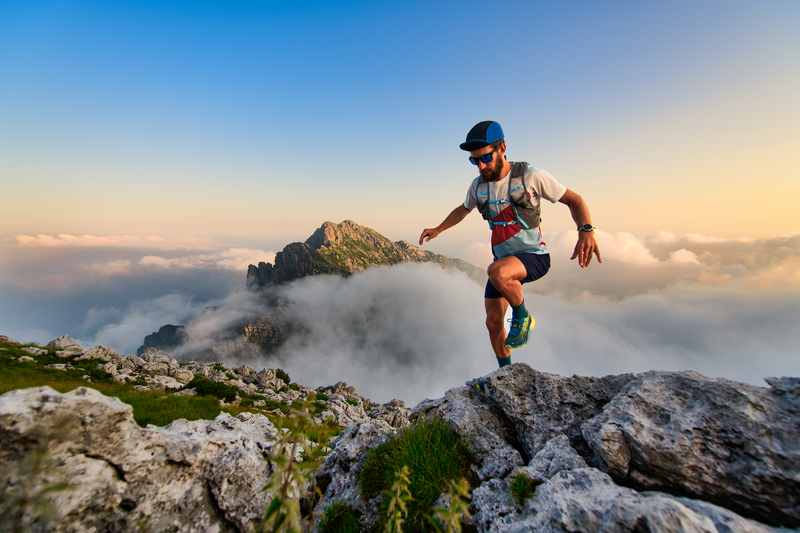
94% of researchers rate our articles as excellent or good
Learn more about the work of our research integrity team to safeguard the quality of each article we publish.
Find out more
MINI REVIEW article
Front. Pharmacol. , 15 January 2020
Sec. Neuropharmacology
Volume 10 - 2019 | https://doi.org/10.3389/fphar.2019.01494
This article is part of the Research Topic Non-Dopaminergic Systems in Parkinson’s Disease View all 11 articles
Parkinson’s disease (PD) is a neurodegenerative disorder characterized by hypokinetic motor features; however, patients also display non-motor symptoms like sleep disorders. The standard treatment for PD is dopamine replacement with L-DOPA; however, symptoms including gait deficits and sleep disorders are unresponsive to L-DOPA. Notably, these symptoms have been linked to aberrant activity in the pedunculopontine nucleus (PPN). Of late, clinical trials involving PPN deep brain stimulation (DBS) have been employed to alleviate gait deficits. Although preclinical evidence implicating PPN cholinergic neurons in gait dysfunction was initially promising, DBS trials fell short of expected outcomes. One reason for the failure of DBS may be that the PPN is a heterogenous nucleus that consists of GABAergic, cholinergic, and glutamatergic neurons that project to a diverse array of brain structures. Second, DBS trials may have been unsuccessful because PPN neurons are susceptible to mitochondrial dysfunction, Lewy body pathology, and degeneration in PD. Therefore, pharmaceutical or gene-therapy strategies targeting specific PPN neuronal populations or projections could better alleviate intractable PD symptoms. Unfortunately, how PPN neuronal populations and their respective projections influence PD motor and non-motor symptoms remains enigmatic. Herein, we discuss normal cellular and neuroanatomical features of the PPN, the differential susceptibility of PPN neurons to PD-related insults, and we give an overview of literature suggesting a role for PPN neurons in motor and sleep deficits in PD. Finally, we identify future approaches directed towards the PPN for the treatment of PD motor and sleep symptoms.
The pedunculopontine tegmental nucleus (PPN), a heterogeneous brainstem structure, consists of glutamatergic, cholinergic, GABAergic, and glycinergic neurons (Mineff et al., 1998; Wang and Morales, 2009; Pienaar and van de Berg, 2013), which co-express neuropeptides including nitric oxide, substance P, atriopeptin, NADPH diaphorase, calcium binding proteins, and corticotropin-releasing factor (Vincent et al., 1983; Standaert et al., 1986; Austin et al., 1995; Martinez-Gonzalez et al., 2012; D’Onofrio et al., 2015). Co-expression of acetylcholine (ACh) with GABA or glutamate has also been reported (Lavoie and Parent, 1994; Wang and Morales, 2009). The PPN is functionally divided into two components, a rostral portion containing GABAergic, glutamatergic, and sparse cholinergic neurons sending projections primarily to motor structures including the substantia nigra and thalamus, and a caudal portion consisting mainly of cholinergic and glutamatergic neurons projecting to structures involved in reward such as the nucleus accumbens (Dautan et al., 2016; Xiao et al., 2016). PPN neurons also send descending projections to cerebellar nuclei, brainstem structures like the pontinus oralis, gigantocellular nucleus, and the spinal cord to modulate movement and muscle tone (Rye et al., 1988; Spann and Grofova, 1989; Martinez-Gonzalez et al., 2014). See Figures 1A, B for PPN efferent projections and their involvement in Parkinson’s disease (PD) symptoms.
Figure 1 Efferent PPN projections and putative involvement in PD symptoms. (A) PPN efferents to brain areas involved in PD motor symptoms, (B) PPN efferents contacting brain areas involved in PD-related sleep disturbances. STR, Striatum; THAL, Thalamus; STN, Sub-thalamic nucleus; GPi, Globus Pallidus Interna; DRN, Dorsal Raphe Nucleus; Med, Medulla; SNr, Substantia Nigra Pars Reticulata; SNc, Substantia Nigra Pars Compacta; H-Thal, Hypothalamus; (Images modified from serviermedical art https://smart.servier.com).
PD is associated with degeneration of nigrostriatal dopamine (DA) neurons; however, PPN cholinergic, glutamatergic, substance P, GABAergic, and glycinergic neurons also degenerate in PD (Hirsch et al., 1987; Jellinger, 1988; Gomez-Gallego et al., 2006; Rinne et al., 2008; Pienaar and van de Berg, 2013). Microglial activation and inflammatory responses occurring in PD likely contribute to PPN neurodegeneration (Elson et al., 2016). Cholinergic loss, in particular, is linked to PD symptom severity, especially akinesia and gait deficits (Pahapill and Lozano, 2000; Rinne et al., 2008; Bohnen et al., 2013). Multiple factors likely contribute to death of cholinergic PPN neurons in PD. For instance, mitochondrial DNA deletion occurs more often in PPN cholinergic neurons than in other PPN neurons (Bury et al., 2017). If substantial, this may inhibit protein translation and energy production. In addition, alpha synuclein aggregates may differentially affect PPN neuronal subtypes, as glutamatergic and cholinergic PPN neurons show more effects of alpha synuclein aggregates than GABAergic neurons (Heinrich et al., as cited by Surmeier, 2018).
Furthermore, it is unknown whether cholinergic loss is a downstream response to prior death of monoaminergic neurons, or whether death of PPN cholinergic neurons precipitates loss of DA neurons (Bensaid et al., 2016; MacLaren et al., 2018). Past research in preclinical models indicates that both processes may occur. Additionally, lesioning both nigrostriatal dopamine and PPN cholinergic systems simultaneously results in more severe degeneration (Bensaid et al., 2016). These results imply that the reciprocal connections shared by PPN cholinergic and nigrostriatal DA neurons may be critical to their survival, and that simultaneous lesion alters cellular activity, potentially contributing to neurodegeneration. Accordingly, following nigrostriatal DA lesion, compensatory increases in activity occur in PPN neurons (Palombo et al., 1990; Carlson et al., 1999; Breit et al., 2001; Zhang et al., 2008). Initial or sustained hyperactivity of PPN glutamatergic or subthalamic nucleus glutamatergic neurons may accelerate nigrostriatal and PPN neurodegeneration and may be exacerbated by simultaneous loss of PPN cholinergic neurons. PPN hyperactivity may also arise due to loss of nigral DA and raphe serotonin afferents in PD, as PPN neurons contain the inhibitory DA D2R and 5HT1AR (Leonard and Llinás, 1994). Conversely, PPN hypoactivity (Jang et al., 2012) caused by increased SNr and GP inputs may lead to more severe PD symptoms (Nandi et al., 2002). Perhaps, PD heterogeneity could be partially explained by the order of cellular degeneration and by differential PPN activity. Future research should investigate the timing of neuronal loss, affected projections, and behaviors resulting from cell loss in these two lesion paradigms.
Although under-investigated, a recent study in preclinical models indicates that galanin may rescue PPN cholinergic neurons in PD. Following SNc lactacystin lesion, galanin is upregulated in rostral PPN cholinergic neurons (Elson et al., 2018). Galanin inhibits cholinergic transmission and may be neuroprotective, as it guards basal forebrain cholinergic neurons from amyloid beta toxicity in Alzheimer’s disease (Ding et al., 2006; Pirondi et al., 2010; Elliot-Hunt et al., 2011). In PD, galanin could reduce the likelihood of apoptosis in response to Lewy bodies or neuroinflammation (however, see also, Crawley et al., 2002).
The role of the PPN in generating locomotion remains controversial; however, early studies suggest PPN involvement in gait, an assertion that has been partially supported and further refined based on recent studies. Early research shows that the PPN and cuneiform nucleus make up the mesencephalic locomotor region (MLR), a functional neurocircuit modulating movement, rhythm, and speed upon electrical stimulation (Garcia-Rill et al., 1987; Garcia-Rill and Skinner, 1988; see Figure 1A for PPN projections related to PD motor symptoms). MLR projections to the lateral paragigantocellular nucleus (LPGi) modulate activity of motorneurons and ventral spinal laminae “central pattern generators” that regulate patterns of locomotor activity (Grillner and Wallen, 1985). In support of the PPN’s role in gait, fMRI studies demonstrate that PPN activity increases during fast imagined walking in healthy individuals (Bakker et al., 2008; Karachi et al., 2010); however, whether the PPN exerts its effects through ascending or descending pathways in actual fast walking is unknown. Although both PPN glutamatergic and cholinergic neurons may exert effects through LGPi efferents, recent data from preclinical models show that these neurons play different roles in locomotion. Based on these studies, glutamatergic neurons control flexor muscles to stop locomotion and are involved in slow-paced movement and exploratory behavior (Caggiano et al., 2018; Josset et al., 2018), whereas cholinergic neurons control extensor muscles and prolong the stance phase of locomotion and can also increase speed (Roseberry et al., 2016).
Ascending PPN efferents are also well-positioned to influence movement, although most existing data on this subject stem from research in preclinical models. Most importantly, the PPN projects to thalamic nuclei, which guide movements via thalamospinal projections. Additionally, although less direct, PPN glutamatergic and cholinergic inputs to SNc and striatum may affect both direct and indirect pathways. Relatedly, PPN cholinergic terminals in SNc drive locomotion (Xiao et al., 2016), whereas the motor outcomes of PPN afferents to striatum remain enigmatic, but are likely modulatory in nature (Dautan et al., 2016; Assous and Tepper, 2019). Finally, PPN cholinergic and GABAergic inputs to SNr exert influence over the nigrostriatal pathway. There, PPN ACh inhibits MSN terminals via M4R, inhibiting movement (Moehle et al., 2017), and PPN GABAergic projections improve motor learning through thalamic disinhibition (Li and Spitzer, 2019). Though PPN neurons likely contribute to normal gait, the role of neurons in the basal forebrain and cuneiform nucleus should also be carefully considered (Xiang et al., 2013; Sarter et al., 2014).
Nearly 20%–60% of PD patients experience gait dysfunction including freezing of gait (FOG) that is not consistently improved by DA replacement therapy (Giladi et al., 2001). FOG and falls are more common in PD patients exhibiting decreased ACh metabolism and reuptake in the thalamus (Bohnen et al., 2009; Bohnen and Albin, 2011; Bohnen et al., 2019), where the PPN is the main source of ACh. Cell death and synaptic inhibition from the GPi and SNr likely contribute to this decrease in cholinergic tone. In animal models, chemogenetic activation of PPN ACh neurons rescues motor deficits, indicating that cholinergic neurons remain which may be suitable therapeutic targets in PD (Pienaar et al., 2015). Similarly, cholinesterase inhibitors decrease falls in some PD patients (Chung et al., 2010).
Given its role in locomotion, the PPN has been considered as a site for deep brain stimulation (DBS) to improve otherwise intractable postural instability, FOG, and falling in PD and atypical Parkinsonism. Results of this approach have been varied. Multiple studies demonstrate that PPN-DBS can ameliorate FOG or general gait parameters in PD patients (Pereira et al., 2008; Ferraye et al., 2010; Khan et al., 2011; Thevathasan et al., 2011; Khan et al., 2012a; Khan et al., 2012b; Mazzone et al., 2014; Welter et al., 2015; Mestre et al., 2016). Although unilateral (Moro et al., 2010) and bilateral (Ferraye et al., 2010) approaches decrease falls, double-blind studies indicate the superiority of bilateral PPN stimulation for improving PD-related gait symptoms (Thevathasan et al., 2010; Thevathasan et al., 2012). Conversely, postural instability is not consistently improved with PPN-DBS (Moro et al., 2010; Thevathasan et al., 2010). Despite symptomatic improvements in some studies, open questions remain on the clinical relevance of PPN-DBS, as it cannot eliminate PD gait symptoms, and exact mechanisms underlying PPN-DBS effects are unknown. Furthermore, side effects ranging from oscillopsia, paresthesia, and even worsening of FOG have occurred in some patients (Hamani et al., 2007).
Multiple factors explain inconsistent PPN-DBS clinical outcomes. First, electrode placements in the PPN of PD patients vary. MRI placements correlated with patient outcomes suggest bilateral lead placement in caudal PPN is most efficacious for gait improvement (Goetz et al., 2018; Khan et al., 2012b). Relatedly, preclinical data show that rostral PPN lead placement has deleterious effects on movement and that caudal PPN controls stepping behavior (Gut and Winn, 2015; Garcia-Rill, 1986; Garcia-Rill and Skinner, 1988; Garcia-Rill, 1991). Second, stimulation parameters for PPN-DBS differ from one study to another. Many paradigms employ frequencies within beta and gamma ranges, but some have also employed stimulation at very low frequencies. Finally, small sample sizes, differences in disease progression, and the heterogeneity of PD signs and symptoms likely contribute to differential results regarding PPN-DBS.
DA replacement therapy with L-DOPA is standard treatment for PD. However, chronic use results in debilitating abnormal involuntary movements termed L-DOPA-induced dyskinesia (LID) in up to 90% of PD patients (Ahlskog and Muenter, 2001). Severe LID can lead to hospitalization and reduced quality of life (Péchevis et al., 2005; Lyoo and Lee, 2011). Although the PPN is not well investigated for its role in L-DOPA’s motor efficacy and in LID, existing evidence from patients undergoing PPN-DBS and preclinical evidence on the PPN’s involvement in motor stereotypy suggest that the PPN may contribute.
For instance, PD patients decrease their L-DOPA dose following long-term treatment with PPN-DBS (Mazzone et al., 2014), suggesting that PPN-DBS has pro-motor effects and may potentiate L-DOPA’s motor efficacy. Prior combination of PPN-DBS with L-DOPA shows additional motor benefit beyond L-DOPA alone (Jenkinson et al., 2008; Stefani et al., 2007). Additionally, combining caudal PPN-DBS with L-DOPA in preclinical models results in attenuation of LID (Gut and Winn, 2015). Similarly, immediate early genes associated with development and expression of LID are upregulated in the PPN of dyskinetic rodents (Bastide et al., 2014), implicating the PPN in LID expression.
The PPN has long been known to contribute to motor stereotypy in preclinical models; contextualizing this literature may provide useful insights into the role of the PPN in LID, as research on the PPN’s contribution to LID is sparse. PPN lesion produces orolingual stereotypy in response to d-amphetamine and apomorphine (Inglis et al., 1994), implying that PPN neurons suppress stereotyped movements in the normal brain. Furthermore, existing evidence shows that PPN GABAergic and cholinergic neurons have differing effects on motor stereotypy. PPN infusion of GABAergic antagonists reduces motor stereotypy produced by apomorphine and SNr microinfusion of muscimol (Childs and Gale, 1983), intimating that PPN GABA projections inhibit SNr neurons to promote motor stereotypy, or that SNr GABA projections to PPN promote motor stereotypy. Conversely, PPN infusion of mAChR antagonists increases motor stereotypy and exacerbates LID (Mathur et al., 1997; Chambers et al., 2019). Taken together, these results suggest that decreasing GABAergic tone or increasing cholinergic tone in the PPN may decrease LID, but this must be examined further. If true, this provides further support for hypoactivity of the PPN in PD. Future research should address specific contributions of PPN neuronal subtypes, and investigate the role of PPN efferents to the SNr and striatum.
The PPN is part of the reticular activating system (RAS), a group of nuclei regulating consciousness and attention. The PPN receives lateral hypothalamus orexin projections and SNr GABA projections that regulate muscle tone during sleep and wakefulness (Takakusaki et al., 2011, see Figure 1B). As mentioned previously, SNr and GPi GABA transmission to PPN is enhanced in PD. Importantly, when GABA B receptors are bound, REM sleep is inhibited (Sakai and Koyama, 1996; Ulloor et al., 2004; Datta, 2007). This alone may account for a significant portion of REM sleep problems in PD.
PPN cholinergic neurons promote EEG desynchronization and changes in state of consciousness via thalamic projections which regulate cortical activity (Hallanger et al., 1987; Steriade et al., 1990; Kobayashi et al., 2002; Mena-Segovia et al., 2008; Kotagal et al., 2012). PPN cholinergic neurons are active during REM sleep and wakefulness, manifesting alpha activity at rest and gamma oscillations related to behavior during waking (Steriade et al., 1991; Steriade et al., 1996; Kezunovic et al., 2011). During REM sleep, cholinergic neurons promote muscle atonia through projections to the subcoeruleus dorsalis (Baghdoyan et al., 1984; Sanford et al., 1994), and nucleus reticularis gigantocellularis neurons which project to motorneurons (Takakusaki et al., 2011). Cholinergic neurons promote REM and pontogeniculooccipital waves characteristic of REM sleep via dorsolateral geniculate nucleus and frontal eye field efferents (Sakai et al., 1976; Callaway et al., 1987; Shouse and Siegel, 1992; Rye, 1997). During slow wave sleep cholinergic neurons also promote nested gamma oscillations associated with memory replay and neuronal plasticity in cortex and hippocampus (Lee and Wilson, 2002).
Sleep disturbances resulting in excessive daytime sleepiness occur in 98% of PD patients (for review see Comella, 2007). While prevalence of PD-related sleep disorders alone is concerning, sleep dysfunction, particularly REM sleep behavior disorder (RBD), is linked to increased risk of cognitive impairment in PD patients (Marion et al., 2008; Gagnon et al., 2009). RBD is present in an estimated 50% of PD patients and is characterized by lack of muscle atonia during REM sleep which leads to patients engaging in complex motor behaviors during sleep. Intriguingly, diagnosis with idiopathic RBD often precedes PD and other synucleinopathies and may represent a prodromal phase of these illnesses (Boeve et al., 2001).
PPN ACh and substance-P-expressing neurons may contribute to the pathogenesis of RBD. Despite similar age of onset and disease duration, PD patients with RBD show decreased cholinergic transmission in cortex and thalamus relative to PD patients without RBD (Kotagal et al., 2012; however see also Bedard et al., 2019). Similarly, AChE inhibitors decrease symptoms of RBD, meaning that RBD may arise due to loss of cholinergic tone (Ringman and Simmons, 2000). Substance P is co-expressed in 27% of caudal PPN ACh neurons and is co-released onto the pontoreticular formation, where it has additive effects beyond those of ACh on initiation and maintenance of sleep (Kohlmeier et al., 2002). Substance P neurons degenerate in PD, which likely contributes to REM sleep atonia in PD patients. Congruent with PPN involvement in sleep dysfunction, PPN-DBS increases sleep efficiency, REM and stage 2 sleep, and decreases awakenings in PD patients (Romigi et al., 2008). Although understudied, these findings demonstrate that PPN-DBS could be used in the future to improve sleep for PD patients.
Interpreting PPN-DBS outcomes is impeded by small sample sizes, differences in electrode placement, stimulation paradigms, and disease progression. Additionally, PPN-DBS paradigms employ open-loop DBS which does not consider the situation and/or symptoms the patient is experiencing, nor brain activity in other areas which influence PD symptoms. Employing closed-loop PPN-DBS may be more effective (Rosin et al., 2011; Hebb et al., 2014; Sun and Morrell, 2014), as different stimulation frequencies have varying effects on PD symptoms. Using machine learning strategies, clinicians could correlate specific patterns of EEG readout with problems with FOG or other symptoms, and then use this information to promote more efficacious PPN-DBS. Future research should also address effects of exercise on the PPN, as recent data show that similar to hippocampal and basal forebrain cholinergic neurons (Molteni et al., 2008; Hall and Savage, 2016), exercise may induce PPN neuroplasticity beneficial for motor learning (Li and Spitzer, 2018). Finally, gene-therapy strategies focusing on neuroprotection through galanin or modulating L-type calcium channels should be considered, as well as gene therapy strategies that target specific PPN projections for symptomatic relief (see Table 1).
Overall, through innervation of ascending and descending motor structures, RAS, and cortex, the PPN is well-positioned to modify PD motor and sleep symptoms. However, to develop effective PPN-centered treatment strategies, there is a need for cell-specific manipulations in the PPN. Currently, this is challenging, as the contribution of specific PPN pathways to gait and RBD symptoms, and pathways that govern PPN’s modulation of L-DOPA’s effects are not well understood. Additionally, receptor types specific to each PPN neuron and how they change in health and disease are not well-defined. Therefore, future research should elucidate the contribution of specific PPN afferent and efferent connections to behavior and identify receptors exclusive to each type of PPN neuron. Additionally, as PD is a heterogeneous disorder, it is especially important that the therapeutic strategies employed are custom-tailored to the patient based on his or her symptoms.
Contributed to writing of the manuscript: NC, CB Created figures: KL.
American Parkinson’s Disease Association Research.
The authors declare that the research was conducted in the absence of any commercial or financial relationships that could be construed as a potential conflict of interest.
Ahlskog, J. E., Muenter, M. D. (2001). Frequency of levodopa-related dyskinesias and motor fluctuations as estimated from the cumulative literature. Movement Disord. 16, 448–458. doi: 10.1002/mds1090
Assous, M., Tepper, J. M. (2019). Excitatory extrinsic afferents to striatal interneurons and interactions with striatal microcircuitry. Eur. J. Neurosci. 49 (5), 593–603. doi: 10.1111/ejn.13881
Austin, M., Rice, P., Mann, J., Arango, V. (1995). Localization of corticotropin-releasing hormone in the human locus coeruleus and pedunculopontine tegmental nucleus: an immunocytochemical and in situ hybridization study. Neuroscience 64, 713–727. doi: 10.1016/0306-4522(94)00420-A
Baghdoyan, H. A., Rodrigo-Angulo, M. L., McCarley, R. W., Hobson, J. A. (1984). Site-specific enhancement and suppression of desynchronized sleep signs following cholinergic stimulation of three brainstem regions. Brain Res. 306, 39–52. doi: 10.1016/0006-8993(84)90354-8
Bakker, M., De Lange, F. P., Helmich, R. C., Scheeringa, R., Bloem, B. R., Toni, I. (2008). Cerebral correlates of motor imagery of normal and precision gait. NeuroImage 41, 998–1010. doi: 10.1016/j.neuroimage.2008.03.020
Bastide, M. F., Dovero, S., Charron, G., Porras, G., Gross, C. E., Fernagut, P.-O., et al. (2014). Immediate-early gene expression in structures outside the basal ganglia is associated to L-DOPA-induced dyskinesia. Neurobiol. Dis. 62, 179–192. doi: 10.1016/j.nbd.2013.09.020
Bedard, M.-A., Aghourian, M., Legault-Denis, C., Postuma, R. B., Soucy, J. P., Gagnon, J.-F., et al. (2019). Brain alterations in idiopathic REM sleep behavior disorder: a PET imaging study with 18F-FEOBV. Sleep Med. 58, 35–41. doi: 10.1016/j.sleep.2018.12.020
Bensaid, M., Michel, P. P., Clark, S. D., Hirsch, E. C., François, C. (2016). Role of pedunculopontine cholinergic neurons in Parkinson’s disease. Exp. Neurol. 275, 209–219. doi: 10.1016/j.expneurol.2015.11.004
Boeve, B. F., Silber, M. H., Ferman, T. J., Lucas, J. A., Parisi, J. E. (2001). Association of REM sleep behavior disorder and neurodegenerative disease may reflect an underlying synucleinopathy. Mov. Disord. 16, 622–630. doi: 10.1002/mds1120
Bohnen, N. I., Albin, R. L. (2011). The cholinergic system and Parkinson disease. Behav. Brain Res. 221 (2), 564–573. doi: 10.1016/j.bbr.2009.12.048
Bohnen, N. I., Frey, K. A., Studenski, S., Kotagal, V., Koeppe, R. A., Scott, P. J., et al. (2013). Gait speed in Parkinson disease correlates with cholinergic degeneration. Neurology 81 (18), 1611–1616. doi: 10.1212/WNL.0b013e3182a9f558
Bohnen, N. I., Kanel, P., Zhou, Z., Koeppe, R. A., Frey, K. A., Dauer, W. T., et al. (2019). Cholinergic system changes of falls and freezing of gait in Parkinson’s disease. Ann. Neurol. 85 (4), 538–549. doi: 10.1002/ana.25430
Bohnen, N. I., Muller, M. L., Koeppe, R. A., Studenski, S. A., Kilbourn, M. A., Frey, K. A., et al (2009). History of falls in Parkinson’s disease is associated with reduced cholinergic activity. Neurology 73, 1670–1676. doi: 10.1212/WNL.0b013e3181c1ded6
Brazhnik, E., McCoy, A. J., Novikov, N., Hatch, C. E., Walters, J. R. (2016). Ventral medial thalamic nucleus promotes synchronization of increased high beta oscillatory activity in the basal ganglia—thalamocortical network of the hemiparkinsonian rat. J. Neursci. 361, 4196–4208. doi: 10.1523/JNEUROSCI.3582-152016
Breit, S., Bouali-Benazzouz, R., Benabid, A.-L., Benazzouz, A. (2001). Unilateral lesion of the nigrostriatal pathway induces an increase of neuronal activity of the pedunculopontine nucleus, which is reversed by the lesion of the subthalamic nucleus in the rat. Eur. J. Neurosci. 14, 1833–1842. doi: 10.1046/j.0953-816x.2001.01800.x
Bury, A. G., Pyle, A., Elson, J. L., Greaves, L., Morris, C. M., Hudson, G., et al. (2017). Mitochondrial DNA changes in pedunculopontine cholinergic neurons in Parkinson disease. Ann. Neurol. 82 (6), 1016–1021. doi: 10.1002/ana.25099
Caggiano, V., Leiras, R., Goni-Erro, H., Masini, D., Bellardita, C., Bouvier, J., et al. (2018). Midbrain circuits that set locomotor speed and gait selection. Nature 553, 455–460. doi: 10.1038/nature25448
Cai, J., Lee, S., Ba, F., Garg, S., Kim, L. J., Liu, A., et al. (2018). Galvanic vestibular stimulation (GVS) augments deficient pedunculopontine nucleus connectivity in mild Parkinson’s disease: fMRI effects of different stimuli. Front. Neurosci. 101, 1–13. doi: 10.3389/fnins.2018.00101
Callaway, C. W., Lydic, R., Baghdoyan, H. A., Hobson, J. A. (1987). Pontogeniculooccipital waves: spontaneous visual system activity during rapid eye movement sleep. Cell. Mol. Neurobiol. 7, 105–149. doi: 10.1007/BF00711551
Carlson, J. D., Pearlsteinb, R. D., Buchholza, J., Iaconoc, R. P., Maedaa, G. (1999). Regional metabolic changes in the pedunculopontine nucleus of unilateral 6-hydroxydopamine Parkinson’s model rats. Brain Res. 828 (1-2), 12–19. doi: 10.1016/S0006-8993(99)01268-8
Chambers, N. E., Meadows, S. M., Taylor, A., Sheena, E., Lanza, K., Conti, M. M., et al (2019). Effects of muscarinic acetylcholine m1 and m4 receptor blockade on dyskinesia in the hemi-parkinsonian rat. Neurosci 409, 180–194. doi: 10.1016/j.neuroscience.2019.04.008
Childs, J. A., Gale, K. (1983). Evidence that the nigrostriatal GABAergic projection mediates stereotypy induced by apomorphine and intranigral muscimol. Life Sciences 33, 1007–1010. doi: 10.1016/0024-3205(83)90758-0
Chung, K. A., Lobb, B. M., Nutt, J. G., Horak, F. B. (2010). Effects of a central cholinesterase inhibitor on reducing falls in Parkinson disease. Neurology 75, 1263–1269. doi: 10.1212/WNL.0b013e3181f6128c
Comella, C. L. (2007). Sleep disorders in Parkinson’s disease. Movement Disord. 22, S367–S373. doi: 10.1002/mds.21682
Crawley, J. N., Mufson, E. J., Hohmann, J. G., Teklemichael, D., Steiner, R. A., Holmbergy, K., et al. (2002). Galanin overexpressing transgenic mice. Neuropeptides 36, 145–156. doi: 10.1054/npep.20020891
D’Onofrio, S., Kezunovic, N., Hyde, J. R., Luster, B., Messias, E., Urbano, F. J., et al. (2015). Modulation of gamma oscillations in the pedunculopontine nucleus by neuronal calcium sensor protein-1: Relevance to schizophrenia and bipolar disorder. J. Neurophysiol. 113, 709–719. doi: 10.1152/jn.008282014
Datta, S. (2007). Activation of pedunculopontine tegmental PKA prevents GABAB receptor activation-mediated rapid eye movement sleep suppression in the freely moving rat. J. Neurophysiol. 97, 3841–3850. doi: 10.1152/jn.00263.2007
Dautan, D., Bay, H. H., Bolam, J. P., Gerdjikov, T. V., Mena-Segovia, J. (2016). Extrinsic innervation of the striatal complex: a whole-brain mapping analysis. Front. Neuroanat. 10 (1), 1–10. doi: 10.3389/fnana.2016.00001
Ding, X., MacTavish, D., Kar, S., Jhamandas, J. H. (2006). Galanin attenuates beta-amyloid (Abeta) toxicity in rat cholinergic basal forebrain neurons. Neurobiol. Dis. 21, 413–420. doi: 10.1016/j.nbd.2005.08.016
Elliot-Hunt, C. R., Holmes, F. E., Hartley, D. M., Perez, S., Mufson, E. J., Wynick, D. (2011). Endogenous galanin protects mouse hippocampal neurons against amyloid beta toxicity in vitro via activation of galanin receptor-2. J. Alzheimers Dis. 25, 455–452. doi: 10.3233/JAD-2011-110011
Elson, J. L., Yates, A., Pienaar, I. S. (2016). Pedunculopontine cell loss and protein aggregation direct microglia activation in parkinsonian rats. Brain Struct. Funct. 221, 2319–2341. doi: 10.1007/s00429-015-1045-4
Elson, J. L., Kochaj, R., Reynolds, R., Pienaar, I. S. (2018). Temporal-spatial profiling of pedunculopontine galanin-cholinergic neurons in the lactacystin rat model of Parkinson’s disease. Neurotoxic. Res. 34 (1), 16–31. doi: 10.1007/s12640-017-9846-2
Ferraye, M. U., Debû, B., Fraix, V., Goetz, L., Ardouin, C., Yelnik, J., et al. (2010). Effects of pedunculopontine nucleus area stimulation on gait disorders in Parkinson’s disease. Brain 133 (1), 205–214. doi: 10.1093/brain/awp229
Gagnon, J. F., Vendette, M., Postuma, R. B., Desjardins, C., Massicotte-Marquez, J., Panisset, M., et al. (2009). Mild cognitive impairment in rapid eye movement sleep behavior disorder and Parkinson’s disease. Ann. Neurol. 66, 39–47. doi: 10.1002/ana.21680
Garcia-Rill, E., Skinner, R. D. (1988). Modulation of rhythmic function in the posterior midbrain. Neurosciences 17, 639–654. doi: 10.1016/0306-4522(88)90295-3
Garcia-Rill, E. (1986). The basal ganglia and the locomotor regions. Brain Res. Rev. 11, 47–63. doi: 10.1016/0165-0173(86)90009-3
Garcia-Rill, E., Houser, C. R., Skinner, R. D., Smith, W., Woodward, D. J. (1987). Locomotion-inducing sites in the vicinity of the pedunculopontine nucleus. Brain Res. Bull. 18, 731– 738. doi: 10.1016/0361-9230(87)90208-5
Garcia-Rill, E. (1991). The pedunculopontine nucleus. Prog. Neurobiol. 36, 363–389. doi: 10.1016/0301-0082(91)90016-T
Giladi, N., Treves, T. A., Simon, E. S., Shabtai, H., Orlov, Y., Kandinov, B., et al. (2001). Freezing of gait in patients with advanced Parkinson’s disease. J. Neural Transm. 108, 53–61. doi: 10.1007/s007020170096
Goetz, L., Bhattacharjee, M., Ferraye, M. U., Fraix, V., Maineri, C., Nosko, D., et al. (2018). Deep brain stimulation of the pedunculopontine nucleus area in Parkinson disease: MRI-based anatomoclinical correlations and optimal target. Neurosurgery 84, 506–518. doi: 10.1093/neuros/nyy151
Gomez-Gallego, M., Fernandez-Villalba, E., Fernandez-Barreiro, A., Herrero, M. T. (2006). Changes in neuronal activity in the pedunculopontine nucleus in chronic MPTP-treated primates: an in situ hybridization study of cytochrome oxidase subunit I, choline acetyl transferase and substance P mRNA expression. J. Neural Transm. 114, 319–326. doi: 10.1007/s00702-006-0547-x
Grillner, S., Wallen, P. (1985). Central pattern generators for locomotion, with special reference to vertebrates. Annu. Rev. Neurosci. 8 (1), 233–261. doi: 10.1146/annurev.ne.08.030185.001313
Gut, N. K., Winn, P. (2015). Deep brain stimulation of different pedunculopontine targets in a novel rodent model of parkinsonism. J. Neurosci. 35, 4792–4803. doi: 10.1523/JNEUROSCI.3646-142015
Hall, J. M., Savage, L. M. (2016). Exercise leads to the re-emergence of the cholinergic/nestin neuronal phenotype within the medial septum/diagonal band and subsequent rescue of both hippocampal ACh efflux and spatial behavior. Exp. Neurol. 278, 62–75. doi: 10.1016/j.expneurol.2016.01.018
Hallanger, A. E., Levey, A. I., Lee, H. J., Rye, D. B., Wainer, B. H. (1987). The origins of cholinergic and other subcortical afferents to the thalamus in the rat. J. Comp. Neurol. 262 (1), 105–124. doi: 10.1002/cne.902620109
Hamani, C., Stone, S., Laxton, A., Lozano, A. M. (2007). The pedunculopontine nucleus and movement disorders: Anatomy and the role for deep brain stimulation. Parkinsonism Relat. Disord. 13, S276–S280. doi: 10.1007/s00702-010-0547-8
Hebb, A. O., Zhang, J. J., Mahoor, M. H., Tsiokos, C., Matlack, C., Chizeck, H. J., et al. (2014). Creating the feedback loop: Closed-loop neurostimulation. Neurosurg. Clin. N. Am. 25, 187–204. doi: 10.1016/j.nec.2013.08.006
Hirsch, E. C., Graybiel, A. M., Duyckaerts, C., Javoy-Agid, F. (1987). Neuronal loss in the pedunculopontine tegmental nucleus in Parkinson disease and in progressive supranuclear palsy. Proc. Natl. Acad. Sci. 84 (16), 5976–5980. doi: 10.1073/pnas.84.165976
Inglis, W. L., Allen, F., Whitelaw, R. B., Latimer, M. P., Brace, H. M., Winn, P. (1994). An investigation into the role of the pedunculopontine tegmental nucleus in the mediation of locomotion and orofacial stereotypy induced by d-amphetamine and apomorphine in the rat. Neuroscience 58, 817–833. doi: 10.1016/0306-4522(94)90459-6
Inkster, L. M., Eng, J. J. (2004). Postural control during a sit-to-stand task in individuals with mild Parkinson’s disease. Exp. Brain Res. 154 (1), 33–38. doi: 10.1007/s00221-003-1629-8
Jang, D. P., Min, H.-K., Lee, S.-Y., Kim, I. Y., Park, H. W., Im, Y. H., et al. (2012). Functional neuroimaging of the 6-OHDA lesion rat model of Parkinson’s disease. Neurosci. Lett. 513 (2), 187–192. doi: 10.1016/j.neulet.2012.02.034
Jellinger, K. (1988). The pedunculopontine nucleus in Parkinson’s disease, progressive supranuclear palsy and Alzheimer’s disease. J. Neurol. Neurosurg. Psychiatry 51 (4), 540–543. doi: 10.1136/jnnp.51.4.540
Jenkinson, N., Nandi, D., Oram, R., Stein, J. F., Aziz, T. Z. (2008). Pedunculopontine nucleus electric stimulation alleviates akinesia independently of dopaminergic mechanisms. Neuroreport 17 (6), 639–641. doi: 10.1097/00001756-200604240-00016
Josset, N., Roussel, M., Lemieux, M., Lafrance-Zoubga, D., Rastqar, A., Bretzner, F. (2018). Distinct contributions of mesencephalic locomotor region nuclei to locomotor control in the freely behaving mouse. Curr. Biol. 28, 884–901. doi: 10.1016/j.cub.2018.02.007
Karachi, C., Grabli, D., Bernard, F. A., Tandé, D., Wattiez, N., Belaid, H., et al. (2010). Cholinergic mesencephalic neurons are involved in gait and postural disorders in Parkinson disease. J. Clin. Invest. 120 (8), 2745–2754. doi: 10.1172/JCI42642
Kezunovic, N., Urbano, F. J., Simon, C., Hyde, J., Smith, K., Garcia-Rill, E. (2011). Mechanism behind gamma band activity in the pedunculopontine nucleus (PPN). Eur. J. Neurosci. 34, 404–415. doi: 10.1111/j.1460-9568.2011.07766.x
Khan, S., Mooney, L., Plaha, P., Javed, S., White, P., Whone, A. L., et al. (2011). Outcomes from stimulation of the caudal zona incerta and pedunculopontine nucleus in patients with Parkinson’s disease. Br. J. Neurosurg. 25 (2), 273–280. doi: 10.3109/02688697.2010.544790
Khan, S., Gill, S. S., Mooney, L., White, P., Whone, A., Brooks, D. J., et al. (2012a). Combined pedunculopontine-subthalamic stimulation in Parkinson disease. Neurology 78, 1090–1095. doi: 10.1212/WNL.0b013e31824e8e96
Khan, S., Javed, S., Mooney, L., White, P., Plaha, P., Whone, A., et al. (2012b). Clinical outcomes from bilateral versus unilateral stimulation of the pedunculopontine nucleus with and without concomitant caudal zona incerta region stimulation in Parkinson’s disease. Br. J. Neurosurg. 26, 722–725. doi: 10.3109/02688697.2012.659297
Kobayashi, Y., Inoue, Y., Yamamoto, M., Isa, T., Aizawa, H. (2002). Contribution of pedunculopontine tegmental nucleus neurons to performance of visually guided saccade task in monkeys. J Neurophysiol. 88, 715–731. doi: 10.1152/jn.2002.88.2.715
Kohlmeier, K. A., Burns, J., Reiner, P. B., Semba, K. (2002). Substance P in the descending cholinergic projection to REM sleep-induction regions of the rat pontine reticular formation: anatomical and electrophysiological analyses. Eur. J. Neurosci. 15, 176–196. doi: 10.1046/j.0953-816x.2001.01829.x
Kotagal, V., Albin, R. L., Müller, M. L., Koeppe, R. A., Chervin, R. D., Frey, K. A., et al. (2012). Symptoms of rapid eye movement sleep behavior disorder are associated with cholinergic denervation in Parkinson disease. Ann. Neurol. 71, 560–568. doi: 10.1002/ana.22691
Lavoie, B., Parent, A. (1994). Pedunculopontine nucleus in the squirrel monkey: cholinergic and glutamatergic projections to the substantia nigra. J. Comp. Neurol. 344 (2), 232–241. doi: 10.1002/cne.903440205
Lee, A. K., Wilson, M. A. (2002). Memory of sequential experience in the hippocampus during slow wave sleep. Neuron 36, 1183–1194. doi: 10.1016/S0896-6273(02)01096-6
Leonard, C. S., Llinás, R. (1994). Serotonergic and cholinergic inhibition of mesopontine cholinergic neurons controlling REM sleep: an in vitro electrophysiological study. Neuroscience 59, 309–330. doi: 10.1016/0306-4522(94)90599-1
Li, H.-Q., Spitzer, N. C. (2018). Exercise enhances motor skill learning by neurotransmitter switching in the adult midbrain. bioRxiv. 1–34. doi: 10.1101/613539
Lyoo, H. C., Lee, M. S. (2011). Rhabdomyolysis induced by severe levodopa induced dyskinesia in a patient with Parkinson’s disease. J. Neurol. 258, 1893–1894. doi: 10.1007/s00415-011-6041-x
MacLaren, D. A., Ljungberg, T. L., Griffin, M. E., Clark, S. D. (2018). Pedunculopontine tegmentum cholinergic loss leads to a progressive decline in motor abilities and neuropathological changes resembling progressive supranuclear palsy. Eur. J. Neurosci. 48 (12), 3477–3497. doi: 10.1111/ejn.14212
Marion, M. H., Qurashi, M., Marshall, G., Foster, O. (2008). Is REM sleep behavior disorder (RBD) a risk factor of dementia in idiopathic Parkinson’s disease? J. Neurol. 255, 192–196. doi: 10.1007/s00415-008-0629-9
Martinez-Gonzalez, C., Wang, H. L., Micklem, B. R., Bolam, J. P., Mena-Segovia, J. (2012). Subpopulations of cholinergic, GABAergic and glutamatergic neurons in the pedunculopontine nucleus contain calcium-binding proteins and are heterogeneously distributed. Eur. J. Neurosci. 35, 723–734. doi: 10.1111/j.1460-9568.2012.08002.x
Martinez-Gonzalez, C., van Andel, J., Bolam, J. P., Mena-Segovia, J. (2014). Divergent motor projections from the pedunculopontine nucleus are differentially regulated in Parkinsonism. Brain Struct. Funct. 219, 1415–1462. doi: 10.1007/s00429-013-0579-6
Mathur, A., Shandarin, A., LaViolette, S. R., Parker, J., Yeomans, J. S. (1997). Locomotion and stereotypy induced by scopolamine: Contributions of muscarinic receptors near the pedunculopontine tegmental nucleus. Brain Res. 775, 144–155. doi: 10.1016/S0006-8993(97)00928-1
Mazzone, P., Paoloni, M., Mangone, M., Santilli, V., Insola, A., Fini, M., et al. (2014). Unilateral deep brain stimulation of the pedunculopontine tegmental nucleus in idiopathic Parkinson’s disease: effects on gait initiation and performance. Gait Posture 40 (3), 357–362. doi: 10.1016/j.gaitpost.2014.05.002
Mena-Segovia, J., Sims, H. M., Magill, P. J., Bolam, J. P. (2008). Cholinergic brainstem neurons modulate cortical gamma activity during slow oscillations. J. Physiol. 586, 2947–2960. doi: 10.1113/jphysiol.2008.153874
Mestre, T. A., Sidiropoulos, C., Hamani, C., Poon, Y. Y., Lozano, A. M., Lang, A. E., et al. (2016). Long-term double-blinded unilateral pedunculopontine area stimulation in Parkinson’s disease. Movement Disord. 31 (10), 1570–1574. doi: 10.1002/mds.26710
Mineff, E. M., Popratiloff, A., Romansky, R., Kazakos, V., Kaimaktschieff, V., Usunoff, K. G., et al. (1998). Evidence for a possible glycinergic inhibitory neurotransmission in the midbrain and rostral pons of the rat studied by gethyrin. Arch. Physiol. Biochem. 106 (3), 210–220. doi: 10.1076/apab.106.3.2104377
Moehle, M. S., Pancani, T., Byun, N., Yohn, S. E., Wilson, G. H., III, Dickerson, J. W., et al. (2017). Cholinergic projections to the substantia nigra pars reticulata inhibit dopamine modulation of basal ganglia through the M4 muscarinic receptor. Neuron 96 (6), 1358–1372. doi: 10.1016/j.neuron.2017.12.008
Molteni, R., Ying, Z., Gomez-Pinilla, F. (2008). Differential effects of acute and chronic exercise on plasticity-related genes in the rat hippocampus revealed by microarray. Eur. J. Neurosci. 16, 1107–1116. doi: 10.1046/j.1460-9568.2002.02158.x
Moro, E., Hamani, C., Poo, Y.-Y, Al-Khairallah, T., Dostrovsky, O., et al. (2010). Unilateral pedunculopontine stimulation improves falls in Parkinson’s disease. Brain 133 (1), 215–224. doi: 10.1093/brain/awp261
Nandi, D., Aziz, T. Z., Giladi, N., Winter, J., Stein, J. F. (2002). Reversal of akinesia in experimental parkinsonism by GABA antagonist microinjections in the pedunculopontine nucleus. Brain 125 (11), 2418–2430. doi: 10.1093/brain/awf259
Okada, K.-I., Kobayashi, Y. (2009). Characterization of oculomotor and visual activities in the primate pedunculopontine tegmental nucleus during visually guided saccade tasks. Eur. J. Neurosci. 30, 2211–2223. doi: 10.1111/j.1460-9568.2009.07009
Pahapil, P. A., Lozano, A. M. (2000). The pedunculopontine nucleus and Parkinson’s disease. Brain 123, 1767–1783. doi: 10.1093/brain/123.9.1767
Palombo, E., Porrino, L. J., Bakiewicz, K. S., Crane, A. M., Sokoloff, L., Kopin, L. J. (1990). Local cerebral glucose utilization in monkeys with hemiparkinsonism induced by intracaratoid infusion of the neurotoxin MPTP. J. Neurosci. 10 (3), 860–869. doi: 10.1523/JNEUROSCI.10-03-008601990
Péchevis, M., Clarke, C. E., Vieregge, P., Khoshnood, B., Deschaseaux-Voinet, C., Berdeaux, G., et al. (2005). Effects of dyskinesias in Parkinson’s disease on quality of life and health-related costs: A prospective European study. Eur. J. Neurol. 12, 956–963. doi: 10.1111/j.1468-1331.2005.01096.x
Pereira, E. A., Muthusamy, K. A., De Pennington, N., Joint, C. A., Aziz, T. Z. (2008). Deep brain stimulation of the pedunculopontine nucleus in Parkinson’s disease. Preliminary experience at Oxford. Br. J. Neurosurg. 22 (sup1), S41–S44. doi: 10.1080/02688690802448335
Pienaar, I. S., van de Berg, W. (2013). A non-cholinergic neuronal loss in the pedunculopontine nucleus of toxin-evoked Parkinsonian rats. Exp. Neurol. 248, 213–223. doi: 10.1016/j.expneurol.2013.06.008
Pienaar, I. S., Gartside, S. E., Sharma, P., De Paola, V., Gretenkord, S., Withers, D., et al. (2015). Pharmacogenetic stimulation of cholinergic pedunculopontine neurons reverses motor deficits in a rat model of Parkinson’s disease. Mol. Neurodegener. 10, 1–22. doi: 10.1186/s13024-015-0044-5
Pirondi, S., Giuliani, A., Del, V. G., Giardino, L., Hokfelt, T., Calza, L. (2010). The galanin receptor 2/3 agonist Gal2-11 protects the SN56 cells against beta-amyloid 25–35 toxicity. J. Neurosci. Res. 88 (5), 1064–1073. doi: 10.1002/jnr.22278
Redgrave, P., Mitchell, I. J., Dean, P. (1987). Descending projections from the superior colliculus in rat: a study using orthograde transport of wheatgerm-agglutinin conjugated horseradish peroxidase. Exp. Brain Res. 68, 147–167. doi: 10.1007/BF00255241
Ringman, J. M., Simmons, J. H. (2000). Treatment of REM sleep behavior disorder with donepezil: a report of three cases. Neurology 55 (6), 870–871. doi: 10.1212/WNL.55.6.870
Rinne, J. O., Ma, S. Y., Lee, M. S., Collan, Y., Röyttä, M. (2008). Loss of cholinergic neurons in the pedunculopontine nucleus in Parkinson’s disease is related to disability of the patients. Parkinson. Relat. Disord. 14 (7), 553–557. doi: 10.1016/j.parkreldis.2008.01.006
Romigi, A., Placidi, F., Peppe, A., Pierantozzi, M., Izzi, F., Brusa, L., et al (2008). Pedunculopontine nucleus stimulation influences REM sleep in Parkinson’s disease. Eur. J. Neurol. 15, e64–e65. doi: 10.1111/j.1468-1331.2008.02167.x
Roseberry, T. K., Lee, A. M., Lalive, A. L., Wilbrecht, L., Bonci, A., Kreitzer, A. C. (2016). Cell-type-specific control of brainstem locomotor circuits by basal ganglia. Cell 164 (3), 526–537. doi: 10.1016/j.cell.2015.12.037
Rosin, B., Slovik, M., Mitelman, R., Rivlin Etzion, M., Haber, S., Israel, Z., et al. (2011). Closed-loop deep brain stimulation is superior in ameliorating parkinsonism. Neuron 72, 370–384. doi: 10.1016/j.neuron.2011.08.023
Rye, D. B., Lee, H. J., Saper, C. B., Wainer, B. H. (1988). Medullary and spinal efferents of the pedunculopontine tegmental nucleus and adjacent mesopontine tegmentum in the rat. J. Comp. Neurol. 269, 315–341. doi: 10.1002/cne.902690302
Rye, D. B. (1997). Contributions of the pedunculopontine region to normal and altered REM sleep. Sleep 20, 757–788. doi: 10.1093/sleep/20.9.757
Sakai, K., Koyama, Y. (1996). Are there cholinergic and non-cholinergic paradoxical sleep-on neurons in the pons. Neuroreport 7, 2449–2453. doi: 10.1097/00001756-199611040-00009
Sakai, K., Petitjean, F., Jouvet, M. (1976). Effects of ponto-mesencephalic lesions and electrical stimulation upon PGO waves and EMPs in unanesthetized cats. Electroencephalogr. Clin. Neruophysiol. 41, 49–63. doi: 10.1016/0013-4694(76)90214-5
Sanford, L. D., Morrison, A. R., Mann, G. I., Harris, J. S., Yoo, L., Ross, R. J. (1994). Sleep patterning and behavior in cats with pontine lesions creating REM without atonia. J. Sleep Res. 527, 213–223. doi: 10.1111/j.1365-2869.1994.tb00136.x
Sarter, M., Albin, R. L., Kucinski, A., Lustig, C. (2014). Where attention falls: increased risk of falls from the converging impact of cortical cholinergic and midbrain dopamine loss on striatal function. Exp. Neurol. 257, 120–129. doi: 10.1016/j.expneurol.2014.04.032
Shouse, M., Siegel, J. M. (1992). Pontine regulation of REM sleep components in cats: Integrity of the pedunculopontine tegmentum (PPT) is important for phasic events but unnecessary for atonia during REM sleep. Brain Res 571, 50–63. doi: 10.1016/0006-8993(92)90508-7
Simon, C., Kezunovic, N., Ye, M., Hyde, J., Hayar, A., Williams, D. K., et al. (2010). Gamma band unit activity and population responses in the pedunculopontine nucleus. J. Neurophys. 104, 463–474. doi: 10.1152/jn.002422010
Spann, B. M., Grofova, I. (1989). Origin of ascending and spinal pathways from the nucleus tegmenti pedunculopontinus in the rat. J. Comp. Neurol. 283, 13–27. doi: 10.1002/cne.902830103
Standaert, D., Saper, C., Rye, D., Wainer, B. (1986). Colocalization of atriopeptin-like immunoreactivity with choline acetyltransferase- and substance—P-like immunoreactivity in the pedunculopontine and laterodorsal tegmental nuclei in the rat. Neuroscience 382, 163–168. doi: 10.1016/0006-8993(86)90126-5
Stefani, A., Lozano, A. M., Peppe, A., Stanzione, P., Galati, S., Tropepi, D., et al. (2007). Bilateral deep brain stimulation of the pedunculopontine and subthalamic nuclei in severe Parkinson’s disease. Brain 130 (6), 1596–1607. doi: 10.1093/brain/awl346
Steriade, M., Datta, S., Paré, D., Oakson, G., Dossi, C. (1990). Neuronal activities in brain-stem cholinergic nuclei related to tonic activation processes in thalamocortical systems. J. Neurosci. 10, 2541–2559. doi: 10.1523/JNEUROSCI.10-08-025411990
Steriade, M., Dossi, R. C., Paré, D., Oakson, G. (1991). Fast oscillations (20-40 Hz) in thalamocortical systems and their potentiation by mesopontine cholinergic nuclei in the cat. PNAS 88, 4396–4400. doi: 10.1073/pnas.88.104396
Steriade, M., Amzica, F., Contreras, D. (1996). Synchronization of Fast (30-40 Hz) spontaneous cortical rhythms during brain activation. J. Neurosci. 16, 392–417. doi: 10.1523/JNEUROSCI.16-01-003921996
Sun, F. T., Morrell, M. J. (2014). Closed-loop neurostimulation: the clinical experience. Neurotherapeutics 11, 553–563. doi: 10.1007/s13311-014-0280-3
Surmeier, J. (2018). Etiology of Parkinson"s disease: Cells and circuits (San Diego, CA: Presentation at Neuroscience of Movement Disorders).
Takakusaki, K., Obara, K., Nozu, T., Okumura, T. (2011). Modulatory effects of the GABAergic basal ganglia neurons on the PPN and the muscle tone inhibitory system in cats. Arch. Italiennes Biol. 149, 383–405. doi: 10.4449/aib.v149i4.1383
Thevathasan, W., Silburn, P. A., Brooker, H., Coyne, T. J., Khan, S., Gill, S. S., et al. (2010). The impact of low-frequency stimulation of the pedunculopontine nucleus region on reaction time in parkinsonism. J. Neurol. Neurosurg. Psychiatry 81 (10), 1099–1104. doi: 10.1136/jnnp.2009.189324
Thevathasan, W., Coyne, T. J., Hyam, J. A., Kerr, G., Jenkinson, N., Aziz, T. Z., et al. (2011). Pedunculopontine nucleus stimulation improves gait freezing in Parkinson disease. Neurosurgery 69 (6), 1248–1254. doi: 10.1227/NEU.0b013e31822b6f71
Thevathasan, W., Cole, M. H., Graepel, C. L., Hyam, J. A., Jenkinson, N., Brittain, J. S., et al. (2012). A spatiotemporal analysis of gait freezing and the impact of pedunculopontine nucleus stimulation. Brain 135 (5), 1446–1454. doi: 10.1093/brain/aws039
Ulloor, J., Mavanji, V., Saha, S., Siwek, D. F., Datta, S. (2004). Spontaneous REM sleepis modulated by the activation of the pedunculopontine tegmental GABAB receptors in the freely moving rat. J. Neurophysiol. 91, 1822–1831. doi: 10.1152/jn.01104.2003
Vincent, S. R., Satoh, K., Armstrong, D. M., Fibiger, H. C. (1983). Substance P in the ascending cholinergic reticular system. Nature 306, 688–691. doi: 10.1038/306688a0
Vitale, C., Marcelli, V., Furia, T., Santangelo, G., Cozzolino, A., Longo, K., et al. (2011). Vestibular impairment and adaptive postural imbalance in Parkinsonian patients with lateral trunk flexion. Mov. Disord. 26, 1458–1463. doi: 10.1002/mds.23657
Wallace, M. N., Fredens, K. (1988). Origin of high acetylcholinesterase activity in the mouse superior colliculus. Exp. Brain Res. 72, 335–346. doi: 10.1007/BF00250255
Wang, H. I., Morales, M. (2009). Pedunculopontine and laterodorsal tegmental nuclei contain distinct populations of cholinergic, glutamatergic, and GABAergic neurons in the rat. Eur. J. Neurosci. 29, 340–358. doi: 10.1111/j.1460-9568.2008.06576.x
Welter, M. L., Demain, A., Ewenczyk, C., Czernecki, V., Lau, B., El Helou, A., et al. (2015). PPNa-DBS for gait and balance disorders in Parkinson’s disease: a double-blind, randomised study. J. Neurol. 262 (6), 1515–1525. doi: 10.1007/s00415-015-7744-1
Xiang, H.-B., Zhu, W.-Z., Guan, X.-H, Ye, D. W. (2013). The cuneiform nucleus may be involved in the regulation of skeletal muscle tone by motor pathway: A virally mediated trans-synaptic tracing study in surgically sympathectomized mice. Brain 136, 1–4. doi: 10.1093/brain/awt123
Xiao, C., Cho, J. R., Zhou, C., Treweek, J. B., Chan, K., McKinney, S. L., et al. (2016). Cholinergic mesopontine signals govern locomotion and reward through dissociable midbrain pathways. Neuron 90, 333–347. doi: 10.1016/j.neuron.2016.03.028
Zhang, Q. J., Liu, J., Wang, Y., Wang, S., Wu, Z. H., Yan, W., et al. (2008). The firing activity of presumed cholinergic and non-cholinergic neurons of the pedunculopontine nucleus in 6-hydroxydopamine-lesioned rats: An in vivo electrophysiological study. Brain Res. 1243, 152–160. doi: 10.1016/j.brainres.2008.09.028
Keywords: Parkinson’s disease, pedunculopontine nucleus, neurodegeneration, gait deficits, non-motor symptoms, REM sleep behavior disorder, pharmacology
Citation: Chambers NE, Lanza K and Bishop C (2020) Pedunculopontine Nucleus Degeneration Contributes to Both Motor and Non-Motor Symptoms of Parkinson’s Disease. Front. Pharmacol. 10:1494. doi: 10.3389/fphar.2019.01494
Received: 21 July 2019; Accepted: 19 November 2019;
Published: 15 January 2020.
Edited by:
Cristina Miguelez, University of the Basque Country, SpainCopyright © 2020 Chambers, Lanza and Bishop. This is an open-access article distributed under the terms of the Creative Commons Attribution License (CC BY). The use, distribution or reproduction in other forums is permitted, provided the original author(s) and the copyright owner(s) are credited and that the original publication in this journal is cited, in accordance with accepted academic practice. No use, distribution or reproduction is permitted which does not comply with these terms.
*Correspondence: Christopher Bishop, Y2Jpc2hvcEBiaW5naGFtdG9uLmVkdQ==
Disclaimer: All claims expressed in this article are solely those of the authors and do not necessarily represent those of their affiliated organizations, or those of the publisher, the editors and the reviewers. Any product that may be evaluated in this article or claim that may be made by its manufacturer is not guaranteed or endorsed by the publisher.
Research integrity at Frontiers
Learn more about the work of our research integrity team to safeguard the quality of each article we publish.