- 1Riphah Institute of Pharmaceutical Sciences, Riphah International University, Islamabad, Pakistan
- 2College of Natural and Health Sciences, Zayed University, Abu Dhabi, United Arab Emirates
Background: Carveol is a natural drug product present in the essential oils of orange peel, dill, and caraway seeds. The seed oil of Carum Carvi has been reported to be antioxidant, anti-inflammatory, anti-hyperlipidemic, antidiabetic, and hepatoprotective.
Methods: The antidiabetic potential of carveol was investigated by employing in-vitro, in-vivo, and in-silico approaches. Moreover, alpha-amylase inhibitory assay and an alloxan-induced diabetes model were used for in-vitro and in-vivo analysis, respectively.
Results: Carveol showed its maximum energy values (≥ -7 Kcal/mol) against sodium-glucose co-transporter, aldose reductase, and sucrose-isomaltase intestinal, whereas it exhibited intermediate energy values (≥ -6 Kcal/mol) against C-alpha glucosidase, glycogen synthase kinases-3β, fructose-1,6-bisphosphatase, phosphoenolpyruvate carboxykinase, and other targets according to in-silico analysis. Similarly, carveol showed lower energy values (≥ 6.4 Kcal/mol) against phosphoenolpyruvate carboxykinase and glycogen synthase kinase-3β. The in-vitro assay demonstrated that carveol inhibits alpha-amylase activity concentration-dependently. Carveol attenuated the in-vivo alloxan-induced (1055.8 µMol/Kg) blood glucose level in a dose- and time-dependent manner (days 1, 3, 6, 9, and 12), compared to the diabetic control group, and further, these results are comparable with the metformin positive control group. Carveol at 394.1 µMol/Kg improved oral glucose tolerance overload in rats compared to the hyperglycemic diabetic control group. Moreover, carveol also attenuated the glycosylated hemoglobin level along with mediating anti-hyperlipidemic and hepatoprotective effects in alloxan-induced diabetic animals.
Conclusions: This study reveals that carveol exhibited binding affinity against different targets involved in diabetes and has antidiabetic, anti-hyperlipidemic, and hepatoprotective actions.
Introduction
Diabetes mellitus (DM) is a leading health issue, having a highly troubling frequency in developing countries. The primary risk factors of DM include a sedentary lifestyle, poor nutritional habits, and obesity (Hu, 2011). Diabetes is an independent risk factor and important comorbidity of several human diseases and increases the risk of death by 1.5–3 times (Capes et al., 2001). The WHO ranked DM the biggest epidemic and the most common cause of functional disabilities (Mathers and Loncar, 2006; Shah et al., 2019). DM is predominantly related to increased blood glucose level associated with alteration in the breakdown of fats, proteins, and carbohydrates due to either a decrease in the production of insulin. The low insulin level in type I diabetes is due to autoimmunity in beta cells of the pancreas (Tuomi, 2005), and the resulting hyperglycemia can be managed by administering insulin injections subcutaneously. In type II diabetes, there is rather a reduction in insulin sensitivity in hepatic, cardiac, and fat cells, and this can be managed by hypoglycemic drugs (Nathan et al., 2009). The foremost risk factors for the progression of anomalous secretion of insulin or resistance to it include genetic defects, aging, viral infection, environmental factors, and a sedentary lifestyle combined with high calorie intake (Saltiel, 2001).
Type II DM has long-term health hazards, including neuropathy, nephropathy, and accelerated atherosclerosis leading to an increased risk of myocardial infarction (MI) (Hanefeld et al., 1996; Viberti, 2005). Furthermore, it makes the human body prone to dyslipidemia, high blood pressure, and obesity (Association, 2014). Moreover, dyslipidemia triggers various cardiovascular complications such as atherosclerosis, MI, hypertension, and obesity-related problems (Ashfaq et al., 2017). Stress also plays an important role in the progression of hyperlipidemia due to the generation of free radicals, which may lead to atherosclerosis and cardiovascular diseases (Arise et al., 2016).
Alloxan is a potent inducer of pancreas toxicity and is therefore used for experimental diabetes induction (Brenna et al., 2003). Alloxan induces a multiphasic blood glucose response when injected into an experimental animal, with consistent fluctuations in the plasma insulin level associated with structural damage to beta cells (Olusanya and Ifeoluwa, 2018). The pathology of DM is attenuated by insulin therapy and orally by hypoglycemic medications such as biguanides and sulfonylureas. However, the therapeutic potential of these oral synthetic antidiabetic agents has been limited by long-term microvascular and macrovascular complications (Stenman et al., 1990; Spiller and Sawyer, 2006).
Carveol (Figure 1) is a natural drug substance present in the essential oils of orange peel, dill (Anethum graveolens L.), and caraway seeds (Crowell et al., 1992). The chemical components are D-limonene, mono-terpenes carveol acetate, and trans- and cis-carveol, glyceryl esters (Singh and Lal, 2008). The seeds of caraway oil (Carum carvi L.) have been described in customary Chinese medicine as antispasmodic, carminative, astringent, anti-inflammatory, anti-cancer, and hepatoprotective (Johri, 2011; Agrahari and Singh, 2014).
This study aims to demonstrate the antidiabetic potential of carveol through molecular docking, in-vitro study, and an in-vivo animal experimentation model using alloxan-induced diabetes and to further evaluate the anti-hyperlipidemic and hepatoprotective effect of carveol.
Materials and Methods
Chemicals
Alloxan monohydrate (CAS number 22-44-11-3), carveol (99-48-9), acarbose (56180-94-0), alpha-amylase (A6255), and metformin hydrochloride (11-15-70-4) were acquired from Sigma-Aldrich Co. LLC, USA. All chemicals were analytical grade (99% HPLC grade).
Animals
Adult Sprague–Dawley (SD) rats of either sex weighing 250–280 g and 7–11 weeks old were obtained from the local breeding facility of Riphah International University. The animals were kept in a standard animal room temperature at 18–22°C under circadian light and dark conditions with access to food and water ad libitum. All the experimental protocols were recommended and approved by the Research and Ethical Committee (REC) of the Riphah Institute of Pharmaceutical Sciences (RIPS) (Approval ID: Ref. No.: REC/RIPS/2019/03).
Computational Studies
The standard drugs used for in-silico analysis were miglitol, metformin, carbenoxolone, thiadiazolidinone-8 (TDZD-8), rosiglitazone, acarbose, and sergliflozin. 3D structures of human protein targets involved in DM were obtained from the online Protein Data Bank (PDB) (www.rcsb.org) (Sussman et al., 1998). The target proteins selected were: alpha-amylase (AA, PDB ID: 3BA1), C-alpha glucosidase (C-AG, PDB ID: 3L4T), aldose reductase (AR, PDB ID: 2R24), phosphoenolpyruvate carboxykinase (PEPCK, PDB ID: 1NI4), fructose-1,6-bisphosphatase (FBP1, PDB ID: 5ZWK), 11β-hydroxysteroid dehydrogenase-1 (11β-HSD1, PDB ID: 3D3E), glycogen synthase kinase-3β (GSK-3β, PDB ID: 6GJO), peroxisome proliferator-activated receptor-γ (PPAR-γ, PDB ID: 4EMA), phosphatidylinositol 3 kinase (PI3K, PDB ID: 1E90), sucrase-isomaltase intestinal (SIMI, PDB ID: 3TOP), and sodium-glucose co-transporter (SGLT, PDB ID: 2XQ2). By using discovery studio visualizer (DSV), water molecules and ligands were removed, and polar H-atoms were added, and the resulting structure was saved in PDB format. Molecular docking was performed by the Auto Dock tool, v.1.5.6, and the PyRx 0.8 docking tool (Duhovny et al., 2002). Docking was executed and evaluated on the basis of atomic contact energy (ACE) value (Kcal/mol). The best poses were evaluated, and the one with the lowest ACE value (Kcal/mol) was selected for evaluation through Biovia DSV. Each complex was assessed in a 3D pattern to check the maximum binding interactions formed between ligands and amino acid residues of the protein targets.
Alpha-Amylase Inhibitory Assay
The antidiabetic potential of the test compound carveol was determined by α-amylase inhibition assay following the standard protocol with minor modification (Kim et al., 2000). The reaction mixture containing 15 μl phosphate buffer (pH 6.8), 25 μl α-amylase enzyme (0.14 U/mL), different concentrations of the sample in normal saline, and 40 μl starch solution (2 mg/mL in potassium phosphate buffer) was incubated at 50°C for 30 min in a 96-well plate followed by addition of 20 μl of 1 M HCl to stop the reaction. Afterward, 90 μl of iodine reagent (5 mM iodine, 5 mM potassium iodide) was added to each well. Negative control was prepared without the sample, whereas blank was prepared without the sample and amylase enzyme, each being replaced by equal quantities of the buffer. Acarbose (250 μM/Kg) was used as a positive control. The absorbance of the reaction plate after incubation was measured at 540 nm. The activity was expressed as percent α-amylase inhibition and calculated by the following equation:
where On = Absorbance of negative control, Os = Absorbance of sample, and Ob = Absorbance of the blank well.
Blood Glucose Levels and Body Weight Measurement
SD rats were adjusted to the laboratory environments and reserved for whole night fasting (12–14 h). The animals were divided into six groups, each containing five animals (n=5). Group I and II were non-diabetic control and diabetic control groups injected with saline (10 mL/Kg) and alloxan monohydrate (1055.8 µMol/Kg), respectively. Groups III, IV, and V were alloxan-induced diabetic rats administered with the test compound at doses of 65.7, 197, and 394.1 µMol/Kg respectively. Group VI was positive control and was injected with metformin (1207.5 µMol/Kg). The blood glucose levels were measured at days 1, 3, 6, 9, and 12, using an Accu-Chek instant glucometer. For the complete treatment period, the body weight of animals was measured at the same regular intervals. For the induction of diabetes, alloxan monohydrate was used (Dunn and McLetchie, 1943). Freshly prepared alloxan solution (1055.8 µMol/Kg) in saline was given to experimental rats via an intra-peritoneal route (Olusanya and Ifeoluwa, 2018). After 48 h, blood glucose levels of experimental rats were measured by using the tail prick methodology. Rats with blood glucose levels ≥ 200 mg/dL were demarcated as hyperglycemic (Saudek et al., 2008).
Oral Glucose Tolerance Test (OGTT)
After 18 h of fasting, rats were placed into four groups. Group I and II were non-diabetic and diabetic control and were injected with saline (10 mL/Kg) and alloxan (1055.8 µMol/Kg), respectively. Group III was a carveol-treated (394.1 µMol/Kg) group. Group IV was positive control and injected with metformin (1207.5 µMol/Kg). Each group was pre-treated, and after 30 min, the D-glucose load (3 g/Kg) was administered orally. The blood glucose level was measured at 0, 30, 60, 90, and 120 min using an Accu-Chek instant glucometer (Rubino and Marescaux, 2004).
Glycosylated Hemoglobin (HbA1C) Test
After 6 weeks of treatment, the HbA1C test was performed for all groups (Asgary et al., 2008). A cardiac puncture methodology was utilized to collect blood samples (Doeing et al., 2003). The HbA1C level was measured in a local laboratory at Islamabad, Pakistan.
Serum Biomarker Analysis
A hepatic functional test was performed for each group (n=5/group). The cardiac puncture method was used to collect blood samples. The lipid profile in terms of TC, TGs, LDL, and HDL and the hepatic functional markers alanine transaminase (ALT), aspartate aminotransferase (AST), alkaline phosphatase (ALP), and total bilirubin (TB) were analyzed in a local laboratory at Islamabad, Pakistan.
Statistical Analysis
Data are presented as mean ± standard error of the mean (SEM). The significance of results was evaluated by analysis of variance (ANOVA), followed by a multiple comparison test. p < 0.05 was considered to be statistically significant. Statistical assessment, preparation of graphs, and evaluation were performed by using Graph Pad Prism 6.
Results
In-Silico Analysis
Against AA, carveol and miglitol showed ACE values of -6.2 and -6.3 Kcal/mol and formed 1 hydrogen bond (H-bond) with ASP197 and 5 H-bonds with ILE312, ASN301, ASP317, and THR314, respectively. Carveol showed hydrophobic interactions with LEU165, HIS299, TRP58, TYR62, and miglitol showed no hydrophobic interactions. Against C-AG, carveol and miglitol showed ACE values of -6.5 and -5.8 Kcal/mol and showed no H-bonds against C-AG and 3 H-bonds with LYS1099, SER1012, and TYR1044, respectively. Carveol showed hydrophobic interactions with HIS1584, TRP1355, TRP1418, TYR1251, PHE1559, and ILE1315, and miglitol showed no hydrophobic interactions. against AR, carveol and metformin exhibited ACE values of -7.1 and -5.3 Kcal/mol and made no H-bonds and 2 H-bonds with SER210 and GLN183, respectively. Carveol showed hydrophobic interactions with TRP20, TRP111, TYR209, SER210, HIS110, and ILE260, and metformin formed hydrophobic interactions with ASP43 and TYR209. Against PEPCK, carveol and metformin exhibited ACE values of -6.5 and -4.7 Kcal/mol and formed no H-bonds and 3 H-bonds with ARG438, GLN112, and GLU89, respectively. Carveol showed hydrophobic interactions with PHE517, PHE525, PHE530, and TRP516, and metformin showed hydrophobic interactions with GLU89. Against FBP1, carveol and metformin showed ACE values of -6.6 and -5.1 Kcal/mol and formed 3 H-bonds with THR171, SER45, and ARG49 and 2 H-bonds with ASP118 and LEU120, respectively. Carveol showed hydrophobic interactions with PRO188, ARG49, and LEU186, and metformin showed hydrophobic interactions with GLU97, GLU98, ASP118, and ASP121. Against 11β-HSD1, carveol and carbenoxolone showed ACE values of -6.6 and -11.2 Kcal/mol and formed 1 H-bond with SER125 and no H-bonds, respectively. Carveol showed hydrophobic interaction with PHE129, HIS135, ASN127, and ALA182 and carbenoxolone with ILE46, ILE121, ALA 223, LEU 217, TYR 177, and TYR183. Against GSK-3β, carveol and TDZD-8 exhibited ACE values of -6.4 and -6.6 Kcal/mol and formed 1 H-bond with ASP200 and 2 H-bonds with LEU88 and GLN89, respectively. Carveol showed hydrophobic interactions with VAL70, VAL110, LEU132, LEU188, ILE62, ALA83, TYR134, and CYS199 and TDZD-8 with PHE67 and VAL87. Against PPAR-γ, carveol and rosiglitazone showed ACE values of -6 and -8.5 Kcal/mol and made 1 H-bond with GLU295 and 4 H-bonds with LEU228, ARG288, and SER289, respectively. Carveol showed hydrophobic interactions with LEU330, LEU333, MET329, ILE326, ARG288, and ALA292 and rosiglitazone with PHE282, CYS285, ALA292, ILE326, LEU330, and HIS449. Against PI3K, carveol and rosiglitazone exhibited ACE values of -6.7 and -7.9 Kcal/mol and formed 1 H-bond with ASP278 and 4 H-bonds with PRO563, PHE497, and THR1043, respectively. Carveol showed hydrophobic interactions with HIS199, HIS693, LYS689, ARG690, MET728, and PRO789 and rosiglitazone with PRO590, LYS591, and ILE1048. Against SIMI, carveol and acarbose exhibited ACE values of -7 and -8.1 Kcal/mol and formed 1 H-bond with GLU1543 and 9 H-bonds with GLN1254, TYR1251, GLN1286, ARG1377, LEU1367, and GLN1372, respectively. Carveol showed hydrophobic interactions with PRO1160, LYS1536, PHE1544, LEU1524, and ALA1554 and acarbose with ILE1587. Against SGLT, carveol and sergliflozin presented ACE values of -7.3 and -9 Kcal/mol and made no H-bonds and 4 H-bonds with SER91, ASN64, ASN142, and GLN428, respectively. Carveol showed hydrophobic interactions with TRP448, LEU444, ALA495, and PHE447 and sergliflozin showed hydrophobic interactions with ILE427 (Figure 2). The ACE (Kcal/mol) values for ligand–complex interactions, the targets, the number of H-bonds, the amino acids employed in forming hydrophobic interactions, and the H-bonds of ligand complexes with targeted protein are presented in Table 1 and Figures S1–S10.
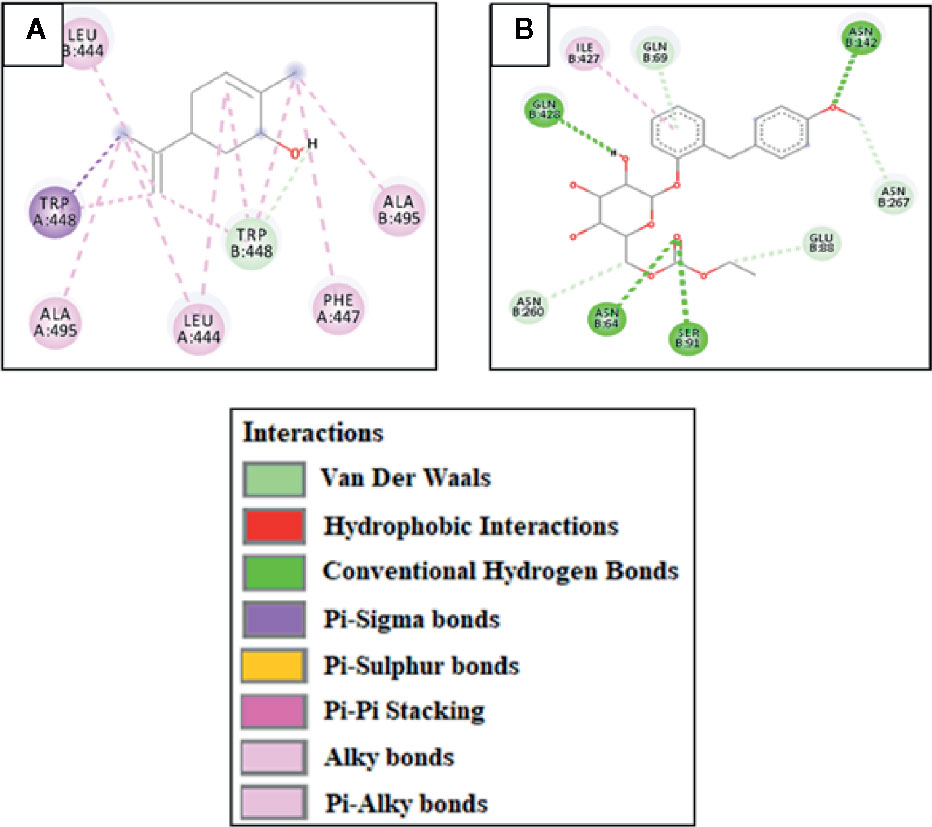
Figure 2 (A) and (B) represent 2D-interactions of carveol and sergliflozin with sodium-glucose co-transporter (SGLT), respectively, evaluated through Biovia Discovery Studio 2016.
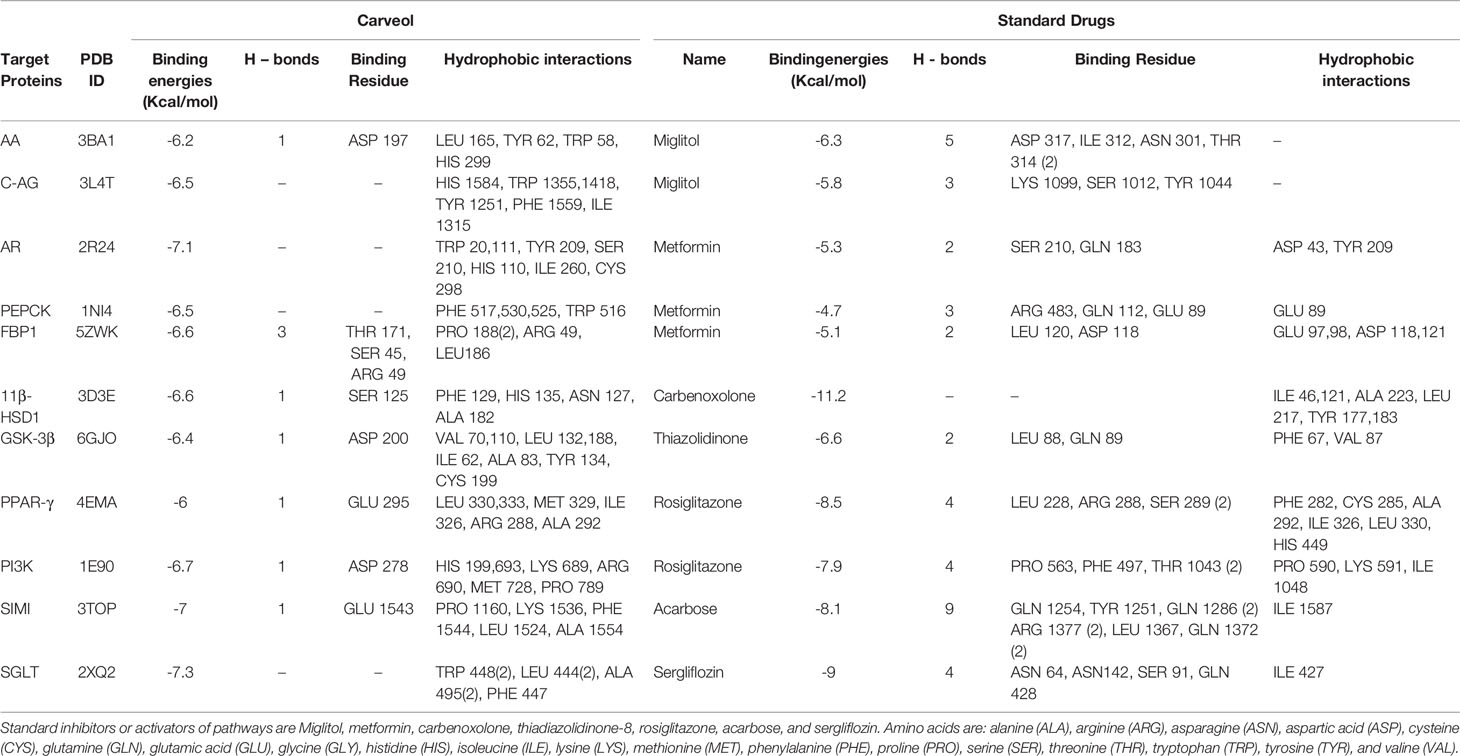
Table 1 Atomic contact energy (ACE) values (Kcal/mol) of best-docked poses of carveol and standard drugs, as well as hydrogen bonds and hydrophobic interactions formed, against AA, alpha-amylase; C-AG, C-alpha Glucosidase; AR, aldose reductase; PEPCK, phosphoenolpyruvate carboxykinase; FBP1, fructose-1,6-bisphosphatase; 11b-HSD1, 11b-hydroxysteroid dehydrogenase-1; GSK-3b, glycogen synthase kinases-3b; PPAR-g, peroxisome proliferator-activated receptor-g; PI3K, phosphatidylinositol 3 kinases; SIMI, sucrase-isomaltase intestinal; SGLT, sodium-glucose co-transporter.
α-Amylase Inhibition
Carveol and acarbose caused α-amylase enzyme inhibition at different concentrations; the results are shown in Table 2.
Effect on Blood Glucose Levels
The levels of blood glucose for the non-diabetic control (saline, 10 mL/Kg), diabetic control alloxan-treated (1055.8 µMol/Kg), carveol-treated (65.7, 197, and 394.1 µMol/Kg), and metformin-treated (positive control) (1207.5 µMol/Kg) groups are shown in Figure 3.
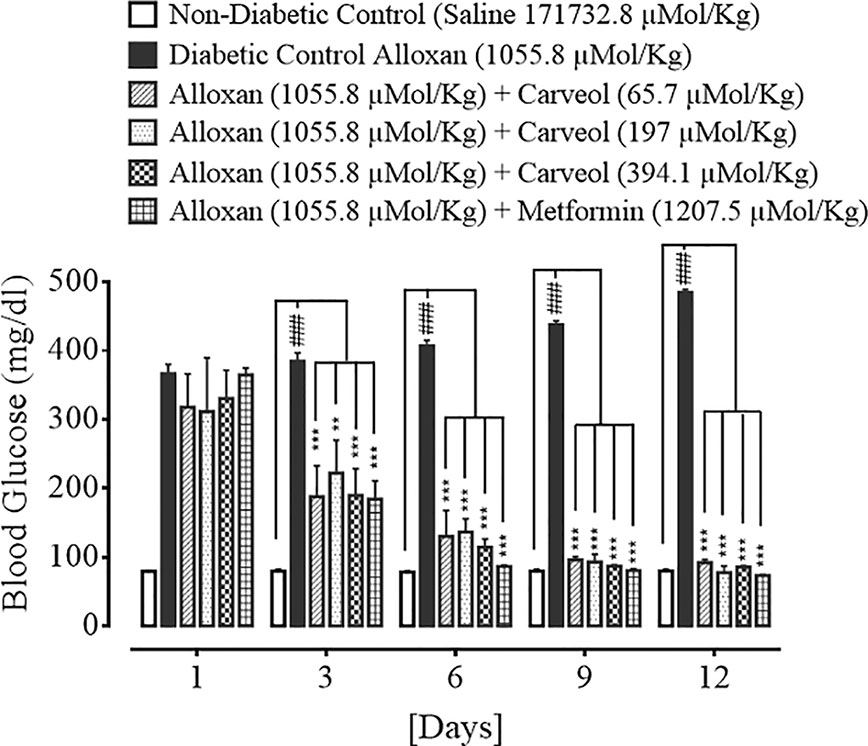
Figure 3 Bar graph representing blood glucose level on different treatment days of the saline-treated group (non-diabetic control), alloxan-treated group (diabetic control), carveol-treated groups at different doses (65.7, 197, 394.1 µMol/Kg), and metformin-treated group against alloxan-induced hyperglycemia in rats. Data presented as mean ± SEM. Statistical analysis used one-way ANOVA, followed by post-hoc Tukey’s test. ###p < 0.001 vs. saline group, **p < 0.01 and ***p < 0.001 comparison of the blood glucose levels of carveol- and metformin-treated groups vs. diabetic control group.
Effect on Body Weight
The body weights of non-diabetic control (saline, 10 mL/Kg), diabetic control alloxan-treated (1055.8 µMol/Kg), carveol-treated (65.7, 197, and 394.1 µMol/Kg), and metformin-treated (positive control) (1207.5 µMol/Kg) groups are shown in Table 3.
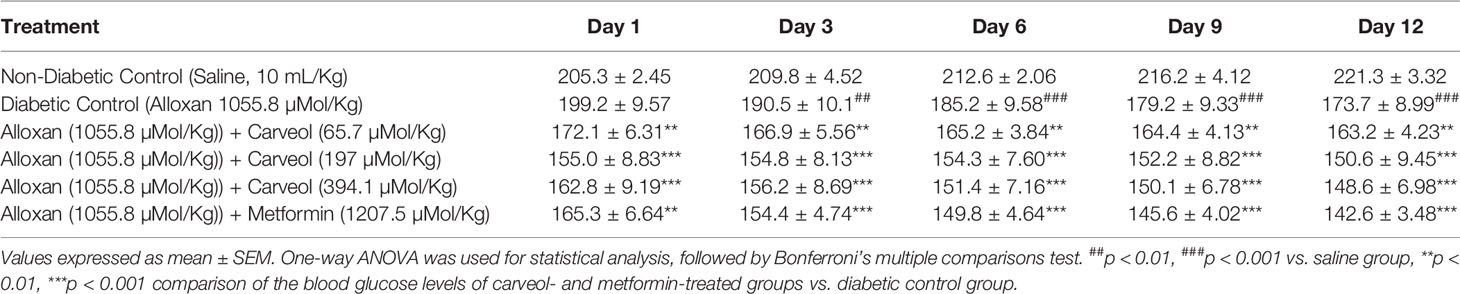
Table 3 Effect of carveol and metformin on different treatment days on body weight (g) of alloxan-induced diabetic rats.
Effect on Glucose Tolerance
The blood glucose levels of non-diabetic control (saline, 10 mL/Kg), diabetic control alloxan-treated (1055.8 µMol/Kg), carveol-treated (394.1 µMol/Kg) and metformin-treated (positive control) (1207.5 µMol/Kg) groups at the different time intervals (0 – 120 min) are shown in Figure 4.
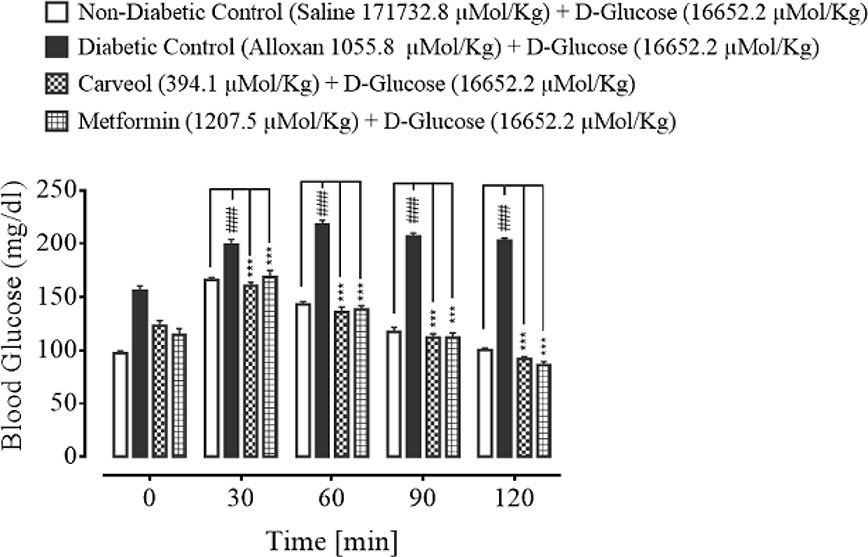
Figure 4 Bar graph representing blood glucose levels at different time intervals (0-120) after administration of oral glucose load (non-diabetic control), alloxan-treated (diabetic control), carveol-treated, and metformin-treated groups. Values expressed as mean ± SEM. One-way ANOVA was used for statistical analysis, followed by post-hoc Tukey’s test. ###p < 0.001 vs. saline group and ***p < 0.001 comparison of the blood glucose levels of carveol- and metformin-treated groups vs. diabetic control group.
Effect on HbA1C
The HbA1C levels of non-diabetic control (saline, 10 mL/Kg), diabetic control alloxan-treated, carveol-treated (65.7, 197, and 394.1 µMol/Kg), and metformin-treated (positive control) (1207.5 µMol/Kg) groups are shown in Table 4.
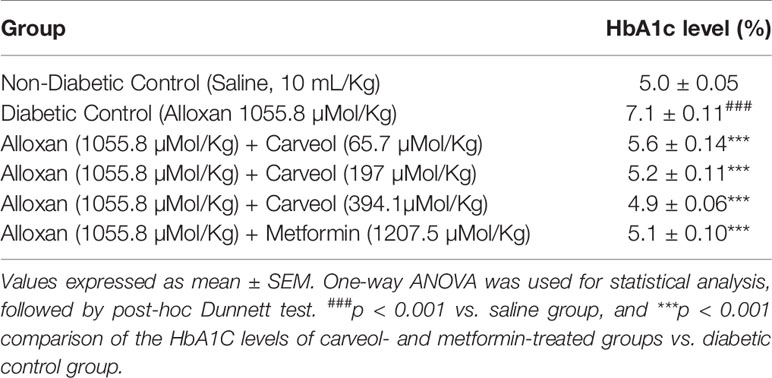
Table 4 Effect of carveol at different doses and metformin on glycosylated hemoglobin A1C (HbA1C) in alloxan-induced diabetic rats.
Effect on Lipid Profile
The serum levels of TGs, TC, LDL, and HDL of non-diabetic control (saline 10 mL/Kg), diabetic control alloxan-treated, carveol-treated (65.7, 197, and 394.1 µMol/Kg), and metformin-treated (positive control) groups are shown in Table 5.
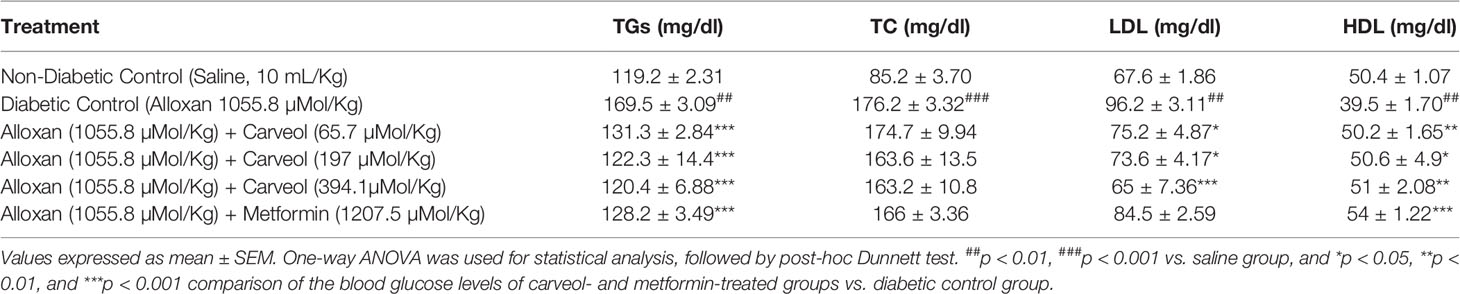
Table 5 Effect of carveol on levels of triglycerides (TGs), total cholesterol (TC), low-density lipoprotein (LDL), and high-density lipoprotein (HDL) in alloxan-induced diabetic rats.
Effect on Hepatic Enzymes
The liver enzyme levels AST, ALT, ALP, and TB of non-diabetic control (saline 10 mL/Kg), diabetic control alloxan-treated (1055.8 µMol/Kg), carveol-treated (65.7, 197, and 394.1 µMol/Kg), and metformin-treated (positive control) (1207.5 µMol/Kg) groups are shown in Table 6.
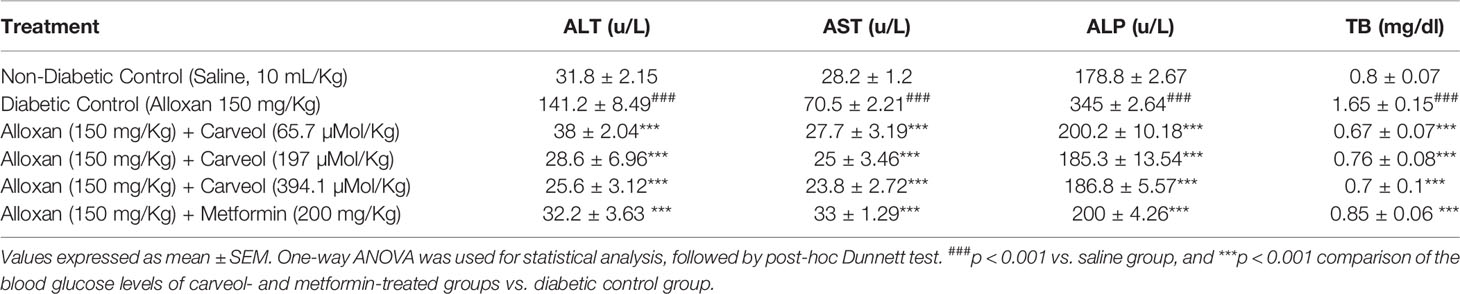
Table 6 Effect of Carveol on levels of alanine transaminase (ALT), aspartate aminotransferase (AST), alkaline phosphatase (ALP), and total bilirubin (TB) in alloxan-induced diabetic rats.
Discussion
Virtual screening, or the in-silico approach, is a procedure through which ligands are docked with respective target proteins by using a fast and cost-effective technique, and it requires computer-assisted programs and software (Prakhov et al., 2010). We demonstrated a comparative study by comparing the results for ligand–protein complexes to those for the standard drugs, obtained from the RCSB and PubChem database. Auto-Dock vina, patch dock, gromacs, and gold suite provide docking of ligands with a possibility of a dozen torsional degrees of freedom. A lower binding value (kcal/mol) reveals reduced energy of desolvation, which depicts stability of the ligand–protein complex (Pecsi et al., 2010). Hydrogen bonding, pi–pi bonding, and other hydrophobic interactions provide valuable strength for structurally complex stabilization. Other hydrophobic interactions play a vital role in increasing the affinity of the ligand towards the protein receptor (Dallakyan and Olson, 2015). E-value, H bonding, and hydrophobic interactions play a key role in the assessment of the binding affinity of ligand and protein complexes. We demonstrated here that carveol manifested the best binding score with the lowest E value against SGLT. Based on the E value against different protein targets, the order of ligand affinity was found to be SGLT > AR > SIMI > PI3K > 11β-HSD1 > FBP1 > C-AG > PEPCK > GSK-3β > AA > PPAR-γ.
The enzyme alpha-amylase and alpha-glucosidase are responsible for the breakdown of carbohydrates and for hydrolyzing starch into glucose before absorption (Chelladurai and Chinnachamy, 2018). Reduction in postprandial hyperglycemic level is due to alpha-amylase inhibition, which delays the small intestine carbohydrate digestion and diminishes the postprandial blood glucose level (Kwon et al., 2007). One of the approaches for treating DM is to inhibit the carbohydrate digesting enzymes such as α-amylase in the GIT and thereby reduce postprandial glucose (Raman et al., 2012). Previous studies demonstrated that flavonoids, tannins, and terpenoids could effectively inhibit alpha-amylase and alpha-glucosidase (Khan et al., 2014). Similarly, we demonstrated that carveol can inhibit α-amylase concentration-dependently, which may be due to its mono-terpenoid nature. Moreover, α-amylase inhibition was performed at various concentrations and compared with the standard drug acarbose at the same concentrations.
In the present study, the antidiabetic effect of carveol against alloxan-induced diabetes in rats was investigated. Administration of alloxan leads to inhibition of insulin secretion, resulting in persistent hyperglycemia or diabetes (Yang et al., 2010). Carveol reversed the blood glucose level in a dose-dependent and time-dependent fashion when compared to the alloxan-treated diabetic group. Furthermore, the results for carveol were comparable to those for the standard metformin group, which was used as a positive control. Metformin lowers the blood glucose level by numerous pathways, including decreasing biogenesis in hepatic tissue, minimizing glucose absorption by the intestine, and improving its peripheral utilization through enhancing insulin sensitivity (Klip and Leiter, 1990). We demonstrated that carveol reduced the body weights of test animals at regular daily intervals. As obesity is directly related to diabetes, a drug with the dual benefits of glycemic and weight control is of interest in diabetes, so this is an attractive outcome of carveol usage. In the glucose-loaded hyperglycemia model, which aims to assess oral glucose tolerance, carveol exhibited considerably better tolerance of glucose overload at regular intervals than did the metformin group. Carveol produced a dose-dependent effect of reducing the HbA1C level and was found to be effective as a long-term antidiabetic agent.
Diabetes is also associated with a variable lipid profile (Virdi et al., 2003), which can be further linked to obesity and renal impairment (Al-Shamaony et al., 1994). The marked hyperlipidemia that characterizes the diabetic state may be a consequence of the abnormal function of lipolytic hormones on the fat depots (Ayeleso et al., 2012). Lowering of serum lipid levels through dietary or drug therapy seems to be associated with a decrease in the risk of vascular disease in diabetes (Ayeleso et al., 2012). The results of the present investigation show that carveol produced a significant decrease in TC, TG, and LDL and a significant increase in HDL as compared to diabetic control. This improvement in the lipid profile status of alloxan-treated rats revealed the anti-hyperlipidemic properties of carveol. It has previously been reported that about 30% of blood cholesterol is circulated in the form of HDL-cholesterol (HDL-C). HDL-C removes cholesterol atheroma from arteries and transports it to the liver for excretion or re-utilization, which is a beneficial approach in cardiovascular diseases (Owolabi et al., 2010). Therefore, the increase in the serum HDL-C level by carveol in hyperglycemic rats indicates that carveol can augment HDL-C effects. Furthermore, our study demonstrated that various hepatic enzymes, such as AST, ALT, and ALP, were significantly increased in the alloxan-induced diabetic control group. However, carveol significantly decreased the level of these enzymes compared to diabetic control. Furthermore, increased gluconeogenesis and ketogenesis might be due to elevated activity of transaminases (Gandhi et al., 2011) associated with hepatocyte damage in experimental animals (Abolfathi et al., 2012). The ability of carveol to attenuate the serum level of ALT, AST, and ALP suggests its hepato-cellular protective effect.
Conclusions
The present study demonstrated that carveol exhibited maximum binding affinity against SGLT, intermediate binding affinity against FBP1, and lower energy values against PEPCK and GSK-3β. Moreover, our in-vitro and in-vivo study suggests that carveol could be a promising therapeutic agent in the management of diabetes. However, extensive exploration is still required to delineate the underlying protective mechanisms of carveol.
Data Availability Statement
All datasets generated and analyzed for this study are included in the article/Supplementary Material.
Ethics Statement
The animal study was reviewed and approved by Ethical Committee, Riphah Institute of Pharmaceutical Sciences (Ref. No.: REC/RIPS/2019/03).
Author Contributions
MA carried out the computational studies, in-vitro and in-vivo experimentation, evaluation of results, and documentation. A-uK, and FS supervised the research project and drafted the initial and final version of the manuscript. LK help in data analysis, revision and with drug Carveol. All authors contributed to the article and approved the submitted version.
Conflict of Interest
The authors declare that the research was conducted in the absence of any commercial or financial relationships that could be construed as a potential conflict of interest.
Acknowledgments
We are thankful to the Riphah Institute of Pharmaceutical Sciences, Riphah International University, Islamabad, for helping us in our research work.
Supplementary Material
The Supplementary Material for this article can be found online at: https://www.frontiersin.org/articles/10.3389/fphar.2020.00919/full#supplementary-material
References
Abolfathi, A. A., Mohajeri, D., Rezaie, A., Nazeri, M. (2012). Protective effects of green tea extract against hepatic tissue injury in streptozotocin-induced diabetic rats. Evid. Based Complement Altern. Med. 2012, 1–10. doi: 10.1155/2012/740671
Agrahari, P., Singh, D. K. (2014). A review on the pharmacological aspects of Carum carvi. J. Biol. Earth Sci. 4, 1–13
Al-Shamaony, L., Al-Khazraji, S. M., Twaij, H. A. (1994). Hypoglycaemic effect of Artemisia herba alba. II. Effect of a valuable extract on some blood parameters in diabetic animals. J. Ethnopharmacol. 43, 167–171. doi: 10.1016/0378-8741(94)90038-8
Arise, R. O., Aburo, O. R., Farohunbi, S. T., Adewale, A. A. (2016). Antidiabetic and antioxidant activities of ethanolic extract of dried flowers of Moringa oleifera in streptozotocin-induced diabetic rats. Acta Fac. Medicae Naiss. 33, 259–272. doi: 10.1515/afmnai-2016-0028
Asgary, S., Parkhideh, S., Solhpour, A., Madani, H., Mahzouni, P., Rahimi, P., et al. (2008). Effect of ethanolic extract of Juglans regia L. on blood sugar in diabetes-induced rats. J. Med. Food. 11, 533–538. doi: 10.1089/jmf.2007.0611
Ashfaq, A., Khan, A. U., Minhas, A. M., Aqeel, T., Assiri, A. M., Bukhari, I. A., et al. (2017). Anti-hyperlipidemic effects of Caralluma edulis (Asclepiadaceae) and Verbena officinalis (Verbenaceae) whole plants against high-fat diet-induced hyperlipidemia in mice. Trop. J. Pharm. Res. 16, 2417–2423.
Association, A. D. (2014). Diagnosis and classification of diabetes mellitus. Diabetes Care 37, 81–90. doi: 10.2337/dc14-S081
Ayeleso, A., Oguntibeju, O. O., Brooks, N. L. (2012). Effects of dietary intake of red palm oil on fatty acid composition and lipid profiles in male Wistar rats. Afr. J. Biotechnol. 11, 8275–8279. doi: 10.5897/AJB11.4080
Brenna, Ø., Qvigstad, G., Brenna, E., Waldum, H. (2003). Cytotoxicity of streptozotocin on neuroendocrine cells of the pancreas and the gut. Dig Dis. Sci. 48, 906–910. doi: 10.1023/A:1023043411483
Capes, S. E., Hunt, D., Malmberg, K., Pathak, P., Gerstein, H. C. (2001). Stress hyperglycemia and prognosis of stroke in nondiabetic and diabetic patients: a systematic overview. Stroke 32, 2426–2432. doi: 10.1161/hs1001.096194
Chelladurai, G. R. M., Chinnachamy, C. (2018). Alpha amylase and Alpha glucosidase inhibitory effects of aqueous stem extract of Salacia oblonga and its GC-MS analysis. Braz. J. Pharm. 54. doi: 10.1590/s2175-97902018000117151
Crowell, P. L., Kennan, W. S., Haag, J. D., Ahmad, S., Vedejs, E., Gould, M. N. (1992). Chemoprevention of mammary carcinogenesis by hydroxylated derivatives of d-limonene. Carcinogenesis 13, 1261–1264. doi: 10.1093/carcin/13.7.1261
Dallakyan, S., Olson, A. J. (2015). Small-molecule library screening by docking with PyRx. Chem. Biol., 243–250. In J. E. H. H. W. C. Hong (Ed.). doi: 10.1007/978-1-4939-2269-7_19
Doeing, D. C., Borowicz, J. L., Crockett, E. T. (2003). Gender dimorphism in differential peripheral blood leukocyte counts in mice using cardiac, tail, foot, and saphenous vein puncture methods. BMC Clin. Pathol. 3, 3. doi: 10.1186/1472-6890-3-3
Duhovny, D., Nussinov, R., Wolfson, H. J. (2002). Efficient unbound docking of rigid molecules. International Workshop on Algorithms in Bioinformatics 185–200. doi: 10.1007/3-540-45784-4_14
Dunn, J. S., McLetchie, N. (1943). Experimental alloxan diabetes in the rat. Lancet 242, 384–387. doi: 10.1016/S0140-6736(00)87397-3
Gandhi, G. R., Ignacimuthu, S., Paulraj, M. G., Sasikumar, P. (2011). Antihyperglycemic activity and antidiabetic effect of methyl caffeate isolated from Solanum torvum Swartz. fruit in streptozotocin induced diabetic rats. Eur. J. Pharmacol. 670, 623–631. doi: 10.1016/j.ejphar.2011.09.159
Hanefeld, M., Fischer, S., Julius, U., Schulze, J., Schwanebeck, U., Schmechel, H., et al. (1996). Risk factors for myocardial infarction and death in newly detected NIDDM: the Diabetes Intervention Study, 11-year follow-up. Diabetologia 39, 1577–1583. doi: 10.1007/s001250050617
Hu, F. B. (2011). Globalization of diabetes: the role of diet, lifestyle, and genes. Diabetes Care 34, 1249–1257. doi: 10.2337/dc11-0442
Johri, R. (2011). Cuminum cyminum and Carum carvi: An update. Pharmacogn. Rev. 5, 63. doi: 10.4103/0973-7847.79101
Khan, M. F., Rawat, A. K., Pawar, B., Gautam, S., Srivastava, A. K., Negi, D. S., et al. (2014). Bioactivity-guided chemical analysis of Melia azedarach L.(Meliaceae), displaying antidiabetic activity. Fitoterapia 98, 98–103. doi: 10.1016/j.fitote.2014.07.014
Kim, J. S., Kwon, C. S., SoN, K. H. (2000). Inhibition of alpha-glucosidase and amylase by luteolin, a flavonoid. Biosci. Biotech. Bioch. 64, 2458–2461. doi: 10.1271/bbb.64.2458
Klip, A., Leiter, L. A. (1990). Cellular mechanism of action of metformin. Diabetes Care 13, 696–704. doi: 10.2337/diacare.13.6.696
Kwon, Y., II, Apostolidis, E., Shetty, K. (2007). Evaluation of pepper (Capsicum annuum) for management of diabetes and hypertension. J. Food Biochem. 31, 370–385. doi: 10.1111/j.1745-4514.2007.00120.x
Mathers, C. D., Loncar, D. (2006). Projections of global mortality and burden of disease from 2002 to 2030. PloS Med. 3, 442. doi: 10.1371/journal.pmed.0030442
Nathan, D. M., Buse, J. B., Davidson, M. B., Ferrannini, E., Holman, R. R., Sherwin, R., et al. (2009). Medical management of hyperglycemia in type 2 diabetes: a consensus algorithm for the initiation and adjustment of therapy: a consensus statement of the American Diabetes Association and the European Association for the Study of Diabetes. Diabetes Care 32, 193–203. doi: 10.2337/dc08-9025
Olusanya, A. R., Ifeoluwa, B. S. (2018). “Antidiabetic and Safety Properties of Ethanolic Leaf Extract of Corchorus olitorius in Alloxan-Induced Diabetic Rats.” in Diabetes Food Plan. doi: 10.5772/intechopen.71529
Owolabi, O., James, D., Ibrahim, A., Folorunsho, O., Bwalla, I., Akanta, F., et al. (2010). Changes in lipid profile of aqueous and ethanolic extract of Blighia sapida in rats. AJMS 2, 177–180.
Pecsi, I., Leveles, I., Harmat, V., Vertessy, B. G., Toth, J. (2010). Aromatic stacking between nucleobase and enzyme promotes phosphate ester hydrolysis in dUTPase. Nucleic Acids Res. 38, 7179–7186. doi: 10.1093/nar/gkq584
Prakhov, N. D., Chernorudskiy, A. L., Gainullin, M. R. (2010). VSDocker: a tool for parallel high-throughput virtual screening using AutoDock on Windows-based computer clusters. J. Bioinform. 26, 1374–1375. doi: 10.1093/bioinformatics/btq149
Raman, B., Krishna, N., Rao, N., Saradhi, P., Rao, B. (2012). Plants with antidiabetic activities and their medicinal values. Int. Res. J. Pharm. 3, 11–15.
Rubino, F., Marescaux, J. (2004). Effect of duodenal–jejunal exclusion in a non-obese animal model of type 2 diabetes: a new perspective for an old disease. Ann. Surg. 239, 1. doi: 10.1097/01.sla.0000102989.54824.fc
Saltiel, A. R. (2001). New perspectives into the molecular pathogenesis and treatment of type 2 diabetes. Cell 104, 517–529. doi: 10.1016/S0092-8674(01)00239-2
Saudek, C. D., Herman, W. H., Sacks, D. B., Bergenstal, R. M., Edelman, D., Davidson, M. B., et al. (2008). A new look at screening and diagnosing diabetes mellitus. J. Clin. Endocrinol. Metab. 93, 2447–2453. doi: 10.1210/jc.2007-2174
Shah, F. A., Li, T., Kury, L. T. A., Zeb, A., Khatoon, S., Liu, G., et al. (2019). Pathological Comparisons of the Hippocampal Changes in the Transient and Permanent Middle Cerebral Artery Occlusion Rat Models. Front. Neurol. 10:1178. doi: 10.3389/fneur.2019.01178
Singh, K., Lal, B. (2008). Ethnomedicines used against four common ailments by the tribal communities of Lahaul-Spiti in western Himalaya. J. Ethnopharmacol. 115, 147–159. doi: 10.1016/j.jep.2007.09.017
Spiller, H. A., Sawyer, T. S. (2006). Toxicology of oral antidiabetic medications. Am. J. Health Syst. Pharm. 63, 929–938. doi: 10.2146/ajhp050500
Stenman, S., Groop, P., Laakkonen, K., Wahlin-Boll, E., Melander, A. (1990). Relationship between sulfonylurea dose and metabolic effect. Diabetes 39, 108. doi: 10.2337/diacare.15.6.737
Sussman, J. L., Lin, D., Jiang, J., Manning, N. O., Prilusky, J., Ritter, O., et al. (1998). Protein Data Bank (PDB): database of three-dimensional structural information of biological macromolecules. IUCr 54, 1078–1084. doi: 10.1107/S0907444998009378
Tuomi, T. (2005). Type 1 and type 2 diabetes: what do they have in common? Diabetes 54, 40–45. doi: 10.2337/diabetes.54.suppl_2.S40
Viberti, G. (2005). Thiazolidinediones—benefits on microvascular complications of type 2 diabetes. J. Diabetes Complicat. 19, 168–177. doi: 10.1016/j.jdiacomp.2004.04.001
Virdi, J., Sivakami, S., Shahani, S., Suthar, A., Banavalikar, M., Biyani, M., et al. (2003). Antihyperglycemic effects of three extracts from Momordica charantia. J. Ethnopharmacol. 88, 107–111. doi: 10.1016/S0378-8741(03)00184-3
Keywords: carveol, molecular docking, alpha-amylase, antidiabetic, anti-hyperlipidemic, hepatoprotective
Citation: Ahmed MS, Khan A-u, Kury LTA and Shah FA (2020) Computational and Pharmacological Evaluation of Carveol for Antidiabetic Potential. Front. Pharmacol. 11:919. doi: 10.3389/fphar.2020.00919
Received: 02 August 2019; Accepted: 05 June 2020;
Published: 29 July 2020.
Edited by:
Michael Heinrich, UCL School of Pharmacy, United KingdomReviewed by:
Hanefi Özbek, Istanbul Medipol University, TurkeyHabib Yaribeygi, Semnan University of Medical Sciences, Iran
Copyright © 2020 Ahmed, Khan, Kury and Shah. This is an open-access article distributed under the terms of the Creative Commons Attribution License (CC BY). The use, distribution or reproduction in other forums is permitted, provided the original author(s) and the copyright owner(s) are credited and that the original publication in this journal is cited, in accordance with accepted academic practice. No use, distribution or reproduction is permitted which does not comply with these terms.
*Correspondence: Arif-ullah Khan, YXJpZi51bGxhaEByaXBoYWguZWR1LnBr; YXJpZnVsbGFoa2hhbjk3OUBob3RtYWlsLmNvbQ==