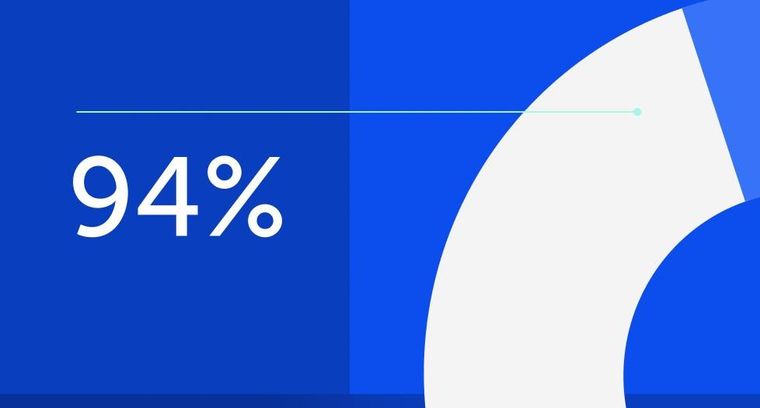
94% of researchers rate our articles as excellent or good
Learn more about the work of our research integrity team to safeguard the quality of each article we publish.
Find out more
ORIGINAL RESEARCH article
Front. Pharmacol., 14 January 2021
Sec. Neuropharmacology
Volume 11 - 2020 | https://doi.org/10.3389/fphar.2020.608887
Mesolimbic dopamine transmission is dysregulated in multiple psychiatric disorders, including addiction. Previous studies found that the endogenous GABAergic steroid (3α,5α)-3-hydroxy-5-pregnan-20-one (allopregnanolone) modulates dopamine levels in the nucleus accumbens and prefrontal cortex. As allopregnanolone is a potent positive allosteric modulator of GABAA receptors, and GABAA receptors can regulate dopamine release, we hypothesized that allopregnanolone would reduce phasic fluctuations in mesolimbic dopamine release that are important in learning and reward processing. We used fast-scan cyclic voltammetry in anesthetized female and male rats to measure dopamine release in the nucleus accumbens evoked by electrical stimulation of the ventral tegmental area, before and after administration of allopregnanolone. Allopregnanolone (7.5–25 mg/kg, IP) reduced evoked dopamine release in both male and female rats, compared to β-cyclodextrin vehicle. In males, all doses of allopregnanolone decreased dopamine transmission, with stronger effects at 15 and 25 mg/kg allopregnanolone. In females, 15 and 25 mg/kg allopregnanolone reduced dopamine release, while 7.5 mg/kg allopregnanolone was no different from vehicle. Since allopregnanolone is derived from progesterone, we hypothesized that high endogenous progesterone levels would result in lower sensitivity to allopregnanolone. Consistent with this, females in proestrus (high progesterone levels) were less responsive to allopregnanolone than females in other estrous cycle stages. Furthermore, 30 mg/kg progesterone reduced evoked dopamine release in males, similar to allopregnanolone. Our findings confirm that allopregnanolone reduces evoked dopamine release in both male and female rats. Moreover, sex and the estrous cycle modulated this effect of allopregnanolone. These results extend our knowledge about the pharmacological effects of neurosteroids on dopamine transmission, which may contribute to their therapeutic effects.
Neuroactive steroids (neurosteroids) are compounds synthesized de novo in neurons that modulate both gene expression and neuronal excitability, the latter through interactions with neurotransmitter receptors (for review, see Porcu et al., 2016; Paul et al., 2020). Neurosteroid levels in serum and brain are dysregulated in multiple psychiatric disorders (e.g., Rapkin et al., 1997; Brambilla et al., 2003; Hellgren et al., 2014; Frau et al., 2020; Hantsoo and Epperson, 2020). Moreover, allopregnanolone, a metabolite of progesterone and a GABAergic neurosteroid, has emerged as a clinically beneficial therapeutic (Milivojevic et al., 2016; Bixo et al., 2017; Meltzer-Brody et al., 2018). For example, progesterone reduced cocaine craving in individuals with cocaine-use disorder (Fox et al., 2013) and this effect was highly correlated with circulating allopregnanolone (Milivojevic et al., 2016).
The mesolimbic dopamine pathway extends from the ventral tegmental area (VTA) to limbic and cortical areas and is involved in complex processes, such as decision making and motivated behavior, and its dysregulation contributes to several psychiatric disorders, including addiction (Volkow et al., 2017). Some strategies of psychoactive treatment aim to directly modify dysregulated dopamine transmission (e.g., German et al., 2015); however, dopamine itself is a difficult therapeutic target due to potential side effects, particularly those affecting movement and motivation (e.g., Kaar et al., 2019). Instead, indirect modulation of dopamine through γ-aminobutyric acid type A (GABAA) receptors (Nikolaus et al., 2018; Lopes et al., 2019; Kramer et al., 2020) may prove to be a useful strategy to regulate dopamine. Benzodiazepines can modulate dopamine (Takada et al., 1993; Finlay et al., 1995; Gómez-A et al., 2017; Brodnik et al., 2019), but these medications can also have both acute and chronic side effects, as well as high dependence and abuse potential (Licata and Rowlett, 2008; Votaw et al., 2019). Previous studies found that neurosteroids can modulate dopamine concentrations. For example, five days of progesterone administration enhanced the alcohol to dose-dependently modulate extracellular dopamine concentrations in the prefrontal cortex (Dazzi et al., 2002). Moreover, the endogenous neurosteroid derived from the hormone deoxycorticosterone, 3α,21-dihydroxy-5α-pregnane-20-one (THDOC) blunted stress-induced increases in dopamine tissue content in the prefrontal cortex (Grobin et al., 1992). Furthermore, intracerebroventricular allopregnanolone also increases tonic dopamine transmission in the nucleus accumbens (NAc) (Rouge-Pont et al., 2002) and modulates tissue content of dopamine (Laconi et al., 2007). However, no studies to date have assessed the regulation of phasic dopamine release–brief dopamine fluctuations resulting from burst-firing–by neurosteroids. This aspect of dopamine release is of interest due to its role in reward-associated learning and addiction (Wightman and Robinson, 2002; Schultz, 2007; Schultz, 2016; Volkow et al., 2017).
As allopregnanolone is inhibitory due to its allosteric actions at GABAA receptors, and based on the above evidence that drugs that enhance GABAA receptor activity reduce dopamine release, we hypothesized that it would inhibit phasic dopamine release. To test this, we used fast-scan cyclic voltammetry, a technique that offers the temporal, spatial, and chemical resolution required to assess fast dopamine release events (Robinson et al., 2003; Robinson et al., 2008). We measured phasic fluctuations in dopamine release evoked by electrical stimulation of the VTA in anesthetized male and female rats before and after intraperitoneal injections of allopregnanolone or β-cyclodextrin vehicle. Progesterone, the precursor to allopregnanolone, has great translational relevance due to its clinical availability when compared to allopregnanolone, and so we also examined its effects on evoked mesolimbic dopamine release. We predicted that both allopregnanolone and progesterone would decrease VTA-evoked dopamine release in the NAc.
Adult male (N = 30, 297 ± 4 g at experiment) and female (N = 27, 216 ± 2 g at experiment) Sprague–Dawley rats (Envigo; Frederick, MD) were used in the present study. Animals were housed in a temperature-controlled environment (21 ± 1°C) with 12-h light/dark cycles and ad libitum access to food and water. Males were housed in groups of 2–3 animals per cage and females in groups of 2–4 per cage. A subset of female rats (n = 19) were assessed for estrous cycle stage by cell morphology after vaginal lavage with 20 µl of saline, immediately after the experiment. Data collection occurred between 1200 and 1700 h each experimental day, for all the male and female animals. Vaginal lavage occurred at the end of the experiment – typically between 1400–1500 h, but occasionally as late as 1700 h. All procedures were approved by the Institutional Animal Care and Use Committee of University of North Carolina at Chapel Hill.
Allopregnanolone, purchased from the late Dr. R. H. Purdy (formerly of the Veterans Medical Research Foundation, San Diego, CA, United States), was added to 45% hydroxypropyl-β-cyclodextrin (Acros Organics, ThermoFisher Scientific, Waltham, MA, #297565000) in water, vortexed, mixed in an ultrasonic water bath for 15–20 min, then stored while continuously stirring at 4°C, and kept for a maximum of two days after preparation. Progesterone (Steraloids, Newport, RI, #Q2600) was added to the 45% hydroxypropyl-β-cyclodextrin solution, vortexed, then stored while continuously stirring at 4°C. The doses of allopregnanolone and progesterone used in the study were selected as doses with neuroactive (anti-seizure) effects but minimal sedative effects in rats (Lonsdale et al., 2006; Wu and Burnham, 2018).
Rats were anesthetized with urethane (50% w/w in saline; 1.2–1.5 g/kg) and secured in a stereotaxic frame on a heated pad. Urethane was chosen due to modest effects on multiple neurotransmitter-gated ion channels (Hara and Harris, 2002Maggi and Meli, 1986) and the lack of effect on dopamine clearance in vivo (Garris et al., 1997; Sabeti et al., 2003). Anterior–posterior (AP), medial–lateral (ML), and dorsal–ventral (DV) positions refer to bregma, and coordinates were obtained from a rat brain atlas (Paxinos and Watson, 1998). A bipolar stimulating electrode (Plastics One, Roanoke, VA, United States, polished tips, 1 mm apart) was placed above the VTA (AP: −5.3, ML: −0.9, DV: −8.1), a recording electrode was placed in the NAc (AP: +1.6, ML: −2.0, DV: −6.2) and an Ag/AgCl reference electrode was implanted in the contralateral cortex. The recording electrode consisted of a single carbon fiber (T650, Thornel/Cytec Industries Inc., Woodland Park, NJ, United States; 6-μm diameter) sealed in a glass capillary (600 µm O.D.). The carbon fiber extended 80–120 µm from the glass seal and was soaked for at least 10 min in isopropyl alcohol to clean the carbon fiber (Bath et al., 2000).
Voltammetric parameters, electrical stimulation parameters, and data acquisition were controlled by a computer using LabVIEW instrumentation software (National Instruments, Austin, TX) as previously described (Shnitko et al., 2016). In brief, voltammetric recordings were made at the carbon-fiber microelectrodes by applying a triangle waveform potential, ramping from −0.4 V to +1.3 V and back to −0.4 V, at a scan rate of 400 V/s. The triangle-waveform was applied at 60 Hz for the first 20 min to condition the electrode, after which the application was reduced to 10 Hz. Dopamine release was evoked by electrical stimulation of the VTA consisting of 24 biphasic, square-wave pulses (60 Hz, 125 μA, 2 ms/phase).
After electrodes were initially placed, dopamine neurons were activated every 2–5 min via electrical stimulation to the VTA and dopamine release was detected at the carbon-fiber electrode. Current was confirmed to be due to oxidation of dopamine and reduction of the ortho-quinone via the background-subtracted cyclic voltammogram. Evoked dopamine release was optimized by moving the stimulating and/or carbon-fiber electrodes ventrally at 100-µm increments. The average final DV placement of the stimulating electrode in the VTA was 8.3 (range: 8.1–8.9 mm) in females and 8.5 (range: 8.1–8.9 mm) in males. The average final DV placement of the carbon-fiber electrode in the NAc was 6.5 mm (range: 6.2–7.1 mm) in females and 6.8 (range: 6.2–7.6 mm) in males. The experimental recording began when evoked dopamine release reached a minimum of 2.5 nA and when the signal-to-noize ratio was higher than 25. Thereafter, dopamine release was evoked at 5-min intervals, and an average of nine electrically-evoked signals were collected before starting the experiment in order to confirm stability of the signal. Next, to ensure that injections did not alter the dopamine signal and that the signal was stable, animals received two saline injections (IP), 15 min apart. Finally, animals received allopregnanolone, progesterone, or vehicle (IP), followed by 60 min of electrochemical recording. The average calibration factor used to estimate dopamine concentration from current was 1 nA = 0.103 ± 0.017 µM, extracted from a library of 107 in vivo electrodes used in the lab that were calibrated in a flow-cell with 1 µM DA in TRIS buffer (Logman et al., 2000; Robinson et al., 2009).
Evoked dopamine was characterized by calculating [DA]max (maximum dopamine concentration) and T½ (the time for dopamine to clear to half maximum concentration) as measures of dopamine release and clearance, respectively (Yorgason et al., 2011; Shnitko et al., 2016). As the effect of neurosteroids on dopamine release was expected to be inhibitory, it was critical that we used stringent inclusion criteria of electrode stability, so that reductions in signal could be attributed to neurosteroid action rather than to time. For inclusion of data from a rat, the nine evoked dopamine signals prior to the injection of neurosteroids or vehicle (45 min, including the two saline injections) were required to vary <15% and exhibit a signal-to-noize ratio >25.
To broadly compare evoked dopamine release and clearance at baseline by sex or by estrous cycle stage, we used Mann-Whitney U tests or Kruskal-Wallis one-way ANOVA on ranks due to the non-normal distribution of the data. For these comparisons, we used raw (not normalized) [DA]max and T½ values. Neurosteroid effects on evoked dopamine over time were assessed using repeated-measures (RM) ANOVA, followed by Holm-Sidak post-hoc comparisons, as appropriate. For these analyses, we used raw T½ values and normalized [DA]max values. Specifically, the raw [DA]max data did not pass the Shapiro-Wilk normality test, and to account for individual variability due to electrode placement or length, [DA]max data were normalized within each rat to the evoked dopamine levels during the saline injections (six evoked signals immediately preceding the neurosteroid or vehicle injections); the transformed data passed the normality test.
For statistical analysis, data were pooled into 15-min bins (three evoked signals/bin), resulting in seven time points (three pre-injection, four post-injection). For the animals that received allopregnanolone (Allo) or vehicle, the pre-injection bins were indicated as BL (basal), Sal (saline) 1, and Sal 2, and the post-injection as Allo 1, Allo 2, Allo 3, and Allo 4. For those that received progesterone (Prog), the bins were BL, Sal 1 and Sal 2, followed by Prog 1 to Prog 4. Effect sizes were considered small, medium, or large if they corresponded to partial η2 of at least 0.0099, 0.0588, and 0.1379, respectively, based on values of ƒ as described by Cohen (Cohen, 1988). Analyses were calculated using SigmaPlot for Windows v. 11 (Systat Software, Inc. San Jose, CA), graphs were made using GraphPad Prism 8.0.0 (GraphPad Software, San Diego, CA), and results are shown as mean ± SEM.
Allopregnanolone (or vehicle) was administered at 7.5, 15, and 25 mg/kg to separate groups of male and female rats. We first compared baseline dopamine signals between males and females and found no difference in [DA]max or T½; these data are reported in detail in the Supplementary Material. Next, as an initial analysis of the effects of dose by sex, we analyzed [DA]max (normalized to saline injection, as described in Statistical Analysis) at the final time bin (45–60 min post-injection, bin Allo 4) representing the maximal effect of allopregnanolone. Allopregnanolone reduced evoked dopamine release at multiple doses in both males and females (Figure 1). The 2-way ANOVA revealed significant main effects of dose (F3,45 = 3.1,
FIGURE 1. Allopregnanolone reduced evoked dopamine release at multiple doses in both males and females. We compared the effect of allopregnanolone and vehicle at 45–60 min post-injection in male and female rats. Evoked dopamine release is displayed as percent baseline (dotted line). We found a main effect of sex, in that males showed lower evoked dopamine than females when collapsed across dose. We also observed a main effect of dose, in that 15 and 25 mg/kg allopregnanolone (collapsed across sex) induced significantly lower evoked dopamine concentrations than vehicle (n = 6–8 per group).
In male rats, all doses of allopregnanolone reduced [DA]max as compared to the vehicle group, although the timing of this effect differed (Figure 2A). The two-way RM ANOVA yielded a significant interaction between dose and time (F18,132 = 2.7,
FIGURE 2. Allopregnanolone decreases evoked dopamine release in the nucleus accumbens of male and female rats. The dashed line indicates the moment when allopregnanolone (or vehicle) was injected. Data are normalized to the average [DA]max after saline injections (SAL 1 and SAL 2); see methods for descriptions of time bins. (A) All three doses of allopregnanolone effectively decreased the evoked dopamine release into the NAc of male rats compared to vehicle group (between-subjects) and compared to the SAL 2 bin (within-subjects). *p < 0.05 7.5 mg/kg vs. vehicle; &p < 0.05 15 mg/kg vs. vehicle; #p < 0.05 25 mg/kg vs. vehicle (Vehicle, n = 6; 7.5 mg/kg, n = 6; 15 mg/kg, n = 7; 25 mg/kg, n = 7). (B) The higher doses of allopregnanolone reduced evoked dopamine transmission in females; all ALLO bins for the 15 and 25 mg/kg doses were significantly lower than SAL 2 bin (within-subjects), and ALLO 2 was significantly lower in 15 and 25 mg/kg groups compared to the vehicle group (between-subjects). &p < 0.05 15 mg/kg vs. vehicle; #p < 0.05 25 mg/kg vs. vehicle (Vehicle, n = 6; 7.5 mg/kg, n = 7; 15 mg/kg, n = 8; 25 mg/kg, n = 6).
FIGURE 3. Examples of evoked dopamine release from individual rats before and after allopregnanolone. Electrochemical scans are shown from a single rat in each group (sex by dose) both before and one hour after the injection of 0.0 (vehicle), 7.5, 15, or 25 mg/kg allopregnanolone. Males are shown on the left and females on the right, with doses in ascending order from top to bottom. In the color plots, the oxidation and reduction currents are expressed in color and plotted by applied potential (y-axis) over time (x-axis). Electrical stimulation of the VTA is indicated by the white triangles, and oxidation of dopamine can be observed as changes in current at approximately 650 mV.
As we observed that 7.5 mg/kg allopregnanolone significantly reduced evoked [DA]max, we conducted a follow-up experiment with a lower dose–5 mg/kg – in a separate group of male rats (n = 6). As described in the Supplementary Material, within-subject analysis confirmed that 5 mg/kg allopregnanolone reduced evoked dopamine release in the final three Allo bins compared to Sal 2 (one-way RM ANOVA: F6,30 = 11.1,
In female rats, only the 15 and 25 mg/kg doses effectively reduced evoked dopamine release (Figure 2B). The two-way RM ANOVA of [DA]max revealed a significant interaction between dose and time (F18,138 = 1.8,
It is possible that the effect of allopregnanolone to decrease dopamine release in the NAc was affected by hormonal fluctuations during the estrous cycle. In rats, the estrous cycle includes proestrus, estrus, metestrus, and diestrus stages, and proestrus is the phase when the plasma levels of progesterone (a precursor to allopregnanolone) are highest (Smith et al., 1975). Moreover, studies have reported higher dopamine release during estrus (Xiao and Becker, 1994; Cummings et al., 2014; Calipari et al., 2017). Thus, we first compared baseline dopamine signals among females in proestrus (n = 7), estrous (n = 5) and metestus/diestrus (n = 7) in the subset of rats for which we determined cycle stage. Dopamine release was highest in rats in estrus, as compared to those in proestrus or metestus/diestrus, although this difference did not reach significance (H2 = 4.29, p < 0.12). We also found no significant difference in T½ across cycle stage; these data are reported in detail in the Supplementary Material. Next, we compared the effect of allopregnanolone on evoked dopamine in a subset of female rats that were assessed for cycle stage (Figure 4). Specifically, we pooled females from the 15 and 25 mg/kg dose groups, and compared rats that were confirmed to be in proestrus (high progesterone, n = 4) to those confirmed to be in diestrus, metestrus or estrus stages (low progesterone, n = 6). For this follow-up comparison, we did not include rats from the vehicle control group, as it contained rats that were in various stages of the estrous cycle, and we did not have sufficient numbers to separate them into proestrus and other stages. A two-way RM ANOVA yielded a significant interaction between time and cycle stage (F6,42 = 3.99,
FIGURE 4. Females in proestrus (high progesterone) are less sensitive to allopregnanolone when compared to females in other estrous stages (low progesterone). The dashed line indicates the moment when allopregnanolone (15 or 25 mg/kg) was injected. Data are normalized to the average [DA]max after saline injections (SAL 1 and SAL 2); see methods for descriptions of time bins. *p < 0.05 low vs. high progesterone levels (Low progesterone, n = 6; High progesterone, n = 4).
As a proof of principle, to assess the effect of allopregnanolone’s precursor on dopamine transmission, we administered 30 mg/kg progesterone to male rats and measured evoked dopamine release in the NAc (Figure 5). Male rats were selected for this experiment due to their greater sensitivity to allopregnanolone as compared to females. For this proof-of-principle study, we did not run a separate vehicle control group, and instead used a within-subject analysis to assess progesterone effects. A one-way RM ANOVA confirmed that progesterone decreased evoked [DA]max (F6,24 = 34.3,
FIGURE 5. Progesterone decreases evoked dopamine release in the nucleus accumbens of male rats. The dashed line indicates the moment when the 30 mg/kg of progesterone was injected. *p < 0.05 vs. SAL 2 (n = 5).
In the present study, we investigated the action of allopregnanolone on the mesolimbic dopamine system, and specifically on phasic fluctuations in dopamine release that are associated with motivated behavior (Schultz, 2016, Schultz, 2019). As allopregnanolone is inhibitory due to its allosteric actions at GABAA receptors, and as other GABAergic compounds reduce evoked dopamine release (Gómez-A et al., 2017; Brodnik et al., 2019), we hypothesized that allopregnanolone would inhibit mesolimbic dopamine release. Our findings confirmed that allopregnanolone reduced electrically evoked dopamine release in the NAc of both male and female rats. Moreover, allopregnanolone action to reduce the evoked dopamine release was stronger in males than in females and stronger in females during the estrous stage characterized by low progesterone.
The ability of allopregnanolone to reduce evoked [DA]max is likely to be due to its action at GABAA receptors, although future studies are needed to confirm this hypothesis. This interpretation is supported by other studies showing that a variety of GABA agonists can reduce mesolimbic dopamine transmission (Smolders et al., 1995; Pitman et al., 2014; Gómez-A et al., 2017; Schelp et al., 2018; Brodnik et al., 2019). More specifically, allopregnanolone blunted dopamine release in an ex vivo slice preparation, an action prevented by the GABAA receptor antagonist bicuculline (Knight et al., 2012). An open question is whether allopregnanolone regulates dopamine release via specific GABAA receptor subtypes. While it is likely that the allopregnanolone effects on dopamine release reported here arise from neurosteroid action at GABAA receptors, we did not confirm this with additional pharmacology. For example, one could block GABAA transmission with bicuculline, which should also prevent any effect of allopregnanolone. However, manipulation of the GABA receptors in vivo would have broad effects and may act at different sites on the GABA receptor, both of which are likely to make its effect on the allopregnanolone response difficult to interpret. A separate but related limitation is that dopamine measurements in the present study were made in anesthetized rats, which may itself alter GABAergic dynamics and, by extension, allopregnanolone effects. Future studies can replicate this study in awake rats to confirm the sensitivity of male and female rats to allopregnanolone.
While GABAA receptors are widely expressed throughout the brain, neurosteroids have greater affinity in some regions for receptors containing the δ subunit (for review, see Belelli and Lambert, 2005), which occur extrasynaptically and have been shown to mediate GABAergic tonic inhibition in different brain regions (Carver et al., 2014; Marowsky and Vogt, 2014), including the VTA (Vashchinkina et al., 2014). While systemic allopregnanolone can produce conditioned place preference (Finn et al., 1997), intracerebroventricular infusion of allopregnanolone produced conditioned place aversion in rats (Beauchamp et al., 2000), and it was shown that this effect was dependent on δ subunits in the VTA (Vashchinkina et al., 2014). Intracerebroventricular allopregnanolone also prevented the increase in extracellular dopamine concentrations in the NAc induced by foot-shock (Motzo et al., 1996). The NAc is also rich in GABAA δ-containing receptors (Maguire et al., 2014). Thus, δ-containing GABAA receptors in the VTA and NAc may regulate mesolimbic dopamine release and participate in the effects of allopregnanolone observed in our study, although future experiments are required to confirm a role of δ subunits. For instance, genetic models such as knock-out mice can be used to determine whether allopregnanolone affects dopamine release through a specific receptor subtype.
We observed that allopregnanolone reductions in evoked dopamine release were more robust in males than in females. Specifically, all doses of allopregnanolone (7.5, 15, and 25 mg/kg) reduced evoked dopamine release compared to vehicle in males, while only the two higher doses reduced evoked dopamine release compared to vehicle controls in females. Other studies confirm similar sex differences in allopregnanolone action; for example, females that received allopregnanolone were less sedated and recovered more rapidly from the neurosteroid effect than males at the same dose (Irwin et al., 2015). Similarly, the α4β3δ GABAA receptor agonist THIP induced significantly greater tonic current in the substantia nigra pars reticulata neurons of young male rats than of females at the same age (Chudomel et al., 2015). We can speculate that the sex variance on the inhibitory action of allopregnanolone could be explained, at least in part, by a greater expression of GABAA receptors in males compared to females (Juptner and Hiemke, 1990). Alternatively, circulating progesterone in cycling females may also contribute to the sex difference, as investigated below.
We found that estrous cycle in females influenced sensitivity to allopregnanolone action. Relevant to this study, there are two peaks of progesterone secretion from the ovaries into blood circulation during the estrous cycle. The first peak of progesterone is small and occurs from the newly formed corporea lutea during the afternoon of metestrus, while the second and larger peak of progesterone arises from the granulosa cells of the preovulatory follicle at proestrous (Nequin et al., 1979; Freeman, 2006). Here we observed that during proestrus, when the circulating levels of progesterone are highest, evoked dopamine release was less affected by allopregnanolone than during other phases of the cycle. Progesterone may directly influence dopamine release by inducing dephosphorylation and inactivation of tyrosine hydroxylase in dopamine neurons (Arbogast and Voogt, 2002), a limiting step in dopamine synthesis, which may result in less dopamine available for release. However, in the present study we did not observe significant differences in baseline dopamine release between females in proestrus versus other stages of the estrous cycle with lower progesterone levels (see Supplementary Material), suggesting that any changes in synthesis did not impact readily-releasable dopamine. Indeed, reported changes in dopamine release across the estrous cycle are tied to fluctuations in estrogen rather than progesterone (Xiao and Becker, 1994; Cummings et al., 2014; Calipari et al., 2017). One straightforward explanation of the present data is that higher levels of circulating progesterone would lead to higher levels of GABAergic metabolites allopregnanolone and THDOC, rendering exogenous allopregnanolone less effective to further alter dopamine release. Moreover, in addition to oscillations in the availability and synthesis of neurosteroids across the ovarian cycle, the expression of δ-containing GABAA receptors in multiple brain regions also fluctuates across the ovarian cycle (Griffiths and Lovick, 2005; Lovick et al., 2005; Maguire et al., 2005; Hantsoo and Epperson, 2020). Such fluctuation may contribute to observations in women across the menstrual cycle; for example, high levels of circulating progesterone during the luteal phase of the menstrual cycle was shown to decrease stress-induced cocaine craving and anxiety (Sinha et al., 2007), phenotypes linked to dopamine and GABA, respectively.
Similarly to allopregnanolone, progesterone reduced evoked dopamine release in males, which is consistent with conversion to its metabolite allopregnanolone. A caveat is that this was a “proof of principle” study and was missing a separate vehicle group. Other researchers have demonstrated that progesterone modulates several types of receptors, but can just activate the GABAA receptor after its conversion to allopregnanolone or other GABAergic metabolites (Callachan et al., 1987). Similar to allopregnanolone, progesterone can be dysregulated in psychiatric disorders (for review, see Bristot et al., 2014). For example, post-traumatic stress disorder in women is associated with a block in conversion of progesterone to allopregnanolone (Pineles et al., 2018). Moreover, progesterone administration to cocaine-dependent subjects increased allopregnanolone in plasma (Milivojevic et al., 2019), normalized cortisol levels, improved mood, and reduced cocaine craving (Milivojevic et al., 2016). Thus, progesterone can elevate allopregnanolone levels in humans, highlighting both neurosteroids as potentially important clinical therapeutics.
The data reported here suggest that females are less sensitive to allopregnanolone regulation of dopamine release, particularly when females are in proestrus, the phase of the rodent cycle accompanied by the highest surge in progesterone. We speculate that higher levels of circulating progesterone, and therefore higher levels of endogenous progesterone metabolites allopregnanolone and THDOC, underlies the lower sensitivity to the effects of exogenous allopregnanolone, due to observations that these neurosteroids can down-regulate various GABAA receptors (Concas et al., 1998; Smith et al., 1998; Belelli and Lambert, 2005, Smith et al., 2007; Wang, 2011). Indeed, THDOC can also regulate dopamine release (Grobin et al., 1992). However, these effects will need to be confirmed in multiple ways. First, the estrous cycle data were determined by a one-time inspection of vaginal cell morphology. A better determination of cycle stage would include assessment over multiple days to track the cycle, blood estradiol and progesterone levels, or ovarian morphology. This would allow full dose-response curves of allopregnanolone and other neuroactive steroids to be conducted in rats at different stages of the estrous cycle. Second, the hypothesis that higher circulating levels of progesterone elevate allopregnanolone levels in blood and/or brain will need to be tested in both in intact, cycling females, and in ovariectomized females administered physiological levels of progesterone. A third area of inquiry would be to study the interaction of progesterone, allopregnanolone and estradiol across the rodent estrous cycle, as estradiol is also known to enhance dopamine release (Xiao and Becker, 1994).
It is known that deficits in GABAergic neurotransmission may contribute to some psychiatric disorders that often include dysregulation of dopamine transmission, such as depression, anxiety, and addiction (Mohler, 2012; Stephens et al., 2017; Duman et al., 2019). Neurosteroids such as allopregnanolone are a promising approach to modulate GABAergic systems to treat psychiatric disorders and the present study extends our understanding of the neuropharmacology of exogenous neurosteroid administration.
The raw data supporting the conclusions of this article will be made available by the authors, without undue reservation.
The animal study was reviewed and approved by Institutional Animal Care and Use Committee of University of North Carolina at Chapel Hill.
AD: Project administration; Investigation; Validation; Formal analysis; Writing-Original Draft; Writing-Review and Editing GM: Project administration; Investigation; Formal analysis; Writing-Review and Editing MM: Investigation; Formal analysis; Writing-Original Draft; Writing-Review and Editing AG-A: Supervision; Investigation; Writing-Review and Editing TO’B: Resources; Writing-Review and Editing CC: Funding acquisition; Conceptualization; Writing-Review and Editing AM: Funding acquisition; Conceptualization; Resources; Writing - Review and Editing DR: Funding acquisition; Conceptualization; Formal analysis; Writing-Original Draft Writing-Review and Editing.
This research was funded by NIH NC TraCS UL1TR001111 project 2KR1011813, NIH P60 AA011605, a Foundation of Hope (Raleigh, NC, United States) research award, and Bowles Center for Alcohol Studies at the University of North Carolina. MHM was supported on “UNC PREP in the Biomedical Sciences” (NIH R25 GM089569). AD and GM were supported on Postdoctoral Program Abroad Fellowships (CAPES)—Coordination of Improvement of Higher Education Personnel (Brazil).
The authors declare that the research was conducted in the absence of any commercial or financial relationships that could be construed as a potential conflict of interest.
The Supplementary Material for this article can be found online at: https://www.frontiersin.org/articles/10.3389/fphar.2020.608887/full#supplementary-material.
AP, anterior-posterior; [DA]max, maximum dopamine concentration; DV, dorsal-ventral; GABA, γ-aminobutyric acid; ML, medial-lateral; NAc, nucleus accumbens; VTA, ventral tegmental area
Arbogast, L. A., and Voogt, J. L. (2002). Progesterone induces dephosphorylation and inactivation of tyrosine hydroxylase in rat hypothalamic dopaminergic neurons. Neuroendocrinology 75, 273–281. doi:10.1159/000057336
Bath, B. D., Michael, D. J., Trafton, B. J., Joseph, J. D., Runnels, P. L., and Wightman, R. M. (2000). Subsecond adsorption and desorption of dopamine at carbon-fiber microelectrodes. Anal. Chem. 72, 5994–6002. doi:10.1021/ac000849y
Beauchamp, M. H., Ormerod, B. K., Jhamandas, K., Boegman, R. J., and Beninger, R. J. (2000). Neurosteroids and reward: allopregnanolone produces a conditioned place aversion in rats. Pharmacol. Biochem. Behav. 67, 29–35. doi:10.1016/s0091-3057(00)00299-9
Belelli, D., and Lambert, J. J. (2005). Neurosteroids: endogenous regulators of the GABA(A) receptor. Nat. Rev. Neurosci. 6, 565–575. doi:10.1038/nrn1703
Bixo, M., Ekberg, K., Poromaa, I. S., Hirschberg, A. L., Jonasson, A. F., Andreen, L., et al. (2017). Treatment of premenstrual dysphoric disorder with the GABAA receptor modulating steroid antagonist Sepranolone (UC1010)-a randomized controlled trial. Psychoneuroendocrinology 80, 46–55. doi:10.1016/j.psyneuen.2017.02.031
Brambilla, F., Biggio, G., Pisu, M. G., Bellodi, L., Perna, G., Bogdanovich-Djukic, V., et al. (2003). Neurosteroid secretion in panic disorder. Psychiatr. Res. 118, 107–116. doi:10.1016/s0165-1781(03)00077-5
Bristot, G., Ascoli, B., Gubert, C., Panizzutti, B., Kapczinski, F., and Rosa, A. R. (2014). Progesterone and its metabolites as therapeutic targets in psychiatric disorders. Expert Opin. Ther. Targets 18, 679–690. doi:10.1517/14728222.2014.897329
Brodnik, Z. D., Batra, A., Oleson, E. B., and Espana, R. A. (2019). Local GABAA receptor-mediated suppression of dopamine release within the nucleus accumbens. ACS Chem. Neurosci. 10, 1978–1985. doi:10.1021/acschemneuro.8b00268
Calipari, E. S., Juarez, B., Morel, C., Walker, D. M., Cahill, M. E., Ribeiro, E., et al. (2017). Dopaminergic dynamics underlying sex-specific cocaine reward. Nat. Commun. 8, 13877. doi:10.1038/ncomms13877
Callachan, H., Cottrell, G. A., Hather, N. Y., Lambert, J. J., Nooney, J. M., and Peters, J. A. (1987). Modulation of the GABAA receptor by progesterone metabolites. Proc. R. Soc. Lond. B Biol. Sci. 231, 359–369. doi:10.1098/rspb.1987.0049
Carver, C. M., Wu, X., Gangisetty, O., and Reddy, D. S. (2014). Perimenstrual-like hormonal regulation of extrasynaptic δ-containing GABAA receptors mediating tonic inhibition and neurosteroid sensitivity. J. Neurosci. 34, 14181–14197. doi:10.1523/JNEUROSCI.0596-14.2014
Chudomel, O., Hasson, H., Bojar, M., Moshe, S. L., and Galanopoulou, A. S. (2015). Age- and sex-related characteristics of tonic GABA currents in the rat substantia nigra pars reticulata. Neurochem. Res. 40, 747–757. doi:10.1007/s11064-015-1523-3
Cohen, J. (1988). Statistical power analysis for the behavioral sciences. Hillsdale, NJ: Lawrence Erlabaum Associates.
Concas, A., Mostallino, M. C., Porcu, P., Follesa, P., Barbaccia, M. L., Trabucchi, M., et al. (1998). Role of brain allopregnanolone in the plasticity of gamma-aminobutyric acid type A receptor in rat brain during pregnancy and after delivery. Proc. Natl. Acad. Sci. USA 95, 13284–13289. doi:10.1073/pnas.95.22.13284
Cummings, J. A., Jagannathan, L., Jackson, L. R., and Becker, J. B. (2014). Sex differences in the effects of estradiol in the nucleus accumbens and striatum on the response to cocaine: neurochemistry and behavior. Drug Alcohol Depend. 135, 22–28. doi:10.1016/j.drugalcdep.2013.09.009
Dazzi, L., Serra, M., Seu, E., Cherchi, G., Pisu, M. G., Purdy, R. H., et al. (2002). Progesterone enhances ethanol-induced modulation of mesocortical dopamine neurons: antagonism by finasteride. J. Neurochem. 83, 1103–1109. doi:10.1046/j.1471-4159.2002.01218.x
Duman, R. S., Sanacora, G., and Krystal, J. H. (2019). Altered connectivity in depression: GABA and glutamate neurotransmitter deficits and reversal by novel treatments. Neuron 102, 75–90. doi:10.1016/j.neuron.2019.03.013
Finlay, J. M., Zigmond, M. J., and Abercrombie, E. D. (1995). Increased dopamine and norepinephrine release in medial prefrontal cortex induced by acute and chronic stress: effects of diazepam. Neuroscience 64, 619–628. doi:10.1016/0306-4522(94)00331-x
Finn, D. A., Phillips, T. J., Okorn, D. M., Chester, J. A., and Cunningham, C. L. (1997). Rewarding effect of the neuroactive steroid 3 alpha-hydroxy-5 alpha-pregnan-20-one in mice. Pharmacol. Biochem. Behav. 56, 261–264. doi:10.1016/s0091-3057(96)00218-3
Fox, H. C., Sofuoglu, M., Morgan, P. T., Tuit, K. L., and Sinha, R. (2013). The effects of exogenous progesterone on drug craving and stress arousal in cocaine dependence: impact of gender and cue type. Psychoneuroendocrinology 38, 1532–1544. doi:10.1016/j.psyneuen.2012.12.022
Frau, R., Traccis, F., and Bortolato, M. (2020). Neurobehavioural complications of sleep deprivation: shedding light on the emerging role of neuroactive steroids. J. Neuroendocrinol. 32, e12792. doi:10.1111/jne.12792
Freeman, M. E. (2006). The neuroendocrine control of the ovarian cycle in the rat. In: Knobil, E., Nieill, J. (Eds.), The Physiology of Reproduction. Academic Press, New York, . 2327–2388.
Garris, P. A., Christensen, J. R., Rebec, G. V., and Wightman, R. M. (1997). Real-time measurement of electrically evoked extracellular dopamine in the striatum of freely moving rats. J. Neurochem. 68, 158–161. doi:10.1046/j.1471-4159.1997.68010152.x
German, C. L., Baladi, M. G., McFadden, L. M., Hanson, G. R., and Fleckenstein, A. E. (2015). Regulation of the dopamine and vesicular monoamine transporters: pharmacological targets and implications for disease. Pharmacol. Rev. 67, 1005–1024. doi:10.1124/pr.114.010397
Gómez-A, A., Fiorenza, A. M., Boschen, S. L., Sugi, A. H., Beckman, D., Ferreira, S. T., et al. (2017). Diazepam inhibits electrically evoked and tonic dopamine release in the nucleus accumbens and reverses the effect of amphetamine. ACS Chem. Neurosci. 8, 300–309. doi:10.1021/acschemneuro.6b00358
Griffiths, J. L., and Lovick, T. A. (2005). GABAergic neurones in the rat periaqueductal grey matter express alpha4, beta1 and delta GABAA receptor subunits: plasticity of expression during the estrous cycle. Neuroscience 136, 457–466. doi:10.1016/j.neuroscience.2005.08.013
Grobin, A. C., Roth, R. H., and Deutch, A. Y. (1992). Regulation of the prefrontal cortical dopamine system by the neuroactive steroid 3a,21-dihydroxy-5a-pregnane-20-one. Brain Res. 578, 351–356. doi:10.1016/0006-8993(92)90270-J
Hantsoo, L., and Epperson, C. N. (2020). Allopregnanolone in premenstrual dysphoric disorder (PMDD): evidence for dysregulated sensitivity to GABA-A receptor modulating neuroactive steroids across the menstrual cycle. Neurobiol. Stress 12, 100213. doi:10.1016/j.ynstr.2020.100213
Hara, K., and Harris, R. A. (2002). The anesthetic mechanism of urethane: the effects on neurotransmitter-gated ion channels. Anesth. Analg. 94, 313–318. doi:10.1097/00000539-200202000-00015
Hellgren, C., Akerud, H., Skalkidou, A., Backstrom, T., and Sundstrom-Poromaa, I. (2014). Low serum allopregnanolone is associated with symptoms of depression in late pregnancy. Neuropsychobiology 69, 147–153. doi:10.1159/000358838
Irwin, R. W., Solinsky, C. M., Loya, C. M., Salituro, F. G., Rodgers, K. E., Bauer, G., et al. (2015). Allopregnanolone preclinical acute pharmacokinetic and pharmacodynamic studies to predict tolerability and efficacy for Alzheimer’s disease. PLoS One 10, e0128313. doi:10.1371/journal.pone.0128313
Juptner, M., and Hiemke, C. (1990). Sex differences in GABAA receptor binding in rat brain measured by an improved in vitro binding assay. Exp. Brain Res. 81, 297–302. doi:10.1007/BF00228119
Kaar, S. J., Natesan, S., McCutcheon, R., and Howes, O. D. (2019). Antipsychotics: mechanisms underlying clinical response and side-effects and novel treatment approaches based on pathophysiology. Neuropharmacology 172, 107704. doi:10.1016/j.neuropharm.2019.107704
Knight, S. R., Davidson, C., Young, A. M., and Gibson, C. L. (2012). Allopregnanolone protects against dopamine-induced striatal damage after in vitro ischaemia via interaction at GABA A receptors. J. Neuroendocrinol. 24, 1135–1143. doi:10.1111/j.1365-2826.2012.02319.x
Kramer, P. F., Twedell, E. L., Shin, J. H., Zhang, R., and Khaliq, Z. M. (2020). Axonal mechanisms mediating gamma-aminobutyric acid receptor type A (GABA-A) inhibition of striatal dopamine release. Elife 9, e55729. doi:10.7554/eLife.55729
Laconi, M. R., Reggiani, P. C., Penissi, A., Yunes, R., and Cabrera, R. J. (2007). Allopregnanolone modulates striatal dopamingergic activity of rats under different gonadal hormones conditions. Neurol. Res. 29, 622–627. doi:10.1179/016164107X166281
Licata, S. C., and Rowlett, J. K. (2008). Abuse and dependence liability of benzodiazepine-type drugs: GABA(A) receptor modulation and beyond. Pharmacol. Biochem. Behav. 90, 74–89. doi:10.1016/j.pbb.2008.01.001
Logman, M. J., Budygin, E. A., Gainetdinov, R. R., and Wightman, R. M. (2000). Quantitation of in vivo measurements with carbon fiber microelectrodes. J. Neurosci. Methods 95, 95–102. doi:10.1016/S0165-0270(99)00155-7
Lonsdale, D., Nylen, K., and McIntyre Burnham, W. (2006). The anticonvulsant effects of progesterone and its metabolites on amygdala-kindled seizures in male rats. Brain Res. 1101, 110–116. doi:10.1016/j.brainres.2006.05.005
Lopes, E. F., Roberts, B. M., Siddorn, R. E., Clements, M. A., and Cragg, S. J. (2019). Inhibition of nigrostriatal dopamine release by striatal GABAA and GABAB receptors. J. Neurosci. 39, 1058–1065. 10.1523/JNEUROSCI.2028-18.2018
Lovick, T. A., Griffiths, J. L., Dunn, S. M., and Martin, I. L. (2005). Changes in GABA(A) receptor subunit expression in the midbrain during the oestrous cycle in Wistar rats. Neuroscience 131, 397–405. doi:10.1016/j.neuroscience.2004.11.010
Maggi, C. A., and Meli, A. (1986). Suitability of urethane anesthesia for physiopharmacological investigations in various systems. Part 1: general considerations. Experientia 42, 109–114. doi:10.1007/BF01952426
Maguire, J. L., Stell, B. M., Rafizadeh, M., and Mody, I. (2005). Ovarian cycle-linked changes in GABA(A) receptors mediating tonic inhibition alter seizure susceptibility and anxiety. Nat. Neurosci. 8, 797–804. doi:10.1038/nn1469
Maguire, E. P., Macpherson, T., Swinny, J. D., Dixon, C. I., Herd, M. B., Belelli, D., et al. (2014). Tonic inhibition of accumbal spiny neurons by extrasynaptic α4βδ GABAA receptors modulates the actions of psychostimulants. J. Neurosci. 34, 823–838. doi:10.1523/JNEUROSCI.3232-13.2014
Marowsky, A., and Vogt, K. E. (2014). Delta-subunit-containing GABAA-receptors mediate tonic inhibition in paracapsular cells of the mouse amygdala. Front. Neural Circ. 8, 27. doi:10.3389/fncir.2014.00027
Meltzer-Brody, S., Colquhoun, H., Riesenberg, R., Epperson, C. N., Deligiannidis, K. M., Rubinow, D. R., et al. (2018). Brexanolone injection in post-partum depression: two multicentre, double-blind, randomised, placebo-controlled, phase 3 trials. Lancet 392, 1058–1070. doi:10.1016/S0140-6736(18)31551-4
Milivojevic, V., Fox, H. C., Sofuoglu, M., Covault, J., and Sinha, R. (2016). Effects of progesterone stimulated allopregnanolone on craving and stress response in cocaine dependent men and women. Psychoneuroendocrinology 65, 44–53. doi:10.1016/j.psyneuen.2015.12.008
Milivojevic, V., Covault, J., Angarita, G. A., Siedlarz, K., and Sinha, R. (2019). Neuroactive steroid levels and cocaine use chronicity in men and women with cocaine use disorder receiving progesterone or placebo. Am. J. Addict. 28, 16–21. doi:10.1111/ajad.12828
Mohler, H. (2012). The GABA system in anxiety and depression and its therapeutic potential. Neuropharmacology 62, 42–53. doi:10.1016/j.neuropharm.2011.08.040
Motzo, C., Porceddu, M. L., Maira, G., Flore, G., Concas, A., Dazzi, L., et al. (1996). Inhibition of basal and stress-induced dopamine release in the cerebral cortex and nucleus accumbens of freely moving rats by the neurosteroid allopregnanolone. J. Psychopharmacol. 10, 266–272. doi:10.1177/026988119601000402
Nequin, L. G., Alvarez, J., and Schwartz, N. B. (1979). Measurement of serum steroid and gonadotropin levels and uterine and ovarian variables throughout 4 day and 5 day estrous cycles in the rat. Biol. Reprod. 20 (3), 659–670. doi:10.1095/biolreprod20.3.659
Nikolaus, S., Wittsack, H. J., Beu, M., Antke, C., De Souza Silva, M. A., Wickrath, F., et al. (2018). GABAergic control of nigrostriatal and mesolimbic dopamine in the rat brain. Front. Behav. Neurosci. 12, 38. doi:10.3389/fnbeh.2018.00038
Paul, S. M., Pinna, G., and Guidotti, A. (2020). Allopregnanolone: from molecular pathophysiology to therapeutics. A historical perspective. Neurobiol. Stress 12, 100215. doi:10.1016/j.ynstr.2020.100215
Paxinos, G., and Watson, C. (1998). “The rat brain in stereotaxic coordinates,”in CD-ROM. 4th Edn (San Diego, CA: Academic Press).
Pineles, S. L., Nillni, Y. I., Pinna, G., Irvine, J., Webb, A., Arditte Hall, K. A., et al. (2018). PTSD in women is associated with a block in conversion of progesterone to the GABAergic neurosteroids allopregnanolone and pregnanolone measured in plasma. Psychoneuroendocrinology 93, 133–141. doi:10.1016/j.psyneuen.2018.04.024
Pitman, K. A., Puil, E., and Borgland, S. L. (2014). GABA(B) modulation of dopamine release in the nucleus accumbens core. Eur. J. Neurosci. 40, 3472–3480. doi:10.1111/ejn.12733
Porcu, P., Barron, A. M., Frye, C. A., Walf, A. A., Yang, S. Y., He, X. Y., et al. (2016). Neurosteroidogenesis today: novel targets for neuroactive steroid synthesis and action and their relevance for translational research. J. Neuroendocrinol. 28, 12351. 10.1111/jne.12351
Rapkin, A. J., Morgan, M., Goldman, L., Brann, D. W., Simone, D., and Mahesh, V. B. (1997). Progesterone metabolite allopregnanolone in women with premenstrual syndrome. Obstet. Gynecol. 90, 709–714. doi:10.1016/S0029-7844(97)00417-1
Robinson, D. L., Venton, B. J., Heien, M. L., and Wightman, R. M. (2003). Detecting subsecond dopamine release with fast-scan cyclic voltammetry in vivo. Clin. Chem. 49, 1763–1773. doi:10.1016/S0029-7844(97)00417-1
Robinson, D. L., Hermans, A., Seipel, A. T., and Wightman, R. M. (2008). Monitoring rapid chemical communication in the brain. Chem. Rev. 108, 2554–2584. doi:10.1021/cr068081q
Robinson, D. L., Howard, E. C., McConnell, S., Gonzales, R. A., and Wightman, R. M. (2009). Disparity between tonic and phasic ethanol-induced dopamine increases in the nucleus accumbens of rats. Alcohol Clin. Exp. Res. 33, 1187–1196. doi:10.1111/j.1530-0277.2009.00942.x
Rouge-Pont, F., Mayo, W., Marinelli, M., Gingras, M., Le Moal, M., and Piazza, P. V. (2002). The neurosteroid allopregnanolone increases dopamine release and dopaminergic response to morphine in the rat nucleus accumbens. Eur. J. Neurosci. 16, 169–173. doi:10.1046/j.1460-9568.2002.02084.x
Schelp, S. A., Brodnik, Z. D., Rakowski, D. R., Pultorak, K. J., Sambells, A. T., Espana, R. A., et al. (2018). Diazepam concurrently increases the frequency and decreases the amplitude of transient dopamine release events in the nucleus accumbens. J. Pharmacol. Exp. Therapeut. 364, 145–155. doi:10.1124/jpet.117.241802
Sabeti, J., Gerhardt, G. A., and Zahniser, N. R. (2003). Chloral hydrate and ethanol, but not urethane, alter the clearance of exogenous dopamine recorded by chronoamperometry in striatum of unrestrained rats. Neurosci Letters 343, 9–12. doi:10.1016/s0304-3940(03)00301-x
Schultz, W. (2016). Dopamine reward prediction-error signalling: a two-component response. Nat. Rev. Neurosci. 17, 183. doi:10.1038/nrn.2015.26
Schultz, W. (2007). Multiple dopamine functions at different time courses. Annu. Rev. Neurosci. 30, 259–288. doi:10.1146/annurev.neuro.28.061604.135722
Schultz, W. (2019). Recent advances in understanding the role of phasic dopamine activity. F1000Res 8, F1000. doi:10.12688/f1000research.19793.1
Shnitko, T. A., Spear, L. P., and Robinson, D. L. (2016). Adolescent binge-like alcohol alters sensitivity to acute alcohol effects on dopamine release in the nucleus accumbens of adult rats. Psychopharmacology (Berl) 233 (3), 361–371. doi:10.1007/s00213-015-4106-8
Sinha, R., Fox, H., Hong, K. I., Sofuoglu, M., Morgan, P. T., and Bergquist, K. T. (2007). Sex steroid hormones, stress response, and drug craving in cocaine-dependent women: implications for relapse susceptibility. Exp. Clin. Psychopharmacol 15, 445–452. doi:10.1037/1064-1297.15.5.445
Smith, M. S., Freeman, M. E., and Neill, J. D. (1975). The control of progesterone secretion during the estrous cycle and early pseudopregnancy in the rat: prolactin, gonadotropin and steroid levels associated with rescue of the corpus luteum of pseudopregnancy. Endocrinology 96, 219–226. doi:10.1210/endo-96-1-219
Smith, S. S., Gong, Q. H., Hsu, F. C., Markowitz, R. S., ffrench-Mullen, J. M., and Li, X. (1998). GABA(A) receptor alpha4 subunit suppression prevents withdrawal properties of an endogenous steroid. Nature 392, 926–930. doi:10.1038/3194
Smith, S. S., Shen, H., Gong, Q. H., and Zhou, X. (2007). Neurosteroid regulation of GABA(A) receptors: focus on the alpha4 and delta subunits. Pharmacol. Ther. 116, 58–76. doi:10.1016/j.pharmthera.2007.03.008
Smolders, I., De Klippel, N., Sarre, S., Ebinger, G., and Michotte, Y. (1995). Tonic GABA-ergic modulation of striatal dopamine release studied by in vivo microdialysis in the freely moving rat. Eur. J. Pharmacol. 284, 83–91. doi:10.1016/0014-2999(95)00369-V
Stephens, D. N., King, S. L., Lambert, J. J., Belelli, D., and Duka, T. (2017). GABAA receptor subtype involvement in addictive behaviour. Gene Brain Behav. 16, 149–184. doi:10.1111/gbb.12321
Takada, K., Murai, T., Kanayama, T., and Koshikawa, N. (1993). Effects of midazolam and flunitrazepam on the release of dopamine from rat striatum measured by in vivo microdialysis. Br. J. Anaesth. 70, 181–185. doi:10.1093/bja/70.2.181
Vashchinkina, E., Manner, A. K., Vekovischeva, O., den Hollander, B., Uusi-Oukari, M., Aitta-Aho, T., et al. (2014). Neurosteroid Agonist at GABAA receptor induces persistent neuroplasticity in VTA dopamine neurons. Neuropsychopharmacology 39, 727–737. doi:10.1038/npp.2013.258
Volkow, N. D., Wise, R. A., and Baler, R. (2017). The dopamine motive system: implications for drug and food addiction. Nat. Rev. Neurosci. 18, 741–752. doi:10.1038/nrn.2017.130
Votaw, V. R., Geyer, R., Rieselbach, M. M., and McHugh, R. K. (2019). The epidemiology of benzodiazepine misuse: a systematic review. Drug Alcohol Depend. 200, 95–114. doi:10.1016/j.drugalcdep.2019.02.033
Wang, M. (2011). Neurosteroids and GABA-A receptor function. Front. Endocrinol. (Lausanne) 2, 44. doi:10.3389/fendo.2011.00044
Wightman, R. M., and Robinson, D. L. (2002). Transient changes in mesolimbic dopamine and their association with ‘reward’. J. Neurochem. 82, 721–735. doi:10.1046/j.1471-4159.2002.01005.x
Wu, Y. V., and Burnham, W. M. (2018). Progesterone, 5a-dihydropogesterone and allopregnanolone’s effects on seizures: a review of animal and clinical studies. Seizure 63, 26–36. doi:10.1016/j.seizure.2018.10.012
Xiao, L., and Becker, J. B. (1994). Quantitative microdialysis determination of extracellular striatal dopamine concentration in male and female rats: effects of estrous cycle and gonadectomy. Neurosci. Lett. 180, 155–158. doi:10.1016/0304-3940(94)90510-x
Keywords: neurosteroid, allopregnanolone, progesterone, voltammetry, dopamine, nucleus accumbens
Citation: Dornellas APS, Macedo GC, McFarland MH, Gómez-A A, O’Buckley TK, Da Cunha C, Morrow AL and Robinson DL (2021) Allopregnanolone Decreases Evoked Dopamine Release Differently in Rats by Sex and Estrous Stage. Front. Pharmacol. 11:608887. doi: 10.3389/fphar.2020.608887
Received: 21 September 2020; Accepted: 05 November 2020;
Published: 14 January 2021.
Edited by:
Brian McCool, Wake Forest School of Medicine, United StatesReviewed by:
Mark J. Ferris, Wake Forest School of Medicine, United StatesCopyright © 2021 Dornellas, Macedo, McFarland, Gómez-A, O'Buckley, Da Cunha, Morrow and Robinson. This is an open-access article distributed under the terms of the Creative Commons Attribution License (CC BY). The use, distribution or reproduction in other forums is permitted, provided the original author(s) and the copyright owner(s) are credited and that the original publication in this journal is cited, in accordance with accepted academic practice. No use, distribution or reproduction is permitted which does not comply with these terms.
*Correspondence: Donita L. Robinson, RExSQHVuYy5lZHU=
†These authors share first authorship
Disclaimer: All claims expressed in this article are solely those of the authors and do not necessarily represent those of their affiliated organizations, or those of the publisher, the editors and the reviewers. Any product that may be evaluated in this article or claim that may be made by its manufacturer is not guaranteed or endorsed by the publisher.
Research integrity at Frontiers
Learn more about the work of our research integrity team to safeguard the quality of each article we publish.