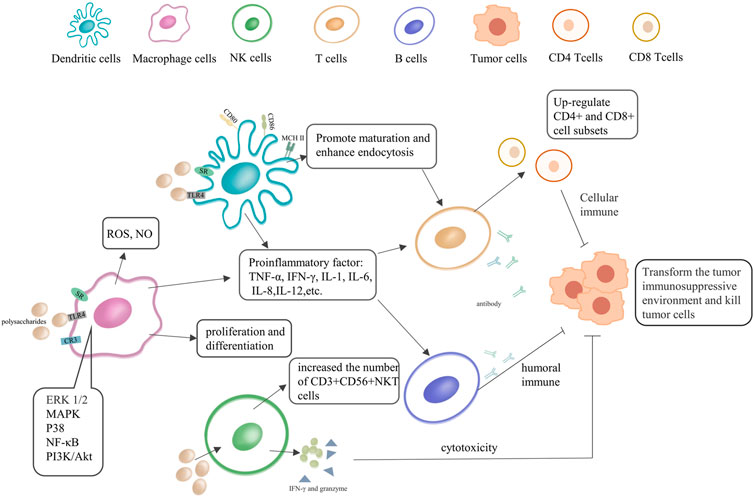
FIGURE 2. The immunoregulatory effect of natural polysaccharides in immune cells. Polysaccharides can directly or indirectly activate dendritic cells (DCs), NK, macrophages, T and B lymphocytes, immune cells. They can also produce antibodies, secrete immune factors, and promote immune activation pathways.
In clinical applications, compared with polysaccharides from other sources, plant and fungal polysaccharides have a large amount of medical experience. This review focuses on selecting some representative fungal and plant polysaccharides, discussing their regulatory effects on immune cells, immune molecules, and immune genes at the cellular and molecular levels (Table 1). The potential of polysaccharides as immunomodulators is also discussed.
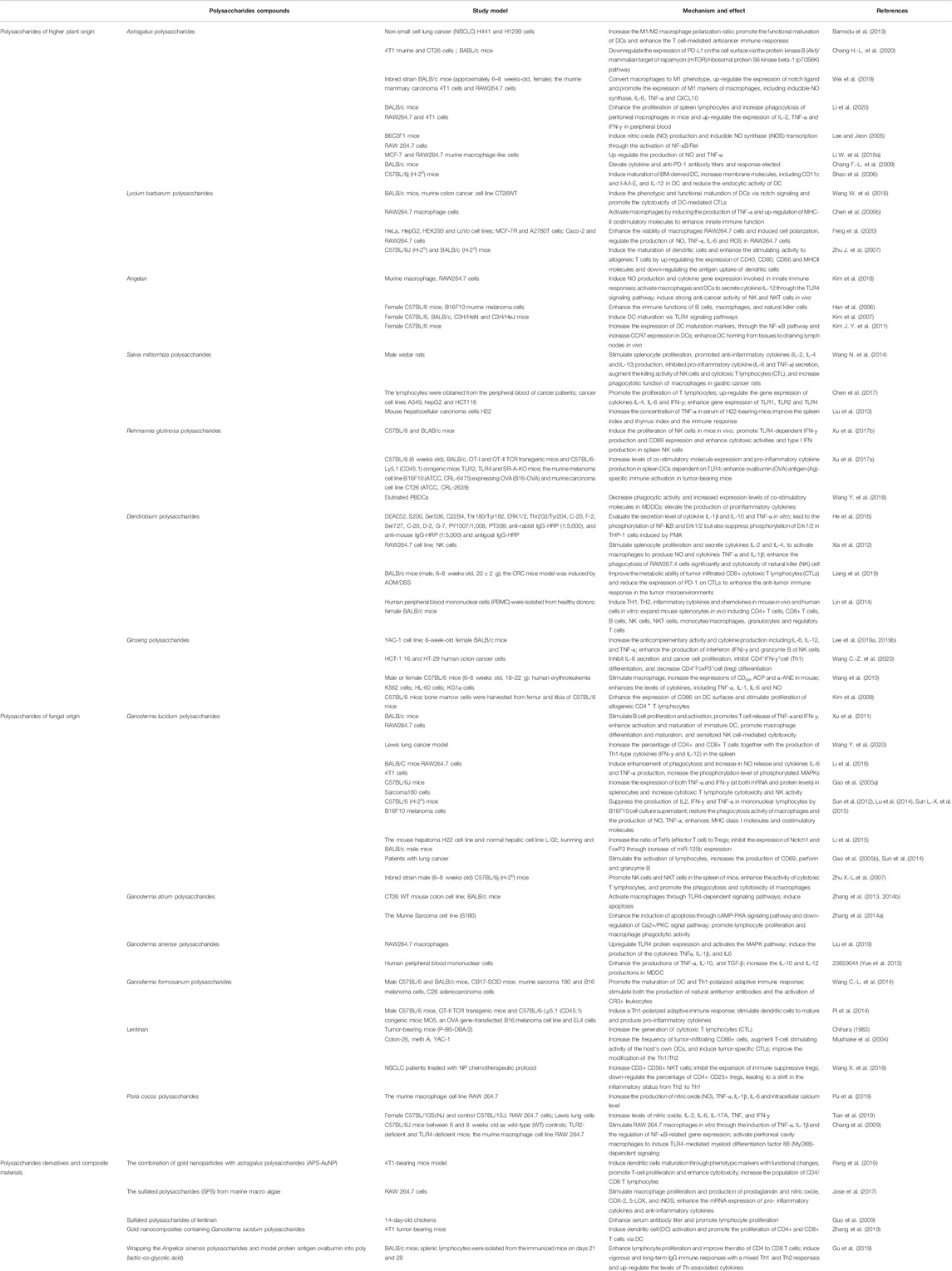
TABLE 1. The immunoregulatory activity of natural polysaccharides and their derivates on immune cells.
Plant Polysaccharides
Astragalus Polysaccharides
Astragali Radix (Huangqi in Chinese), the dried root of Astragalus membranaceus, a commonly used Chinese medicine, has been proven to be effective in enhancing the immune system and treating pathological diseases, even cancer (Chang F.-L. et al., 2020). The astragalus polysaccharide (APS) is the main active component of A. membranaceus extract, and the combined treatment strategy used with other drugs (such as cancer chemotherapy and immunosuppressants drugs) has also been proved to obviously reduce the toxicity of these drugs and enhance the therapeutic effect (Li et al., 2020).
Bamodu et al. (2019) proved that astragalus polysaccharides can significantly increase the polarization rate of M1/M2 macrophages in non-small cell lung cancer (NSCLC) cell lines, regulate the M1/M2 macrophage pool (M1 macrophages produce pro-inflammatory factors and enhance the expression of MHC-II and costimulatory molecules), enhancing the body’s immune response. Using the results in vitro of the clinical samples of the NSCLC cohort it was confirmed that APS can also promote the functional maturation of DCs, enhance the T cell-mediated anti-cancer immune response, and synergistically enhance the therapeutic effect of cisplatin, which proves that it is a substitute in the clinical feasibility of immunotherapy. In addition, further studies by Wei et al. (2019) showed that APS induced increased gene expression of M1 markers (including iNOS, IL-6, TNF-α and CXCL10), and induced macrophages to polarize to the M1 phenotype through the notch signaling pathway. In addition, APS can activate macrophages and release NO and TNF-α by activating TLR4 and NF-κB/Rel, directly preventing the growth of cancer cells (Li W. et al., 2019). Furthermore, research by Lee and Jeon (2005) showed that APS stimulate macrophages to express the iNOS gene by activating NF-κB/Rel.
Shao et al. (2006) confirmed through mice experiments that APS-treated DCs secreted higher levels of IL-12, and showed a more mature state, with long protuberances, while untreated DCs showed shorter protuberances than stimulated DCs. Besides, it was confirmed that APS could up-regulate the membrane expression of MHC and costimulatory molecules on DC. Du et al. (2012) have also proved this point, and believed that APS can further induce CD4+T cells to produce IL-4, IL-2, and IFN-γ, enhance the expression of IFN-γ in CD8+T cells, and induce the strong activity of CTL. These studies also make APS a potential herbal medicine that can be used to increase the anti-tumor effect of DC therapy.
In recent years, with the rise of immune agents, the immunomodulatory function of traditional Chinese medicine on immune checkpoints has also aroused great interest. Previous studies (Chang F.-L. et al., 2020) have shown that APS can significantly inhibit the growth of melanoma cells in transgenic mice and reduce the expression of PD-L1 in tumors, suggesting that the anti-tumor immunosuppressive mechanism of APS may also be related to the regulation of PD-1/PD-L1 signal pathway. A study demonstrated that there was no significant difference in tumor inhibition compared with anti-PD-1 combined with ixabepilone or APS, indicating that APS can maintain an effective dose of anti-PD-1 antibody in vivo, and delay the progression of tumor or tumorigenesis by increasing the activity of T cells, which may improve the synergistic effect (Chang F.-L. et al., 2020). In addition, another research showed that APS enhances the chemotherapy by stimulating host immunity through reducing the PD-L1 expression of tumor surface (Chang H.-L. et al., 2020). It can reduce the expression of pro-inflammatory cytokines, promote the maturation of DCs and it also reduced the M2 macrophage population in patients with lung cancer (Huang et al., 2019). Based on the above data, we have reasons to think that APS can be used in conjunction with ICI to improve the immune cell infiltration and enhance therapeutic effects.
Lycium barbarum Polysaccharides
The Lycium barbarum polysaccharides (LBP) are one of the main active components in the Lycium barbarum fruit (Goji in Chinese). It is a mixture of proteoglycans and polysaccharides and their biological activities are mainly reflected in the antioxidant, anticancer, regulation of immune activity and cytoprotective effects on normal cells (Ho et al., 2009; Wu et al., 2010). Several studies have confirmed that administration of LBP can induce phenotypic and functional maturation of DCs, induce immunogenicity, enhance Th1 response and activate T lymphocytes (Chen et al., 2009a; Wang W. et al., 2018).
Chen et al. (2009b) have shown that LBP can activate macrophages by activating transcription factors NF-κB and AP-1, can induce the production of TNF-α, and up-regulate MHC II molecules. Feng et al. (2020) also observed that LBP significantly enhanced the release of NO, TNF-α, and IL-6 from RAW264.7 macrophages, and significantly promoted the production of ROS. During phagocytosis, it can help eliminate intracellular pathogens (Khatua and Acharya, 2019). Furthermore, LBP induce the maturation of dendritic cells and enhance the stimulating activity to allogeneic T cells (Zhu J. et al., 2007; Tang et al., 2012). In addition, LBP increased the expression of IL-2 and the TNF-α in both mRNA and protein levels of human peripheral blood mononuclear cells (Gan et al., 2003). It is suggested that the combination of LBP and subunit vaccine with weak immunogenicity can improve the level of protective immune response. Furthermore, polysaccharides can not only improve the anti-tumor capabilities, but also avoid tissue damage caused by superfluous production of inflammatory factors (Graff et al., 2009; Liu et al., 2018).
These results suggest that an appropriate amount of LBP not only has the potential to be used as an immunologic adjuvant, but also can prevent immune injury caused by excessive activation of macrophages (Feng et al., 2020). In addition, LBP can be used as a very valuable adjuvant to cancer therapy (such as chemotherapy, radiotherapy, and immunotherapy), reducing the side effects of other types of cancer mediated by apoptosis, and enhancing the antineoplastic effects of other forms of cancer (Tang et al., 2012).
Angelan
Angelan is a type of polysaccharide isolated from the water-soluble part of the Angelica sinensis (Oliv.) Diels (Dang Gui in Chinese) extract. It can activate both the innate and the acquired immune system, enhance the immune function of B cells, macrophages, and natural killer cells, indirectly activate cytotoxic and helper T cells, and directly inhibit cancer cell adhesion to inhibit tumor growth and metastasis (Han et al., 2006; Kim et al., 2018).
Han et al. (2006) found that Angelan can significantly prolong the survival rate and reduce the frequency of lung metastasis in melanoma transplanted mice, and combined with doxorubicin, it can significantly enhance the therapeutic effect and inhibit tumor growth. Moreover, Kim et al. (2007) found that a mature DC treated with Angelan was more effective than an immature DC in inhibiting the growth of B16F10 tumor in the syngeneic murine tumor model. The results showed that Angelan induces DC maturation through the TLR4 signal pathway, suggesting that Angelan may be used in DC immunotherapy. After further study, they found that Angelica polysaccharides up-regulate the expression of MHC-I/II, CD80 and CD86 through the NF-κB pathway, increase the expression of CCR7 in DCs, promote the maturation of DC and the migration of CCL19 in vivo. In the B16-F10 syngeneic tumor model, Angelan can enhance the anti-tumor activity of DC (Kim J. Y. et al., 2011). These data show that Angelan can help overcome the shortcomings of DC-based cancer immunotherapy and enhance the therapeutic effect.
Salvia miltiorrhiza Polysaccharides
The Salvia miltiorrhiza polysaccharide (SMP) is a kind of natural polymer that has antioxidant properties and immunomodulatory activity (Wang N. et al., 2014). SMP has been proved to stimulate the proliferation of T lymphocytes in mice, activate the host’s collective immune response, and play an anti-tumor effect (Chen et al., 2017). Furthermore, there are no obvious side effects. A study (Liu et al., 2013) showed that SMP can significantly increase spleen and thymus index of mice, and improve the ability of immune regulation. Chen et al. (2017) used T lymphocytes and homologous tumor cell lines from patients with lung, liver, and colon cancer to demonstrate that SMP specifically promotes the proliferation of peripheral blood T lymphocytes and enhances their cytotoxicity in tumor patients in a dose-dependent manner. In addition, the gastric cancer rats experiments (Wang N. et al., 2014) showed that SMP significantly stimulates the proliferation of splenocytes, promotes the production of anti-inflammatory cytokines (IL-2, IL-4 and IL-10), inhibits the secretion of pro-inflammatory cytokines (IL-6 and TNF-α), enhances the killing activity of NK cells and cytotoxic T lymphocyte (CTL), as well as promotes the phagocytosis of macrophages in them. Furthermore, SMP significantly increased the total intracellular granzyme B and IFN-γ. These studies suggest that the addition of SMP to the treatment can improve the immune function and help improve the quality of life of patients. Therefore, SMP is also a valuable immunomodulator for the treatment of tumors with immunosuppression.
Rehmannia glutinosa Polysaccharides
The Rehmannia glutinosa polysaccharide (RGP), one of the active components of R. glutinosa Libosch., has strong immunomodulatory properties and anti-tumor activities, and it is involved in maintaining homeostasis in vivo (Zhang et al., 2008). RGP stimulate host immune response by inducing dendritic cell maturation and activating NK cells to induce anticancer effect.
Xu et al. (2017b) have verified the stimulating effect of RGP on NK cells and DCs. RGP can induce the number of NK cells in the peripheral blood and the proliferation of NK cells in the spleen and whole blood of C57BL/6 mice. Furthermore, the RGP treatment also promoted the production of IFN-γ dependent on TLR 4 and the up-regulation of CD69 expression on NK cells in splenic. The killing activity of NK cells treated with RGP against Yac-1 cells was enhanced, and the production of I-IFN finally inhibited the growth of CT26 lung tumor. In addition, tumor-bearing mice experiments showed that RGP could induce the expression of DC costimulatory molecules and the production of pro-inflammatory cytokines in splenic dendritic cells dependent on TLR4, enhance the antigen presentation of DC, and promote the production of IFN-γ by CD4 and CD8T cells. Moreover, the combination of RGP and Ag effectively inhibited the growth of CT26 tumor and B16 melanoma in BLAB/c and C57BL/6 mice. RGP induces the maturation of dendritic cells and activates antigen specific immune response in tumor-bearing mice, which promotes the infiltration of T cells into tumor (Xu et al., 2017a). Subsequently, Wang Y. et al. (2018) confirmed this in human cell experiments in vitro. RGP treatment significantly decreased the phagocytic activity of MDDC and induced the activation of T cells. Besides, RGP can upregulate costimulatory molecules and production of proinflammatory cytokines in both MDDC and PBDC subsets. These data suggest that RGP may play a role as an immunostimulatory molecule and it is expected to be used as an effective adjuvant in the immunotherapy of tumors.
Dendrobium Polysaccharides
Dendrobium polysaccharide, one of the main active components of the traditional Chinese herbal medicine “Dendrobium,” has the effects of antioxidation, immune enhancement, and anti-tumor. Researchers compared different kinds of Dendrobium from different producing areas and found that most of the crude polysaccharides or purified polysaccharides extracted from Dendrobium plants can enhance the function of immune cells, increase the secretion of cytokines, activate macrophages. In particular, the polysaccharides extracted from Dendrobium officinale polysaccharide (DOP) and Dendrobium huoshanense polysaccharide (DHP) have better immunomodulatory activity (Meng et al., 2013; Yue et al., 2020).
Several studies have shown that DOP can stimulate the proliferation of splenocytes, and secrete cytokines IL-2 and IL-4, to induce morphological changes of macrophages, thus promoting the production of cytokines TNF-α, IL-6, IL-1 β, and NO. Further, they enhance the phagocytic activity of RAW267.4 macrophages and significantly enhance the killing activity of NK cells, which may be involved in the early immune response (Xia et al., 2012; He et al., 2016; Yue et al., 2020). In addition, it was observed that DOP could inhibit the growth of tumor cells in mice with sarcoma 180 cells, which can be related to its mechanism (Yue et al., 2020). Liang et al. (2019) found in the colorectal cancer model that DOP can enhance the metabolic ability of tumor infiltrating CD8+ CTL, reduce the loss of mitochondria the expression of PD-1 on CTL, thus enhancing the anti-tumor immune response of TME.
DHP can induce the production of Th1, Th2, inflammatory cytokines, and chemokines in mouse and human cells in vivo and in vitro. Additionally, it expanded mouse spleen cells in vivo, including CD4+T cells, CD8+T cells, B cells, NK cells, monocytes/macrophages, granulocytes and Tregs, and had strong anti-inflammatory ability (Lin et al., 2014). These data show that DHP can be used as an immune synergistic agent to exert its potential in immunotherapy in the future.
Ginseng Polysaccharides
According to the different extraction parts, we divided the ginseng polysaccharides into polysaccharides extracted from the roots of Panax ginseng (GSP) and polysaccharides extracted from the fruits of Panax ginseng (GBP). Some studies have shown that the berry of Panax ginseng has a much stronger pharmacological activity than its root (Lee et al., 2019a; Lee et al., 2019b).
The anticancer effect of GBP isolated from ginseng berries may be due to the increased activity of innate immune cells, such as macrophages and NK cells (Lee et al., 2019a). GBP can promote the production of IL-6, IL-12, and TNF-α as well as increase the expression of mRNA in mouse peritoneal macrophages. Furthermore, it significantly increases the killing activity of YAC-1 tumor cells to NK cells and the production of granzyme B. It can also inhibit the lung metastatic activity of B16-BL6 melanoma cells (Lee et al., 2019a; Lee et al., 2019b). In addition, GBP can significantly inhibit the differentiation of Th1 cells and the differentiation of Treg cells (hindering the body’s immune response to malignant tumors), and the differentiation of Treg cells Down-regulation may help the anti-cancer potential of GBP and reshape the tumor microenvironment (Wang C.-Z. et al., 2020).
However, ginseng polysaccharides (GSP) mostly regulate immunity through macrophages and dendritic cells. Wang et al. (2010) showed that mouse peritoneal macrophages (PMs) treated with GSP could induce tumor killing activity, enhance phagocytic activity and the expression of CD68 and show the ability to produce cytokines and cytotoxic molecules. Kim et al. (2009) proved that GSP can induce the maturation of mouse bone marrow-derived DC, enhance the expression of CD86 on the surface of DCs, and significantly promote the proliferation of allogeneic CD4+T lymphocytes.
Taken together, these results suggest that ginseng polysaccharides have strong anti-tumor activities by stimulating macrophages and they may act as an immunomodulator against diseases such as cancer.
Fungal Polysaccharides
Ganoderma Polysaccharides
The main active components of Ganoderma are polysaccharides and triterpenoids, among which the polysaccharide fraction is responsible for antitumor and immunomodulatory effects. The main mechanism of anti-tumor activity of Ganoderma polysaccharides is stimulating the host’s defense response, activating lymphocytes to enhance the immunogenicity of tumor cells, rather than killing tumor cells directly. However, Ganoderma plants are rich in species and widely studied. Therefore, we will divide it into the following 4 parts to elaborate: Ganoderma lucidum polysaccharide (GLP), Ganoderma atrum polysaccharide (PSG-1), Ganoderma sinense polysaccharide (GSP-2) and Ganoderma formosanum polysaccharides (PS-F2).
GLP, a polysaccharide extracted from Ganoderma lucidum, can regulate the function of a variety of immune cells, including macrophages, dendritic cells, NK cells, T cells, and B cells. It can stimulate the proliferation and activation of B cells, promote the release of TNF-γ and IFN-α from T cells, enhance the activation and maturation of immature DCs, increase the phosphorylation level of MAPK and promote the differentiation and maturation of macrophages, and sensitize NK cell-mediated cytotoxicity (Xu et al., 2011). GLPs can effectively promote the activation and maturation of immature DCs, and they are more inclined to Th1 reaction (Zeng et al., 2019).
We further summarized the regulatory role of GLP in tumor microenvironment in different cancers. Li et al. (2018) found that in 4T1 breast cancer BALB/c mice, GLP can significantly inhibit tumor growth and induce macrophages to inhibit the survival and migration of cancer cells in vitro. For lung cancer, GLP antagonized the immunosuppression of lung cancer tumor cells and stimulated the activation of lymphocytes in lung cancer patients to improve immune function (Gao et al., 2005b; Sun et al., 2014). A research on lewis lung cancer mouse (Wang Y. et al., 2020) further concluded that GLP can inhibit tumor growth and regulate the differentiation and inhibition of myeloid-derived suppressor cells (MDSCs) to enhance antitumor immune response. After GLP treatment, there were fewer MDSCs in both spleen and tumor tissues and the production of Th1-type cytokines together with the percentage of CD4+T and CD8+T cells was increased in the spleen of mice, indicating a better immune infiltration microenvironment. For melanoma, several studies (Sun et al., 2012; Lu et al., 2014; Sun L.-X. et al., 2015) have shown that GLP can completely or partially improve the inhibitory effect of B16F10 cells on the production of IL-2, IFN-γ and TNF-α by mononuclear lymphocytes in the culture supernatant of B16F10 cells. In addition, it can also promote the proliferation and activation of lymphocytes induced by melanoma cells, increasing the production of CD69 and IFN-γ, and enhancing the expression of MHC-I and costimulatory molecules to induce more effective immune cells mediated cytotoxicity and control tumor progression. Then, GLP can inhibit the accumulation of Treg and inhibit the growth of liver cancer by inducing miR-125 in hepatoma-bearing mice (Li et al., 2015).
In addition, GLP can also antagonize the immunosuppressive effect caused by drugs by restoring the function of immune effector cells (including macrophages, NK cells and NKT cells) (Zhu X.-L. et al., 2007). Therefore, when combined with chemotherapy or other therapies, GLPs can alleviate treatment-induced immunosuppression, enhance the anti-cancer effect of them and improve the health status (Zhu X.-L. et al., 2007; Zeng et al., 2019).
PSG-1, a polysaccharide extracted from Ganoderma atrum, may against drug-induced immunosuppression by increasing levels of TNF-α and IL-2 and promoting immune effort cell survival (Li et al., 2017). In CT26-bearing mice, PSG-1 induced apoptosis by enhancing the antitumor immune response and activate macrophages through TLR4-dependent signaling pathways to inhibit tumor growth (Zhang et al., 2013; Zhang et al., 2014b). In another studies, PSG-1 can increase cAMP and PKA activities and promote lymphocyte proliferation and macrophage phagocytic activity to activating host immune function in tumor-bearing mice (Zhang et al., 2014a).
GSP-2, a polysaccharide extracted from Ganoderma sinense, which specific induced the overexpression of TLR4 and activated the MAPK pathway, promotes cytokine secretion and immune modulation in macrophages (Liu et al., 2019). It can stimulate the proliferation of PBMC and increase the secretion of TNF-α, IL-10 and transforming growth factor-β and enhance the ability of monocyte-derived DCs to produce IL10 and IL-12(Yue et al., 2013). These findings suggested that GSP-2 could be used as an adjuvant in immunosuppressed tumor patients.
PS-F2, a polysaccharide extracted from Ganoderma formosanum, stimulates tumor-specific cellular and humoral immune responses by promoting the maturation of DC and Th1-polarized adaptive immune response (Wang C.-L. et al., 2014). At the same time, the adjuvant function of PS-F2 was also investigated in another experiment, which demonstrated that PS-F2 stimulated dendritic cells to mature and produce pro-inflammatory cytokines in vitro. Their studies also confirmed that PS-F2, when used as an adjuvant, can play a role in anti-tumor vaccines by inducing a Th-1polarized adaptive immune response (Pi et al., 2014).
In short, polysaccharides extracted from Ganoderma have almost no adverse effects on the human body, and other immune enhancers rarely have this advantage. They can regulate the activities of neutrophils, NK cells, NKT cells, dendritic cells, and the complement system, enhancing the immune response and anti-tumor activity (Gao et al., 2005a). In addition, the ability to reverse the immunosuppressive microenvironment caused by multiple drugs also makes it a potential choice of adjuvant for tumor immunotherapy.
Lentinan
Lentinan (LNT) is a compound extracted from the edible mushroom Lentinus edodes, which has a direct anti-tumor effect and a type of immunomodulatory activity. Its active component is β-(1→3)-D-glucan. It can activate nonspecific cytotoxicity in vivo and enhance cytotoxic T cell activity, NK cell activity, and humoral immune response mediated by helper T cells, induce Th1 polarization, and improve the balance between Th1 and Th2 (Chihara, 1983; Ina et al., 2013). It is reported that LNT has been used as a biological response regulator for cancer chemotherapy, being able of improving the quality of life and prolong the survival time of patients (Mushiake et al., 2004).
It has been previously proved that CSF increases after the application of lentinan in vivo, which may act on immunomodulatory macrophages, resulting in an increase in the production of IL-1, thus activating the function of helper T cells. In the tumor-bearing environment, most of the immune cells are in a state of inhibition, while lentinan can restore the immunosuppression of allogeneic reactive killer cells to the normal level, and the killer cells produced by spleen cells can also recover from zero to about 40%. Lentinan is the first adjuvant proved to enhance cytotoxic T lymphocyte response in vivo (Chihara, 1983). In an animal experiment (Mushiake et al., 2004), lentinan was proved to increase the number of CD86 + cells infiltrated by tumor to activate DC function. In addition, lentinan can stimulate the production of killer T cells and NK cells, restore the ratio of killer/suppressor T cells, and up-regulate the killing effect on tumor cells mediated by NK cells (Ina et al., 2013).
The combination of lentinan with leukocytes can enhance the cytotoxicity mediated by ADCC and complement by activating CR3, induce the production of IL-12 and enhance the anti-tumor effect of mAbs. A study in vivo has clearly shown that lentinan combined with trastuzumab can significantly inhibit tumor growth (Cheung et al., 2002). Many studies have shown that lentinan, as a non-specific BRM, has an effect on the immune regulation of various cancers (including gastrointestinal cancer, breast cancer, lung cancer): the survival rate and quality of life have been significantly improved during the one-year follow-up, and the short-term evaluation of the objective response and disease progression has also been significantly improved. Moreover, lentinan was associated with a lower incidence of adverse events than chemotherapy alone (Ina et al., 2013; Wang et al., 2017; Zhang et al., 2018). In vitro, the antitumor effect of LNT was also significantly enhanced when combined with monoclonal antibody and gemcitabine (Hong et al., 2004; Sun M. et al., 2015).
Furthermore, Wang X. et al. (2018) showed that in patients with NSCLC, lentinan significantly increased the number of CD3+CD56+NKT cells, up-regulated CD4+ and CD8+ cell subsets, increased the levels of IFN-γ, TNF-α, and IL-12, and decreased the levels of IL-10 and TGF-β1 in patients with NSCLC. It is confirmed that lentinan can not only enhance the cellular immune function and promote anti-tumor benefit through combined immunotherapy, but also inhibit the expansion of immunosuppressive Tregs. Lentinan-based chemical immunotherapy is a promising anti-tumor strategy by promoting the proliferation of cytotoxic T cells and then increasing the inflammatory chemokines/cytokines. At the same time, the proportion of CD4+CD25+Tregs in NSCLC patients treated with lentinan was down-regulated, resulting in the transformation of the inflammatory state from Th2 to Th1. In view of these, a synergistic effect of immunotherapy with lentinan and monoclonal antibody can be expected.
Poria cocos Polysaccharides
The Poria cocos polysaccharides (PCPs), the main bioactive components extracted from the sclerotia of Poria cocos (Schw.) Wolf, are composed of ribose, arabinose, xylose, mannose, glucose, and galactose. Studies have shown that PCPs have anti-tumor, immunomodulatory, antioxidant, and mitogenic effects (Li X. et al., 2019; Pu et al., 2019).
PCP can enhance the innate immunity, improve the proportion of lymphocytes, enhance the phagocytosis of macrophages by activating varieties of immune cells, and regulate the specific immunity by activating T cells. Tian et al. (2019) found that PCPs can directly interact with the surface TLR of macrophages, induce the secretion of NO, IL-2, IL-6, TNF, IFN-γ, and IL-17A, increase the organ immune activity index, play an immunomodulatory role, and reduce the tumor burden. In different animal models, PCPs combined with chemotherapeutic drugs, such as 5-FU (Li X. et al., 2019), can further improve the therapeutic effects and reduce the adverse reactions associated with chemotherapeutic drugs. Chang et al. (2009) found that PCPs can stimulate RAW264.7 macrophages in vitro by inducing TNF-α and IL-1β as well as by regulating the expression of NF-κB-related genes. Therefore, PCP can be considered as a new and potential immune stimulant.
Polysaccharide Derivatives
Polysaccharide has almost no cytotoxic effect on human body, and its safety is extremely high, but it also has significant defects: rapid elimination, short half-life and lack of targeting in vivo, which may hinder its sustained pharmacological activity and prevent it from exerting its due effect (Pang et al., 2019). Many researches have shown that polysaccharides with functional groups have more immunostimulatory activity than those without functional groups (Ferreira et al., 2015). The structural modification of polysaccharides can significantly improve immune activity. In the aspect of chemical modifications, we screened sulfation, carboxymethylation, acetylation and phosphorylation as representatives to review the effects (Chen and Huang, 2018).
Sulfated polysaccharides can enhance the phagocytic function of macrophages, stimulate macrophages to secrete NO, IL-6, and other interleukin, and enhance the ability of immune regulation (Kim J.-K. et al., 2011; Jose et al., 2017). The sulfation treatment could significantly improve the immune enhancement activity of seaweed polysaccharides (Jose et al., 2017) and lentinan (Guo et al., 2009). In addition, the sulfated function groups of polysaccharides can enhance cytotoxicity, which are related to the substitution position of its groups: substitution on C-6 > C-4> C-2 of galactose > C-2 of anhydrogalactose (Liang et al., 2014). Furthermore, the Carboxylation and acetylation groups can enhance the water solubility of polysaccharides (Xu et al., 2019). When the degree of substitution of carboxymethyl is 0.5–0.6, carboxymethylation can significantly improve the maturation induction ability of DCs and gain an immunomodulatory effect (Huang G. et al., 2016). The modification of the phosphate group will significantly enhance the expression of B cells and DCs on the surface of CD86 and CD69, and promote the production of IL-10, enhancing the immunosuppressive activity of polysaccharides (Chen and Huang, 2018).
Natural Polysaccharides Composite Materials
Beyond the modification of derivatives, the rise and application of new materials also provide a wide range of research directions for the modification of natural polysaccharides. The addition of nanocomposites may enhance their pharmacological activities (Pang et al., 2019). The nano-drug delivery system has been widely used in the targeted drug delivery system, and it shows advantages in tumor therapy (Chen and Huang, 2018; Yu et al., 2018).
Compared with other nanoparticles (NPs), gold nanoparticles (AuNPs) have a high surface area-to-volume ratio, dispersion, stability, and biocompatibility, therefore, it is one of the most promising ways for the application of nanotechnology (Cabuzu et al., 2015; Okoampah et al., 2020). The combination of gold nanoparticles with astragalus polysaccharides (APS-AuNP) can give astragalus polysaccharides longer peripheral circulation and more aggregation in immune organs or tumors, resulting in stronger regional and systemic anti-tumor effects. It can not only induce phenotypic maturation and functional changes of DCs, but also further promote T cell proliferation and cytotoxicity. Compared with free APS and other AuNP, APS-AuNP has a stronger immunomodulatory effect on DCs. Further, animal experiments have confirmed that APS-AuNP has excellent efficacy in inhibiting primary tumor growth and reducing lung metastatic nodules, and it has a significant ability to assist tumor microenvironment reconstruction and systematic anti-tumor immune response (Pang et al., 2019). Besides APS-AuNP, Zhang et al. (2019) have also shown that gold nanocomposites containing Ganoderma lucidum polysaccharides (GLP-Au) can effectively induce the activation of DCs, increase the expression of CD80/CD86/CD40/MHCII, and promote the proliferation of CD4+ and CD8+T cells in splenocytes. The combination of GLP-Au and doxorubicin could strongly inhibit the tumor growth and lung metastasis of 4T1, restore the weight loss caused by doxorubicin, and increase the percentage of memory T cells in CD4+/CD44+.
Moreover, there is a vaccine delivery system based on NPs, which can control the release of antigens and promote the immune response to cancer. A novel NPs-based vaccine delivery system (ASP-PLGA/OVA) can be prepared by wrapping the immunopotentiator Angelica sinensis polysaccharide (ASP) and model protein antigen ovalbumin (OVA) into poly (lactic-co-glycolic acid; PLGA). Mice treated with ASP-PLGA/OVA nanoparticles can promote lymphocyte proliferation and increase the ratio of CD4/CD8T cells, thus inducing a strong cellular immune response. ASP-PLGA/OVA nanoparticles can induce a strong and persistent immune response to Th1/Th2 mixed response and up-regulate the level of Th-related cytokines (Gu et al., 2019).
The above studies show that the new material of polysaccharides can make up for the shortcomings of natural polysaccharides to a certain extent, stimulate strong and sustained antibody responses through a variety of ways, and induce cellular immune responses, making them an effective and safe vaccine delivery and adjuvant system to improve cancer immunotherapy.
Summary and Perspectives
This review focuses on the anti-tumor activity obtained by polysaccharide compounds in stimulating or activating macrophages, dendritic cells, and NK cells to optimize the non-specific immunity, thereby regulating T cell function and, ultimately, enhancing the specific immunity. Most natural products have multiple “targets” that may affect different kinds of signaling pathways. Polysaccharide compounds can extensively regulate immune mechanisms through various pathways, such as TLR4, NF-κB, and notch pathways, which act on DCs, macrophages, and NK cells to achieve the balance and improvement of the immune microenvironment. The infiltration of activated immune effector cells in tumors is significantly related to the improvement of the prognosis of tumor diseases. Recent studies have found that dendritic cells and other immune cells are a key part of immunotherapy. The PD-L1 blockade can activate DC function, thereby generating a powerful anti-cancer T cell immunity (Mayoux et al., 2020), and that natural polysaccharides can also make efforts on it. A variety of immune cells stimulate the adaptive immunity, which increases the theoretical feasibility for subsequent studies of polysaccharides as immune adjuvants.
The components of plant polysaccharides and fungal polysaccharides have a lot in common, which makes them may have similar biological activities. However, there are great differences in structure between them, which determine the tendency of their biological functions to a certain extent. Plant polysaccharides are mainly pectin polysaccharides, whose immune-enhancing effects are mostly considered as 1→3, 6-branched galactose residues, the rhamnoides galacturonic acid and other structures and functional groups. While fungal polysaccharides are represented by β-glucan and its derivatives, they seem like to have better anti-tumor abilities and stronger immunomodulatory effects (Jiang et al., 2010). However, technical limitations such as separation and purification of polysaccharides prevent further structural and functional linkages, suggesting that we will need to link more common structures of different polysaccharides with their biological activities in the future. Plant and fungal polysaccharides had similar effects in activating macrophages and promoting their phagocytosis and cytokine release. However, for dendritic cells, plant polysaccharides, such as LBP (Zhu J. et al., 2007), have strong abilities to induce maturation, and are more likely to improve the tumor immune response. Regarding NK cells, generally speaking, fungal polysaccharides mostly promote their cytotoxicity and enhance anti-tumor activity, while plant polysaccharides promote the release of cytokines, such as interferon-γ and granzyme. In addition, different polysaccharides have preferences for the therapeutic effect of different cancers. Fungal polysaccharides may be more influential in hormone/endocrine-related tumors. Ganoderma lucidum polysaccharides have strong killing effect on liver cancer and colon cancer cell lines, but have no effect on osteosarcoma cells; compared with liver cancer, LNT has a better effect on breast cancer (Pang et al., 2018). Therefore, when we are faced with complex polysaccharides, we not only need to understand its basic structure, but also should compare and analyze it from multiple angles, combined with laboratory results, to comprehensively explore its possible ways of action.
Not only the extraction sources of polysaccharides, the structure, molecular weight of different polysaccharides, main chain and branched chain, functional group modification, spatial conformation and so on are also categories that we need to consider. Polysaccharides with special structure and functional group modification may have higher immunostimulatory activity. The polysaccharides with β-(1–3) bond and β-(1–6) branched chain on the main chain have anti-tumor activity, and their anti-tumor function may be obtained by regulating host immunity, such as (β1→4)-, (β1→3)-, and (β1→6)-D-glucans or (α1→3)-, (α1→4)-, and (α1→6)-d-glucans have better immune enhancement (Ferreira et al., 2015). In addition, the functional group modification mentioned in this review is also one of the leading factors in the biological activity of polysaccharides. we considered that triple-helixconformation can promote the release of TNF-α by macrophages and monocytes and enhance their immunostimulatory activity, and some experimental studies have shown that when breaking the triple helix conformation, the inhibitory activity of polysaccharides on sarcoma growth in mice is decreased. Single helix and polysaccharides without helix but with other residues may have immunostimulatory activity. Therefore, there is still a partially shared structure with the same biological activity between polysaccharides.
Although laboratory research has made some progress regarding the use of natural polysaccharides as immune adjuvants, there still are many challenges in the process of transferring them into clinical applications. The main problems, such as low oral availability and difficulty in targeting organs and tissues, need to be solved urgently. In response to these difficulties, researchers have made some remarks. First of all, different ways of administration can be chosen to improve their efficacy according to their biological characteristics. In the second place, different polysaccharides have complementary advantages, and two or more polysaccharides can be selected for synthesis [the combination of GLP and Polyporus umbellatus polysaccharides can enhance the innate immune function of mice (Gao et al., 2005a)] to improve their immune efficacy. Thirdly, combined with new materials, such as gold nanoparticles, polysaccharides can improve the bioavailability. Compared with free GLP, GLP-Au-induced CD80, CD86, CD40, and MHCII increased in a dose-dependent manner, and had a better immunostimulatory effect on DC maturation, with a statistically significant difference (Zhang et al., 2019). In addition, combining polysaccharides with liposomes (Huang Y. et al., 2016), or changing their properties to increase acetyl groups (Yue et al., 2020), or combining with proteins to form polypeptide compounds (Chen et al., 2009a) can improve their medicinal efficiency to varying degrees. Last but not least, it’s of vital for polysaccharides with the presence of triple-helix conformation of (β1→3)-D-glucans, function groups, branching degree of pectic polysaccharides, and Type II arabinogalactans to enhance immunostimulatory activity. We found that most plant polysaccharides have not been widely used in clinical preparations, while fungal polysaccharides are relatively widely used. Although the fundamental mechanism has not been fully explained, there are great differences in their clinical applications. We can reasonably infer that the dissimilarity may be due to the different molecular structure and bioavailability of their polysaccharides. In the future, we can organize the chain and conformation to form a database about polysaccharides, integrate its structural heterogeneity and function as much as possible, make it easy to obtain and analyze, and fully understand its biological activity.
In order to maximize the strengths and avoid weaknesses as much as possible, while fully exerting their potential, the efficiency of action needs to be improved. In short, the coordinated efforts of researchers and clinicians are essential to improve the drug defects of natural substances themselves. And we should also conduct further clinical trials to better develop the potential of natural phytochemicals for drug development.
Author Contributions
The research project was designed by YL, XW, CS and JW; YL collected the literature, drew structures; YL and XW wrote the manuscript and checked the Tables and Figures as well as grammar of manuscript; XM and CL revised the manuscript. CS and JW participated in and helped draft the manuscript. All authors have read and approved the final version of the manuscript.
Funding
National Natural Science Foundation of China (Grant Number 81973677) CS.
Conflict of Interest
The authors declare that the research was conducted in the absence of any commercial or financial relationships that could be construed as a potential conflict of interest.
Supplementary Material
The Supplementary Material for this article can be found online at: https://www.frontiersin.org/articles/10.3389/fphar.2021.621813/full#supplementary-material.
Glossary
ADCC antibody-dependent cell-mediated cytotoxicity
ADCP antibody-dependent cellular phagocytosis
APC antigen presenting cell
APS the Astragalus polysaccharide
AuNPs gold nanoparticles
BRM biological response regulators
cDC conventional DC
CR3 complement receptor 3
CTL cytotoxic T lymphocyte
CXCL CXC chemokine ligand
DC dendritic cell
DHP Dendrobium huoshanense polysaccharides
DOP Dendrobium officinale polysaccharides
ERK1/2 extracellular regulated protein kinases 1/2
GLP Ganoderma lucidum polysaccharides
GM-CSF granulocyte-macrophage colony-stimulating factor
GSP ginseng polysaccharides
GSP-2 Ganoderma sinense polysaccharide
ICI immune checkpoint inhibitor
IFN-γ ; interferon-γ
IL-12 interleukin-12
iNOS inducible nitric oxide synthase
LBP Lycium barbarum polysaccharides
LPS lipopolysaccharide
LNT lentinan
MAPK mitogen-activated protein kinase
MDSC myeloid-derived suppressor cell
MDDC bone marrow derived dendritic cell
MHC II major histocompatibility complex II
M1 M1 macrophages
M2 M2 macrophages
NKs natural killer cells
NO nitric oxide
NPs nanoparticles
NSCLC non-small cell lung cancer
PBDC peripheral blood dendritic cell
pDC DC-specific precursor
PCPs Poria cocos polysaccharides
PMs peritoneal macrophages
PS-F2 Ganoderma formosanum polysaccharides
PSG-1 Ganoderma atrum polysaccharide
RGP Rehmannia glutinosa polysaccharide
ROS reactive oxygen species
SMP Salvia miltiorrhiza polysaccharide
SR scavenger receptor
TAM tumor-associated macrophages
Th1 T helper 1 cell
Th2 T helper 2 cell
TLR4 toll-like receptors 4
TME tumor microenvironment
TNF-α tumor necrosis factor-α
Treg regulatory T cell
References
Anfossi, N., André, P., Guia, S., Falk, C. S., Roetynck, S., Stewart, C. A., et al. (2006). Human NK cell education by inhibitory receptors for MHC class I. Immunity 25 (2), 331–342. doi:10.1016/j.immuni.2006.06.013
Bamodu, O. A., Kuo, K.-T., Wang, C.-H., Huang, W.-C., Wu, A. T. H., Tsai, J.-T., et al. (2019). Astragalus polysaccharides (PG2) enhances the M1 polarization of macrophages, functional maturation of dendritic cells, and T cell-mediated anticancer immune responses in patients with lung cancer. Nutrients 11 (10), 2264. doi:10.3390/nu11102264
Bonnardel, J., and Guilliams, M. (2018). Developmental control of macrophage function. Curr. Opin. Immunol. 50, 64–74. doi:10.1016/j.coi.2017.12.001
Cabuzu, D., Cirja, A., Puiu, R., and Grumezescu, A. (2015). Biomedical applications of gold nanoparticles. Ctmc 15 (16), 1605–1613. doi:10.2174/1568026615666150414144750
Chan, C. W., and Housseau, F. (2008). The 'kiss of death' by dendritic cells to cancer cells. Cell Death Differ 15 (1), 58–69. doi:10.1038/sj.cdd.4402235
Chang, F.-L., Tsai, K.-C., Lin, T.-Y., Yang, T.-W., Lo, Y.-N., Chen, W.-C., et al. (2020). Astragalus membranaceus-derived anti-programmed death-1 monoclonal antibodies with immunomodulatory therapeutic effects against tumors. Biomed. Res. Int. 2020, 1. doi:10.1155/2020/3415471
Chang, H.-H., Yeh, C.-H., and Sheu, F. (2009). A novel immunomodulatory protein from Poria cocos induces Toll-like receptor 4-dependent activation within mouse peritoneal macrophages. J. Agric. Food Chem. 57 (14), 6129–6139. doi:10.1021/jf9011399
Chang H.-L., H.-L., Kuo, Y.-H., Wu, L.-H., Chang, C.-M., Cheng, K.-J., Tyan, Y.-C., et al. (2020). The extracts of Astragalus membranaceus overcome tumor immune tolerance by inhibition of tumor programmed cell death protein ligand-1 expression. Int. J. Med. Sci. 17 (7), 939–945. doi:10.7150/ijms.42978
Chen, F., and Huang, G. (2018). Preparation and immunological activity of polysaccharides and their derivatives. Int. J. Biol. Macromolecules 112, 211–216. doi:10.1016/j.ijbiomac.2018.01.169
Chen, Y., Li, H., Li, M., Niu, S., Wang, J., Shao, H., et al. (2017). Salvia miltiorrhiza polysaccharide activates T Lymphocytes of cancer patients through activation of TLRs mediated -MAPK and -NF-κB signaling pathways. J. Ethnopharmacology 200, 165–173. doi:10.1016/j.jep.2017.02.029
Chen, Z., Lu, J., Srinivasan, N., Tan, B. K. H., and Chan, S. H. (2009a). Polysaccharide-protein complex fromLycium barbarumL. Is a novel stimulus of dendritic cell immunogenicity. J. Immunol. 182 (6), 3503–3509. doi:10.4049/jimmunol.0802567
Chen, Z., Soo, M. Y., Srinivasan, N., Tan, B. K. H., and Chan, S. H. (2009b). Activation of macrophages by polysaccharide-protein complex fromLycium barbarumL. Phytother. Res. 23 (8), 1116–1122. doi:10.1002/ptr.2757
Cheung, N.-K. V., Modak, S., Vickers, A., and Knuckles, B. (2002). Orally administered β-glucans enhance anti-tumor effects of monoclonal antibodies. Cancer Immunol. Immunother. 51 (10), 557–564. doi:10.1007/s00262-002-0321-3
Chihara, G. (1983). Preclinical evaluation of lentinan in animal models. Adv. Exp. Med. Biol. 166, 189–197. doi:10.1007/978-1-4757-1410-4_16
Chiossone, L., Dumas, P.-Y., Vienne, M., and Vivier, E. (2018). Natural killer cells and other innate lymphoid cells in cancer. Nat. Rev. Immunol. 18 (11), 671–688. doi:10.1038/s41577-018-0061-z
Del Cornò, M., Gessani, S., and Conti, L. (2020). Shaping the innate immune response by dietary glucans: any role in the control of cancer?. Cancers 12 (1), 155. doi:10.3390/cancers12010155
Deng, L. J., Qi, M., Li, N., Lei, Y. H., Zhang, D. M., and Chen, J. X. (2020). Natural products and their derivatives: promising modulators of tumor immunotherapy. J. Leukoc. Biol. 108 (2), 493–508. doi:10.1002/JLB.3MR0320-444R
Du, X., Zhao, B., Li, J., Cao, X., Diao, M., Feng, H., et al. (2012). Astragalus polysaccharides enhance immune responses of HBV DNA vaccination via promoting the dendritic cell maturation and suppressing Treg frequency in mice. Int. Immunopharmacology 14 (4), 463–470. doi:10.1016/j.intimp.2012.09.006
Duan, Q., Zhang, H., Zheng, J., and Zhang, L. (2020). Turning cold into hot: firing up the tumor microenvironment. Trends Cancer 6 (7), 605–618. doi:10.1016/j.trecan.2020.02.022
Feng, L., Xiao, X., Liu, J., Wang, J., Zhang, N., Bing, T., et al. (2020). Immunomodulatory effects of Lycium barbarum polysaccharide extract and its uptake behaviors at the cellular level. Molecules 25 (6), 1351. doi:10.3390/molecules25061351
Ferreira, S. S., Passos, C. P., Madureira, P., Vilanova, M., and Coimbra, M. A. (2015). Structure-function relationships of immunostimulatory polysaccharides: a review. Carbohydr. Polym. 132, 378–396. doi:10.1016/j.carbpol.2015.05.079
Fridman, W. H., Zitvogel, L., Sautès–Fridman, C., and Kroemer, G. (2017). The immune contexture in cancer prognosis and treatment. Nat. Rev. Clin. Oncol. 14 (12), 717–734. doi:10.1038/nrclinonc.2017.101
Gan, L., Zhang, S.-H., Liu, Q., and Xu, H.-B. (2003). A polysaccharide-protein complex from Lycium barbarum upregulates cytokine expression in human peripheral blood mononuclear cells. Eur. J. Pharmacol. 471 (3), 217–222. doi:10.1016/s0014-2999(03)01827-2
Gao, Y., Gao, H., Chan, E., Tang, W., Xu, A., Yang, H., et al. (2005a). Antitumor activity and underlying mechanisms of ganopoly, the refined polysaccharides extracted from Ganoderma lucidum, in mice. Limm 34 (2), 171–198. doi:10.1081/imm-5581310.1081/imm-200055813
Gao, Y., Tang, W., Dai, X., Gao, H., Chen, G., Ye, J., et al. (2005b). Effects of water-SolubleGanoderma lucidumPolysaccharides on the immune functions of patients with advanced lung cancer. J. Med. Food 8 (2), 159–168. doi:10.1089/jmf.2005.8.159
Garris, C. S., and Luke, J. J. (2020). Dendritic cells, the T-cell-inflamed tumor microenvironment, and immunotherapy treatment response. Clin. Cancer Res. 26 (15), 3901–3907. doi:10.1158/1078-0432.CCR-19-1321
Ginefra, P., Lorusso, G., and Vannini, N. (2020). Innate immune cells and their contribution to T-cell-based immunotherapy. Ijms 21 (12), 4441. doi:10.3390/ijms21124441
Gordon, S., and Plüddemann, A. (2017). Tissue macrophages: heterogeneity and functions. BMC Biol. 15 (1), 53. doi:10.1186/s12915-017-0392-4
Graff, J. C., Kimmel, E. M., Freedman, B., Schepetkin, I. A., Holderness, J., Quinn, M. T., et al. (2009). Polysaccharides derived from Yamoa (Funtumia elastica) prime γδ T cells in vitro and enhance innate immune responses in vivo. Int. Immunopharmacology 9 (11), 1313–1322. doi:10.1016/j.intimp.2009.07.015
Gu, P., Liu, Z., Sun, Y., Ou, N., Hu, Y., Liu, J., et al. (2019). Angelica sinensis polysaccharide encapsulated into PLGA nanoparticles as a vaccine delivery and adjuvant system for ovalbumin to promote immune responses. Int. J. Pharmaceutics 554, 72–80. doi:10.1016/j.ijpharm.2018.11.008
Guo, Z., Hu, Y., Wang, D., Ma, X., Zhao, X., Zhao, B., et al. (2009). Sulfated modification can enhance the adjuvanticity of lentinan and improve the immune effect of ND vaccine. Vaccine 27 (5), 660–665. doi:10.1016/j.vaccine.2008.11.038
Han, S., Lee, C., Kang, M., Yoon, Y., Kang, J., Lee, K., et al. (2006). Pectic polysaccharide isolated from Angelica gigas Nakai inhibits melanoma cell metastasis and growth by directly preventing cell adhesion and activating host immune functions. Cancer Lett. 243 (2), 264–273. doi:10.1016/j.canlet.2005.11.040
Haslam, A., and Prasad, V. (2019). Estimation of the percentage of US patients with cancer who are eligible for and respond to checkpoint inhibitor immunotherapy drugs. JAMA Netw. Open 2 (5), e192535. doi:10.1001/jamanetworkopen.2019.2535
He, T.-B., Huang, Y.-P., Yang, L., Liu, T.-T., Gong, W.-Y., Wang, X.-J., et al. (2016). Structural characterization and immunomodulating activity of polysaccharide from Dendrobium officinale. Int. J. Biol. Macromolecules 83, 34–41. doi:10.1016/j.ijbiomac.2015.11.038
Ho, Y.-S., Yu, M.-S., Yik, S.-Y., So, K.-F., Yuen, W.-H., Chang, R. C.-C., et al. (2009). Polysaccharides from wolfberry antagonizes glutamate excitotoxicity in rat cortical neurons. Cell Mol Neurobiol 29 (8), 1233–1244. doi:10.1007/s10571-009-9419-x
Hollern, D. P., Xu, N., Thennavan, A., Glodowski, C., Garcia-Recio, S., Mott, K. R., et al. (2019). B cells and T follicular helper cells mediate response to checkpoint inhibitors in high mutation burden mouse models of breast cancer. Cell 179 (5), 1191–1206. doi:10.1016/j.cell.2019.10.028e1121
Hollingsworth, R. E., and Jansen, K. (2019). Turning the corner on therapeutic cancer vaccines. NPJ Vaccin. 4, 7. doi:10.1038/s41541-019-0103-y
Hong, F., Yan, J., Baran, J. T., Allendorf, D. J., Hansen, R. D., Ostroff, G. R., et al. (2004). Mechanism by which orally administered β-1,3-glucans enhance the tumoricidal activity of antitumor monoclonal antibodies in murine tumor models. J. Immunol. 173 (2), 797–806. doi:10.4049/jimmunol.173.2.797
Hou, C., Chen, L., Yang, L., and Ji, X. (2020). An insight into anti-inflammatory effects of natural polysaccharides. Int. J. Biol. Macromolecules 153, 248–255. doi:10.1016/j.ijbiomac.2020.02.315
Huang, G., Chen, X., and Huang, H. (2016). Chemical modifications and biological activities of polysaccharides. Cdt 17 (15), 1799–1803. doi:10.2174/1389450117666160502151004
Huang, W.-C., Kuo, K.-T., Bamodu, O. A., Lin, Y.-K., Wang, C.-H., Lee, K.-Y., et al. (2019). Astragalus polysaccharide (PG2) ameliorates cancer symptom clusters, as well as improves quality of life in patients with metastatic disease, through modulation of the inflammatory cascade. Cancers 11 (8), 1054. doi:10.3390/cancers11081054
Huang, Y., Qin, T., Huang, Y., Liu, Z., Bo, R., Hu, Y., et al. (2016). Rehmannia glutinosa polysaccharide liposome as a novel strategy for stimulating an efficient immune response and their effects on dendritic cells. Ijn 11, 6795–6808. doi:10.2147/IJN.S119108
Hume, D. A., Ross, I. L., Himes, S. R., Sasmono, R. T., Wells, C. A., and Ravasi, T. (2002). The mononuclear phagocyte system revisited. J. Leukoc. Biol. 72 (4), 621–627.
Hume, D. A. (2006). The mononuclear phagocyte system. Curr. Opin. Immunol. 18 (1), 49–53. doi:10.1016/j.coi.2005.11.008
Ina, K., Kataoka, T., and Ando, T. (2013). The use of lentinan for treating gastric cancer. Acamc 13 (5), 681–688. doi:10.2174/1871520611313050002
Jiang, M.-H., Zhu, L., and Jiang, J.-G. (2010). Immunoregulatory actions of polysaccharides from Chinese herbal medicine. Expert Opin. Ther. Targets 14 (12), 1367–1402. doi:10.1517/14728222.2010.531010
Jiao, R., Liu, Y., Gao, H., Xiao, J., and So, K. F. (2016). The anti-oxidant and antitumor properties of plant polysaccharides. Am. J. Chin. Med. 44 (3), 463–488. doi:10.1142/s0192415x16500269
Jose, G. M., and Kurup, G. M. (2017). The efficacy of sulfated polysaccharides from modulated immune functions of RAW 264.7 cells. Biomed. Pharmacother. 88, 677–683. doi:10.1016/j.biopha.2017.01.094
Khatua, S., and Acharya, K. (2019). Alkali treated antioxidative crude polysaccharide from Russula alatoreticula potentiates murine macrophages by tunning TLR/NF-κB pathway. Sci. Rep. 9 (1), 1713. doi:10.1038/s41598-018-37998-2
Kim, J.-K., Cho, M. L., Karnjanapratum, S., Shin, I.-S., and You, S. G. (2011). In vitro and in vivo immunomodulatory activity of sulfated polysaccharides from Enteromorpha prolifera. Int. J. Biol. Macromolecules 49 (5), 1051–1058. doi:10.1016/j.ijbiomac.2011.08.032
Kim, J. Y., Kim, Y. J., Kim, J. S., Ryu, H. S., Lee, H. K., Kang, J. S., et al. (2011). Adjuvant effect of a natural TLR4 ligand on dendritic cell-based cancer immunotherapy. Cancer Lett. 313 (2), 226–234. doi:10.1016/j.canlet.2011.09.009
Kim, J. Y., Yoon, Y. D., Ahn, J. M., Kang, J. S., Park, S.-K., Lee, K., et al. (2007). Angelan isolated from Angelica gigas Nakai induces dendritic cell maturation through toll-like receptor 4. Int. Immunopharmacology 7 (1), 78–87. doi:10.1016/j.intimp.2006.08.017
Kim, M.-H., Byon, Y.-Y., Ko, E.-J., Song, J.-Y., Yun, Y.-S., Shin, T., et al. (2009). Immunomodulatory activity of ginsan, a polysaccharide of panax ginseng, on dendritic cells. Korean J. Physiol. Pharmacol. 13 (3), 169–173. doi:10.4196/kjpp.2009.13.3.169
Kim, S. H., Lee, S. W., Park, H. J., Lee, S. H., Im, W. K., Kim, Y. D., et al. (2018). Anti-cancer activity of Angelica gigas by increasing immune response and stimulating natural killer and natural killer T cells. BMC Complement. Altern. Med. 18 (1). doi:10.1186/s12906-018-2277-7
Larionova, I., Kazakova, E., Patysheva, M., and Kzhyshkowska, J. (2020). Transcriptional, epigenetic and metabolic programming of tumor-associated macrophages. Cancers 12 (6), 1411. doi:10.3390/cancers12061411
Law, A. M. K., Valdes-Mora, F., and Gallego-Ortega, D. (2020). Myeloid-derived suppressor cells as a therapeutic target for cancer. Cells 9 (3), 561. doi:10.3390/cells9030561
Lee, D.-Y., Park, C. W., Lee, S. J., Park, H.-R., Kim, S. H., Son, S.-U., et al. (2019a). Anti-cancer effects of panax ginseng berry polysaccharides via activation of immune-related cells. Front. Pharmacol. 10, 1411. doi:10.3389/fphar.2019.01411
Lee, D.-Y., Park, C. W., Lee, S. J., Park, H.-R., Seo, D. B., Park, J. Y., et al. (2019b). Immunostimulating and antimetastatic effects of polysaccharides purified from ginseng berry. Am. J. Chin. Med. 47 (4), 823–839. doi:10.1142/S0192415X19500435
Lee, K. Y., and Jeon, Y. J. (2005). Macrophage activation by polysaccharide isolated from Astragalus membranaceus. Int. Immunopharmacology 5 (7-8), 1225–1233. doi:10.1016/j.intimp.2005.02.020
Li, A., Shuai, X., Jia, Z., Li, H., Liang, X., Su, D., et al. (2015). Ganoderma lucidum polysaccharide extract inhibits hepatocellular carcinoma growth by downregulating regulatory T cells accumulation and function by inducing microRNA-125b. J. Transl Med. 13, 100. doi:10.1186/s12967-015-0465-5
Li, L.-F., Liu, H.-B., Zhang, Q.-W., Li, Z.-P., Wong, T.-L., Fung, H.-Y., et al. (2018). Comprehensive comparison of polysaccharides from Ganoderma lucidum and G. sinense: chemical, antitumor, immunomodulating and gut-microbiota modulatory properties. Sci. Rep. 8 (1), 6172. doi:10.1038/s41598-018-22885-7
Li, W.-J., Li, L., Zhen, W.-Y., Wang, L.-F., Pan, M., Lv, J.-Q., et al. (2017). Ganoderma atrum polysaccharide ameliorates ROS generation and apoptosis in spleen and thymus of immunosuppressed mice. Food Chem. Toxicol. 99, 199–208. doi:10.1016/j.fct.2016.11.033
Li, W., Hu, X., Wang, S., Jiao, Z., Sun, T., Liu, T., et al. (2020). Characterization and anti-tumor bioactivity of astragalus polysaccharides by immunomodulation. Int. J. Biol. Macromolecules 145, 985–997. doi:10.1016/j.ijbiomac.2019.09.189
Li, W., Song, K., Wang, S., Zhang, C., Zhuang, M., Wang, Y., et al. (2019). Anti-tumor potential of astragalus polysaccharides on breast cancer cell line mediated by macrophage activation. Mater. Sci. Eng. C 98, 685–695. doi:10.1016/j.msec.2019.01.025
Li, X., Ma, L., and Zhang, L. (2019). Molecular basis for Poria cocos mushroom polysaccharide used as an antitumor drug in China. Prog. Mol. Biol. Transl Sci. 163, 263–296. doi:10.1016/bs.pmbts.2019.02.011
Liang, J., Li, H., Chen, J., He, L., Du, X., Zhou, L., et al. (2019). Dendrobium officinale polysaccharides alleviate colon tumorigenesis via restoring intestinal barrier function and enhancing anti-tumor immune response. Pharmacol. Res. 148, 104417. doi:10.1016/j.phrs.2019.104417
Liang, W., Mao, X., Peng, X., and Tang, S. (2014). Effects of sulfate group in red seaweed polysaccharides on anticoagulant activity and cytotoxicity. Carbohydr. Polym. 101, 776–785. doi:10.1016/j.carbpol.2013.10.010
Lin, J., Chang, Y.-J., Yang, W.-B., Yu, A. L., and Wong, C.-H. (2014). The multifaceted effects of polysaccharides isolated from Dendrobium huoshanense on immune functions with the induction of interleukin-1 receptor antagonist (IL-1ra) in monocytes. PLoS One 9 (4), e94040. doi:10.1371/journal.pone.0094040
Liu, D., Su, J., Lin, J., Qian, G., Chen, X., Song, S., et al. (2018). Activation of AMPK-dependent SIRT-1 by astragalus polysaccharide protects against ochratoxin A-induced immune stress in vitro and in vivo. Int. J. Biol. Macromolecules 120, 683–692. doi:10.1016/j.ijbiomac.2018.08.156
Liu, K.-S., Zhang, C., Dong, H.-L., Li, K.-K., Han, Q.-B., Wan, Y., et al. (2019). GSP-2, a polysaccharide extracted from Ganoderma sinense, is a novel toll-like receptor 4 agonist. PLoS One 14 (8), e0221636. doi:10.1371/journal.pone.0221636
Liu, L., Jia, J., Zeng, G., Zhao, Y., Qi, X., He, C., et al. (2013). Studies on immunoregulatory and anti-tumor activities of a polysaccharide from Salvia miltiorrhiza Bunge. Carbohydr. Polym. 92 (1), 479–483. doi:10.1016/j.carbpol.2012.09.061
Liu, L., Nie, S., and Xie, M. (2016). Tumor microenvironment as a new target for tumor immunotherapy of polysaccharides. Crit. Rev. Food Sci. Nutr. 56, S85–S94. doi:10.1080/10408398.2015.1077191
Locati, M., Mantovani, A., and Sica, A. (2013). Macrophage activation and polarization as an adaptive component of innate immunity. Adv. Immunol. 120, 163–184. doi:10.1016/B978-0-12-417028-5.00006-5
Lu, J., Sun, L.-X., Lin, Z.-B., Duan, X.-S., Ge, Z.-H., Xing, E.-H., et al. (2014). Antagonism by Ganoderma lucidum polysaccharides against the suppression by culture supernatants of B16F10 melanoma cells on macrophage. Phytother. Res. 28 (2), 200–206. doi:10.1002/ptr.4980
Mantovani, A., Sica, A., Sozzani, S., Allavena, P., Vecchi, A., and Locati, M. (2004). The chemokine system in diverse forms of macrophage activation and polarization. Trends Immunol. 25 (12), 677–686. doi:10.1016/j.it.2004.09.015
Mayoux, M., Roller, A., Pulko, V., Sammicheli, S., Chen, S., Sum, E., et al. (2020). Dendritic cells dictate responses to PD-L1 blockade cancer immunotherapy. Sci. Transl. Med. 12 (534), eaav7431. doi:10.1126/scitranslmed.aav7431
Meng, L.-Z., Lv, G.-P., Hu, D.-J., Cheong, K.-L., Xie, J., Zhao, J., et al. (2013). Effects of polysaccharides from different species of Dendrobium (Shihu) on macrophage function. Molecules 18 (5), 5779–5791. doi:10.3390/molecules18055779
Mougel, A., Terme, M., and Tanchot, C. (2019). Therapeutic cancer vaccine and combinations with antiangiogenic therapies and immune checkpoint blockade. Front. Immunol. 10, 467. doi:10.3389/fimmu.2019.00467
Mushiake, H., Tsunoda, T., Nukatsuka, M., Shimao, K., Fukushima, M., and Tahara, H. (2004). Dendritic cells might be one of key factors for eliciting antitumor effect by chemoimmunotherapy in vivo. Cancer Immunol. Immunother. 54 (2), 120–128. doi:10.1007/s00262-004-0585-x
Nefedova, Y., Huang, M., Kusmartsev, S., Bhattacharya, R., Cheng, P., Salup, R., et al. (2004). Hyperactivation of STAT3 is involved in abnormal differentiation of dendritic cells in cancer. J. Immunol. 172 (1), 464–474. doi:10.4049/jimmunol.172.1.464
Okoampah, E., Mao, Y., Yang, S., Sun, S., Zhou, C., and surfaces, B. B. (2020). Gold nanoparticles-biomembrane interactions: from fundamental to simulation. Colloids Surf. B: Biointerfaces 196, 111312. doi:10.1016/j.colsurfb.2020.111312
Pang, G., Wang, F., and Zhang, L. W. (2018). Dose matters: direct killing or immunoregulatory effects of natural polysaccharides in cancer treatment. Carbohydr. Polym. 195, 243–256. doi:10.1016/j.carbpol.2018.04.100
Pang, G., Zhang, S., Zhou, X., Yu, H., Wu, Y., Jiang, T., et al. (2019). Immunoactive polysaccharide functionalized gold nanocomposites promote dendritic cell stimulation and antitumor effects. Nanomedicine 14 (10), 1291–1306. doi:10.2217/nnm-2018-0390
Pastò, A., Consonni, F. M., and Sica, A. (2020). Influence of innate immunity on cancer cell stemness. Ijms 21 (9), 3352. doi:10.3390/ijms21093352
Paul, S., and Lal, G. (2017). The molecular mechanism of natural killer cells function and its importance in cancer immunotherapy. Front. Immunol. 8, 1124. doi:10.3389/fimmu.2017.01124
Pi, C.-C., Chu, C.-L., Lu, C.-Y., Zhuang, Y.-J., Wang, C.-L., Yu, Y.-H., et al. (2014). Polysaccharides from Ganoderma formosanum function as a Th1 adjuvant and stimulate cytotoxic T cell response in vivo. Vaccine 32 (3), 401–408. doi:10.1016/j.vaccine.2013.11.027
Pierce, S., Geanes, E. S., and Bradley, T. (2020). Targeting natural killer cells for improved immunity and control of the adaptive immune response. Front. Cel. Infect. Microbiol. 10, 231. doi:10.3389/fcimb.2020.00231
Pu, Y., Liu, Z., Tian, H., and Bao, Y. (2019). The immunomodulatory effect of Poria cocos polysaccharides is mediated by the Ca2+/PKC/p38/NF-κB signaling pathway in macrophages. Int. Immunopharmacology 72, 252–257. doi:10.1016/j.intimp.2019.04.017
Shao, P., Zhao, L.-H., Chen, Z., and Pan, J.-P. (2006). Regulation on maturation and function of dendritic cells by Astragalus mongholicus polysaccharides. Int. Immunopharmacology 6 (7), 1161–1166. doi:10.1016/j.intimp.2006.02.009
Shapouri-Moghaddam, , A., Mohammadian, , S., Vazini, , H., Taghadosi, , M., Esmaeili, , S., Mardani, , F., et al. (2018). Macrophage plasticity, polarization, and function in health and disease. J. Cell. Physiol. 233 (9), 6425–6440. doi:10.1002/jcp.26429
Sun, L.-X., Li, W.-D., Lin, Z.-B., Duan, X.-S., Li, X.-F., Yang, N., et al. (2014). Protection against lung cancer patient plasma-induced lymphocyte suppression byGanoderma LucidumPolysaccharides. Cell Physiol Biochem 33 (2), 289–299. doi:10.1159/000356669
Sun, L.-X., Li, W.-D., Lin, Z.-B., Duan, X.-S., Xing, E.-H., Jiang, M.-M., et al. (2015). Cytokine production suppression by culture supernatant of B16F10 cells and amelioration by Ganoderma lucidum polysaccharides in activated lymphocytes. Cell Tissue Res. 360 (2), 379–389. doi:10.1007/s00441-014-2083-6
Sun, L.-X., Lin, Z.-B., Duan, X.-S., Lu, J., Ge, Z.-H., Li, X.-F., et al. (2012). Enhanced MHC class I and costimulatory molecules on B16F10 cells byGanoderma lucidumpolysaccharides. J. Drug Target. 20 (7), 582–592. doi:10.3109/1061186X.2012.697167
Sun, M., Zhao, W., Xie, Q., Zhan, Y., and Wu, B. (2015). Lentinan reduces tumor progression by enhancing gemcitabine chemotherapy in urothelial bladder cancer. Surg. Oncol. 24 (1), 28–34. doi:10.1016/j.suronc.2014.11.002
Tang, W.-M., Chan, E., Kwok, C.-Y., Lee, Y.-K., Wu, J.-H., Wan, C.-W., et al. (2012). A review of the anticancer and immunomodulatory effects of Lycium barbarum fruit. Inflammopharmacol 20 (6), 307–314. doi:10.1007/s10787-011-0107-3
Tao, S., Zhao, Z., Zhang, X., Guan, X., Wei, J., Yuan, B., et al. (2020). The role of macrophages during breast cancer development and response to chemotherapy. Clin. Transl Oncol. 22 (11), 1938–1951. doi:10.1007/s12094-020-02348-0
Tian, H., Liu, Z., Pu, Y., and Bao, Y. (2019). Immunomodulatory effects exerted by Poria Cocos polysaccharides via TLR4/TRAF6/NF-κB signaling in vitro and in vivo. Biomed. Pharmacother. 112, 108709. doi:10.1016/j.biopha.2019.108709
Trefny, M. P., Kaiser, M., Stanczak, M. A., Herzig, P., Savic, S., Wiese, M., et al. (2020). PD-1+ natural killer cells in human non-small cell lung cancer can be activated by PD-1/PD-L1 blockade. Cancer Immunol. Immunother. 69 (8), 1505–1517. doi:10.1007/s00262-020-02558-z
Wang, C.-L., Lu, C.-Y., Hsueh, Y.-C., Liu, W.-H., and Chen, C.-J. (2014). biotechnologyActivation of antitumor immune responses by Ganoderma formosanum polysaccharides in tumor-bearing mice. Appl. Microbiol. Biotechnol. 98 (22), 9389–9398. doi:10.1007/s00253-014-6027-6
Wang, C.-Z., Hou, L., Wan, J.-Y., Yao, H., Yuan, J., Zeng, J., et al. (2020). Ginseng berry polysaccharides on inflammation-associated colon cancer: inhibiting T-cell differentiation, promoting apoptosis, and enhancing the effects of 5-fluorouracil. J. Ginseng Res. 44 (2), 282–290. doi:10.1016/j.jgr.2018.12.010
Wang, H., Cai, Y., Zheng, Y., Bai, Q., Xie, D., and Yu, J. (2017). Efficacy of biological response modifier lentinan with chemotherapy for advanced cancer: a meta-analysis. Cancer Med. 6 (10), 2222–2233. doi:10.1002/cam4.1156
Wang, J., Zuo, G., Li, J., Guan, T., Li, C., Jiang, R., et al. (2010). Induction of tumoricidal activity in mouse peritoneal macrophages by ginseng polysaccharide. Int. J. Biol. Macromolecules 46 (4), 389–395. doi:10.1016/j.ijbiomac.2010.02.007
Wang, N., Yang, J., Lu, J., Qiao, Q., Wu, T., Du, X., et al. (2014). A polysaccharide from Salvia miltiorrhiza Bunge improves immune function in gastric cancer rats. Carbohydr. Polym. 111, 47–55. doi:10.1016/j.carbpol.2014.04.061
Wang , N., Zhao , T., Li , S., Li, Y., Wang , Y., Li , D., et al. (2019). Fibroblast growth factor 21 ameliorates pancreatic fibrogenesis via regulating polarization of macrophages. Exp. Cell Res. 382 (1), 111457. doi:10.1016/j.yexcr.2019.06.002
Wang, W., Liu, M., Wang, Y., Yang, T., Li, D., Ding, F., et al. (2018). Lycium barbarum polysaccharide promotes maturation of dendritic cell via notch signaling and strengthens dendritic cell mediated T lymphocyte cytotoxicity on colon cancer cell CT26-WT. Evidence-Based Complement. Altern. Med. 2018, 1. doi:10.1155/2018/2305683
Wang, X, Wang, Y, Zhou, Q., Peng, M., Zhang, J., Chen, M., et al. (2018). Immunomodulatory effect of lentinan on aberrant T subsets and cytokines profile in non-small cell lung cancer patients. Pathol. Oncol. Res. 26 (1), 499–505. doi:10.1007/s12253-018-0545-y
Wang, Y., Fan, X., and Wu, X. (2020). Ganoderma lucidum polysaccharide (GLP) enhances antitumor immune response by regulating differentiation and inhibition of MDSCs via a CARD9-NF-κB-Ido pathway. Biosci. Rep. 40 (6). doi:10.1042/bsr20201170
Wang, Y., Kwak, M., Lee, P. C.-W., and Jin, J.-O. (2018). Rehmannia glutinosa polysaccharide promoted activation of human dendritic cells. Int. J. Biol. Macromolecules 116, 232–238. doi:10.1016/j.ijbiomac.2018.04.144
Wculek, S. K., Cueto, F. J., Mujal, A. M., Melero, I., Krummel, M. F., and Sancho, D. (2020). Dendritic cells in cancer immunology and immunotherapy. Nat. Rev. Immunol. 20 (1), 7–24. doi:10.1038/s41577-019-0210-z
Wei, W., Li, Z.-P., Bian, Z.-X., and Han, Q.-B. (2019). Astragalus polysaccharide RAP induces macrophage phenotype polarization to M1 via the notch signaling pathway. Molecules 24 (10), 2016. doi:10.3390/molecules24102016
Wu, H.-T., He, X.-J., Hong, Y.-K., Ma, T., Xu, Y.-P., and Li, H.-H. (2010). Chemical characterization of Lycium barbarum polysaccharides and its inhibition against liver oxidative injury of high-fat mice. Int. J. Biol. Macromolecules 46 (5), 540–543. doi:10.1016/j.ijbiomac.2010.02.010
Xia, L., Liu, X., Guo, H., Zhang, H., Zhu, J., and Ren, F. (2012). Partial characterization and immunomodulatory activity of polysaccharides from the stem of Dendrobium officinale (Tiepishihu) in vitro. J. Funct. Foods 4 (1), 294–301. doi:10.1016/j.jff.2011.12.006
Xu, L., Kwak, M., Zhang, W., Zeng, L., Lee, P. C.-W., and Jin, J.-O. (2017a). Rehmannia glutinosa polysaccharide induces toll-like receptor 4 dependent spleen dendritic cell maturation and anti-cancer immunity. Oncoimmunology 6 (7), e1325981. doi:10.1080/2162402X.2017.1325981
Xu, L., Zhang, W., Zeng, L., and Jin, J.-O. (2017b). Rehmannia glutinosa polysaccharide induced an anti-cancer effect by activating natural killer cells. Int. J. Biol. Macromolecules 105 (Pt 1), 680–685. doi:10.1016/j.ijbiomac.2017.07.090
Xu, Y., Wu, Y.-j., Sun, P.-l., Zhang, F.-m., Linhardt, R. J., and Zhang, A.-q. (2019). Chemically modified polysaccharides: synthesis, characterization, structure activity relationships of action. Int. J. Biol. Macromolecules 132, 970–977. doi:10.1016/j.ijbiomac.2019.03.213
Xu, Z., Chen, X., Zhong, Z., Chen, L., and Wang, Y. (2011). Ganoderma lucidum polysaccharides: immunomodulation and potential anti-tumor activities. Am. J. Chin. Med. 39 (1), 15–27. doi:10.1142/S0192415X11008610
Yin, M., Zhang, Y., and Li, H. (2019). Advances in research on immunoregulation of macrophages by plant polysaccharides. Front. Immunol. 10. doi:10.3389/fimmu.2019.00145
Yu, H., Pardoll, D., and Jove, R. (2009). STATs in cancer inflammation and immunity: a leading role for STAT3. Nat. Rev. Cancer 9 (11), 798–809. doi:10.1038/nrc2734
Yu, Y., Shen, M., Song, Q., and Xie, J. (2018). Biological activities and pharmaceutical applications of polysaccharide from natural resources: a review. Carbohydr. Polym. 183, 91–101. doi:10.1016/j.carbpol.2017.12.009
Yue, G. G. L., Chan, B. C. L., Han, X.-Q., Cheng, L., Wong, E. C. W., Leung, P. C., et al. (2013). Immunomodulatory activities ofGanoderma sinensePolysaccharides in human immune cells. Nutr. Cancer 65 (5), 765–774. doi:10.1080/01635581.2013.788725
Yue, H., Zeng, H., and Ding, K. (2020). A review of isolation methods, structure features and bioactivities of polysaccharides from Dendrobium species. Chin. J. Nat. Medicines 18 (1), 1–27. doi:10.1016/s1875-5364(20)30001-7
Zeng, P., Chen, Y., Zhang, L., and Xing, M. (2019). Ganoderma lucidum polysaccharide used for treating physical frailty in China. Prog. Mol. Biol. Transl Sci. 163, 179–219. doi:10.1016/bs.pmbts.2019.02.009
Zhang, R.-X., Li, M.-X., and Jia, Z.-P. (2008). Rehmannia glutinosa: review of botany, chemistry and pharmacology. J. Ethnopharmacology 117 (2), 199–214. doi:10.1016/j.jep.2008.02.018
Zhang, S., Nie, S., Huang, D., Huang, J., Feng, Y., Xie, M., et al. (2014b). A polysaccharide from Ganoderma atrum inhibits tumor growth by induction of apoptosis and activation of immune response in CT26-bearing mice. J. Agric. Food Chem. 62 (38), 9296–9304. doi:10.1021/jf503250d
Zhang, S., Nie, S., Huang, D., Huang, J., Feng, Y., Xie, M., et al. (2014a). Ganoderma atrum polysaccharide evokes antitumor activity via cAMP-PKA mediated apoptotic pathway and down-regulation of Ca2+/PKC signal pathway. Food Chem. Toxicol. 68, 239–246. doi:10.1016/j.fct.2014.03.020
Zhang, S., Nie, S., Huang, D., Li, W., and Xie, M. (2013). Immunomodulatory effect of Ganoderma atrum polysaccharide on CT26 tumor-bearing mice. Food Chem. 136, 1213–1219. doi:10.1016/j.foodchem.2012.08.090
Zhang, S., Pang, G., Chen, C., Qin, J., Yu, H., Liu, Y., et al. (2019). Effective cancer immunotherapy by Ganoderma lucidum polysaccharide-gold nanocomposites through dendritic cell activation and memory T cell response. Carbohydr. Polym. 205, 192–202. doi:10.1016/j.carbpol.2018.10.028
Zhang, Y., Zhang, M., Jiang, Y., Li, X., He, Y., Zeng, P., et al. (2018). Lentinan as an immunotherapeutic for treating lung cancer: a review of 12 years clinical studies in China. J. Cancer Res. Clin. Oncol. 144 (11), 2177–2186. doi:10.1007/s00432-018-2718-1
Zhu, J., Zhao, L., Zhao, X., and Chen, Z. (2007). Lycium barbarum polysaccharides regulate phenotypic and functional maturation of murine dendritic cells. Cel Biol. Int. 31 (6), 615–619. doi:10.1016/j.cellbi.2006.12.002
Zhu, X.-L., Chen, A.-F., and Lin, Z.-B. (2007). Ganoderma lucidum polysaccharides enhance the function of immunological effector cells in immunosuppressed mice. J. Ethnopharmacology 111 (2), 219–226. doi:10.1016/j.jep.2006.11.013
Keywords: natural polysaccharides, tumor immunotherapy, antigen presenting cells, natural killer cells, natural adjuvant
Citation: Li Y, Wang X, Ma X, Liu C, Wu J and Sun C (2021) Natural Polysaccharides and Their Derivates: A Promising Natural Adjuvant for Tumor Immunotherapy. Front. Pharmacol. 12:621813. doi: 10.3389/fphar.2021.621813
Received: 27 October 2020; Accepted: 15 March 2021;
Published: 14 April 2021.
Edited by:
Jiang-Jiang Qin, Chinese Academy of Sciences (CAS), ChinaReviewed by:
Wen Zhou, Guangzhou University of Chinese Medicine, ChinaQiang Yu, Nanchang University, China
Copyright © 2021 Li, Wang, Ma, Liu, Wu and Sun. This is an open-access article distributed under the terms of the Creative Commons Attribution License (CC BY). The use, distribution or reproduction in other forums is permitted, provided the original author(s) and the copyright owner(s) are credited and that the original publication in this journal is cited, in accordance with accepted academic practice. No use, distribution or reproduction is permitted which does not comply with these terms.
*Correspondence: Jibiao Wu, d3VqaWJpYW8xOTYzQDE2My5jb20=; Changgang Sun, c2NnZG9jdG9yQDEyNi5jb20=
†These authors have contributed equally to this work