- 1Laboratory of Cellular and Molecular Angiogenesis, Department of Life Sciences and Systems Biology, University of Torino, Torino, Italy
- 2Laboratoire de Cell Physiology, Université de Lille, Department of Life Sciences, Univ. Lille, Inserm, U1003—PHYCEL, Lille, France
- 3Univ. Lille, CNRS, INSERM, CHU Lille, Centre Oscar Lambret, UMR 9020-UMR 1277-Canther-Cancer Heterogeneity, Plasticity and Resistance to Therapies, Lille, France
- 4Institut Universitaire de France (IUF), Paris, France
Transient Receptor Potential (TRP) cations channels, as key regulators of intracellular calcium homeostasis, play a central role in the essential hallmarks of cancer. Among the multiple pathways in which TRPs may be involved, here we focus our attention on the ones involving small guanosine triphosphatases (GTPases), summarizing the main processes associated with the metastatic cascade, such as migration, invasion and tumor vascularization. In the last decade, several studies have highlighted a bidirectional interplay between TRPs and small GTPases in cancer progression: TRP channels may affect small GTPases activity via both Ca2+-dependent or Ca2+-independent pathways, and, conversely, some small GTPases may affect TRP channels activity through the regulation of their intracellular trafficking to the plasma membrane or acting directly on channel gating. In particular, we will describe the interplay between TRPC1, TRPC5, TRPC6, TRPM4, TRPM7 or TRPV4, and Rho-like GTPases in regulating cell migration, the cooperation of TRPM2 and TRPV2 with Rho GTPases in increasing cell invasiveness and finally, the crosstalk between TRPC1, TRPC6, TRPM8, TRPV4 and both Rho- and Ras-like GTPases in inducing aberrant tumor vascularization.
Introduction
Ca2+ signaling plays a central role in the regulation of many important cellular functions and indeed, not surprisingly, a dysregulation of Ca2+ homeostasis has been observed in various pathological conditions, including tumorigenesis (Monteith et al., 2017). Changes in Ca2+ signaling leading to dysfunctions in cancer cells are due to alterations in the so-called “Ca2+-signaling toolkit” (Berridge et al., 2003) which includes, among others, ion channels, responsible for altered fluxes within the cell, and several Ca2+-dependent effectors, which mediate various signal transduction pathways in response to altered Ca2+ homeostasis.
In recent years, the involvement of Ca2+-permeable channels in neoplastic diseases has been extensively investigated and a direct correlation between their dysregulation and cancer development has been shown (Monteith et al., 2017). Among them, Transient Receptor Potential (TRP) channels have revealed an important involvement in the regulation of many signaling pathways associated with tumor progression (Yang and Kim, 2020). One of the key features of TRP channels is their polymodal activation mechanism and their involvement in different signal transduction pathways. Among the multiple pathways TRPs are signaling through, the ones involving specific intracellular messengers belonging to the family of small guanosine triphosphatases (GTPases) are emerging as essential in tumorigenesis in the last decade. Therefore, in this review, we will focus on the interplay between TRPs and small GTPases, and its role in several aspects of the metastatic process (Aspenström, 2004; Clayton and Ridley, 2020).
The metastatic cascade leads to spread of malignant cells from the primary tumor through the lymph or blood circulation to establish secondary growth in a distant organ; it is a multistep process involving highly complex structural and functional alterations within cancer cells. Primary tumor cells are primed for dissemination by the process of epithelial-mesenchymal transition (EMT), during which they assume a more aggressive mesenchymal-like phenotype. This phenotypic switch allows them to detach from the primary tumor mass and to acquire migratory and invasive properties, in order to move from their original location, migrate and invade the extracellular matrix (ECM) and endothelium to spread to secondary sites and form metastases (Roche, 2018). All the steps involved in metastatic cascade (EMT, cell migration, invasion and tumor vascularization) are regulated by the intracellular Ca2+ concentration (Berridge et al., 2003) and specifically by the TRP-mediated calcium influx (Fels et al., 2018), as well as by the most important small GTPases (Rho-like and Ras-like) involved in cytoskeletal dynamics and cell polarity (Ungefroren et al., 2018). Here, we discuss TRPs and small GTPases contribution in cancer progression, focusing on signaling pathways involving a direct interplay between TRPs and small GTPases in three main metastatic cancer hallmarks: migration, invasion and tumor vascularization.
TRP Channels
TRP channels mainly act as signal transducers by altering membrane potential or intracellular Ca2+ concentration in response to various environmental stimuli, including physical-chemical stimuli, such as temperature, pH changes, osmolarity, and pressure as well as endogenous and exogenous ligands (Nilius and Owsianik, 2011). TRP channels have been shown to play a central role in carcinogenesis as well as in various late stages of tumor progression. In particular, it has been shown that changes in the expression of TRP channels are correlated with the progression of different types of cancer. To date, most changes involving TRP proteins do not involve mutations in the TRP gene but rather dysregulation of the wild-type TRP protein expression levels, depending on the stage of cancer (Gkika and Prevarskaya, 2011; Lehen’kyi and Prevarskaya, 2011; Bernardini et al., 2015; Shapovalov et al., 2016). Moreover, several recent studies have highlighted a direct correlation between cancer patient survival and TRP channel expression. Tumor differential expression of the main TRP channels discussed in this review and its correlation with patients’ survival are summarized in Table 1.
These observations strongly indicate that TRPs play a significant role in cancer progression and more specifically in many processes underlying the metastatic cascade, making them promising candidates as both molecular biomarkers and therapeutic targets in various types of cancer (Gkika and Prevarskaya, 2011; Fiorio Pla and Gkika, 2013; Bernardini et al., 2015; Lastraioli et al., 2015; Litan and Langhans, 2015; Fels et al., 2018). It has been shown that TRP-mediated effects on metastatic cancer cell behavior are mainly associated to their Ca2+ permeability. Indeed, through the regulation of intracellular Ca2+ concentration, both in the cytosol and within subcellular organelles, TRP channels play a key role in many Ca2+-dependent signaling pathways, including those associated with the metastatic cascade such as EMT, migration, invasion and tumor vascularization (Iamshanova et al., 2017). In response to different environmental challenges during the metastatic cascade, like hypoxic, acidic and mechanical cues, cancer cells “re-program” TRPs expression and “misuse” their functions in order to put in place and sustain a more aggressive, metastatic phenotype. However, although most of the TRP-mediated pathways involved in cancer progression are due to alteration of Ca2+ homeostasis, it has been also demonstrated an involvement of these channels independent from their Ca2+ permeability. As an example, TRPM7 regulation on cell migration is mainly due to Mg2+ influx through the channel (Abed and Moreau, 2009; Su et al., 2011). Similarly, the Na+-selective TRPM4 channel has been implicated in cancer migration, although it is inherently Ca2+-impermeable (Vennekens and Nilius, 2007; Cáceres et al., 2015; Holzmann et al., 2015). On the other hand, TRPs’ involvement in the metastatic cascade may also be pore-independent, extending the interest in TRPs beyond the field of ion channels (Vrenken et al., 2015). Indeed, TRPM7 promotes many of its biological effects through its peculiar intrinsic kinase activity (Desai et al., 2012; Faouzi et al., 2017; Cai et al., 2018). Moreover, we recently demonstrated the role of TRPM8 in inhibiting vascular endothelial cell migration, which is independent from the pore function of the channel (Genova et al., 2017).
It is therefore evident that, despite the countless advances made in recent years in the study of TRP channels, there are still many aspects to be explored in order to better characterize the main TRP-mediated pathways involved in tumor progression and thus be able to develop new cancer therapies that use TRPs as therapeutic targets.
Small GTPases
The family of small GTPases is composed of a large group of structurally and functionally related proteins, subgrouped into six families: Ras, Rho, Arf, Rab, Ran, and RGK. Among them, Ras-like and Rho-like GTPases are the most well characterized. Mechanistically, small GTPases are molecular switches that cycle between an active GTP- bound form and an inactive GDP-bound form. More specifically, these enzymes, once bound to GTP, can catalyse its hydrolysis to GDP and this reaction then results in a conformational change which causes the inactivation of the proteins (Vetter and Wittinghofer, 2001; Cherfils and Zeghouf, 2013). The cycling between GTP- and GDP-bound states is tightly regulated by specific GTPases activating protein (GAPs), which act as negative regulators, promoting the GDP-bound state by increasing the hydrolysis activity of small GTPases, and by guanine exchange factors (GEFs), which act as positive regulators, guiding the replacement of the hydrolysed GDP for a GTP, thus promoting the enzyme active state (Mishra and Lambright, 2016). Moreover, a third family of regulatory proteins called guanine-nucleotide dissociation inhibitors (GDIs) inhibits small GTPases activity by controlling their intracellular localization: GDIs bind to GTPases in their inactive GDP-bound state and sequester them in the cytosol, thus preventing their translocation to intracellular membranes, where activation occurs (Mishra and Lambright, 2016). Indeed, it has been shown that spatial and temporal distributions of the different small GTPases, as well as of their regulators, are important determinants in signaling by small GTPases, determining many aspects of cell behavior (Yarwood et al., 2006; Cherfils and Zeghouf, 2013; Moissoglu and Schwartz, 2014).
The conformational changes following the binding to GTP allow the association of small GTPases with a large number of potential effector proteins such as enzymes and scaffold proteins, which mediate the specific biological effects of each GTPases. Thanks to their ability to interact with a wide number of downstream targets and to co-ordinately activate several molecular processes required for a particular cellular response, small GTPases function as signaling switches in numerous cellular processes. In general, it has been found that Ras-like GTPases are mainly implicated in regulating cell cycle, differentiation and proliferation, (Shields et al., 2000), whereas Rho-like GTPases are mainly involved in cell morphology, cytoskeletal dynamics and cell polarity (Ridley, 2001). Moreover, Rab GTPases play a key role in many cellular functions, by controlling intracellular trafficking between organelles through vesiculotubular carriers and thus ensuring the spatiotemporal regulation of vesicle traffic (Stenmark, 2009). Considering the central role of Ca2+ signaling in many of these processes, it is not surprising that small GTPases activity was found to be strongly related to Ca2+ homeostasis (Aspenström, 2004). More specifically, their activation/inactivation may occur through Ca2+-dependent mechanisms acting on specific GEF/GAP proteins or directly on them. Moreover, some GTPases have revealed a direct influence on calcium signaling by regulating the activity of certain calcium channels, including TRPs, by itself or through their effectors (Koopman et al., 2003; Aspenström, 2004; Iftinca et al., 2007; Correll et al., 2008). Finally, several small GTPases collaborate with calcium signaling through the activation of specific Ca2+-related effectors involved in cellular processes, such as cell adhesion, cell migration and exocytosis (Cullen and Lockyer, 2002; Aspenström, 2004; Bader et al., 2004).
Small GTPases expression results dysregulated in several tumors and has been correlated with the progression of the disease (Sahai and Marshall, 2002; Svensmark and Brakebusch, 2019). Without a doubt, Ras is still the most studied small GTPase in cancer, due to the role played by mutated ras genes in the pathogenesis of human tumors (Bos, 1989; Hobbs et al., 2016; Li et al., 2018). Indeed, oncogenic mutations on H-ras, K-Ras, and N-Ras genes have been detected in several tumor types, although the incidence varies greatly (Bos, 1989; Prior et al., 2012; Li et al., 2018). Moreover, some members of the Ras superfamily like Rap1, resulted involved in cancer hallmarks such as migration and angiogenesis, due to their key role in integrin-mediated “inside-out” signaling events (Chrzanowska-wodnicka et al., 2008; Boettner and Van Aelst, 2009; Carmona et al., 2009). As regards the Rho superfamily, its role in many aspects of the metastatic cascade, including EMT, cell migration, invasion and angiogenesis, has been well established (Bryan and D’Amore, 2007; Ungefroren et al., 2018; Clayton and Ridley, 2020).
TRPs-Small GTPases Interplay in Metastatic Cancer
Migration
One of the key steps in the metastatic cascade involves the acquisition of motility by cancer cells. This results from a complex coordination between cytoskeleton dynamics, cellular contractility and cell adhesion rearrangements. Cell migration is a dynamic process characterized by the cycling of four principal steps: after an initial phase in which cell spreading increases about twice with the generation of protrusions at the leading edge and cell adhesion to the ECM increases, then spreading is reduced and thanks to the generation of traction forces cell compacts and detaches at the trailing edge, allowing for cellular movement (Haws et al., 2016). Due to their role as key regulators of cytoskeletal dynamics and cell polarity, it is not surprising that Rho GTPases play a central role in controlling cell migration (Clayton and Ridley, 2020). This process is triggered by cell acquisition of a front-rear end polarity due to the formation of protrusive structures, called filopodia and lamellipodia, at the front edge, opposite to a retracting trailing edge (Llense and Etienne-Manneville, 2015). Filopodia consist of actin filaments organized as long parallel bundles and their formation is dependent on Rho GTPase Cdc42 activity, whereas lamellipodia result from the subsequent Rho GTPase Rac1-mediated branching of actin filaments. The extension of actin-based protrusions is accompanied by the formation at the leading edge of new adhesions that link integrins on the plasma membrane to the F-actin cytoskeleton through talin, vinculin, and focal adhesion kinases (FAKs) and which can mature into focal adhesions (FAs) in a process Rho GTPase RhoA- dependent. Contemporary, RhoA also mediates cytoskeletal rearrangements that lead to the formation of stress fibers, structures composed of bundles of actin and myosin II that have a high contractile capability. The force necessary to pull the cell body forward is engendered by the association of mature FAs with the end of stress fibers and by the actin-myosin cytoskeleton contraction. Finally, the disassembly of FAs in the rear of the migrating cells supports cell retraction at the trailing edge, allowing for cell detachment and movement.
A direct involvement of TRP channels activity in all processes associated with cell migration, including cytoskeletal rearrangement, FA turnover and cellular contractility, has been well established (Fiorio Pla and Gkika, 2013; Canales et al., 2019). Therefore, it is not surprising that several studies have highlighted a tight interplay between TRP channels and Rho GTPases in controlling cell migration during cancer progression. As depicted in Figure 1, this interplay is characterized by a bidirectional communication, in which TRP channels promote actin cytoskeleton reorganization through a cation-dependent activation of Rho GTPases, and, conversely, some small GTPases cause changes in TRP channel location, protein–protein interactions and channel gating, thereby modulating their function. More specifically, as described in the following paragraphs, TRPC5, TRPC6, TRPC1, TRPM7, TRPM4, and TRPV4 have been found to affect cell migration through a direct interaction with some small GTPases belonging to the Rho family.
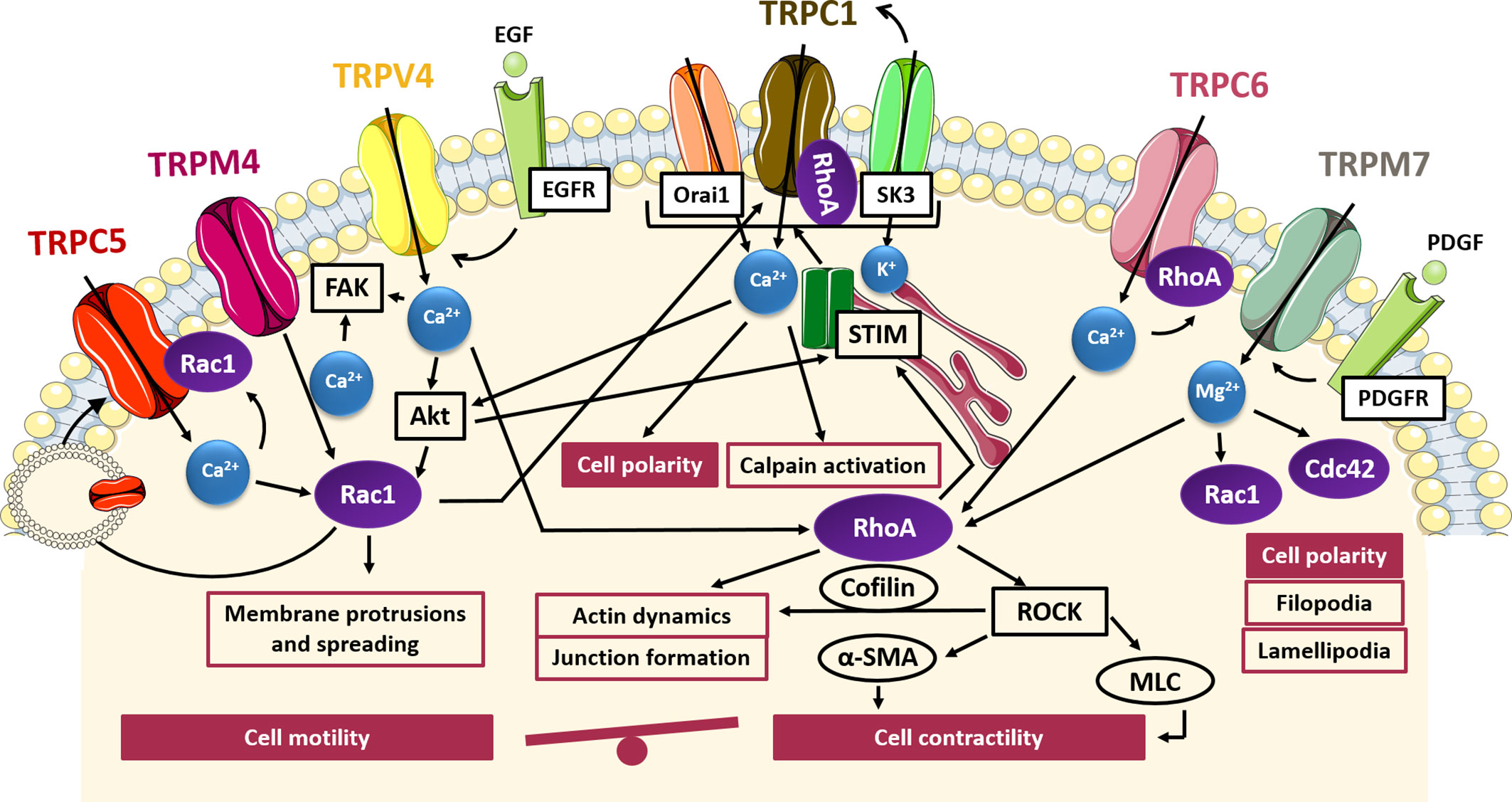
Figure 1 TRP- small GTPases signaling pathways interplay in cell migration. Cartoon depicting TRP channels signaling pathways affecting cell motility and contractility through GTPases. TRPC5, TRPM4 and TRPV4 induce the formation of protrusions and spreading via Rac1 activation in a Ca2+-dependent manner and at the same time Rac1 promote the translocation of TRPC5 into the plasma membrane; Rac1 and RhoA through SOCE activation induce TRPC1-mediated cell polarization for directional cell migration; TRPM7 control polarized cell movement through the regulation of Rac1 and Cdc42 in a Mg2+-dependent way; TRPM7, TRPV4, and TRPC6 contribute to actin dynamics and cell contractility through the Mg2+- or Ca2+-mediated activation of RhoA/ROCK pathways. FAK, focal adhesion kinase; Akt, protein-kinase B; EGF, epidermal growth factor; EGFR, epidermal growth factor receptor; PDGF, platelet-derived growth factor; PDGFR, platelet-derived growth factor receptor; Orai1, calcium release-activated calcium channel; SK3, small conductance calcium-activated potassium channel 3; STIM, stromal interaction molecule 1; ROCK, Rho-associated protein kinase; α-SMA, alfa-smooth muscle actin; MLC, myosin light chain.
Indeed, TRPC5 and TRPC6 channels have been identified as antagonist regulators of actin remodelling and cell motility in fibroblast and kidney podocytes, mediating the activation of Rac1 and RhoA, respectively (Tian et al., 2010). It has been shown that TRPC5 and TRPC6 trigger antagonistic and mutually inhibitory pathways associated with the maintenance of the balance between contractility and motility. In particular, TRPC5-mediated Ca2+ influx induces Rac1 activation, thereby enhancing motility and cell migration. Conversely, TRPC6-mediated Ca2+ influx stimulates an increase in RhoA activation, thereby promoting stress fibers formation, cell contractility and the subsequent inhibition of cell migration (Tian et al., 2010) or the disruption of podocytes architecture in glomerular renal diseases, which may lead to proteinuria (Jiang et al., 2011). Interestingly, the functional coupling between TRPC5 and TRPC6 with Rac1 and RhoA respectively, gives rise to two distinct molecular complexes predominantly localized to discrete membrane compartments, as detected by co-immunoprecipitation and immunofluorescence (Tian et al., 2010). Of note, the presence of constitutively active Rac1 has been shown to affect TRPC5 channel localization, leading to an increase in plasma membrane abundance of TRPC5 with respect to TRPC6, which, conversely, is predominant in cells expressing constitutively active RhoA. In this regard, RhoA seemed to have no effect on the dynamics of TRPC6 insertion into the membrane, whereas Rac1 was found to promote translocation of TRPC5 into the plasma membrane, according to other findings (Bezzerides et al., 2004). This close interdependence between TRPC5 and Rac1 could suggest a positive feedback mechanism in which the Rac1-mediated TRPC5 insertion from a vesicular pool into the cell membrane leads to enhanced TRPC5-mediated Ca2+ influx, which in turn triggers the activation of Rac1 and the subsequent migratory phenotype. Although a direct correlation between TRPC5/TRPC6 and Rac1/RhoA has not yet been established in cancer models, it is possible to speculate that a similar interplay may also occur in the regulation of cancer cells migration, taking into account the evidence for direct involvement of TRPC5 and TRPC6 in the increased migratory potential of several cell types (Greka et al., 2003; Xu et al., 2006; Rampino et al., 2007), including some tumors such as colon cancer and glioblastoma (Chigurupati et al., 2010; Chen L. et al. 2017; Chen Z. et al., 2017). However, whether these effects on tumor migration are dependent on the impact of TRPC5 and TRPC6 activity on cell contractility and motility remains to be clarified.
Similarly, TRPC1 has shown a correlation with an increased migratory phenotype in some tumors, such as glioblastoma, osteosarcoma, thyroid, pancreatic and colon carcinoma (Dong et al., 2010; Asghar et al., 2015; Huang et al., 2015; Guéguinou et al., 2016; Lepannetier et al., 2016). One of the key steps in cell migration is the establishment of a functional and morphological polarity along the axis of movement. In this context, it has been demonstrated that TRPC1 is localized to lipid raft domains at the leading edge of migrating cells and plays a role in determining their polarity and directionality (Fabian et al., 2008; Bomben et al., 2011; Huang et al., 2015). For instance, it has been shown that silencing of TRPC1 in transformed renal epithelial Madin–Darby Canine Kidney-Focus (MDCK-F) cells leads to a decrease in migration associated with a failure of cell polarization and an impaired lamellipodia formation. This effect is due to the partial loss of the local Ca2+ gradient at the front edge, needed to establish and maintain the axis of movement in migrating cells (Fabian et al., 2008). The same results were also shown in U2OS bone osteosarcoma cells, in which TRPC1 inhibition or knockdown correlates with a decrease in the percentage of polarized cells and the consequent reduction in cell migration (Huang et al., 2015). However, the link between TRPC1-mediated Ca2+ gradients and actin dynamics, as well as the possible involvement of TRPC1-mediated Rho GTPases activation in these processes has not been yet fully characterized. Nonetheless, a direct interaction between TRPC1 and RhoA has been characterized in intestinal epithelial cells (IECs) and in pulmonary arterial endothelial cells (Mehta et al., 2003; Chung et al., 2015). Indeed, TRPC1-RhoA interaction regulates TRPC1-mediated Ca2+ influx through SOCE, stimulating IECs migration after wounding (Chung et al., 2015). A reduction of RhoA/TRPC1 complexes, induced by downregulation or inactivation of either small GTPase or TRP channel, is associated with an inhibition of Ca2+ influx after store depletion and the decrease in wound healing after injury (Chung et al., 2015). Besides RhoA, a mechanism involving TRPC1 and Rac1 in promoting colon cancer cell migration has recently been proposed (Guéguinou et al., 2016). Indeed, in HCT-116 colon cancer cells TRPC1 and Rac1 are involved in a complex positive feedback loop in which EGF-induced SOCE activates both Rac1 and STIM1 through Akt pathway; in turn, STIM1 activation promotes translocation of TRPC1 and Orai1 into lipid rafts where SK3 is located and thereby triggers SOCE mediated by the complex SK3/TRPC1/Orai1. At the same time, Akt-mediated Rac1 activation enhances SOCE and thereby SOCE-dependent cell migration through Akt pathway, with subsequent lamellipodia formation and calpain activation. Taken together these data suggest a direct interplay between TRPC1 and Rho GTPases in controlling cell polarity and actin rearrangements during cancer cell migration.
TRPM7 is involved in directional migration in different cell types including migrating fibroblasts, osteoblasts, astrocytes and endothelial cells (ECs) (Abed and Moreau, 2009; Wei et al., 2009; Baldoli et al., 2013; Zeng et al., 2015). Similarly to TRPC1, TRPM7 has been shown to be involved in Ca2+ gradient formation, contributing to cell polarization and directional migration (Wei et al., 2009). In particular, TRPM7 is positively correlated with platelet-derived growth factor (PDGF)-induced Mg2+ influx as well as high-calcium microdomains “Ca2+ flickers”, most active at the leading lamella of migrating cells (Abed and Moreau, 2009; Wei et al., 2009). Interestingly, several data have shown that the effects of TRPM7 in controlling cytoskeleton and polarized cell movement are independent of its kinase activity and are associated with its channel function. This has been clearly demonstrated in fibroblasts and neuroblastoma cells where re-expression of TRPM7, as well as a kinase-inactive mutant of TRPM7 on knock out cells, reverted phenotypic changes in cell polarization enhancing cell spreading and adhesion (Clark et al., 2008; Su et al., 2011). In particular, it has been shown that Mg2+ plays a central role in TRPM7-mediated control of directional migration in fibroblasts and osteoblasts (Abed and Moreau, 2009; Su et al., 2011). The effects observed by TRPM7 depletion in cell morphology, disruption of actin filaments and myosin fibers and a decrease in the number of FAs, correlates with decreased activity of RhoA GTPase, suggesting a role for TRPM7 in RhoA regulation (Su et al., 2011). Besides its interaction with RhoA, TRPM7 is also functionally coupled with Rac1 and Cdc42: indeed, TRPM7 knockdown prevents Rac1 and Cdc42 activation, with a subsequent deficiency in their ability to form lamellipodia and impaired polarized cell movements (Su et al., 2011). Although Rho GTPases, differing from Ras and Rab proteins, do not require Mg2+ for high-affinity nucleotide binding, it has been shown that Mg2+ plays a role in regulating nucleotide binding and hydrolysis kinetics in the GEF- and GAP-catalyzed reactions of Rho family GTPases (Zhang et al., 2000). In particular, RhoGAPs exploit Mg2+ to achieve high catalytic efficiency and specificity and, conversely, RhoGEFs are negatively regulated by free Mg2+, since the presence of Mg2+ significantly decreases the intrinsic dissociation rates of the nucleotides. This finding may suggest that one role of GEFs is to displace bound Mg2+ from Rho proteins in order to efficiently perform their function and dissociate nucleotide from Rho GTPases (Zhang et al., 2000). Taken together this data revealed an interesting and so far little investigated interplay between TRP channel and Rho GTPases in controlling cell migration mediated by Mg2+ homeostasis.
More recently, another member of the TRPM subfamily, TRPM4, has been recognized as the first TRP channel to be part of the adhesome that is the set of protein components of FAs required for migration and contractility (Cáceres et al., 2015). Indeed, it has been shown that TRPM4 localises to FAs in different cell types and that its suppression impaires FAs relocation and lamellipodia formation, leading to a reduced cellular spreading and migration in mouse embryonic fibroblasts. Actually, FA turnover plays a key role in cell migration, contributing to the generation of the traction forces necessary for cellular movements. TRPM4-mediated effects on cell migration are at least partially due to the activation of Rac1 GTPase. Indeed, it has been observed that upon silencing TRPM4 the serum-induced activation of Rac1 and lamellipodial distribution are significantly reduced, suggesting a direct cooperation between TRPM4 and Rac1 in the regulation of cellular spreading. Moreover, it has been demonstrated that TRPM4 pharmacological inhibition caused retarded skin wound healing in vivo, affecting cell contractility (Cáceres et al., 2015). TRPM4 has been found to affect the migratory behaviour of many cell types, including prostate cancer cells (Holzmann et al., 2015). Although TRPM4 itself is Ca2+-impermeable, its contribution to cell migration through the regulation of intracellular Ca2+ signaling has been well established (Vennekens and Nilius, 2007). For instance, in androgen-insensitive prostate cancer cells, it has been shown that TRPM4 acts as an important negative feedback regulator of SOCE, thus promoting cell migration (Holzmann et al., 2015). However, further investigations are needed to deepen the knowledge of the molecular mechanism underlying the pro-migratory effect of TRPM4 on prostate cancer cell migration.
To date, several experimental pieces of evidence have revealed a critical role of TRPV4 in regulating the migratory properties of many tumors, including liver, breast and gastric cancer (Vriens et al., 2004; Lee et al., 2016; Xie et al., 2017). However, little is known about the molecular mechanism driving this process. Nonetheless, it has been shown that TRPV4 is involved in the dynamics of trailing adhesions, likely through an interplay with other cation channels or proteins present at the FA sites (Mrkonjić et al., 2015). A direct correlation between TRPV4 and RhoA/ROCK pathways has been revealed in cardiac fibroblast remodelling and myofibroblast contraction. In particular, it has been shown that after stimulation with growth factors, TRPV4 contributes to cell contractility by increasing the actin protein α-SMA expression and incorporation into stress fibers, through the Ca2+-mediated activation of RhoA/ROCK pathways (Tomasek et al., 2006; Adapala et al., 2013; Thodeti et al., 2013). Another study reported a role of TRPV4 in the modulation of adherent-junctions, mediated by the TRPV4-dependent activation of Rho GTPases, thereby promoting actin fibers organization and junctions formation (Sokabe and Tominaga, 2010). Furthermore, the exogenous up-regulation of TRPV4 in breast cancer has been shown to aid actin dynamics and lead to higher activation of cofilin, a downstream protein effector of RhoA/ROCK pathways that promotes actin filaments depolymerisation, thus conferring cellular “softness” and promoting transendothelial migration (Lee et al., 2016). Accordingly, TRPV4 knockdown reduced migration, invasion and transendothelial migration in breast cancer cells (Lee et al., 2016). Finally, a recent study of Ou-yang and co-worker (Ou-yang et al., 2018) has described the Akt/Rac1 signaling pathway through which TRPV4 promotes migration and invasion in glioma cancer cells (Ou-yang et al., 2018). Mechanistically, agonist-mediated TRPV4 activation promoted the activation of Rac1 by targeting Akt for phosphorylation, thus enhancing glioma cell migration and invasion (Ou-yang et al., 2018). Accordingly, in gastric cancer, TRPV4-mediated Ca2+ influx promotes cell migration through the activation of the downstream Akt/β-catenin pathways (Xie et al., 2017). Collectively, these data support a direct interplay between TRPV4 and small GTPases in controlling cytoskeletal remodeling aimed to confer migratory phenotypes.
Invasion
In order to reach lymph- and bloodstreams and to colonize sites distant from the primary tumor, cancer cells have to acquire, beyond migratory phenotype, the ability to degrade ECM. Consequently, invasion is another key step of the metastatic cascade. Invasiveness of cancer cells comes from their ability to produce special protrusions called invadopodia, which are actin-rich protrusions of the plasma membrane with proteolytic activity. Once matured, invadopodia recruit proteolytic enzymes such as membrane-matrix metalloproteinases (MMPs), which are endopeptidases able to locally degrade ECM, allowing cell invasion. Among them, MMP-2 and MMP-9 are considered the most important in metastasis, since they were often aberrantly expressed in tumors (Jabłońska-trypuć et al., 2016). Both TRP channels and small GTPases have been implicated in increased tumor invasiveness through the induction of MMPs expression (Betson and Braga, 2003; Yang and Kim, 2020). Indeed, TRPM2, TRPM7, TRPM8, TRPV2, and TRPC1 have shown to cause upregulation of MMP9 in a Ca2+-dependent manner in gastric, bladder, oral squamous, prostate and thyroid cancer cells respectively (Monet et al., 2010; Okamoto et al., 2012; Asghar et al., 2015; Cao et al., 2016; Chen L. et al. 2017; Chen Z. et al., 2017; Almasi et al., 2019). Additionally, TRPM2, TRPM7, and TRPC1 activity have been also correlated with MMP2 production in gastric, lung, pancreatic and thyroid cancer (Asghar et al., 2015; Rybarczyk et al., 2017; Liu et al., 2018; Almasi et al., 2019). On the other hand, Rho and Rac GTPases activation has been correlated with increased MMPs expression in different cancer cell types (Zhuge and Xu, 2001; Abécassis et al., 2003; Santibáñez et al., 2010; Jacob et al., 2013).
TRPV2 and TRPM2 have been shown to affect cell invasiveness through signaling pathways involving small GTPases, which are summarized in Figure 2.
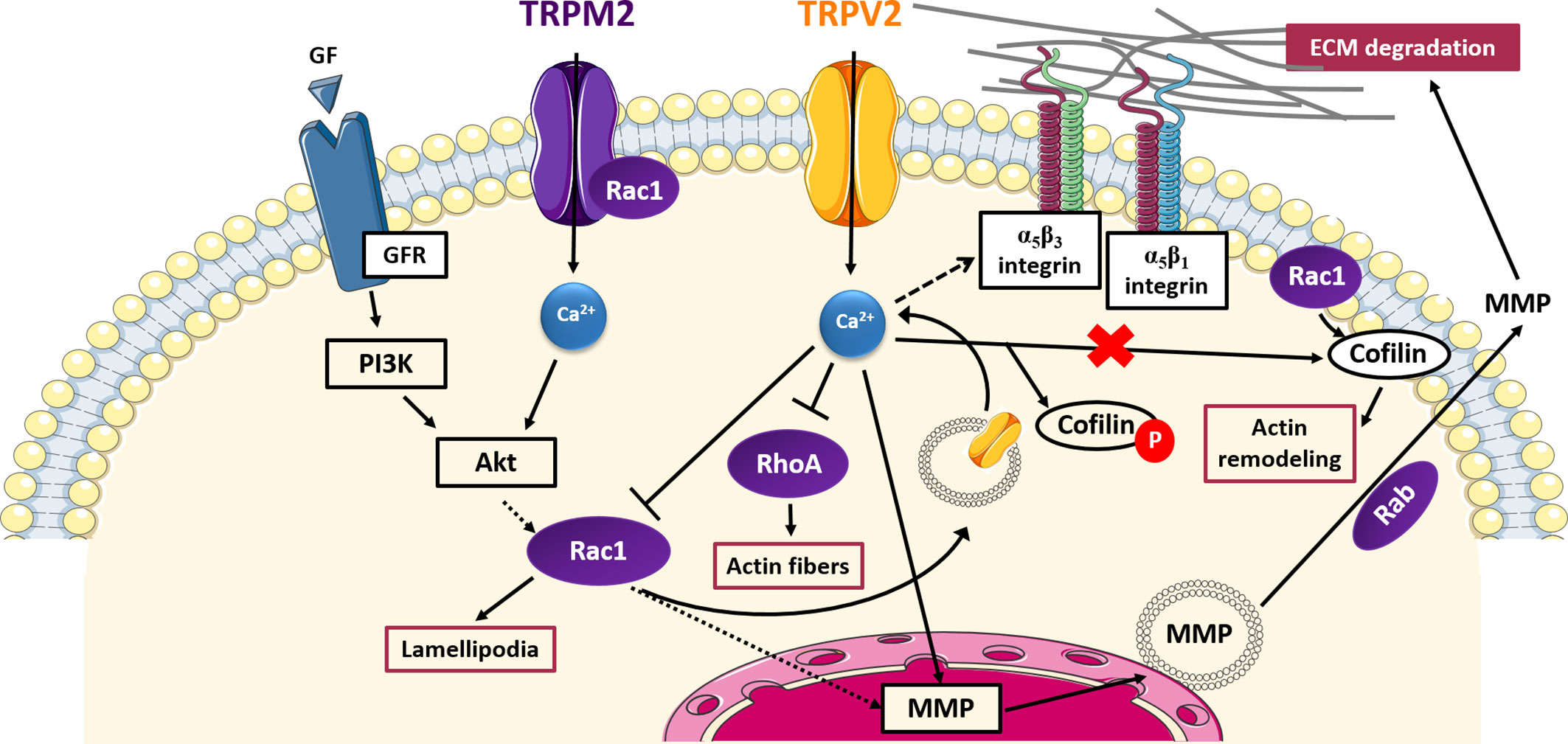
Figure 2 TRP- small GTPases signaling pathways interplay in cell invasion. Cartoon depicting TRP channels signaling pathways affecting cell invasiveness through GTPases. Rac1 promotes the translocation of TRPV2 into the plasma membrane and thus TRPV2-mediated increased in MMPs expression; TRPV2 affects also cell adhesion and invasion interfering with integrin-mediated signalling and inhibiting Rac1, RhoA, and cofilin activation by Rac1 in a Ca2+-dependent manner; TRPM2 and Rac1 physically interact with each other, mutually influencing their activity and lead to an increase in MMPs production; MMPs exocytosis is mediated by the Rab superfamily of small GTPases. GF, growth factor; GFR, growth factor receptor; PI3K, phosphoinositide-3 kinase; Akt, protein-kinase B; MMP, membrane-matrix metalloproteinase; ECM, extracellular matrix.
In particular, TRPV2 has been found to positively correlate with prostate cancer (PCa) invasiveness, promoting PCa progression to the aggressive castration-resistant stage (Monet et al., 2010). More specifically, it has been shown that siRNA-mediated silencing of TRPV2 leads to a decrease in MMP-2 and MMP-9 expression, reducing growth and invasive properties of PC3 prostate tumors established in nude mice xenografts (Monet et al., 2010). The mechanism by which the Ca2+ influx mediated by TRPV2 is linked with the up-regulation of MMPs has not been characterized. However, another study has revealed that TRPV2 trafficking to the plasma membrane correlates with enhanced cell migration and invasion in PCa, via the phosphoinositide 3-kinase (PI3K) pathway (Oulidi et al., 2013). Taking into account evidence of a PI3K-mediated activation of Rac1 in several tumor models (Haws et al., 2016; Ungefroren et al., 2018) and considering that Rac1 has been found to regulate TRPV2 intracellular trafficking in fibrosarcoma cells (Nagasawa and Kojima, 2015), it is possible to speculate that in PCa a PI3K-mediated Rac1 activation may allow the translocation to the plasma membrane of the “de novo” expressed TRPV2 in PC3 cells, thus giving rise of the TRPV2-mediated increase of cytosolic Ca2+ concentration responsible for MMPs overexpression. Confirming this hypothesis, Rac1 has been related to the up-regulation of MMP-2 and MMP-9 in fibrosarcoma (Zhuge and Xu, 2001) and transformed keratinocytes (Santibáñez et al., 2010), respectively. Unlike in PCa, TRPV2 has been found to suppress the invasiveness of fibroblast-like synoviocytes (FLS), which have an aggressive and invasive behaviour resembling that of cancer cells (Laragione et al., 2015). TRPV2 activation has been associated both in vitro and in vivo with reduced cell invasiveness and a down-regulation of the IL-1β-induced expression of MMP-2 and MMP-3 (Laragione et al., 2015). More recently, the cell signaling events mediating this TRPV2 suppressive activity have been characterized (Laragione et al., 2019). Interestingly, a direct interplay between TRPV2 channel function and RhoA/Rac1 GTPases activity in suppressing FLS cell invasion has been proposed (Laragione et al., 2019). In particular, it has been shown that, upon stimulation with the commercially-available TRPV2-specific agonist O1821, the channel nearly disappears from the plasma membrane, as well as integrins αν, β1, and β3, involved in cell binding to ECM. Concomitantly, a decrease in cell adhesion and the number of thick actin filaments and a reduction in lamellipodia formation are observed. Mechanistically, it has been found that O1821-induced TRPV2 activation causes a decrease in both RhoA and Rac1 activation, giving rise to the observed inhibition of actin filaments and lamellipodia formation, respectively. Moreover, TRPV2 activation significantly increases levels of phosphorylated (inactive) cofilin and affects the localization of active cofilin keeping it in the cytosol away from cell protrusions and lamellipodia, in which normally it exerts its function on actin remodeling, upon activation by Rac1. Considering the active involvement of Rac1 in TRPV2 trafficking found by Nagasawa and Kojima (2015), Rac1 inhibition through TRPV2 described by Laragione et al. might also explain the observed reduction of TRPV2 expression on the plasma membrane after channel stimulation, suggesting an intriguing negative feedback loop between TRPV2 and Rac1 regulation. Indeed, TRPV2 activation on the plasma membrane may inhibit in a Ca2+-dependent manner Rac1 activation, which in turn resulted in a decrease of TRPV2 expression on the cell surface. Taken together these data account for a possible two-sided interplay between Rac1 and TRPV2, based on which TRPV2 may exert both pro- and anti-invasiveness activities depending on cell type. In the first case Rac1 is activated by the PI3K pathway, thus allowing the overexpression of the constitutively active TRPV2 on the plasma membrane and the increased calcium flow responsible for MMPs overexpression and the increased cell invasiveness (Nagasawa and Kojima, 2015); in the second case TRPV2, upon activation by external stimuli, causes Rac1 inactivation and the inhibition of pro-invasive intracellular pathways as well as the expression of TRPV2 in the membrane through a negative feedback mechanism (Laragione et al., 2019). Therefore, in an interesting interchangeable relationship, TRPV2 may act as either modulator or effector of Rac1, depending on cell type, thus reflecting the bivalent activity showed by the channel in cell invasiveness.
TRPM2 offers another potential field of investigation on TRP-small GTPase relationship in cancer cells’ ability to invade surrounding tissues. In fact, it has been recently proven that TRPM2 causes an increase in MMP-9 production in gastric cancer (Almasi et al., 2019). As in the case of TRPV4, the function of TRPM2 appears to be regulated by the Akt pathway, known to regulate the activity of several GTPases including Rac1 (Ho et al., 2010), whose interaction with TRPM2 has been already well established in response to oxidative stress (Gao et al., 2014) (Figure 2). In this regard, it has been demonstrated that TRPM2 and Rac1 physically interact with each other, mutually influencing their activity, similarly to what was observed between TRPC5 and Rac1 in podocytes previously described (Bezzerides et al., 2004; Tian et al., 2010). Thus, we could speculate a TRPM2-Akt-Rac1 axis in the modulation of MMP-9 expression in gastric cancer.
Tumor Vascularization
Tumor vascularization, is a critical step in the metastatic cascade since the formation of new blood vessels is crucial not only to provide sufficient oxygen and nutrients and thus promoting the continuous growth of tumors but also to drive tumor spread and metastasis. Tumor vascularization, promoted by the tumor cells themselves through the secretion of several growth factors, results aberrant and leads to the formation of new vessels characterized by abnormal morphology, irregular blood flow, and distribution, non-uniform pericyte coverage and hyper-permeability (Carmeliet and Jain, 2000; Carmeliet and Jain, 2011). TRP channels are widely expressed within vascular ECs and several data correlate aberrant TRP channels expression and/or activity with tumor vascularization, thanks to their high sensitivity to both pro-angiogenic signals and subtle changes in the local microenvironment (Brossa et al., 2019; Negri et al., 2020; Scarpellino et al., 2020). TRP channels have been related to critical steps of tumor vascularization, including cell adhesion, cell migration, enhanced permeability and in vitro angiogenesis (Fiorio Pla and Gkika, 2013). Similarly, several Rho and Ras small GTPases, thanks to their contribution to actin dynamics, cell contractility and integrin-mediated “inside-out” signaling events, have been found to be dysregulated during tumor vascularization (Carmona et al., 2009; Chrzanowska-Wodnicka, 2010). Some common pathways involving both TRP channels and small GTPases in determining aberrant tumor vascularization have been described, such as those concerning TRPV4, TRPM8, TRPC1, and TRPC6, depicted in Figure 3.
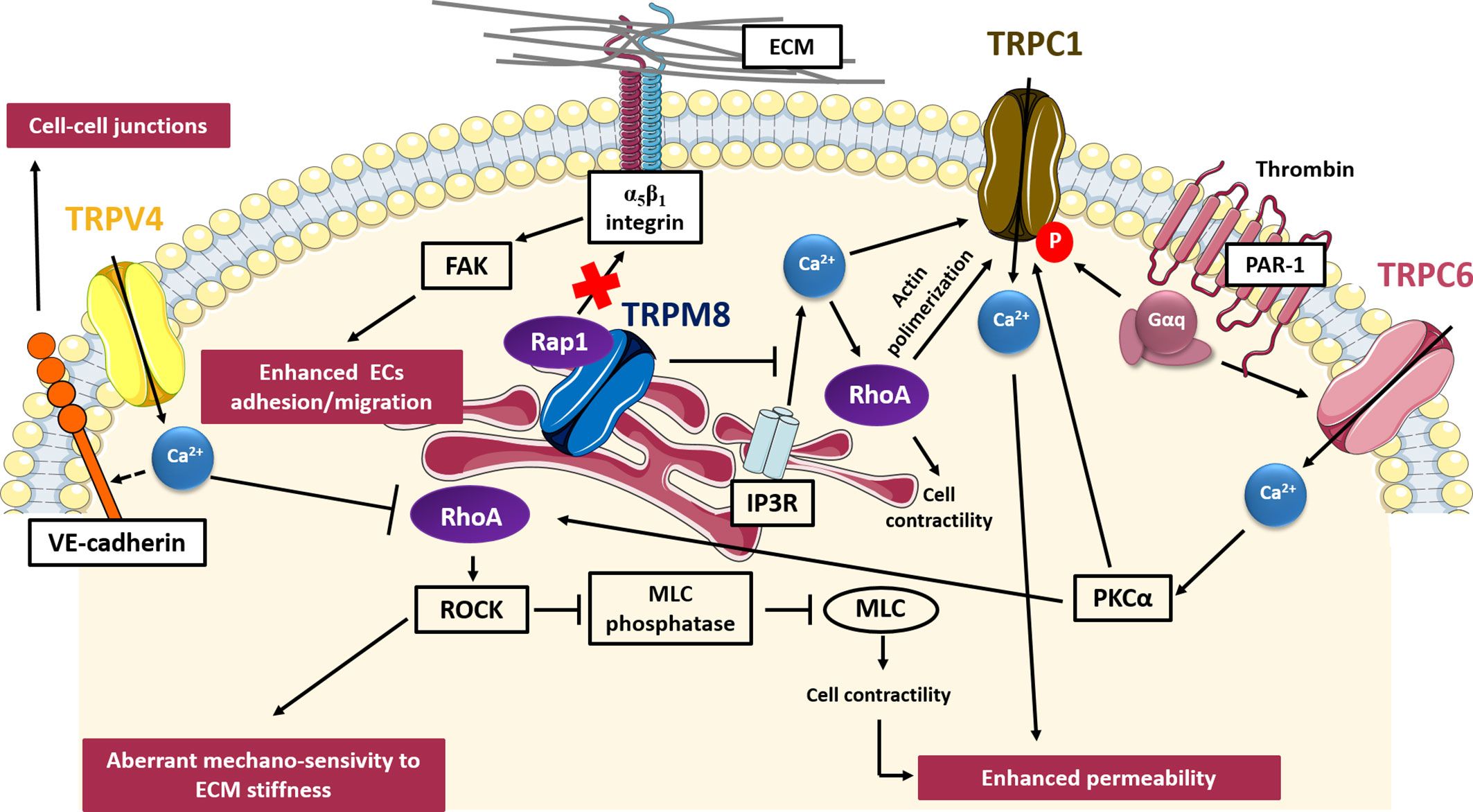
Figure 3 TRP- small GTPases signaling pathways interplay in aberrant tumor vascularization. Cartoon depicting TRP channels signaling pathways affecting tumor vascularization through GTPases. TRPV4 induces aberrant mechano-sensivity to ECM through the Ca2+-dependent inhibition of Rho/ROCK pathway; TRPC1 and TRPC6 enhance vessels permeability affecting cell contractility through a Ca2+-mediated regulation of RhoA; on the contrary, TRPM8 exerts a protective role in tumor vasculature permeability, inhibiting the store-operated RhoA activation and subsequent cell contraction; TRPM8 also inhibits ECs adhesion and migration, impairing activation of β1-integrin through the intracellular retention of Rap1. VE-cadherin, vascular E-cadherin; FAK, focal adhesion kinase; ROCK, Rho-associated protein kinase; MLC, myosin light chain; PKCα, protein kinase C alpha; IP3R, inositol trisphosphate receptor; PAR-1, protease-activated receptor-1.
TRPV4 has been the first TRP channel to be clearly implicated in tumor angiogenesis, although it can have both pro-angiogenic and anti-angiogenic effects depending on tumor type. In particular, TRPV4-mediated Ca2+ signals are implicated in tumor-derived endothelial cells (TECs) migration via a membrane-stretch activated arachidonic acid release and subsequent actin remodeling and TRPV4 insertion in the plasma membrane (Fiorio Pla et al., 2012). These data are in accordance with previous results showing that TRPV4 is required for shear stress EC reorientation in an integrin-dependent manner (Thodeti et al., 2009). However, in different TECs models, TRPV4 results to be down-regulated as compared to normal tissues. Moreover, this down-regulation is correlated with aberrant mechano-sensitivity of TECs towards ECM stiffness and subsequently with enhanced cell motility and abnormal angiogenesis. Subcutaneous injection of Lewis Lung Carcinoma cells in TRPV4 knockout mice leads to increased vascular density, higher vessel diameter, and reduced pericyte coverage, overall resulting in enhanced tumor growth (Adapala et al., 2016). More recently, it has also been shown by the same group that TRPV4 silencing causes a significant decrease in VE-cadherin expression at cell-to-cell junctions, with the subsequent increase in vascular leakage (Cappelli et al., 2019). Accordingly, overexpression or pharmacological stimulation of TRPV4 with GSK1016790A has been shown to lead to a “normalization” of the vascular endothelium, enhancing the permeability of chemotherapeutic drugs, and basically blocking tumor growth (Adapala et al., 2016). In particular, TRPV4-mediated “normalization” of aberrant capillary-like tubules in vitro is achieved by restoring the mechano-sensitivity of ECs toward ECM stiffness through the blockade of basal Rho activity (Adapala et al., 2016). In this contest, it was previously shown that the aberrant TECs mechano-sensitivity of TECs in response to ECM stiffness and cyclic strain results, at least partially, due to constitutively high level of Rho/ROCK basal activities (Ghosh et al., 2008). Moreover, ECs isolated from TRPV4 knockout (KO) mice display higher basal Rho activity as compared to EC WT and the inhibition of the Rho/ROCK pathway in TRPV4KO mice results in vessel normalization, confirming the role of TRPV4 as an important modulator of Rho activity also in vivo (Thoppil et al., 2016).
In agreement with other recent findings which have highlighted non-conducting functions of TRP channels in many processes, including the regulation of cytoskeletal dynamics (Vrenken et al., 2015), our recent study (Genova et al., 2017) has unveiled that TRPM8 inhibits ECs migration in a Ca2+-independent manner. TRPM8 is mainly localized in the endoplasmatic reticulum (ER) in ECs and its expression results remarkably down-regulated in breast TECs (BTECs) as compared to healthy human microvascular ECs (HMECs) and human umbilical vein ECs (HUVECs) (Genova et al., 2017). Mechanistically, TRPM8 inhibits ECs migration and spheroid sprouting by trapping Rap1 intracellularly, thereby preventing its relocation toward the plasma membrane, which is required to activate β1-integrin signaling. Inactive Rap1 (GDP- bound) physically interacts with the N-terminal tail of TRPM8 and interestingly, its retention within the cytosol occurs also in the presence of a TRPM8 pore mutant, demonstrating that TRPM8 inhibitory effects on ECs migration and adhesion by trapping Rap1 are independent from its channel function (Genova et al., 2017). Curiously, TRPM8 activation through specific channel agonists (icilin and menthol) or endogenous activators such as prostate-specific antigen (PSA), significantly reduces the amount of active Rap1-GTP bound and enhanced its inhibitory effect on migration, thus raising questions about the possible effects of agonists on TRPM8 besides pore gating. TRPM8-mediated inhibition of Rap1 cytoplasm–plasma membrane trafficking impairs the activation of inside-out signaling pathway known to trigger the conformational activation of β1-integrin and, consequently, cell adhesion and migration. Indeed, icilin-stimulated TRPM8 significantly inhibits active-β1 integrins as revealed by immunofluorescence assays. In addition, agonist-induced TRPM8 stimulation leads to a significant decrease in FAK phosphorylation, suggesting the involvement of FAK as a downstream effector of the β1-integrin pathway affected by TRPM8 (Genova et al., 2017). This study has highlighted how TRP channels may regulate GTPases activity not only by the generation of local Ca2+ fluxes, but also acting through physical interactions which affect their intracellular localization and thus their activity. Indeed, as well as TRP channels, also small GTPases are used for spatial and temporal control of cell behavior, as reported by several studies (Yarwood et al., 2006; Wang et al., 2012; Moissoglu and Schwartz, 2014). Another evidence of a TRPM8 interplay with small GTPases in controlling ECs behavior comes from a study of Sun and coworkers (Sun et al., 2014), which has revealed a role of TRPM8 in vasoconstriction and hypertension through attenuating RhoA/Rho kinase pathway. In particular, the authors have shown that TRPM8 activation by menthol attenuates vasoconstriction by inhibiting the RhoA/ROCK pathway in wild-type mice, but not in TRPM8KO mice (Sun et al., 2014). TRPM8 effect is associated with inhibition of intracellular calcium release from the sarcoplasmic reticulum, thus reducing Ca2+-mediated activation of RhoA/ROCK and ECs contraction. Since several experiments have correlated ECs contraction with vessel permeability (Hicks et al., 2010), this study could suggest a protective role of TRPM8 not only in ECs migration, but also in tumor vasculature permeability.
The increased permeability shown by tumor vessels may be induced by several factors, including hypoxia and inflammatory signals, such as thrombin. In this regard, TRPC6 and TRPC1 have been implicated in thrombin-induced hyper-permeabilization of ECs through the RhoA/ROCK signaling pathway. Thrombin is a serine-protease which binds specifically to the protease-activated receptor-1 (PAR-1) on endothelial cell surface, inducing a signaling cascade, which results in an increase in ECs contraction, changes in cell shape and finally, in the development of tiny inter-endothelial junctional gaps that lead to increased endothelial permeability. Interestingly, TRPC6 activation is promoted by thrombin stimulation through the α subunit of G protein-coupled receptors (Gαq) (Singh et al., 2007). Then, TRPC6-mediated Ca2+ influx leads to the activation of PKCα and thereby induces RhoA activity and ECs contraction, with subsequent cell shape changes, inter-endothelial gaps formation and increase in EC permeability (Singh et al., 2007). Indeed, upon thrombin stimulation it has been shown that RhoA activates its downstream effector ROCK, which in turn promotes myosin light chain (MLC) phosphatase regulatory subunit phosphorylation, reducing its phosphatase activity (Birukova et al., 2004). TRPC6 have also been found to play a central role in determining the angiogenic potential of glioma cells, since its inhibition affected EC tube formation in vitro by reducing the number of branch points (Chigurupati et al., 2010). Moreover, TRPC6 has been shown to exert pro-angiogenic effects by affecting vascular endothelial growth factor (VEGF)-induced calcium influx in ECs. Although there are not robust data on GTPases-mediated TRPC6 functions in angiogenesis, recently Zahra and coworkers defined an important role of RhoA in ECs proliferation, migration, invasion and sprouting triggered by important angiogenesis inducers, including VEGF (Zahra et al., 2019), thus suggesting a possible interplay between TRPC6 and RhoA in VEGF-induced angiogenesis.
Similarly to TRPC6, also TRPC1 has been found to have a role in increasing ECs permeability through RhoA activation in response to thrombin stimulation. In this regard, an intriguing mechanism depicting RhoA as a TRPC1 modulator in human pulmonary arterial endothelial cells has been proposed by Mehta and co-worker (Mehta et al., 2003). They showed that RhoA, upon thrombin-induced activation, forms a complex with IP3R and TRPC1, which then translocates to the plasma membrane, where TRPC1 can mediate a store depletion-induced Ca2+ entry and the resultant increase in endothelial permeability. Moreover, it has been demonstrated that RhoA-induced TRPC1-IP3R association is dependent on actin filament polymerization, since its inhibition hinders both the association and Ca2+ entry (Mehta et al., 2003). Moreover, TRPC1 serine/threonine phosphorylation by PKCα is crucial for inducing Ca2+ influx and consequently EC permeability (Ahmmed et al., 2004). However, the mechanism by with PKCα integrates with RhoA in ECs to trigger TRPC1-mediated Ca2+ influx remained to be characterized. Nonetheless, considering the previously described role of PKCα as a downstream effector of TRPC6 (Singh et al., 2007), it is possible that the PKCα- dependent RhoA-induced TRPC1 activation gives rise to a positive feedback loop that overall leads to a persistent increase endothelial permeability. This study provides an example of pathway in which small GTPases do not act as TRP Ca2+-dependent effectors, but rather as modulators of TRP channel activity, influencing protein-protein interactions and channel gating, and thus corroborating the bidirectional nature of this complex interplay.
Conclusions and Perspectives
TRPs and small GTPases show a direct interplay in cancer progression, characterized by a bidirectional communication, whereby TRP channels have been shown to affect small GTPases activity via both Ca2+-dependent or Ca2+–independent pathways, and conversely some small GTPases may affect TRP channels activity through the regulation of their intracellular trafficking to the plasma membrane or acting directly on channel gating (Table 2). In most cases, TRP-GTPase interaction is mediated by Ca2+ signals, triggered by TRP-mediated Ca2+ influx through the plasma membrane induced by growth factors, specific ligands or mechanical stimuli. In some specific cases, such as TRPM7, TRP-mediated small GTPases activation/inhibition may be supported by Mg2+ rather than Ca2+ homeostasis regulation. Moreover, also Ca2+-impermeable TRP channels like TRPM4 have been found to regulate small GTPases activity, probably through an indirect control on other Ca2+-signaling pathways. Finally, alternative regulatory pathways that go beyond the canonical ones involving cation homeostasis have been characterized for TRP-mediated small GTPases regulation. For instance, it has been shown that some TRPs, such as TRPM8 in ECs, may act similarly to a GDI-like protein, inhibiting small GTPases activity by physically trapping and restraining them within a specific cellular compartment and thus preventing their switch to the active form that generally occurs at the plasma membrane. Although in most cases small GTPases act as TRP effectors, there are some evidence about the role of small GTPases as modulators of TRP channel activity. Indeed, it has been shown that some small GTPases may affect TRPs channel activity by influencing channel trafficking, gene expression, protein-protein interactions and channel gating. In some cases, positive feedback loop mechanisms, wherein TRP channels activate small GTPases which in turn increase TRPs insertion to the plasma membrane, have also been described. In addition, some studies have highlighted that effector/modulator roles may be interchangeable between TRPs and small GTPases depending on cell type. For instance, it has been shown that Rac1 may act as either modulator or effector of TRPV2 activity, thus determining opposite effects on cell invasion, depending on cell type.
In addition to the examples of a direct TRP-small GTPases interaction in metastatic cancer hallmarks reported in this review, many studies suggest other possible signal transduction pathways associated with tumor progression involving both TRPs and small GTPases. For instance, they have both been implicated in the regulation of MMPs production through pathways like IP3K/Akt and Hsp90α-uPA-MMP2 that offer many points of contact in between them (Koike et al., 2000; Zhuge and Xu, 2001; Yang et al., 2015; Luo et al., 2016; Rybarczyk et al., 2017; Liu et al., 2018). However, to date, a direct correlation between TRPs and small GTPases in these signal transduction pathways has not yet been established but remains to be deeper clarified. Moreover, several studies have reported a role of both these two superfamilies of molecules in promoting another key step of the metastatic cascade that is EMT. In response to the same growth factor/cytokines-induced signaling pathways both TRPs and small GTPases are able to induce the up-regulation of mesenchymal-like markers such as vimentin and the down-regulation of epithelial-like markers such as E-cadherin through the direct regulation of transcriptional factors including STAT3, Snail and Twist (Simon et al., 2000; Davis et al., 2014; Liu et al., 2014; Yang et al., 2015; Chen L. et al., 2017; Kim et al., 2018 Liu et al., 2019; Seiz et al., 2020). However, a synergistic cooperation between TRPs and Rho GTPases during the early stage of growth-factor induced EMT is still only speculation, since to the best knowledge of the authors a direct correlation between TRPs and small GTPases effects on EMT has not yet been characterized. In conclusion, the interplay between TRP channels and small GTPase in cancer progression is only partially investigated to date and further investigations are required to shed light on many other aspects of this interesting crosstalk in cancer not well known.
Author Contributions
DG conceived the study. GC provided wrote and edited the manuscript and designed the figures. AP revised the manuscript. All authors contributed to the article and approved the submitted version.
Funding
GC was supported by University of Torino as part of the PhD Program in Complex Systems for Life Sciences. DG is supported by the Institut National du Cancer (INCa- PLBIO14-213) and the Institut Universitaire de France (IUF). GC, AP, and DG were supported by the International Associated Laboratory (LAI) agreement (CaPancInv). AP was supported by grants from the Italian Ministry of Instruction, University and Research (MIUR), PRIN grant “Leveraging basic knowledge of ion channel network in cancer for innovative therapeutic strategies (LIONESS)” (grant number 20174TB8KW).
Conflict of Interest
The authors declare that the research was conducted in the absence of any commercial or financial relationships that could be construed as a potential conflict of interest.
Abbreviations
EC, endothelial cell; ECM, extracellular matrix; EGF, epidermal growth factor; EMT, epithelial to mesenchymal transition; ER, endoplasmatic reticulum; FA, focal adhesion; FAK, focal adhesion kinase; GAP, GTPases activating protein; GEF, guanine exchange factor; GDI, guanine-nucleotide dissociation inhibitors; GDP, guanosine diphosphate; GTP, guanosine triphosphate; GTPase, guanosine triphosphatases; MLC, myosin light chain; MMP, membrane-matrix metalloproteinases; PCa, prostate cancer; PI3K, phosphoinositide 3-kinase; PFGF, platelet-derived growth factor; TEC, tumor endothelial cell; TRP, transient receptor potential; VEGF, vascular endothelial growth factor.
References
Abécassis, I., Olofsson, B., Schmid, M. (2003). RhoA Induces MMP-9 Expression at CD44 Lamellipodial Focal Complexes and Promotes HMEC-1 Cell Invasion. Exp. Cell Res. 291 (2), 363–376. doi: 10.1016/j.yexcr.2003.08.006
Abed, E., Moreau, R. (2009). Importance of Melastatin-like Transient Receptor Potential 7 and Magnesium in the Stimulation of Osteoblast Proliferation and Migration by Platelet-Derived Growth Factor. Am. J. Physiol. - Cell Physiol. 297 (2), 360–368. doi: 10.1152/ajpcell.00614.2008
Adapala, R. K., Thoppil, R. J., Luther, D. J., Paruchuri, S., Meszaros, J. G., Chilian, W. M., et al. (2013). Cellular Cardiology TRPV4 Channels Mediate Cardiac Fi Broblast Differentiation by Integrating Mechanical and Soluble Signals. J. Mol. Cell. Cardiol. 54, 45–52. doi: 10.1016/j.yjmcc.2012.10.016
Adapala, R. K., Thoppil, R. J., Ghosh, K., Cappelli, H., Dudley, A. C., Paruchuri, S., et al. (2016). Activation of Mechanosensitive Ion Channel TRPV4 Normalizes Tumor Vasculature and Improves Cancer Therapy. J. Autism Dev. Disord. 35 (3), 314–322. doi: 10.1097/CCM.0b013e31823da96d.Hydrogen
Ahmmed, G. U., Mehta, D., Vogel, S., Holinstat, M., Paria, B. C., Tiruppathi, C., et al. (2004). Protein Kinase Cα Phosphorylates the TRPC1 Channel and Regulates Store-Operated Ca 2 Entry in Endothelial Cells *. J. Biol. Chem. 279 (20), 20941–20949. doi: 10.1074/jbc.M313975200
Almasi, S., Sterea, A. M., Fernando, W., Clements, D. R., Marcato, P., Hoskin, D. W., et al. (2019). TRPM2 Ion Channel Promotes Gastric Cancer Migration, Invasion and Tumor Growth through the AKT Signaling Pathway. Sci. Rep. 9 (1), 4182. doi: 10.1038/s41598-019-40330-1
Asghar, M. Y., Magnusson, M., Kemppainen, K., Sukumaran, P., Löf, C., Pulli, I., et al. (2015). Transient Receptor Potential Canonical 1 (TRPC1) Channels as Regulators of Sphingolipid and VEGF Receptor Expression: Implications for Thyroid Cancer Cell Migration and Proliferation. J. Biol. Chem. 290 (26), 16116–16131. doi: 10.1074/jbc.M115.643668
Ashida, S., Nakagawa, H., Katagiri, T., Furihata, M., Iiizumi, M., Anazawa, Y., et al. (2004). Molecular Features of the Transition from Prostatic Intraepithelial Neoplasia (PIN) to Prostate Cancer: Genome-Wide Gene-Expression Profiles of Prostate Cancers and PINs. Cancer Res. 64 (17), 5963–5972. doi: 10.1158/0008-5472.CAN-04-0020
Aspenström, P. (2004). Integration of Signalling Pathways Regulated by Small GTPases and Calcium. Biochim. Biophys. Acta - Mol. Cell Res. 1742 (1–3), 51–58. doi: 10.1016/j.bbamcr.2004.09.029
Azimi, I., Milevskiy, M. J. G., Kaemmerer, E., Turner, D., Yapa, K. T. D. S., Brown, M. A., et al. (2017). TRPC1 Is a Differential Regulator of Hypoxia-Mediated Events and Akt Signalling in PTEN-Deficient Breast Cancer Cells. J. Cell Sci. 130 (14), 2292–2305. doi: 10.1242/jcs.196659
Bader, M. F., Doussau, F., Chasserot-Golaz, S., Vitale, N., Gasman, S (2004). Coupling Actin and Membrane Dynamics during Calcium-Regulated Exocytosis: A Role for Rho and ARF GTPases. Biochim. Biophys. Acta - Mol. Cell Res. 1742 (1–3), 37–49. doi: 10.1016/j.bbamcr.2004.09.028
Baldoli, E., Castiglioni, S., Maier, J. A.M. (2013). Regulation and Function of TRPM7 in Human Endothelial Cells: TRPM7 as a Potential Novel Regulator of Endothelial Function. PloS One 8 (3), e598915. doi: 10.1371/journal.pone.0059891
Bernardini, M., Pla, A. F., Prevarskaya, N., Gkika, D. (2015). Human Transient Receptor Potential (TRP) Channels Expression Profiling in Carcinogenesis. Int. J. Dev. Biol. 59 (7–9), 399–406. doi: 10.1387/ijdb.150232dg
Berridge, M. J., Bootman, M. D., Roderick, H. L. (2003). CALCIUM SIGNALLING : DYNAMICS, HOMEOSTASIS AND REMODELLING. Nat. Rev. Mol. Cell Biol. 4 (7), 517–529. doi: 10.1038/nrm1155
Betson, M., Braga, V. M.M. (2003). Tumor Progression : Small GTPases and Loss of Cell – Cell Adhesion. Bioessays 25 (5), 452–635. doi: 10.1002/bies.10262
Bezzerides, V. J., Ramsey, I. S., Kotecha, S., Greka, A., Clapham, D. E. (2004). Rapid Vesicular Translocation and Insertion of TRP Channels. Nat. Cell Biol. 6 (8), 709–720. doi: 10.1038/ncb1150
Bidaux, G., Roudbaraki, M., Merle, C., Crépin, A., Delcourt, P., Slomianny, C., et al. (2005). Evidence for Specific TRPM8 Expression in Human Prostate Secretory Epithelial Cells: Functional Androgen Receptor Requirement. Endoc.-Rel. Cancer 12 (2), 367–382. doi: 10.1677/erc.1.00969
Birukova, A. A., Smurova, K., Birukov, K. G., Kaibuchi, K., Garcia, J. G.N., Verin, A. D. (2004). Role of Rho GTPases in Thrombin-Induced Lung Vascular Endothelial Cells Barrier Dysfunction. Microvascular 67, 64–77. doi: 10.1016/j.mvr.2003.09.007
Boettner, B., Van Aelst, L. (2009). Control of Cell Adhesion Dynamics by Rap1 Signaling. Curr. Opin. Cell Biol. 21 (5), 684–693. doi: 10.1016/j.ceb.2009.06.004
Bomben, V. C., Turner, K. L., Barclay, T. C., Sontheimer, H. (2011). Transient Receptor Potential Canonical Channels Are Essential for Chemotactic Migration of Human Malignant Gliomas. J. Cell Physiol. 226 (7), 1879–1888. doi: 10.1161/CIRCULATIONAHA.110.956839
Brossa, A., Buono, L., Fallo, S., Fiorio Pla, A., Munaron, L., Bussolati, B. (2019). Alternative Strategies to Inhibit Tumor Vascularization. Int. J. Mol. Sci. 20 (24), 6180. doi: 10.3390/ijms20246180
Bryan, B. A., D’Amore, P. A. (2007). What Tangled Webs They Weave: Rho-GTPase Control of Angiogenesis. Cell. Mol. Life Sci. 64 (16), 2053–2065. doi: 10.1007/s00018-007-7008-z
Cáceres, M., Ortiz, L., Recabarren, T., Romero, A., Colombo, A., Leiva-Salcedo, E., et al. (2015). TRPM4 Is a Novel Component of the Adhesome Required for Focal Adhesion Disassembly, Migration and Contractility. PloS One 10 (6), 1–23. doi: 10.1371/journal.pone.0130540
Cai, N., Lou, L., Al-Saadi, N., Tetteh, S., Runnels, L. W. (2018). The Kinase Activity of the Channel-Kinase Protein TRPM7 Regulates Stability and Localization of the TRPM7 Channel in Polarized Epithelial Cells. J. Biol. Chem. 293 (29), 11491–11504. doi: 10.1074/jbc.RA118.001925
Canales, J., Morales, D., Blanco, C., Rivas, J., Diaz, N., Angelopoulos, I., et al. (2019). A Tr(i)p to Cell Migration: New Roles of Trp Channels in Mechanotransduction and Cancer. Front. Physiol. 10:757 (JUN). doi: 10.3389/fphys.2019.00757
Cao, R., Meng, Z., Liu, T., Wang, G., Qian, G., Cao, T. (2016). Decreased TRPM7 Inhibits Activities and Induces Apoptosis of Bladder Cancer Cells via ERK1 / 2 Pathway. Oncotarget 7 (45), 72941–72960. doi: 10.18632/oncotarget.12146
Cappelli, H. C., Kanugula, A. K., Adapala, R. K., Amin, V., Sharma, P., Midha, P., et al. (2019). Mechanosensitive TRPV4 Channels Stabilize VE-Cadherin Junctions to Regulate Tumor Vascular Integrity and Metastasis. Cancer Lett. 442, 15–20. doi: 10.1016/j.canlet.2018.07.042
Caprodossi, S., Lucciarini, R., Amantini, C., Nabissi, M., Canesin, G., Ballarini, P., et al. (2008). Transient Receptor Potential Vanilloid Type 2 (TRPV2) Expression in Normal Urothelium and in Urothelial Carcinoma of Human Bladder: Correlation with the Pathologic Stage. Eur. Urol. 54 (3), 612–620. doi: 10.1016/j.eururo.2007.10.016
Carmeliet, P., Jain, R. K. (2000). Angiogenesis in Cancer and Other Diseases. Nature 407 (6801), 249–257. doi: 10.1038/35025220
Carmeliet, P., Jain, R. K. (2011). Principles and Mechanisms of Vessel Normalization for Cancer and Other Angiogenic Diseases. Nat. Rev. Drug Discovery 10 (6), 417–427. doi: 10.1038/ndr3455
Carmona, G., Go, S., Orlandi, A., Ba, T., Jugold, M., Kiessling, F., et al. (2009). Role of the Small GTPase Rap1 for Integrin Activity Regulation in Endothelial Cells and Angiogenesis. Blood 113 (2), 488–497. doi: 10.1182/blood-2008-02-138438
Chen, J. P., Wang, J., Luan, Y., Wang, C. X., Li, W. H., Zhang, J. B., et al. (2015). TRPM7 Promotes the Metastatic Process in Human Nasopharyngeal Carcinoma. Cancer Lett. 356 (2), 483–490. doi: 10.1016/j.canlet.2014.09.032
Chen, L., Cao, R., Wang, G., Yuan, L., Qian, G. (2017). Downregulation of TRPM7 Suppressed Migration and Invasion by Regulating Epithelial – Mesenchymal Transition in Prostate Cancer Cells. Med. Oncol. 34 (7), 1–11. doi: 10.1007/s12032-017-0987-1
Chen, Z., Zhu, Y., Dong, Y., Zhang, P., Han, X., Jin, J., et al. (2017). Overexpression of TrpC5 Promotes Tumor Metastasis via the HIF-1α-Twist Signaling Pathway in Colon Cancer. Clin. Sci. 131 (19), 2439–2450. doi: 10.1042/CS20171069
Cherfils, J., Zeghouf, M. (2013). Regulation of Small GTPases by GEFs, GAPs, and GDIs. Physiol. Rev. 93 (1), 269–309. doi: 10.1152/physrev.00003.2012
Chigurupati, S., Venkataraman, R., Barrera, D., Naganathan, A., Madan, M., Paul, L., et al. (2010). Receptor Channel TRPC6 Is a Key Mediator of Notch-Driven Glioblastoma Growth and Invasiveness. Cancer Res. 6 (11), 418–428. doi: 10.1158/0008-5472.CAN-09-2654
Chrzanowska-wodnicka, M., Kraus, A. E., Gale, D., Ii, G. C.W., Vansluys, J. (2008). Defective Angiogenesis, Endothelial Migration, Proliferation, and MAPK Signaling in Rap1b-Deficient Mice. Blood 111 (5), 2647–2656. doi: 10.1182/blood-2007-08-109710
Chrzanowska-Wodnicka, M. (2010). Regulation of Angiogenesis by a Small GTPase Rap1. Vasc. Pharmacol. 53 (1–2), 1–10. doi: 10.1016/j.vph.2010.03.003
Chung, H. K., Rathor, N., Wang, S. R., Wang, J. Y., Rao, J. N. (2015). RhoA Enhances Store-Operated Ca2+ Entry and Intestinal Epithelial Restitution by Interacting with TRPC1 after Wounding. Am. J. Physiol. - Gastrointest. Liver Physiol. 309 (9), G759–G767. doi: 10.1152/ajpgi.00185.2015
Clark, K., Middelbeek, J., Lasonder, E., Dulyaninova, N. G., Morrice, A., Ryazanov, A. G., et al. (2008). TRPM7 Regulates Myosin IIA Filament Stability and Protein Localization by Heavy Chain Phosphorylation. J. Mol. Biol. 378 (4), 790–803. doi: 10.1016/j.jmb.2008.02.057
Clayton, N. S., Ridley, A. J. (2020). Targeting Rho GTPase Signaling Networks in Cancer. Front. Cell Dev. Biol. 8:222. doi: 10.3389/fcell.2020.00222
Correll, R. N., Pang, C., Niedowicz, D. M., Finlin, B. S., Andres, D. A. (2008). The RGK Family of GTP-Binding Proteins: Regulators of Voltage-Dependent Calcium Channels and Cytoskeleton Remodeling. Cell. Signal. 20 (2), 292–300. doi: 10.1016/j.cellsig.2007.10.028
Cullen, P. J., Lockyer, P. J. (2002). Integration of Calcium and Ras Signalling. Nat. Rev. Mol. Cell Biol. 3 (5), 339–348. doi: 10.1038/nrm808
Davis, F. M., Azimi, I., Faville, R. A., Peters, A. A., Jalink, K., Putney, J. W., Jr., et al. (2014). Induction of Epithelial – Mesenchymal Transition (EMT ) in Breast Cancer Cells Is Calcium Signal Dependent. Oncogene 33 (18), 2307–2316. doi: 10.1038/onc.2013.187
Desai, B. N., Krapivinsky, G., Navarro, B., Krapivinsky, L., Carter, B. C., Febvay, S., et al. (2012). Cleavage of TRPM7 Releases the Kinase Domain from the Ion Channel and Regulates Its Participation in Fas-Induced Apoptosis. Dev. Cell 22 (6), 1149–1162. doi: 10.1016/j.devcel.2012.04.006
Dhennin-duthille, I., Gautier, M., Faouzi, M., Brevet, M., Vaudry, D., Ahidouch, A., et al. (2011). Cellular Physiology and Biochemistr y Biochemistry High Expression of Transient Receptor Potential Channels in Human Breast Cancer Epithelial Cells and Tissues: Correlation with Pathological Parameters. Cell Physiol. Biochem. 28, 813–822.
Dong, H., Shim, K. N., Li, J. M. J., Estrema, C., Ornelas, T. A., Nguyen, F., et al. (2010). Molecular Mechanisms Underlying Ca2+-Mediated Motility of Human Pancreatic Duct Cells. Am. J. Physiol. - Cell Physiol. 299 (6), 1493–1503. doi: 10.1152/ajpcell.00242.2010
Elbaz, M., Ahirwar, D., Xiaoli, Z., Zhou, X., Lustberg, M., Nasser, M. W., et al. (2018). TRPV2 Is a Novel Biomarker and Therapeutic Target in Triple Negative Breast Cancer. Oncotarget 9 (71), 33459–33470. doi: 10.18632/oncotarget.9663
Fabian, A., Fortmann, T., Dieterich, P., Riethmüller, C., Schön, P., Mally, S., et al. (2008). TRPC1 Channels Regulate Directionality of Migrating Cells. Pflugers Archiv. Eur. J. Physiol. 457 (2), 475–484. doi: 10.1007/s00424-008-0515-4
Faouzi, M., Kilch, T., Horgen, F. D., Fleig, A., Penner, R. (2017). The TRPM7 Channel Kinase Regulates Store-Operated Calcium Entry. J. Physiol. 595 (10), 3165–3180. doi: 10.1113/JP274006
Fels, B., Bulk, E., Pethő, Z., Schwab, A. (2018). The Role of TRP Channels in the Metastatic Cascade. Pharmaceuticals 11 (2), 48. doi: 10.3390/ph11020048
Fiorio Pla, A. F., Gkika, D. (2013). Emerging Role of TRP Channels in Cell Migration : From Tumor Vascularization to Metastasis. Front. Physiol. 4, 1–12. doi: 10.3389/fphys.2013.00311
Fiorio Pla, A., Ong, H. L., Cheng, K. T., Brossa, A., Bussolati, B., Lockwich, T., et al. (2012). TRPV4 Mediates Tumor-Derived Endothelial Cell Migration via Arachidonic Acid-Activated Actin Remodeling. Oncogene 31 (2), 200–212. doi: 10.1016/j.physbeh.2017.03.040
Fuessel, S., Sickert, D., Meye, A., Klenk, U., Schmidt, U., Schmitz, M., et al. (2003). Multiple Tumor Marker Analyses (PSA, HK2, PSCA, Trp-P8) in Primary Prostate Cancers Using Quantitative RT-PCR. Int. J. Oncol. 23 (1), 221–228. doi: 10.3892/ijo.23.1.221
Gao, G., Wang, W., Tadagavadi, R. K., Briley, N. E., Love, M., II, Miller, B. A., et al. (2014). TRPM2 Mediates Ischemic Kidney Injury and Oxidant Stress through RAC1. J. Clin. Invest. 124 (11), 4989–5001. doi: 10.1172/JCI76042.us
Genova, T., Grolez, G. P., Camillo, C., Bernardini, M., Bokhobza, A., Richard, E., et al. (2017). TRPM8 Inhibits Endothelial Cell Migration via a Nonchannel Function by Trapping the Small GTPase Rap1. J. Cell Biol. 216 (7), 2107–2130. doi: 10.1083/jcb.201506024
Ghosh, K., Thodeti, C. K., Dudley, A. C., Mammoto, A., Klagsbrun, M., Ingber, D. E. (2008). Tumor-Derived Endothelial Cells Exhibit Aberrant Rho-Mediated Mechanosensing and Abnormal Angiogenesis in Vitro. Proc. Natl. Acad. Sci. U. S. A. 105 (32), 11305–11310. doi: 10.1073/pnas.0800835105
Gkika, D., Prevarskaya, N. (2011). TRP Channels in Prostate Cancer: The Good, the Bad and the Ugly? Asian J. Androl. 13 (5), 673–676. doi: 10.1038/aja.2011.18
Greka, A., Navarro, B., Oancea, E., Duggan, A., Clapham, D. E. (2003). TRPC5 Is a Regulator of Hippocampal Neurite Length and Growth Cone Morphology. Nat. Neurosci. 6 (8), 837–845. doi: 10.1038/nn1092
Guéguinou, M., Harnois, T., Crottes, D., Uguen, A., Deliot, N., Gambade, A., et al. (2016). SK3/TRPC1/Orai1 Complex Regulates SOCE-Dependent Colon Cancer Cell Migration: A Novel Opportunity to Modulate Anti- EGFR MAb Action by the Alkyl-Lipid Ohmline. Oncotarget 7 (24), 36168–36184. doi: 10.18632/oncotarget.8786
Guilbert, A., Dhennin-Duthille, I., Hiani, Y. E. L., Haren, N., Khorsi, H., Sevestre, H., et al. (2008). Expression of TRPC6 Channels in Human Epithelial Breast Cancer Cells. BMC Cancer 8, 1–11. doi: 10.1186/1471-2407-8-125
Guilbert, A., Gautier, M., Dhennin-Duthille, I., Rybarczyk, P., Sahni, J., Sevestre, H., et al. (2013). Transient Receptor Potential Melastatin 7 Is Involved in Oestrogen Receptor-Negative Metastatic Breast Cancer Cells Migration through Its Kinase Domain. Eur. J. Cancer 49 (17), 3694–3707. doi: 10.1016/j.ejca.2013.07.008
Haws, H. J., Mcneil, M. A., Hansen, M. D.H. (2016). Control of Cell Mechanics by RhoA and Calcium Fl Uxes during Epithelial Scattering. Tissue Barriers 4 (3), 1–155. doi: 10.1080/21688370.2016.1187326
Hicks, K., Neil, R. G.O., Dubinsky, W. S., Brown, R. C., Hicks, K., O Neil, R. G., et al. (2010). TRPC-Mediated Actin-Myosin Contraction Is Critical for BBB Disruption Following Hypoxic Stress. Am. J. Physiol. Cell Physiol. 298, 1583–1593. doi: 10.1152/ajpcell.00458.2009
Ho, H.-h., Chang, C.-s., Ho, W.-c., Liao, S.-y., Wu, C.-h. (2010). Anti-Metastasis Effects of Gallic Acid on Gastric Cancer Cells Involves Inhibition of NF- j B Activity and Downregulation of PI3K / AKT / Small GTPase Signals. Food Chem. Toxicol. 48 (8–9), 2508–2516. doi: 10.1016/j.fct.2010.06.024
Hobbs, G. A., Der, C. J., Rossman, K. L. (2016). RAS Isoforms and Mutations in Cancer at a Glance. J. Cell Sci. 129 (7), 1287–1292. doi: 10.1242/jcs.182873
Holzmann, C., Kappel, S., Kilch, T., Jochum, M. M., Urban, S. K., Jung, V., et al. (2015). Transient Receptor Potential Melastatin 4 Channel Contributes to Migration of Androgen-Insensitive Prostate Cancer Cells. Oncotarget 6 (39), 41783–41793. doi: 10.18632/oncotarget.6157
Huang, Y. W., Chang, S. J., Harn, H., II, Huang, H. T., Lin, H. H., Shen, M. R., et al. (2015). Mechanosensitive Store-Operated Calcium Entry Regulates the Formation of Cell Polarity. J. Cell. Physiol. 230 (9), 2086–2097. doi: 10.1002/jcp.24936
Iamshanova, O., Pla, A. F., Prevarskaya, N. (2017). Molecular Mechanisms of Tumour Invasion: Regulation by Calcium Signals. J. Physiol. 595 (10), 3063–3075. doi: 10.1113/JP272844
Iftinca, M., Hamid, J., Chen, L., Varela, D., Tadayonnejad, R., Altier, C., et al. (2007). Regulation of T-Type Calcium Channels by Rho-Associated Kinase. Nat. Neurosci. 10 (7), 854–860. doi: 10.1038/nn1921
Jabłońska-trypuć, A., Matejczyk, M., Rosochacki, S. (2016). Matrix Metalloproteinases (MMPs ), the Main Extracellular Matrix (ECM ) Enzymes in Collagen Degradation, as a Target for Anticancer Drugs Enzymes in Collagen Degradation, as a Target for Anticancer Drugs. J. Enzyme Inhib. Med. Chem. 31 (sup1), 177–183. doi: 10.3109/14756366.2016.1161620
Jacob, A., Jing, J., Lee, J., Schedin, P., Gilbert, S. M., Peden, A. A., et al. (2013). Rab40b Regulates Trafficking of MMP2 and MMP9 during Invadopodia Formation and Invasion of Breast Cancer Cells. J. Cell Sci. 126 (Pt20), 4647–4658. doi: 10.1242/jcs.126573
Jiang, L. H., Gamper, N., Beech, D. J. (2011). Properties and Therapeutic Potential of Transient Receptor Potential Channels with Putative Roles in Adversity: Focus on TRPC5, TRPM2 and TRPA1. Curr. Drug Targets 12 (5), 724–736. doi: 10.2174/138945011795378568
Kim, J. G., Islam, R., Cho, J. Y., Jeong, H., Cap, K. C., Park, Y., et al. (2018). Regulation of RhoA GTPase and Various Transcription Factors in the RhoA Pathway. J. Cell. Physiol. 233 (9), 6381–6392. doi: 10.1002/jcp.26487
Koike, T., Kuzuya, M., Asai, T., Kanda, S., Cheng, X. W. (2000). Activation of MMP-2 by Clostridium Difficile Toxin B in Bovine Smooth Muscle Cells. Biochem. Biophys. Res. Commun. 46, 43–46. doi: 10.1006/bbrc.2000.3630
Koopman, W. J. H., Bosch, R. R., Van Emst-De Vries, S. E., Spaargaren, M., De Pont Jan Joep, H. H. M., Willems, P. H. G. M. (2003). R-Ras Alters Ca2+ Homeostasis by Increasing the Ca2+ Leak across the Endoplasmic Reticular Membrane. J. Biol. Chem. 278 (16), 13672–13679. doi: 10.1074/jbc.M211256200
Laragione, T., Cheng, K. F., Tanner, M. R., He, M., Beeton, C., Al-Abed, Y., et al. (2015). THE CATION CHANNEL TRPV2 IS A NEW SUPPRESSOR OF ARTHRITIS SEVERITY, JOINT DAMAGE AND SYNOVIAL FIBROBLAST INVASION. Clin. Immunol. 158 (2), 183–192. doi: 10.1016/j.clim.2015.04.001
Laragione, T., Harris, C., Gulko, P. S. (2019). TRPV2 Suppresses Rac1 and RhoA Activation and Invasion in Rheumatoid Arthritis Fibroblast-like Synoviocytes. Int. Immunopharmacol. 70, 268–273. doi: 10.1016/j.intimp.2019.02.051
Lastraioli, E., Iorio, J., Arcangeli, A. (2015). Ion Channel Expression as Promising Cancer Biomarker. Biochim. Biophys. Acta - Biomembr. 1848 (10), 2685–2702. doi: 10.1016/j.bbamem.2014.12.016
Lee, W. H., Choong, L. Y., Mon, N. N., Lu, S., Lin, Q., Pang, B., et al. (2016). “TRPV4 Regulates Breast Cancer Cell Extravasation, Stiffness and Actin Cortex. Sci. Rep. 6 (May), 1–16. doi: 10.1038/srep27903
Lee, W. H., Choong, L. Y., Jin, T. H., Mon, N. N., Chong, S., Liew, C. S., et al. (2017). TRPV4 Plays a Role in Breast Cancer Cell Migration via Ca2+-Dependent Activation of AKT and Downregulation of E-Cadherin Cell Cortex Protein. Oncogenesis 6 (5), e338. doi: 10.1038/oncsis.2017.39
Lehen’kyi, V., Prevarskaya, N. (2011). “Oncogenic TRP Channels.” in Transient Receptor Potential Channels. Advances in Experimental Medicine and Biology. Ed. Islam, M. (United States: Springer), 929–945.
Lepannetier, S., Zanou, N, Yerna, X., Emeriau, N., Dufour, I., Masquelier, J., et al. (2016). Sphingosine-1-Phosphate-Activated TRPC1 Channel Controls Chemotaxis of Glioblastoma Cells. Cell Calcium 60 (6), 373–383. doi: 10.1016/j.ceca.2016.09.002
Li, S., Balmain, A., Counter, C. M. (2018). A Model for RAS Mutation Patterns in Cancers: Finding the Sweet Spot. Nat. Rev. Cancer 18 (12), 767–777. doi: 10.1038/s41568-018-0076-6
Litan, A., Langhans, S. A. (2015). Cancer as a Channelopathy: Ion Channels and Pumps in Tumor Development and Progression. Front. Cell. Neurosci. 9:86 (March). doi: 10.3389/fncel.2015.00086
Liu, J., Chen, Y., Shuai, S., Ding, D. (2014). TRPM8 Promotes Aggressiveness of Breast Cancer Cells by Regulating EMT via Activating AKT / GSK-3 β Pathway. Tumour Biol. 35 (9), 8969–8977. doi: 10.1007/s13277-014-2077-8
Liu, K., Xu, S.-h., Chen, Z., Zeng, Q.-x., Li, Z.-j., Chen, Z.-m. (2018). TRPM7 Overexpression Enhances the Cancer Stem Cell-like and Metastatic Phenotypes of Lung Cancer through Modulation of the Hsp90 α / UPA / MMP2 Signaling Pathway. BMC Cancer 18 (1), 1167. doi: 10.1186/s12885-018-5050-x
Liu, L., Wu, N., Wang, Y., Zhang, X., Xia, B., Tang, J., et al. (2019). TRPM7 Promotes the Epithelial – Mesenchymal Transition in Ovarian Cancer through the Calcium-Related PI3K / AKT Oncogenic Signaling. J. Exp. Clin. Cancer Res. 38 (1), 106–121. doi: 10.1186/s13046-019-1061-y
Llense, F., Etienne-Manneville, S. (2015). “Front-to-Rear Polarity in Migrating Cells.” in Cell Polarity 1. Ed. Klaus, E. (United States: Springer), 115–146. doi: 10.1007/978-3-319-14463-4_5
Luo, Y., Wu, J.-y., Lu, M.-h., Shi, Z., Na, N., Di, J.-m. (2016). Carvacrol Alleviates Prostate Cancer Cell Proliferation, Migration, and Invasion through Regulation of PI3K / Akt and MAPK Signaling Pathways. Oxid. Med. Cell Longev. 2016, 1469693. doi: 10.1155/2016/1469693
Mehta, D., Ahmmed, G. U., Paria, B. C., Holinstat, M., Voyno-Yasenetskaya, T., Tiruppathi, C., et al. (2003). RhoA Interaction with Inositol 1,4,5-Trisphosphate Receptor and Transient Receptor Potential Channel-1 Regulates Ca2+ Entry: Role in Signaling Increased Endothelial Permeability. J. Biol. Chem. 278 (35), 33492–33500. doi: 10.1074/jbc.M302401200
Meng, X., Cai, C., Wu, J., Cai, S., Ye, C., Chen, H., et al. (2013). TRPM7 Mediates Breast Cancer Cell Migration and Invasion through the MAPK Pathway. Cancer Lett. 333 (1), 96–102. doi: 10.1016/j.canlet.2013.01.031
Middelbeek, J., Kuipers, A. J., Henneman, L., Visser, D., Eidhof, I., Van Horssen, R., et al. (2012). TRPM7 Is Required for Breast Tumor Cell Metastasis. Cancer Res. 72 (16), 4250–4261. doi: 10.1158/0008-5472.CAN-11-3863
Mishra, A. K., Lambright, D. G. (2016). Small GTPases and Their GAPs. Biopolymers 105 (8), 431–448. doi: 10.1002/bip.22833
Moissoglu, K., Schwartz, M. A. (2014). Spatial and Temporal Control of Rho GTPase Functions. Cell. Logistics 4 (2), e943618. doi: 10.4161/21592780.2014.943618
Monet, M., Lehen, V., Gackiere, F., Firlej, V., Vandenberghe, M., Roudbaraki, M., et al. (2010). Role of Cationic Channel TRPV2 in Promoting Prostate Cancer Migration and Progression to Androgen Resistance. Cancer Res. 70 (3), 1225–1236. doi: 10.1158/0008-5472.CAN-09-2205
Monteith, G. R., Prevarskaya, N., Roberts-Thomson, S. J. (2017). The Calcium–cancer Signalling Nexus. Nat. Rev. Cancer 17 (6), 367–380. doi: 10.1038/nrc.2017.18
Mrkonjić, S., Garcia-Elias, A., Pardo-Pastor, C., Bazellières, E., Trepat, X., Vriens, J., et al. (2015). TRPV4 Participates in the Establishment of Trailing Adhesions and Directional Persistence of Migrating Cells. Pflugers Archiv. Eur. J. Physiol. 467 (10), 2107–2119. doi: 10.1007/s00424-014-1679-8
Nagasawa, M., Kojima, I. (2015). Translocation of TRPV2 Channel Induced by Focal Administration of Mechanical Stress. Physiol. Rep. 3, 1–12. doi: 10.14814/phy2.12296
Narayan, G., Bourdon, V., Chaganti, S., Arias-Pulido, H., Nandula, S. V., Rao, P. H., et al. (2007). Gene Dosage Alterations Revealed by CDNA Microarray Analysis in Cervical Cancer: Identification of Candidate Amplified and Overexpressed Genes. Genes Chromosomes Cancer 46 (4), 373–384. doi: 10.1002/gcc.20418
Negri, S., Faris, P., Berra-Romani, R., Guerra, G., Moccia, F. (2020). Endothelial Transient Receptor Potential Channels and Vascular Remodeling: Extracellular Ca2 + Entry for Angiogenesis, Arteriogenesis and Vasculogenesis. Front. Physiol. 10, 1618 (January). doi: 10.3389/fphys.2019.01618
Nilius, B., Owsianik, G. (2011). The Transient Receptor Potential Family of Ion Channels. Genome Biol. 12 (3), 218. doi: 10.1186/gb-2011-12-3-218
Okamoto, Y., Ohkubo, T., Ikebe, T., Yamazaki, J. U. N. (2012). Blockade of TRPM8 Activity Reduces the Invasion Potential of Oral Squamous Carcinoma Cell Lines. Int. J. Oncol. 40 (5), 1431–1440. doi: 10.3892/ijo.2012.1340
Oulidi, A., Bokhobza, A., Gkika, D., Vanden Abeele, F., Lehen, V., Houcine Ouafik, L., et al. (2013). TRPV2 Mediates Adrenomedullin Stimulation of Prostate and Urothelial Cancer Cell Adhesion, Migration and Invasion. PLoS One 8 (5), 1–7. doi: 10.1371/journal.pone.0064885
Ou-yang, Q., Li, B., Xu, M., Liang, H. (2018). TRPV4 Promotes the Migration and Invasion of Glioma Cells via AKT/Rac1 Signaling. Biochem. Biophys. Res. Commun. 503 (2), 876–881. doi: 10.1016/j.bbrc.2018.06.090
Prior, I. A., Lewis, P. D., Mattos, C. (2012). A Comprehensive Survey of Ras Mutations in Cancer. Cancer Res. 72 (10), 2457–2467. doi: 10.1158/0008-5472.CAN-11-2612.A
Rampino, T., Gregorini, M., Guidetti, C., Broggini, M., Marchini, S., Bonomi, R., et al. (2007). KCNA1 and TRPC6 Ion Channels and NHE1 Exchanger Operate the Biological Outcome of HGF / Scatter Factor in Renal Tubular Cells. Growth Factors 25, 382–391. doi: 10.1080/08977190801892184
Ridley, A. J. (2001). Rho Family Proteins : Coordinating Cell Responses. Growth Factors 11 (12), 471–477. doi: 10.1016/s0962-8924(01)02153-5
Roche, J. (2018). The Epithelial-to-Mesenchymal Transition in Cancer. Cancers 10 (3), 10–13. doi: 10.3390/cancers10020052
Rybarczyk, P., Gautier, M., Hague, F., Dhennin-Duthille, I., Chatelain, D., Kerr-Conte, J., et al. (2012). Transient Receptor Potential Melastatin-Related 7 Channel Is Overexpressed in Human Pancreatic Ductal Adenocarcinomas and Regulates Human Pancreatic Cancer Cell Migration. Int. J. Cancer 131 (6), 851–861. doi: 10.1002/ijc.27487
Rybarczyk, P., Vanlaeys, A., Brassart, B., Dhennin-duthille, I., Chatelain, D., Sevestre, H., et al. (2017). The Transient Receptor Potential Melastatin 7 Channel Regulates Pancreatic Cancer Cell Invasion through the Hsp90 α / UPA / MMP2. Neoplasia 19 (4), 288–300. doi: 10.1016/j.neo.2017.01.004
Sahai, E., Marshall, C. J. (2002). RHO - GTPases and Cancer. Nat. Rev. Cancer 2 (2), 133–142. doi: 10.1038/nrc725
Santibáñez, J. F., Kocic, J., Fabra, A., Cano, A., Quintanilla, M. (2010). Rac1 Modulates TGF- b 1-Mediated Epithelial Cell Plasticity and MMP9 Production in Transformed Keratinocytes. FEBS Lett. 584 (11), 2305–2310. doi: 10.1016/j.febslet.2010.03.042
Scarpellino, S., Munaron, L., Cantelmo, A. R., Fiorio Pla, A. (2020). Calcium-Permeable Channels in Tumor Vascularization: Peculiar Sensors of Microenvironmental Chemical and Physical Cues. Rev. Physiol. Biochem. Pharmacol. doi: 10.1007/112_2020_32
Seiz, J. R., Klinke, J., Scharlibbe, L., Lohfink, D., Heipel, M. (2020). Different Signaling and Functionality of Rac1 and Rac1b in the Progression of Lung Adenocarcinoma. Biol. Chem. 401 (4), 517–531. doi: 10.1515/hsz-2019-0329
Shapovalov, G., Ritaine, A., Skryma, R., Prevarskaya, N. (2016). Role of TRP Ion Channels in Cancer and Tumorigenesis. Semin. Immunopathol. 38 (3), 357–369. doi: 10.1007/s00281-015-0525-1
Shields, J. M., Pruitt, K., Shaub, A., Der, C. J. (2000). Understanding Ras : ‘ It Ain ‘ t over ‘ Til It ‘ s over ‘. Trends Cell Biol. 10 (4), 147–154. doi: 10.1016/s0962-8924(00)01740-2
Simon, A. R., Vikis, H. G., Steward, S., Fanburg, B. L., Cochran, B. H., Guan, K.-L. (2000). Regulation of STAT3 by Direct Binding to the Racl GTPase. Science 290 (5489), 144–147. doi: 10.1126/science.290.5489.144
Singh, J., Manickam, P., Shmoish, M., Natik, S., Denyer, G., Handelsman, D., et al. (2006). Annotation of Androgen Dependence to Human Prostate Cancer-Associated Genes by Microarray Analysis of Mouse Prostate. Cancer Lett. 237 (2), 298–304. doi: 10.1016/j.canlet.2005.06.008
Singh, I., Knezevic, N., Ahmmed, G. U., Kini, V., Malik, A. B., Mehta, D. (2007). Gαq -TRPC6-Mediated Ca 2+ Entry Induces RhoA Activation and Resultant Endothelial Cell Shape Change in Response to Thrombin. J. Biol. Chem. 282 (11), 7833–7843. doi: 10.1074/jbc.M608288200
Sokabe, T., Tominaga, M. (2010). The TRPV4 Cation Channel. Communicative Integr. Biol. 3 (6), 619–621. doi: 10.4161/cib.3.6.13461
Stenmark, H. (2009). Rab GTPases as Coordinators of Vesicle Traffic. Nat. Rev. Mol. Cell Biol. 10 (8), 513–525. doi: 10.1038/nrm2728
Su, L.-t., Liu, W., Chen, H.-c., An, O. G. A.-p., Habas, R., Runnels, L. W., et al. (2011). TRPM7 Regulates Polarized Cell Movements. Biochem. J. 434 (3), 513–521. doi: 10.1042/BJ20101678
Sumoza-Toledo, A., Espinoza-Gabriel, M., II, Montiel-Condado, D. (2016). Evaluación Del Canal TRPM2 Como Biomarcador En Cáncer de Mama Mediante El Análisis de Bases de Datos Públicos. Boletin Medico Del Hosp. Infantil Mexico 73 (6), 397–404. doi: 10.1016/j.bmhimx.2016.10.001
Sun, J., Yang, T., Wang, P., Ma, S., Zhu, Z., Pu, Y., et al. (2014). Activation of Cold-Sensing Transient Receptor Potential Melastatin Subtype 8 Antagonizes Vasoconstriction and Hypertension through Attenuating RhoA/Rho Kinase Pathway. Hypertension 63 (6), 1354–1363. doi: 10.1161/HYPERTENSIONAHA.113.02573
Svensmark, J. H., Brakebusch, C. (2019). Rho GTPases in Cancer: Friend or Foe? Oncogene 38 (50), 7447–7456. doi: 10.1038/s41388-019-0963-7
Thodeti, C. K., Matthews, B., Ravi, A., Mammoto, A., Ghosh, K., Bracha, A. L., et al. (2009). TRPV4 Channels Mediate Cyclic Strain-Induced Endothelial Cell Reorientation through Integrin-to-Integrin Signaling. Circ. Res. 104 (9), 1123–1130. doi: 10.1161/CIRCRESAHA.108.192930
Thodeti, C. K., Paruchuri, S., Meszaros, J. G. (2013). A TRP to Cardiac Fibroblast Differentiation. Channels 7 (3), 211–214. doi: 10.4161/chan.24328
Thoppil, R. J., Cappelli, H. C., Adapala, R. K., Kanugula, A. K., Paruchuri, S., Thodeti, C. K. (2016). TRPV4 Channels Regulate Tumor Angiogenesis via Modulation of Rho/Rho Kinase Pathway. Oncotarget 7 (18), 25849–25861. doi: 10.18632/oncotarget.8405
Tian, D., Jacobo, S. M.P., Billing, D., Rozkalne, A., Gage, S. D., Anagnostou, T., et al. (2010). Antagonistic Regulation Od Actin Dynamics and Cell Motility by TRPC5 and TRPC6 Channels. Sci. Signal. 3 (145), 1–25. doi: 10.1126/scisignal.2001200.Antagonistic
Tomasek, J. J., Vaughan, M. B., Kropp, B. P., Gabbiani, G., Martin, M. D., Hinz, B. (2006). Contraction of Myofibroblasts in Granulation Tissue Is Dependent on Rho / Rho Kinase / Myosin Light Chain Phosphatase Activity. Wound Repair Regen. 14 (3), 313–320. doi: 10.1111/j.1743-6109.2006.00126.x
Tsavaler, L., Shapero, M. H., Morkowski, S., Laus, R. (2001). Trp-P8, a Novel Prostate-Specific Gene, Is up-Regulated in Prostate Cancer and Other Malignancies and Shares High Homology with Transient Receptor Potential Calcium Channel Proteins. Cancer Res. 61 (9), 3760–3769.
Ungefroren, H., Witte, D., Lehnert, H. (2018). The Role of Small GTPases of the Rho/Rac Family in TGF-β-Induced EMT and Cell Motility in Cancer. Dev. Dynamics 247 (3), 451–461. doi: 10.1002/dvdy.24505
Vennekens, R., Nilius, B. (2007). “Insights into TRPM4 Function, Regulation and Physiological Role.” in Transient Receptor Potential (TRP) Channels. Handbook of Experimental Pharmacology. Eds. Flockerzi, V., Nilius, B. (United States: Springer), 269–285.
Vetter, I. R., Wittinghofer, A. (2001). The Guanine Nucleotide-Binding Switch in Three Dimensions. Science 294 (5545), 1299–1304. doi: 10.1126/science.1062023
Vrenken, K. S., Jalink, K., van Leeuwen, F. N., Middelbeek, J. (2015). Beyond Ion-Conduction: Channel-Dependent and -Independent Roles of TRP Channels during Development and Tissue Homeostasis. Biochim. Biophys. Acta - Mol. Cell Res. 1863 (6), 1436–1446. doi: 10.1016/j.bbamcr.2015.11.008
Vriens, J., Janssens, A., Prenen, J., Nilius, B., Wondergem, R. (2004). TRPV Channels and Modulation by Hepatocyte Growth Factor / Scatter Factor in Human Hepatoblastoma (HepG2 ) Cells. Cell Calcium 36 (1), 19–28. doi: 10.1016/j.ceca.2003.11.006
Wang, Y., He, H., Srivastava, N., Vikarunnessa, S., Chen, Y. B., Jiang, J., et al. (2012). Plexins Are GTPase-Activating Proteins for Rap and Are Activated by Induced Dimerization. Sci. Signaling 5 (207), 1–25. doi: 10.1126/scisignal.2002636
Wei, C., Wang, X., Chen, M., Ouyang, K., Song, L.-S., Cheng, H. (2009). Calcium Flickers Steer Cell Migration. Nature 457 (7231), 901–905. doi: 10.1038/nature07577.Calcium
Xiao, N., Jiang, L. M., Ge, B., Zhang, T. Y., Zhao, X. K., Zhou, X. (2014). Over-Expression of TRPM8 Is Associated with Poor Prognosis in Urothelial Carcinoma of Bladder. Tumor Biol. 35 (11), 11499–11504. doi: 10.1007/s13277-014-2480-1
Xie, R., Xu, J., Xiao, Y., Wu, J., Wan, H., Tang, B., et al. (2017). Calcium Promotes Human Gastric Cancer via a Novel Coupling of Calcium-Sensing Receptor and TRPV4 Channel. Cancer Res. 77 (23), 6499–6512. doi: 10.1158/0008-5472.CAN-17-0360
Xu, S.-z., Muraki, K., Zeng, F., Li, J., Sukumar, P., Dedman, A. M., et al. (2006). A Sphingosine-1 – Phosphate – Activated Calcium Channel Controlling Vascular Smooth Muscle Cell Motility. Cell. Pharmacol. 98 (11), 1381–1389. doi: 10.1161/01.RES.0000225284.36490.a2.A
Yang, D., Kim, J. (2020). Emerging Role of Transient Receptor Potential (TRP) Channels in Cancer Progression. BMB Rep. 53 (3), 125–132. doi: 10.5483/BMBRep.2020.53.3.016
Yang, J., Liu, W., Lu, X., Fu, Y., Li, L., Luo, Y. (2015). High Expression of Small GTPase Rab3D Promotes Cancer Progression and Metastasis. Oncotarget 6 (13), 11125–11138. doi: 10.18632/oncotarget.3575
Yarwood, S., Cullen, P. J., Kupzig, S. (2006). The GAP1 Family of GTPase-Activating Proteins : Spatial and Temporal Regulators of Small GTPase Signalling. Biochem. Soc. Trans. 34 (5), 846–850. doi: 10.1042/BST0340846
Yee, N. S., Kazi, A. A., Li, Q., Yang, Z., Berg, A., Yee, R. K. (2015). Aberrant Over-Expression of TRPM7 Ion Channels in Pancreatic Cancer: Required for Cancer Cell Invasion and Implicated in Tumor Growth and Metastasis. Biol. Open 4 (4), 507–514. doi: 10.1242/bio.20137088
Yue, D., Wang, Y., Xiao, J. Y., Wang, P., Ren, C. S. (2009). Expression of TRPC6 in Benign and Malignant Human Prostate Tissues. Asian J. Androl. 11 (5), 541–547. doi: 10.1038/aja.2009.53
Zahra, F. T., Sajib, Md S., Ichiyama, Y., Akwii, R. G., Tullar, P. E., Cobos, C., et al. (2019). Endothelial RhoA GTPase Is Essential for in Vitro Endothelial Functions but Dispensable for Physiological in Vivo Angiogenesis. Sci. Rep. 9 (1), 1–15. doi: 10.1038/s41598-019-48053-z
Zeng, Z., Leng, T., Feng, X., Sun, H., Inoue, K., Zhu, L., et al. (2015). Silencing TRPM7 in Mouse Cortical Astrocytes Impairs Cell Proliferation and Migration via ERK and JNK Signaling Pathways. PloS One 10 (3), 1–19. doi: 10.1371/journal.pone.0119912
Zhang, B., Zhang, Y., Wang, Z. X., Zheng, Y. (2000). The Role of Mg2+ Cofactor in the Guanine Nucleotide Exchange and GTP Hydrolysis Reactions of Rho Family GTP-Binding Proteins. J. Biol. Chem. 275 (33), 25299–25307. doi: 10.1074/jbc.M001027200
Zhang, S. S., Wen, J., Yang, F., Cai, X. L., Yang, H., Luo, K. J., et al. (2013). High Expression of Transient Potential Receptor C6 Correlated with Poor Prognosis in Patients with Esophageal Squamous Cell Carcinoma. Med. Oncol. 30 (3), 607. doi: 10.1007/s12032-013-0607-7
Zhao, W., Xu, H. (2016). High Expression of TRPM8 Predicts Poor Prognosis in Patients with Osteosarcoma. Oncol. Lett. 12 (2), 1373–1379. doi: 10.3892/ol.2016.4764
Zhou, K., Zhang, S. S., Yan, Y., Zhao, S. (2014). Overexpression of Transient Receptor Potential Vanilloid 2 Is Associated with Poor Prognosis in Patients with Esophageal Squamous Cell Carcinoma. Med. Oncol. 31 (7), 17. doi: 10.1007/s12032-014-0017-5
Zhuge, Y., Xu, J. (2001). Rac1 Mediates Type I Collagen-Dependent MMP-2 Activation. J. Biol. Chem. 276 (19), 16248–16256. doi: 10.10974/jbc.M01090200
Keywords: transient receptor potential channels, small guanosine triphosphatases, cancer hallmarks, metastasis, molecular signaling, migration, invasion, tumor vascularization
Citation: Chinigò G, Fiorio Pla A and Gkika D (2020) TRP Channels and Small GTPases Interplay in the Main Hallmarks of Metastatic Cancer. Front. Pharmacol. 11:581455. doi: 10.3389/fphar.2020.581455
Received: 08 July 2020; Accepted: 11 September 2020;
Published: 29 September 2020.
Edited by:
Sébastien Roger, Université de Tours, FranceReviewed by:
Isaac Jardin, University of Extremadura, SpainOsama F. Harraz, University of Vermont, United States
Copyright © 2020 Chinigò, Fiorio Pla and Gkika. This is an open-access article distributed under the terms of the Creative Commons Attribution License (CC BY). The use, distribution or reproduction in other forums is permitted, provided the original author(s) and the copyright owner(s) are credited and that the original publication in this journal is cited, in accordance with accepted academic practice. No use, distribution or reproduction is permitted which does not comply with these terms.
*Correspondence: Dimitra Gkika, ZGltaXRyYS5na2lrYUB1bml2LWxpbGxlLmZy