- 1São Paulo State University (Unesp), Institute of Biosciences, Humanities and Exact Sciences (Ibilce), São Paulo, Brazil
- 2Department of Clinical and Toxicological Analysis, Faculty of Pharmaceutical Sciences, University of São Paulo, São Paulo, Brazil
- 3From the Post-Graduation in Structural and Functional Biology, Federal University of São Paulo, São Paulo, Brazil
- 4Department of Surgical Research, Northwick Park Institute for Medical Research, University College London, London, United Kingdom
Skin graft successful depends on reduction of local inflammation evoked by the surgical lesion and efficient neovascularization to nutrition the graft. It has been shown that N-terminal portion of the Annexin A1 protein (AnxA1) with its anti-inflammatory properties induces epithelial mucosa repair and presents potential therapeutic approaches. The role of AnxA1 on wound healing has not been explored and we investigated in this study the effect of the peptide Ac2–26 (N-terminal AnxA1 peptide Ac2–26; AnxA12–26) on heterologous skin scaffolds transplantation in BALB/c mice, focusing on inflammation and angiogenesis. Treatment with AnxA12–26, once a day, from day 3–60 after scaffold implantation improved the take of the implant, induced vessels formation, enhanced gene and protein levels of the vascular growth factor-A (VEGF-A) and fibroblast influx into allograft tissue. It also decreased pro- while increasing anti-inflammatory cytokines. The pro-angiogenic activity of AnxA12–26 was corroborated by topical application of AnxA12–26 on the subcutaneous tissue of mice. Moreover, treatment of human umbilical endothelial cells (HUVECs) with AnxA12–26 improved proliferation, shortened cycle, increased migration and actin polymerization similarly to those evoked by VEGF-A. The peptide treatment instead only potentiated the tube formation induced by VEGF-A. Collectively, our data showed that AnxA12–26 treatment favors the tissue regeneration after skin grafting by avoiding exacerbated inflammation and improving the angiogenesis process.
Introduction
Skin grafting has been employed to treat several acute and chronic wounds, and the success of the process is depending on an appropriated inflammatory reaction, neovascularization, granulation tissue formation, re-epithelialization, and tissue remodeling (Barrientos et al., 2008). Hence, skin grafting is a highly complex process, mediated by numerous growth factors and inflammatory modulators (Leoni et al., 2015). The healing of wounds begins with clots formation, influx of inflammatory cells, fibroblast proliferation, and capillary damage with subsequent migration and proliferation of endothelial cells to the transplanted area. Then, angiogenesis takes place to deliver nutrients and oxygen to the wound bed, and to improve fibroblast proliferation (Bergers and Benjamin, 2003; Moon and West, 2008; Micallef et al., 2012; Yoo et al., 2013).
Annexin A1 (AnxA1) is a 37 kDa protein positively regulated by glucocorticoids and binds to membrane phospholipids in a calcium-dependent manner (Perretti and D’Acquisto, 2009; Xu et al., 2013). AnxA1 is a member of a family of 13 proteins identified in mammals (Raynal and Pollard, 1994; Hoehenwarter et al., 2008) that contains a small N-terminal region, varying in length and composition, and a central domain consisting of 4–8 repetitions of a highly conserved aminoacid sequence. The N-terminal domain is unique to each member of the annexin superfamily, and it has been extensively shown that this region is responsible for the anti-inflammatory actions of AnxA1 (Perretti and Flower, 2004; Solito et al., 2006; Perretti and D’Acquisto, 2009). In this context, the N-terminal AnxA1 mimetic peptide Ac2–26 (AnxA12–26) inhibits neutrophil migration in inflammatory sites, epithelial cell proliferation, phagocytosis of apoptotic neutrophils by macrophages and neutrophil apoptosis, with especial role on the resolution of the inflammation (Gobbetti and Cooray, 2016). Although AnxA1 is able to interact to membrane phospholipids, the AnxA1 effects are dependent on phosphorylation and interaction with formyl–peptide receptors (FPR), especially FPR2, a G-protein coupled receptor; meanwhile the peptide Ac2–26 is able to interact to both FPR1 and FPR2 (Cooray et al., 2013).
Endogenous AnxA1 is involved in muscle and intestinal epithelial cells repair, as these processes are impaired or delayed in AnxA1 null mice (Leikina et al., 2015; Leoni et al., 2015). Furthermore, the benefits of the use of recombinant AnxA1 or its related peptides, such as AnxA12–26, on mucosal epithelial repair have been fully demonstrated (Leoni and Nusrat, 2016). Hence, along with pro-resolutive actions on inflammation, the beneficial results of AnxA1 or its related peptides on tissue repair have pointed out evidences to therapeutic applications of AnxA1 N-terminal peptides (Gobbetti and Cooray, 2016). However, the role of AnxA1 on skin repair is not well established. Secreted AnxA1 is not essential to skin wound healing, as wound inflammation, closure and the formation of granulation tissue were not altered in AnxA1 null mice (Kreft et al., 2016). Conversely, we recently showed, for the first time, that the systemic pharmacological treatment using AnxA12–26 in mice increased skin allograft survival by inducing the resolution of inflammation, as it caused impaired migration of neutrophils into tissue and enhanced apoptosis of these cells in the site of allograft transplantation (Teixeira et al., 2016).
In order to establish the mechanisms of AnxA12–26 on skin healing process, in this study we investigated the systemic actions of the AnxA12–26 on heterologous skin transplantation in mice, focusing on inflammation and angiogenesis. Altogether, our data suggested that Ac2–26 peptide acts as a facilitating agent in skin allograft transplantation, by limiting local inflammation, inducing fibroblast proliferation and angiogenesis.
Materials and Methods
Animals
Male BALB/c wild-type mice, weighing 25–30 g, 6–8 weeks old, were kept on a 12 h light-dark cycle and allowed food and water ad libitum. They were anesthetized with ketamine (20 mg/kg) and xylazine solution (2 mg/kg) before each experimental procedure. All experiments were performed according to protocols approved by the Brazilian Society of Science of Laboratory Animals (SBCAL) for proper care and use of experimental animals, and it was approved by the Ethics Committee in Animal Experimentation of São Paulo State University of Sao Jose do Rio Preto (Nos. 074/2013 and 065/2012). The total number of animals used in the in vivo experiments was 60.
Dermis Harvesting and Scaffold Production
Scaffolds were produced at the Northwick Park Institute for Medical Research, London, United Kingdom. Fresh porcine skin was obtained from Large-White/Landrace crossbred pigs after euthanasia. This study was performed according to the regulatory guidelines of the United Kingdom Home Office. Procedures of skin harvesting and scaffold production were described by Mimura et al. (2016). The detailed method is described in the Supplementary Material.
Heterologous Transplantation
To carry out heterologous transplantation we used porcine decellularized skin (scaffolds). Mice were anesthetized, and the surgical procedures were carried out according to Mimura et al. (2016). Details of technical procedures are described in the Supplementary Material. Transplanted mice were subjected to daily administration of either PBS or AnxA12–26 (Ac-AMVSEFLKQAWFIENEEQEYVQTVK, Invitrogen, United States) (n = 5 animals/group) and sacrificed on days 3, 10, 15, and 60 after transplantation. Pharmacological treatments started 3 days before heterologous skin transplantation. The AnxA12–26 (100 μg/day diluted in sterile PBS) was administrated intraperitoneally (Teixeira et al., 2016).
Processing of Skin Fragments for Histological Analysis
Samples were fixed for 24 h in 4% paraformaldehyde solution at room temperature. They underwent the process of multiple washes in distilled water, dehydration in graded alcohol, embedding in paraffin wax, sectioning to 5 μm, staining with haematoxylin and eosin (HE) and analyzing on an Axioskop 2-Mot Plus Zeiss microscope (Carl Zeiss, Jena, Germany).
Quantitative Reverse Transcription Polymerase Chain Reaction (qRT-PCR)
Briefly, total RNA was extracted from the scaffold transplanted area of mice using a commercially available kit (QiagenRNeasy Mini Kit; Qiagen, Hilden, Germany). Tissues were collected from mice subjected to daily administration of either PBS or AnxA12–26 (n = 5 animals/group) and sacrificed at 3, 10, 15, and 60 days after transplantation procedure. Pharmacological treatments started 3 days before heterologous skin transplantation. AnxA12–26 (100 μg/day diluted in sterile PBS) was i.p. administrated. Specificities of the technique here employed are described in the Supplementary Material.
Multiplex Assays
Transplanted tissues were macerated in liquid nitrogen and placed in clean, 1.5 mL tubes to which 500 μL of a solution containing protease inhibitor cocktail (GE Healthcare, Amersham, United Kingdom) and Tween 20 (1 μL) (Sigma-Aldrich, Poole, Dorset, United Kingdom) was added to quantify inflammatory mediators interleukin (IL)1β, IL-6, tumor necrosis factor-α (TNF-α), IL-17, and interferon-γ (INF-γ). The description of experimental procedure is described in the Supplementary Material and tissues were collected after the treatments described above.
Dorsal Skinfold Chamber
The dorsal skinfold chamber was implanted in mice under anesthesia, as previously described by Harder et al. (2004). Saline (10 μL) (control), AnxA12–26 peptide (0.4 μg), and/or VEGF-A (10 ng) (n = 5 animals/group) were locally applied as previously described by Drewes et al. (2012). Treatments were carried out on the 4th, 5th, and 6th days after chamber implantation. The images obtained before (day 4) and after treatment (day 9) were quantified according to Dellian et al. (1996) and Drewes et al. (2012). The representative scheme of tissue analysis is described in the Supplementary Material.
Cell Culture and Experimental Procedures
Human umbilical vessel endothelial cells (HUVEC) (ATCC-CRL-2873TM) were cultured in 75 cm2 plastic culture flasks with DMEM (Invitrogen, Carlsbad, CA, United States) supplemented with 10% fetal bovine serum, L-glutamine (200 mM), streptomycin (0.1 mg/mL) and penicillin (100 U/mL) (Cultilab, Brazil), at 37°C in a humid atmosphere containing 5% CO2. Cells were used up to the 3rd passage. HUVEC proliferation, migration and tube formation were performed according to Drewes et al. (2015). The detailed experimental procedures are described in the Supplementary Material. HUVECs were seeded (1 × 104 cells/well) and, after cell adhesion, were incubated with PBS (control), AnxA12–26 peptide (30 μM) and/or VEGF-A (10 ng/mL) (Cell Signaling Technology, Danvers, MA, Unites States) for 24, 48, or 72 h to measure proliferation. Semi confluent HUVECs in the matrigel were disrupted with a pipette tip, creating a “groove” in the center of the well. After, the cells gently washed and incubated with PBS (control), AnxA12–26 peptide (1, 10, or 30 μM) and/or VEGF-A (50 ng/mL), for 12 h, to measure the migration into matrigel; 2 × 104 cells/well were incubated with PBS (control), AnxA12–26 peptide (1, 10, or 30 μM) and/or VEGF-A (50 ng/mL) for 2 h and cells were plated under the Matrigel® (Corning, Corning, NY, Unites States) layer to form capillary-like structures for 6 h.
F-actin Staining by Confocal Microscopy Assay
Human umbilical endothelial cells (1 × 104 cells/well) were seeded on a glass-bottom culture dish and once adhered, they were treated with PBS (control), AnxA12–26 peptide (30 μM) and/or VEGF-A (50 ng/mL) for 2 h. Immediately after the treatment protocol, cells were stained using an F-actin kit (Cytoskeleton, Inc., Denver, CO, United States) and visualized by confocal microscopy (Carl Zeiss LSM 780-NLO, Germany). The description of experimental procedure is described in the Supplementary Material.
F-actin Quantitation With a Fluorescent Plate Reader
HUVECs were cultured to reach 80–90% confluence in 48-well plates. After treatment with PBS (control), AnxA12–26 peptide (30 μM) and/or VEGF-A (50 ng/mL) for 2 h, cells went through the same staining procedure as described above. After being stained, the cells were washed three times with PBS and then 100 μL of wash buffer were added in each well and the fluorescence was read by a Synergy H1 (BioTek®, Peru, NY, United States) fluorescent plate reader (excitation wavelength of 535 nm and an emission wavelength of 585 nm). Results are expressed as the median of fluorescence intensity.
Ultrastructural Immunocytochemical Analysis
To detect the FPR1 (Abcam Cambridge, United Kingdom) and AnxA1 (Zymed Laboratories, Cambridge, United Kingdom), ultrathin sections (∼70 nm) of LR Gold embedded-HUVECs were treated with VEGF-A, AnxA12–26 or both for 2 h and submitted to several steps before being incubated with IgG antibodies conjugated to 20 and 10 nm colloidal gold (British Biocell, United Kingdom). The complete description of the methods is described in the Supplementary Material.
Statistical Analysis
Data were analyzed using Prisma® GraphPad software version 5.00. The results were presented as mean ± standard error of the mean (SEM) and statistical analysis was performed by analysis of variance for multiple comparisons (ANOVA), followed by the Bonferroni adjustment or Student’s t-test. P < 0.05 were considered to indicate statistically significant results.
Results
AnxA12–26 Treatment Induces Angiogenesis, Fibroblasts Influx, and Reduces Inflammatory Cytokines Secretion in Transplanted Tissue
Systemic treatment of transplanted mice with AnxA12–26 peptide augmented the number of blood vessels on day 10, which was further enhanced up to the 60th day (Figures 1A–D). Moreover, AnxA12–26 treatment induced augumentation on the number of vessels and migration of fibroblasts into the scaffold (Figures 1A–E), and also enhanced the mRNA levels of mediators involved in the recovery of the transplanted tissue, as detected by greater levels of transforming growth factor-β1 (TGF-β1), myofibroblasts α smooth muscle actin (α-SMA), fibroblast growth factor basic (FGF-β) (Figures 1F–H), and mRNA and protein levels of VEGF-A (Figures 1I,J). In the inflammatory scenario, we detected reduced levels of the pro-inflammatory mediators IL-1β, IL-6, TNF-α, IL-17, and IFN-γ during the first days after transplantation in the tissue collected from AnxA12–26 treated mice (Figures 2A–E).
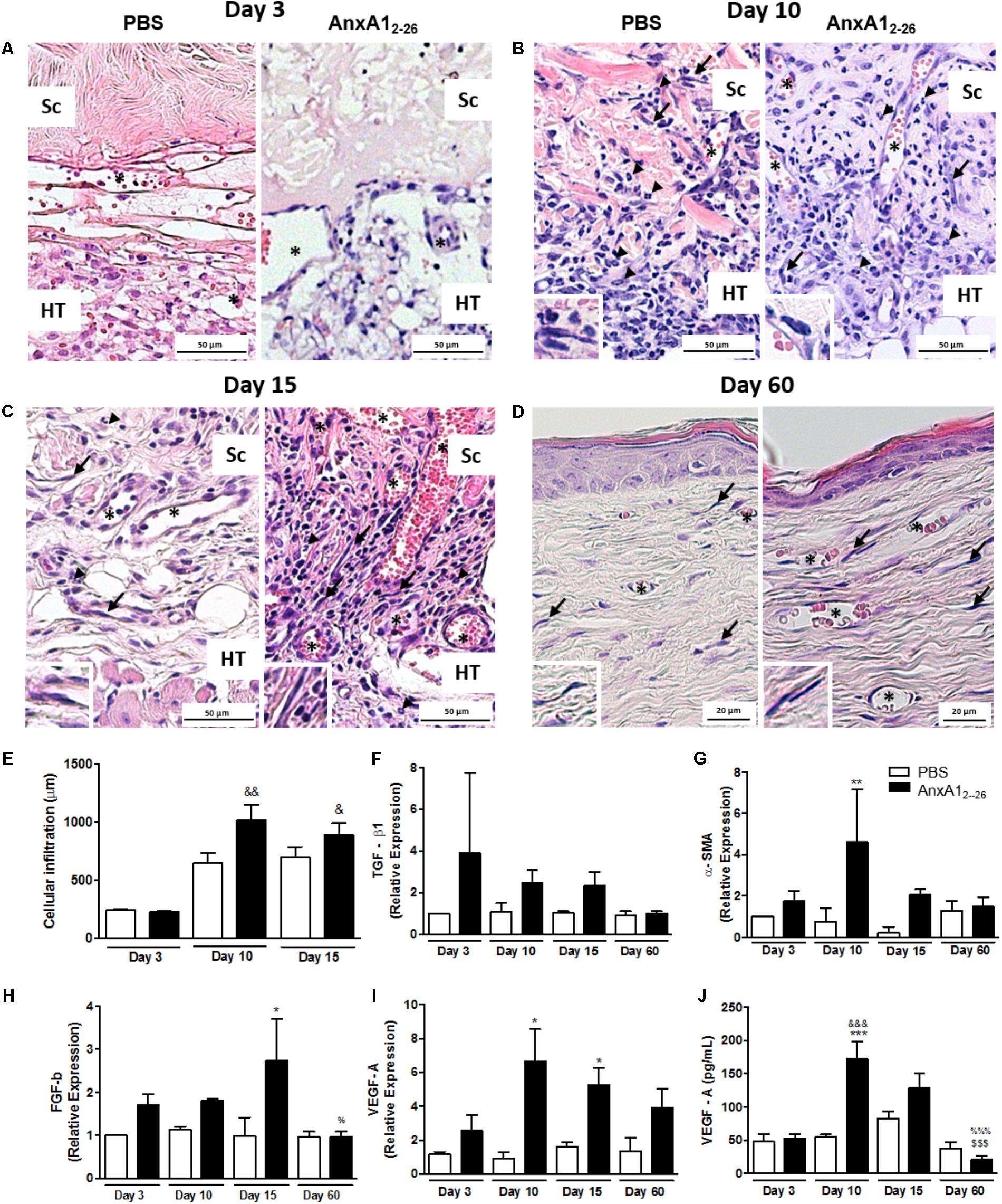
FIGURE 1. AnxA12–26 treatment improves the heterologous transplantation and induces angiogenesis. Histopathological analyses of skin transplanted fragments without (PBS) and with AnxA12–26 peptide treatment after 3 (A), 10 (B), 15 (C), and 60 (D) days post-surgery. Cell infiltration (E), TGF-β (F), α-SMA (G), FGF-b (H), and VEGF-A (I) gene expression and VEGF-A protein (J) in the transplanted tissue. Host tissue (TH), transplanted scaffold (Sc), vessels (∗), fibroblasts (arrows). The inserts show high magnifications of the fibroblasts. The values express the mean ± SEM of five animals per group (ANOVA followed by the Bonferroni’s test). ∗p < 0.05, ∗∗p < 0.01, and ∗∗∗p < 0.001 vs. respective PBS; &p < 0.05, &&p < 0.01 and &&&p < 0.001 vs. day 3; $$$p < 0.001 vs. day 10; %p < 0.05 and %%%p < 0.001 vs. day 15. Staining: hematoxylin & eosin.
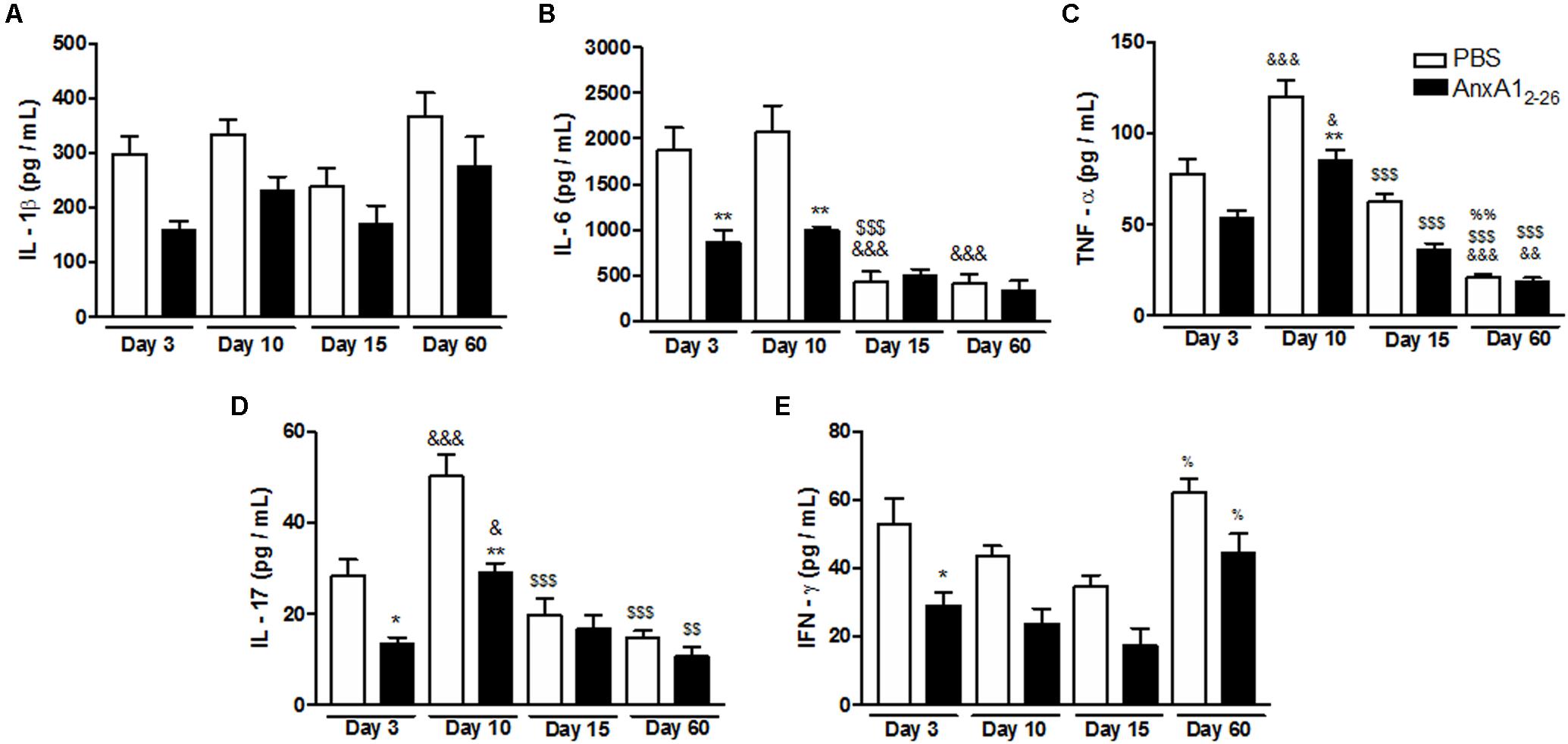
FIGURE 2. AnxA12–26 treatment reduces the inflammatory response in the heterologous transplantation. Levels of IL-1β (A), IL-6 (B), TNF-α (C), IL-17 (D), and IFN-γ (E) in transplanted tissue extracts at days 3, 10, 15, and 60 post-surgery quantified by Multiplex. Data indicate the mean ± SEM of data obtained from five animals per group (ANOVA followed by the Bonferroni’s test). ∗p < 0.05 and ∗∗p < 0.01 vs. respective PBS; &p < 0.05, &&p < 0.01, and &&&p < 0.001 vs. day 3; $$p < 0.01 and $$$p < 0.001 vs. day 10; %p < 0.05 and %%p < 0.01 vs. day 15.
AnxA12–26 Treatment Induces in vivo Angiogenesis
To assess if AnxA12–26 induces angiogenesis on absence of allograft transplantation, the dorsal skinfold chamber was implanted in mice and topical treatments started 3 days later. This protocol has been employed to avoid interference of surgical stress observed until 48 h of surgery (Drewes et al., 2012). The images obtained showed that local application of VEGF-A, AnxA12–26 or both simultaneously elevated the number of vessels in the dorsal subcutaneous tissue (Figure 3). It is noteworthy to mention that angiogenesis induced by VEGF-A treatment was similar in wild type (WT) and AnxA1 null mice (Supplementary Figure 2), showing that endogenous AnxA1 is not relevant to the VEGF-A induced angiogenesis in the skin. As previously mentioned, it was recently showed the normal skin repair in AnxA1 null mice (Kreft et al., 2016).
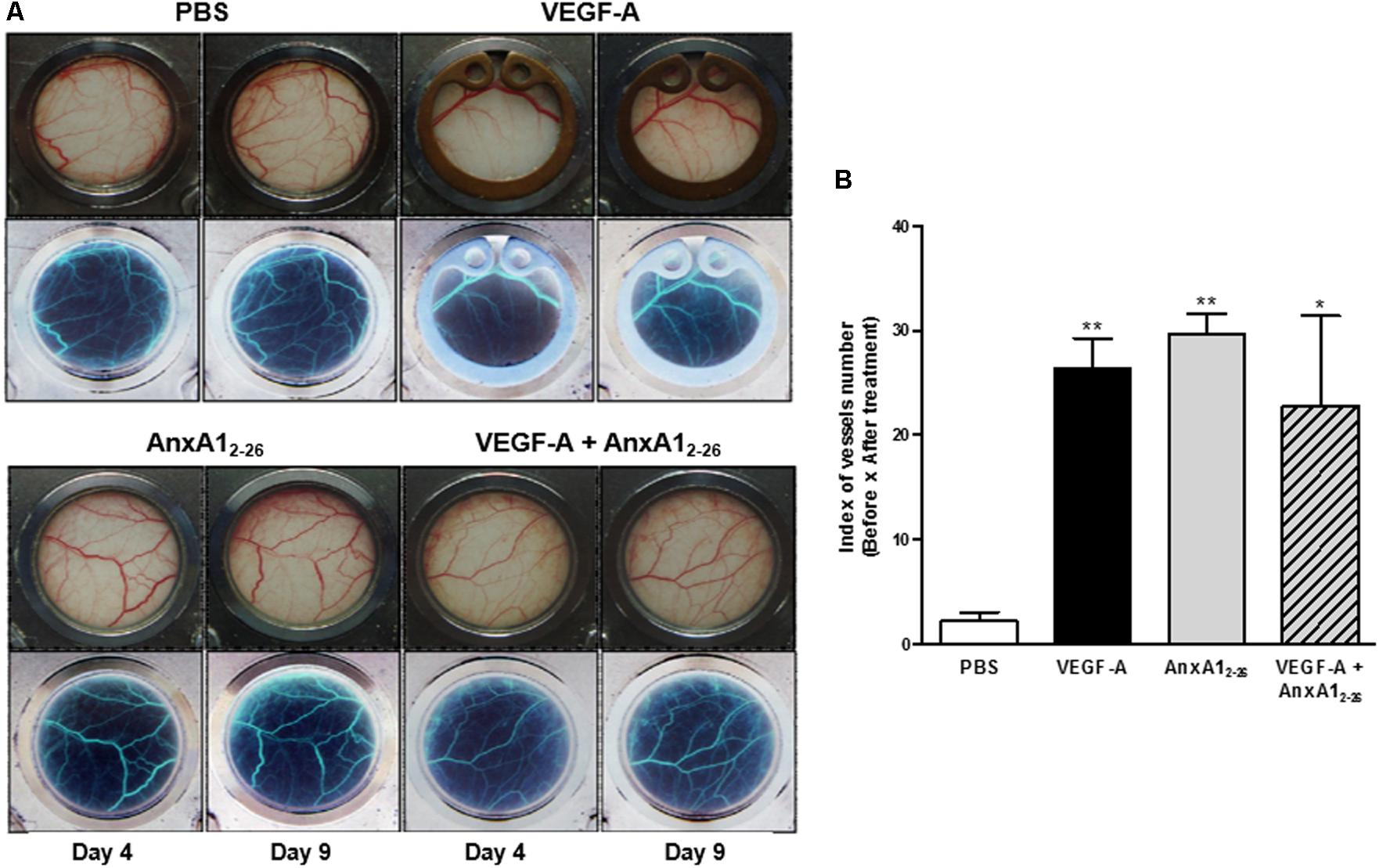
FIGURE 3. AnxA12–26 increases angiogenesis in a dorsal chamber model in BALB/c mice. Mice were topically treated with Saline (10 μL), AnxA12–26 (1 mg/kg), and/or VEGF-A (10 ng/10 μL) in the dorsal skin. The treatments were administrated once per day, every 2 days, resulting three applications in each mouse. Representative images of the microcirculatory network of dorsal skin were obtained before (day 4) and after (day 9) treatments (A). The images in the upper panel represent the stained normal tissue and in the lower panel, the same computational images obtained after inverting the colors are displayed (A). The quantification of vessels is represented in B. The values express the mean ± SEM of five animals per group (ANOVA followed by Bonferroni’s Multiple Comparison Test). ∗p < 0.05, ∗∗p < 0.01 vs. PBS.
In vitro AnxA12–26 Treatment Induces Endothelial Proliferation and Migration
As expected, incubation with VEGF-A significantly stimulated the proliferation of HUVECs. Treatment with AnxA12–26 peptide induced cell proliferation and enhanced the effect caused by VEGF-A after 48 h of co-incubation (Figure 4A). Moreover, treatment with VEGF-A or AnxA12–26 peptide reduced the percentage of cells in phases G0/G1 and elevated cells in phase S. Cell proliferation and alterations on cell cycle were further augmented by co-treatment with VEGF-A and AnxA12–26 peptide (Figure 4B). It is noteworthy to mention that treatments did not induce HUVEC toxicity, measured by the amount of apoptotic and necrotic cells in flow cytometry analysis (Supplementary Table 1).
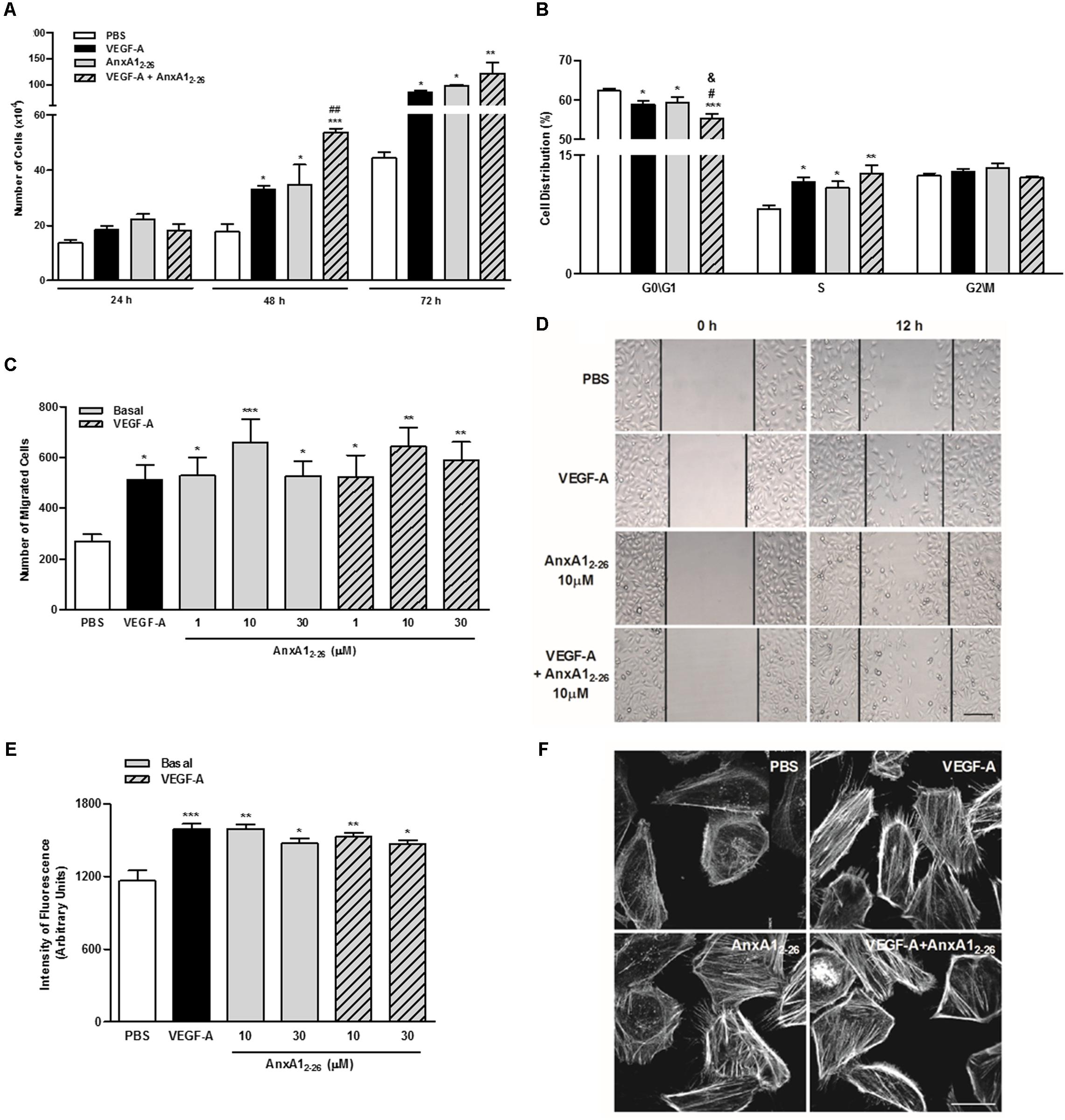
FIGURE 4. AnxA12–26 increases endothelial cell migration and actin polymerization. HUVECs (1 × 104 cells/well) were incubated with PBS (control), AnxA12–26 (30 μM), and/or VEGF-A (10 or 50 ng/mL) and cell proliferation was evaluated at 24, 48, and 72 h. Results are expressed as the mean ± SEM of cells of two independent experiments in triplicate (A). HUVECs were incubated with different treatments for 48 h, later labeled with PI (50 μg/mL) and the cell cycle phases were evaluated (ANOVA followed by the Tukey’s multiple comparisons test) (B). HUVEC migration was evaluated after 12 h of incubation with PBS (control), AnxA12–26 (1, 10, or 30 μM) and/or VEGF-A (50 ng/mL). Cell migration was monitored with images obtained before (0 h) and after (12 h) treatments (C,D). HUVECs (1 × 104 cells/well) were incubated with different treatments for 2 h and later incubated with rhodamine-phalloidin to evaluate actin polymerization. The intensity of fluorescence was monitored using a fluorescent plate reader (E) and by confocal microscopy (F). Scale bar = 10 μm. Results are expressed as the mean ± SEM of cells of two independent experiments in triplicate (ANOVA followed by the Bonferroni’s test). ∗p < 0.05; ∗∗p < 0.01 and ∗∗∗p < 0.001 vs. PBS; #p < 0.05 and ##p < 0.01 vs. VEGF; &p < 0.05 vs. AnxA12–26.
Moreover, AnxA12–26 treatment augmented the migration of HUVECs, similarly, to that evoked by VEGF-A treatment. Furthermore, co-treatment with the peptide and VEGF-A did not cause further migration in comparison with those induced by isolated treatments (Figures 4C,D). The fluorescent plate reader and confocal analyses of F-actin, detected by phalloidin binding, showed that all treatments, VEGF-A, AnxA12–26 or VEGF-A plus AnxA12–26, enhanced actin polymerization (Figures 4E,F).
Capillary-like tube formation is representative of the latter phase of angiogenesis, as is the primary organization of coalesced endothelial cells. Here we verified that VEGF-A treatment increased the number of tubes, an event not detected in cells treated with only AnxA12–26 peptide. Nevertheless, AnxA12–26 treatment further enhanced the effect produced by VEGF-A (Figures 5A,B). The analyses of adhesion molecules involved in homotypic endothelial cell adhesion showed that all treatments, similarly, enhanced PECAM-1 expression on cell membrane (Figure 5C).
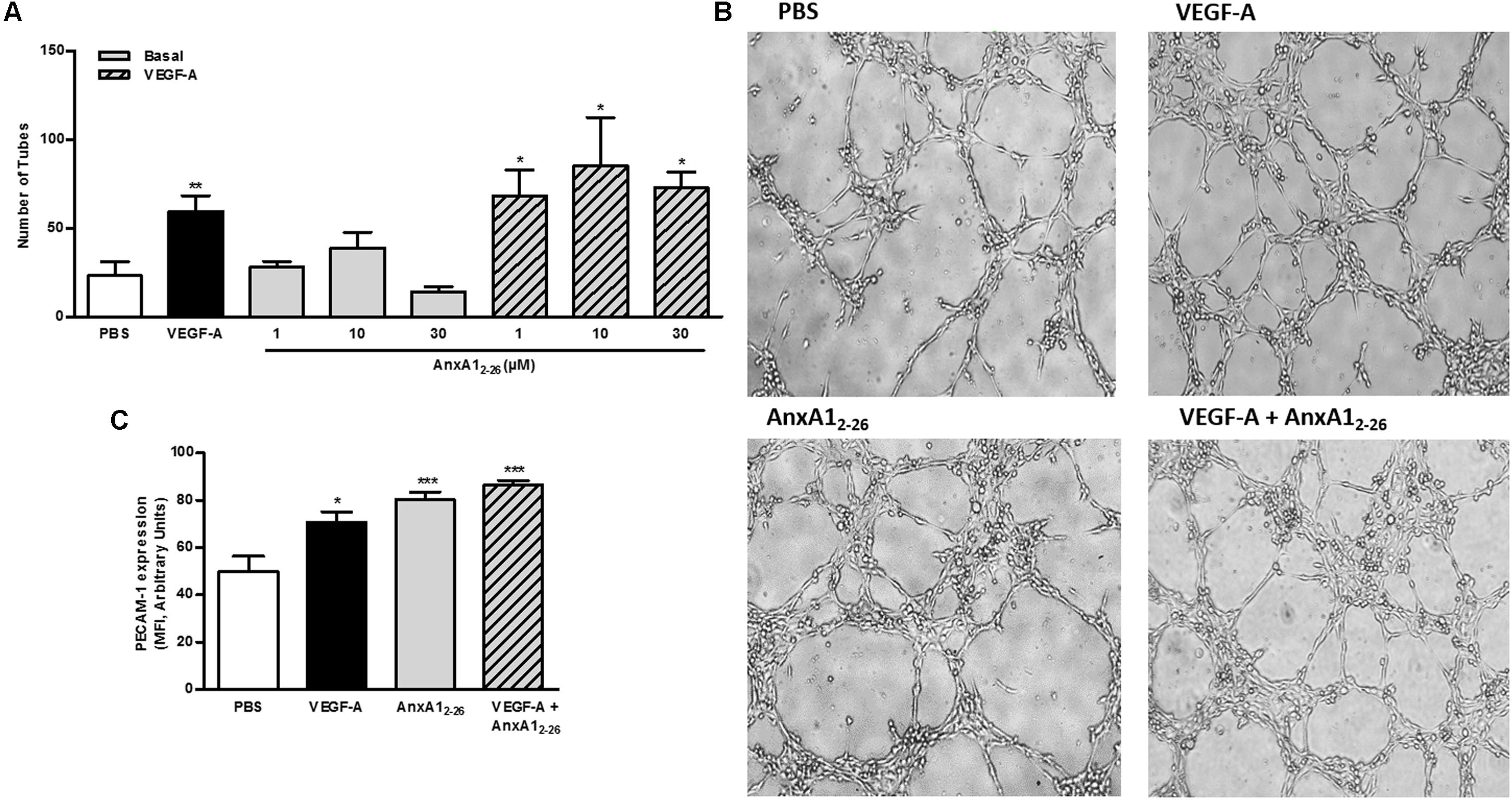
FIGURE 5. AnxA12–26 does not induce tube formation. HUVECs (2 × 104 cells/well) were incubated with PBS (control), AnxA12–26 (30 μM), and/or VEGF (50 ng/mL) for 6 h on Matrigel®and the number of tube structures were quantified using an optical microscope (A,B). PECAM-1 expression was evaluated by flow cytometry (C). Scale bar = 10 μm. Results are expressed as the mean ± SEM of two independent experiments in triplicate (ANOVA followed by the Tukey’s multiple comparisons test). ∗p < 0.05, ∗∗p < 0.01; ∗∗∗p < 0.001 vs. PBS.
VEGF-A or AnxA12–26 Treatments Reduce FPR1 Receptor on Membrane of HUVEC
Images obtained with ultrastructural immunocytochemistry showed that the AnxA1 and FPR1 is downregulated in HUVEC after VEGF-A, AnxA12–26 or VEGF-A plus AnxA12–26 treatments (Figures 6A,C). In these cells, gold particles were detected throughout the cytosol, with a significant proportion being observed also in the plasma membrane (Figure 6A). No labeling was detected in sections incubated with the control non-immune sheep serum (Figure 6B).
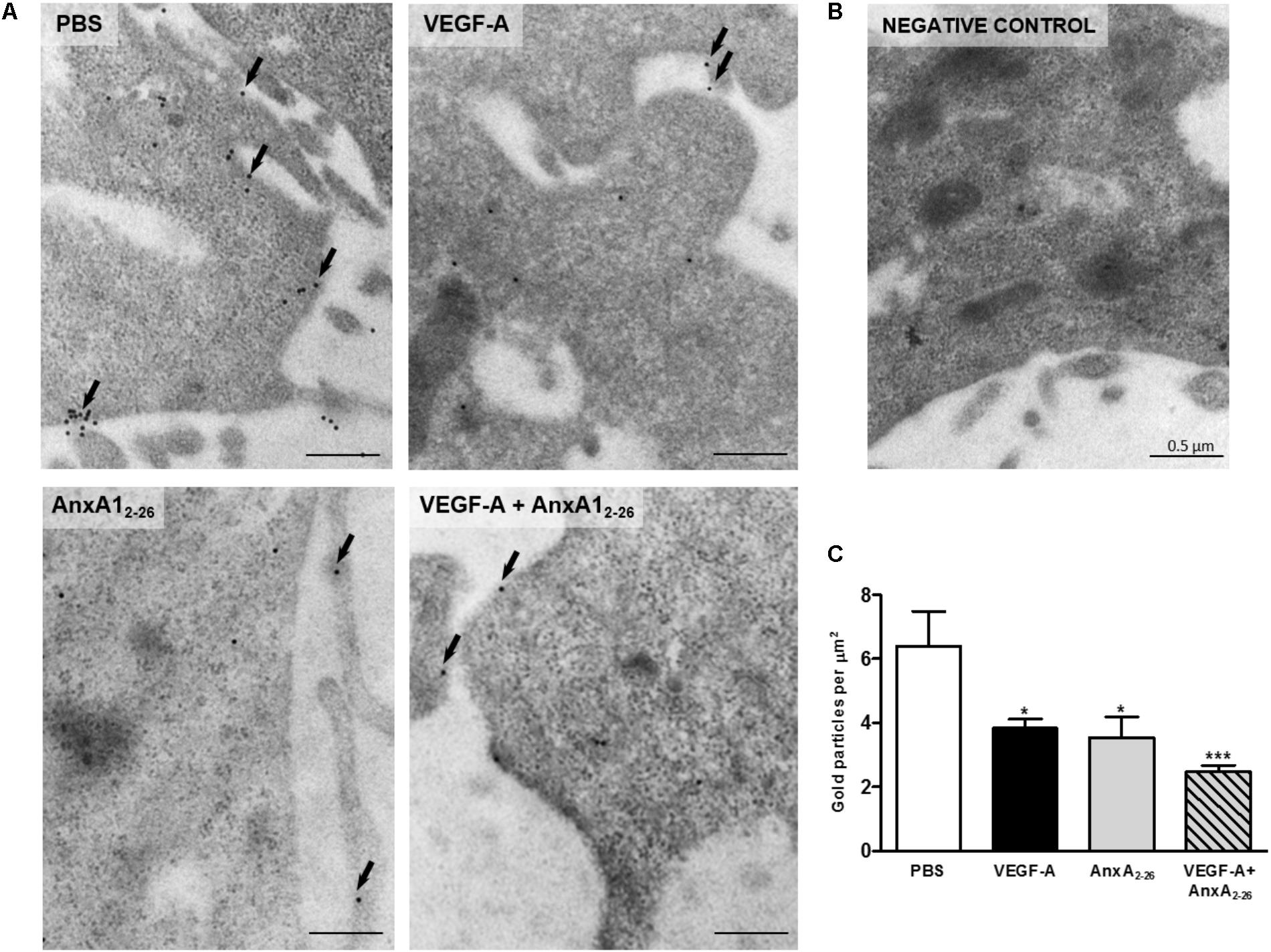
FIGURE 6. VEGF-A and AnxA12–26 decreases FPR1 expression on HUVECs. FPR1 was detected in the plasma membrane (arrows) and cytoplasm of cells under all experimental conditions (A). Negative control (B). Density of FPR1 immunogold particles in HUVECs (C). Scale bar = 0.5 μm. Data are mean ± SEM of distinct cells analyzed (n = 12–20/group) for each condition (ANOVA followed by the Bonferroni’s test). ∗p < 0.05, ∗∗∗p < 0.001 vs. PBS.
Discussion
In our previous study using a model of mice skin regeneration after decellularized non-crosslinked porcine skin scaffold implantation, we showed that systemic treatment with AnxA12–26 modified the microenvironment of the transplanted area, by limiting the exacerbated inflammatory response at the beginning of the process and favoring angiogenesis and regeneration of the transplanted tissue (Mimura et al., 2016). Furthermore, local application of this peptide evoked angiogenesis in the subcutaneous tissue of mice, and the functional control of the process after treatment seems to be related more prominently to endothelial cell proliferation and migration. However, the ability of AnxA12–26 only to potentiate in vitro tubulogenesis caused by VEGF-A and to induce the angiogenesis in in vivo conditions, infers that AnxA12–26 may act as a co-adjuvant to growth factors on new vessel formation. Together, our data highlight the mechanisms of AnxA12–26 on skin regeneration and the potential application of the peptide as pharmacological tool.
Previous study of our group had already shown that systemic AnxA12–26 treatment reduced the infiltration of neutrophils into the transplanted tissue and led them to apoptosis (Teixeira et al., 2016), and here we corroborated the anti-inflammatory actions of the peptide by the impaired secretion of pro-inflammatory cytokines at the beginning phase of the tissue regeneration. It is commonly known that acellular skin is not able to promote a specific inflammatory reaction in response to a local graft (Sengor et al., 2005; Ngo et al., 2011), but inflammation occurs due to invasive surgical procedures, and the exacerbated inflammatory reaction impairs tissue regeneration (Diegelmann and Evans, 2004). Since the initial phase, the AnxA12–26 was able to reduce the expression of IL-1β, INF-γ, IL-6, TNF-α, and IL-17, corroborating studies with in vivo and in vitro models of retina autoimmune disease and uveitis in rodents and human (Girol et al., 2013; Yazid et al., 2015; Cardin et al., 2017). Lima et al. (2017) showed that AnxA1 can acts at multiple regulatory levels to promote resolution of inflammation and may be a common mechanism that account for the pro-resolving actions of pro-resolving molecules.
In the proliferation phase, we have showed that AnxA12–26 treatment enhanced the cellular infiltration of myofibroblasts within the transplanted scaffold, and elevated the levels of regenerative factors, such as FGFs and TGF-β (Mimura et al., 2016). During granulation process, fibroblasts are gradually transformed into myofibroblasts (Hinz, 2016), and they are the predominant mediators of the contractile process (Li et al., 2007). FGFs are growth factor family is also involved in the angiogenesis process, fibroblasts and epithelial cell proliferation, and consequently in the wound healing, allowing for maintenance of transplants (Martin et al., 1992; Lu and Huang, 2013). TGF-β is a growth factor responsible for the differentiation and activation of myofibroblasts (showed by α-SMA-positive cells) (Hinz, 2016; Mimura et al., 2016).
Assuredly, systemic administration of AnxA12–26 enhanced the number of new vessels in the transplanted tissue and enhanced local gene and protein expression of VEGF-A, showing a pro-angiogenic action of the peptide. The direct role of AnxA1 on angiogenesis has not been described, as data regarding AnxA1 on angiogenesis were obtained in in vivo and in vitro tumorigenesis conditions, and data suggest that the tumor microenvironment is determinant to angiogenic actions of AnxA1 (Oh et al., 2004; Yi and Schnitzer, 2009; Anbalagan et al., 2014). Remarkably, our in vivo data showed the ability of AnxA12–26 to induce angiogenesis in the subcutaneous tissue, equivalent to that caused by VEGF-A.
Human umbilical endothelial cell was employed to elucidate the direct actions of Ac2–26 on angiogenesis pathways due to well-established data obtained with these cells in the steps of the complex process of vessel formation. We showed here the direct ability of AnxA12–26 to induce cell proliferation, especially accomplished by arresting the cell cycle rather than inhibiting death mechanisms. Furthermore, proliferation and cell arrest were further augmented in AnxA12–26 and VEGF-A co-treated cells, showing synergic effect of AnxA12–26 and VEGF-A on cell cycle phases. AnxA1 actions on cell proliferation are controversial, as both pro- and anti-proliferative actions have been described in different types of cancers (Leoni et al., 2013; Biaoxue et al., 2014; Gastardelo et al., 2014; Liu et al., 2014) and inflammatory cells (Jia et al., 2013). However, our unprecedented data show the direct proliferative role of AnxA12–26 on endothelial cells in the absence of other stimuli.
Furthermore, our results corroborate the actions of AnxA12–26 on cell migration, by acting on the formation of cytoskeletal and protein actin projection at the leading edge of migrating cells, as previously described in muscle, epithelial cells, and fibroblasts (Bizzarro et al., 2012a,b). The pivotal role of AnxA1 in endothelial cell migration had already been shown in VEGF-A induced migration, as the actions of VEGF-A on endothelial cell locomotion depend on the activation of p38/MAP-KAP kinase-2/LIMK1, which phosphorylates endogenous AnxA1, and leads to actin cytoskeletal remodeling (Côté et al., 2010; Pin et al., 2012). However, the direct effect of AnxA12–26 on migration of endothelial cells is shown for the first time in this study.
In vitro angiogenesis, quantified by terminal tubulogenesis, was only enhanced by VEGF-A treatment, and AnxA12–26 co-treatment potentiated the VEGF-A effect. The inability of the peptide to evoke the organization of vessels, which depends on a perfect homotypic cell binding, could be unexpectedly, as it has been shown that endogenous AnxA1 mediates the interendothelial cell tight junctions on blood–brain barrier, which stabilizes tight and adherence junctions (Park et al., 2010; Cristante et al., 2013). Moreover, our data showed AnxA12–26 treatment, similarly, to VEGF-A, enhanced the expression of PECAM-1, which is also an important molecule of endothelial cell homotypic interaction (Harris and Nelson, 2010; Park et al., 2015). Hence, it is plausible to suppose that actions AnxA1 and related peptides are different, even though the peptide also binds to FPR1 further than FPR2. Nonetheless, the ability of AnxA12–26 to induce PECAM-1 expression is shown here for the first time, and the molecular basis of this effect will be further investigated regarding the relevance of endothelial PECAM-1 in several pathophysiological conditions, such as cardiovascular and immune diseases (Marelli-Berg et al., 2013; Privratsky and Newman, 2014).
The effects observed here may be dependent on AnxA12–26/FPR1 pathway, as we also showed that endogenous AnxA1, which binds only to FPR2, does not play a pivotal role on in vivo angiogenesis induced by VEGF-A. The role of FPR2 on angiogenesis is dual, as serum amyloid A induces functional neovascularization by acting on FPR2 of endothelial cells. Conversely activation of FPR2 on endothelial cells by inflammatory resolving mediators, such as lipoxin A4, reduces the neovascularization on the resolution of inflammation (Prevete et al., 2015). The participation of FPR1 on angiogenesis has been preferentially shown in cancer conditions, and depends on a complex scenario in the tumor microenvironment. Activation of FPR1 on cancer gastric cells reduced the local angiogenesis and cancer growth, depending on pro-resolving mediators of inflammation, such as metabolic activity of lipoxygenases (ALOX5/15) (Prevete et al., 2015, 2017). Differently, the D1 and D2 linear sequences of uroquinase-type plasminogen activator (uPAR) induced angiogenesis by binding to FPR1 on endothelial cells (Prevete et al., 2015), and the blockage of the interaction of uPAR with the receptor that prevents capillary-like tubes formation in co-culture with chondrosarcoma cells (Ingangi et al., 2016). We here showed that VEGF-A or AnxA12–26 treatments down regulated the membrane expression FPR1 in HUVEC, suggesting a direct role of the receptor on angiogenesis process.
Together, data herein presented identified the bioactive AnxA1 derivative peptide AnxA12–26 as a possible therapeutic agent to promote skin regeneration in allograft transplantation, with specific modulation of inflammation and angiogenesis. Moreover, the interrelationship of AnxA12–26 or VEGF-A with FPR1 on endothelial cells opens new pathways to be investigated in order to understand the modulation of angiogenesis process on graft transplantation.
Author Contributions
JL and CD performed all in vitro experiments with HUVEC and intravital microscopy studies, and analyzed the data obtained. JL performed all dorsal skinfold chamber experiments and analyzed the data obtained. KM performed all transplants experiments and analyzed the data obtained. CZ contributed to RNA extraction. CG performed the immune electron microscopy with HUVECs. TA contributed to the production and assessment of the scaffolds. KG performed the production of porcine skin scaffolds and molecular analysis and contributed to the manuscript review. SF and SO supervised the in vitro and in vivo studies, analyzed the data, and contributed to the writing of the manuscript.
Funding
We thank the São Paulo Research Foundation (FAPESP) for its financial support (Grants to SO 2012/21603-2, 2016/02012-4, and SF 2014/07328-4). JL was granted a Master FAPESP supporting fellowship (Grant 2013/07487-2). KM and CD are granted a Ph.D. FAPESP supporting fellowship (Grants 2012/13041-4 and 2010/19802-1). SO and SF are Research Fellows of the Conselho Nacional de Desenvolvimento Científico e Tecnológico (CNPq).
Conflict of Interest Statement
The authors declare that the research was conducted in the absence of any commercial or financial relationships that could be construed as a potential conflict of interest.
Acknowledgments
The authors thank the Centre of Facility and Support to Research (CEFAP) of Institute of Biomedical Science of University of São Paulo for technical assistance.
Supplementary Material
The Supplementary Material for this article can be found online at: https://www.frontiersin.org/articles/10.3389/fphar.2018.01015/full#supplementary-material
References
Anbalagan, D., Yap, G., Yuan, Y., Pandey, V. K., Lau, W. H., Arora, S., et al. (2014). Annexin-A1 regulates MicroRNA-26b∗ and MicroRNA-562 to directly target NF-κB and angiogenesis in breast cancer cells. PLoS One 9:e114507. doi: 10.1371/journal.pone.0114507
Barrientos, S., Stojadinovic, O., Golinko, M. S., Brem, H., and Tomic-Canic, M. (2008). Growth factors and cytokines in wound healing. Wound Repair Regen. 16, 585–601. doi: 10.1111/j.1524-475X.2008.00410.x
Bergers, G., and Benjamin, L. E. (2003). Tumorigenesis and the angiogenic switch. Nat. Rev. Cancer 3, 401–410. doi: 10.1038/nrc1093
Biaoxue, R., Xiguang, C., and Shuanying, Y. (2014). Annexin A1 in malignant tumors: current opinions and controversies. Int. J. Biol. Markers 29, e8–e20. doi: 10.5301/jbm.5000046
Bizzarro, V., Fontanella, B., Carratù, A., Belvedere, R., Marfella, R., Parente, L., et al. (2012a). Annexin A1 N-terminal derived peptide Ac2-26 stimulates fibroblast migration in high glucose conditions. PLoS One 7:e45639. doi: 10.1371/journal.pone.0045639
Bizzarro, V., Petrella, A., and Parente, L. (2012b). Annexin A1: novel roles in skeletal muscle biology. J. Cell. Physiol. 227, 3007–3015. doi: 10.1002/jcp.24032
Cardin, L. T., Sonehara, N. M., Mimura, K. K. O., Ramos Dinarte dos Santos, A., da Silva, W. A., Sobral, L. M., et al. (2017). ANXA1 Ac2–26 peptide, a possible therapeutic approach in inflammatory ocular diseases. Gene 614, 26–36. doi: 10.1016/j.gene.2017.02.032
Cooray, S. N., Gobbetti, T., Montero-Melendez, T., McArthur, S., Thompson, D., Clark, A. J. L., et al. (2013). Ligand-specific conformational change of the G-protein-coupled receptor ALX/FPR2 determines proresolving functional responses. Proc. Natl. Acad. Sci. U.S.A. 110, 18232–18237. doi: 10.1073/pnas.1308253110
Côté, M. C., Lavoie, J. R., Houle, F., Poirier, A., Rousseau, S., and Huot, J. (2010). Regulation of vascular endothelial growth factor-induced endothelial cell migration by LIM kinase 1-mediated phosphorylation of annexin 1. J. Biol. Chem. 285, 8013–8021. doi: 10.1074/jbc.M109.098665
Cristante, E., McArthur, S., Mauro, C., Maggioli, E., Romero, I. A., Wylezinska-Arridge, M., et al. (2013). Identification of an essential endogenous regulator of blood-brain barrier integrity, and its pathological and therapeutic implications. Proc. Natl. Acad. Sci. U.S.A. 110, 832–841. doi: 10.1073/pnas.1209362110
Dellian, M., Witwer, B. P., Salehi, H. A., Yuan, F., and Jain, R. K. (1996). Quantitation and physiological characterization of angiogenic vessels in mice: effect of basic fibroblast growth factor, vascular endothelial growth factor/vascular permeability factor, and host microenvironment. Am. J. Pathol. 149, 59–71.
Diegelmann, R. F., and Evans, M. C. (2004). Wound healing: an overview of acute, fibrotic and delayed healing. Front. Biosci. 9:283–289. doi: 10.2741/1184
Drewes, C. C., Dias, R. Y., Branco, V. G., Cavalcante, M. F., Souza, J. G., Abdalla, D. S. P., et al. (2015). Post-transcriptional control of Amblyomin-X on secretion of vascular endothelial growth factor and expression of adhesion molecules in endothelial cells. Toxicon 101, 1–10. doi: 10.1016/j.toxicon.2015.04.002
Drewes, C. C., Dias, R. Y. S., Hebeda, C. B., Simons, S. M., Barreto, S. A., Ferreira, J. M., et al. (2012). Actions of the Kunitz-type serine protease inhibitor amblyomin-X on VEGF-A-induced angiogenesis. Toxicon 60, 333–340. doi: 10.1016/j.toxicon.2012.04.349
Gastardelo, T. S., Cunha, B. R., Raposo, L. S., Maniglia, J. V., Cury, P. M., Lisoni, F. C. R., et al. (2014). Inflammation and cancer: role of annexin A1 and FPR2/ALX in proliferation and metastasis in human laryngeal squamous cell carcinoma. PLoS One 9:e111317. doi: 10.1371/journal.pone.0111317
Girol, A. P., Mimura, K. K. O., Drewes, C. C., Bolonheis, S. M., Solito, E., Farsky, S. H. P., et al. (2013). Anti-inflammatory mechanisms of the annexin A1 protein and its mimetic peptide Ac2-26 in models of ocular inflammation in vivo and in vitro. J. Immunol. 190, 5689–5701. doi: 10.4049/jimmunol.1202030
Gobbetti, T., and Cooray, S. N. (2016). Annexin A1 and resolution of inflammation: tissue repairing properties and signalling signature. Biol. Chem. 397, 981–993. doi: 10.1515/hsz-2016-0200
Harder, Y., Amon, M., Erni, D., and Menger, M. D. (2004). Evolution of ischemic tissue injury in a random pattern flap: a new mouse model using intravital microscopy. J. Surg. Res. 121, 197–205. doi: 10.1016/j.jss.2004.03.026
Harris, E. S., and Nelson, W. J. (2010). VE-cadherin: at the front, center, and sides of endothelial cell organization and function. Curr. Opin. Cell Biol. 22, 651–658. doi: 10.1016/j.ceb.2010.07.006
Hoehenwarter, W., Tang, Y., Ackermann, R., Pleissner, K.-P., Schmid, M., Stein, R., et al. (2008). Identification of proteins that modify cataract of mouse eye lens. Proteomics 8, 5011–5024. doi: 10.1002/pmic.200800380
Ingangi, V., Bifulco, K., Yousif, A. M., Ragone, C., Motti, M. L., Rea, D., et al. (2016). The urokinase receptor-derived cyclic peptide [SRSRY] suppresses neovascularization and intravasation of osteosarcoma and chondrosarcoma cells. Oncotarget 7, 54474–54487. doi: 10.18632/oncotarget.9976
Jia, Y., Morand, E. F., Song, W., Cheng, Q., Stewart, A., and Yang, Y. H. (2013). Regulation of lung fibroblast activation by annexin A1. J. Cell. Physiol. 228, 476–484. doi: 10.1002/jcp.24156
Kreft, S., Klatt, A. R., Straßburger, J., Pöschl, E., Flower, R. J., Eming, S., et al. (2016). Skin wound repair is not altered in the absence of endogenous AnxA1 or AnxA5, but pharmacological Concentrations of AnxA4 and AnxA5 inhibit wound hemostasis. Cells Tissues Organs 201, 287–298. doi: 10.1159/000445106
Leikina, E., Defour, A., Melikov, K., Van der Meulen, J. H., Nagaraju, K., Bhuvanendran, S., et al. (2015). Annexin A1 deficiency does not affect myofiber repair but delays regeneration of injured muscles. Sci. Rep. 5:18246. doi: 10.1038/srep18246
Leoni, G., Alam, A., Neumann, P., Lambeth, J. D., Cheng, G., Mccoy, J., et al. (2013). Orchestrate Epithelial Repair. J. Clin. Invest. 123, 1–12. doi: 10.1172/JCI65831DS1
Leoni, G., Neumann, P.-A., Sumagin, R., Denning, T. L., and Nusrat, A. (2015). Wound repair: role of immune-epithelial interactions. Mucosal Immunol. 8, 959–968. doi: 10.1038/mi.2015.63
Leoni, G., and Nusrat, A. (2016). Annexin A1: shifting the balance towards resolution and repair. Biol. Chem. 397, 971–979. doi: 10.1515/hsz-2016-0180
Li, J., Chen, J., and Kirsner, R. (2007). Pathophysiology of acute wound healing. Clin. Dermatol. 25, 9–18. doi: 10.1016/j.clindermatol.2006.09.007
Lima, K. M., Vago, J. P., Caux, T. R., Negreiros-Lima, G. L., Sugimoto, M. A., Tavares, L. P., et al. (2017). The resolution of acute inflammation induced by cyclic AMP is dependent on annexin A1. J. Biol. Chem. 292, 13758–13773. doi: 10.1074/jbc.M117.800391
Liu, A., Huang, W., Zeng, G., Ma, X., Zhou, X., Wang, Y., et al. (2014). Expression of the Annexin A1 gene is associated with suppression of growth, invasion and metastasis of nasopharyngeal carcinoma. Mol. Med. Rep. 10, 3059–3067. doi: 10.3892/mmr.2014.2656
Lu, G., and Huang, S. (2013). Bioengineered skin substitutes: key elements and novel design for biomedical applications. Int. Wound J. 10, 365–371. doi: 10.1111/j.1742-481X.2012.01105.x
Marelli-Berg, F. M., Clement, M., Mauro, C., and Caligiuri, G. (2013). An immunologist’s guide to CD31 function in T-cells. J. Cell Sci. 126, 2343–2352. doi: 10.1242/jcs.124099
Martin, P., Hopkinson-Woolley, J., and McCluskey, J. (1992). Growth factors and cutaneous wound repair. Prog. Growth Factor Res. 4, 25–44.
Micallef, L., Vedrenne, N., Billet, F., Coulomb, B., Darby, I. A., and Desmoulière, A. (2012). The myofibroblast, multiple origins for major roles in normal and pathological tissue repair. Fibrogene. Tissue Repair 5:S5. doi: 10.1186/1755-1536-5-S1-S5
Mimura, K. K. O., Moraes, A. R., Miranda, A. C., Greco, R., Ansari, T., Sibbons, P., et al. (2016). Mechanisms underlying heterologous skin scaffold-mediated tissue remodeling. Sci. Rep. 6:35074. doi: 10.1038/srep35074
Moon, J. J., and West, J. L. (2008). Vascularization of engineered tissues: approaches to promote angio-genesis in biomaterials. Curr. Top. Med. Chem. 8, 300–310.
Ngo, M.-D., Aberman, H. M., Hawes, M. L., Choi, B., and Gertzman, A. A. (2011). Evaluation of human acellular dermis versus porcine acellular dermis in an in vivo model for incisional hernia repair. Cell Tissue Banking 12, 135–145. doi: 10.1007/s10561-011-9245-5
Oh, P., Li, Y., Yu, J., Durr, E., Krasinska, K. M., Carver, L. A., et al. (2004). Subtractive proteomic mapping of the endothelial surface in lung and solid tumours for tissue-specific therapy. Nature 429, 629–635. doi: 10.1038/nature02580
Park, S., Sorenson, C. M., and Sheibani, N. (2015). PECAM-1 isoforms, eNOS and endoglin axis in regulation of angiogenesis. Clin. Sci. 129, 217–234. doi: 10.1042/CS20140714
Park, S.-Y., Jang, W.-J., Yi, E.-Y., Jang, J.-Y., Jung, Y., Jeong, J.-W., et al. (2010). Melatonin suppresses tumor angiogenesis by inhibiting HIF-1alpha stabilization under hypoxia. J. Pineal Res. 48, 178–184.
Perretti, M., and D’Acquisto, F. (2009). Annexin A1 and glucocorticoids as effectors of the resolution of inflammation. Nat. Rev. Immunol. 9, 62–70. doi: 10.1038/nri2470
Perretti, M., and Flower, R. J. (2004). Annexin 1 and the biology of the neutrophil. J. Leukoc. Biol. 76, 25–29.
Pin, A.-L., Houle, F., Fournier, P., Guillonneau, M., Paquet, ÉR., Simard, M. J., et al. (2012). Annexin-1-mediated endothelial cell migration and angiogenesis are regulated by vascular endothelial growth factor (VEGF)-induced inhibition of miR-196a expression. J. Biol. Chem. 287, 30541–30551. doi: 10.1074/jbc.M112.393561
Prevete, N., Liotti, F., Illiano, A., Amoresano, A., Pucci, P., de Paulis, A., et al. (2017). Formyl peptide receptor 1 suppresses gastric cancer angiogenesis and growth by exploiting inflammation resolution pathways. OncoImmunology 6:e1293213. doi: 10.1080/2162402X.2017.1293213
Prevete, N., Liotti, F., Marone, G., Melillo, R. M., and de Paulis, A. (2015). Formyl peptide receptors at the interface of inflammation, angiogenesis and tumor growth. Pharmacol. Res. 102, 184–191. doi: 10.1016/j.phrs.2015.09.017
Privratsky, J. R., and Newman, P. J. (2014). PECAM-1: regulator of endothelial junctional integrity. Cell Tissue Res. 355, 607–619. doi: 10.1007/s00441-013-1779-3
Raynal, P., and Pollard, H. B. (1994). Annexins: the problem of assessing the biological role for a gene family of multifunctional calcium- and phospholipid-binding proteins. Biochim. Biophys. Acta 1197, 63–93.
Sengor, A., Aydin, O., Mola, F., and Gürbüz, Y. (2005). Evaluation of alloderm and autologous skin in quadriceps muscles of rats for injection laryngoplasty. Eur. Arch. Otorhinolaryngol. 262, 107–112. doi: 10.1007/s00405-004-0756-6
Solito, E., Christian, H. C., Festa, M., Mulla, A., Tierney, T., Flower, R. J., et al. (2006). Post-translational modification plays an essential role in the translocation of annexin A1 from the cytoplasm to the cell surface. FASEB J. 20, 1498–1500. doi: 10.1096/fj.05-5319fje
Teixeira, R. A. P., Mimura, K. K. O., Araujo, L. P., Greco, K. V., and Oliani, S. M. (2016). The essential role of annexin A1 mimetic peptide in the skin allograft survival. J. Tissue Eng. Regen. Med. 10, E44–E53. doi: 10.1002/term.1773
Xu, X., Yang, X., Xing, C., Zhang, S., and Cao, J. (2013). miRNA: the nemesis of gastric cancer (Review). Oncol. Lett. 6, 631–641. doi: 10.3892/ol.2013.1428
Yazid, S., Gardner, P. J., Carvalho, L., Chu, C. J., Flower, R. J., Solito, E., et al. (2015). Annexin-A1 restricts Th17 cells and attenuates the severity of autoimmune disease. J. Autoimmun. 58, 1–11. doi: 10.1016/j.jaut.2014.12.004
Yi, M., and Schnitzer, J. E. (2009). Impaired tumor growth, metastasis, angiogenesis and wound healing in annexin A1-null mice. Proc. Natl. Acad. Sci. U.S.A. 106, 17886–17891. doi: 10.1073/pnas.0901324106
Keywords: Ac2–26 peptide, dorsal skinfold chamber, HUVEC, VEGF-A, cytokines, fibroblast
Citation: Lacerda JZ, Drewes CC, Mimura KKO, Zanon CF, Ansari T, Gil CD, Greco KV, Farsky SHP and Oliani SM (2018) Annexin A12–26 Treatment Improves Skin Heterologous Transplantation by Modulating Inflammation and Angiogenesis Processes. Front. Pharmacol. 9:1015. doi: 10.3389/fphar.2018.01015
Received: 19 June 2018; Accepted: 20 August 2018;
Published: 10 September 2018.
Edited by:
Mauro Perretti, Queen Mary University of London, United KingdomReviewed by:
Giustino Orlando, Università degli Studi “G. d’Annunzio” Chieti - Pescara, ItalyClaudio Ferrante, Università degli Studi “G. d’Annunzio” Chieti - Pescara, Italy
Copyright © 2018 Lacerda, Drewes, Mimura, Zanon, Ansari, Gil, Greco, Farsky and Oliani. This is an open-access article distributed under the terms of the Creative Commons Attribution License (CC BY). The use, distribution or reproduction in other forums is permitted, provided the original author(s) and the copyright owner(s) are credited and that the original publication in this journal is cited, in accordance with accepted academic practice. No use, distribution or reproduction is permitted which does not comply with these terms.
*Correspondence: Sonia Maria Oliani, c29uaWEub2xpYW5pQHVuZXNwLmJy
†These authors have contributed equally to this work