- 1School of Bioscience and Biopharmaceutics, Guangdong Province Key Laboratory for Biotechnology Drug Candidates, Guangdong Pharmaceutical University, Guangzhou, China
- 2Department of Ultrasound, Sun Yat-sen Memorial Hospital, Sun Yat-sen University, Guangzhou, China
- 3Manufacturing, Commonwealth Scientific and Industrial Research Organisation, Clayton, VIC, Australia
Ruthenium complexes are a new generation of metal antitumor drugs that are currently of great interest in multidisciplinary research. In this review article, we introduce the applications of ruthenium complexes in the diagnosis and therapy of tumors. We focus on the actions of ruthenium complexes on DNA, mitochondria, and endoplasmic reticulum of cells, as well as signaling pathways that induce tumor cell apoptosis, autophagy, and inhibition of angiogenesis. Furthermore, we highlight the use of ruthenium complexes as specific tumor cell probes to dynamically monitor the active biological component of the microenvironment and as excellent photosensitizer, catalyst, and bioimaging agents for phototherapies that significantly enhance the diagnosis and therapeutic effect on tumors. Finally, the combinational use of ruthenium complexes with existing clinical antitumor drugs to synergistically treat tumors is discussed.
Introduction
Chemotherapy is an important modality for cancer treatment. Since the introduction of metal chemotherapeutics represented by cisplatinum (Figure 1A), numerous metal agents have been developed as antitumor drugs, and platinum-based drugs have become the focus of metal-based antitumor drug research (Harper et al., 2010; Burger et al., 2011; Wang X. et al., 2015). In recent years, the platinum-based drugs have become the first line of anti-cancer drugs because of their significant antitumor efficacy (Jakupec et al., 2008; Gasser et al., 2011; Wang and Guo, 2013). However, there are increasing reports that platinum-based anticancer drugs have severe side effects including myelotoxicity, peripheral neuropathy et al. (Galanski, 2006; Samimi et al., 2007). Therefore, researchers have turned their attention to other potential metal antitumor drugs. Ruthenium complexes have shown remarkable antitumor activity among the numerous metal compounds studied; they possess various advantages over platinum drugs, such as potent efficacy, low toxicity, less drug resistance, and are expected to become a new generation of clinical metal antitumor drugs (Abid et al., 2016; Thota, 2016; Southam et al., 2017).
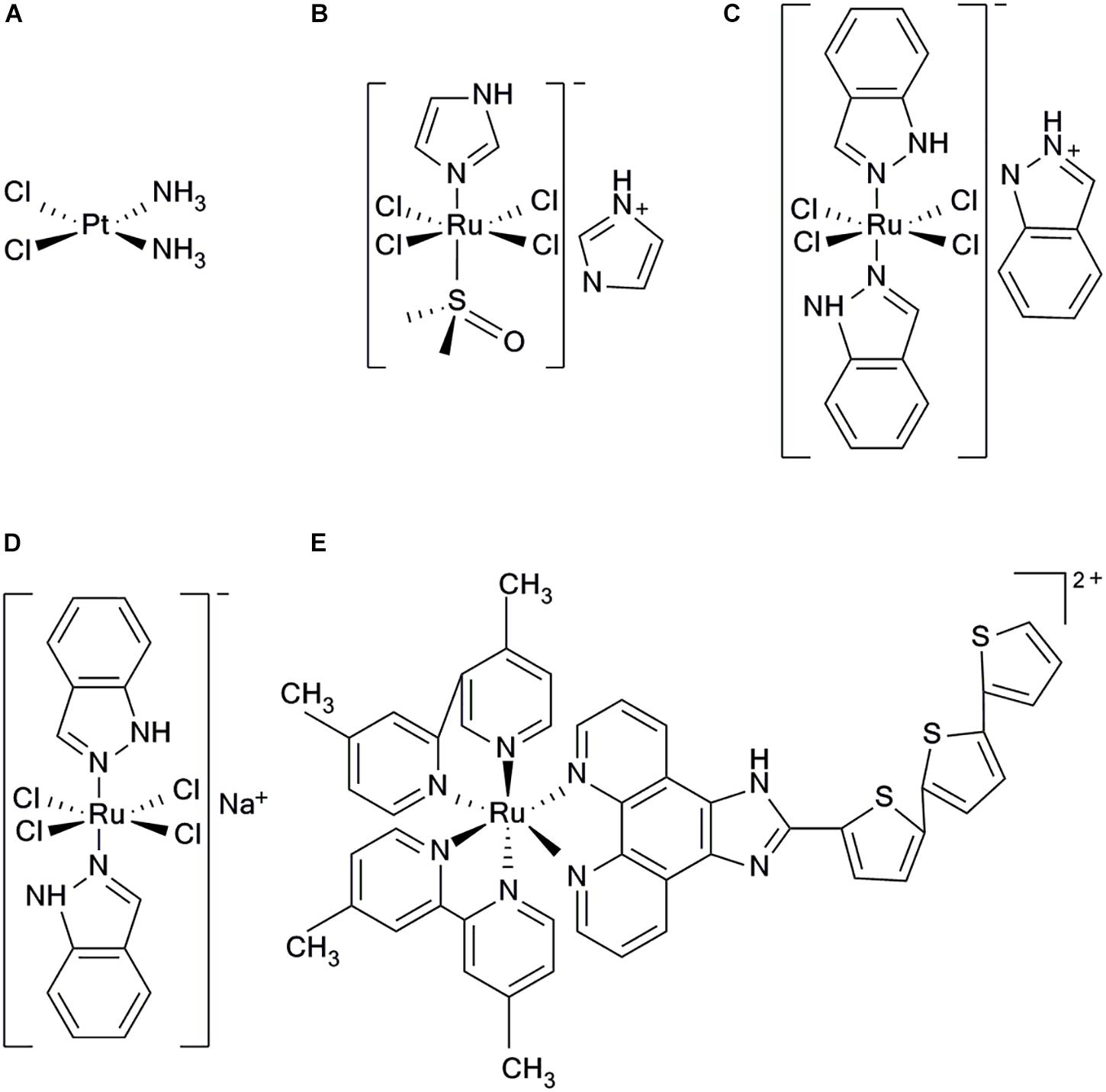
FIGURE 1. Structure of five clinical complexes; (A) Cisplatinum, (B) NAMI-A, (C) KP1019, (D) KP1339, and (E) TLD1443.
There are three main oxidation states of ruthenium compounds. The high oxidation state of Ru(IV) compound is unstable, which limited its further development (Duan et al., 2009). Ru(III) complexes have good stability of thermodynamics and kinetics, and can be used as prodrugs under biological circumstances of hypoxia, acidic pH and high level glutathione, showing antitumor effect by reducing to corresponding Ru(II) counterparts in vivo (Minchinton and Tannock, 2006; Antonarakis and Emadi, 2010). Ru(II) can directly kill tumor cells via multiple mechanisms (Zeng et al., 2015). Ru(II) complexes have great photophysical and chemical properties as well as multiple exchanging ligands. Combining with their applicability as nanomaterials and they have demonstrated significant antitumor efficacy (Poynton et al., 2017). Generally, the thermodynamic and kinetic stability of Ru(II) compounds are higher than Ru(III) due to their lower oxidation states (Duan et al., 2009). In addition, the nature and net charge of the ligands play important roles in the kinetics of Ru(II) compounds hydration (Abid et al., 2016). Many Ru(II) compounds showed better antitumor activities than their corresponding Ru(III) counterpart in vivo (Minchinton and Tannock, 2006; Hartinger et al., 2013). Generally speaking, the following options are viable in improving the water solubility of ruthenium compounds. (i) modifying the ligand structures; (ii) constructing the supramolecular ruthenium compounds; (iii) encapsulating ruthenium compounds into nanomaterial systems. (Suss-Fink, 2010; Jiang et al., 2012; Schmitt et al., 2012).
All the following ruthenium complexes that have progressed to clinical studies, NAMI-A {ImH[trans-RuCl4(dmso) (imidazole)]} (Figure 1B), KP1019 {indazolium trans-[tetrachlorobis(1H-indazole)ruthenate(III)]} (Figure 1C), and KP1339, are Ru(III) complexes (Webb et al., 2013). NAMI-A showed potent inhibitory efficacy on tumor metastasis. However, the phase II clinical studies revealed that it caused severe side effects in patients and, therefore, further investigations were not undertaken (Bergamo et al., 2003; Alessio et al., 2004). KP1019 had also failed to be investigated because of its poor water solubility, severe side effects and unsatisfactory efficacy for clinical study, (Hartinger et al., 2006, 2008). To improve the low water solubility of KP1019, researchers designed a more soluble sodium salt complex, KP1339 [Na(trans-RuCl4 (Ind)2)] (Figure 1D), which is currently used in clinical studies (Heffeter et al., 2010). Using the potent photophysical and chemical properties of Ru(II) complex, researchers have synthesized a photosensitizer TLD1443 (Figure 1E), which has immensely enhanced photodynamic therapy (Zeng et al., 2017a). It has a significant therapeutic efficacy on bladder cancer and is currently in phase II clinical trials (Smithen et al., 2017).
Based on the characteristics of ruthenium compound, optimizing its structure with relevant modification is a good strategy to improve its targeting capability and antitumor activity (Blanck et al., 2012). Researchers designed a series of lipophilic ruthenium complexes that effectively increase the uptake efficiency of tumor cells (Svensson et al., 2010; Matson et al., 2011). They found that the difference in the length of alkyl ether chains contributed to the different organelle-targeting properties of ruthenium complexes. Coupling of targeted polypeptides with ruthenium complexes is another effective way to enhance their targeting capability (Chakrabortty et al., 2017). In addition, encapsulating ruthenium complexes into nanomaterials can improve their targeting capability through the enhanced permeation and retention (EPR) effect (Frasconi et al., 2013; Wei et al., 2015). Capitalizing the properties of Ru(II) complexes, researchers have designed a series of nanoruthenium complexes including, Ru(II)-selenium nanoparticles (Sun et al., 2013), Ru(II)-gold nanocomplexes (Rogers et al., 2014), Ru(II)-silicon nanocomplexes (Frasconi et al., 2013), Ru(II)-carbon nanotubes (Wang N. et al., 2015), and some organic and biometallic nanoruthenium complexes (Chakrabortty et al., 2017) with direct antitumor effects. These nanoruthenium complexes can also be used as a good catalyst, photosensitizer and tracer to enhance the therapeutic effect (Chakrabortty et al., 2017).
Antitumor Targets and Mechanisms of Ruthenium Complexes
Ruthenium complexes show multiple targets and diverse mechanisms for its antitumor properties (Figure 2). Some ruthenium complexes act on telomere DNA, some interfere with replication and transcription of DNA, and others inhibit related enzymes (Kurzwernhart et al., 2012; Jain et al., 2018). Furthermore, ruthenium complexes can block the cell cycle (Kou et al., 2012; Wang et al., 2016; De Carvalho et al., 2018) and induce the formation of DNA photocrosslinking products to prevent RNA polymerization enzymes or exonucleases from binding to DNA, thereby causing tumor cell apoptosis (Le Gac et al., 2009; Rickling et al., 2010). Studies have found that some dinuclear and polynuclear Ru(II) polypyridyl complexes bind stably to the G-quadruplex (G4-DNA) structure of telomere DNA (Hiyama et al., 1995; Ambrus et al., 2006), inhibiting telomerase activity and blocking the function of DNA replication, thus, preventing normal cells from developing into immortalized tumor cells (Rajput et al., 2006; Shi et al., 2008). Ruthenium complexes have good topoisomerase (Topo) inhibitory activity (Kurzwernhart et al., 2012); however, some studies have found that inhibition of one type of Topo increases the activity of others (Crump et al., 1999; Vey et al., 1999). To solve this problem, studies have been conducted to synthesize a ruthenium complex with dual inhibitory property on Topo I and Topo II, which significantly inhibits tumor cell proliferation (Du et al., 2011; Zhang et al., 2013). Researchers have also designed a ruthenium complex with dual inhibitory effects on G4-DNA and Topo (Liao et al., 2015), achieving multitarget synergy with strong apoptosis promoting effects on tumor cells. In addition, Hurley and co-workers reported a ruthenium complex with dual stabilizing effects on Topo and G4-DNA, which also inhibited some drug resistant tumor cells (Kim et al., 2003).
In addition, it was found that ruthenium complexes accumulate more in organelles, such as mitochondria, endoplasmic reticulum, and lysosome, than in nucleus (Puckett and Barton, 2007; Groessl et al., 2011). A number of studies have revealed that mitochondria is a key target of ruthenium complexes (Wang et al., 2014; Liu et al., 2015; Wan et al., 2017), because ruthenium complexes can quickly decrease the membrane potential of mitochondria, leading to mitochondrial dysfunction or activating mitochondrial apoptosis pathways. Furthermore, this effect promoted the expression of pro-apoptotic members of the B-cell lymphoma-2 (Bcl-2) family, releasing cytochrome c (Cyto C), and activating cascade reactions of the caspase family members to induce tumor cell apoptosis. The endoplasmic reticulum is a key participant in tumor cell apoptosis, autophagy, and drug resistance and, thus, is a target in antitumor research (Sano et al., 2012; Fernandez et al., 2015). Ruthenium complexes can target the endoplasmic reticulum, cause oxidative stress or endoplasmic reticulum stress (ERS), and induce tumor cell apoptosis by activating caspase family members (Gill et al., 2013; Sano and Reed, 2013). In addition, ruthenium complexes can target another significant participant in autophagy, the lysosomes, inducing autolysosome production and hydrolase release (Tan et al., 2010; Castonguay et al., 2012; Chen et al., 2016). Thereby, they increase apoptosis of tumor cells (Yuan et al., 2015).
A very important feature of ruthenium complexes is that it is effective against many platinum resistant tumors. Gasser et al. found that [Ru(dppz)2(CppH)]2+ (CppH = 2-(20-pyridyl)-pyrimidine-4-carboxylic acid)] accumulated in the mitochondria. Moreover, this Ru(II) complex showed more cytotoxic effect in cisplatin-resistant A2780/CP70 cells than cisplatin and less cytotoxic than cisplatin in normal MRC-5 cells (Pierroz et al., 2012). Dyson and co-workers also designed some ruthenium complexes which contained ethacrynic acid (EA) ligands that inhibited cisplatin resistant A2780cisR cells (Ang et al., 2007). Moreover, Chao’s and Chen’s group designed a series of mitochondria-targeted Ru(II) complexes, based on a 2-phenylimidazo[4,5-f] [1,10]phenanthroline (PIP) Ru(II) polypyridyl complexes. These complexes induced apoptosis via a mitochondrial pathway and were effective against cisplatin resistant tumor cells (Li et al., 2012c; Wang et al., 2014; Yu et al., 2014).
The membrane structure as a “protective barrier” not only regulates the entry of drug molecules into cells, but also acts as a direct target of drug molecules, effectively killing tumor cells. A number of studies have confirmed that ruthenium complexes directly act on cell membrane, changing its permeability to allow cellular content to flow out of cells and induce cell apoptosis (Deng et al., 2017). Using the photophysical properties of Ru(II) complexes, researchers designed a Ru(II) polypyridine complex that accumulates on mitochondrial membrane and tumor surface membrane. These complexes emit red phosphorescence and produce a large amount of 1O2, thereby causing cytotoxicity and inducing cell apoptosis (Hess et al., 2017; Pal et al., 2018). Chao and colleagues synthesized Ru(II) pyridine complexes with two-photon performance and 1O2 yield, which could serve as a photosensitizer to simultaneously target surface membrane and mitochondrial membrane of human cervical carcinoma (HeLa) cells, achieving a dual killing effect (Qiu et al., 2017).
The Use of Ruthenium Complexes in Diagnosis and Treatment of Tumors
The effective diagnosis and treatment of tumors is a major clinical challenge. Ruthenium complexes have shown promising application prospects to this difficulty. The combination of development and applications of subcellular targeting probes and bio-imaging technologies with the understanding of the occurrence and physiological development of tumors, is expected to facilitate the achievement of tumor-specific diagnosis and therapy. Ru(II) complexes have the advantages of considerable photothermal stability, large stokes shift, long luminescence lifetime, and low toxicity (Gill et al., 2009). They are ideal photosensitizers, catalysts, and imaging agents in phototherapy, and could serve as excellent probes and tracers for subcellular structure localization. Thomas and colleagues reported a lipophilic Ru(II) complex that can be used as a fluorescent probe, targeting the mitochondria and endoplasmic reticulum of human breast cancer cell (MCF-7), and it showed comparable cytotoxicity to that of cisplatin (Gill et al., 2013). In addition to targeting and imaging tumor subcellular structures, ruthenium complexes can also detect and specifically recognize biological components of the microenvironment. As a significant active ingredient in organisms, the level of thiol in tumor tissues can change rapidly. Specific recognition of the thiol level is important for tumor diagnosis and therapy (Dirican et al., 2016; Inal et al., 2017). The Ru(II)-gold nanocomplex synthesized by Chao and co-workers could be used as a specific two-photon probe for thiol level, as it detected biothiol levels in living HeLa cells and mouse hippocampus using two-photon microscopy, which provides a potent tool for molecular biology research in tumors (Zhang et al., 2014). The oxygen allotrope O2 is an indispensable source of metabolic energy and could be specifically identified and used to monitor the local metabolites of tumor cells, which would facilitate tumor diagnosis and therapy. Keyes and colleagues found that a peptide-bridged dinuclear Ru(II) complex as the mitochondrial fluorescent probe can monitor the dynamic changes of O2 concentration in mitochondria of HeLa cell, which could be used to monitor the malignant proliferation of tumor cells (Martin et al., 2014). The non-oxygen-dependent Ru(II) complex has been used as a photosensitizer in treating hypoxic tumors. This complex overcomes the limitations of low-depth-effect and low cell killing efficiency of phototherapy, significantly increasing 1O2 production and fluorescence efficiency, thus, enhancing cytotoxicity of ruthenium complex and showing potent therapeutic effects (Volker et al., 2014; Sadhu et al., 2015; Cuello-Garibo et al., 2017).
The development of DNA structure recognition and imaging probes enables us to understand the pathogenesis of cancer at the genetic level, which has enhanced the study of antitumor drugs. Using the optical switch effect of Ru(II) complex to DNA (Augustyn et al., 2007), a Ru(II) polypyridine complex as a DNA secondary structure recognition probe was designed. The Barton research team reported a selective Ru(II) complex for DNA mismatch detection and fluorescence localization, which effectively reduces the risk of carcinogenesis caused by base mismatches (McConnell et al., 2012). DNA bulge structures are caused by the DNA recombination process, which is likely to cause a frameshift mutation in DNA replication. This structure binds more tightly to DNA repair proteins than it does to normal double-stranded DNA, making the bulge structures a potential binding site for therapeutic drugs (Pieniazek et al., 2011). Keene and colleagues synthesized a series of binuclear Ru(II) complexes that selectively recognize and bind to DNA bulge structures via electrostatic interaction and zonal action, and have DNA-targeted repair function (Mulyana et al., 2011; Li et al., 2012a). Z-DNA induces gene deletion, translocation, and other instability (Dumat et al., 2016). Tridentate complexes, [Ru(tpy)(ptn)]2+ and [Ru(dmtpy)(ptn)]2+, were designed to induce Z-DNA transforms into a stable B-DNA dominant conformation, which effectively decreased the risk of mutations (Li et al., 2012b).
In addition to DNA imaging, some complexes were synthesized by coupling fluorescent Ru(II) complexes with histone deacetylase inhibitors (HDACIs). These complexes specifically recognize and image proteins (Kurzwernhart et al., 2012). Further investigation has found that it not only images and inhibits HDACs, but also produces a large amount of reactive oxygen species (ROS) under light irradiation, showing comparable cytotoxicity to that of cisplatin. Thus, it induces apoptosis of some tumor cells. Photoacoustic imaging (PA) is a novel imaging technique for tissue imaging based on optical absorption coefficients under the action of an imaging agent (Levi et al., 2014). Liu and co-workers used poly(nisopropylacrylamide) as a thermal response switch and [Ru(bpy)2(tip)]2+ as a photosensitizer in combination with gold nanomaterials to synthesize the Ru(II) complex pRu-pNIPAM@RBT (Chen et al., 2017). Under optical stimulation, this complex produces high heat and large amounts of ROS in tumor tissues, and it showed synergistic action in photothermal therapy (PTT) and photodynamic therapy (PDT) against tumors. Ruthenium complexes are good imaging agents for PA. Combination of infrared thermal imaging quantitative analysis and PA data, can be effectively used to distinguish healthy and tumor tissues, which has significantly improved the accuracy and efficiency of tumor therapy (Su et al., 2010).
At the organizational level, tumor cell proliferation and metastasis depend on adequate nutrient supply and angiogenesis. Therefore, blocking tumor angiogenesis is also a key strategy to inhibit tumor growth and migration (Gau et al., 2017). Studies have found that some ruthenium complexes have good antiangiogenic effects and effectively inhibit tumor growth (Silva Sousa et al., 2016). Liu and colleagues designed a fluorescent Ru(II)-selenium nanoparticles (Ru-SeNPs) that significantly inhibited the proliferation of liver carcinoma HepG2 cells. In vivo experiments in tumor bearing mice revealed that NAMI-A potently inhibited tumor angiogenesis and migration (Vacca et al., 2002). In another study, the nitric oxide synthase (NOS) pathway was found to play an important role in tumor angiogenesis (Chakraborty and Ain, 2017). Increasing NO levels is positively correlated with tumor growth and migration. Drugs that interfere with the NOS pathway can inhibit tumor angiogenesis. It has been observed that NAMI-A inhibits vascular endothelial growth factor (VEGF)-mediated angiogenesis in tumor tissues by scavenging NO (Morbidelli et al., 2003).
Synergistic Effect of Ruthenium Complexes
Drug combinations are common therapeutic strategies in clinical practices. Combinational drug molecules act on multiple targets and pathways simultaneously, which could enhance their synergistic effects, reduce dosage and side effects, and reduce the risk of drug resistance (Lehar et al., 2009). A ruthenium complex was combined with a second-line antitumor agent ketoconazole (KTZ) in hormone-refractory cancer therapy to form a RuCl2(KTZ)2 complex, which showed a favorable synergistic effect (Bozic et al., 2013). The combination of these two agents in a C8161 melanoma cell line significantly enhanced the expression of caspase-3 and promoted tumor cell apoptosis. Mechanistic studies have shown that RuCl2(KTZ)2 has mitochondrial targeting effects, releasing mitochondrial cytochrome c and activating superoxide dismutase (Mn-SOD), thereby facilitating apoptosis. In the melanoma (WM164) cell line, RuCl2(KTZ)2 displayed a stronger inhibitory effect on tumor cell growth than cisplatin, and induced apoptosis by activating poly-ADP ribose polymerase (PARP) fragmentation and the proapoptotic factor Bcl-2-associated X protein (Bax) expression. RuCl2(KTZ)2 acts on the P53 signaling pathway to effectively inhibit the proliferation of a variety of adherent tumor cells, and synergizes the anti-epidermal growth factor receptor (EGFR) inhibitor C225MAb to kill resistant spheroids (Gelfo et al., 2016).
Berger and colleagues studied the combinations of ruthenium complexes and first-line anticancer drugs. They found that the clinical drug, ruthenium complex KP1339 combined with multi-kinase inhibitor sorafenib was more effective in the therapy of hepatoma (Hep3B) than KP1339 or sorafenib alone (Heffeter et al., 2013). Specifically, the mean survival of patients was extended by 3.9-fold by the combination, whereas KP1339 and sorafenib alone extended it by 2.4-and 1.9-fold, respectively. The combination of both agents effectively inhibited sorafenib-resistant tumor cells. In-depth investigations have found that the combination substantially increased their intracellular accumulation and, thereby, interfered with the DNA synthesis process, rendering the cells unable to perform effective mitosis, and enhancing apoptosis induction.
In clinical studies, NAMI-A combined with gemcitabine, better inhibited the activity of non-small cell lung cancer cells and reduced tolerance compared with the use of gemcitabine alone, but the combination of both had significant side effects such as neutropenia, anemia, and renal impairment (Leijen et al., 2015). Sava and co-workers identified promising drug combinations with synergistic potential using high-throughput screening (Bergamo et al., 2015). NAMI-A and doxorubicin were shown to have a potent synergistic antitumor efficacy. NAMI-A effectively increased the accumulation of doxorubicin in breast carcinoma. In in vivo studies of mouse MCa mammary carcinoma, this combination increased inhibition of tumor metastasis by 70%, compared to the use of doxorubicin alone. In a lung metastasis preclinical tumor model in mice, both agents demonstrated promising synergistic effects (Marien et al., 2017). However, there were noticeable side effects when the maximum doses were used.
The tumor vasculature is poorly organized, resulting in extravascular permeation of drug molecules (Pries et al., 2010). In addition, the decreased blood flow and oxygen supply affects drug uptake, which is also a major obstacle to effective tumor therapy (Siemann and Horsman, 2015). Studies on the combination of ovarian carcinoma chemotherapeutic doxorubicin and a ruthenium complex RAPTA-C have demonstrated that this combination significantly promoted the apoptosis of A2780 ovarian carcinoma cells compared with either single drug alone (Weiss et al., 2015). Normalization of tumor vasculature induced by apoptosis reduces vascular extravasation, and provides adequate oxygen for oxygen-dependent phototherapy, achieving synergism (Goel et al., 2011). These studies provide valid evidence for the interaction between anti-angiogenesis and antitumor effects.
Conclusion and Perspectives
Investigation of the antitumor activity of ruthenium complexes has led to gratifying achievements and the identification of some promising antitumor compounds (Chen et al., 2016; Alves de Souza et al., 2017; Zeng et al., 2017b; Zhao et al., 2018). The ruthenium complex showed more potent activities than platinum drugs, and has a significant inhibitory effect on platinum-resistant tumor (Zeng et al., 2016). The peculiarity of ruthenium compounds suggests that the research methods used for investigating platinum-based drugs may not fully be applied in these agents, because the cytotoxic mechanisms of cisplatin and ruthenium are different. The primary target of cisplatin is DNA, but the target of some ruthenium complexes is mitochondria or endoplasmic reticulum. Although they can both regulate cell apoptosis and cell cycle, cisplatin induces a large number of genes related to DNA damage, P53 and apoptosis, while some ruthenium complexes facilitate the expression of oxidative stress and ER stress (Licona et al., 2017).
The existing research achievements should be combined with molecular biology and nanomaterials, applying the advantage of existing tools and methods to develop antitumor drugs with better therapeutic effects, based on these complexes. This prospect is extremely enlightening, and antitumor drugs with better efficacy than that of existing chemotherapeutic drugs, which are ineffective in treating certain tumors, could be developed. Furthermore, the prospective agents could be effective against tumors that have developed drug resistance for their potent efficacy (Wang N. et al., 2015; Purushothaman et al., 2018). The results of clinical studies should be reflectively considered in determining the reasons for the failure of the clinical investigations of NAMI-A and KP1019, which could lead to design drugs with less side effects, greater selectivity, and higher bioavailability. For example, KP1339, the sodium salt of KP1019, which is currently in clinical studies, has better water solubility and transmembrane absorption efficiency than KP1019 (Bytzek et al., 2016). The Ru(II) complex TLD1443, as a promising photosensitizer, significantly enhanced the efficacy of phototherapy and produced less toxicity in vitro and in vivo (Smithen et al., 2017).
Numerous breakthroughs have been made in the diagnosis and therapy of tumors using ruthenium complexes (Thota et al., 2018). As a probe, the ruthenium complex could be used for target localization and imaging of DNA, the mitochondria, endoplasmic reticulum, and lysosomes, achieving specific identification and dynamic monitoring of thiol and O2 in tumors (Martin et al., 2014; Zhang et al., 2014). As a tracer, it enhances the understanding of the physiological development of tumors at the genetic level (Wilson et al., 2016; Xu et al., 2016). As photosensitizers and catalysts, these complexes have significant synergistic effects with phototherapies such as PDT, PTT, and photoactivated chemotherapy (PACT) (Chen et al., 2017). The combination of ruthenium complexes and PA imaging technology has significantly improved the accuracy and effectiveness of tumor diagnosis and therapy (Chen et al., 2014). In the therapy of tumors using drug combinations, ruthenium complexes have shown favorable efficacy. The Ru(II) complex combined with KTZ significantly inhibited the proliferation of C8161 melanoma cells and directly killed cisplatin-resistant spheroids (Bozic et al., 2013). KP1339 combined with the first-line anticancer drug sorafenib for hepatic carcinoma, demonstrated a remarkable therapeutic effect (Heffeter et al., 2013). Furthermore, NAMI-A combined with gemcitabine enhanced the inhibitory effect on non-small cell lung cancer while NAMI-A combined with doxorubicin showed potent inhibitory effects on lung metastasis in vivo (Bergamo et al., 2015). RAPTA-C and doxorubicin showed synergistically enhanced therapeutic effects on ovarian cancer and some solid tumors (Weiss et al., 2015). However, studies on the synergistic effect of ruthenium complexes are rare, because there are some uncertain factors such as the mechanism of drug synergy and how to choose drugs that cooperate with ruthenium complexes (Zhao et al., 2013; Madani Tonekaboni et al., 2018).
In conclusion, the results of the investigations on drugs combinations with ruthenium complexes are currently unsatisfactory. Perhaps the development and use of high-throughput screening technology and algorithm analysis tools are a viable strategy to promote the study of drug synergistic effects (Aviolat et al., 2018).
Presently, the mechanism of action of ruthenium complexes is unclear, and further research is still needed. Before the ruthenium complex can be used clinically, numerous problems need to be addressed, including strategies to improve the hydrolysis of ruthenium complexes to achieve effective absorption and better metabolism, as well as enhance their cellular penetration to achieve targeted tumor cell death. Furthermore, methods to avoid and alleviate the side effects of ruthenium complexes, enhance their efficacy via synergism, and overcome drug resistance are imperative. The solution to these problems would provide a promising direction for the design and screening of ruthenium complexes, which are of great significance for their use in clinical diagnosis and therapy of tumors.
Author Contributions
KL and Z-ZZ drafted and wrote the manuscript. J-QW conceived the idea for the manuscript. H-BB and X-JH provided critical analysis and language editing. All authors contributed to the writing and final approval of the manuscript.
Funding
This work was supported by the National Science Foundation of China (No. 21771042) and Natural Science Foundation of Guangdong Province (No. 2016A030310298).
Conflict of Interest Statement
The authors declare that the research was conducted in the absence of any commercial or financial relationships that could be construed as a potential conflict of interest.
References
Abid, M., Shamsi, F., and Azam, A. (2016). Ruthenium complexes: an emerging ground to the development of metallopharmaceuticals for cancer therapy. Mini Rev. Med. Chem. 16, 772–786. doi: 10.2174/1389557515666151001142012
Alessio, E., Mestroni, G., Bergamo, A., and Sava, G. (2004). Ruthenium antimetastatic agents. Curr. Top. Med. Chem. 4, 1525–1535. doi: 10.2174/1568026043387421
Alves de Souza, C. E., Alves, de Souza, H. M., Stipp, M. C., Corso, C. R., Galindo, C. M., et al. (2017). Ruthenium complex exerts antineoplastic effects that are mediated by oxidative stress without inducing toxicity in Walker-256 tumor-bearing rats. Free Radic. Biol. Med. 110, 228–239. doi: 10.1016/j.freeradbiomed.2017.06.011
Ambrus, A., Chen, D., Dai, J., Bialis, T., Jones, R. A., and Yang, D. (2006). Human telomeric sequence forms a hybrid-type intramolecular G-quadruplex structure with mixed parallel/antiparallel strands in potassium solution. Nucleic Acids Res. 34, 2723–2735. doi: 10.1093/nar/gkl348
Ang, W. H., De Luca, A., Chapuis-Bernasconi, C., Juillerat-Jeanneret, L., Lo Bello, M., and Dyson, P. J. (2007). Organometallic ruthenium inhibitors of glutathione-S-transferase P1-1 as anticancer drugs. Chem. Med. Chem. 2, 1799–1806. doi: 10.1002/cmdc.200700209
Antonarakis, E. S., and Emadi, A. (2010). Ruthenium-based chemotherapeutics: are they ready for prime time? Cancer Chemother. Pharmacol. 66, 1–9. doi: 10.1007/s00280-010-1293-1
Augustyn, K. E., Stemp, E. D., and Barton, J. K. (2007). Charge separation in a ruthenium-quencher conjugate bound to DNA. Inorg. Chem. 46, 9337–9350. doi: 10.1021/ic701276t
Aviolat, H., Nomine, Y., Gioria, S., Bonhoure, A., Hoffmann, D., Ruhlmann, C., et al. (2018). SynAggreg: a multifunctional high-throughput technology for precision study of amyloid aggregation and systematic discovery of synergistic inhibitor compounds. J. Mol. Biol. doi: 10.1016/j.jmb.2018.09.009 [Epub ahead of print].
Bergamo, A., Messori, L., Piccioli, F., Cocchietto, M., and Sava, G. (2003). Biological role of adduct formation of the ruthenium(III) complex NAMI-A with serum albumin and serum transferrin. Invest. New Drugs 21, 401–411. doi: 10.1023/A:1026243000320
Bergamo, A., Riedel, T., Dyson, P. J., and Sava, G. (2015). Preclinical combination therapy of the investigational drug NAMI-A+ with doxorubicin for mammary cancer. Invest. New Drugs 33, 53–63. doi: 10.1007/s10637-014-0175-5
Blanck, S., Maksimoska, J., Baumeister, J., Harms, K., Marmorstein, R., and Meggers, E. (2012). The art of filling protein pockets efficiently with octahedral metal complexes. Angew. Chem. Int. Ed. Engl. 51, 5244–5246. doi: 10.1002/anie.201108865
Bozic, I., Reiter, J. G., Allen, B., Antal, T., Chatterjee, K., Shah, P., et al. (2013). Evolutionary dynamics of cancer in response to targeted combination therapy. eLife 2:e00747. doi: 10.7554/eLife.00747
Burger, H., Loos, W. J., Eechoute, K., Verweij, J., Mathijssen, R. H., and Wiemer, E. A. (2011). Drug transporters of platinum-based anticancer agents and their clinical significance. Drug Resist. Updat. 14, 22–34. doi: 10.1016/j.drup.2010.12.002
Bytzek, A. K., Koellensperger, G., Keppler, B. K., and G Hartinger, C. (2016). Biodistribution of the novel anticancer drug sodium trans-[tetrachloridobis(1H-indazole)ruthenate(III)] KP-1339/IT139 in nude BALB/c mice and implications on its mode of action. J. Inorg. Biochem. 160, 250–255. doi: 10.1016/j.jinorgbio.2016.02.037
Castonguay, A., Doucet, C., Juhas, M., and Maysinger, D. (2012). New ruthenium(II)–letrozole complexes as anticancer therapeutics. J. Med. Chem. 55, 8799–8806. doi: 10.1021/jm301103y
Chakrabortty, S., Agrawalla, B. K., Stumper, A., Vegi, N. M., Fischer, S., Reichardt, C., et al. (2017). Mitochondria targeted protein-ruthenium photosensitizer for efficient photodynamic applications. J. Am. Chem. Soc. 139, 2512–2519. doi: 10.1021/jacs.6b13399
Chakraborty, S., and Ain, R. (2017). Nitric-oxide synthase trafficking inducer is a pleiotropic regulator of endothelial cell function and signaling. J. Biol. Chem. 292, 6600–6620. doi: 10.1074/jbc.M116.742627
Chen, G., Xu, M., Zhao, S., Sun, J., Yu, Q., and Liu, J. (2017). Pompon-like RuNPs-Based theranostic nanocarrier system with stable photoacoustic imaging characteristic for accurate tumor detection and efficient phototherapy guidance. ACS Appl. Mater. Interfaces 9, 33645–33659. doi: 10.1021/acsami.7b10553
Chen, L., Li, G., Peng, F., Jie, X., Dongye, G., Cai, K., et al. (2016). The induction of autophagy against mitochondria-mediated apoptosis in lung cancer cells by a ruthenium (II) imidazole complex. Oncotarget 7, 80716–80734. doi: 10.18632/oncotarget.13032
Chen, Y., Lei, W., Jiang, G., Hou, Y., Li, C., Zhang, B., et al. (2014). Fusion of photodynamic therapy and photoactivated chemotherapy: a novel Ru(II) arene complex with dual activities of photobinding and photocleavage toward DNA. Dalton Trans. 43, 15375–15384. doi: 10.1039/c4dt01755b
Crump, M., Lipton, J., Hedley, D., Sutton, D., Shepherd, F., Minden, M., et al. (1999). Phase I trial of sequential topotecan followed by etoposide in adults with myeloid leukemia: a national cancer Institute of Canada clinical trials group study. Leukemia 13, 343–347. doi: 10.1038/sj.leu.2401308
Cuello-Garibo, J. A., Meijer, M. S., and Bonnet, S. (2017). To cage or to be caged? The cytotoxic species in ruthenium-based photoactivated chemotherapy is not always the metal. Chem. Commun. 53, 6768–6771. doi: 10.1039/c7cc03469e
De Carvalho, N. C., Neves, S. P., Dias, R. B., Valverde, L. F., Sales, C. B. S., Rocha, C. A. G., et al. (2018). A novel ruthenium complex with xanthoxylin induces S-phase arrest and causes ERK1/2-mediated apoptosis in HepG2 cells through a p53-independent pathway. Cell Death Dis. 9:79. doi: 10.1038/s41419-017-0104-6
Deng, Z., Gao, P., Yu, L., Ma, B., You, Y., Chan, L., et al. (2017). Ruthenium complexes with phenylterpyridine derivatives target cell membrane and trigger death receptors-mediated apoptosis in cancer cells. Biomaterials 129, 111–126. doi: 10.1016/j.biomaterials.2017.03.017
Dirican, N., Dirican, A., Sen, O., Aynali, A., Atalay, S., Bircan, H. A., et al. (2016). Thiol/disulfide homeostasis: a prognostic biomarker for patients with advanced non-small cell lung cancer? Redox. Rep. 21, 197–203. doi: 10.1179/1351000215y.0000000027
Du, K. J., Wang, J. Q., Kou, J. F., Li, G. Y., Wang, L. L., Chao, H., et al. (2011). Synthesis, DNA-binding and topoisomerase inhibitory activity of ruthenium(II) polypyridyl complexes. Eur. J. Med. Chem. 46, 1056–1065. doi: 10.1016/j.ejmech.2011.01.019
Duan, L., Fischer, A., Xu, Y., and Sun, L. (2009). Isolated seven-coordinate Ru(IV) dimer complex with [HOHOH]- bridging ligand as an intermediate for catalytic water oxidation. J. Am. Chem. Soc. 131, 10397–10399. doi: 10.1021/ja9034686
Dumat, B., Larsen, A. F., and Wilhelmsson, L. M. (2016). Studying Z-DNA and B- to Z-DNA transitions using a cytosine analogue FRET-pair. Nucleic Acids Res. 44:e101. doi: 10.1093/nar/gkw114
Fernandez, A., Ordonez, R., Reiter, R. J., Gonzalez-Gallego, J., and Mauriz, J. L. (2015). Melatonin and endoplasmic reticulum stress: relation to autophagy and apoptosis. J. Pineal. Res. 59, 292–307. doi: 10.1111/jpi.12264
Frasconi, M., Liu, Z., Lei, J., Wu, Y., Strekalova, E., Malin, D., et al. (2013). Photoexpulsion of surface-grafted ruthenium complexes and subsequent release of cytotoxic cargos to cancer cells from mesoporous silica nanoparticles. J. Am. Chem. Soc. 135, 11603–11613. doi: 10.1021/ja405058y
Galanski, M. (2006). Recent developments in the field of anticancer platinum complexes. Recent Pat. Anticancer Drug Discov. 1, 285–295. doi: 10.2174/157489206777442287
Gasser, G., Sosniak, A. M., and Metzler-Nolte, N. (2011). Metal-containing peptide nucleic acid conjugates. Dalton Trans. 40, 7061–7076. doi: 10.1039/c0dt01706j
Gau, D., Veon, W., Capasso, T. L., Bottcher, R., Shroff, S., Roman, B. L., et al. (2017). Pharmacological intervention of MKL/SRF signaling by CCG-1423 impedes endothelial cell migration and angiogenesis. Angiogenesis 20, 663–672. doi: 10.1007/s10456-017-9560-y
Gelfo, V., Rodia, M. T., Pucci, M., Dall’Ora, M., Santi, S., Solmi, R., et al. (2016). A module of inflammatory cytokines defines resistance of colorectal cancer to EGFR inhibitors. Oncotarget 7, 72167–72183. doi: 10.18632/oncotarget.12354
Gill, M. R., Cecchin, D., Walker, M. G., Mulla, R. S., Battaglia, G., Smythe, C., et al. (2013). Targeting the endoplasmic reticulum with a membrane-interactive luminescent ruthenium(ii) polypyridyl complexdagger Electronic supplementary information (ESI) available: experimental details, characterization of 2 and Fig. S1-S6. See doi: 10.1039/c3sc51725jClick here for additional data file. Chem. Sci. 4, 4512–4519. doi: 10.1039/c3sc51725j
Gill, M. R., Garcia-Lara, J., Foster, S. J., Smythe, C., Battaglia, G., and Thomas, J. A. (2009). A ruthenium(II) polypyridyl complex for direct imaging of DNA structure in living cells. Nat. Chem. 1, 662–667. doi: 10.1038/nchem.406
Goel, S., Duda, D. G., Xu, L., Munn, L. L., Boucher, Y., Fukumura, D., et al. (2011). Normalization of the vasculature for treatment of cancer and other diseases. Physiol. Rev. 91, 1071–1121. doi: 10.1152/physrev.00038.2010
Groessl, M., Zava, O., and Dyson, P. J. (2011). Cellular uptake and subcellular distribution of ruthenium-based metallodrugs under clinical investigation versus cisplatin. Metallomics 3, 591–599. doi: 10.1039/c0mt00101e
Harper, B. W., Krause-Heuer, A. M., Grant, M. P., Manohar, M., Garbutcheon-Singh, K. B., and Aldrich-Wright, J. R. (2010). Advances in platinum chemotherapeutics. Chemistry 16, 7064–7077. doi: 10.1002/chem.201000148
Hartinger, C. G., Groessl, M., Meier, S. M., Casini, A., and Dyson, P. J. (2013). Application of mass spectrometric techniques to delineate the modes-of-action of anticancer metallodrugs. Chem. Soc. Rev. 42, 6186–6199. doi: 10.1039/c3cs35532b
Hartinger, C. G., Jakupec, M. A., Zorbas-Seifried, S., Groessl, M., Egger, A., Berger, W., et al. (2008). KP1019, a new redox-active anticancer agent–preclinical development and results of a clinical phase I study in tumor patients. Chem. Biodivers. 5, 2140–2155. doi: 10.1002/cbdv.200890195
Hartinger, C. G., Zorbas-Seifried, S., Jakupec, M. A., Kynast, B., Zorbas, H., and Keppler, B. K. (2006). From bench to bedside–preclinical and early clinical development of the anticancer agent indazolium trans-[tetrachlorobis(1H-indazole)ruthenate(III)] (KP1019 or FFC14A). J. Inorg. Biochem. 100, 891–904. doi: 10.1016/j.jinorgbio.2006.02.013
Heffeter, P., Atil, B., Kryeziu, K., Groza, D., Koellensperger, G., Korner, W., et al. (2013). The ruthenium compound KP1339 potentiates the anticancer activity of sorafenib in vitro and in vivo. Eur. J. Cancer 49, 3366–3375. doi: 10.1016/j.ejca.2013.05.018
Heffeter, P., Bock, K., Atil, B., Reza Hoda, M. A., Korner, W., Bartel, C., et al. (2010). Intracellular protein binding patterns of the anticancer ruthenium drugs KP1019 and KP1339. J. Biol. Inorg. Chem. 15, 737–748. doi: 10.1007/s00775-010-0642-1
Hess, J., Huang, H., Kaiser, A., Pierroz, V., Blacque, O., Chao, H., et al. (2017). Evaluation of the medicinal potential of two ruthenium(II) polypyridine complexes as one- and two-photon photodynamic therapy photosensitizers. Chemistry 23, 9888–9896. doi: 10.1002/chem.201701392
Hiyama, E., Hiyama, K., Yokoyama, T., Matsuura, Y., Piatyszek, M. A., and Shay, J. W. (1995). Correlating telomerase activity levels with human neuroblastoma outcomes. Nat. Med. 1, 249–255. doi: 10.1038/nm0395-249
Inal, B. B., Emre, H. O., Baran, O., Ahmedov, M., Ozdemir, A. F., Kemerdere, R., et al. (2017). Dynamic thiol-disulphide homeostasis in low-grade gliomas: preliminary results in serum. Clin. Neurol. Neurosurg. 161, 17–21. doi: 10.1016/j.clineuro.2017.08.002
Jain, S. S., Anderson, C. M., Sapse, I. A., Lundgren, S. H., Freer, A. K., Hoang, H., et al. (2018). A ruthenium-platinum metal complex that binds to sarcin ricin loop RNA and lowers mRNA expression. Chem. Commun. 54, 8987–8990. doi: 10.1039/c8cc02131g
Jakupec, M. A., Galanski, M., Arion, V. B., Hartinger, C. G., and Keppler, B. K. (2008). Antitumour metal compounds: more than theme and variations. Dalton Trans. 183–194. doi: 10.1039/b712656p
Jiang, Q., Song, C., Nangreave, J., Liu, X., Lin, L., Qiu, D., et al. (2012). DNA origami as a carrier for circumvention of drug resistance. J. Am. Chem. Soc. 134, 13396–13403. doi: 10.1021/ja304263n
Kim, M. Y., Duan, W., Gleason-Guzman, M., and Hurley, L. H. (2003). Design, synthesis, and biological evaluation of a series of fluoroquinoanthroxazines with contrasting dual mechanisms of action against topoisomerase II and G-quadruplexes. J. Med. Chem. 46, 571–583. doi: 10.1021/jm0203377
Kou, J. F., Qian, C., Wang, J. Q., Chen, X., Wang, L. L., Chao, H., et al. (2012). Chiral ruthenium(II) anthraquinone complexes as dual inhibitors of topoisomerases I and II. J. Biol. Inorg. Chem. 17, 81–96. doi: 10.1007/s00775-011-08316
Kurzwernhart, A., Kandioller, W., Bartel, C., Bachler, S., Trondl, R., Muhlgassner, G., et al. (2012). Targeting the DNA-topoisomerase complex in a double-strike approach with a topoisomerase inhibiting moiety and covalent DNA binder. Chem. Commun. 48, 4839–4841. doi: 10.1039/c2cc31040f
Le Gac, S., Rickling, S., Gerbaux, P., Defrancq, E., Moucheron, C., and Kirsch-De Mesmaeker, A. (2009). A photoreactive ruthenium(II) complex tethered to a guanine-containing oligonucleotide: a biomolecular tool that behaves as a “seppuku molecule”. Angew. Chem. Int. Ed. Engl. 48, 1122–1125. doi: 10.1002/anie.200804503
Lehar, J., Krueger, A. S., Avery, W., Heilbut, A. M., Johansen, L. M., Price, E. R., et al. (2009). Synergistic drug combinations tend to improve therapeutically relevant selectivity. Nat. Biotechnol. 27, 659–666. doi: 10.1038/nbt.1549
Leijen, S., Burgers, S. A., Baas, P., Pluim, D., Tibben, M., van Werkhoven, E., et al. (2015). Phase I/II study with ruthenium compound NAMI-A and gemcitabine in patients with non-small cell lung cancer after first line therapy. Invest. New Drugs 33, 201–214. doi: 10.1007/s10637-014-0179-1
Levi, J., Sathirachinda, A., and Gambhir, S. S. (2014). A high-affinity, high-stability photoacoustic agent for imaging gastrin-releasing peptide receptor in prostate cancer. Clin. Cancer Res. 20, 3721–3729. doi: 10.1158/1078-0432.ccr-13-3405
Li, F., Weber, D. K., Morgan, J. L., Collins, J. G., and Keene, F. R. (2012a). An approach to therapeutic agents through selective targeting of destabilised nucleic acid duplex sequences. Dalton Trans. 41, 6528–6535. doi: 10.1039/c2dt12146h
Li, L. Y., Jia, H. N., Yu, H. J., Du, K. J., Lin, Q. T., Qiu, K. Q., et al. (2012b). Synthesis, characterization, and DNA-binding studies of ruthenium complexes [Ru(tpy)(ptn)]2+ and Ru(dmtpy)(ptn)]2+. J. Inorg. Biochem. 113, 31–39. doi: 10.1016/j.jinorgbio.2012.03.008
Li, L., Wong, Y. S., Chen, T., Fan, C., and Zheng, W. (2012c). Ruthenium complexes containing bis-benzimidazole derivatives as a new class of apoptosis inducers. Dalton Trans. 41, 1138–1141. doi: 10.1039/c1dt11950h
Liao, G., Chen, X., Wu, J., Qian, C., Wang, Y., Ji, L., et al. (2015). Ruthenium(II) polypyridyl complexes as dual inhibitors of telomerase and topoisomerase. Dalton Trans. 44, 15145–15156. doi: 10.1039/c4dt03585b
Licona, C., Spaety, M. E., Capuozzo, A., Ali, M., Santamaria, R., Armant, O., et al. (2017). A ruthenium anticancer compound interacts with histones and impacts differently on epigenetic and death pathways compared to cisplatin. Oncotarget 8, 2568–2584. doi: 10.18632/oncotarget.13711
Liu, J., Chen, Y., Li, G., Zhang, P., Jin, C., Zeng, L., et al. (2015). Ruthenium(II) polypyridyl complexes as mitochondria-targeted two-photon photodynamic anticancer agents. Biomaterials 56, 140–153. doi: 10.1016/j.biomaterials.2015.04.002
Madani Tonekaboni, S. A., Soltan Ghoraie, L., Manem, V. S. K., and Haibe-Kains, B. (2018). Predictive approaches for drug combination discovery in cancer. Brief Bioinform. 19, 263–276. doi: 10.1093/bib/bbw104
Marien, E., Hillen, A., Vanderhoydonc, F., Swinnen, J. V., and Vande Velde, G. (2017). Longitudinal microcomputed tomography-derived biomarkers for lung metastasis detection in a syngeneic mouse model: added value to bioluminescence imaging. Lab. Invest. 97, 24–33. doi: 10.1038/labinvest.2016.114
Martin, A., Byrne, A., Burke, C. S., Forster, R. J., and Keyes, T. E. (2014). Peptide-bridged dinuclear Ru(II) complex for mitochondrial targeted monitoring of dynamic changes to oxygen concentration and ROS generation in live mammalian cells. J. Am. Chem. Soc. 136, 15300–15309. doi: 10.1021/ja508043q
Matson, M., Svensson, F. R., Norden, B., and Lincoln, P. (2011). Correlation between cellular localization and binding preference to RNA, DNA, and phospholipid membrane for luminescent ruthenium(II) complexes. J. Phys. Chem. B 115, 1706–1711. doi: 10.1021/jp109530f
McConnell, A. J., Lim, M. H., Olmon, E. D., Song, H., Dervan, E. E., and Barton, J. K. (2012). Luminescent properties of ruthenium(II) complexes with sterically expansive ligands bound to DNA defects. Inorg. Chem. 51, 12511–12520. doi: 10.1021/ic3019524
Minchinton, A. I., and Tannock, I. F. (2006). Drug penetration in solid tumours. Nat. Rev. Cancer 6, 583–592. doi: 10.1038/nrc1893
Morbidelli, L., Donnini, S., Filippi, S., Messori, L., Piccioli, F., Orioli, P., et al. (2003). Antiangiogenic properties of selected ruthenium(III) complexes that are nitric oxide scavengers. Br. J. Cancer 88, 1484–1491. doi: 10.1038/sj.bjc.6600906
Mulyana, Y., Weber, D. K., Buck, D. P., Motti, C. A., Collins, J. G., and Keene, F. R. (2011). Oligonuclear polypyridylruthenium(II) complexes incorporating flexible polar and non-polar bridges: synthesis, DNA-binding and cytotoxicity. Dalton Trans. 40, 1510–1523. doi: 10.1039/c0dt01250e
Pal, M., Nandi, U., and Mukherjee, D. (2018). Detailed account on activation mechanisms of ruthenium coordination complexes and their role as antineoplastic agents. Eur. J. Med. Chem. 150, 419–445. doi: 10.1016/j.ejmech.2018.03.015
Pieniazek, S. N., Hingorani, M. M., and Beveridge, D. L. (2011). Dynamical allosterism in the mechanism of action of DNA mismatch repair protein MutS. Biophys. J. 101, 1730–1739. doi: 10.1016/j.bpj.2011.08.039
Pierroz, V., Joshi, T., Leonidova, A., Mari, C., Schur, J., Ott, I., et al. (2012). Molecular and cellular characterization of the biological effects of ruthenium(II) complexes incorporating 2-pyridyl-2-pyrimidine-4-carboxylic acid. J. Am. Chem. Soc. 134, 20376–20387. doi: 10.1021/ja307288s
Poynton, F. E., Bright, S. A., Blasco, S., Williams, D. C., Kelly, J. M., and Gunnlaugsson, T. (2017). The development of ruthenium(ii) polypyridyl complexes and conjugates for in vitro cellular and in vivo applications. Chem. Soc. Rev. 4, 7706–7756. doi: 10.1039/c7cs00680b
Pries, A. R., Hopfner, M., le Noble, F., Dewhirst, M. W., and Secomb, T. W. (2010). The shunt problem: control of functional shunting in normal and tumour vasculature. Nat. Rev. Cancer 10, 587–593. doi: 10.1038/nrc2895
Puckett, C. A., and Barton, J. K. (2007). Methods to explore cellular uptake of ruthenium complexes. J. Am. Chem. Soc. 129, 46–47. doi: 10.1021/ja0677564
Purushothaman, B., Arumugam, P., Ju, H., Kulsi, G., Samson, A. A. S., and Song, J. M. (2018). Novel ruthenium(II) triazine complex [Ru(bdpta)(tpy)]2+ co-targeting drug resistant GRP78 and subcellular organelles in cancer stem cells. Eur. J. Med. Chem. 156, 747–759. doi: 10.1016/j.ejmech.2018.07.048
Qiu, K., Wang, J., Song, C., Wang, L., Zhu, H., Huang, H., et al. (2017). Crossfire for two-pphoton photodynamic therapy with fluorinated ruthenium (II) photosensitizers. ACS Appl. Mater. Interfaces 9, 18482–18492. doi: 10.1021/acsami.7b02977
Rajput, C., Rutkaite, R., Swanson, L., Haq, I., and Thomas, J. A. (2006). Dinuclear monointercalating RuII complexes that display high affinity binding to duplex and quadruplex DNA. Chemistry 12, 4611–4619. doi: 10.1002/chem.200501349
Rickling, S., Ghisdavu, L., Pierard, F., Gerbaux, P., Surin, M., Murat, P., et al. (2010). A rigid dinuclear ruthenium(II) complex as an efficient photoactive agent for bridging two guanine bases of a duplex or quadruplex oligonucleotide. Chemistry 16, 3951–3961. doi: 10.1002/chem.200902817
Rogers, N. J., Claire, S., Harris, R. M., Farabi, S., Zikeli, G., Styles, I. B., et al. (2014). High coating of Ru(II) complexes on gold nanoparticles for single particle luminescence imaging in cells. Chem. Commun. 50, 617–619. doi: 10.1039/c3cc47606e
Sadhu, K. K., Lindberg, E., and Winssinger, N. (2015). In cellulo protein labelling with Ru-conjugate for luminescence imaging and bioorthogonal photocatalysis. Chem. Commun. 51, 16664–16666. doi: 10.1039/c5cc05405b
Samimi, G., Kishimoto, S., Manorek, G., Breaux, J. K., and Howell, S. B. (2007). Novel mechanisms of platinum drug resistance identified in cells selected for resistance to JM118 the active metabolite of satraplatin. Cancer Chemother. Pharmacol. 59, 301–312. doi: 10.1007/s00280-006-0271-0
Sano, R., Hou, Y. C., Hedvat, M., Correa, R. G., Shu, C. W., Krajewska, M., et al. (2012). Endoplasmic reticulum protein BI-1 regulates Ca2+-mediated bioenergetics to promote autophagy. Genes Dev. 26, 1041–1054. doi: 10.1101/gad.184325.111
Sano, R., and Reed, J. C. (2013). ER stress-induced cell death mechanisms. Biochim. Biophys. Acta 1833, 3460–3470. doi: 10.1016/j.bbamcr.2013.06.028
Schmitt, F., Freudenreich, J., Barry, N. P., Juillerat-Jeanneret, L., Suss-Fink, G., and Therrien, B. (2012). Organometallic cages as vehicles for intracellular release of photosensitizers. J. Am. Chem. Soc. 134, 754–757. doi: 10.1021/ja207784t
Shi, S., Liu, J., Yao, T., Geng, X., Jiang, L., Yang, Q., et al. (2008). Promoting the formation and stabilization of G-quadruplex by dinuclear RuII complex Ru2(obip)L4. Inorg. Chem. 47, 2910–2912. doi: 10.1021/ic7021209
Siemann, D. W., and Horsman, M. R. (2015). Modulation of the tumor vasculature and oxygenation to improve therapy. Pharmacol. Ther. 153, 107–124. doi: 10.1016/j.pharmthera.2015.06.006
Silva Sousa, E. H., Ridnour, L. A., Gouveia, F. S. Jr., Silva da Silva, C. D., Wink, D. A., de Franca Lopes, L. G., et al. (2016). Thiol-activated HNO release from a ruthenium antiangiogenesis complex and HIF-1alpha inhibition for cancer therapy. ACS Chem. Biol. 11, 2057–2065. doi: 10.1021/acschembio.6b00222
Smithen, D. A., Yin, H., Beh, M. H., Hetu, M., Cameron, T. S., McFarland, S. A., et al. (2017). Synthesis and photobiological activity of Ru(II) dyads derived from pyrrole-2-carboxylate thionoesters. Inorg. Chem. 56, 4121–4132. doi: 10.1021/acs.inorgchem.7b00072
Southam, H. M., Butler, J. A., Chapman, J. A., and Poole, R. K. (2017). The microbiology of ruthenium complexes. Adv. Microb. Physiol. 71, 1–96. doi: 10.1016/bs.ampbs.2017.03.001
Su, J. L., Wang, B., Wilson, K. E., Bayer, C. L., Chen, Y. S., Kim, S., et al. (2010). Advances in clinical and biomedical applications of photoacoustic imaging. Expert Opin. Med. Diagn. 4, 497–510. doi: 10.1517/17530059.2010.529127
Sun, D., Liu, Y., Yu, Q., Zhou, Y., Zhang, R., Chen, X., et al. (2013). The effects of luminescent ruthenium(II) polypyridyl functionalized selenium nanoparticles on bFGF-induced angiogenesis and AKT/ERK signaling. Biomaterials 34, 171–180. doi: 10.1016/j.biomaterials.2012.09.031
Suss-Fink, G. (2010). Arene ruthenium complexes as anticancer agents. Dalton Trans. 39, 1673–1688. doi: 10.1039/b916860p
Svensson, F. R., Matson, M., Li, M., and Lincoln, P. (2010). Lipophilic ruthenium complexes with tuned cell membrane affinity and photoactivated uptake. Biophys. Chem. 149, 102–106. doi: 10.1016/j.bpc.2010.04.006
Tan, C., Lai, S., Wu, S., Hu, S., Zhou, L., Chen, Y., et al. (2010). Nuclear permeable ruthenium(II) beta-carboline complexes induce autophagy to antagonize mitochondrial-mediated apoptosis. J. Med. Chem. 53, 7613–7624. doi: 10.1021/jm1009296
Thota, S. (2016). Editorial: anticancer ruthenium complexes in drug discovery and medicinal chemistry. Mini Rev. Med. Chem. 16:771. doi: 10.2174/138955751610160503003405
Thota, S., Rodrigues, D. A., Crans, D. C., and Barreiro, E. J. (2018). Ru(II) compounds: next-generation anticancer metallotherapeutics? J. Med. Chem. 61, 5805–5821. doi: 10.1021/acs.jmedchem.7b01689
Vacca, A., Bruno, M., Boccarelli, A., Coluccia, M., Ribatti, D., Bergamo, A., et al. (2002). Inhibition of endothelial cell functions and of angiogenesis by the metastasis inhibitor NAMI-A. Br. J. Cancer 86, 993–998. doi: 10.1038/sj.bjc.6600176
Vey, N., Kantarjian, H., Beran, M., O’Brien, S., Cortes, J., Koller, C., et al. (1999). Combination of topotecan with cytarabine or etoposide in patients with refractory or relapsed acute myeloid leukemia: results of a randomized phase I/II study. Invest. New Drugs 17, 89–95. doi: 10.1023/A:1006271618635
Volker, T., Dempwolff, F., Graumann, P. L., and Meggers, E. (2014). Progress towards bioorthogonal catalysis with organometallic compounds. Angew. Chem. Int. Ed. Engl. 53, 10536–10540. doi: 10.1002/anie.201404547
Wan, D., Tang, B., Wang, Y. J., Guo, B. H., Yin, H., Yi, Q. Y., et al. (2017). Synthesis and anticancer properties of ruthenium (II) complexes as potent apoptosis inducers through mitochondrial disruption. Eur. J. Med. Chem. 139, 180–190. doi: 10.1016/j.ejmech.2017.07.066
Wang, J. Q., Zhang, P. Y., Qian, C., Hou, X. J., Ji, L. N., and Chao, H. (2014). Mitochondria are the primary target in the induction of apoptosis by chiral ruthenium(II) polypyridyl complexes in cancer cells. J. Biol. Inorg. Chem. 19, 335–348. doi: 10.1007/s00775-013-1069-2
Wang, J.-Q., Zhao, Z.-Z., Bo, H.-B., and Chen, Q.-Z. (2016). Synthesis, characterization, and antitumor properties of ruthenium(II) anthraquinone complexes. J. Coord. Chem. 69, 177–189. doi: 10.1080/00958972.2015.1120291
Wang, N., Feng, Y., Zeng, L., Zhao, Z., and Chen, T. (2015). Functionalized multiwalled carbon nanotubes as carriers of ruthenium complexes to antagonize cancer multidrug resistance and radioresistance. ACS Appl. Mater. Interfaces 7, 14933–14945. doi: 10.1021/acsami.5b03739
Wang, X., Wang, X., and Guo, Z. (2015). Functionalization of platinum complexes for biomedical applications. Acc Chem. Res. 48, 2622–2631. doi: 10.1021/acs.accounts.5b00203
Wang, X., and Guo, Z. (2013). Targeting and delivery of platinum-based anticancer drugs. Chem. Soc. Rev. 42, 202–224. doi: 10.1039/c2cs35259a
Webb, M. I., Wu, B., Jang, T., Chard, R. A., Wong, E. W., Wong, M. Q., et al. (2013). Increasing the bioavailability of Ru(III) anticancer complexes through hydrophobic albumin interactions. Chemistry 19, 17031–17042. doi: 10.1002/chem.201302671
Wei, L., Lu, J., Xu, H., Patel, A., Chen, Z. S., and Chen, G. (2015). Silver nanoparticles: synthesis, properties, and therapeutic applications. Drug Discov. Today 20, 595–601. doi: 10.1016/j.drudis.2014.11.014
Weiss, A., Bonvin, D., Berndsen, R. H., Scherrer, E., Wong, T. J., Dyson, P. J., et al. (2015). Angiostatic treatment prior to chemo- or photodynamic therapy improves anti-tumor efficacy. Sci. Rep. 5:8990. doi: 10.1038/srep08990
Wilson, R., Espinosa-Diez, C., Kanner, N., Chatterjee, N., Ruhl, R., Hipfinger, C., et al. (2016). MicroRNA regulation of endothelial TREX1 reprograms the tumour microenvironment. Nat. Commun. 7:13597. doi: 10.1038/ncomms13597
Xu, L., Liu, Y. Y., Chen, L. M., Xie, Y. Y., Liang, J. X., and Chao, H. (2016). Mitochondria-targeted ruthenium (II) polypyridyl complexes with benzofuran group for live cell imaging. J. Inorg. Biochem. 159, 82–88. doi: 10.1016/j.jinorgbio.2016.02.028
Yu, Q., Liu, Y., Xu, L., Zheng, C., Le, F., Qin, X., et al. (2014). Ruthenium(II) polypyridyl complexes: cellular uptake, cell image and apoptosis of HeLa cancer cells induced by double targets. Eur. J. Med. Chem. 82, 82–95. doi: 10.1016/j.ejmech.2014.05.040
Yuan, J., Lei, Z., Wang, X., Zhu, F., and Chen, D. (2015). Ruthenium complex Lambda-WH0402 induces hepatocellular carcinoma LM6 (HCCLM6) cell death by triggering the Beclin-1-dependent autophagy pathway. Metallomics 7, 896–907. doi: 10.1039/c5mt00010f
Zeng, L., Chen, Y., Huang, H., Wang, J., Zhao, D., Ji, L., et al. (2015). Cyclometalated ruthenium(II) anthraquinone complexes exhibit strong anticancer activity in hypoxic tumor cells. Chemistry 21, 15308–15319. doi: 10.1002/chem.201502154
Zeng, L., Chen, Y., Liu, J., Huang, H., Guan, R., Ji, L., et al. (2016). Ruthenium(II) complexes with 2-phenylimidazo[4,5-f][1,10]phenanthroline derivatives that strongly combat cisplatin-resistant tumor cells. Sci. Rep. 6:19449. doi: 10.1038/srep19449
Zeng, L., Gupta, P., Chen, Y., Wang, E., Ji, L., Chao, H., et al. (2017a). The development of anticancer ruthenium(ii) complexes: from single molecule compounds to nanomaterials. Chem. Soc. Rev. 46, 5771–5804. doi: 10.1039/c7cs00195a
Zeng, L., Kuang, S., Li, G., Jin, C., Ji, L., and Chao, H. (2017b). A GSH-activatable ruthenium(ii)-azo photosensitizer for two-photon photodynamic therapy. Chem. Commun. 53, 1977–1980. doi: 10.1039/c6cc10330h
Zhang, P., Wang, J., Huang, H., Chen, H., Guan, R., Chen, Y., et al. (2014). RuNH2@AuNPs as two-photon luminescent probes for thiols in living cells and tissues. Biomaterials 35, 9003–9011. doi: 10.1016/j.biomaterials.2014.07.021
Zhang, P., Wang, J., Huang, H., Qiao, L., Ji, L., and Chao, H. (2013). Chiral ruthenium(II) complexes with phenolic hydroxyl groups as dual poisons of topoisomerases I and IIalpha. Dalton Trans. 42, 8907–8917. doi: 10.1039/c3dt50472g
Zhao, S., Nishimura, T., Chen, Y., Azeloglu, E. U., Gottesman, O., Giannarelli, C., et al. (2013). Systems pharmacology of adverse event mitigation by drug combinations. Sci. Transl. Med. 5:206ra140. doi: 10.1126/scitranslmed.3006548
Keywords: ruthenium complexes, antitumor, diagnosis and therapy, drug combinations, synergistic effect
Citation: Lin K, Zhao Z-Z, Bo H-B, Hao X-J and Wang J-Q (2018) Applications of Ruthenium Complex in Tumor Diagnosis and Therapy. Front. Pharmacol. 9:1323. doi: 10.3389/fphar.2018.01323
Received: 27 September 2018; Accepted: 29 October 2018;
Published: 19 November 2018.
Edited by:
Zhe-Sheng Chen, St. John’s University, United StatesReviewed by:
Leli Zeng, Shenzhen University, ChinaQingbin Cui, Guangzhou Medical University, China
Lili Liu, Guangdong Provincial Occupational Disease Prevention Hospital, China
Copyright © 2018 Lin, Zhao, Bo, Hao and Wang. This is an open-access article distributed under the terms of the Creative Commons Attribution License (CC BY). The use, distribution or reproduction in other forums is permitted, provided the original author(s) and the copyright owner(s) are credited and that the original publication in this journal is cited, in accordance with accepted academic practice. No use, distribution or reproduction is permitted which does not comply with these terms.
*Correspondence: Xiao-Juan Hao, eGlhb2p1YW4uaGFvQGNzaXJvLmF1 Jin-Quan Wang, d2FuZ2ppbnF1YW5AZ2RwdS5lZHUuY24=