- 1College of Pharmacy, Chengdu University of Traditional Chinese Medicine, Chengdu, China
- 2Department of Pharmacy, PLA General Hospital, Beijing, China
Cholestatic liver disease is caused by the obstruction of bile synthesis, transport, and excretion in or outside the liver by a variety of reasons. Long-term persistent cholestasis in the liver can trigger inflammation, necrosis, or apoptosis of hepatocytes. Bile acid nuclear receptors have received the most attention for the treatment of cholestasis, while the drug development for bile acid nuclear receptors has made considerable progress. However, the targets regulated by bile acid receptor drugs are limited. Thus, as anticipated, intervention in the expression of bile acid nuclear receptors alone will not yield satisfactory clinical results. Therefore, this review comprehensively summarized the literature related to cholestasis, analyzed the molecular mechanism that bile acid damages cells, and status of drug development. It is hoped that this review will provide some reference for the research and development of drugs for cholestasis treatment in the future.
Introduction
Bile acid is an important component of bile, accounting for about 85% of the solid composition of bile, is the main metabolite of cholesterol metabolism, which can participate in the regulation of physiological function, such as cholesterol, sugar, and lipid metabolism (Song et al., 2015). After being synthesized in the liver, bile acid is secreted into the gallbladder through the bile duct, then enters the small intestine to participate in food digestion, and finally re-absorbs into the liver again. Another 5% of bile acids are excreted through feces (Martinot et al., 2017). Bile acids are mainly conjugated bile acids in the hepato-intestinal circulation and cannot pass through the cell membrane, so the metabolic process of bile acids requires the participation of a variety of metabolic enzymes, nuclear receptors and transporters, as well as the bile acids transport membrane system in the hepato-intestinal circulation (Trauner et al., 2017).
Cholestasis is an obstacle in the secretion and excretion of bile acid, which results in bile acid not flowing into the small intestine but flowing into the blood reversely. The toxic bile acid accumulated in the liver and systemic circulation for a long time can cause damage to the bile duct and liver cells, and severe cases can cause liver fibrosis and cirrhosis (Yan et al., 2017). Clinically, it is common in acute and chronic liver diseases such as primary sclerosing cholangitis (PSC), primary biliary cirrhosis (PBC), viral hepatitis, and drug-induced liver injury (de Vries and Beuers, 2017), and the main clinical manifestations are jaundice, pruritus, liver dysfunction, etc. (Wagner and Trauner, 2016).
Currently, numerous studies have investigated the pathogenesis of cholestasis. The mechanism of bile acid homeostasis is currently the hottest research hotspot. The key signaling pathways that regulate bile acid metabolism, such as bile acid synthesis-related targets farnesoid X receptor (FXR) (Farr et al., 2020), cholesterol 7-alpha hydroxy-lase (CYP7A1) (Chambers et al., 2019), short heterodimer partner (SHP) (Keitel et al., 2019), and oxysterol 12α-hydroxylase (CYP8B1) (Zhong et al., 2018), bile acid absorption-related targets Na+/taurocholate cotransporter (NTCP) (Li et al., 2019), bile acid transport targets bile salt export pump (BSEP), multidrug resistance-associated protein 2 (MRP2) (Wang et al., 2019), multidrug resistance-associated protein 3 (MRP3) (Song et al., 2019), and organic anion transporter family member 3A1 (OATP3A1) (Pan et al., 2018), and the bile acid detoxification-related targets pregnane X receptor (PXR) (Fan et al., 2019) and constitutive androstane receptor (CAR) (Shin and Wang, 2019), are the most concerned pathogenesis of cholestasis.
The discovery of the pathogenesis of cholestasis has pushed the research of cholestatic liver disease. Some small-molecule compound ligands are also found for the key targets regulating bile acid metabolism, which provides a clear direction for the discovery of cholestasis drugs and also provide better options for the treatment of patients with cholestasis. FXR agonist obeticholic acid (OCA) can significantly improve the symptoms and liver function of patients with cholestasis who do not respond to ursodeoxycholic acid (UDCA) (Bowlus et al., 2019). This gives us new hope for cholestatic liver diseases. However, some evidence has also found that OCA can also aggravate the symptoms of itching (Mayer et al., 2020). This leads us to rethink whether bile acid receptor drugs are the most promising treatments for patients with cholestatic liver disease. Besides, we also found that several studies have found that some non-bile acid receptor signaling pathways also play an important role in bile acid synthesis. However, the current research on non-nuclear receptor-targeted drugs is rare. So whether non-bile acid receptor ligands can be a new choice of drugs for cholestatic liver disease is also a question worthy of consideration. Therefore, this review focuses on the latest research progress about non-bile acid nuclear receptor mechanisms that regulate bile acid synthesis, hydrophobic bile acid toxicity mechanism, and current main drugs targeting bile acid receptor, hoping to provide some reference for the pathogenesis research and drug discovery research of cholestatic liver disease.
Signals of Hydrophobic Bile Acid-Induced Liver Cell Death
Bile acids are composed of two types of bile acids: hydrophilic bile acids and hydrophobic bile acids. Hydrophobic bile acids, including glycocholic acid (GCA), cholic acid (CA), lithocholic acid (LCA), chenodeoxycholic acid (CDCA), and deoxycholic acid (DCA) are a major factor in inducing liver cell death (Thomas et al., 2008).
“Hydrophobic Bile Acid-Death Ligand” Signals
Some reported evidence suggest that cholestasis-related hepatocyte apoptosis is related to death receptors (Sodeman et al., 2000). Activation of the TNF-related apoptosis-inducing ligand receptor (TRAILR) and Fas death receptor signaling pathway is an important pathway for hepatocyte apoptosis induced by bile acid. Bile acids activate Fas-related death signals in a ligand-dependent and -dependent hepatocyte apoptosis manner. Bile acid stimulates intracellular vesicles associated with the Golgi complex and the trans-Golgi network, and transfers Fas-containing vesicles to hepatocyte membranes, initiating a ligand-dependent death signaling pathway, while increasing Fas density on the surface of hepatocytes to making it more sensitive to Fas agonists. Bile acid-mediated apoptosis of hepatocytes not only activates ligand-independent death receptor oligomerization, but also regulates the sensitivity of death receptor-related signaling pathways. Death receptor-mediated apoptosis of hepatocytes is regulated by different apoptotic signals. On the death-inducing signaling complex (DISC), bile acid stimulates the phosphorylation of cFLIP to reduce the binding of two different isoforms of cFLIP long (cFLIP-L) and cFLIP short (cFLIP-S) to Fas-associated death domain (FADD) in DISC, and then reduce the recruitment of cFLIP to DISC, promoting the activation of caspases 8 and 10 (Higuchi et al., 2003; Higuchi and Gores, 2003). Activated caspases 8 and 10 cleave bid into tBid and enter it mitochondria with Bax to induce mitochondrial dysfunction and promote the release of cytochrome c. The released cytochrome c binds to apoptosis-activating factor-1 (Apaf-1) to promote the activation of Caspase9. And caspase9 further activates Caspase3/6/7, which eventually leads to liver cell death. Besides, bile acids can also directly cause Bax translocation into mitochondria, which can also lead to the release of cytochrome c and the downstream effectors of caspases signaling pathway. Bile acids can also stimulate mitochondrial respiratory chain to stimulate the production of reactive oxygen species (ROS) and cause mitochondrial membrane permeability transition (MPT), and release cytochrome c (Rodrigues et al., 1998; Higuchi et al., 2002) (Figure 1).
“Intestinal Flora-Hydrophobic Bile Acid-Inflammation” Signals
Bile acids are mainly secreted by the liver, and 95% of them are reabsorbed into the ileum (Chavez-Talavera et al., 2019). Intestinal flora can regulate the body’s metabolism (Feng et al., 2019) and produce a large number of metabolites in vivo, which are important signal regulators and energy substrates (Swann et al., 2011). Intestinal flora modify bile acid molecules by debinding water, epimerization, and dehydroxylation. Bile salt hydrolase (BSH) in bacteria is the key enzyme for bile acid degradation in the intestinal tract (Joyce et al., 2014). It was found that Bacteroides, Lactobacillus, and Bifidobacterium can release primary bile acid by BSH (Swann et al., 2011). The primary bile acid is broken down into secondary bile acid (Sun et al., 2019), and esterified to make them more hydrophobic (Ridlon et al., 2016) by some other intestinal flora. Hydrophobic bile acid (such as DCA) in the secondary bile acid is cytotoxic and can cause liver cell damage (Xie et al., 2016) after reabsorption by liver. Moreover, intestinal flora can also modulate the synthesis of bile acids in an FXR dependent manner (Ridlon et al., 2013) and by influencing CYP7A1, Cyp8b1, CYP27A1 (Sayin et al., 2013).
Primary bile acids (DCA and CDCA) has been demonstrated to increase intestinal permeability (Raimondi et al., 2008). The intestinal LPS enters the blood circulation through the intestinal barrier and combines with the Toll-like receptor 4 (TLR4) receptor to further participate in the oxidative stress response and increase the overproduction of intracellular ROS in liver, reducing the activity of various antioxidant enzymes (Singh and Li, 2012; Chassaing et al., 2014). LPS can also induce macrophages to secrete IL-1, TNF-α to promote the release of adhesion molecules, stimulate the inflammatory response, and cause neutrophils to produce excess RO (Weber-Mzell et al., 2006). Hydrophobic bile acid is also a factor that stimulates inflammation, which can activate the production of inflammatory mediators (Allen et al., 2010). For example, CDCA and DCA up-regulate the expression of early growth response gene-1 (Egr-1) by activating the epithelial growth factor receptor (EGFR) to cause the production of vascular endothelial cell adhesion molecule-1 (VCAM-1), IL-1β, and IL-10 in liver cells (Yan et al., 2000). The pro-inflammatory factors produced by hepatocytes further stimulate and activate a variety of inflammatory cells, such as macrophages and neutrophils, to increase the degree of the inflammatory response in liver (Wintermeyer et al., 2009).
“Nuclear Receptor-Apoptotic Protein” Signals
FXR can also regulate the synthesis of bile acids in the process of cholestasis through negative feedback regulation (Sinal et al., 2000). Unlike cFLIP, FXR in the cytoplasm does not directly inhibit the activation of caspase 8, but binds to caspase 8 to prevent the activation and conduction of apoptotic signals in a ligand-independent pathway. This is the fact that FXR agonists do not effectively improve the death receptor-induced hepatocyte death. Therefore, FXR in the liver cytoplasm can inhibit the over activation of caspase 8 by cooperating with cFLIP (Mattisson et al., 2017). However, in the process of liver injury, high levels of TRAIL, TNFα, and FasL in the blood circulation rapidly reduced the expression level of FXR before the activation of apoptosis signal, indicating that the decrease of FXR in the hepatocyte is the primary condition for the activation of apoptosis signal. NAD-dependent protein deacetylase sirtuin-1 (SIRT1) is a member of the silent information regulatory protein family and NAD-dependent deacetylase, which can affect many biological processes, including inflammation, glycolipid metabolism, and so on (Kulkarni et al., 2016). In the pathological state of cholestasis, toxic bile acids such as taurodeoxycholic acid (TDCA), taurocholic acid (TCA), and DCA can reduce the expression level of SIRT1 in hepatocytes (Zhao et al., 2019). SIRT is also the transcription regulator of FXR, and the FXR Lys217 is the main deacetylation binding site of FXR regulated by SIRT1 (Kemper et al., 2009). It can regulate the activity of FXR through deacetylation protein and histone (Purushotham et al., 2012). In addition, in mammals, peroxisome proliferator-activated receptor-γ coactivator 1α (PGC-1α) can be activated by SIRT1 through deacetylation and regulate the activity of FXR (Purushotham et al., 2012) (Figure 1).
“Hydrophobic Bile Acid-Mitochondrial” Signals
Hepatocytes are rich in mitochondria. While producing adenosine triphosphate (ATP), mitochondria are also the main source of ROS, so hepatocytes are also the main target of ROS attack (Czaja, 2007). Under pathological conditions, excessive bile acids interfere with the mitochondrial respiratory complex and the electronic chain transmission process to decouple the oxidative respiratory chain, resulting in the generation of a large number of ROS. The ROS further stimulated mitochondrial permeability transition pore (MPTP) to leads to an irreversible open state, which in turn causes a large amount of high-molecular substances in the cytoplasm to enter the mitochondria, triggers mitochondrial hypertonicity and swells, and causes the mitochondrial membrane damage, ATP hydrolysis, and the release of Smac/DIABLO, cytochrome C, apoptosis-inducing factor (AIF) is released, eventually leading to hepatocyte apoptosis (Yerushalmi et al., 2001). Excess ROS can also increase the synthesis of active oxygen clusters in mitochondria by oxidation of antioxidants in mitochondria, further amplifying oxidative stress response. Simultaneously, excessive ROS can damage the dynamic balance of mitochondrial fusion and division related proteins and induce apoptosis (Wasiak et al., 2007).
On the other hand, excessive ROS can cause mitochondrial membrane depolarization, release cytochrome c to cytoplasm, activate caspase cascade, and induce apoptosis through caspase-3 related signaling pathway (Paradies et al., 2009). After a mitochondrial injury, glutamic oxalacetic transaminase (AST) in mitochondria will be released to the cytoplasm, and into the blood circulation (Perez et al., 2006).
“Hydrophobic Bile Acid-Endoplasmic Reticulum” Signals
Hydrophobic bile acids can release calcium ions into the cytoplasm by inducing endoplasmic reticulum stress (ERS). The increased concentration of Ca2+ causes mitochondria to generate and release a large number of ROS, while the high level of ROS in the cytoplasm causes the increase of Ca2+ concentration. Moreover, hydrophobic bile acids directly stimulate mitochondria to release ROS and excessive ROS in the cytoplasm induces Ca2+ in the endoplasmic reticulum to enter the cytoplasm, and further stimulates mitochondria to produce excess ROS, causing a vicious cycle of oxidative stress in liver cells. All ERS sensors, including IRE1, are activated when there is an imbalance between the ER unfolded protein and chaperone protein (Tabas and Ron, 2011). ERS can activate JNK through IRE1 (Urano et al., 2000). JNK combines with Sab on mitochondria to inhibit mitochondrial respiration and ROS production (Win et al., 2014). C/EBP homologous protein (CHOP) is rarely expressed in the physiological state, but is expressed in large amounts when ERS occurs. ER oxidase 1α (ERO1α) is the direct target of ER-dependent oxidative stress induced by CHOP (Marciniak et al., 2004). ERO1α increases the level of Ca2+ in the cytoplasm by activating the ER calcium release channel IP3R1 (Li et al., 2009).
The role of CaMKII in ERS-induced apoptosis may be a part of the positive feedback amplification loop (Li et al., 2009). ROS in the cytoplasm can lead to the activation of Ca2+-dependent CaMKII (Biswas et al., 1999; Pizzo and Pozzan, 2007; Erickson et al., 2008). More importantly, another important downstream signal of chop CaMKII signaling pathway is the STAT1 signaling pathway (Li et al., 2010). When ERS occurs, another important mechanism of apoptosis induced by CHOP is the inhibition of the survival-promoting protein Bcl-2 (McCullough et al., 2001; Fu et al., 2010). Bcl-2 inhibits mitochondrial permeabilization and apoptosis through BH3 only proteins (including Bax, Bad, and Bim). Some studies have proved that Bim plays an important role in the apoptosis induced by ERS mediated by CHOP, and found that Bim knockout mice have a protective effect on apoptosis, and ERS increased the expression level of Bim (Puthalakath et al., 2007). Another study also found that there was an increase in the expression of Bax, which was dependent on CHOP, in ERS (Santos et al., 2009) (Figure 1).
Main Bile Acid Receptor Drugs
FXR Agonists
Farnesoid X receptor (FXR) is a bile acid nuclear receptor, which is highly expressed in liver and intestinal tissues, and plays an important role in the synthesis, absorption, metabolism, transport, and excretion of bile acids. FXR target genes, including SHP, BSEP, I-BABP, CYP3A4, SULT2A1, UGT2B4, etc. negatively regulate the uptake and synthesis of bile acids, and positively regulate genes responsible for bile acid excretion and detoxification (Fiorucci and Baldelli, 2009). The unconjugated bile acids produced in hepatocytes are mainly detoxified by the liver detoxification enzyme, such as CYP3A4, UGT1A1, and SULT2A1, to form bile acids with high water solubility, which are eliminated by the kidneys (Guengerich, 2001; Fujiwara et al., 2012). The main nuclear receptor of CYP3A4 is FXR. Therefore, FXR can not only regulate the synthesis of bile acids by feedback, but also accelerate the excretion of bile acids by increasing the hydrophilicity of hydrophobic bile acids. The FXR agonist OCA has been used clinically for the treatment of PBC. Studies have shown that it significantly improves the levels of serum ALP, ALT, AST of PBC patients. And, the long-term effect is good, but there are certain side effects (Kowdley et al., 2018). GW4064 is a non-steroidal FXR agonist. Animal experiments show that it can induce SHP in a FXR-selectively dependent manner, thereby reducing the expression of CYP8B1 and CYP7A1, and up-regulating the expression of NTCP (Moscovitz et al., 2016). WAY-362450 is also a highly selective FXR agonist, which can increase the expression of FXR, reduce the expression of CYP8B1 and CYP7A1 proteins, and reduce liver damage (Wu et al., 2014). In an ethanol-induced cholestatic liver injury study, it was found that intestinal FXR agonist Fexaramine can activate FXR, up-regulate the expression of SHP protein, reduce the expression of CYP7A1, and then reduce serum ALT levels in mice (Hartmann et al., 2018). As an FXR agonist, LJN 452 is more selective than GW4060, has better safety and tolerability in healthy volunteers, and is already undergoing phase II clinical trials in PBC patients (Tully et al., 2017). Ly2562175 is also a highly selective FXR agonist. Experiments have found that it has a significant effect on regulating blood lipids. It can reduce TG and LDL levels, and increase HDL levels. However, the indicators for regulating bile acid have not been evaluated (Genin et al., 2015). As a synthetic FXR agonist, GS-9674 mainly activates the expression of FGF19 by activating FXR on intestinal epithelial cells. FGF19 enters the liver to exert an inhibitory effect on bile acid synthesis. GS-9674 is currently in a phase II clinical trial for the treatment of PSC (Khanna and Jones, 2017).
Traditional Chinese medicine (TCM) and its active ingredients also have significant advantages in the treatment of cholestasis. It has been proved that geniposide, an effective component of Gardenia jasminoides, can reduce the synthesis of bile acid by activating FXR, SHP, and OST β and reducing the expression of CYP7A1, Cyp8b1, and CYP27A1 (Wang et al., 2017). Resveratrol, one of the effective components of Polygonum cuspidatum, can regulate the bile acid homeostasis by inducing the expression of FXR and up-regulating the expression of BSEP, NTCP, and MRP2, thus reducing cholestasis (Ding et al., 2018). Applying a mouse model of cholestasis, it was found that the effective ingredient of Alisma orientale B23-acetate (AB23A) can activate bile acid synthesis negative feedback FXR signal, and then promote bile acid efflux and regulate bile acid metabolism (Meng et al., 2015). Corilagin, as one of the active ingredients of Erodium stephaniahum, can significantly improve serum liver function indexes of cholestasis rats, and regulate antioxidant and anti-inflammatory mechanisms (Jin et al., 2013). It can also activate FXR, SHP1, SHP2, UGT2B4, BSEP, MRP2, and SULT2A1 expression, down-regulate CYP7A1 and NTCP protein expression to improve cholestasis (Yang et al., 2018). Studies have shown that auraptene, the active ingredient in Citrus reticulata, reduces bile acid synthesis by activating the expression of FXR to reduce CYP7A1 and CYP8B1, and increases bile acid transporters (such as BSEP and MRP2) to increase bile acid transport (Gao et al., 2017). Emodin in Rheum palmatum can up-regulate the gene and protein expression of BSEP, FXR1, and FXR2 by activating the FXR/BSEP signaling pathway to reduce liver injury (Xiong et al., 2019). In addition, gentiopicrin, the main active ingredient in Gentiana macrophylla, can improve cholestatic liver injury by up-regulating the expression of FXR and MRP4 and reducing the expression of CYP7A1 (Han et al., 2018) (Figure 2).
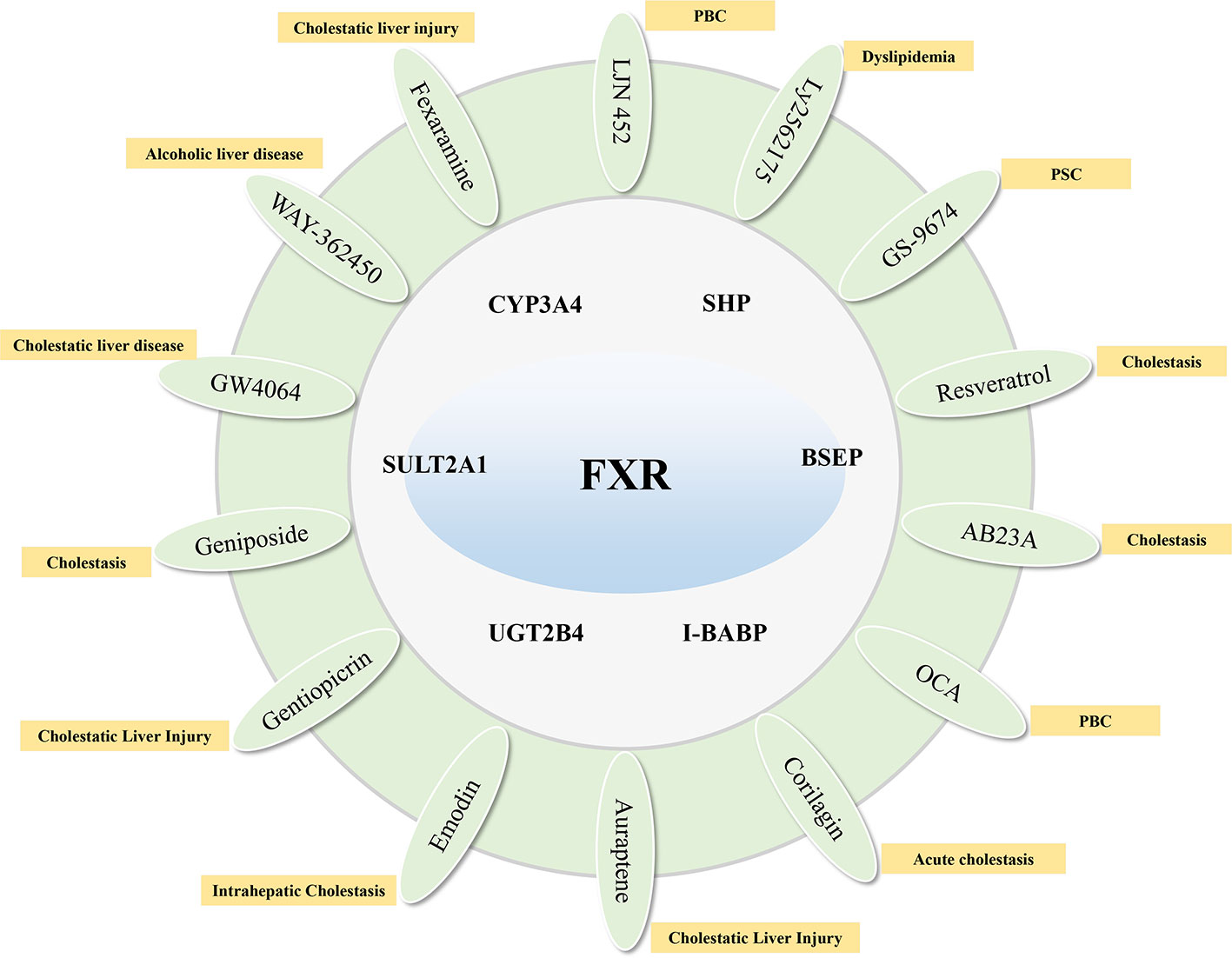
Figure 2 Compounds that target FXR to activate their target genes and related cholestatic liver diseases.
PXR Agonists
PXR is mainly responsible for regulating detoxification-related metabolic enzymes in the liver and small intestine, promoting the degradation of bile acids, and reducing the synthesis of bile acids (Kliewer and Willson, 2002; Jurica et al., 2016). The target genes for PXR include Cyp3a11 (Yamasaki et al., 2018), CYP7A1, CYP8B1, Cpy2b10, Oatp1a4, Oatp4 (Staudinger et al., 2001; Pavek, 2016), CYP3A4, MDR1 (Geick et al., 2001), MRP2 (Kast et al., 2002), MRP3 (Teng et al., 2003), SULT2A1 (Fang et al., 2007), UGT1A1/3/4 (Gardner-Stephen et al., 2004; van Dijk et al., 2015). In addition to inducing the bile acid efflux system, PXR can also activate the bile acid and bilirubin detoxification system (Jung et al., 2006; Fiorucci and Baldelli, 2009). Studies have shown that the activation of PXR can significantly reduce the bile acid synthesis by inhibiting the expression of CYP7A1, and the activation of PXR by FXR further enhances this effect (Staudinger et al., 2001).
Rifampin, an activator of PXR, promotes bilirubin excretion by inducing expression of SULT2A1, UGT1A1, and MRP2, and accelerates bile acid metabolism by increasing expression of CYP3A4 (Marschall et al., 2005; Li et al., 2013). Moreover, it can improve pruritus symptoms of patients with cholestasis (European Association for the Study of the 2009) and liver function biochemical indicators in PBC patients (Bachs et al., 1989). Rifampin is safe for up to 2 weeks (Khurana and Singh, 2006). However, atorvastatin as a PXR agonist cannot improve cholestasis in patients with PBC (Stojakovic et al., 2007).
There are also some ingredients in herbs that can reduce cholestasis by activating the expression of PXR. Schisandrin B in Schisandra chinensis can reduce the bile acid levels in lithocholic acid-induced mice by activating PXR to induce the expression of Ugt1a1, Cyp3a11, and Oatp2 (Zeng et al., 2017). Tanshinone IIA is also a PXR agonist with significant liver protection. It is capable of preventing ANIT-induced cholestatic liver injury by improving the expression of CYP3A4, and simultaneously up-regulating the expression of Cyp3a11, Cyp3a13, and Mdr1 (Zhang X. et al., 2015). Schisandrins A, Schisandrins B, and Schisandrol B from Schisandra chinensis have also been reported to activate PXR expression in primary hepatocytes. Among them, Schisandrins A is also the main active ingredient in Wuzhi Tablets for the treatment of intrahepatic cholestasis (Mu et al., 2006; Zeng et al., 2016). Interestingly, due to the similarities in structural and functional between CAR and PXR, some active ingredients in herbs can activate both the targets simultaneously. Studies have reported that the praeruptorin A and C in Peucedanum praeruptorum can simultaneously activate the expression of PXR and CAR and the expression of CYP3A4 (Huang et al., 2013; Zhou et al., 2013) (Figure 3).
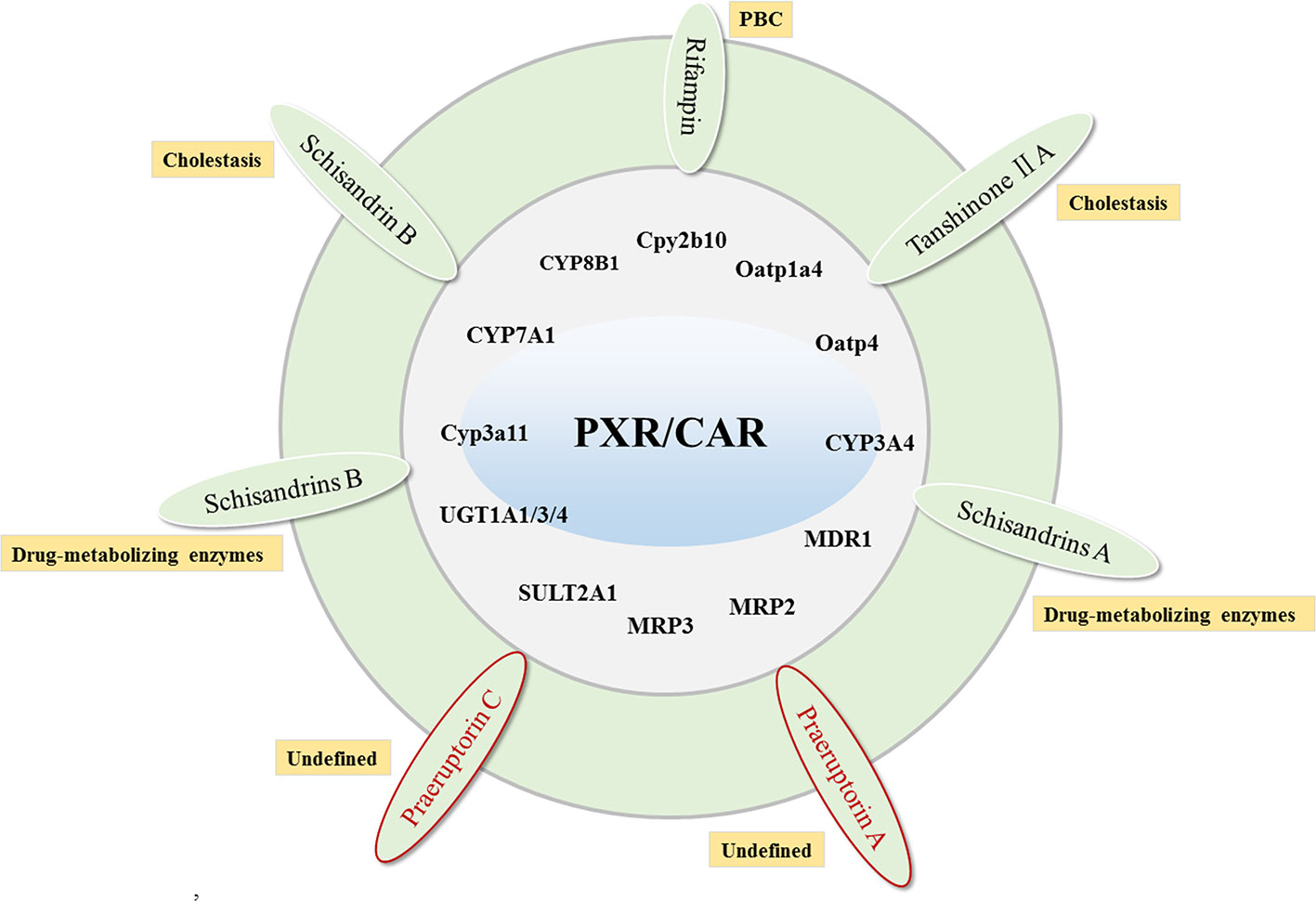
Figure 3 The compounds that target PXR/CAR to activate their target genes and related cholestatic liver diseases. Red labeled drugs are compounds that activate both PXR and CAR.
CAR Agonists
CAR is one of the members of the orphan nuclear receptor superfamily. Its function and structure are very similar to PXR and are mainly expressed in liver and intestine. Studies have demonstrated that the activation of CAR can regulate UGT1A1, organic anion transporter SLC21A6, and MRP2 to accelerate the metabolism of bilirubin (Huang et al., 2003). More importantly, The CAR knockout mice cannot induce UGT1A1 expression, and also make mice more sensitive to toxic bile acids (Barbier et al., 2003). CAR shares common target genes with PXR and FXR, indicating that in addition to FXR agonists, CAR agonists may also be a potential treatment for cholestasis. CAR can activate CYP2B, CYP3A4, Sult2a1, UGT1A, MRP2 Mrp3, and MRP4 to induce bile acid excretion (Fiorucci and Baldelli, 2009) and resist hydrophobic bile acid-induced liver toxicity. At present, CAR agonist research is relatively rare. In addition to the praeruptorin A and C mentioned above, which can improve cholestasis by activating CAR, studies have found some CAR natural product agonists, such as berberine (Zhang et al., 2016), Arecoline (Ling et al., 2014), artemisinin (Burk et al., 2005), and 6,7-dimethylesculetin(Huang et al., 2004), etc. (Figure 3), but these CAR agonists have not been proven effective for cholestatic liver disease.
FXR-PXR/CAR and FXR-Non-Bile Acid Nuclear Receptor Targets Coactivator
From the above, FXR, as a key target for regulating bile acids, has consistently been a promising target for the treatment of cholestatic liver disease. However, OCA targeting FXR has not shown satisfactory results in the clinical treatment of cholestatic liver disease. This leads us to think differently about drug development strategies that only intervention in bile acids and FXRs. It is well known that PXR and CAR are similar in structure and function, which can regulate the transcription and translation of bile acid metabolism enzymes and transporters related to bilirubin clearance, and play a detoxifying role in the liver (Kakizaki et al., 2011). Therefore, we speculate that drugs that can simultaneously regulate FXR and PXR/CAR may be more promising for the treatment of cholestatic liver disease. In addition, non-bile acid receptor also plays a key role in bile acid synthesis and bile acid-induced liver cell damage. Therefore, we also propose that drugs that can simultaneously regulate FXR and non-bile acid receptors are also important directions for drug development in the treatment of cholestatic liver disease. At present, some related compounds have been found. For example, Geniposide can simultaneously regulate the expression of FXR, PXR, NF-κB, Bax, and Bcl-2, and has a great effect on various liver diseases such as cholestasis and liver inflammation (Rong et al., 2017; Wang et al., 2017; Hu et al., 2018). Swertiamarin has been found to have a significant effect on improving cholestasis. It can simultaneously regulate FXR, PXR, bile acid transporters Mrp3, Mdr1, and Mrp4 and detoxification enzymes (Cyp3a, Ugt2b, Sult2a1, and Gsta1), increase the water solubility of hydrophobic bile acids, remove the combined bile acids (Feng et al., 2015; Zhang L. et al., 2015). Formononetin can improve cholestasis through Sirt1-FXR signal pathway and alleviate liver inflammation through JNK inflammatory signal pathway (Yang et al., 2019). In addition, formononetin can decrease acetaminophen induced hepatotoxicity by increasing Nrf2 activity (Jin et al., 2017). Resveratrol has a therapeutic effect on ANIT induced cholestasis by regulating the FXR pathway, and it also has a very good improvement on non-FXR target genes, such as liver inflammatory factors TNFα, IL-6, and IL-1β, as well as oxidation factor COX-2 (Ding et al., 2018). In addition, resveratrol can also regulate the PI3K-Akt signal pathway (Shu et al., 2020). It can be concluded that there are few researches on this kind of coactivators, and the only existing researches are still in the stage of laboratory research, which also puts forward a longer-term requirement for future drug development of cholestatic liver disease.
Conclusions
The pathogenesis of cholestasis has been studied relatively clearly, which has provided strong support for the drug development of cholestatic liver disease. However, at present, very few drugs are used clinically for the treatment of cholestatic liver disease. After decades of effort, UDCA has been clinically preferred for the treatment of cholestatic liver disease (Wunsch et al., 2014; Ma et al., 2016), but some patients do not respond. With an in-depth understanding of the pathogenesis of cholestasis, nuclear receptors have been discovered to play a key role in bile acid metabolism. And bile acid receptor agonists are recognized as the most promising drugs for the treatment of cholestatic liver diseases, such as FXR agonist OCA, which can significantly improve patients who do not respond to UDCA. This gives us a temporary glimmer of hope. However, the side effects aggravating the pruritus symptoms in patients with PBC have led us to rethink the development of therapeutic drugs for cholestatic liver disease. It is well known that, while regulating the bile acid synthesis with FXR as the key target, PXR and CAR regulate the bile acid metabolism enzymes and transporters related to bilirubin clearance, and play a detoxifying role in the liver. In addition, non-bile acid receptor targets also play a key role in bile acid synthesis and bile acid-induced liver cell damage. Therefore, we speculate that drugs that can simultaneously regulate FXR and PXR/CAR or FXR and non-bile acid receptor targets may be more promising for the treatment of cholestatic liver disease. It is worth mentioning that due to the strong hepatotoxicity of hydrophobic bile acids, the research and development of drugs that directly target the activation of hepatocyte detoxification enzymes (CYP3A4, UGT1A1, and SULT2A1) to accelerate the metabolism of hydrophobic bile acids to hydrophilic bile acids is also a very promising strategy for the development of drugs for the treatment of cholestasis. However, there are few studies on this research strategy, and the existing researches are still in the laboratory research stage, which puts forward longer-term requirements for the future drug development of cholestatic liver disease.
Author Contributions
YZ designed the research framework and direction. SW wrote the paper. XM helped to organize the literature or provide writing ideas.
Funding
This work is financially supported by grants from the National Natural Science Foundation of China (81874365).
Conflict of Interest
The authors declare that the research was conducted in the absence of any commercial or financial relationships that could be construed as a potential conflict of interest.
References
Allen, K., Kim, N. D., Moon, J. O., Copple, B. L. (2010). Upregulation of early growth response factor-1 by bile acids requires mitogen-activated protein kinase signaling. Toxicol. Appl. Pharmacol. 243, 63–67. doi: 10.1016/j.taap.2009.11.013
Bachs, L., Pares, A., Elena, M., Piera, C., Rodes, J. (1989). Comparison of rifampicin with phenobarbitone for treatment of pruritus in biliary cirrhosis. Lancet 1, 574–576. doi: 10.1016/S0140-6736(89)91608-5
Barbier, O., Villeneuve, L., Bocher, V., Fontaine, C., Torra, I. P., Duhem, C., et al. (2003). The UDP-glucuronosyltransferase 1A9 enzyme is a peroxisome proliferator-activated receptor alpha and gamma target gene. J. Biol. Chem. 278, 13975–13983. doi: 10.1074/jbc.M300749200
Biswas, G., Adebanjo, O. A., Freedman, B. D., Anandatheerthavarada, H. K., Vijayasarathy, C., Zaidi, M., et al. (1999). Retrograde Ca2+ signaling in C2C12 skeletal myocytes in response to mitochondrial genetic and metabolic stress: a novel mode of inter-organelle crosstalk. EMBO J. 18, 522–533. doi: 10.1093/emboj/18.3.522
Bowlus, C. L., Pockros, P. J., Kremer, A. E., Pares, A., Forman, L. M., Drenth, J. P. H., et al. (2019). Long-Term Obeticholic Acid Therapy Improves Histological Endpoints in Patients With Primary Biliary Cholangitis. Clin. Gastroenterol. Hepatol. 18 (5). doi: 10.1016/j.cgh.2019.09.050
Burk, O., Arnold, K. A., Nussler, A. K., Schaeffeler, E., Efimova, E., Avery, B. A., et al. (2005). Antimalarial artemisinin drugs induce cytochrome P450 and MDR1 expression by activation of xenosensors pregnane X receptor and constitutive androstane receptor. Mol. Pharmacol. 67, 1954–1965. doi: 10.1124/mol.104.009019
Chambers, K. F., Day, P. E., Aboufarrag, H. T., Kroon, P. A. (2019). Polyphenol Effects on Cholesterol Metabolism via Bile Acid Biosynthesis, CYP7A1: A Review. Nutrients 11 (11). doi: 10.3390/nu11112588
Chassaing, B., Etienne-Mesmin, L., Gewirtz, A. T. (2014). Microbiota-liver axis in hepatic disease. Hepatology 59, 328–339. doi: 10.1002/hep.26494
Chavez-Talavera, O., Haas, J., Grzych, G., Tailleux, A., Staels, B. (2019). Bile acid alterations in nonalcoholic fatty liver disease, obesity, insulin resistance and type 2 diabetes: what do the human studies tell? Curr. Opin. Lipidol. 30, 244–254. doi: 10.1097/MOL.0000000000000597
Czaja, M. J. (2007). Cell signaling in oxidative stress-induced liver injury. Semin. Liver Dis. 27, 378–389. doi: 10.1055/s-2007-991514
de Vries, E., Beuers, U. (2017). Management of cholestatic disease in 2017. Liver Int. 37 (Suppl 1), 123–129. doi: 10.1111/liv.13306
Ding, L., Zhang, B., Li, J., Yang, L., Wang, Z. (2018). Beneficial effect of resveratrol on alphanaphthyl isothiocyanateinduced cholestasis via regulation of the FXR pathway. Mol. Med. Rep. 17, 1863–1872. doi: 10.3892/mmr.2017.8051
Erickson, J. R., Joiner, M. L., Guan, X., Kutschke, W., Yang, J., Oddis, C. V., et al. (2008). A dynamic pathway for calcium-independent activation of CaMKII by methionine oxidation. Cell 133, 462–474. doi: 10.1016/j.cell.2008.02.048
European Association for the Study of the, L (2009). EASL Clinical Practice Guidelines: management of cholestatic liver diseases. J. Hepatol. 51, 237–267. doi: 10.1016/j.jhep.2009.04.009
Fan, S., Liu, C., Jiang, Y., Gao, Y., Chen, Y., Fu, K., et al. (2019). Lignans from Schisandra sphenanthera protect against lithocholic acid-induced cholestasis by pregnane X receptor activation in mice. J. Ethnopharmacol. 245, 112103. doi: 10.1016/j.jep.2019.112103
Fang, H. L., Strom, S. C., Ellis, E., Duanmu, Z., Fu, J., Duniec-Dmuchowski, Z., et al. (2007). Positive and negative regulation of human hepatic hydroxysteroid sulfotransferase (SULT2A1) gene transcription by rifampicin: roles of hepatocyte nuclear factor 4alpha and pregnane X receptor. J. Pharmacol. Exp. Ther. 323, 586–598. doi: 10.1124/jpet.107.124610
Farr, S., Stankovic, B., Hoffman, S., Masoudpoor, H., Baker, C., Taher, J., et al. (2020). Bile acid treatment and FXR agonism lower postprandial lipemia in mice. Am. J. Physiol. Gastrointest. Liver Physiol. 318 (4). doi: 10.1152/ajpgi.00386.2018
Feng, X. C., Du, X., Chen, S., Yue, D., Cheng, Y., Zhang, L., et al. (2015). Swertianlarin, isolated from Swertia mussotii Franch, increases detoxification enzymes and efflux transporters expression in rats. Int. J. Clin. Exp. Pathol. 8, 184–195.
Feng, W., Ao, H., Peng, C., Yan, D. (2019). Gut microbiota, a new frontier to understand traditional Chinese medicines. Pharmacol. Res. 142, 176–191. doi: 10.1016/j.phrs.2019.02.024
Fiorucci, S., Baldelli, F. (2009). Farnesoid X receptor agonists in biliary tract disease. Curr. Opin. Gastroenterol. 25, 252–259. doi: 10.1097/MOG.0b013e328324f87e
Fu, H. Y., Okada, K., Liao, Y., Tsukamoto, O., Isomura, T., Asai, M., et al. (2010). Ablation of C/EBP homologous protein attenuates endoplasmic reticulum-mediated apoptosis and cardiac dysfunction induced by pressure overload. Circulation 122, 361–369. doi: 10.1161/CIRCULATIONAHA.109.917914
Fujiwara, R., Chen, S., Karin, M., Tukey, R. H. (2012). Reduced expression of UGT1A1 in intestines of humanized UGT1 mice via inactivation of NF-kappaB leads to hyperbilirubinemia. Gastroenterology 142, 109–118 e2. doi: 10.1053/j.gastro.2011.09.045
Gao, X., Fu, T., Wang, C., Ning, C., Kong, Y., Liu, Z., et al. (2017). Computational discovery and experimental verification of farnesoid X receptor agonist auraptene to protect against cholestatic liver injury. Biochem. Pharmacol. 146, 127–138. doi: 10.1016/j.bcp.2017.09.016
Gardner-Stephen, D., Heydel, J. M., Goyal, A., Lu, Y., Xie, W., Lindblom, T., et al. (2004). Human PXR variants and their differential effects on the regulation of human UDP-glucuronosyltransferase gene expression. Drug Metab. Dispos. 32, 340–347. doi: 10.1124/dmd.32.3.340
Geick, A., Eichelbaum, M., Burk, O. (2001). Nuclear receptor response elements mediate induction of intestinal MDR1 by rifampin. J. Biol. Chem. 276, 14581–14587. doi: 10.1074/jbc.M010173200
Genin, M. J., Bueno, A. B., Agejas Francisco, J., Manninen, P. R., Bocchinfuso, W. P., Montrose-Rafizadeh, C., et al. (2015). Discovery of 6-(4-{[5-Cyclopropyl-3-(2,6-dichlorophenyl)isoxazol-4-yl]methoxy}piperidin-1-yl)- 1-methyl-1H-indole-3-carboxylic Acid: A Novel FXR Agonist for the Treatment of Dyslipidemia. J. Med. Chem. 58, 9768–9772. doi: 10.1021/acs.jmedchem.5b01161
Guengerich, F. P. (2001). Common and uncommon cytochrome P450 reactions related to metabolism and chemical toxicity. Chem. Res. Toxicol. 14, 611–650. doi: 10.1021/tx0002583
Han, H., Xu, L., Xiong, K., Zhang, T., Wang, Z. (2018). Exploration of Hepatoprotective Effect of Gentiopicroside on Alpha-Naphthylisothiocyanate-Induced Cholestatic Liver Injury in Rats by Comprehensive Proteomic and Metabolomic Signatures. Cell Physiol. Biochem. 49, 1304–1319. doi: 10.1159/000493409
Hartmann, P., Hochrath, K., Horvath, A., Chen, P., Seebauer, C. T., Llorente, C., et al. (2018). Modulation of the intestinal bile acid/farnesoid X receptor/fibroblast growth factor 15 axis improves alcoholic liver disease in mice. Hepatology 67, 2150–2166. doi: 10.1002/hep.29676
Higuchi, H., Gores, G. J. (2003). Bile acid regulation of hepatic physiology: IV. Bile acids and death receptors. Am. J. Physiol. Gastrointest. Liver Physiol. 284, G734–G738.
Higuchi, H., Bronk, S. F., Taniai, M., Canbay, A., Gores, G. J. (2002). Cholestasis increases tumor necrosis factor-related apoptotis-inducing ligand (TRAIL)-R2/DR5 expression and sensitizes the liver to TRAIL-mediated cytotoxicity. J. Pharmacol. Exp. Ther. 303, 461–467. doi: 10.1124/jpet.102.040030
Higuchi, H., Yoon, J. H., Grambihler, A., Werneburg, N., Bronk, S. F., Gores, G. J. (2003). Bile acids stimulate cFLIP phosphorylation enhancing TRAIL-mediated apoptosis. J. Biol. Chem. 278, 454–461. doi: 10.1074/jbc.M209387200
Hu, X., Yu, D., Zhuang, L., Zhou, M., Shi, Z., Jin, G., et al. (2018). Geniposide improves hepatic inflammation in diabetic db/db mice. Int. Immunopharmacol. 59, 141–147. doi: 10.1016/j.intimp.2018.03.035
Huang, W., Zhang, J., Chua, S. S., Qatanani, M., Han, Y., Granata, R., et al. (2003). Induction of bilirubin clearance by the constitutive androstane receptor (CAR). Proc. Natl. Acad. Sci. U. S. A. 100, 4156–4161. doi: 10.1073/pnas.0630614100
Huang, W., Zhang, J., Moore, D. D. (2004). A traditional herbal medicine enhances bilirubin clearance by activating the nuclear receptor CAR. J. Clin. Invest. 113, 137–143. doi: 10.1172/JCI200418385
Huang, L., Bi, H. C., Li, Y. H., Zhang, J. Q., Kuang, S. Y., Zhang, L., et al. (2013). Regulation of human pregnane X receptor and its target gene cytochrome P450 3A by praeruptorin A isolated from the herbal medicine Peucedanum praeruptorum. Planta Med. 79, 1509–1515. doi: 10.1055/s-0033-1350795
Jin, F., Cheng, D., Tao, J. Y., Zhang, S. L., Pang, R., Guo, Y. J., et al. (2013). Anti-inflammatory and anti-oxidative effects of corilagin in a rat model of acute cholestasis. BMC Gastroenterol. 13, 79. doi: 10.1186/1471-230X-13-79
Jin, F., Wan, C., Li, W., Yao, L., Zhao, H., Zou, Y., et al. (2017). Formononetin protects against acetaminophen-induced hepatotoxicity through enhanced NRF2 activity. PLoS One 12, e0170900. doi: 10.1371/journal.pone.0170900
Joyce, S. A., MacSharry, J., Casey, P. G., Kinsella, M., Murphy, E. F., Shanahan, F., et al. (2014). Regulation of host weight gain and lipid metabolism by bacterial bile acid modification in the gut. Proc. Natl. Acad. Sci. U. S. A. 111, 7421–7426. doi: 10.1073/pnas.1323599111
Jung, D., Mangelsdorf, D. J., Meyer, U. A. (2006). Pregnane X receptor is a target of farnesoid X receptor. J. Biol. Chem. 281, 19081–19091. doi: 10.1074/jbc.M600116200
Jurica, J., Dovrtelova, G., Noskova, K., Zendulka, O. (2016). Bile acids, nuclear receptors and cytochrome P450. Physiol. Res. 65, S427–S440. doi: 10.33549/physiolres.933512
Kakizaki, S., Takizawa, D., Tojima, H., Horiguchi, N., Yamazaki, Y., Mori, M. (2011). Nuclear receptors CAR and PXR; therapeutic targets for cholestatic liver disease. Front. Biosci (Landmark Ed). 16, 2988–3005. doi: 10.2741/3893
Kast, H. R., Goodwin, B., Tarr, P. T., Jones, S. A., Anisfeld, A. M., Stoltz, C. M., et al. (2002). Regulation of multidrug resistance-associated protein 2 (ABCC2) by the nuclear receptors pregnane X receptor, farnesoid X-activated receptor, and constitutive androstane receptor. J. Biol. Chem. 277, 2908–2915. doi: 10.1074/jbc.M109326200
Keitel, V., Droge, C., Haussinger, D. (2019). Targeting FXR in Cholestasis. Handb. Exp. Pharmacol. 256, 299–324. doi: 10.1007/164_2019_231
Kemper, J. K., Xiao, Z., Ponugoti, B., Miao, J., Fang, S., Kanamaluru, D., et al. (2009). FXR acetylation is normally dynamically regulated by p300 and SIRT1 but constitutively elevated in metabolic disease states. Cell Metab. 10, 392–404. doi: 10.1016/j.cmet.2009.09.009
Khanna, A., Jones, D. E. (2017). Novel strategies and therapeutic options for the management of primary biliary cholangitis. Therap. Adv. Gastroenterol. 10, 791–803. doi: 10.1177/1756283X17728669
Khurana, S., Singh, P. (2006). Rifampin is safe for treatment of pruritus due to chronic cholestasis: a meta-analysis of prospective randomized-controlled trials. Liver Int. 26, 943–948. doi: 10.1111/j.1478-3231.2006.01326.x
Kliewer, S. A., Willson, T. M. (2002). Regulation of xenobiotic and bile acid metabolism by the nuclear pregnane X receptor. J. Lipid Res. 43, 359–364.
Kowdley, K. V., Luketic, V., Chapman, R., Hirschfield, G. M., Poupon, R., Schramm, C., et al. (2018). A randomized trial of obeticholic acid monotherapy in patients with primary biliary cholangitis. Hepatology 67, 1890–1902. doi: 10.1002/hep.29569
Kulkarni, S. R., Soroka, C. J., Hagey, L. R., Boyer, J. L. (2016). Sirtuin 1 activation alleviates cholestatic liver injury in a cholic acid-fed mouse model of cholestasis. Hepatology 64, 2151–2164. doi: 10.1002/hep.28826
Li, G., Mongillo, M., Chin, K. T., Harding, H., Ron, D., Marks, A. R., et al. (2009). Role of ERO1-alpha-mediated stimulation of inositol 1,4,5-triphosphate receptor activity in endoplasmic reticulum stress-induced apoptosis. J. Cell Biol. 186, 783–792. doi: 10.1083/jcb.200904060
Li, G., Scull, C., Ozcan, L., Tabas, I. (2010). NADPH oxidase links endoplasmic reticulum stress, oxidative stress, and PKR activation to induce apoptosis. J. Cell Biol. 191, 1113–1125. doi: 10.1083/jcb.201006121
Li, F., Lu, J., Cheng, J., Wang, L., Matsubara, T., Csanaky, I. L., et al. (2013). Human PXR modulates hepatotoxicity associated with rifampicin and isoniazid co-therapy. Nat. Med. 19, 418–420. doi: 10.1038/nm.3104
Li, T., Xu, L., Zheng, R., Wang, X., Li, L., Ji, H., et al. (2019). Picroside II protects against cholestatic liver injury possibly through activation of farnesoid X receptor. Phytomedicine 68, 153153. doi: 10.1016/j.phymed.2019.153153
Ling, H. Y., Yao, Q. X., Qi, Z. Q., Yang, S. S., He, J. Q., Zhang, K. F., et al. (2014). The role of arecoline on hepatic insulin resistance in type 2 diabetes rats. Zhongguo Ying Yong Sheng Li Xue Za Zhi. 30, 208–212.
Ma, H., Zeng, M., Han, Y., Yan, H., Tang, H., Sheng, J., et al. (2016). A multicenter, randomized, double-blind trial comparing the efficacy and safety of TUDCA and UDCA in Chinese patients with primary biliary cholangitis. Med. (Baltimore). 95, e5391. doi: 10.1097/MD.0000000000005391
Marciniak, S. J., Yun, C. Y., Oyadomari, S., Novoa, I., Zhang, Y., Jungreis, R., et al. (2004). CHOP induces death by promoting protein synthesis and oxidation in the stressed endoplasmic reticulum. Genes Dev. 18, 3066–3077. doi: 10.1101/gad.1250704
Marschall, H. U., Wagner, M., Zollner, G., Fickert, P., Diczfalusy, U., Gumhold, J., et al. (2005). Complementary stimulation of hepatobiliary transport and detoxification systems by rifampicin and ursodeoxycholic acid in humans. Gastroenterology 129, 476–485. doi: 10.1016/j.gastro.2005.05.009
Martinot, E., Sedes, L., Baptissart, M., Lobaccaro, J. M., Caira, F., Beaudoin, C., et al. (2017). Bile acids and their receptors. Mol. Asp. Med. 56, 2–9. doi: 10.1016/j.mam.2017.01.006
Mattisson, I. Y., Bjorkbacka, H., Wigren, M., Edsfeldt, A., Melander, O., Fredrikson, G. N., et al. (2017). Elevated Markers of Death Receptor-Activated Apoptosis are Associated with Increased Risk for Development of Diabetes and Cardiovascular Disease. EBioMedicine 26, 187–197. doi: 10.1016/j.ebiom.2017.11.023
Mayer, L. S., Kaser, R., Bottler, T. (2020). [Autoimmune mediated cholestatic liver diseases]. Dtsch Med. Wochenschr. 145, 296–305. doi: 10.1055/a-0944-8805
McCullough, K. D., Martindale, J. L., Klotz, L. O., Aw, T. Y., Holbrook, N. J. (2001). Gadd153 sensitizes cells to endoplasmic reticulum stress by down-regulating Bcl2 and perturbing the cellular redox state. Mol. Cell Biol. 21, 1249–1259. doi: 10.1128/MCB.21.4.1249-1259.2001
Meng, Q., Chen, X. L., Wang, C. Y., Liu, Q., Sun, H. J., Sun, P. Y., et al. (2015). Alisol B 23-acetate protects against ANIT-induced hepatotoxity and cholestasis, due to FXR-mediated regulation of transporters and enzymes involved in bile acid homeostasis. Toxicol. Appl. Pharmacol. 283, 178–186. doi: 10.1016/j.taap.2015.01.020
Moscovitz, J. E., Kong, B., Buckley, K., Buckley, B., Guo, G. L., Aleksunes, L. M. (2016). Restoration of enterohepatic bile acid pathways in pregnant mice following short term activation of Fxr by GW4064. Toxicol. Appl. Pharmacol. 310, 60–67. doi: 10.1016/j.taap.2016.08.021
Mu, Y., Zhang, J., Zhang, S., Zhou, H. H., Toma, D., Ren, S., et al. (2006). Traditional Chinese medicines Wu Wei Zi (Schisandra chinensis Baill) and Gan Cao (Glycyrrhiza uralensis Fisch) activate pregnane X receptor and increase warfarin clearance in rats. J. Pharmacol. Exp. Ther. 316, 1369–1377. doi: 10.1124/jpet.105.094342
Pan, Q., Zhang, X., Zhang, L., Cheng, Y., Zhao, N., Li, F., et al. (2018). Solute Carrier Organic Anion Transporter Family Member 3A1 Is a Bile Acid Efflux Transporter in Cholestasis. Gastroenterology 155, 1578–1592 e16. doi: 10.1053/j.gastro.2018.07.031
Paradies, G., Petrosillo, G., Paradies, V., Ruggiero, F. M. (2009). Role of cardiolipin peroxidation and Ca2+ in mitochondrial dysfunction and disease. Cell Calcium. 45, 643–650. doi: 10.1016/j.ceca.2009.03.012
Pavek, P. (2016). Pregnane X Receptor (PXR)-Mediated Gene Repression and Cross-Talk of PXR with Other Nuclear Receptors via Coactivator Interactions. Front. Pharmacol. 7, 456. doi: 10.3389/fphar.2016.00456
Perez, M. J., Macias, R., II, Marin, J. J. (2006). Maternal cholestasis induces placental oxidative stress and apoptosis. Protective effect of ursodeoxycholic acid. Placenta 27, 34–41. doi: 10.1016/j.placenta.2004.10.020
Pizzo, P., Pozzan, T. (2007). Mitochondria-endoplasmic reticulum choreography: structure and signaling dynamics. Trends Cell Biol. 17, 511–517. doi: 10.1016/j.tcb.2007.07.011
Purushotham, A., Xu, Q., Lu, J., Foley, J. F., Yan, X., Kim, D. H., et al. (2012). Hepatic deletion of SIRT1 decreases hepatocyte nuclear factor 1alpha/farnesoid X receptor signaling and induces formation of cholesterol gallstones in mice. Mol. Cell Biol. 32, 1226–1236. doi: 10.1128/MCB.05988-11
Puthalakath, H., O’Reilly, L. A., Gunn, P., Lee, L., Kelly, P. N., Huntington, N. D., et al. (2007). ER stress triggers apoptosis by activating BH3-only protein Bim. Cell 129, 1337–1349. doi: 10.1016/j.cell.2007.04.027
Raimondi, F., Santoro, P., Barone, M. V., Pappacoda, S., Barretta, M. L., Nanayakkara, M., et al. (2008). Bile acids modulate tight junction structure and barrier function of Caco-2 monolayers via EGFR activation. Am. J. Physiol. Gastrointest. Liver Physiol. 294, G906–G913. doi: 10.1152/ajpgi.00043.2007
Ridlon, J. M., Alves, J. M., Hylemon, P. B., Bajaj, J. S. (2013). Cirrhosis, bile acids and gut microbiota: unraveling a complex relationship. Gut Microbes. 4, 382–387. doi: 10.4161/gmic.25723
Ridlon, J. M., Harris, S. C., Bhowmik, S., Kang, D. J., Hylemon, P. B. (2016). Consequences of bile salt biotransformations by intestinal bacteria. Gut Microbes. 7, 22–39. doi: 10.1080/19490976.2015.1127483
Rodrigues, C. M., Fan, G., Wong, P. Y., Kren, B. T., Steer, C. J. (1998). Ursodeoxycholic acid may inhibit deoxycholic acid-induced apoptosis by modulating mitochondrial transmembrane potential and reactive oxygen species production. Mol. Med. 4, 165–178. doi: 10.1007/BF03401914
Rong, Y. P., Huang, H. T., Liu, J. S., Wei, L. (2017). Protective Effects of Geniposide on Hepatic Ischemia/Reperfusion Injury. Transplant. Proc. 49, 1455–1460. doi: 10.1016/j.transproceed.2017.02.063
Santos, C. X., Tanaka, L. Y., Wosniak, J., Laurindo, F. R. (2009). Mechanisms and implications of reactive oxygen species generation during the unfolded protein response: roles of endoplasmic reticulum oxidoreductases, mitochondrial electron transport, and NADPH oxidase. Antioxid. Redox Signal. 11, 2409–2427. doi: 10.1089/ars.2009.2625
Sayin, S., II, Wahlstrom, A., Felin, J., Jantti, S., Marschall, H. U., Bamberg, K., et al. (2013). Gut microbiota regulates bile acid metabolism by reducing the levels of tauro-beta-muricholic acid, a naturally occurring FXR antagonist. Cell Metab. 17, 225–235. doi: 10.1016/j.cmet.2013.01.003
Shin, D. J., Wang, L. (2019). Bile Acid-Activated Receptors: A Review on FXR and Other Nuclear Receptors. Handb. Exp. Pharmacol. 256, 51–72. doi: 10.1007/164_2019_236
Shu, L., Zhao, H., Huang, W., Hou, G., Song, G., Ma, H. (2020). Resveratrol Upregulates mmu-miR-363-3p via the PI3K-Akt Pathway to Improve Insulin Resistance Induced by a High-Fat Diet in Mice. Diabetes Metab. Syndr. Obes. 13, 391–403. doi: 10.2147/DMSO.S240956
Sinal, C. J., Tohkin, M., Miyata, M., Ward, J. M., Lambert, G., Gonzalez, F. J. (2000). Targeted disruption of the nuclear receptor FXR/BAR impairs bile acid and lipid homeostasis. Cell 102, 731–744. doi: 10.1016/S0092-8674(00)00062-3
Singh, N., Li, L. (2012). Reduced oxidative tissue damage during endotoxemia in IRAK-1 deficient mice. Mol. Immunol. 50, 244–252. doi: 10.1016/j.molimm.2012.01.011
Sodeman, T., Bronk, S. F., Roberts, P. J., Miyoshi, H., Gores, G. J. (2000). Bile salts mediate hepatocyte apoptosis by increasing cell surface trafficking of Fas. Am. J. Physiol. Gastrointest. Liver Physiol. 278, G992–G999. doi: 10.1152/ajpgi.2000.278.6.G992
Song, Y., Xu, C., Shao, S., Liu, J., Xing, W., Xu, J., et al. (2015). Thyroid-stimulating hormone regulates hepatic bile acid homeostasis via SREBP-2/HNF-4alpha/CYP7A1 axis. J. Hepatol. 62, 1171–1179. doi: 10.1016/j.jhep.2014.12.006
Song, C. W., Qiu, W., Zhou, X. Q., Feng, X. C., Chen, W. S. (2019). Elevated hepatic MDR3/ABCB4 is directly mediated by MiR-378a-5p in human obstructive cholestasis. Eur. Rev. Med. Pharmacol. Sci. 23, 2539–2547. doi: 10.26355/eurrev_201903_17402
Staudinger, J. L., Goodwin, B., Jones, S. A., Hawkins-Brown, D., MacKenzie, K., II, LaTour, A., et al. (2001). The nuclear receptor PXR is a lithocholic acid sensor that protects against liver toxicity. Proc. Natl. Acad. Sci. U. S. A. 98, 3369–3374. doi: 10.1073/pnas.051551698
Stojakovic, T., Putz-Bankuti, C., Fauler, G., Scharnagl, H., Wagner, M., Stadlbauer, V., et al. (2007). Atorvastatin in patients with primary biliary cirrhosis and incomplete biochemical response to ursodeoxycholic acid. Hepatology 46, 776–784. doi: 10.1002/hep.21741
Sun, L., Pang, Y., Wang, X., Wu, Q., Liu, H., Liu, B., et al. (2019). Ablation of gut microbiota alleviates obesity-induced hepatic steatosis and glucose intolerance by modulating bile acid metabolism in hamsters. Acta Pharm. Sin. B. 9, 702–710. doi: 10.1016/j.apsb.2019.02.004
Swann, J. R., Want, E. J., Geier, F. M., Spagou, K., Wilson, I. D., Sidaway, J. E., et al. (2011). Systemic gut microbial modulation of bile acid metabolism in host tissue compartments. Proc. Natl. Acad. Sci. U. S. A. 108 (Suppl 1), 4523–4530. doi: 10.1073/pnas.1006734107
Tabas, I., Ron, D. (2011). Integrating the mechanisms of apoptosis induced by endoplasmic reticulum stress. Nat. Cell Biol. 13, 184–190. doi: 10.1038/ncb0311-184
Teng, S., Jekerle, V., Piquette-Miller, M. (2003). Induction of ABCC3 (MRP3) by pregnane X receptor activators. Drug Metab. Dispos. 31, 1296–1299. doi: 10.1124/dmd.31.11.1296
Thomas, C., Pellicciari, R., Pruzanski, M., Auwerx, J., Schoonjans, K. (2008). Targeting bile-acid signalling for metabolic diseases. Nat. Rev. Drug Discovery 7, 678–693. doi: 10.1038/nrd2619
Trauner, M., Fuchs, C. D., Halilbasic, E., Paumgartner, G. (2017). New therapeutic concepts in bile acid transport and signaling for management of cholestasis. Hepatology 65, 1393–1404. doi: 10.1002/hep.28991
Tully, D. C., Rucker, P. V., Chianelli, D., Williams, J., Vidal, A., Alper, P. B., et al. (2017). Discovery of Tropifexor (LJN452), a Highly Potent Non-bile Acid FXR Agonist for the Treatment of Cholestatic Liver Diseases and Nonalcoholic Steatohepatitis (NASH). J. Med. Chem. 60, 9960–9973. doi: 10.1021/acs.jmedchem.7b00907
Urano, F., Wang, X., Bertolotti, A., Zhang, Y., Chung, P., Harding, H. P., et al. (2000). Coupling of stress in the ER to activation of JNK protein kinases by transmembrane protein kinase IRE1. Science 287, 664–666. doi: 10.1126/science.287.5453.664
van Dijk, R., Kremer, A. E., Smit, W., van den Elzen, B., van Gulik, T., Gouma, D., et al. (2015). Characterization and treatment of persistent hepatocellular secretory failure. Liver Int. 35, 1478–1488. doi: 10.1111/liv.12603
Wagner, M., Trauner, M. (2016). Recent advances in understanding and managing cholestasis. F1000Res 5. doi: 10.12688/f1000research.8012.1
Wang, L., Wu, G., Wu, F., Jiang, N., Lin, Y. (2017). Geniposide attenuates ANIT-induced cholestasis through regulation of transporters and enzymes involved in bile acids homeostasis in rats. J. Ethnopharmacol. 196, 178–185. doi: 10.1016/j.jep.2016.12.022
Wang, J., Fu, T., Dong, R., Wang, C., Liu, K., Sun, H., et al. (2019). Hepatoprotection of auraptene from the peels of citrus fruits against 17alpha-ethinylestradiol-induced cholestasis in mice by activating farnesoid X receptor. Food Funct. 10, 3839–3850. doi: 10.1039/C9FO00318E
Wasiak, S., Zunino, R., McBride, H. M. (2007). Bax/Bak promote sumoylation of DRP1 and its stable association with mitochondria during apoptotic cell death. J. Cell Biol. 177, 439–450. doi: 10.1083/jcb.200610042
Weber-Mzell, D., Zaupa, P., Petnehazy, T., Kobayashi, H., Schimpl, G., Feierl, G., et al. (2006). The role of nuclear factor-kappa B in bacterial translocation in cholestatic rats. Pediatr. Surg. Int. 22, 43–49. doi: 10.1007/s00383-005-1599-y
Win, S., Than, T. A., Fernandez-Checa, J. C., Kaplowitz, N. (2014). JNK interaction with Sab mediates ER stress induced inhibition of mitochondrial respiration and cell death. Cell Death Dis. 5, e989. doi: 10.1038/cddis.2013.522
Wintermeyer, P., Cheng, C. W., Gehring, S., Hoffman, B. L., Holub, M., Brossay, L., et al. (2009). Invariant natural killer T cells suppress the neutrophil inflammatory response in a mouse model of cholestatic liver damage. Gastroenterology 136, 1048–1059. doi: 10.1053/j.gastro.2008.10.027
Wu, W., Zhu, B., Peng, X., Zhou, M., Jia, D., Gu, J. (2014). Activation of farnesoid X receptor attenuates hepatic injury in a murine model of alcoholic liver disease. Biochem. Biophys. Res. Commun. 443, 68–73. doi: 10.1016/j.bbrc.2013.11.057
Wunsch, E., Trottier, J., Milkiewicz, M., Raszeja-Wyszomirska, J., Hirschfield, G. M., Barbier, O., et al. (2014). Prospective evaluation of ursodeoxycholic acid withdrawal in patients with primary sclerosing cholangitis. Hepatology 60, 931–940. doi: 10.1002/hep.27074
Xie, G., Wang, X., Huang, F., Zhao, A., Chen, W., Yan, J., et al. (2016). Dysregulated hepatic bile acids collaboratively promote liver carcinogenesis. Int. J. Cancer 139, 1764–1775. doi: 10.1002/ijc.30219
Xiong, X. L., Ding, Y., Chen, Z. L., Wang, Y., Liu, P., Qin, H., et al. (2019). Emodin Rescues Intrahepatic Cholestasis via Stimulating FXR/BSEP Pathway in Promoting the Canalicular Export of Accumulated Bile. Front. Pharmacol. 10, 522. doi: 10.3389/fphar.2019.00522
Yamasaki, Y., Kobayashi, K., Inaba, A., Uehara, D., Tojima, H., Kakizaki, S., et al. (2018). Indirect activation of pregnane X receptor in the induction of hepatic CYP3A11 by high-dose rifampicin in mice. Xenobiotica 48, 1098–1105. doi: 10.1080/00498254.2017.1400128
Yan, S. F., Fujita, T., Lu, J., Okada, K., Shan Zou, Y., Mackman, N., et al. (2000). Egr-1, a master switch coordinating upregulation of divergent gene families underlying ischemic stress. Nat. Med. 6, 1355–1361. doi: 10.1038/82168
Yan, J., Xie, G., Liang, C., Hu, Y., Zhao, A., Huang, F., et al. (2017). Herbal medicine Yinchenhaotang protects against alpha-naphthylisothiocyanate-induced cholestasis in rats. Sci. Rep. 7, 4211. doi: 10.1038/s41598-017-04536-5
Yang, F., Wang, Y., Li, G., Xue, J., Chen, Z. L., Jin, F., et al. (2018). Effects of corilagin on alleviating cholestasis via farnesoid X receptor-associated pathways in vitro and in vivo. Br. J. Pharmacol. 175, 810–829. doi: 10.1111/bph.14126
Yang, S., Wei, L., Xia, R., Liu, L., Chen, Y., Zhang, W., et al. (2019). Formononetin ameliorates cholestasis by regulating hepatic SIRT1 and PPARalpha. Biochem. Biophys. Res. Commun. 512, 770–778. doi: 10.1016/j.bbrc.2019.03.131
Yerushalmi, B., Dahl, R., Devereaux, M. W., Gumpricht, E., Sokol, R. J. (2001). Bile acid-induced rat hepatocyte apoptosis is inhibited by antioxidants and blockers of the mitochondrial permeability transition. Hepatology 33, 616–626. doi: 10.1053/jhep.2001.22702
Zeng, H., Li, D., Qin, X., Chen, P., Tan, H., Zeng, X., et al. (2016). Hepatoprotective Effects of Schisandra sphenanthera Extract against Lithocholic Acid-Induced Cholestasis in Male Mice Are Associated with Activation of the Pregnane X Receptor Pathway and Promotion of Liver Regeneration. Drug Metab. Dispos. 44, 337–342. doi: 10.1124/dmd.115.066969
Zeng, H., Jiang, Y., Chen, P., Fan, X., Li, D., Liu, A., et al. (2017). Schisandrol B protects against cholestatic liver injury through pregnane X receptors. Br. J. Pharmacol. 174, 672–688. doi: 10.1111/bph.13729
Zhang, L., Cheng, Y., Du, X., Chen, S., Feng, X., Gao, Y., et al. (2015). Swertianlarin, an Herbal Agent Derived from Swertia mussotii Franch, Attenuates Liver Injury, Inflammation, and Cholestasis in Common Bile Duct-Ligated Rats. Evid. Based Complement Alternat. Med. 2015, 948376. doi: 10.1155/2015/948376
Zhang, X., Ma, Z., Liang, Q., Tang, X., Hu, D., Liu, C., et al. (2015). Tanshinone IIA exerts protective effects in a LCA-induced cholestatic liver model associated with participation of pregnane X receptor. J. Ethnopharmacol. 164, 357–367. doi: 10.1016/j.jep.2015.01.047
Zhang, L., Miao, X. J., Wang, X., Pan, H. H., Li, P., Ren, H., et al. (2016). Antiproliferation of berberine is mediated by epigenetic modification of constitutive androstane receptor (CAR) metabolic pathway in hepatoma cells. Sci. Rep. 6, 28116. doi: 10.1038/srep28116
Zhao, Q., Liu, F., Cheng, Y., Xiao, X. R., Hu, D. D., Tang, Y. M., et al. (2019). Celastrol Protects From Cholestatic Liver Injury Through Modulation of SIRT1-FXR Signaling. Mol. Cell Proteomics. 18, 520–533. doi: 10.1074/mcp.RA118.000817
Zhong, D., Xie, Z., Huang, B., Zhu, S., Wang, G., Zhou, H., et al. (2018). Ganoderma Lucidum Polysaccharide Peptide Alleviates Hepatoteatosis via Modulating Bile Acid Metabolism Dependent on FXR-SHP/FGF. Cell Physiol. Biochem. 49, 1163–1179. doi: 10.1159/000493297
Keywords: cholestasis, bile acid, liver injury, mechanism, drug
Citation: Wei S, Ma X and Zhao Y (2020) Mechanism of Hydrophobic Bile Acid-Induced Hepatocyte Injury and Drug Discovery. Front. Pharmacol. 11:1084. doi: 10.3389/fphar.2020.01084
Received: 26 May 2020; Accepted: 03 July 2020;
Published: 16 July 2020.
Edited by:
Ruixin Zhu, Tongji University, ChinaReviewed by:
Jing Yuan, Children’s Hospital of Capital Institute of Pediatrics, ChinaChenghai Liu, Shanghai University of Traditional Chinese Medicine, China
Copyright © 2020 Wei, Ma and Zhao. This is an open-access article distributed under the terms of the Creative Commons Attribution License (CC BY). The use, distribution or reproduction in other forums is permitted, provided the original author(s) and the copyright owner(s) are credited and that the original publication in this journal is cited, in accordance with accepted academic practice. No use, distribution or reproduction is permitted which does not comply with these terms.
*Correspondence: Yanling Zhao, emhhb3lsMjg1NUAxMjYuY29t