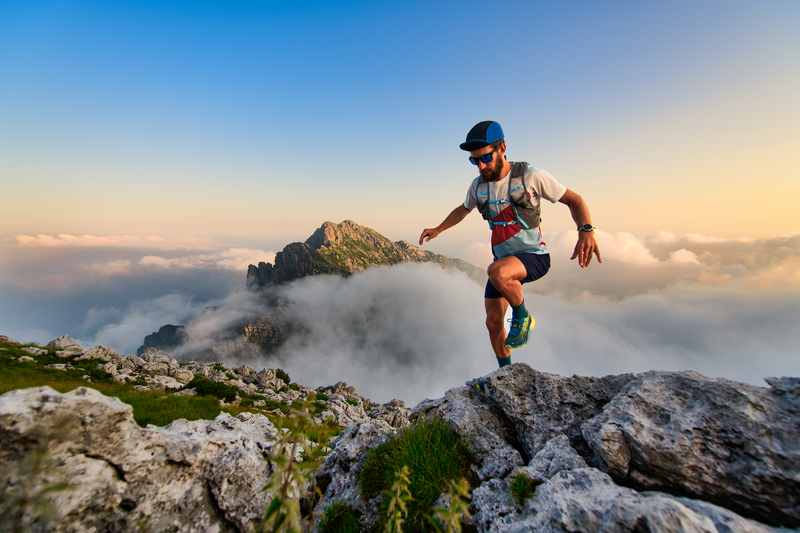
95% of researchers rate our articles as excellent or good
Learn more about the work of our research integrity team to safeguard the quality of each article we publish.
Find out more
MINI REVIEW article
Front. Pharmacol. , 20 March 2019
Sec. Translational Pharmacology
Volume 10 - 2019 | https://doi.org/10.3389/fphar.2019.00248
This article is part of the Research Topic Cell-Free Production Platforms for Developing Tools and Applications in Pharmacology View all 8 articles
The translation system is a sophisticated machinery that synthesizes proteins from 20 canonical amino acids. Recently, the repertoire of such composition has been expanded by the introduction of non-canonical amino acids (ncAAs) with the genetic code expansion strategy, which provides proteins with designed properties and structures for protein studies and engineering. Although the genetic code expansion strategy has been mostly implemented by using living cells as the host, a number of limits such as poor cellular uptake or solubility of specific ncAA substrates and the toxicity of target proteins have hindered the production of certain ncAA-modified proteins. To overcome those challenges, cell-free protein synthesis (CFPS) has been applied as it allows the precise control of reaction components. Several approaches have been recently developed to increase the purity and efficiency of ncAA incorporation in CFPS. Here, we summarized recent development of CFPS with an emphasis on its applications in generating site-specific protein post-translational modifications by the genetic code expansion strategy.
The translational machinery in nature provides a new avenue of chemistry in which synthetic biologists could prospect beyond the repertoire of 20 canonical amino acids (Liu and Schultz, 2010). Generally, an orthogonal pair of aminoacyl-tRNA synthetase (AARS) and its cognate tRNA, which does not cross-react with endogenous AARSs and tRNAs in the host, is used or evolved to direct the incorporation of a non-canonical amino acid (ncAA) into the specific site of target proteins (Mukai et al., 2017) (Figure 1). Because of diversified functional groups, ncAAs endow proteins with modified structures, functions, and interactions (O’Donoghue et al., 2013). This approach, named genetic code expansion, has paved the way for various applications in biological studies (Chin, 2014; Li and Liu, 2014; Neumann-Staubitz and Neumann, 2016). It has also been exploited to design new therapeutics and vaccines for pharmaceutical applications (Axup et al., 2012; Wang et al., 2014).
Figure 1. An overview of genetic code expansion. The evolved aminoacyl-tRNA synthetase (AARS) charges the tRNA with the non-canonical amino acid (ncAA). The tRNA harbors the CUA anticodon, which can read the introduced amber stop codon UAG in the mRNA, bringing the ncAA at the designed position in target proteins. AA: canonical amino acid.
Recently, cell-free protein synthesis (CFPS) has stepped into the field of genetic code expansion (Hong et al., 2014). Even though with limitations such as the need for folding chaperones and the lack of natural membranes for membrane protein synthesis, CFPS also has a number of unique advantages over living cells for protein synthesis. First, the open system allows convenient manipulation of the protein synthesis environment and facilitates appropriate protein folding due to the considerable distance between the ribosomes (Rosenblum and Cooperman, 2014). Second, it permits the synthesis of proteins toxic to cells (Worst et al., 2015). Third, it has no limit for cellular uptake and solubility of target ncAAs (Bundy and Swartz, 2010). Fourth, CFPS does not need to grow, collect, and break cells to obtain target proteins, thus saving time for downstream experiments, which is especially important for unstable proteins.
It becomes crucial to study post-translational modifications (PTMs) because of their significant role in various cellular functions and biological processes (Lothrop et al., 2013). Besides being mostly reversible, competitions among different PTMs for the same residues and limited knowledge of modifying enzymes make it difficult to synthesize homogeneously modified proteins for studying. To overcome these challenges, the genetic code expansion strategy has been applied in PTM studies (Liu et al., 2011; Chen et al., 2018). Here, we summarized the applications of CFPS in generating proteins with PTMs through the genetic code expansion strategy.
The first in vitro work of site-specifically incorporating phosphotyrosine (pTyr) into proteins was performed to generate modified luciferase analogs (Arslan et al., 1997). In this work, pTyr was attached to the di-nucleotide pdCpA and then linked to the tRNA transcript lacking the last two nucleotides (CA) by T4 RNA ligase. The pTyr-charged tRNA with a CUA anticodon was used to suppress the UAG codon in the luciferase mRNA transcript to incorporate pTyr in the designed site of luciferase. The similar approach was also used to incorporate a pTyr analog, phosphonotyrosine into luciferase. However, the incorporation efficiency was relatively low. To increase the yield, a nitrophenylethyl-caged pTyr was synthesized to mask the negative charges of the phosphate as the elongation factor (EF-Tu) binds poorly with negatively charged amino acids (Rothman et al., 2005). The caging group could be easily removed by the UV light after incorporating into the protein. The same approach was also applied to generate phosphoserine (pSer)- and phosphothreonine (pThr)-modified proteins. By using vasodilator-stimulated phosphoprotein (VASP) that is involved in cell migration processes as a target protein, the phosphoprotein generated by CFPS was shown to be active as expected. Another analog of pTyr, phosphonoamino-phenylalanine, was later incorporated into protein by two steps. First, the azido-phenylalanine-modified protein was generated by CFPS with the amber-suppression system. Then, the azido-phenylalanine side chain was modified into phosphonoamino-phenylalanine through the Staudinger-phosphite reaction (Serwa et al., 2009).
It has been a challenge for decades to study serine phosphorylation both structurally and functionally. Although many attempts were made by using mimics such as aspartate or glutamate, they could not reflect the real situation due to charge and size differences. Efforts made to incorporate pSer in vivo by the genetic code expansion strategy have been successful (Park et al., 2011; Heinemann et al., 2012; Pirman et al., 2015; Rogerson et al., 2015). However, some issues such as endogenous phosphatases in host cells, which could remove phosphorylation, still exist.
In order to tackle these limitations, CFPS was applied to achieve pure and high yield of serine phosphorylation at multiple sites in proteins (Oza et al., 2015). One issue with the amber-suppression approach is the competition with the release factor-1 (RF1) for the stop codon. Thus, in this study, a genomically recoded RF1-deficient strain (E. coli C321.ΔA) in which all 321 TAG codons in the genome were substituted with TAA codons to improve cell growth and increase purity of ncAA incorporation (Lajoie et al., 2013) was used to generate the CFPS system. By using human mitogen-activated ERK activating kinase 1 (MEK1) as a validating example, 1 mg of phosphorylated MEK1 was obtained from 2 ml of CFPS reaction mixtures (Oza et al., 2015). This was a dramatic increase in protein yield compared to that of the in vivo pSer incorporation system in which only 1 μg of phosphorylated MEK1 was obtained from 1 L of cell culture (Park et al., 2011).
Although RF1-deficient systems could eliminate the early termination of target proteins in which the amber stop codon is used as the signal of ncAA incorporation, the suppression of the amber codon by endogenous near-cognate tRNAs could bring canonical amino acids into the desired site to cause impurity (Aerni et al., 2014). It is lethal to remove those near-cognate tRNAs from cells due to their essential role in normal protein synthesis. However, CFPS has no such limitations because all the translation components such as RFs and tRNAs can be modulated. Taking the advantage of CFPS, we have recently developed a method to increase the purity of pSer-containing proteins in the RF1-deficient background by reducing the amount of near-cognate tRNAs for the amber stop codon (Gan and Fan, 2017) (Figure 2). In this work, we first used an RF1-specific RNA aptamer to eliminate the RF1 activity in the CFPS system. Then, we applied antisense oligos to reduce the amount of near cognate tRNAs for the amber stop codon (tRNATyr, tRNALys, and one tRNAGln isoacceptor). Without affecting the protein yield, the purity of pSer incorporation at the amber codon increased significantly.
Figure 2. Increasing the fidelity of ncAA incorporation in CFPS. To remove competitions from the release factor-1 (RF1) and near-cognate tRNAs (tRNATyr as a representative) for the amber stop codon UAG for ncAA incorporation, RF1-sepcific aptamer and near-cognate tRNA-specific antisense DNA oligos were used, respectively.
For phosphoprotein synthesis, one challenge of using living cells is the poor uptake of negatively charged phosphoamino acids. Even for phosphoserine that is an intermediate in serine biosynthesis, the concentration of the free amino acid is still relatively low in cells (Steinfeld et al., 2014). Another issue with living cells is endogenous phosphatases, making phosphoprotein dephosphorylated easily. CFPS is able to solve both problems ideally. First, CFPS is an open system and has no issue of amino acid uptake. Second, the PURE system contains only translation components without any phosphatases. Furthermore, enzymatic syntheses of pTyr- and pThr-charged tRNAs have been recently established (Fan et al., 2016; Zhang et al., 2017), making it convenient for biological laboratories to generate pTyr- and pThr-containing proteins without multi-step organic synthesis of pTyr- or pThr-charged tRNAs by chemical ligation.
Lysine acetylation is one of the most well-studied PTMs because of its crucial role in DNA replication, DNA repair, and transcription (Sterner and Berger, 2000). To study lysine acetylation without concerning about endogenous deacetylases in cells, non-deacetylatable analogs of Nε -acetyllysine (AcK) such as Nε -thio-acetyllysine (ThioAcK) and Nε -seleno-acetyllysine (SeAcK) were proposed. ThioAcK, which has only a single atom replacement, has been shown to be the most comparable analog of AcK in structures and functions in our recent in vivo studies with the genetic code expansion strategy (Venkat et al., 2017).
Actually, ThioAcK has already been incorporated into proteins in a previous work by combining flexizyme and the PURE (protein synthesis using purified recombinant elements) system (Xiong et al., 2016). Flexizyme is a catalytic RNA, which is able to mediate the aminoacylation of the tRNA with a wide range of ncAAs, providing scientists with a facile method to obtain ncAA-charged tRNAs without labor-consuming AARS engineering (Morimoto et al., 2011). The PURE system contains affinity-purified protein components of the translational machinery including initiation factors, elongation factors, release factors, ribosome recycling factors, and 20 AARSs (Shimizu et al., 2001). In this work, flexizyme was first used to generate suppressor tRNAs charged with AcK, ThioAcK, or SeAcK, individually. Then, the aminoacylated suppressor tRNA was added into the PURE ΔRF1 system to incorporate AcK or its analogs at K9 and K56 positions of histone H3. Results showed highly efficient incorporation at both positions. Furthermore, by using two suppressor tRNAs harboring different anticodons, the study also made the dual incorporation of AcK and ThioAcK in the same protein possible, paving the way for future studies that can lead to deeper understanding of the crosstalk between PTMs.
Besides flexizyme-mediated tRNA aminoacylation, enzyme-catalyzed tRNA charging by evolved pyrrolysyl-tRNA synthetase (PylRS) variants has also been used to generate site-specifically acetylated proteins such as histone H4 to study inter- and intra-nucleosome interactions by CFPS (Wakamori et al., 2015). As the charged tRNAPyl can be recycled for another round of tRNA aminoacylation, the protein yield was much higher than those generated by chemical or ribozyme-dependent aminoacylation. Advancements of CFPS for ncAA incorporation followed the development of RF1-deficient strains, which were designed to aid UAG codon reassignment. As the host strain for the CFPS system, BL21-based RF1-deficient cells were optimized for cell growth by engineering an essential gene sucB (Mukai et al., 2011). The optimized CFPS could generate full-length histone H4 with four lysine residue acetylated simultaneously with the yield of 0.2 mg protein per 1 ml reaction, while the yield of the same protein produced in living cells was 1 mg per 1 L culture.
Lysine methylation is another important PTM of lysine residues that modulates epigenetic statuses and various other biological processes including heterochromatin development and transcription (Lanouette et al., 2014). Different from lysine acetylation, lysine methylation has various forms including mono-, di-, and tri-methylation, which have different effects on protein functions (Martin and Zhang, 2005). Furthermore, due to the similarity between methylated lysine (MeK) and lysine itself, it is difficult to evolve AARSs specifically for MeK only. Thus, genetic incorporation of lysine methylation in living cells has not succeeded.
On the other hand, evolved AARSs for MeK incorporation are not necessary for CFPS, which has already been used to produce site-specifically methylated proteins. To introduce mono-methyllysine, di-methyllysine, and tri-methyllysine, frameshift and amber-suppressor tRNAs charged with MeKs were synthesized by chemical aminoacylation and supplied to an E. coli CFPS system (Tokuda et al., 2011). The MeKs were successfully incorporated into streptavidin and histone H3 in a quantitative, position-specific manner.
Similar to the AcK incorporation, RF1-deficient strains have also been used for MeK incorporation in CFPS (Yanagisawa et al., 2014). In order to study the impact of lysine methylation in histone H3, cell lysates of RF1-deficient strains were used to incorporate Nε -(tert-butyloxycarbonyl)-Nε -methyl-L-lysine (BocKme1), a protected mono-MeK analog, by an engineered PylRS variant first, which was then removed by trifluoroacetic acid. Up to four lysine residues could be modified at the same time by the CFPS system. Compared with the genetic incorporation in living cells, the CFPS system has an important advantage in which the amounts of proteins obtained per consumed BocKme1 were considerably larger (10–20 folds) than those of the cell-based system. This is also true for other cell-based ncAA incorporation in which a large proportion of ncAAs is wasted in cell growth media without efficient approaches to recycle. Thus, CFPS is a more cost-efficient choice when target ncAAs are expensive or need special synthesis.
In this review, we summarized the applications of CFPS in generating proteins with PTMs by the genetic code expansion strategy. Generally, major differences between these approaches are the methods to get ncAA-charged suppressor tRNAs. The traditional chemical ligation method needs extensive organic synthesis, which could not be practical for biological laboratories. Flexizyme-mediated tRNA charging is relatively easy to handle and also could provide a wide range of ncAA candidates. However, suppressor tRNAs cannot be recycled, making the protein yield relatively low. Evolved AARSs for specific ncAAs could overcome this issue to increase the incorporation efficiency. But, AARS engineering is time- and labor-consuming and may not be successful. So, researchers should choose appropriate approaches, which fit for their own laboratory setup and project needs. There are also many other successful works of using CFPS to generate proteins with PTMs such as glycosylation (Tarui et al., 2001; Jaroentomeechai et al., 2018) and myristoylation (Suzuki et al., 2010; Yamauchi et al., 2010) through enzymatic modifications, which were not discussed in this review.
CFPS has led to many break-through in various fields. In structural biology, CFPS could generate high yields of cytotoxic proteins for crystallization (Avenaud et al., 2004). CFPS can also increase the incorporation efficiency of selenomethionine into protein to help crystallographers with protein phasing (Kigawa et al., 2002). In the diagnostics and therapeutics, CFPS has been used for generating viral particles and detecting mutations in marker genes by coupling transcription and translation of specific RT-PCR or PCR amplified products (Cello et al., 2002; Gite et al., 2003). In the field of synthetic biology, CFPS has grown to emerge as an assuring approach for incorporating ncAAs at specific sites in proteins, setting the stage for studying protein interactions and PTMs. The course of development for CFPS in genetic code expansion would be prominently concentrated on building better genetic incorporation systems and more efficient cell extracts. This will not only be in terms of reducing the cost of the systems but also making it readily available to the ever-changing scientific needs.
A promising application of CFPS in PTM studies would be to generate a single protein with multiple PTMs simultaneously. Although most of studies generated proteins with only one type of PTMs, it is known that proteins usually have multiple PTMs, which co-regulate protein functions. Thus, it is necessary to generate multi-modified proteins. Recently, we have shown that the most-widely used genetic incorporation systems, which are derived from the Methanocaldococcus jannaschii tyrosyl-tRNA synthetase for incorporation of tyrosine or phenylalanine analogs, the Methanosarcina species PylRS for incorporation of lysine derivatives, or the Methanococcus maripaludis phosphoseryl-tRNA synthetase for pSer/pThr incorporation, are orthogonal to each other, making it possible to produce dual- or tri-modified proteins (Venkat et al., 2018). However, multiple ncAA incorporation in living cells needs to introduce a number of plasmids, which usually causes strong growth effects to lower protein yields. CFPS has no such concerns for cell growth; thus, it could be the first choice to generate proteins with multiple PTMs simultaneously.
All authors listed have made a substantial, direct and intellectual contribution to the work, and approved it for publication.
This work was supported by the Ralph E. Powe Junior Faculty Enhancement Award from Oak Ridge Associated Universities to CF, the grant from Arkansas Biosciences Institute to CF, and the start-up fund from University of Arkansas to CF.
The authors declare that the research was conducted in the absence of any commercial or financial relationships that could be construed as a potential conflict of interest.
Aerni, H. R., Shifman, M. A., Rogulina, S., O’Donoghue, P., and Rinehart, J. (2014). Revealing the amino acid composition of proteins within an expanded genetic code. Nucleic Acids Res. 43:e8. doi: 10.1093/nar/gku1087
Arslan, T., Mamaev, S. V., Mamaeva, N. V., and Hecht, S. M. (1997). Structurally modified firefly luciferase. Effects of amino acid substitution at position 286. J. Am. Chem. Soc. 119, 10877–10887. doi: 10.1021/ja971927a
Avenaud, P., Castroviejo, M., Claret, S., Rosenbaum, J., Megraud, F., and Menard, A. (2004). Expression and activity of the cytolethal distending toxin of Helicobacter hepaticus. Biochem. Biophys. Res. Commun. 318, 739–745. doi: 10.1016/j.bbrc.2004.04.089
Axup, J. Y., Bajjuri, K. M., Ritland, M., Hutchins, B. M., Kim, C. H., Kazane, S. A., et al. (2012). Synthesis of site-specific antibody-drug conjugates using unnatural amino acids. Proc. Natl. Acad. Sci. 109, 16101–16106. doi: 10.1073/pnas.1211023109
Bundy, B. C., and Swartz, J. R. (2010). Site-specific incorporation of p-propargyloxyphenylalanine in a cell-free environment for direct protein-protein click conjugation. Bioconjug. Chem. 21, 255–263. doi: 10.1021/bc9002844
Cello, J., Paul, A. V., and Wimmer, E. (2002). Chemical synthesis of poliovirus cDNA: generation of infectious virus in the absence of natural template. Science 297, 1016–1018. doi: 10.1126/science.1072266
Chen, H., Venkat, S., McGuire, P., Gan, Q., and Fan, C. (2018). Recent development of genetic code expansion for posttranslational modification studies. Molecules 23:1662. doi: 10.3390/molecules23071662
Chin, J. W. (2014). Expanding and reprogramming the genetic code of cells and animals. Annu. Rev. Biochem. 83, 379–408. doi: 10.1146/annurev-biochem-060713-035737
Fan, C., Ip, K., and Soll, D. (2016). Expanding the genetic code of Escherichia coli with phosphotyrosine. FEBS Lett. 590, 3040–3047. doi: 10.1002/1873-3468.12333
Gan, Q., and Fan, C. (2017). Increasing the fidelity of noncanonical amino acid incorporation in cell-free protein synthesis. Biochim. Biophys. Acta Gen. Subj. 1861, 3047–3052. doi: 10.1016/j.bbagen.2016.12.002
Gite, S., Lim, M., Carlson, R., Olejnik, J., Zehnbauer, B., and Rothschild, K. (2003). A high-throughput nonisotopic protein truncation test. Nat. Biotechnol. 21, 194–197. doi: 10.1038/nbt779
Heinemann, I. U., Rovner, A. J., Aerni, H. R., Rogulina, S., Cheng, L., Olds, W., et al. (2012). Enhanced phosphoserine insertion during Escherichia coli protein synthesis via partial UAG codon reassignment and release factor 1 deletion. FEBS Lett. 586, 3716–3722. doi: 10.1016/j.febslet.2012.08.031
Hong, S. H., Kwon, Y. C., and Jewett, M. C. (2014). Non-standard amino acid incorporation into proteins using Escherichia coli cell-free protein synthesis. Front. Chem. 2:34. doi: 10.3389/fchem.2014.00034
Jaroentomeechai, T., Stark, J. C., Natarajan, A., Glasscock, C. J., Yates, L. E., Hsu, K. J., et al. (2018). Single-pot glycoprotein biosynthesis using a cell-free transcription-translation system enriched with glycosylation machinery. Nat. Commun. 9:2686. doi: 10.1038/s41467-018-05110-x
Kigawa, T., Yamaguchi-Nunokawa, E., Kodama, K., Matsuda, T., Yabuki, T., Matsuda, N., et al. (2002). Selenomethionine incorporation into a protein by cell-free synthesis. J. Struct. Funct. Genom. 2, 29–35. doi: 10.1023/A:1013203532303
Lajoie, M. J., Rovner, A. J., Goodman, D. B., Aerni, H. R., Haimovich, A. D., Kuznetsov, G., et al. (2013). Genomically recoded organisms expand biological functions. Science 342, 357–360. doi: 10.1126/science.1241459
Lanouette, S., Mongeon, V., Figeys, D., and Couture, J. F. (2014). The functional diversity of protein lysine methylation. Mol. Syst. Biol. 10:724. doi: 10.1002/msb.134974
Li, X., and Liu, C. C. (2014). Biological applications of expanded genetic codes. ChemBioChem 15, 2335–2341. doi: 10.1002/cbic.201402159
Liu, C. C., and Schultz, P. G. (2010). Adding new chemistries to the genetic code. Annu. Rev. Biochem. 79, 413–444. doi: 10.1146/annurev.biochem.052308.105824
Liu, W. R., Wang, Y. S., and Wan, W. (2011). Synthesis of proteins with defined posttranslational modifications using the genetic noncanonical amino acid incorporation approach. Mol. BioSyst. 7, 38–47. doi: 10.1039/c0mb00216j
Lothrop, A. P., Torres, M. P., and Fuchs, S. M. (2013). Deciphering post-translational modification codes. FEBS Lett. 587, 1247–1257. doi: 10.1016/j.febslet.2013.01.047
Martin, C., and Zhang, Y. (2005). The diverse functions of histone lysine methylation. Nat. Rev. Mol. Cell Biol. 6, 838–849. doi: 10.1038/nrm1761
Morimoto, J., Hayashi, Y., Iwasaki, K., and Suga, H. (2011). Flexizymes: their evolutionary history and the origin of catalytic function. Acc. Chem. Res. 44, 1359–1368. doi: 10.1021/ar2000953
Mukai, T., Lajoie, M. J., Englert, M., and Soll, D. (2017). Rewriting the genetic code. Annu. Rev. Microbiol. 71, 557–577. doi: 10.1146/annurev-micro-090816-093247
Mukai, T., Yanagisawa, T., Ohtake, K., Wakamori, M., Adachi, J., Hino, N., et al. (2011). Genetic-code evolution for protein synthesis with non-natural amino acids. Biochem. Biophys. Res. Commun. 411, 757–761. doi: 10.1016/j.bbrc.2011.07.020
Neumann-Staubitz, P., and Neumann, H. (2016). The use of unnatural amino acids to study and engineer protein function. Curr. Opin. Struct. Biol. 38, 119–128. doi: 10.1016/j.sbi.2016.06.006
O’Donoghue, P., Ling, J., Wang, Y. S., and Soll, D. (2013). Upgrading protein synthesis for synthetic biology. Nat. Chem. Biol. 9, 594–598. doi: 10.1038/nchembio.1339
Oza, J. P., Aerni, H. R., Pirman, N. L., Barber, K. W., Ter Haar, C. M., Rogulina, S., et al. (2015). Robust production of recombinant phosphoproteins using cell-free protein synthesis. Nat. Commun. 6:8168. doi: 10.1038/ncomms9168
Park, H.-S., Hohn, M. J., Umehara, T., Guo, L.-T., Osborne, E. M., Benner, J., et al. (2011). Expanding the genetic code of Escherichia coli with phosphoserine. Science 333, 1151–1154. doi: 10.1126/science.1207203
Pirman, N. L., Barber, K. W., Aerni, H. R., Ma, N. J., Haimovich, A. D., Rogulina, S., et al. (2015). A flexible codon in genomically recoded Escherichia coli permits programmable protein phosphorylation. Nat. Commun. 6:8130. doi: 10.1038/ncomms9130
Rogerson, D. T., Sachdeva, A., Wang, K., Haq, T., Kazlauskaite, A., Hancock, S. M., et al. (2015). Efficient genetic encoding of phosphoserine and its nonhydrolyzable analog. Nat. Chem. Biol. 11, 496–503. doi: 10.1038/nchembio.1823
Rosenblum, G., and Cooperman, B. S. (2014). Engine out of the chassis: cell-free protein synthesis and its uses. FEBS Lett. 588, 261–268. doi: 10.1016/j.febslet.2013.10.016
Rothman, D. M., Petersson, E. J., Vazquez, M. E., Brandt, G. S., Dougherty, D. A., and Imperiali, B. (2005). Caged phosphoproteins. J. Am. Chem. Soc. 127, 846–847. doi: 10.1021/ja043875c
Serwa, R., Wilkening, I., Del Signore, G., Muhlberg, M., Claussnitzer, I., Weise, C., et al. (2009). Chemoselective Staudinger-phosphite reaction of azides for the phosphorylation of proteins. Angew. Chem. Int. Ed. Eng. 48, 8234–8239. doi: 10.1002/anie.200902118
Shimizu, Y., Inoue, A., Tomari, Y., Suzuki, T., Yokogawa, T., Nishikawa, K., et al. (2001). Cell-free translation reconstituted with purified components. Nat. Biotechnol. 19, 751–755. doi: 10.1038/90802
Steinfeld, J. B., Aerni, H. R., Rogulina, S., Liu, Y., and Rinehart, J. (2014). Expanded cellular amino acid pools containing phosphoserine, phosphothreonine, and phosphotyrosine. ACS Chem. Biol. 9, 1104–1112. doi: 10.1021/cb5000532
Sterner, D. E., and Berger, S. L. (2000). Acetylation of histones and transcription-related factors. Microbiol. Mol. Biol. Rev. 64, 435–459. doi: 10.1128/MMBR.64.2.435-459.2000
Suzuki, T., Moriya, K., Nagatoshi, K., Ota, Y., Ezure, T., Ando, E., et al. (2010). Strategy for comprehensive identification of human N-myristoylated proteins using an insect cell-free protein synthesis system. Proteomics 10, 1780–1793. doi: 10.1002/pmic.200900783
Tarui, H., Murata, M., Tani, I., Imanishi, S., Nishikawa, S., and Hara, T. (2001). Establishment and characterization of cell-free translation/glycosylation in insect cell (Spodoptera frugiperda 21) extract prepared with high pressure treatment. Appl. Microbiol. Biotechnol. 55, 446–453. doi: 10.1007/s002530000534
Tokuda, Y., Watanabe, T., Horiike, K., Shiraga, K., Abe, R., Muranaka, N., et al. (2011). Biosynthesis of proteins containing modified lysines and fluorescent labels using non-natural amino acid mutagenesis. J. Biosci. Bioeng. 111, 402–407. doi: 10.1016/j.jbiosc.2010.12.012
Venkat, S., Nannapaneni, D. T., Gregory, C., Gan, Q., McIntosh, M., and Fan, C. (2017). Genetically encoding thioacetyl-lysine as a non-deacetylatable analog of lysine acetylation in Escherichia coli. FEBS Open Bio. 7, 1805–1814. doi: 10.1002/2211-5463.12320
Venkat, S., Sturges, J., Stahman, A., Gregory, C., Gan, Q., and Fan, C. (2018). Genetically incorporating two distinct post-translational modifications into one protein simultaneously. ACS Synth. Biol. 7, 689–695. doi: 10.1021/acssynbio.7b00408
Wakamori, M., Fujii, Y., Suka, N., Shirouzu, M., Sakamoto, K., Umehara, T., et al. (2015). Intra-and inter-nucleosomal interactions of the histone H4 tail revealed with a human nucleosome core particle with genetically-incorporated H4 tetra-acetylation. Sci. Rep. 5:17204. doi: 10.1038/srep17204
Wang, N., Li, Y., Niu, W., Sun, M., Cerny, R., Li, Q., et al. (2014). Construction of a live-attenuated HIV-1 vaccine through genetic code expansion. Angew. Chem. Int. Ed. 53, 4867–4871. doi: 10.1002/anie.201402092
Worst, E. G., Exner, M. P., De Simone, A., Schenkelberger, M., Noireaux, V., Budisa, N., et al. (2015). Cell-free expression with the toxic amino acid canavanine. Bioorg. Med. Chem. Lett. 25, 3658–3660. doi: 10.1016/j.bmcl.2015.06.045
Xiong, H., Reynolds, N. M., Fan, C., Englert, M., Hoyer, D., Miller, S. J., et al. (2016). Dual genetic encoding of acetyl-lysine and non-deacetylatable thioacetyl-lysine mediated by flexizyme. Angew. Chem. Int. Ed. 55, 4083–4086. doi: 10.1002/anie.201511750
Yamauchi, S., Fusada, N., Hayashi, H., Utsumi, T., Uozumi, N., Endo, Y., et al. (2010). The consensus motif for N-myristoylation of plant proteins in a wheat germ cell-free translation system. FEBS J. 277, 3596–3607. doi: 10.1111/j.1742-4658.2010.07768.x
Yanagisawa, T., Takahashi, M., Mukai, T., Sato, S., Wakamori, M., Shirouzu, M., et al. (2014). Multiple site-specific installations of Nε-monomethyl-L-lysine into histone proteins by cell-based and cell-free protein synthesis. ChemBioChem 15, 1830–1838. doi: 10.1002/cbic.201402291
Keywords: genetic code expansion, cell-free protein synthesis, non-canonical amino acid, post-translational modification, protein phosphorylation, protein acetylation, protein methylation
Citation: Venkat S, Chen H, Gan Q and Fan C (2019) The Application of Cell-Free Protein Synthesis in Genetic Code Expansion for Post-translational Modifications. Front. Pharmacol. 10:248. doi: 10.3389/fphar.2019.00248
Received: 29 December 2018; Accepted: 26 February 2019;
Published: 20 March 2019.
Edited by:
Frank Bernhard, Goethe-Universität Frankfurt am Main, GermanyReviewed by:
Yi Ma, South China University of Technology, ChinaCopyright © 2019 Venkat, Chen, Gan and Fan. This is an open-access article distributed under the terms of the Creative Commons Attribution License (CC BY). The use, distribution or reproduction in other forums is permitted, provided the original author(s) and the copyright owner(s) are credited and that the original publication in this journal is cited, in accordance with accepted academic practice. No use, distribution or reproduction is permitted which does not comply with these terms.
*Correspondence: Chenguang Fan, Y2YwMjFAdWFyay5lZHU=
Disclaimer: All claims expressed in this article are solely those of the authors and do not necessarily represent those of their affiliated organizations, or those of the publisher, the editors and the reviewers. Any product that may be evaluated in this article or claim that may be made by its manufacturer is not guaranteed or endorsed by the publisher.
Research integrity at Frontiers
Learn more about the work of our research integrity team to safeguard the quality of each article we publish.