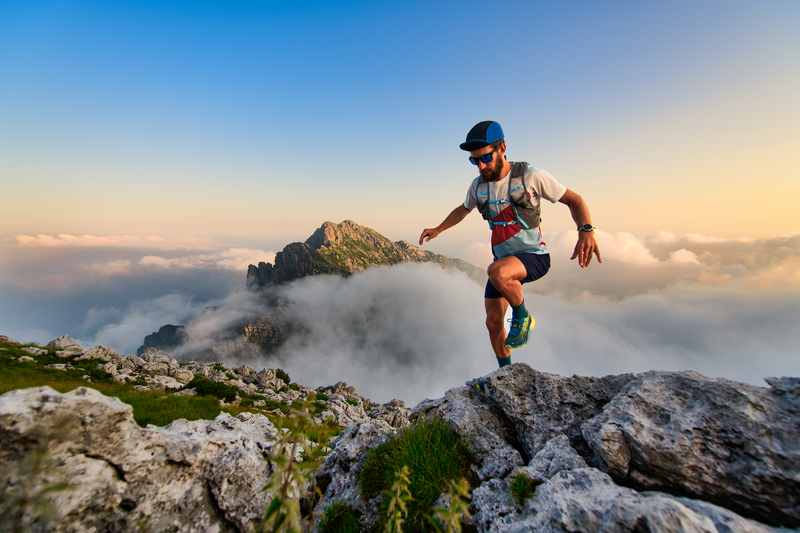
94% of researchers rate our articles as excellent or good
Learn more about the work of our research integrity team to safeguard the quality of each article we publish.
Find out more
ORIGINAL RESEARCH article
Front. Pharmacol. , 05 May 2021
Sec. Gastrointestinal and Hepatic Pharmacology
Volume 12 - 2021 | https://doi.org/10.3389/fphar.2021.586010
The flavonoid quercitrin has a strong antioxidant property. It is also reported to have a protective effect on the liver. However, the mechanism by which it exerts a protective effect on the liver is not fully understood. The objective of this article is to confirm the protective effect of quercitrin extracted from Albiziae flos on acetaminophen (APAP)-induced liver injury and to explain its mechanism. In the in vivo study, quercitrin was administered orally to BALB/c mice at a dose of 50, 100, and 200 mg/kg for seven consecutive days. APAP (300 mg/kg) was injected intraperitoneally after a last dose of quercitrin was administered. Determination of alanine aminotransferase (ALT), aspartate aminotransferase (AST), lactate dehydrogenase (LDH), interleukin 6 (IL-6), tumor necrosis factor α (TNF-α), reactive oxygen species (ROS), superoxide dismutase (SOD), glutathione (GSH), glutathione peroxidase (GSH-Px), catalase (CAT), and malondialdehyde (MDA) levels showed that quercitrin effectively attenuated APAP-induced acute liver injury in mice. Results of the in vitro study showed that quercitrin reduced the levels of ROS, protected mitochondria from damage, and restored the activity of mitochondrial complex I in APAP-treated L-02 cells. The addition of rotenone which is an inhibitor of complex I blocked the protective effect of quercitrin. The expression of mitochondrial complex I was also maintained by quercitrin. Our results suggest that quercitrin can maintain the level of mitochondrial complex I in injured cells and restore its activity, which reduces the production of ROS, protects the mitochondria from oxidative stress, and has a protective effect on the liver.
The liver which is one of the important organs in the human body is responsible for metabolizing ingested compounds and removing toxic substances (Mitra and Metcalf, 2012). Injury to the liver can be caused by hepatotoxic compounds like acetaminophen (APAP), for example. APAP is widely used clinically as an antipyretic and an analgesic drug (Lee, 2017). Although it is safe and effective at therapeutic doses, higher doses of APAP are hepatotoxic and can cause acute liver injury. Abuse of APAP is currently the main cause for failure of the liver (Yan et al., 2018). N-acetylcysteine (NAC) is currently the only U.S. Food and Drug Administration–approved antidote for treating toxicity of APAP. However, long-term use of NAC can cause toxic side effects and damage regeneration of the liver (Yang et al., 2009). Hence, newer alternative drugs are urgently needed to treat APAP-induced acute liver injury.
Numerous studies have shown that excessive APAP exposure can lead to mitochondrial dysfunction, increased ATP consumption, and increased generation of reactive oxygen species (ROS) and oxidative stress (Subramanya et al., 2018). All of these factors ultimately result in injury to the liver (Masubuchi et al., 2005; Jaeschke and Bajt, 2006). Respiration is accomplished by the electron transport chain (ETC) located on the mitochondrial inner membrane, and the mitochondrial complex I is an important component of the ETC (Guo et al., 2018). Reduction in the activity of complex I results in electron leakage, which in turn leads to the generation of ROS and mitochondrial dysfunction (Dröse et al., 2016). The activity of mitochondrial complex I is regulated by the mitochondrial negative regulatory protein methylation–controlled J (MCJ) protein (Hatle et al., 2013). A previously published study has reported that excessive APAP can increase the expression of MCJ in mice (Barbier-Torres et al., 2017). MCJ binds to mitochondrial complex I, prevents the formation of supercomplexes, and inhibits the activity of complex I. This results in an increased production of mitochondrial ROS and oxidative stress (Ramachandran et al., 2018). Restoring the activity of mitochondrial complex I is therefore one of the potential targets for liver protection.
Flavonoids are a group of natural substances with variable phenolic structures (Panche et al., 2016). Studies have reported that flavonoids reduced APAP-induced liver injury due to their powerful antioxidant effect (Kuang et al., 2017; Li et al., 2017; Shao et al., 2017; Chowdhury et al., 2019). Therefore, we have investigated the protective effect of Albiziae flos (dry inflorescence or the bud of Albizia julibrissin Durazz.), a traditional Chinese medicine rich in flavonoids, on APAP-induced acute liver injury and found that its active ingredient is quercitrin (Que). As a natural flavonoid with a wide range of pharmacological activities, quercitrin has been proven to have antioxidant, anti-inflammatory, antitumor, and antidepressant effects (Camuesco et al., 2004; Li et al., 2016; Xiong et al., 2020). Quercitrin is known to have protective effects on liver injury; however, its mechanism of action remains to be further elucidated (Raja et al., 2007; Truong et al., 2016).
In this study, we established the efficacy of quercitrin in attenuating APAP-induced acute liver injury and studied its effect on complex I to further clarify its mechanism of action at a molecular level.
Albiziae flos was purchased from Shizhentang Badong Pharmaceutical (Lot: 19021502, Enshi, China). APAP, silymarin (Sil), and rotenone were purchased from Macklin (Shanghai, China). The hematoxylin and eosin (H & E) dye kit was purchased from Baiqiandu (Wuhan, China). Alanine aminotransferase (ALT), aspartate aminotransferase (AST), lactate dehydrogenase (LDH), superoxide dismutase (SOD), glutathione (GSH), glutathione peroxidase (GSH-Px), catalase (CAT), and malondialdehyde (MDA) commercial kits were purchased from Nanjing Jiancheng Bioengineering Institute (Nanjing, China). The BCA protein concentration assay kit was purchased from Biosharp (Hefei, China). Dihydroethidium (DHE), 4′,6-diamidino-2-phenylindole (DAPI), and RIPA lysis buffer were purchased from Beyotime (Shanghai, China). Interleukin 6 (IL-6) and tumor necrosis factor α (TNF-α) ELISA kits were purchased from Elabscience (Wuhan, China). The electron transport chain complex I assay kit and mitochondrial protein extraction kit were purchased from Nanjing Jiancheng Bioengineering Institute (Nanjing, China). DCFH-DA and JC-1 were purchased from Solarbio (Beijing, China). F4/80, apoptosis-inducing factor (AIF), endonuclease G (EndoG), NDUFS3, and GAPDH antibodies were purchased from Proteintech (Rosemont, IL, United States). The anti-rabbit secondary antibody was purchased from Cell Signaling Technology (Danvers, MA, United States). The ELC chemiluminescence kit and nitrocellulose (NC) membrane were purchased from Bio-Rad (Hercules, CA, United States). HPLC grade solvents were purchased from Tedia (Fairfield, OH, United States). All other solvents were of analytical grade (Sinopharm Chemical Reagent, Shanghai, China).
The powder of Albiziae flos (10 kg) was extracted with 80% EtOH (100 L). The combined extracts were concentrated by a rotary evaporator. The concentrate was successively extracted with petroleum ether, ethyl acetate, and n-butanol. The ethyl acetate extract was further separated on the silica gel column (100–200 mesh), and three subfractions were obtained by gradient elution with trichloromethane-methanol (6:1–2:1). The solvent of subfraction 2 was recovered, and the remaining extract was fully mixed with trichloromethane–methanol (1:1) solution and then filtered after standing overnight. The filtrate residue was dried, and the yellow powder was obtained as quercitrin. The purity was detected by a 1260 high-performance liquid chromatograph (HPLC) (Agilent, CA, United States), and the structure was analyzed by a Xevo G2-XS QTOF mass spectrometer (Waters, MA, United States) and Bruker Avance III 800 MHz instrument (Bruker, Karlsruhe, Germany).
Male BALB/c mice of 18–22 g body weight were provided by the Hubei Provincial Center for Disease Control and Prevention. The mice were kept at a temperature of 24 ± 2°C and a humidity of 50 ± 5% and were exposed to 12 h of light and 12 h of darkness each day. All animals had free access to food and water. All animal protocols were approved by the Experimental Animal Ethics Committee of the Hubei University of Chinese Medicine (NO. HUCMS202006011).
Following one week of adaptive feeding, 60 mice were randomly separated into following six groups (n = 10): a control group, a model group (APAP group), three treatment groups (APAP +50 mg/kg Que group; APAP +100 mg/kg Que group; APAP +200 mg/kg Que group), and a positive control group (APAP +100 mg/kg Sil group).
APAP (300 mg/kg) was dissolved in sterile physiological saline; quercitrin and silymarin were formulated as a suspension with 0.5% (w/v) CMC-Na. Mice in the three treatment groups were orally administered with quercitrin suspension for seven consecutive days, and a single APAP (300 mg/kg) was injected intraperitoneally after 1 h of the last administration of quercitrin. The positive control group was administered in the similar manner. Control group and APAP group mice were orally administered with 0.5% CMC-Na solution for seven consecutive days. One hour after the last administration of CMC-Na solution, mice in the control group were intraperitoneally injected with saline, whereas mice in the APAP group were intraperitoneally injected with a single dose of APAP (300 mg/kg). The volume of oral administration and intraperitoneal injection was 0.1 ml/10 g.
In order to eliminate the effect of diet on APAP modeling, all mice were not given any food for 15 h before intraperitoneal injection and were given food following the intraperitoneal injection. All mice were sacrificed after they were treated with APAP for 14 h, and their blood samples and liver tissues were collected for further analysis. The blood samples were centrifuged (4°C, 4000 rpm, and 10 min) to separate the serum and stored at −20°C. After weighing the liver tissues, one part was fixed in 4% paraformaldehyde and the other was stored at −80°C.
The liver tissues were fixed with 4% paraformaldehyde for 24 h and then embedded in paraffin. Next, the tissues were cut into 5-μm sections. Hematoxylin and eosin (H&E) were used for staining, and the F4/80 antibody was used for immunohistochemical staining.
To determine the levels of ROS, the frozen liver tissues were cut into 5-μm sections, stained with DHE, and then counterstained with DAPI. The sections were examined under the Nikon Eclipse C1 fluorescence microscope imaging system (Nikon Instruments, Tokyo, Japan).
ALT and AST were determined according to the following steps. In short, 5 μL of diluted serum was incubated with 20 μL of ALT/AST matrix solution at 37°C for 30 min, and then, 20 μL of 2,4-dinitrophenylhydrazine hydrochloride solution was added to incubate at 37°C for another 20 min. Finally, 200 μL 0.4 M NaOH was added to stop the reaction. After 15 min at room temperature, absorbance at 510 nm was read by a SPARK 10 M microplate reader (Tecan, Switzerland), and the ALT/AST activity was calculated according to the standard curve. The LDH activity was determined according to the manufacturer’s instructions.
The levels of IL-6 and TNF-α in the serum were determined according to the instructions provided by the manufacturers of ELISA kits. The absorbance at 450 nm was measured using a SPARK 10 M microplate reader (Tecan, Switzerland).
Appropriate amount of thawed frozen liver tissue was taken, and 9-fold volume of 4°C physiological saline was added to homogenize and centrifuged (4°C, 4000 rpm and 10 min). Following centrifugation, the supernatant was collected. Protein concentrations in liver homogenates were measured using the BCA protein concentration assay kit. The SOD, GSH, GSH-Px, CAT, and MDA content in the liver homogenates were determined according to the instructions provided by the respective assay kits.
The L-02 (HL-0072) cell line was obtained from the Key Laboratory of Traditional Chinese Medicine Resources and Chemistry of Hubei Province (Wuhan, China). L-02 cells were cultured with RPMI-1640 medium comprising 10% fetal bovine serum (FBS) in a 5% CO2 incubator at 37°C and humid atmosphere.
L-02 cells (1 × 105 cells/well) were sowed in 96-well plates and divided into five groups: a control group, a model group (APAP group), and three treatment groups. The cells were cultured overnight, and after checking for cell adherence, the cells in the treatment groups were treated with quercitrin at final concentrations of 20, 40, and 80 μM for 15 min. Next, APAP (10 mM) was added to each group, except the control group. After 24 h, each group was incubated with MTT (5 mg/mL) for 4 h. Following this, the supernatant was removed carefully, and 150 μL of DMSO was added to each well to completely dissolve the purple crystals of formazan. The optical density (OD) value at 490 nm was measured by using a microplate reader. In order to determine the effect of rotenone, a complex I inhibitor, on quercitrin activity, rotenone (10 μM) was added into the wells after 5 h of treatment by APAP.
L-02 cells (2.5 × 105 cells/well) were seeded in 6-well plates and divided into four groups: a control group, a drug group [Que group, cells were treated with quercitrin (80 μM) only], a model group [APAP group, cells were treated with APAP (10 mM) only], and a treatment group (APAP + Que group, cells were incubated with quercitrin for 15 min and then treated with APAP). After 24 h incubation, ROS was determined by flow cytometry (FACSCalibur, BD Company, NJ, United States) after staining with DCFH-DA. The mitochondrial membrane potential was observed with a fluorescence microscope (Nikon Instruments, Tokyo, Japan) after staining with JC-1.
Total mitochondrial proteins of L-02 cells were extracted by using a mitochondrial protein extraction kit. Complex I catalyzes the conversion of reduced nicotinamide adenine dinucleotide (NADH) to nicotinamide adenine dinucleotide (NAD). Therefore, the activity of complex I was calculated by measuring the decrease in absorbance at 340 nm to determine the conversion rate of NADH according to the manufacturer’s instructions.
Total protein extracts of cells were prepared using RIPA lysis buffer. Protein concentrations were determined in all samples using the BCA protein concentration assay kit. Protein (equal concentration) samples were separated by running them on a 10% SDS-PAGE gel and transferred onto an NC membrane. This NC membrane was blocked using 5% (w/v) nonfat milk at room temperature for 2 h. Following blocking, the membrane was incubated with the primary antibody at 4°C overnight. The next day, the membrane was incubated with the anti-rabbit secondary antibody for 2 h at room temperature. The protein bands were detected by chemiluminescence using the ECL reagent and photographed using a FluorChem FC3 gel imager.
All data are presented as mean ± standard deviation (SD). A one-way analysis of variance (ANOVA) was used for data analysis. p < 0.05 or p < 0.01 represented statistically significant difference.
Active screening showed that quercitrin was the main active ingredient in Albiziae flos, so quercitrin was extracted for subsequent experiments (Supplementary Figures S1, S2; Supplementary Table S1). The obtained yellow powder was easily soluble in methanol, and its purity was more than 95% by HPLC (Figure 1A). Mass spectrometry showed that its HR-ESI-MS m/z 471.0881 ([M + Na]+, calcd. 471.0903) and m/z 449.1083 ([M + H]+, calcd. 449.1084), which indicated that the molecular formula of this compound was C21H20O11 (Figure 1B). Finally, 1H- and 13C-nuclear magnetic resonance (NMR) analysis showed that the yellow powder was quercitrin (Supplementary Table S2, Figure 1C).
FIGURE 1. Structural identification of quercitrin. (A) HPLC-UV chromatogram obtained at 210 nm. (B) MS (top) and MS/MS (bottom) data for quercitrin. (C) Structural formula of quercitrin.
In the beginning, we evaluated the protective effect of quercitrin on the liver. We first did a gross examination of the livers of the mice. Histopathological results are presented in Figure 2A. Compared with the control group, the liver cells in the APAP group were necrotic in a great area, as indicated by the black arrow; the necrotic area was filled with red blood cells, as indicated by the yellow arrow; and neutrophils were aggregated, as indicated by the red arrow. Moreover, the cellular structure of the liver tissue in quercitrin-pretreated mice tended to be normal compared with the APAP group. In these quercitrin-pretreated mice, the inflammatory infiltration was significantly decreased, and only a few hepatocytes were edema, as indicated by green arrows. The necrotic area of the liver tissue was determined. Massive necrotic areas occurred in the APAP group. In contrast, quercitrin pretreatment effectively reduced liver cell necrosis in a dose-dependent manner (Figure 2B). Furthermore, the liver organ coefficients were calculated. The liver organ coefficient of the APAP group was significantly augmented compared with the control group (p < 0.01). Quercitrin 100 and 200 mg/kg pretreatment significantly decreased the organ coefficient of the liver (p < 0.01) (Figure 2C).
FIGURE 2. Effect of quercitrin on APAP-induced acute liver injury in mice. (A) Pathological evaluation of the liver tissue of mice (H & E staining 200×). Black arrows show cell nuclei fragmentation; yellow arrows show hepatic sinusoidal congestion; red arrow shows neutrophil aggregation; green arrows show hepatocyte edema. (B) Necrotic area of the liver tissue. (C) Effect of quercitrin on liver organ coefficients of mice. (D–F) Analysis of ALT, AST, and LDH contents in the serum of mice. The experimental data are presented as mean ± SD, ##p < 0.01, compared with the control group; **p < 0.01, compared with the APAP group.
There was a significant increase in serum levels of ALT, AST, and LDH in the APAP group compared with the control group (p < 0.01). Furthermore, quercitrin pretreatment significantly reduced ALT, AST, and LDH in serum compared with the APAP group (p < 0.01) and exhibited a dose-dependent effect (Figures 2D–F). The abovementioned findings indicated that quercitrin could diminish APAP-induced acute liver injury in mice and improve APAP-induced liver enlargement and cell injury.
In order to further confirm that quercitrin has a protective effect on the liver, the levels of inflammatory factors were determined. Results of the F4/80 protein content are shown in Figure 3A, and the brown part is the expression of F4/80. The sections were analyzed by integrated optical density (IOD), and the results were consistent with those observed in the sections (Figure 3B). F4/80 expression in the APAP group increased significantly when compared with the control group (p < 0.01). Pretreatment with quercitrin and silymarin significantly reduced the increase in F4/80 caused by APAP (p < 0.01). The above results demonstrated that APAP increased the macrophage content in the liver, which decreased after quercitrin treatment.
FIGURE 3. Effect of quercitrin on APAP-induced inflammation. (A) Effect of quercitrin on F4/80 expression in the liver tissue of mice (200×). (B) IOD of immunohistochemistry. (C,D) Effect of quercitrin on serum IL-6 and TNF-α levels in APAP-induced liver injury mice. The experimental data are expressed as mean ± SD, ##p < 0.01, compared with the control group; **p < 0.01, compared with the APAP group.
Additionally, the serum levels of IL-6 and TNF-α in the APAP group were significantly increased when compared with the control group (p < 0.01). Compared with the APAP group, the levels of IL-6 and TNF-α in the serum of mice pretreated with quercitrin were significantly reduced (p < 0.01) (Figures 3C,D). The reduction of pro-inflammatory cytokines also indicated that quercitrin can attenuate APAP-induced liver injury.
Next, the effect of quercitrin on APAP-induced oxidative stress was evaluated. The ROS fluorescent sections were observed, and the sections were analyzed by IOD analysis (Figures 4A,B). There was a significant augmentation of the ROS levels in the APAP group compared with the control group (p < 0.01). In addition, there was a significant decrease in the ROS levels in the three treatment groups compared with the APAP group (p < 0.01).
FIGURE 4. Effect of quercitrin on APAP-induced oxidative stress. (A) ROS frozen sections of the liver tissue of mice (DHE staining 200×). (B) IOD of frozen sections. (C–F) SOD, GSH, GSH-Px, and CAT levels in the liver of mice. (G) MDA content in the liver of mice. The experimental data are expressed as mean ± SD, ##p < 0.01, compared with the control group; *p < 0.05, **p < 0.01, compared with the APAP group.
The results of the antioxidant enzymes assay showed that SOD, GSH, GSH-Px, and CAT levels in the APAP group were significantly reduced compared with the control group (p < 0.01). Pretreatment with quercitrin significantly increased the levels of SOD, GSH, GSH-Px, and CAT (p < 0.05, p < 0.01) and showed a dose-dependent effect. The efficacy of silymarin was comparable to that of quercitrin at 100 mg/ml (Figures 4C–F). In addition, the content of MDA in the APAP group was significantly increased when compared with the control group (p < 0.01). Pretreatment with quercitrin 100 and 200 mg/kg significantly reduced the levels of MDA when compared with the APAP group (p < 0.05, p < 0.01) (Figure 4G). Above results indicated that quercitrin can alleviate APAP-induced acute liver injury by reducing oxidative stress injury in the liver of mice.
First, the in vitro activity of quercitrin was determined. 80 μM quercitrin significantly increased cell viability, APAP (10 mM) decreased cell viability to 66.26 ± 1.54%, while pretreatment with quercitrin restored cell viability (p < 0.01) (Figures 5A,B). These results demonstrated that quercitrin could effectively reduce APAP-induced L-02 cell injury.
FIGURE 5. Effect of quercitrin on the activity and ROS levels of APAP-treated L-02 cells. (A) Effect of quercitrin on the viability of L-02 cells. (B) Effect of quercitrin on the viability of APAP-treated L-02 cells. (C) Effect of quercitrin on ROS levels in L-02 cells. The experimental data are expressed as mean ± SD, and the experiment was repeated three times, ##p < 0.01, compared with the control group; **p < 0.01, compared with the APAP group.
Next, we studied the effect of quercitrin on the ROS levels of L-02 cells. There was no significant difference in the levels of ROS between the Que group and the control group. However, the levels of ROS in the APAP group were significantly higher than those in the control group (p < 0.01). The ROS levels decreased significantly in the APAP + Que group when compared with the APAP group (p < 0.01) (Figure 5C). The above results demonstrated that pretreatment with quercitrin did not affect the ROS levels of normal cells but decreased the ROS levels of L-02 cells which were treated with APAP.
Mitochondrial damage leads to depolarization, and the fluorescence of the JC-1 changes from red to green. Due to the decrease in mitochondrial membrane potential, JC-1 aggregates (red fluorescence) decreased and monomers (green fluorescence) increased in the APAP group. Pretreatment with quercitrin resulted in a significant increase in red fluorescence, indicating that mitochondrial depolarization was prevented (Figure 6A). In addition, the expression of apoptosis-related proteins AIF and EndoG in the APAP group increased significantly compared with the control group (p < 0.01), while the expression of proteins in the APAP + Que group decreased significantly compared with the APAP group (p < 0.01) (Figures 6B,C). The above results showed that quercitrin can prevent mitochondrial dysfunction caused by APAP.
FIGURE 6. Effect of quercitrin on mitochondria. (A) Effect of quercitrin on mitochondrial membrane potential. (B) Western blot analysis of AIF and EndoG in L-02 cells. (C) Western blot densitometric analysis. The experimental data are expressed as mean ± SD, and the experiment was repeated three times, ##p < 0.01, compared with the control group; **p < 0.01, compared with the APAP group.
Although the activity of the complex I in the Que group was slightly increased when compared with the control group, there was no significant difference. The activity of the complex I in the APAP group was however significantly decreased when compared with the control group (p < 0.01). The complex I activity of the APAP + Que group was significantly increased when compared with the APAP group (p < 0.05) (Figure 7A). The results indicated that excessive levels of APAP results in a significant decrease in mitochondrial complex I activity. Pretreatment with quercitrin (80 μM) can restore the activity of complex I.
FIGURE 7. Effect of quercitrin on mitochondrial complex I. (A) Effect of quercitrin on the activity of complex I in L-02 cells. (B) Effect of APAP and rotenone treatment on L-02 cells viability in the blank group and Que group. (C) Western blot analysis of NDUFS3 (complex I) in L-02 cells. (D) Western blot densitometric analysis. The experimental data are expressed as mean ± SD, and the experiment was repeated three times, ##p < 0.01, compared with the control group; *p < 0.05, **p < 0.01, compared with the APAP group; ΔΔP < 0.01, the APAP-treated blank group was compared with the control group, the Que group was compared with the blank group, and the APAP + rotenone group was compared with the APAP group.
Additionally, the blank and Que groups were treated with APAP in the presence and absence of the complex I inhibitor. The smallest survival was seen in the L-02 cells in the blank group treated with APAP. Quercitrin improved the survival rate of L-02 cells treated with APAP. The combined treatment of APAP and rotenone reduced the cell survival of the Que group when compared with the Que group treated with APAP (p < 0.01). In contrast, the survival of the blank group treated with APAP could not be reduced further by rotenone (Figure 7B). Rotenone reduced the protective effect of quercitrin on APAP-induced cell injury by inhibiting the activity of complex I. This result indicated that the protective effect of quercitrin on the liver protection is via restoring the activity of complex I.
The expression of NDUFS3 (a well-characterized subunit of complex I) in each group was detected by Western blot. The expression of NDUFS3 (complex I) protein in the APAP group was significantly reduced when compared with the control group (p < 0.01). Pretreatment with quercitrin significantly increased the expression of NDUFS3 (complex I) in the APAP + Que group when compared with the APAP group (p < 0.01) (Figures 7C,D). The above results indicate that quercitrin maintained the activity of complex I by maintaining its protein content and thus attenuated APAP-induced cell injury.
Quercitrin is a flavonoid with various pharmacological activities. It is isolated from the ethyl acetate extract of Albiziae flos. We studied the mechanisms of quercitrin, which could be of a potential benefit in the attenuation APAP-induced acute liver injury.
APAP can increase the permeability of the cell membrane of the liver and released the enzymes in the cell and increased the levels of ALT, AST, and LDH in serum (Naguib et al., 2014). Quercitrin significantly reduced the levels of the abovementioned factors in the serum of mice treated with APAP and improved the pathological characteristics of the liver, indicating that quercitrin can alleviate APAP-induced liver injury in mice. The inflammatory response occurs in many pathological processes and thus becomes one of the important indicators of cell injury (Jaeschke and Ramachandran, 2020). Studies have demonstrated that oxidative stress caused by APAP leads to necrosis of hepatocytes and the release of damage-associated molecular patterns (DAMPs), including HMGB1, mtDNA, nuclear DNA, and others (Jaeschke et al., 2013). DAMPs can stimulate various inflammatory cells, mainly liver macrophages, thereby triggering pro-inflammatory transcriptional activation and the release of inflammatory mediators, such as IL-6 and TNF-α (Ramachandran et al., 2018; Wu et al., 2018; Shan and Ju, 2019). Therefore, the inflammatory response can represent the severity of APAP-induced acute liver injury. In this study, the levels of IL-6 and TNF-α in the serum of mice treated with quercitrin were decreased, indicating a lower degree of liver injury. This again suggested that quercitrin could attenuate APAP-induced acute liver injury.
ROS, including superoxide anion, singlet oxygen, hydroxyl radical, and hydrogen peroxide, can cause serious damage to nucleic acids, proteins, and membrane structures by increasing oxidative stress (Zorov et al., 2006). Many pathological oxidative damages are initially caused by ROS (Murphy, 2009). Previously published studies have demonstrated that the ROS content in the liver is significantly increased by APAP (Takemoto et al., 2014; Jiang et al., 2015; Guo et al., 2019). In addition, oxidative stress is also an important cause of APAP-induced acute liver injury (Du et al., 2016). SOD, GSH, GSH-Px, and CAT are the important enzymes for combating oxidative stress in the body (Lei et al., 2015). They protect cells and tissues from oxidative stress by directly antagonizing and neutralizing free radicals and eliminating ROS (Jodynis-Liebert et al., 2005). MDA is an imperative marker of lipid peroxidation, which is frequently used to assess the degree of tissue oxidative damage (Gaweł et al., 2004). Quercitrin reduced ROS levels in the liver of APAP-treated mice. Moreover, it also inhibited the decrease in SOD, GSH, GSH-Px, and CAT levels and the production of MDA in the liver of APAP-treated mice. These findings suggested that quercitrin decreases APAP-induced oxidative stress in mice liver cells through antioxidants.
Mitochondrial complex I is a protein complex which comprises 44 subunits (Guerrero-Castillo et al., 2017). It is also a part of the electron transport chain. Complex I is one of the important sources for the production of ROS (Onukwufor et al., 2019). The decreased activity of complex I causes the leaked electrons to directly react with oxygen or other electron acceptors, triggering a series of reactions under the action of enzymes or nonenzymes to generate various types of reactive oxygen species (Lenaz et al., 2016; Hu et al., 2017; Wang et al., 2017). MCJ protein is a chaperone molecule on the inner membrane of mitochondria, which can interact with complex I, thereby interfering with the formation of the supercomplex formed by complex I. It plays a negative role in the regulation of the respiratory chain (Genova and Lenaz, 2014). A recently published study demonstrated that the excess metabolites produced by APAP can increase the level of MCJ in the liver. This causes the interaction of MCJ with the complex, thereby interfering with the formation of the supercomplex in mitochondria, and leads to reduced ATP production and increased ROS production, which ultimately results in injury to the liver (Barbier-Torres et al., 2017). Thus, restoring the activity of complex I may be an effective treatment for APAP-induced acute liver injury.
A study on the mechanism showed that quercitrin pretreatment significantly inhibited the increase in ROS in L-02 cells induced by APAP. Furthermore, quercitrin also restored the mitochondrial membrane potential and reduced the expression of apoptosis-related proteins AIF and EndoG, which are related to mitochondrial damage (Kapoor et al., 2013). This suggested that quercitrin may prevent mitochondrial dysfunction by regulating the activity of complex I. Complex I activity assays indicated that quercitrin pretreatment restored complex I activity in injured cells. In addition, the presence of rotenone, which is a complex I inhibitor, inhibited the protective effect of quercitrin. This result further confirmed that quercitrin could protect cells by maintaining the activity of complex I and reducing the production of ROS in cells. Finally, the Western blot results demonstrated that quercitrin maintained the expression of complex I in L-02 cells which were treated with APAP. The above results indicate that the maintenance of complex I activity plays a role in quercitrin alleviating APAP-induced liver injury.
In conclusion, our results indicate that quercitrin maintains the activity of complex I by maintaining the expression of complex I, thereby reducing ROS production in mitochondria to prevent oxidative stress and ultimately reduces the cell injury induced by APAP, which indicates that quercitrin has potential value in further clinical applications.
The raw data supporting the conclusions of this article will be made available by the authors, without undue reservation, to any qualified researcher.
The animal study was reviewed and approved by the Experimental Animal Ethics Committee of the Hubei University of Chinese Medicine.
HW and YaY designed the study; WX, ZY, and TW performed the research; SW, YX, and YuY collected and analyzed the data; WX and ZY wrote the paper.
This research was funded by the National Natural Science Foundation of China, grant number 31570343. This study was financially supported by the Key Laboratory of Traditional Chinese Medicine Resources and Chemistry of Hubei Province, Hubei University of Chinese Medicine, Wuhan, Hubei, China.
The authors declare that the research was conducted in the absence of any commercial or financial relationships that could be construed as a potential conflict of interest.
The Supplementary Material for this article can be found online at: https://www.frontiersin.org/articles/10.3389/fphar.2021.586010/full#supplementary-material
Barbier-Torres, L., Iruzubieta, P., Fernández-Ramos, D., Delgado, T. C., Taibo, D., Guitiérrez-de-Juan, V., et al. (2017). The Mitochondrial Negative Regulator MCJ is a Therapeutic Target for Acetaminophen-Induced Liver Injury. Nat. Commun. 8, 2068. doi:10.1038/s41467-017-01970-x
Camuesco, D., Comalada, M., Rodríguez-Cabezas, M. E., Nieto, A., Lorente, M. D., Concha, A., et al. (2004). The Intestinal Anti-Inflammatory Effect of Quercitrin is Associated with an Inhibition in iNOS Expression. Br. J. Pharmacol. 143, 908–918. doi:10.1038/sj.bjp.0705941
Chowdhury, A., Lu, J., Zhang, R., Nabila, J., Gao, H., Wan, Z., et al. (2019). Mangiferin Ameliorates Acetaminophen-Induced Hepatotoxicity Through APAP-Cys and JNK Modulation. Biomed. Pharmacother. 117, 109097. doi:10.1016/j.biopha.2019.109097
Dröse, S., Stepanova, A., and Galkin, A. (2016). Ischemic A/D Transition of Mitochondrial Complex i and its Role in ROS Generation. Biochim. Biophys. Acta (Bba) - Bioenerg. 1857, 946–957. doi:10.1016/j.bbabio.2015.12.013
Du, K., Ramachandran, A., and Jaeschke, H. (2016). Oxidative Stress during Acetaminophen Hepatotoxicity: Sources, Pathophysiological Role and Therapeutic Potential. Redox Biol. 10, 148–156. doi:10.1016/j.redox.2016.10.001
Gaweł, S., Wardas, M., Niedworok, E., and Wardas, P. (2004). [Malondialdehyde (MDA) as a Lipid Peroxidation Marker]. Wiad. Lek. 57, 453–455.
Genova, M. L., and Lenaz, G. (2014). Functional Role of Mitochondrial Respiratory Supercomplexes. Biochim. Biophys. Acta (Bba) - Bioenerg. 1837, 427–443. doi:10.1016/j.bbabio.2013.11.002
Guerrero-Castillo, S., Baertling, F., Kownatzki, D., Wessels, H. J., Arnold, S., Brandt, U., et al. (2017). The Assembly Pathway of Mitochondrial Respiratory Chain Complex I. Cel Metab. 25, 128–139. doi:10.1016/j.cmet.2016.09.002
Guo, H., Sun, J., Li, D., Hu, Y., Yu, X., Hua, H., et al. (2019). Shikonin Attenuates Acetaminophen-Induced Acute Liver Injury via Inhibition of Oxidative Stress and Inflammation. Biomed. Pharmacother. 112, 108704. doi:10.1016/j.biopha.2019.108704
Guo, R., Gu, J., Zong, S., Wu, M., and Yang, M. (2018). Structure and Mechanism of Mitochondrial Electron Transport Chain. Biomed. J. 41, 9–20. doi:10.1016/j.bj.2017.12.001
Hatle, K. M., Gummadidala, P., Navasa, N., Bernardo, E., Dodge, J., Silverstrim, B., et al. (2013). MCJ/DnaJC15, an Endogenous Mitochondrial Repressor of the Respiratory Chain That Controls Metabolic Alterations. Mol. Cell Biol. 33, 2302–2314. doi:10.1128/mcb.00189-13
Hu, H., Nan, J., Sun, Y., Zhu, D., Xiao, C., Wang, Y., et al. (2017). Electron Leak from NDUFA13 within Mitochondrial Complex I Attenuates Ischemia-Reperfusion Injury via Dimerized STAT3. Proc. Natl. Acad. Sci. USA 114, 11908–11913. doi:10.1073/pnas.1704723114
Jaeschke, H., and Bajt, M. L. (2006). Intracellular Signaling Mechanisms of Acetaminophen-Induced Liver Cell Death. Toxicol. Sci. 89, 31–41. doi:10.1093/toxsci/kfi336
Jaeschke, H., and Ramachandran, A. (2020). Mechanisms and Pathophysiological Significance of Sterile Inflammation During Acetaminophen Hepatotoxicity. Food Chem. Toxicol. 138, 111240. doi:10.1016/j.fct.2020.111240
Jaeschke, H., Williams, C. D., McGill, M. R., Xie, Y., and Ramachandran, A. (2013). Models of Drug-Induced Liver Injury for Evaluation of Phytotherapeutics and Other Natural Products. Food Chem. Toxicol. 55, 279–289. doi:10.1016/j.fct.2012.12.063
Jiang, J., Briedé, J. J., Jennen, D. G. J., Van Summeren, A., Saritas-Brauers, K., Schaart, G., et al. (2015). Increased Mitochondrial ROS Formation by Acetaminophen in Human Hepatic Cells Is Associated with Gene Expression Changes Suggesting Disruption of the Mitochondrial Electron Transport Chain. Toxicol. Lett. 234, 139–150. doi:10.1016/j.toxlet.2015.02.012
Jodynis-Liebert, J., Matławska, I., Bylka, W., and Murias, M. (2005). Protective Effect of Aquilegia Vulgaris (L.) on APAP-Induced Oxidative Stress in Rats. J. Ethnopharmacology 97, 351–358. doi:10.1016/j.jep.2004.11.027
Kapoor, R., Rizvi, F., and Kakkar, P. (2013). Naringenin Prevents High Glucose-Induced Mitochondria-Mediated Apoptosis Involving AIF, Endo-G and Caspases. Apoptosis 18, 9–27. doi:10.1007/s10495-012-0781-7
Kuang, Y., Lin, Y., Li, K., Song, W., Ji, S., Qiao, X., et al. (2017). Screening of Hepatoprotective Compounds from Licorice against Carbon Tetrachloride and Acetaminophen Induced HepG2 Cells Injury. Phytomedicine 34, 59–66. doi:10.1016/j.phymed.2017.08.005
Lee, W. M. (2017). Acetaminophen (APAP) Hepatotoxicity-Isn't it Time for APAP to Go Away? J. Hepatol. 67, 1324–1331. doi:10.1016/j.jhep.2017.07.005
Lei, X. G., Zhu, J.-H., Cheng, W.-H., Bao, Y., Ho, Y.-S., Reddi, A. R., et al. (2016). Paradoxical Roles of Antioxidant Enzymes: Basic Mechanisms and Health Implications. Physiol. Rev. 96, 307–364. doi:10.1152/physrev.00010.2014
Lenaz, G., Tioli, G., Falasca, A. I., and Genova, M. L. (2016). Complex I Function in Mitochondrial Supercomplexes. Biochim. Biophys. Acta (Bba) - Bioenerg. 1857, 991–1000. doi:10.1016/j.bbabio.2016.01.013
Li, H., Gao, H., Liu, F., and Liu, J. (2017). Quercitrin Suppresses Hepatocellular Carcinoma Metastasis and Angiogenesis by Targeting the Nrf2 Signaling Pathway. Oncotarget 5. doi:10.18632/oncotarget.23777
Li, X., Jiang, Q., Wang, T., Liu, J., and Chen, D. (2016). Comparison of the Antioxidant Effects of Quercitrin and Isoquercitrin: Understanding the Role of the 6″-OH Group. Molecules 21, 1246. doi:10.3390/molecules21091246
Masubuchi, Y., Suda, C., and Horie, T. (2005). Involvement of Mitochondrial Permeability Transition in Acetaminophen-Induced Liver Injury in Mice. J. Hepatol. 42, 110–116. doi:10.1016/j.jhep.2004.09.015
Mitra, V., and Metcalf, J. (2012). Metabolic Functions of the Liver. Anaesth. Intensive Care Med. 13, 54–55. doi:10.1016/j.mpaic.2011.11.006
Murphy, M. P. (2009). How Mitochondria Produce Reactive Oxygen Species. Biochem. J. 417, 1–13. doi:10.1042/BJ20081386
Naguib, Y. M., Azmy, R. M., Samaka, R. M., and Salem, M. F. (2014). Pleurotus Ostreatus Opposes Mitochondrial Dysfunction and Oxidative Stress in Acetaminophen-Induced Hepato-Renal Injury. BMC Complement. Altern. Med. 14, 494. doi:10.1186/1472-6882-14-494
Onukwufor, J. O., Berry, B. J., and Wojtovich, A. P. (2019). Physiologic Implications of Reactive Oxygen Species Production by Mitochondrial Complex I Reverse Electron Transport. Antioxidants 8, 285. doi:10.3390/antiox8080285
Panche, A. N., Diwan, A. D., and Chandra, S. R. (2016). Flavonoids: an Overview. J. Nutr. Sci. 5, e47. doi:10.1017/jns.2016.41
Raja, S., Ahamed, K. F. H. N., Kumar, V., Mukherjee, K., Bandyopadhyay, A., and Mukherjee, P. K. (2007). Antioxidant Effect of Cytisus Scoparius Against Carbon Tetrachloride Treated Liver Injury in Rats. J. Ethnopharmacology 109, 41–47. doi:10.1016/j.jep.2006.06.012
Ramachandran, A., Visschers, R. G. J., Duan, L., Akakpo, J. Y., and Jaeschke, H. (2018). Mitochondrial Dysfunction as a Mechanism of Drug-Induced Hepatotoxicity: Current Understanding and Future Perspectives. J. Clin. Transl. Res. 4, 75–100. doi:10.18053/jctres.04.201801.005
Shan, Z., and Ju, C. (2019). Hepatic Macrophages in Drug-Induced Liver Injury. Liver Res. 3, 170–175. doi:10.1016/j.livres.2019.11.002
Shao, S.-Y., Yang, Y.-N., Feng, Z.-M., Jiang, J.-S., and Zhang, P.-C. (2017). New Iridoid Glycosides from the Fruits of Forsythia Suspensa and Their Hepatoprotective Activities. Bioorg. Chem. 75, 303–309. doi:10.1016/j.bioorg.2017.10.006
Subramanya, S., Venkataraman, B., Meeran, M., Goyal, S., Patil, C., and Ojha, S. (2018). Therapeutic Potential of Plants and Plant Derived Phytochemicals against Acetaminophen-Induced Liver Injury. Ijms 19, 3776. doi:10.3390/ijms19123776
Takemoto, K., Hatano, E., Iwaisako, K., Takeiri, M., Noma, N., Ohmae, S., et al. (2014). Necrostatin-1 Protects against Reactive Oxygen Species (ROS)-induced Hepatotoxicity in Acetaminophen-Induced Acute Liver Failure. FEBS Open Bio 4, 777–787. doi:10.1016/j.fob.2014.08.007
Truong, V.-L., Ko, S.-Y., Jun, M., and Jeong, W.-S. (2016). Quercitrin from Toona Sinensis (Juss.) M.Roem. Attenuates Acetaminophen-Induced Acute Liver Toxicity in HepG2 Cells and Mice through Induction of Antioxidant Machinery and Inhibition of Inflammation. Nutrients 8, 431. doi:10.3390/nu8070431
Wang, X., Wu, Q., Liu, A., Anadón, A., Rodríguez, J.-L., Martínez-Larrañaga, M.-R., et al. (2017). Paracetamol: Overdose-Induced Oxidative Stress Toxicity, Metabolism, and Protective Effects of Various Compoundsin Vivo and In Vitro. Drug Metab. Rev. 49, 395–437. doi:10.1080/03602532.2017.1354014
Wu, K., Fan, J., Huang, X., Wu, X., and Guo, C. (2018). Hepatoprotective Effects Exerted by Poria Cocos Polysaccharides against Acetaminophen-Induced Liver Injury in Mice. Int. J. Biol. Macromolecules 114, 137–142. doi:10.1016/j.ijbiomac.2018.03.107
Xiong, W.-c., Wu, H.-z., Xiong, Y.-y., Liu, B., Xie, Z.-t., Wu, S.-t., et al. (2020). Network Pharmacology-Based Research of Active Components of Albiziae Flos and Mechanisms of its Antidepressant Effect. Curr. Med. Sci. 40, 123–129. doi:10.1007/s11596-020-2155-7
Yan, M., Huo, Y., Yin, S., and Hu, H. (2018). Mechanisms of Acetaminophen-Induced Liver Injury and its Implications for Therapeutic Interventions. Redox Biol. 17, 274–283. doi:10.1016/j.redox.2018.04.019
Yang, R., Miki, K., He, X., Killeen, M. E., and Fink, M. P. (2009). Prolonged Treatment with N-Acetylcystine Delays Liver Recovery from Acetaminophen Hepatotoxicity. Crit. Care 13, R55. doi:10.1186/cc7782
Keywords: quercitrin, acute liver injury, acetaminophen, complex I, mitochondrial dysfunction, reactive oxygen species
Citation: Xiong W, Yuan Z, Wang T, Wu S, Xiong Y, Yao Y, Yang Y and Wu H (2021) Quercitrin Attenuates Acetaminophen-Induced Acute Liver Injury by Maintaining Mitochondrial Complex I Activity. Front. Pharmacol. 12:586010. doi: 10.3389/fphar.2021.586010
Received: 22 July 2020; Accepted: 19 April 2021;
Published: 05 May 2021.
Edited by:
Raffaele Capasso, University of Naples Federico II, ItalyReviewed by:
Jia-bo Wang, Capital Medical University, ChinaCopyright © 2021 Xiong, Yuan, Wang, Wu, Xiong, Yao, Yang and Wu. This is an open-access article distributed under the terms of the Creative Commons Attribution License (CC BY). The use, distribution or reproduction in other forums is permitted, provided the original author(s) and the copyright owner(s) are credited and that the original publication in this journal is cited, in accordance with accepted academic practice. No use, distribution or reproduction is permitted which does not comply with these terms.
*Correspondence: Yanfang Yang, eXlmMDIwNEBoYnRjbS5lZHUuY24=; Hezhen Wu, aGV6aF93dUAxNjMuY29t
†These authors have contributed equally to this work
Disclaimer: All claims expressed in this article are solely those of the authors and do not necessarily represent those of their affiliated organizations, or those of the publisher, the editors and the reviewers. Any product that may be evaluated in this article or claim that may be made by its manufacturer is not guaranteed or endorsed by the publisher.
Research integrity at Frontiers
Learn more about the work of our research integrity team to safeguard the quality of each article we publish.