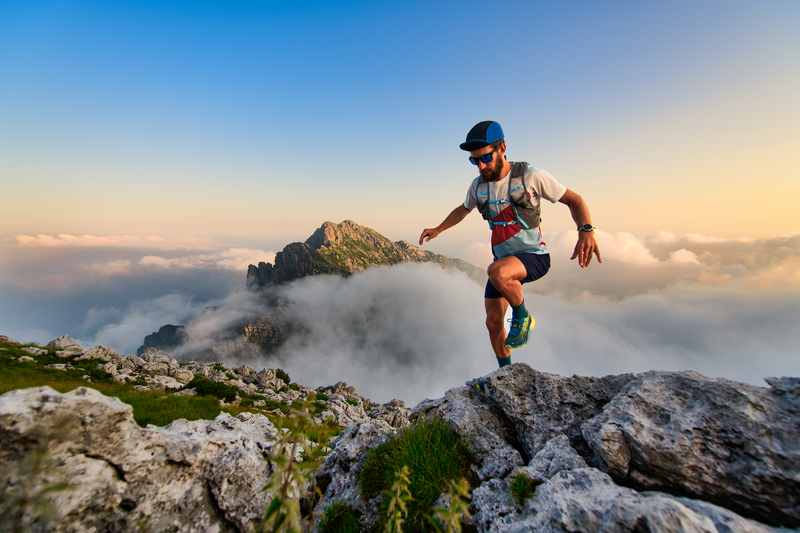
95% of researchers rate our articles as excellent or good
Learn more about the work of our research integrity team to safeguard the quality of each article we publish.
Find out more
ORIGINAL RESEARCH article
Front. Pharmacol. , 29 March 2019
Sec. Neuropharmacology
Volume 10 - 2019 | https://doi.org/10.3389/fphar.2019.00309
This article is part of the Research Topic New Paradigms in Neuroscience and Related Targets for Drug Discovery, Volume I View all 21 articles
Gene-targeted mice with deficient AMPA receptor GluA1 subunits (Gria1-/- mice) show robust hyperlocomotion in a novel environment, suggesting them to constitute a model for hyperactivity disorders such as mania, schizophrenia and attention deficit hyperactivity disorder. This behavioral alteration has been associated with increased neuronal activation in the hippocampus, and it can be attenuated by chronic treatment with antimanic drugs, such as lithium, valproic acid, and lamotrigine. Now we found that systemic cannabidiol strongly blunted the hyperactivity and the hippocampal c-Fos expression of the Gria1-/- mice, while not affecting the wild-type littermate controls. Acute bilateral intra-dorsal hippocampal infusion of cannabidiol partially blocked the hyperactivity of the Gria1-/- mice, but had no effect on wild-types. The activation of the inhibitory DREADD receptor hM4Gi in the dorsal hippocampus by clozapine-N-oxide robustly inhibited the hyperactivity of the Gria1-/- mice, but had no effect on the locomotion of wild-type mice. Our results show that enhanced neuronal excitability in the hippocampus is associated with pronounced novelty-induced hyperactivity of GluA1 subunit-deficient mice. When this enhanced response of hippocampal neurons to novel stimuli is specifically reduced in the hippocampus by pharmacological treatment or by chemogenetic inhibition, Gria1-/- mice recover from behavioral hyperactivity, suggesting a hippocampal dysfunction in hyperactive behaviors that can be treated with cannabidiol.
Neurological and psychiatric brain diseases cause vast harm and excessive costs to affected individuals and the society at large (DiLuca and Olesen, 2014). There are many clinical evidence-based pharmacological and non-pharmacological treatment options (Millan et al., 2015a,b), but their efficacy should be greatly improved. Unfortunately, there has been a slow progress in neuropsychiatric drug development, especially for schizophrenia, schizoaffective disorder and bipolar disorder, justifying further efforts in finding testable mechanisms, drug candidates and novel targets (Vazquez et al., 2017).
Animal models play an important role in testing mechanisms how neuronal modulations and drug effects are mediated into behavioral responses (Cryan and Slattery, 2007; Forrest et al., 2014), even if they cannot reproduce a full repertoire of symptoms in often heterogeneous brain diseases. Especially difficult are the models of psychosis, since in animals the behavioral outcomes are usually related to motor functions as they are most easily quantifiable. In addition to lithium therapy, presently there are no specific drugs for the treatment of bipolar disorder with mania (Millan et al., 2015b). A simple symptom in that illness is the mania-like hyperactivity, which can be habituated in a constant environment. A mouse line with deficient AMPA-type glutamate receptor GluA1 subunits [the Gria1-/- mouse line (Zamanillo et al., 1999)] has been proposed as an animal model for hyperactive disorders, including schizoaffective disorder and bipolar disorder with mania (Fitzgerald et al., 2010; Barkus et al., 2011; Procaccini et al., 2011). This is based on (1) robust novelty-induced hyperactivity that is eventually habituated, leading to unaltered diurnal home-cage activity in the Gria1-/- mice (Vekovischeva et al., 2001; Fitzgerald et al., 2010; Sanderson et al., 2010; Procaccini et al., 2011), (2) consistent attenuation of this behavior by chronic treatment with drugs having antimanic efficacy in patients (Maksimovic et al., 2014a,b), and (3) linkage equilibrium of GRIA1-gene polymorphisms in psychotic disorders (Ripke et al., 2013, 2014; Devor et al., 2017).
The basic characteristics of Gria1-/- mice do not deviate from Gria1+/+ wild-type littermates (WT). Physical health, body weight, food consumption, nociception, neurological, motor, sexual, sensory functions, and circadian rhythm appear normal (Vekovischeva et al., 2001, 2004; Bannerman et al., 2004; Hartmann et al., 2004; Feyder et al., 2007; Chourbaji et al., 2008; Fitzgerald et al., 2010; Procaccini et al., 2011). Extensive behavioral comparison of Gria1-/- and Gria1+/+ mice suggests a schizophrenia- and depressive-like phenotype. Deficiency in prepulse inhibition of an acoustic startle reflex is indicative of psychosis-related properties (Wiedholz et al., 2008) and increased learned helplessness as deficits in coping skills in aversive situations indicative of depressive phenotype of Gria1-/- mice (Chourbaji et al., 2008). Short term spatial working memory of Gria1-/- mice is disrupted (Reisel et al., 2002; Sanderson et al., 2009). However, despite the prominent role of GluA1-type AMPA receptors in hippocampal synaptic plasticity Gria1-/- mice can form spatial reference memory (Zamanillo et al., 1999).
The most striking and reproducible behavioral response of Gria1-/- mice has been hyperactivity provoked by a novel environment. They have normal locomotion compared to their WT littermates in a familiar home-cage environment (Wiedholz et al., 2008; Procaccini et al., 2011), but when transferred to novel environment, they double their locomotor activity (Vekovischeva et al., 2001; Bannerman et al., 2004; Chourbaji et al., 2008; Fitzgerald et al., 2010; Procaccini et al., 2011) and fail to habituate (Barkus et al., 2011; Sanderson and Bannerman, 2012).
This increased activity is sustained, and it takes 30 to 40 min before the Gria1-/- mice show signs of habituation to the novelty. Thus, any confrontation with a novel signal, such as a new object or littermate, induce an aberrant reaction in Gria1-/- mice due to the lack of habituation (Wiedholz et al., 2008). Similarly, in sociability and resident-intruder tests Gria1-/- mice show remarkably low level of intermale aggression, suggesting poor ability to adapt to social encounters (Vekovischeva et al., 2004).
We have recently found that Gria1-/- mice react to novel environment by increased neuronal activation of the hippocampi, as shown by the enhanced immediate early gene expression (Procaccini et al., 2011). In the present study, we used Gria1-/- mice to analyze the effect of systemic application and acute hippocampal infusion of the non-psychoactive phytocannabinoid cannabidiol (CBD) (Izzo et al., 2009) on the hyperactivity and hippocampal c-Fos expression in Gria1-/- and controls. CBD has been shown potential as treatment for schizophrenia (Schubart et al., 2014). We also tested whether selective inhibition of the dorsal hippocampal principal neurons is sufficient to down-regulate the hyperactivity by using the activation of the virus-mediated, cell-type specific expression of an inhibitory designer receptors exclusively activated by designer drug (DREADD) (Roth, 2016), the hM4Gi receptor (Armbruster et al., 2007).
In our experiments, both the CBD and DREADD approaches attenuated the behavioral response to novelty of Gria1-/- mice, indicating that the paradoxical hippocampal activation is associated with hyperactivity of this mouse model. Importantly, acute infusion of CBD specifically into the hippocampus reduced the abnormal hyperactivity of the Gria1-/- knockout mice but did not affect the spontaneous activity of the wild-type littermates.
Gria1-/- mice (Gria1-/-, Gria1tm1Rsp; MGI:2178057) and their Gria1+/+ wild-type littermate controls (WT) were from heterozygous breeding, generated by inactivation of the Gria1 gene (Zamanillo et al., 1999) and genotyped as described (Vekovischeva et al., 2001). The Gria1-/- mouse line is available at the Jackson Laboratory (B6N.129-Gria1tm1Rsp/J, stock number: 019011). Mice were group-housed under standard laboratory conditions (12-h light-dark cycle; lights on at 6:00 A.M.; temperature 20–23°C; relative humidity 50–60%; aspen chip beddings). For locomotor activity, a total of 24 Gria1-/- (14.12 ± 0.92 weeks; 24.58 ± 1.03 g) and 23 WT mice (11.14 ± 0.66 weeks; 24.63 ± 0.92 g) were used.
All experimental procedures were approved by the State Provincial Government of Southern Finland (ESAVI-0010026/041003/2010). All efforts were made to minimize the number and suffering of animals.
Locomotor activity in a novel environment after acute systemic cannabidiol treatments was observed in plastic cages (40 cm × 30 cm × 20 cm) as described in detail (Procaccini et al., 2011; Maksimovic et al., 2014b). At least 1 h before the tests was allowed for the animals to habituate to the experimental room. Horizontal movements of eight mice, placed in visually isolated cages in a sound-attenuated room at the light intensity of 175 lx, were simultaneously recorded for 2 h using EthoVision Color-Pro 3.0 video tracking software (Noldus Information Technology, Wageningen, Netherlands). The same method, but only during 30-min recordings, was used to assess the effects of intrahippocampal CBD infusions and chemogenetic inhibition of hippocampal neurons (see below).
The animals were quickly decapitated after 2-h novelty-exploration, the brains dissected, frozen on dry ice and stored at -80°C. Fourteen-μm thick sections were cut on a cryostat (Leica CM 3050 S; Leica Microsystem, Nußloch, Germany), thaw-mounted on Fisher Superfrost Plus slides (Menzel-Glaeser, Braunschweig, Germany) and stored at -80°C. To obtain sections of the ventral hippocampi, we changed the plane of cutting to horizontal one after we had collected the coronal sections until Bregma -2.4 mm (Franklin and Paxinos, 2008) as described earlier (Procaccini et al., 2013; Maksimovic et al., 2014b).
In immunohistochemistry, the protocol described in Procaccini et al. (2011) was followed. In brief, the sections were thawed, air-dried, marked with hydrophobic pen (Daido Sangyo, Tokyo, Japan) and all incubations performed using so-called liquid bubble technique. The sections were fixed with ice-cold 4% paraformaldehyde in Tris-buffered saline (TBS; in mM: Tris, 50; NaCl, 150; pH 7.4) for 10 min. As a washing medium, we used TBS supplemented with 0.05% Tween 20 (TBST) between incubations. Endogenous peroxidases were blocked by 0.3% H2O2 in methanol. Endogenous proteins were blocked with 10% normal horse serum (Sigma-Aldrich) and avidin blocking solution (Avidin/Biotin blocking kit; Vector Laboratories, Burlingame, CA, United States) diluted in TBST containing 1% bovine serum albumin (BSA; Sigma-Aldrich). Sections were incubated with goat anti-c-Fos antibody (1:1000; Santa Cruz Biotechnology, Santa Cruz, CA, United States) in TBST/1% BSA and biotin blocking solution (Avidin/Biotin blocking kit) overnight at 4°C, followed by 30 min in biotinylated horse anti-goat secondary antibody (1:200; Vector Laboratories). Avidin–biotin peroxidase complex (Vectastain Standard Elite; Vector Laboratories) and diaminobenzidine with nickel sulfate intensification (DAB Substrate kit; Vector Laboratories) were used for visualization. Then, the sections were dehydrated in gradual ethanol solutions (70, 96, and 99.5%), rinsed in Histoclear (National Diagnostic, Atlanta, GA, United States) and finally coverslipped with DPX mounting medium (BHD Chemicals, Poole, United Kingdom).
In quantifying the c-Fos-positive cells, photomicrographs from anatomically-matched sections were captured using a light microscope with a 10× objective (Leica DMR, Leica Microsystems, Wetzlar, Germany) and a CCD camera (Leica DC 300). The analysis was carried out blind to the treatment and genotype. Detection of c-Fos-positive cells was automatic, using ImageJ software (National Institutes of Health, Bethesda, MD, United States), by setting a constant threshold and ‘region of interest’ area based on brain atlas (Franklin and Paxinos, 2008). Analysis comprised bilaterally-obtained values from one or two sections (for dorsal and ventral hippocampi) of 12 brain areas (as shown in Figure 2 and Supplementary Table S1). Apart from the hippocampus, expression of c-Fos protein was studied in those regions that may have a role in explorative locomotor task or where relatively high c-Fos expression is observed (Montag-Sallaz et al., 1999).
The hippocampus was targeted with CBD injections by microinjection cannulae. Mice were anesthetized with isoflurane (induction 5%, 2% maintenance; Vetflurane, Virbac, Carros, France), and implanted with bilateral 26-gauge stainless steel guide cannulae (Plastics One, Roanoke, VA, United States) into the dorsal dentate gyrus using a stereotactic device (David Kopf Instruments, Tujunga, CA, United States). Dental cement (Simplex Rapid, Associated Dental Products, Ltd., Swindon, United Kingdom) and two stainless steel screws were used to secure the cannula placement. The coordinates used were 1.9 mm posterior to bregma, 1.0 mm lateral to midline, and 1.0 mm ventral to skull level. Stainless steel obturators were inserted to keep cannulae unobstructed during the 1-week recovery period. Animals’ recovery was monitored daily and none showed signs of distress. Prior to experiments, the mice were gently handled and CBD was injected through the guide cannulae by using 31-gauge internal stainless-steel injectors protruding an additional 1.0 mm below the guide cannulae. The injectors were connected to a microsyringe (Hamilton, Bonaduz, Switzerland) via polyethylene tubing. CBD (5 μg/side) or vehicle was injected in a volume of 0.2 μL at 0.1 μL/min by an infusion pump (kDScientific, Holliston, MA, United States). After the injection, the injectors remained in place for 1 min to allow solutions to diffuse into the tissue. The measurement of novelty-induced locomotor activity was recorded immediately after the injections as described above. After the experiments, the injector tip placements were confirmed histologically.
Dorsal hippocampal dentate gyrus was stereotactically targeted in isoflurane anesthesia as described above for CBD infusions. To each mouse, a total volume of 0.15 μL of AAV5-CaMKIIα-hM4Gi-mCherry viral vector (3.4 × 1012 genome copies/mL, University of North Carolina, Gene Therapy Center, Chapel Hill, NC, United States) was injected through a 31-gauge injection cannula using a microsyringe and an injection pump as described above. The animals recovered for 3 weeks. Thirty minutes prior to behavioral experiments the animals were administered clozapine-N-oxide (CNO) at 3 mg/kg i.p. in home cage. Non-DREADD-transduced mice acted as controls to test for DREADD receptor-non-specific effects of CNO. The measurement of novelty-induced locomotor activity was recorded immediately after the injections. After the experiments, the animals were deeply anesthetized with pentobarbital (Mebunat, Orion Pharma, Espoo, Finland), transcardially perfused with 0.1 M phosphate-buffered saline (PBS) followed by 4% paraformaldehyde in PBS. Brains were removed, post-fixed overnight at 4°C, and cryoprotected in 30% sucrose in PBS at 4°C until sunk. Forty-μm-thick coronal sections were cut by cryostat (Leica CM 3050 S; Leica Microsystem, Nußloch, Germany). To visualize the DREADD receptors, mCherry tag immunohistochemistry was performed. In brief, brain sections were washed at RT in 0.1 M PBS, blocked with 1% BSA (Merck, Darmstadt, Germany) supplemented with 0.3% Triton X-100 (Fisher Scientific, Fair Lawn, NJ, United States) in 0.1 M PBS. Sections were then incubated overnight at RT in primary antibody (rabbit anti-mCherry, 1:800, ab167453, Abcam, Cambridge, United Kingdom) diluted in blocking buffer, washed in PBS, incubated in secondary antibody (donkey anti-rabbit AlexaFluor 594, 1:1000, ab150076, Abcam) for 2 h at RT, washed in PBS, and mounted on microscope slides and coverslipped. Digital images were captured with a Zeiss Axioimager Z1 microscope using Apotome optical sectioning (Zeiss, Oberkochen, Germany) and Hamamatsu Orca R2 CCD camera (Hamamatsu, Japan).
The doses of cannabidiol (CBD, from THC Pharm GmbH, Frankfurt, Germany) were based on literature (Zuardi et al., 1991; Moreira and Guimaraes, 2005; Long et al., 2006, 2010, 2012; Gururajan et al., 2011, 2012) and on preliminary dose–response experiments. The drug was dissolved in a mixture of ethanol:Tween 80:saline (1:1:18, which was used as a vehicle for controls) (Todd and Arnold, 2016) and injected (i.p.) in a volume of 10 ml/kg. For intrahippocampal injection, CBD was dissolved in 1% Tween 80 and 10% DMSO in sterile saline. Clozapine-N-oxide (CNO, Sequoia Research Products, Ltd., Pangbourne, United Kingdom) was dissolved in 0.5% DMSO in saline.
Data are expressed as means ± standard errors of the mean (SEM). Statistical analyses were carried out using IBM SPSS Statistics 21 software (IBM SPSS, Inc., Somers, NY, United States) using (repeated measures) two-way ANOVA (genotype, treatment) followed by a Bonferroni post hoc test. Statistical significance was set at P < 0.05.
In keeping with our previous results (Procaccini et al., 2013; Maksimovic et al., 2014b), the Gria1-/- mice showed robust hyperlocomotion as compared to the WT mice, when placed into an unfamiliar environment (Figures 1A,B, ANOVA for genotype effect, F1.49 = 33.09, P < 0.001). They gradually habituated over the observation period of 2 h (time effect F23,1127 = 56.89, P < 0.001), although not down to the average level of WT mice (time × genotype interaction F23,1127 = 2.23, P < 0.01), as determined by the comparison of the mean path length of the last three timebins (genotype effect, F1,10 = 6.48, P < 0.05). CBD treatment dose-dependently attenuated the novelty-induced hyperlocomotion of Gria1-/- mice (treatment effect, F4.49 = 4.16, P < 0.01; genotype effect, F1.49 = 33.09, P < 0.001), while the locomotor activity of WT mice remained unaltered (treatment × genotype interaction, F4.49 = 3.74, P < 0.01; Figure 1, Bonferroni post-test, vehicle vs. CBD, P > 0.05). Post hoc comparisons showed that all doses of CBD (15, 60, and 100 mg/kg), except for the lowest dose (5 mg/kg), brought the hyperactivity of Gria1-/- mice back to the level of WT mice (Figure 1C, locomotor activity analyzed from the beginning of the trial until 30 min, and Figure 1D, locomotor activity analyzed over the whole 2 h trial, Bonferroni post-test, P > 0.05 for the genotype difference within all doses, except for 5 mg/kg, when it was P < 0.001). The 5 mg/kg dose seemed to fail to rescue the hyperactivity of the Gria1-/- mice in the beginning of the trial, but to facilitate the habituation in the later stage of the trial (Figures 1A,B, time × genotype × treatment interaction F92,1127 = 1.85, P < 0.001).
Figure 1. Dose–response analysis of cannabidiol on novelty-induced hyperlocomotion of Gria1-/- mice. (A,B) Distance traveled in a novel environment in 5-min periods after treatment with vehicle and doses of cannabidiol (mg/kg, i.p.). (C) Distance traveled from the beginning of the trial until 30 min. (D) Total distance traveled during the whole 2 h test trial. Means ± SEMs are shown for 5–6 mice per group. ∗∗∗P < 0.001 for the significances of the differences between WT and Gria1-/- mice after vehicle treatment; #P < 0.05, ##P < 0.01, and ###P < 0.001 between vehicle and CBD within the same genotype (Bonferroni post-test). Veh, vehicle; CBD, cannabidiol.
As the lowest effective dose of cannabidiol to block the novelty-induced hyperactivity in Gria1-/- mice was 15 mg/kg (Figures 1A,B), we analyzed the brain regional c-Fos protein expression after this dose (Figure 2). In the dentate gyrus granule cell layer of the dorsal hippocampus, the number of c-Fos-positive cells of the vehicle-treated Gria1-/- mice was significantly increased compared to the number of c-Fos positive cells in WT mice (Figure 2A, genotype effect, F1,20 = 9.32, P < 0.01). The CBD treatment of WT mice did not alter the number of c-Fos positive cells in the dentate gyrus, but CBD normalized the number of c-Fos positive cells in that of Gria1-/- mice (Figure 2B, treatment effect F1,20 = 7.34, P < 0.05; genotype × treatment interaction F1,20 = 6.90, P < 0.05). However, in the CA1 subfield, CBD treatment decreased c-Fos-positive cell counts in both Gria1-/- and WT mice (treatment effect, F1,20 = 7.35, P < 0.05). The c-Fos cell count in CA3 was equal between the genotypes (F1,20 = 0.001, P > 0.05) and not affected by the CBD treatment (F1,20 = 0.12, P > 0.05).
Figure 2. Expression of c-Fos protein in hippocampal subregions of the Gria1-/- and WT mice after a 2-h exposure to a novel environment with pre-treatment with vehicle or cannabidiol (15 mg/kg). (A) Representative images of the dorsal hippocampus. (B) Counts of c-Fos+ cells in the subregions of the dorsal hippocampus. Open bars for the vehicle- (VEH) treated and closed bars for cannabidiol- (CBD) treated groups. (C) Representative images of the ventral hippocampus. (D) Counts of c-Fos+ cells in the subregions of the ventral hippocampus. Means ± SEMs are shown for 4–6 mice per group. ∗P < 0.5, ∗∗P < 0.01 for the significances of the differences between genotypes after the same treatment, #P < 0.05, ##P < 0.01 for the difference from the vehicle within the same genotype (Bonferroni post-test). Scale bar: 100 μm.
In the ventral hippocampus (Figures 2C,D), novelty-induced hyperactivity did not change the number of c-Fos positive cells in dentate gyrus subfield (genotype effect for DG, F1,19 = 0.24, P > 0.05). In the CA1 subfield, Gria1-/- mice had slightly higher number of c-Fos positive cells (genotype effect, F1,19 = 5.04, P < 0.05), but CBD treatment failed to reduce the cell count significantly (treatment effect F1,19 = 1.76, P > 0.05; genotype × treatment interaction F1,19 = 1.00, P > 0.05). In the CA3 subfield, novelty-induced hyperactivity did not change the number of c-Fos positive cells, although a non-significant trend was detected (genotype effect, F1,19 = 3.22, P > 0.05; genotype × treatment interaction, F1,19 = 4.13, P = 0.056).
The numbers of c-Fos-positive cells after treatment with CBD in extra-hippocampal brain regions are summarized in Supplementary Table S1. In the basolateral amygdala, CBD treatment reduced the number of c-Fos-positive cells in WT animals only (genotype × treatment interaction F1,20 = 4.74, P < 0.05). In the lateral septum, the number of c-Fos-positive cells increased in WT mice after CBD treatment (genotype × treatment interaction F1,19 = 8.54, P < 0.01). In the prelimbic cortex, the greater number of c-Fos-positive cells was observed in WT mice than in Gria1-/- mice, a difference that disappeared after CBD treatment (genotype × treatment interaction, F1,20 = 4.72, P < 0.05). Thus, compared to the hippocampus, extra-hippocampal brain areas of wild-type mice were hardly affected by the CBD. Only in the lateral septal nucleus of Gria1-/- mice the c-Fos response to CBD injection was opposite.
The c-Fos-based neuronal activation mapping revealed the hippocampal complex as a main locus of novelty-induced hyperactivity in Gria1-/- mice (Figure 2 and Procaccini et al., 2011). Because systemic CBD both decreased the novelty-induced hyperactivity and re-adjusted the c-Fos positive cell score in the hippocampal dentate gyrus of Gria1-/- mice (Figures 1, 2), we sought to determine whether infusion of CBD into the dorsal hippocampus is sufficient to down-regulate the hyperactivity. We found that the intrahippocampal CBD microinjection (Figure 3A) significantly decreased, but did not completely reverse, the novelty-induced hyperactivity of Gria1-/- mice (Figures 3B,C), while not changing locomotor activity of WT mice (genotype effect, F1,16 = 49.08, P < 0.001; genotype × treatment interaction, F1,16 = 9.39, P < 0.01), supporting our hypothesis that the attenuation of the hyperactivity by CBD of Gria1-/- mice is mainly mediated by its action in the hippocampus.
Figure 3. Intrahippocampal infusion of cannabidiol decreases novelty-induced hyperlocomotion of Gria1-/- mice. Animals were given an infusion with vehicle or cannabidiol (5 μg/side) followed by recording of distance moved for 30 min. (A) Injector tip placements within the dorsal hippocampus for all experimental groups. The brain images were modified with permission from Paxinos and Franklin (2001). (B) Distance traveled in a novel environment in 5-min periods. (C) Total distance traveled during 30-min trials. ∗∗P < 0.01 and ∗∗∗P < 0.001 for the significances of the differences between the genotypes after vehicle; #P < 0.05 between vehicle and CBD within the same genotype (Bonferroni post-test). N = 10 for both genotypes. Veh, vehicle; CBD, cannabidiol.
The c-Fos-based neuronal activation mapping has revealed the hippocampal complex as a main locus of novelty-induced overactivity in Gria1-/- mice (Figure 2 and Procaccini et al., 2011). We used the clozapine-N-oxide (CNO) activation of the Gi-DREADD receptors (hM4Gi; Armbruster et al., 2007) to inhibit neuronal activity in the dorsal hippocampus of Gria1-/- mice and to reveal the anatomical and cellular substrate for the hyperlocomotion phenotype of the Gria1-/- mice (Figures 4A,B). Gria1-/- mice that received these bilateral AAV5-CaMKIIα-hM4Gi-mCherry injections in the dorsal hippocampus, CNO-induced DREADD inhibition of hippocampal CaMKIIα-expressing, excitatory cells decreased hyperlocomotion of Gria1-/- mice to the level of WT littermates (Figures 4C,D). In the WTs, CNO-induced DREADD inhibition in the same cell population had no modifying effect on the locomotor activity (treatment effect, F1,16 = 5.61, P < 0.05; genotype effect, F1,16 = 12.11, P < 0.01; genotype × treatment interaction, F1,16 = 10.27, P < 0.01). The effect persisted until the Gria1-/- mice habituated to the locomotor activity level of the WT mice (Figure 4C, time effect, F5,80 = 9.63, P < 0.001; time × genotype interaction, F5,80 = 7.46, P < 0.001; time × genotype × treatment interaction, F5,80 = 3.26, P < 0.01). In contrast, in DREADD-non-expressing mice, the drug CNO did not change the locomotor activity of either genotype (treatment effect, F1,17 = 0.47, P > 0.05; treatment × genotype interaction, F1,17 = 0.49, P > 0.05) and the Gria1-/- mice displayed hyperlocomotion (genotype effect, F1,17 = 15.61, P < 0.001) irrespective of the CNO treatment (Figures 4E,F).
Figure 4. Chemogenetic inhibition of novelty-induced hyperlocomotion in Gria1-/- mice. (A) Virus injection resulted in hM4Gi-mCherry expression in the dorsal hippocampus. Hb, habenula; fi, fimbria; scale bar: 1 mm. (B) A close-up photograph displaying transduced neurons and neurites in the dentate gyrus. No transduced neurites were observed outside the hippocampus (not shown). Gr, granule cell layer; Mol, molecular layer; scale bar: 50 μm. (C) Distance traveled in a novel environment in 5-min periods in mice transduced with DREADDs. (D) Total distance traveled during the 30-min trial in mice transduced with DREADDs. ∗∗P < 0.01 and ∗∗∗P < 0.001 for the significances of the differences between the genotypes after vehicle; #P < 0.05 and ##P < 0.01 between vehicle and CNO treatments within the same genotype (Bonferroni post-test), n = 10 for both genotypes. (E) Distance traveled in a novel environment in 5-min periods in non-DREADD expressing WT and Gria1-/- control mice. ∗∗∗P < 0.001 for the significances within drug treatment and between genotypes (ANOVA). (F) Total distance traveled during the 30-min trial in non-DREADD-expressing control mice. ∗P < 0.05 between genotypes and within drug treatment (Bonferroni post-test). n = 11–14. Veh, vehicle; CNO, clozapine-N-oxide.
Increased novelty-induced hyperactivity has been the most consistent and robust behavioral phenotypic alteration in mice with deficient AMPA-type glutamate receptor GluA1 subunits (for review see, Sanderson et al., 2008). In the present study, Gria1-/- mice again reproduced the hyperactive phenotype, also after stresses of the surgeries and intracerebral infusions, indicating that the model is stable for these kinds of experimental scrutiny.
The hippocampus is considered a most important brain area for spatial learning and adaptation (Bannerman et al., 2014; Moser et al., 2017). Inhibition of hippocampal principal neurons by DREADD receptors after CNO strongly blocked the novelty-induced hyperactivity in Gria1-/- mice, without affecting the locomotor activity of wild-type mice, indicating that the hippocampus is abnormally activated in the mutants during extensive exploration of novel environments. Such an increased hippocampal activity of Gria1-/- mice can be visualized by increased numbers of c-Fos expressing cells during exposure to unfamiliar surroundings [the present study (Procaccini et al., 2011)]. Both acute and chronic drug treatments have reduced both hippocampal c-Fos expression and behavioral hyperactivity (Procaccini et al., 2013; Maksimovic et al., 2014a,b).
Our data, using hippocampus specific Gi-DREADD and hippocampal infusion of CBD indicate that the hippocampal dysfunction is responsible for the impaired habituation of GluA1 deficient mice to new environments or situations. This view finds support by an inducible hippocampal deletion of GluA1 subunits from principal neurons during late adolescence which phenocopied the hyperactivity as seen in mice with global reduction of GluA1 (Inta et al., 2014). Since the DREADD system targeted mainly the dentate gyrus granule cells but was detected also in cornu ammonis regions (Figure 4A), it is likely that the DREADD-driven inhibition recruited more extensively the hippocampal formation leading to complete normalization of the novelty-induced hyperactivity in Gria1-/- mice. Similarly, the restoration of GluA1 in the hippocampus had attenuates the open field hyperactivity of Gria1-/- mice (Freudenberg et al., 2016). In our critical control experiment (MacLaren et al., 2016), the drug used to activate the DREADD receptors, clozapine-N-oxide (CNO) at the dose of 3 mg/kg, did not alter locomotor activities of the mouse lines without the DREADD expression (Figures 4E,F). This result is in line with the recent report that showed only higher than 5 mg/kg doses of CNO having partial clozapine-like effects in mice (Manvich et al., 2018).
Although hyperactivity and responses to novelty are often linked to increased dopaminergic mechanisms (Boekhoudt et al., 2016) and the Gria1-/- mice have been suggested to show hyperdopaminergic phenotype (Wiedholz et al., 2008), our previous experiments failed to find differential c-Fos expression between the Gria1-/- and WTs in the midbrain and striatal dopaminergic regions after exposure to a novel environment (Procaccini et al., 2011) and the hyperactive, hippocampally restricted GluA1 subunit-deficient mice had normal striatal dopamine levels (Inta et al., 2014). However, pharmacological antagonism of dopamine D2 receptors has been shown to non-selectively reduce hyperactivity in both Gria1-/- and WT mice (Wiedholz et al., 2008), making it difficult to fully exclude the role of putatively enhanced dopamine mechanisms in the Gria1-/- mice. Furthermore, recent neuronal pathway modulation on regulation of habituation and novelty preference in mice has indicated important interactive roles of the medial habenula and interpeduncular nucleus with the VTA, with the habenula activating the interpeduncular nucleus to recognize familiarity and the VTA inhibiting the interpeduncular nucleus to promote novelty seeking (Molas et al., 2017).
Despite that the dopaminergic dysfunction has a recognized role in schizoaffective disorders and might play a role in Gria1-/- mice, there is evidence rather pointing to indirect effects of cannabinoids on dopamine cell firing, by altering glutamate neurotransmission (Colizzi et al., 2016). The Gria1-/- mouse model has been sensitive to drugs that downregulate glutamate neurotransmission including several antiepileptic drugs and AMPA antagonists (Procaccini et al., 2013; Maksimovic et al., 2014a,b). CBD shows little target selectivity and has multiple actions on various receptors and ion channels (Izzo et al., 2009; Ibeas Bih et al., 2015). While our data on attenuation of locomotor hyperactivity by silencing glutamatergic neurons via Gi-coupled DREADDs could imply the involvement of the inhibitory CB1 receptors, the inhibition of the presynaptic glutamate release via CBD action on the cannabinoid receptors have been largely dismissed (Ibeas Bih et al., 2015). One of the most interesting and relevant CBD actions is the inhibition of the G protein-coupled receptor 55 (GPR55). Hippocampally expressed G-protein-coupled receptor 55 (GPR55) induces Ca2+ release from presynaptic stores leading to increased synaptic neurotransmitter release (Sylantyev et al., 2013; Hurst et al., 2017). As GPR55 colocalizes presynaptically with the vesicular glutamate transporter 1 (Sylantyev et al., 2013), glutamate is the likely neurotransmitter for the GPR55-induced increase of excitatory transmitter release. Importantly, CBD inhibits GPR55-mediated excitatory drive in hippocampal synapses (Sylantyev et al., 2013), which may suggest a pharmacological significance in damping overactivated network within the hippocampal circuits (Kaplan et al., 2017). While a direct measurement of glutamate release in response to novelty-induced hyperactivity in Gria1-/- mice needs to be established, previous data suggest a role for glutamate release in novel environments (Bianchi et al., 2003). Therefore, the GPR55-regulated glutamate release provides a possible mechanism for the CBD-mediated downregulation of hippocampal excitatory activity and behavioral hyperactivity in Gria1-/- mice.
In addition to the activation of GPR55, CBD appears also to possess agonistic properties at serotonergic Gi-coupled 5-HT1A receptors, through which it can induce anxiolytic-like effects and mediate adaptation to stress (Joca et al., 2003; Russo et al., 2005; Rock et al., 2012). These receptors are localized at presynaptic terminals of glutamatergic synapses and their activation suppresses glutamatergic signaling (Cai et al., 2002). Thus, CBD actions on 5-HT1A receptors might have contributed to the decrease in hippocampal neuronal overactivation in Gria1-/- mice.
The intrahippocampal CBD injection partially rescued the novelty-induced hyperactive phenotype in the Gria1-/- mice. Similar doses as in our study (bilaterally 5 μg/side) have been previously injected into the brain parenchyma, although not into the hippocampus. A 10-fold lower dose of CBD (0.4 μg/side) facilitated fear extinction, when injected bilaterally to rat infralimbic cortex (Do Monte et al., 2013). A higher unilateral periaqueductal CBD dose of 10 μg was required to produce an anxiolytic-like effect in rats, while 20 μg dose failed to affect the behavioral output (Campos and Guimaraes, 2008). In a summary, these studies suggest that bilateral 5 μg/side dose of CBD reaches appropriate concentration intrahippocampally to have an effect on various hippocampally expressed CBD targets, although the affinity of CBD to them might vary (Ibeas Bih et al., 2015).
Overall, our data suggest that silencing hippocampal glutamatergic neurons and systemic and intrahippocampal pharmacological treatment with CBD alleviate behavioral hyperactivity in Gria1-/- mice. CBD in this study has reproduced the effects of glutamate-modulating drug-treatments in blunting the excessive hyperlocomotion and the hyperactivity of the dorsal hippocampus in Gria1-/- mice (Procaccini et al., 2013; Maksimovic et al., 2014a,b). Although the precise brain circuitry and pharmacological targets involved in the CBD effect require further elucidation, our data contribute to the possibility that CBD actions on glutamatergic transmission in the hippocampus could be therapeutically applied to dampen hyperexcitable hippocampal and other brain circuitries.
All datasets generated for this study are included in the manuscript and/or the Supplementary Files.
TA-a, MM, and ERK originated the study and wrote the first draft of the manuscript. TA-a, MM, and KD performed the experiments and statistical analyses. RS provided some mouse cohorts. All authors contributed to manuscript revision, read and approved the submitted version.
The study was partially supported by the Academy of Finland, The Finnish Foundation for Alcohol Studies and the Sigrid Juselius Foundation (TA-a, ERK), the Emil Aaltonen Foundation (MM) and by the Ingeborg-Ständer Foundation (RS). The one access publication fee is paid by the Library of the University of Helsinki.
The authors declare that the research was conducted in the absence of any commercial or financial relationships that could be construed as a potential conflict of interest.
The Supplementary Material for this article can be found online at: https://www.frontiersin.org/articles/10.3389/fphar.2019.00309/full#supplementary-material
Armbruster, B. N., Li, X., Pausch, M. H., Herlitze, S., and Roth, B. L. (2007). Evolving the lock to fit the key to create a family of G protein-coupled receptors potently activated by an inert ligand. Proc. Natl. Acad. Sci. U.S.A. 104, 5163–5168. doi: 10.1073/pnas.0700293104
Bannerman, D. M., Deacon, R. M., Brady, S., Bruce, A., Sprengel, R., Seeburg, P. H., et al. (2004). A comparison of GluR-A-deficient and wild-type mice on a test battery assessing sensorimotor, affective, and cognitive behaviors. Behav. Neurosci. 118, 643–647. doi: 10.1037/0735-7044.118.3.643
Bannerman, D. M., Sprengel, R., Sanderson, D. J., McHugh, S. B., Rawlins, J. N., Monyer, H., et al. (2014). Hippocampal synaptic plasticity, spatial memory and anxiety. Nat. Rev. Neurosci. 15, 181–192. doi: 10.1038/nrn3677
Barkus, C., Feyder, M., Graybeal, C., Wright, T., Wiedholz, L., Izquierdo, A., et al. (2011). Do GluA1 knockout mice exhibit behavioral abnormalities relevant to the negative or cognitive symptoms of schizophrenia and schizoaffective disorder? Neuropharmacology 62, 1263–1272. doi: 10.1016/j.neuropharm.2011.06.005
Bianchi, L., Ballini, C., Colivicchi, M. A., Della Corte, L., Giovannini, M. G., and Pepeu, G. (2003). Investigation on acetylcholine, aspartate, glutamate and GABA extracellular levels from ventral hippocampus during repeated exploratory activity in the rat. Neurochem. Res. 28, 565–573. doi: 10.1023/A:1022881625378
Boekhoudt, L., Omrani, A., Luijendijk, M. C., Wolterink-Donselaar, I. G., Wijbrans, E. C., van der Plasse, G., et al. (2016). Chemogenetic activation of dopamine neurons in the ventral tegmental area, but not substantia nigra, induces hyperactivity in rats. Eur. Neuropsychopharmacol. 26, 1784–1793. doi: 10.1016/j.euroneuro.2016.09.003
Cai, X., Gu, Z., Zhong, P., Ren, Y., and Yan, Z. (2002). Serotonin 5-HT1A receptors regulate AMPA receptor channels through inhibiting Ca2+/calmodulin-dependent kinase II in prefrontal cortical pyramidal neurons. J. Biol. Chem. 277, 36553–36562. doi: 10.1074/jbc.M203752200
Campos, A. C., and Guimaraes, F. S. (2008). Involvement of 5HT1A receptors in the anxiolytic-like effects of cannabidiol injected into the dorsolateral periaqueductal gray of rats. Psychopharmacology 199, 223–230. doi: 10.1007/s00213-008-1168-x
Chourbaji, S., Vogt, M. A., Fumagalli, F., Sohr, R., Frasca, A., Brandwein, C., et al. (2008). AMPA receptor subunit 1 (GluR-A) knockout mice model the glutamate hypothesis of depression. FASEB J. 22, 3129–3134. doi: 10.1096/fj.08-106450
Colizzi, M., McGuire, P., Pertwee, R. G., and Bhattacharyya, S. (2016). Effect of cannabis on glutamate signalling in the brain: a systematic review of human and animal evidence. Neurosci. Biobehav. Rev. 64, 359–381. doi: 10.1016/j.neubiorev.2016.03.010
Cryan, J. F., and Slattery, D. A. (2007). Animal models of mood disorders: recent developments. Curr. Opin. Psychiatry 20, 1–7. doi: 10.1097/YCO.0b013e3280117733
Devor, A., Andreassen, O. A., Wang, Y., Maki-Marttunen, T., Smeland, O. B., Fan, C. C., et al. (2017). Genetic evidence for role of integration of fast and slow neurotransmission in schizophrenia. Mol. Psychiatry 22, 792–801. doi: 10.1038/mp.2017.33
DiLuca, M., and Olesen, J. (2014). The cost of brain diseases: a burden or a challenge? Neuron 82, 1205–1208. doi: 10.1016/j.neuron.2014.05.044
Do Monte, F. H., Souza, R. R., Bitencourt, R. M., Kroon, J. A., and Takahashi, R. N. (2013). Infusion of cannabidiol into infralimbic cortex facilitates fear extinction via CB1 receptors. Behav. Brain. Res. 250, 23–27. doi: 10.1016/j.bbr.2013.04.045
Feyder, M., Wiedholz, L., Sprengel, R., and Holmes, A. (2007). Impaired associative fear learning in mice with complete loss or haploinsufficiency of AMPA GluR1 receptors. Front. Behav. Neurosci. 1:4. doi: 10.3389/neuro.08.004.2007
Fitzgerald, P. J., Barkus, C., Feyder, M., Wiedholz, L. M., Chen, Y. C., Karlsson, R. M., et al. (2010). Does gene deletion of AMPA GluA1 phenocopy features of schizoaffective disorder? Neurobiol. Dis. 40, 608–621. doi: 10.1016/j.nbd.2010.08.005
Forrest, A. D., Coto, C. A., and Siegel, S. J. (2014). Animal models of psychosis: current state and future directions. Curr. Behav. Neurosci. Rep. 1, 100–116. doi: 10.1007/s40473-014-0013-2
Franklin, K. B. J., and Paxinos, G. (2008). The Mouse Brain in Stereotaxic Coordinates, 3rd Edn, Amsterdam: Elsevier.
Freudenberg, F., Resnik, E., Kolleker, A., Celikel, T., Sprengel, R., and Seeburg, P. H. (2016). Hippocampal GluA1 expression in Gria1(-/-) mice only partially restores spatial memory performance deficits. Neurobiol. Learn. Mem. 135, 83–90. doi: 10.1016/j.nlm.2016.07.005
Gururajan, A., Taylor, D. A., and Malone, D. T. (2011). Effect of cannabidiol in a MK-801-rodent model of aspects of schizophrenia. Behav. Brain Res. 222, 299–308. doi: 10.1016/j.bbr.2011.03.053
Gururajan, A., Taylor, D. A., and Malone, D. T. (2012). Cannabidiol and clozapine reverse MK-801-induced deficits in social interaction and hyperactivity in Sprague-Dawley rats. J. Psychopharmacol. 26, 1317–1332. doi: 10.1177/0269881112441865
Hartmann, B., Ahmadi, S., Heppenstall, P. A., Lewin, G. R., Schott, C., Borchardt, T., et al. (2004). The AMPA receptor subunits GluR-A and GluR-B reciprocally modulate spinal synaptic plasticity and inflammatory pain. Neuron 44, 637–650. doi: 10.1016/j.neuron.2004.10.029
Hurst, K., Badgley, C., Ellsworth, T., Bell, S., Friend, L., Prince, B., et al. (2017). A putative lysophosphatidylinositol receptor GPR55 modulates hippocampal synaptic plasticity. Hippocampus 27, 985–998. doi: 10.1002/hipo.22747
Ibeas Bih, C., Chen, T., Nunn, A. V., Bazelot, M., Dallas, M., and Whalley, B. J. (2015). Molecular targets of cannabidiol in neurological disorders. Neurotherapeutics 12, 699–730. doi: 10.1007/s13311-015-0377-3
Inta, D., Vogt, M. A., Elkin, H., Weber, T., Lima-Ojeda, J. M., Schneider, M., et al. (2014). Phenotype of mice with inducible ablation of GluA1 AMPA receptors during late adolescence: relevance for mental disorders. Hippocampus 24, 424–435. doi: 10.1002/hipo.22236
Izzo, A. A., Borrelli, F., Capasso, R., Di Marzo, V., and Mechoulam, R. (2009). Non-psychotropic plant cannabinoids: new therapeutic opportunities from an ancient herb. Trends Pharmacol. Sci. 30, 515–527. doi: 10.1016/j.tips.2009.07.006
Joca, S. R., Padovan, C. M., and Guimaraes, F. S. (2003). Activation of post-synaptic 5-HT(1A) receptors in the dorsal hippocampus prevents learned helplessness development. Brain Res. 978, 177–184. doi: 10.1016/S0006-8993(03)02943-3
Kaplan, J. S., Stella, N., Catterall, W. A., and Westenbroek, R. E. (2017). Cannabidiol attenuates seizures and social deficits in a mouse model of Dravet syndrome. Proc. Natl. Acad. Sci. U.S.A. 114, 11229–11234. doi: 10.1073/pnas.1711351114
Long, L. E., Chesworth, R., Huang, X. F., McGregor, I. S., Arnold, J. C., and Karl, T. (2010). A behavioural comparison of acute and chronic Delta9-tetrahydrocannabinol and cannabidiol in C57BL/6JArc mice. Int. J. Neuropsychopharmacol. 13, 861–876. doi: 10.1017/S1461145709990605
Long, L. E., Chesworth, R., Huang, X. F., Wong, A., Spiro, A., McGregor, I. S., et al. (2012). Distinct neurobehavioural effects of cannabidiol in transmembrane domain neuregulin 1 mutant mice. PLoS One 7:e34129. doi: 10.1371/journal.pone.0034129
Long, L. E., Malone, D. T., and Taylor, D. A. (2006). Cannabidiol reverses MK-801-induced disruption of prepulse inhibition in mice. Neuropsychopharmacology 31, 795–803. doi: 10.1038/sj.npp.1300838
MacLaren, D. A., Browne, R. W., Shaw, J. K., Krishnan Radhakrishnan, S., Khare, P., Espana, R. A., et al. (2016). Clozapine N-oxide administration produces behavioral effects in long-evans rats: implications for designing DREADD experiments. eNeuro 3:ENEURO.0219-16.2016. doi: 10.1523/eneuro.0219-16.2016
Maksimovic, M., Aitta-Aho, T., and Korpi, E. R. (2014a). Reversal of novelty-induced hippocampal c-Fos expression in GluA1 subunit-deficient mice by chronic treatment targeting glutamatergic transmission. Eur. J. Pharmacol. 745, 36–45. doi: 10.1016/j.ejphar.2014.10.005
Maksimovic, M., Vekovischeva, O. Y., Aitta-aho, T., and Korpi, E. R. (2014b). Chronic treatment with mood-stabilizers attenuates abnormal hyperlocomotion of GluA1-subunit deficient mice. PLoS One 9:e100188. doi: 10.1371/journal.pone.0100188
Manvich, D. F., Webster, K. A., Foster, S. L., Farrell, M. S., Ritchie, J. C., Porter, J. H., et al. (2018). The DREADD agonist clozapine N-oxide (CNO) is reverse-metabolized to clozapine and produces clozapine-like interoceptive stimulus effects in rats and mice. Sci. Rep. 8:3840. doi: 10.1038/s41598-018-22116-z
Millan, M. J., Goodwin, G. M., Meyer-Lindenberg, A., and Ogren, S. O. (2015a). 60 years of advances in neuropsychopharmacology for improving brain health, renewed hope for progress. Eur. Neuropsychopharmacol. 25, 591–598. doi: 10.1016/j.euroneuro.2015.01.015
Millan, M. J., Goodwin, G. M., Meyer-Lindenberg, A., and Ove Ogren, S. (2015b). Learning from the past and looking to the future: emerging perspectives for improving the treatment of psychiatric disorders. Eur. Neuropsychopharmacol. 25, 599–656. doi: 10.1016/j.euroneuro.2015.01.016
Molas, S., Zhao-Shea, R., Liu, L., DeGroot, S. R., Gardner, P. D., and Tapper, A. R. (2017). A circuit-based mechanism underlying familiarity signaling and the preference for novelty. Nat. Neurosci. 20, 1260–1268. doi: 10.1038/nn.4607
Montag-Sallaz, M., Welzl, H., Kuhl, D., Montag, D., and Schachner, M. (1999). Novelty-induced increased expression of immediate-early genes c-fos and arg 3.1 in the mouse brain. J. Neurobiol. 38, 234–246. doi: 10.1002/(SICI)1097-4695(19990205)38:2<234::AID-NEU6>3.0.CO;2-G
Moreira, F. A., and Guimaraes, F. S. (2005). Cannabidiol inhibits the hyperlocomotion induced by psychotomimetic drugs in mice. Eur. J. Pharmacol. 512, 199–205. doi: 10.1016/j.ejphar.2005.02.040.
Moser, E. I., Moser, M. B., and McNaughton, B. L. (2017). Spatial representation in the hippocampal formation: a history. Nat. Neurosci. 20, 1448–1464. doi: 10.1038/nn.4653
Paxinos, G., and Franklin, K. B. J. (2001). The Mouse Brain in Stereotaxic Coordinates. New York, NY: Academic Press
Procaccini, C., Aitta-aho, T., Jaako-Movits, K., Zharkovsky, A., Panhelainen, A., Sprengel, R., et al. (2011). Excessive novelty-induced c-Fos expression and altered neurogenesis in the hippocampus of GluA1 knockout mice. Eur. J. Neurosci. 33, 161–174. doi: 10.1111/j.1460-9568.2010.07485.x
Procaccini, C., Maksimovic, M., Aitta-Aho, T., Korpi, E. R., and Linden, A. M. (2013). Reversal of novelty-induced hyperlocomotion and hippocampal c-Fos expression in GluA1 knockout male mice by the mGluR2/3 agonist LY354740. Neuroscience 250, 189–200. doi: 10.1016/j.neuroscience.2013.07.010
Reisel, D., Bannerman, D. M., Schmitt, W. B., Deacon, R. M., Flint, J., Borchardt, T., et al. (2002). Spatial memory dissociations in mice lacking GluR1. Nat. Neurosci. 5, 868–873. doi: 10.1038/nn910
Ripke, S., Neale, B. M., Corvin, A., Walters, J. T., Farh, K. H., Holmans, P. A., et al. (2014). Biological insights from 108 schizophrenia-associated genetic loci. Nature 511, 421–427. doi: 10.1038/nature13595.
Ripke, S., O’Dushlaine, C., Chambert, K., Moran, J. L., Kahler, A. K., Akterin, S., et al. (2013). Genome-wide association analysis identifies 13 new risk loci for schizophrenia. Nat. Genet. 45, 1150–159. doi: 10.1038/ng.2742
Rock, E. M., Bolognini, D., Limebeer, C. L., Cascio, M. G., Anavi-Goffer, S., Fletcher, P. J., et al. (2012). Cannabidiol, a non-psychotropic component of cannabis, attenuates vomiting and nausea-like behaviour via indirect agonism of 5-HT(1A) somatodendritic autoreceptors in the dorsal raphe nucleus. Br. J. Pharmacol. 165, 2620–2634. doi: 10.1111/j.1476-5381.2011.01621.x
Roth, B. L. (2016). DREADDs for neuroscientists. Neuron 89, 683–694. doi: 10.1016/j.neuron.2016.01.040
Russo, E. B., Burnett, A., Hall, B., and Parker, K. K. (2005). Agonistic properties of cannabidiol at 5-HT1a receptors. Neurochem. Res. 30, 1037–1043. doi: 10.1007/s11064-005-6978-1
Sanderson, D. J., and Bannerman, D. M. (2012). The role of habituation in hippocampus-dependent spatial working memory tasks: evidence from GluA1 AMPA receptor subunit knockout mice. Hippocampus 22, 981–994. doi: 10.1002/hipo.20896
Sanderson, D. J., Good, M. A., Seeburg, P. H., Sprengel, R., Rawlins, J. N., and Bannerman, D. M. (2008). The role of the GluR-A (GluR1) AMPA receptor subunit in learning and memory. Prog. Brain Res. 169, 159–178. doi: 10.1016/S0079-6123(07)00009-X
Sanderson, D. J., Good, M. A., Skelton, K., Sprengel, R., Seeburg, P. H., Rawlins, J. N., et al. (2009). Enhanced long-term and impaired short-term spatial memory in GluA1 AMPA receptor subunit knockout mice: evidence for a dual-process memory model. Learn. Mem. 16, 379–386. doi: 10.1101/lm.1339109
Sanderson, D. J., McHugh, S. B., Good, M. A., Sprengel, R., Seeburg, P. H., Rawlins, J. N., et al. (2010). Spatial working memory deficits in GluA1 AMPA receptor subunit knockout mice reflect impaired short-term habituation: evidence for Wagner’s dual-process memory model. Neuropsychologia 48, 2303–2315. doi: 10.1016/j.neuropsychologia.2010.03.018
Schubart, C. D., Sommer, I. E., Fusar-Poli, P., de Witte, L., Kahn, R. S., and Boks, M. P. (2014). Cannabidiol as a potential treatment for psychosis. Eur. Neuropsychopharmacol. 24, 51–64. doi: 10.1016/j.euroneuro.2013.11.002
Sylantyev, S., Jensen, T. P., Ross, R. A., and Rusakov, D. A. (2013). Cannabinoid- and lysophosphatidylinositol-sensitive receptor GPR55 boosts neurotransmitter release at central synapses. Proc. Natl. Acad. Sci. U.S.A. 110, 5193–5198. doi: 10.1073/pnas.1211204110
Todd, S. M., and Arnold, J. C. (2016). Neural correlates of interactions between cannabidiol and Delta(9) -tetrahydrocannabinol in mice: implications for medical cannabis. Br. J. Pharmacol. 173, 53–65. doi: 10.1111/bph.13333
Vazquez, G. H., Camino, S., Tondo, L., and Baldessarini, R. J. (2017). Potential novel treatments for bipolar depression: ketamine, fatty acids, anti-inflammatory agents, and probiotics. CNS Neurol. Disord. Drug. Targets 16, 858–869. doi: 10.2174/1871527316666170728165648
Vekovischeva, O. Y., Aitta-Aho, T., Echenko, O., Kankaanpaa, A., Seppala, T., Honkanen, A., et al. (2004). Reduced aggression in AMPA-type glutamate receptor GluR-A subunit-deficient mice. Genes Brain Behav. 3, 253–265. doi: 10.1111/j.1601-1848.2004.00075.x
Vekovischeva, O. Y., Zamanillo, D., Echenko, O., Seppala, T., Uusi-Oukari, M., Honkanen, A., et al. (2001). Morphine-induced dependence and sensitization are altered in mice deficient in AMPA-type glutamate receptor-A subunits. J. Neurosci. 21, 4451–4459. doi: 10.1523/JNEUROSCI.21-12-04451.2001
Wiedholz, L. M., Owens, W. A., Horton, R. E., Feyder, M., Karlsson, R. M., Hefner, K., et al. (2008). Mice lacking the AMPA GluR1 receptor exhibit striatal hyperdopaminergia and ‘schizophrenia-related’ behaviors. Mol. Psychiatry 13, 631–640. doi: 10.1038/sj.mp.4002056
Zamanillo, D., Sprengel, R., Hvalby, O., Jensen, V., Burnashev, N., Rozov, A., et al. (1999). Importance of AMPA receptors for hippocampal synaptic plasticity but not for spatial learning. Science 284, 1805–1811. doi: 10.1126/science.284.5421.1805
Keywords: AMPA receptors, cannabidiol, DREADD, hippocampus, c-Fos, hyperactivity, novelty, hM4Gi
Citation: Aitta-aho T, Maksimovic M, Dahl K, Sprengel R and Korpi ER (2019) Attenuation of Novelty-Induced Hyperactivity of Gria1-/- Mice by Cannabidiol and Hippocampal Inhibitory Chemogenetics. Front. Pharmacol. 10:309. doi: 10.3389/fphar.2019.00309
Received: 13 December 2018; Accepted: 13 March 2019;
Published: 29 March 2019.
Edited by:
Salvatore Salomone, Università degli Studi di Catania, ItalyReviewed by:
Emmanuel Valjent, Centre National de la Recherche Scientifique (CNRS), FranceCopyright © 2019 Aitta-aho, Maksimovic, Dahl, Sprengel and Korpi. This is an open-access article distributed under the terms of the Creative Commons Attribution License (CC BY). The use, distribution or reproduction in other forums is permitted, provided the original author(s) and the copyright owner(s) are credited and that the original publication in this journal is cited, in accordance with accepted academic practice. No use, distribution or reproduction is permitted which does not comply with these terms.
*Correspondence: Esa R. Korpi, ZXNhLmtvcnBpQGhlbHNpbmtpLmZp
†These authors have contributed equally to this work
Disclaimer: All claims expressed in this article are solely those of the authors and do not necessarily represent those of their affiliated organizations, or those of the publisher, the editors and the reviewers. Any product that may be evaluated in this article or claim that may be made by its manufacturer is not guaranteed or endorsed by the publisher.
Research integrity at Frontiers
Learn more about the work of our research integrity team to safeguard the quality of each article we publish.