- 1Graduate School, Beijing University of Chinese Medicine, Beijing, China
- 2Research Center for Chinese Medical Analysis and Transformation, Beijing University of Chinese Medicine, Beijing, China
- 3Department of Traditional Chinese Medicine Oncology, China-Japan Friendship Hospital, Beijing, China
Chemotherapy-induced peripheral neuropathy (CIPN) is a serious dose-limiting toxicity of many anti-neoplastic agents, especially paclitaxel, and oxaliplatin. Up to 62% of patients receiving paclitaxel regimens turn out to develop CIPN. Unfortunately, there are so few agents proved effective for prevention or management of CIPN. The reason for the current situation is that the mechanisms of CIPN are still not explicit. Traditional Chinese Medicine (TCM) has unique advantages for dealing with complex diseases. Wen-Luo-Tong (WLT) is a TCM ointment for topical application. It has been applied for prevention and management of CIPN clinically for more than 10 years. Previous animal experiments and clinical studies had manifested the availability of WLT. However, due to the unclear mechanisms of WLT, further transformation has been restricted. To investigate the therapeutic mechanisms of WLT, a metabolomic method on the basis of UPLC- MS was developed in this study. Multivariate analysis techniques, such as principal component analysis (PCA) and partial least squares discriminate analysis (PLS-DA), were applied to observe the disturbance in the metabolic state of the paclitaxel-induced peripheral neuropathy (PIPN) rat model, as well as the recovering tendency of WLT treatment. A total of 19 significant variations associated with PIPN were identified as biomarkers. Results of pathway analysis indicated that the metabolic disturbance of pathways of linoleic acid (LA) metabolism and glycerophospholipid metabolism. WLT attenuated mechanical allodynia and rebalanced the metabolic disturbances of PIPN by primarily regulating LA and glycerophospholipid metabolism pathway. Further molecular docking analysis showed some ingredients of WLT, such as hydroxysafflor yellow A (HSYA), icariin, epimedin B and 4-dihydroxybenzoic acid (DHBA), had high affinity to plenty of proteins within these two pathways.
Introduction
Chemotherapy-induced peripheral neuropathy (CIPN) is a common treatment-related adverse effect of many anti-neoplastic agents. It is a serious dose-limiting toxicity characterized by distal, symmetrical, sensory peripheral neuropathy. These sensory neuropathy symptoms generally present as paraesthesia, numbness and/or pain, which severely affect the patient's quality of life (QOL). Although recent advances in cancer treatment have led to prolonged survival of patients, many treatment-related adverse effects remain unsolved (Wolf et al., 2008; Hershman et al., 2011, 2014). There are few established agents recommended for the prevention or management of CIPN to cancer survivals undergoing anti-neoplastic treatment with neurotoxic agents (Hershman et al., 2014), such as paclitaxel, oxaliplatin, vinorelbine, et al. Paclitaxel, as an effective anti-neoplastic agent, is widely used as first-line treatment for various types of cancer, including of breast, ovarian, and non-small cell lung cancer. However, CIPN is a significant problem for patients receiving paclitaxel regimens. Up to 62% of patients turn out to develop CIPN after paclitaxel regimens (Lee and Swain, 2006; Argyriou et al., 2008; Reyes-Gibby et al., 2009). It has been reported that mitochondrial damage (André et al., 2000; Bernardi et al., 2006; Flatters and Bennett, 2006; Martin et al., 2009), intraepidermal nerve fibers degeneration (Flatters and Bennett, 2006), ion channels alteration (Xiao et al., 2007; Nieto et al., 2008; Zhang et al., 2014), transient receptors potential (TRP) family (Alessandri-Haber et al., 2008; Materazzi et al., 2012), inflammation and immune status (Ledeboer et al., 2007; Dutra et al., 2015; Li D. et al., 2015) are associated with paclitaxel-induced peripheral neuropathy (PIPN). However, the mechanisms remain unclear. An explicit mechanism is the precondition of discovery for eutherapeutic drug or treatment, thus it's very important and urgent to understand its mechanism.
After more than several decades of efforts, the treatment against cancer and its complications still appears far from being satisfying. It has gradually become clear that the “one gene-one target-one drug” model has failed for drug discovery. It appears that monotherapy strategy will be replaced by rational combination targeted therapy (Haefner, 2006). It is necessary to analyze the systems' response to drug treatments, not just one target or pathway. Therefore, is very important to reveal the abnormal metabolisms through a systematic perspective for understanding the mechanisms of disease, as well as for developing drugs. Metabolomics is a collection of powerful tools for detecting, identifying and quantifying the endogenous metabolites that are involved in the metabolisms, and then interpreting biological changes of the internal environment (Nicholson and Lindon, 2008). It has been increasingly used in the researches aiming to explore the mechanisms of diseases or treatments, as it could provide comprehensive metabolic information of organisms (Weckwerth, 2003, 2010; Glinski and Weckwerth, 2006; Fang and Gonzalez, 2014; Wang et al., 2015).
Currently, drug combinations and multi-target therapies have been proposed as a promising therapeutic strategy for improving anti-neoplastic effects, and relieving the side effects. Traditional Chinese Medicine (TCM) deals with diseases from the perspective of holism. The TCM decoctions are commonly composed of several herbs with complex constituents of compound. Metabolomics has been used as important technique for interpreting pharmacological mechanisms of TCM formulae under the guidance of systematic theory (Li et al., 2011; Li and Zhang, 2013). Indeed, there have been an increasing number of TCM studies applying metaboliomics (Lu et al., 2014; Cao et al., 2015; Wang et al., 2015, 2017). Wen-Luo-Tong (WLT) is an herbal formula ointment for topical application. It had been applied to prevention and management for CIPN in clinical practice for more than 10 years. The formula was constituted by epimedium herb, geranium wilfordii, cassia twig, and carthamus tinctorius (Table 1). The components are supposed to act synergistically to achieve the TCM treatment principle of Warming and Activating Meridian, Promoting Blood Circulation and Alleviating Pain. Moreover, previous clinical trial and animal experiment manifested it had effect of analgesic and neuroprotection over CIPN. The clinical trial showed that after 7 days WLT treatment, numerical rating scale (NRS) in trial group was significantly decreased while control group did not. And the response rate of pain relief was 85.07% in trial group while 44.12% in control group (p < 0.01). In addition, symptoms of 75.00% subjects in trial group were improved by grading, while 35.29% in control group. The animal experiment showed WLT treatment alleviated oxaliplatin-induced mechanical allodynia and mechanical hyperalgesia. Degenerations in the nuclear, and nucleolar areas of neurons in dorsal root ganglion (DRG) were attenuated. In the spinal dorsal horn, hypertrophy and activation of glial fibrillary acidic portein (GFAP) positive astrocytes were averted, and the level of GFAP mRNA decreased significantly (Lou et al., 2008, 2014; Deng et al., 2017). Therefore, decipher the mechanisms of WLT based on metabolomics will provide a global insight into the actions of neurotoxic agents and WLT, which helps to understand the mechanisms and further helps to discovery new drug or treatment.
Our study aimed to investigate the metabolic disturbances of PIPN through untargeted metabolomic assays. Additionally, taking WLT as probe, metabolomic alterations were analyzed to provide some clues for CIPN mechanism explorations.
Materials and Methods
Paclitaxel and WLT Preparation
Paclitaxel (6 mg/ml, Beijing Union Pharmaceuticals, Beijing, China) was diluted with saline (1:3) (Li D. et al., 2015). WLT decoction was authenticated by both Ethics Committee and Pharmaceutical Department of China-Japan Friendship Hospital. Herbs of WLT (Table 1) were purchased from pharmacy of China-Japan Friendship Hospital (Beijing, China). WLT decoction was prepared into granula with individual package (composes and dosage of each package showed in Table 1) by the Pharmaceutical Department of China-Japan Friendship Hospital, following the technological process shown in Supplementary Figure 1. (Manufacturer production lot number: 201610211416).
Animal Experiments and Sample Collection
Female Sprague-Dawley rats weighing 160–200 g were purchased from Beijing Vital River Laboratory Animal Technology Co., Ltd. (Beijing, China). All rats were housed in automatically controlled environmental conditions, using a 12 h light–dark cycle (lights on from 08:00 to 20:00) with free access to food and water. All animal experiment protocols were approved by the Animal Care and Welfare Committee of China-Japan Friendship Hospital (Beijing, China), approval No. 160105. The investigation was conducted in accordance with the ethical principles of animal use and care.
After 3 days adaption, rats were randomly allocated into three groups as follows: PTX, WLT, Control. Animals of PTX and WLT were intraperitoneally (i.p.) injected (8 mg/kg, cumulative dose of 24 mg/kg) on 3 alternate days (d1, 4, 7) (Li D. et al., 2015). Animals of Control received an equivalent volume of saline. One package of WLT (Table 1) was dissolved into 1,000 ml deionized water (40°C) before application. Animals in WLT group shared one batch of WLT solution together. Animals took pediluvium of WLT or water for 30 min, twice a day (Supplementary Figure 4). The intervention was initiated 1 day before paclitaxel administration and lasted for 11 days (d0-10). Rats in PTX and Control took pediluvium of deionized water. The route of operation was as follow: behavioral test, pediluvium, intraperitoneal injection (Deng et al., 2016).
Mechanical paw withdrawal threshold (PWT) was tested before, during, and after paclitaxel treatment (d0, 2, 4, 6, 8, and 10) by an experimenter blinded to treatment groups (Li D. et al., 2015). Rats were placed in elevated plexiglas chambers upon a wire mesh floor and allowed to acclimate for 15 min prior to measuring the PWT. PWT was tested using calibrated von Frey filaments according to the “up and down” method (Dixon, 1980; Chaplan et al., 1994). The development of mechanical allodynia was evidenced by a significant (p < 0.05) reduction in mean absolute PWT (g) at forces that failed to elicit withdrawal responses before treatment (baseline or D0). PWT testing was done prior to any other administration or intervention.
Blood and tissue samples were collected on day 10 after tests and interventions. Rats were anesthetized with intraperitoneal injection of sodium pentobarbital (50 mg/kg). Blood samples were centrifuged at 3,000 rpm for 10 min. Then 500 μL plasma was extracted. All the samples were stored in a −80°C freezer for further analysis. L4–6 spinal cord segments were removed, fixed in 4% paraformaldehyde overnight, embedded in paraffin and cut into 5 μm thickness sections. The scheme of the whole experiment was shown in Figure 1.
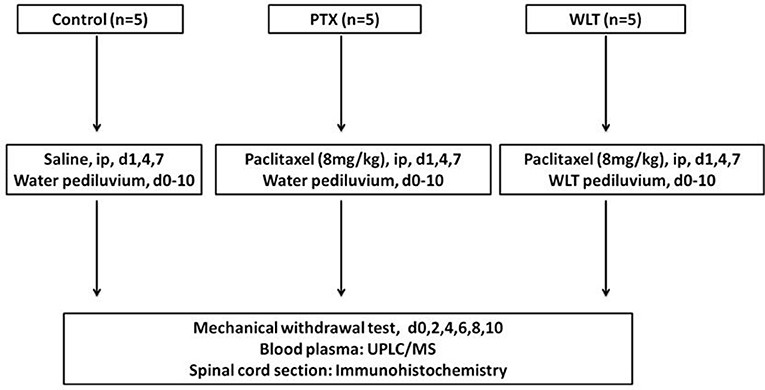
Figure 1. Experimental design. Animals of Control, PTX and WLT received paclitaxel/saline injection and WLT/water pediluvium during the experiment. The PIPN model was constructed by administration of paclitaxel at 8 mg/kg by intraperitoneal injection on d1, 4, 7. WLT solution was given tropically by pediiluvium twice a day for 11 consecutive days (d0-10). The behavior assay was monitored every other day (d0, 2, 4, 6, 8, 10). The rats were finally euthanized on day 10. Plasma and spinal cord samples were collected for preparation and analysis.
Immunohistochemistry
Sections of the L4–6 spinal cord were processed for immunohistochemistry using a primary antibody against Chemokine (C-X3-C motif) ligand 1 (CX3CL1) (1:200, Bosterbio, China). Following incubation overnight at 4°C, sections were incubated in a horseradish peroxidase-conjugated secondary antibody for 4 h at room temperature. The color was developed with 3,3′-diaminobenzidine (DAB).
Instrumentation for LC-MS
An ultra-performance liquid chromatography-electrospray ionization-mass spectrometer (UPLC-ESI-MS) was used. It was equipped with hybrid quadrupole Orbitrap mass spectrometer and ThermoFisher Scientific Q Exactive (Thermo Fisher Scientific, Inc., Waltham, MA, United States). The sample solutions were separated using a HSS C18 column (2.1 × 100 mm, 1.7 μm, Waters) at 45°C. For C18 separation, mobile phase A was water and mobile phase B was acetonitrile. Both A and B contained 0.1% formic acid and 1 mmol/L ammonium acetate. The flow rate was 300 μL/min and the injection volume was 1 μL. The positive and negative HESI-II spray voltages were 3.7 and 3.5 kV, respectively, the heated capillary temperature was 320°C, the sheath gas pressure was 30 psi, the auxiliary gas setting was 10 psi, and the heated vaporizer temperature was 300°C. Both the sheath gas and the auxiliary gas were nitrogen. The collision gas was also nitrogen at a pressure of 1.5 mTorr. The parameters of the full mass scan were as follows: a resolution of 70,000, an auto gain control target under 1 × 106, a maximum isolation time of 50 ms, and an m/z range 150–1,500. The calibration was customized for the analysis of Q Exactive to keep the mass tolerance of 5 ppm. The parameters of the dd-MS2 scan were as follows: a resolution of 17,500, an auto gain control target under 1 × 105, a maximum isolation time of 50 ms, a loop count of top 10 peaks, an isolation window of m/z 2, normalized collision energy of 30 V and an intensity threshold under 1 × 105. The LC-MS system was controlled using Xcalibur 2.2 SP1.48 software (Thermo Fisher Scientific, United States), and data were collected and processed with the same software.
All data obtained from the assays in the two systems of both positive and negative ion modes were processed using Progenesis QI data analysis software (Nonlinear Dynamics, Newcastle, UK) for imputing raw data, peak alignment, picking, and normalization to produce peak intensities for retention time (tR) and m/z data pairs. The ranges of automatic peak picking for the C18 assays were between 0.7 and 19 min. Then, the adduct ions of each “feature” (m/z, tR) were deconvoluted, and these features were identified in the human metabolome database (HMDB, http://www.hmdb.ca/) and Lipidmaps (http://www.lipidmaps.org/).
Quality Control
To monitor the system's stability and performance as well as the reproducibility of the samples, quality control samples (QCs) were prepared by pooling equal volumes of each sample. The pretreatment of QCs was in accord with real samples. For repeatable metabolic analyses, three features of the analytical system must be stable: (i) retention time, (ii) signal intensity and (iii) mass accuracy. In this study, three QCs were continuously injected at the beginning of the run. QCs are then injected at regular intervals throughout the analytical run in order to provide data from which repeatability can be assessed. The features were selected based on their coefficients of variation (CVs) with QCs; features with CVs over 15 % were eliminated.
Statistical Analysis
Statistical analysis of behavioral test was performed using SPSS 19.0 (IBM, USA). Results of pathological scores were analyzed using IPP 6.0 (Intel, USA). The multivariate data analysis, principal component analysis (PCA) and partial least squares discriminate analysis (PLS-DA) were conducted by EZinfo 3.0 (Umetrics, Sweden). Metabolism pathway analysis was conducted by MetaboAnalyst 3.0 (http://www.metaboanalyst.ca/). Enzymes related to metabolites were collected by HMDB 4.0 (http://www.hmdb.ca/). Protein-protein interactions with confidence >0.9 in the STRING 10.5 (https://string-db.org) were used to construct a network containing relationships between enzymes and proteins. The structures of WLT ingredients were downloaded from PubChem (http://pubchem.ncbi.nlm.nih.gov). The structures of proteins were obtained from the Protein Data Bank (PDB, https://www.rcsb.org/). Protein structures not available from PDB was homology modeled by (https://www.swissmodel.expasy.org) docking was carried out with Discovery Studio 3.5 (BIOVIA, USA). Parametric data was analyzed by ANOVA or Student's t-test for, and non-parametric data by Mann–Whitney U-test or Kruskal-Wallis. Differences were considered the results with P-values < 0.05.
Results
WLT Attenuated Paclitaxel-Induced Peripheral Neuropathy
Consistent with previous studies (Huang et al., 2014; Li D. et al., 2015), administration with paclitaxel (3 × 8 mg/kg, cumulative dose 24 mg/kg, equivalent to average dose of clinical practice) induced marked mechanical allodynia (Figure 2A). The PWT of both WLT and PTX was greatly decreased compared with Control group. However, co-administrated with WLT alleviated PWT decrease. PWT of PTX group was decreased significantly from 20.5 ± 5.88 to 4.5 ± 0.93 g. Statistical difference between PTX and Control had been shown since day 4. While that of WLT group was from 20.5 ± 5.88 to 8.0 ± 1.07 g. Statistical difference between PTX and WLT had been shown since day 6. According to previous studies, it had been revealed that up-regulation of CX3CL1 was involved with the neuropathic pain induced by nerve injury. Immunochemistry staining of our study showed that paclitaxel increased the expression of CX3CL1 in neurons and dendrites of spinal cord sections. Meanwhile, compared with PTX group, CX3CL1 expression in WLT was markedly down-regulated (Figure 2B). The results of behavioral test and immunochemistry demonstrated that WLT attenuated paclitaxel-induced peripheral neuropathy.
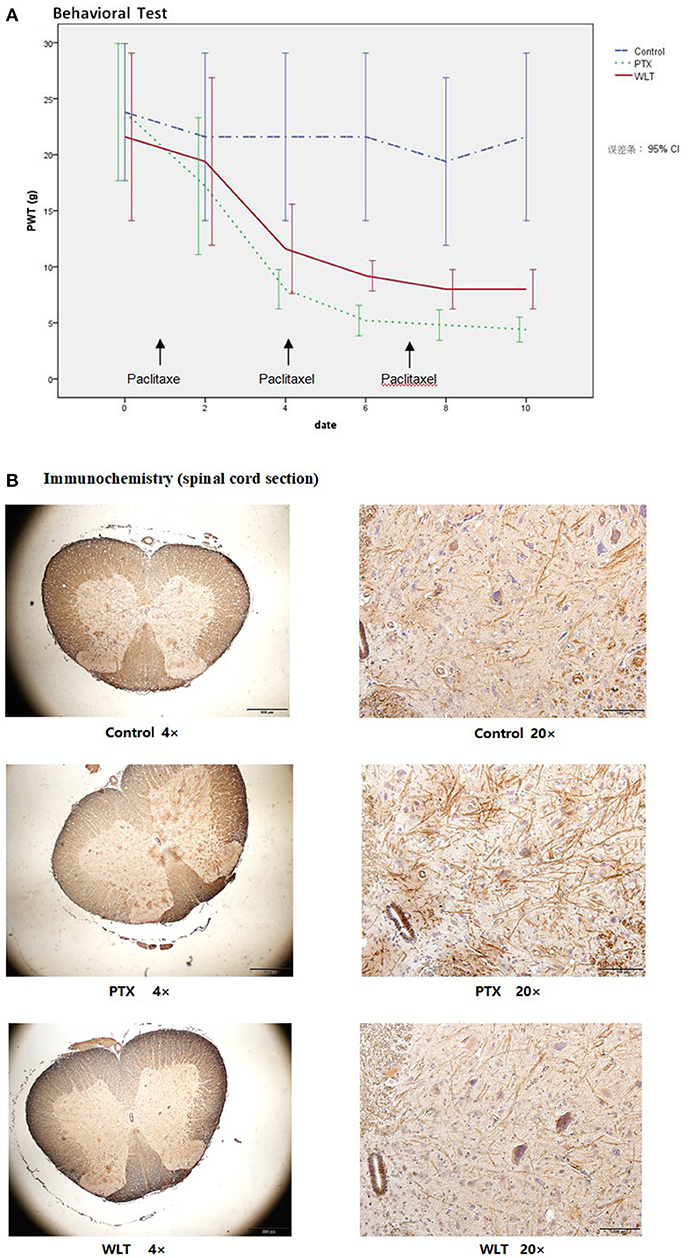
Figure 2. WLT attenuated Paclitaxel-induced Peripheral Neuropathy. (A) Paw withdraw threshold (PWT) of PTX and WLT group was decreased significantly after injection of paclitaxel (8 mg/kg, i.p.). Statistical difference had been shown since day 4. Compared with PTX group, WLT attenuated the mechanical allodynia. Significant difference was shown since day 6 (p < 0.05). (B) Immunochemistry staining showed that paclitaxel induced up-regulation of CX3CL1 expression in neurons and dendrites. The expression of CX3CL1 in WLT was down-regulated.
Metabolic Disturbances of Paclitaxel-Induced Peripherial Neuropathy
PCA manifested paclitaxel induced obvious metabolic disturbances. QCs were applied in assessing system stability. The PCA plots showed a clear discrimination between Control and PTX groups, while QCs highly clustered (Supplementary Figure 2), indicating that the PIPN model was successfully duplicated and our analytical method was of good reproducibility and stability.
To identify the significant metabolic disturbances of PIPN, PLS-DA models of PTX and Control were further performed. The score plots demonstrated a satisfactory separation between Control and PTX (Figure 3A, positive: R2X = 0.799, Q2 = 0.642; Figure 3B, negative: R2X = 0.906, Q2 = 0.847). The variable importance in the projection (VIP) value of each variable in the model was ranked according to its contribution to the classification. The VIP list of the retention time-exact mass pairs was obtained from the PLS-DA using EZinfo 3.0. To select the potential biomarkers worthy of preferential study in the next step, these differential metabolites were validated using the Student's t-test. The critical p-value was set to 0.05 for the significantly different variables in this study. Following the criteria above, 19 differential metabolites were identified (Table 2). Most of the metabolites were lipids (3 phosphatidylcholines and 6 lysophospholipid), fatty acid or organic acid (LA, N-Undecylbenzenesulfonic acid, and Ricinoleic acid), ketones ((±)-(Z)-2-(5-Tetradecenyl) cyclobutanone and Suprofen S-oxide). To show the variation trend of the significant metabolites, a heatmap was drawn according to relative concentrations of the differential metabolites (Figure 4A). As shown in Figure 4A, 12 metabolites were down-regulated in PTX, including all the PCs and lysoPCs, Indoxyl sulfate, Suprofen S-oxide, and 4-ethylphenylsulfate. The other 7 metabolites were up-regulated. These results revealed the potential role of lipids in the metabolic response to paclitaxel.
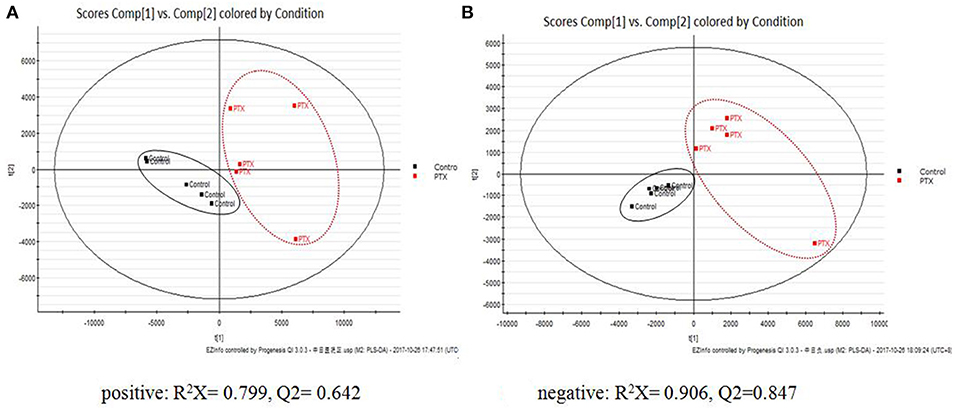
Figure 3. PLS-DA Plots of PIPN Metabolic Disturbances. The PLS-DA plots showed clear separation between Control and PTX. (A) Positive: R2X = 0.799, Q2 = 0.642. (B) Negative: R2X = 0.906, Q2 = 0.847.
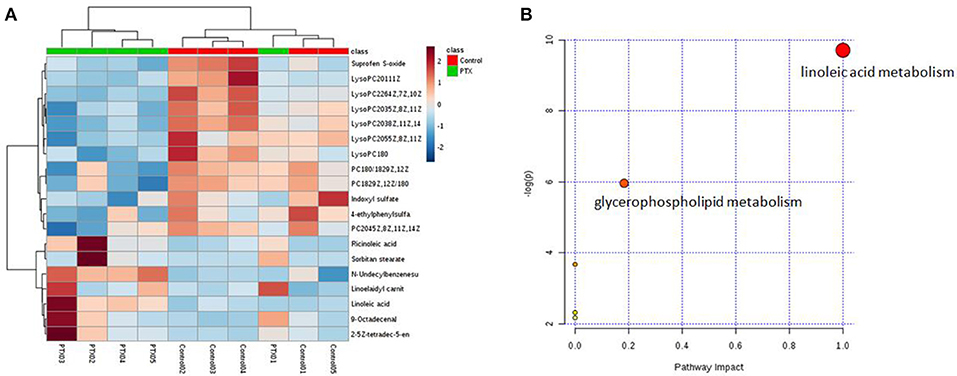
Figure 4. Metabolites variation analysis. (A) Heatmap of metabolite concentrations. Heatmap showed the relative concentrations of differential metabolites in each sample. (B) Metabolism Pathway Affected by Paclitaxel Treatment. The result of the significant metabolites analysis showed that paclitaxel primarily disturbed the linoleic acid and glycerophospholipid metabolisms. Linoleic acid metabolism was a preferred target pathway of paclitaxel (impact 1.0).
To further investigate the metabolism pathway affected by paclitaxel treatment, the significant metabolites were analyzed using Metaboanalyst 3.0 (http://www.metaboanalyst.ca/), a free, web-based tool that combines metabolites with the pathway analysis to help researchers to identify the relevant pathways under their study conditions (Xia et al., 2015; Xia and Wishart, 2016). Metabolites in Table 2 were input into Metaboanalyst and the metabolic networks were depicted (Figure 4B). The result showed that paclitaxel primarily disturbed the LA metabolism (impact 1.0) and glycerophospholipid metabolism (impact 0.18), which was in accord with published literatures (Patwardhan et al., 2010; Wang et al., 2013; Sisignano et al., 2016). And LA metabolism was considered as a preferred target pathway of paclitaxel due to its significant impact score. LA, PC(20:4(5Z,8Z,11Z,14Z)/18:0), PC(18:0/18:2(9Z,12Z)), PC(18:2(9Z,12Z)/18:0) were key metabolites of LA metabolism pathway. While LysoPC(20:5(5Z,8Z,11Z, 14Z,17Z)), LysoPC(22:6(4Z,7Z,10Z,13Z,16Z,19Z)), LysoPC(20:3 (8Z,11Z, 14Z)), LysoPC(18:0), LysoPC(20:3(5Z,8Z,11Z)), LysoPC(20:1(11Z)) were key metabolites of glycerophospholipid metabolism pathway (Supplementary Figure 3). Thus, paclitaxel induced peripheral neuropathy by primarily targeting LA metabolism and glycerophospholipid metabolism pathways.
WLT Rebalanced the Metabolic Disturbances
WLT treatment rebalanced the alteration of most metabolites disturbed by paclitaxel. The expression of 14 disturbed metabolites appeared to be rebalanced, including most of the key metabolites in LA metabolism and glycerophospholipid metabolism pathways (Figure 5). Especially, LA, PC(20:4(5Z,8Z,11Z,14Z)/18:0), LysoPC(20:3(5Z,8Z,11Z)), LysoPC(20:3(8Z,11Z,14Z)), LysoPC(20:5(5Z,8Z,11Z,14Z,17Z)), LysoPC(20:1(11Z)), LysoPC(22:6(4Z,7Z,10Z,13Z,16Z,19Z)), LysoPC(18:0) were rebalanced after WLT treatment. In addition, indoxyl sulfate, linoelaidyl carnitine, 4-ethylphenylsulfate, and Suprofen S-oxide were also regulated by WLT treatment.
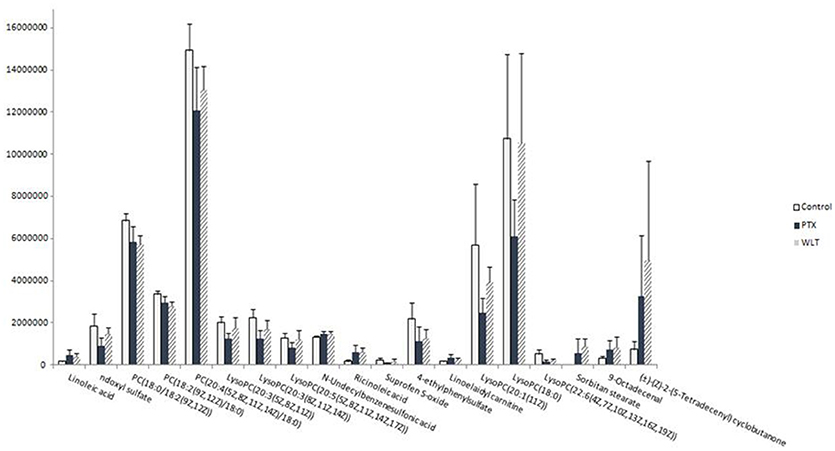
Figure 5. Effects of WLT on Regulating the Metabolic Disturbances. WLT rebalanced most metabolic disturbances caused by paclitaxel.
To investigate the underlying mechanism of WLT, the metabolite–protein interaction networks were constructed. Nineteen enzymes related to the regulated metabolites were collected from HMDB (Table 3). Next, interaction networks of the enzymes and proteins were constructed by STRING. Interactions with highest confidence (interaction score = 0.9) were considered as active interactions (Figure 6A). As shown, pla2g15, lypla1, and plb1 were 3 most interactive enzymes. Their interaction networks of the enzymes and proteins were analyzed (Figures 6B–D). Correspondingly, the 27 proteins with highest confidence (interaction score = 0.9) were considered as possible targets network for the metabolites (Table 4). Additionally, the metabolite-related enzymes and proteins were mapped in the biological pathways to explore the metabolite-related signal pathways in DAVID 6.8. The 2 important biological pathways, including LA metabolism pathway, and glycerophospholipid metabolism pathway, were showed in Figure 7. The potential targets of WLT were marked in the pathways.
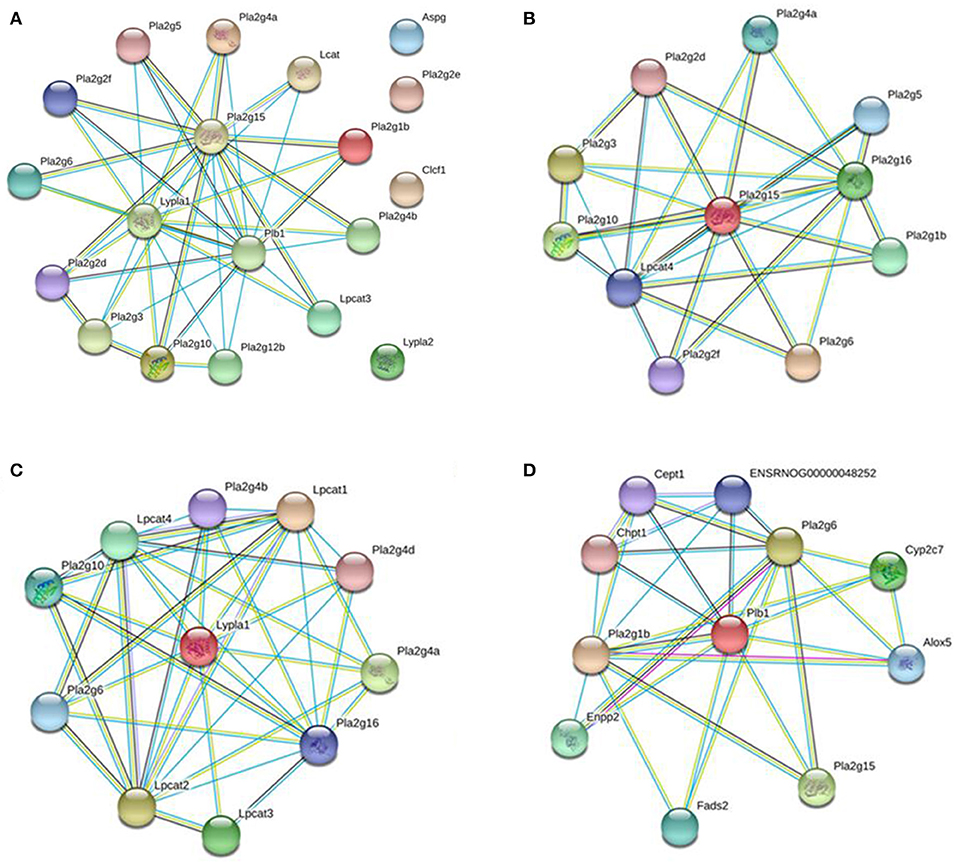
Figure 6. Interaction Networks of Enzymes and Proteins. (A) Functional interaction networks of the key enzymes were collected and input into the STRING database to analyze interactions between them (interaction score = 0.9). Pla2g15, lypla1, and plb1 were 3 most interactive enzymes. Functional interaction networks of pla2g15 (B), lypla1 (C), plb1 (D). Only proteins with highest confidence (interaction score = 0.9) were considered as possible targets network for the metabolites.
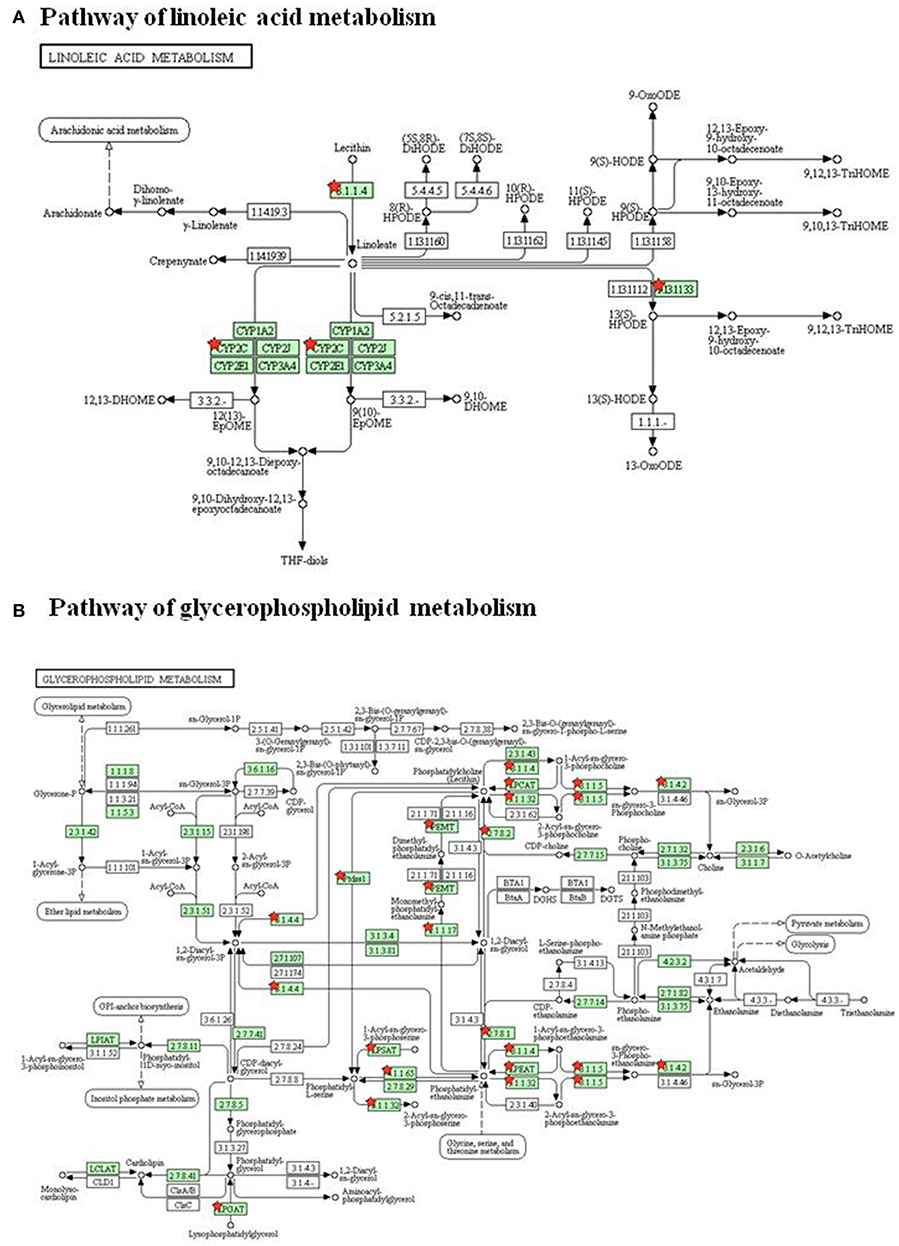
Figure 7. Biological Pathways of WLT treatment. (A) Pathway of linoleic acid metabolism. (B) Pathway of glycerophospholipid metabolism. The red stars mark potential targets of WLT in pathways.
To further investigate the affinity between ingredients and potential targets of WLT, molecular docking was performed. A previous pharmaceutical study of WLT identified that HSYA, icariin, epimedin B and 4-DHBA (Table 5) penetrated through the skin, and they had significant protective effects on Schwann cells after injured by chemotherapy (Lin et al., 2017). Therefore, molecular docking was performed between these 4 active ingredients and the potential protein network to predict the probable targets of WLT against PIPN. The docking results revealed that there were high affinities between HSYA and alox15, alox5, cept1, cyp2c7, pla2g1b, pla2g10, pla2g4a, pla2g4d, pla2g7, and ptgs1. Similarly, high affinities were seen between Epimedin B, Icariin and alox5, chpt1, pla2g1b, pla2g4a, pla2g7, ptgs1, as well as between 4-DHBA and most key proteins except pafah2, pla2g16 (Table 6).
Discussion
CIPN is a serious dose-limiting toxicity of many anti-neoplastic agents, especially paclitaxel and oxaliplatin. Because the mechanisms are not explicit, there is hardly any effective agent for the prevention or management of CIPN. Previous studies showed that WLT, a topical applied ointment with herbal formula, was helpful to prevent and alleviate CIPN. This study was aimed to explore the mechanisms behind it. We identified 19 significant changed metabolites of PIPN, most of which were lipids (LysoPCs or PCs), organic acids, and ketones. Metabolism pathway study demonstrated that paclitaxel induced peripheral neuropathy mainly by targeting LA metabolism pathway and glycerophospholipid metabolism pathway. Correspondingly, WLT attenuated symptoms of PIPN by primarily rebalancing these two pathways. As a pilot study, we explored the methodology of metabolomics and network pharmacology on PIPN. The findings of pathways and potential targets might provide important clues for further mechanism studies and clinical improvement.
CIPN is a complex topic. Although the anti-neoplastic features are well described, no explicit pathophysiological process can be identified to explain the various neuropathies (Addington and Freimer, 2016). The neurotoxic side effects may be related to multifactorial metabolism pathways. Studies have documented that processes of neurotransmitter signaling, such as glutamate and γ-aminobutyric acid, are involved with CIPN occurrence (Cata et al., 2006; Carozzi et al., 2015). Caspase signaling is also found to contribute to PIPN, leading to the generation of reactive oxygen species (ROS), as well as potential apoptosis (Park et al., 2004). Additionally, pathways activate inflammatory cytokines, such as TNF-α, MAPK, and CX3CL1, are involved with CIPN as well (Cavaletti et al., 2000; Zhang et al., 2010; Brandolini et al., 2017). Moreover, recent evidences indicated that activation of TRPV1 during chemotherapy sensitizes pain pathways. Recent studies identified members of the TRP family of ion channels (TRPV1, TRPA1, and TRPV4) as contributors to mechanical allodynia during PIPN. However, it remains unclear which endogenous mediators were involved with paclitaxel-induced activation or sensitization of TRP channels, as paclitaxel cannot directly activate TRP channels (Alessandri-Haber et al., 2008; Chen et al., 2011; Jang et al., 2012; Materazzi et al., 2012; Hara et al., 2013; Boyette-Davis et al., 2015; Li Y. et al., 2015). In present study, we found that LA metabolism pathway was a key pathways contributed to PIPN. LA is a doubly unsaturated fatty acid, also known as an omega-6 fatty acid. It is used in the biosynthesis of prostaglandins (via arachidonic acid) and cell membranes. This finding is supported by other studies. Paclitaxel is an inducer of some Cytochrome-P450 epoxygenases (e.g., CYP2C8, CYP2C9), which can metabolize ω-6 fatty acids, such as arachidonic acid (AA) and LA (Dai et al., 2001). Another study identified that 9,10-EpOME (9,10-epoxy-12Z-octadecenoic acid), a CYP metabolite of LA, was strongly synthesized in DRGs after paclitaxel injection. Injection of 9,10-EpOME alone caused significant reduction of the mechanical thresholds of wild-type mice too. Further, decreasing 9,10-EpOME concentrations in DRGs and in plasma reversed mechanical hypersensitivity in paclitaxel treated mice (Sisignano et al., 2016). Glycerophospholipid are the main component of neural membranes. The main function of glycerophospholipids in the neural membranes is to provide stability, permeability and fluidity through specific alterations in their compositions (Glynn, 2013; Ruiz et al., 2015). Previous study demonstrated that the proportion of glycerophospholipid decreased significantly in diabetic neuropathy rats (Kuruvilla and Eichberg, 1998).
According to TCM theory, CIPN was blamed to Meridian obstruction due to cold congealing. Consequently, based on syndrome differentiation and the holistic theory, WLT was developed to act the effect of Warming and Activating Meridian, Promoting Blood Circulation, and Alleviating Pain. It was constitute of four herbs, which were epimedium herb, geranium wilfordii, cassia twig, and carthamus tinctorius. Respectively, epimedium herb, acting as Monarch (the most important constituent), was used to tonify Kidney Yang and dispel Wind-Damp. Geranium wilfordii, acting as Minister (the second important constituent), was used to expel Wind and dredging Collat. cassia twig, acting as Assistance (a constituent helps Monarch or/and Minister to achieve their effects), was used to warm and activate Meridian and reinforce Yang to promote the flow of Qi. Carthamus tinctorius, acting as Assistance (a constituent helps Monarch or/and Minister to achieve their effects), was used to promote blood circulation and remove Meridian obstruction. The herbs of WLT were supposed to act synergistically and achieve the effect of Warming and Activating Meridian, Promoting Blood Circulation and Alleviating Pain. Meanwhile, some pharmacological studies of herbs also indicated that constituents of WLT could be helpful for alleviating neuropathy (Kou et al., 2013; Sun et al., 2016). A study showed that epimedium extract promotes peripheral nerve regeneration in rats (Oh et al., 2015). Another study showed icariin, a flavonoid glycosides extracted from epimedium herb, promoted the expression of nNOs after neural injury (Shindel et al., 2010). It was also reported that cassia twig, geranium wilfordii and carthamus tinctorius had anti-inflammatory, antioxidative, neurorotective and analgesic effects (Gan et al., 2010; Wang et al., 2014; Yue et al., 2014; Gunawardena et al., 2015; Huang et al., 2015; Jo et al., 2017). According to previous study, we took 4 active ingredients of WLT as probe to conduct molecular docking with potential targets obtained from metabolomic analysis. Published literatures showed their neuroprotective effects. A study revealed that HSYA showed neuroprotection through attenuating oxidative stress, inflammatory response, and neural apoptosis (Pei et al., 2017). Another study showed that Icariin could be a potential agent for the treatment of paclitaxel-induced neuropathic pain in a SIRT1-dependent manner (Gui et al., 2018). Another study suggested that 3,4-DHBA prevented neuronal cell damage by interfering with the increase of Ca2+ (Ban et al., 2007). The docking results showed complex interactions between them, demonstrating the characteristics of multi-components and multi-targets. The ingredients showed high affinity to most proteins, except pafah2, pla2g16, and ENSRNOG00000048252. Some proteins showed complex interactions with more than 1 ingredient, such as alox15, alox5, cept1, chpt1, cyp4f6, cyp2c7, pla2g1b, pla2g10, pla2g4a, pla2g4d, pla2g7, and ptgs1. The acting sites in LA pathway and glycerophospholipid pathway of these proteins were marked in Figure 7.
Conclusion
In this study, LC-MS based non-targeted metabolomics method revealed the metabolic disturbances induced by paclitaxel, and the effects of WLT on alleviating PIPN. Paclitaxel primarily disturbed the LA and glycerophospholipid metabolism pathways to induce peripheral neuropathy. Further study demonstrated that WLT attenuated PIPN by regulating these two pathways in the manner of multi-target interference.
Author Contributions
FW participated in research design, carried out most of the studies, performed statistical analysis, and wrote the manuscript. WX performed data analysis and contributed to the writing and modification of the manuscript. BD and CD provided professional advices for research design and manuscript revising. SL, MW, and YG participated in the animal experiments. LJ provided professional advices for research design, statistical analysis, and manuscript revising.
Funding
This study was supported by National Natural Science Foundation of China (grant No. 81173421 and 81603462), and Beijing Municipal Science & Technology Commission (grant No. Z151100003815019).
Conflict of Interest Statement
The authors declare that the research was conducted in the absence of any commercial or financial relationships that could be construed as a potential conflict of interest.
The reviewer JC declared a shared affiliation, with no collaboration, with one of the authors, FW, to the handling editor at the time of the review.
Supplementary Material
The Supplementary Material for this article can be found online at: https://www.frontiersin.org/articles/10.3389/fphar.2018.00956/full#supplementary-material
References
Addington, J., and Freimer, M. (2016). Chemotherapy-induced peripheral neuropathy: an update on the current understanding. F1000Res 5, 1460–1466. doi: 10.12688/f1000research.8053.1
Alessandri-Haber, N., Dina, O. A., Joseph, E. K., Reichling, D. B., and Levine, J. D. (2008). Interaction of transient receptor potential vanilloid 4, integrin, and SRC tyrosine kinase in mechanical hyperalgesia. J. Neurosci. 28, 1046–1057. doi: 10.1523/JNEUROSCI.4497-07.2008
André, N., Braguer, D., Brasseur, G., Gonçalves, A., Lemesle-Meunier, D., Guise, S., et al. (2000). Paclitaxel induces release of cytochrome c from mitochondria isolated from human neuroblastoma cells. Cancer Res. 60, 5349–5353.
Argyriou, A. A., Koltzenburg, M., Polychronopoulos, P., Papapetropoulos, S., and Kalofonos, H. P. (2008). Peripheral nerve damage associated with administration of taxanes in patients with cancer. Crit. Rev. Oncol. Hematol. 66, 218–228. doi: 10.1016/j.critrevonc.2008.01.008
Ban, J. Y., Cho, S. O., Jeon, S. Y., Bae, K., Song, K. S., and Seong, Y. H. (2007). 3,4-dihydroxybenzoic acid from Smilacis chinae rhizome protects amyloid beta protein (25-35)-induced neurotoxicity in cultured rat cortical neurons. Neurosci. Lett. 420, 184–188. doi: 10.1016/j.neulet.2007.05.009
Bernardi, P., Krauskopf, A., Basso, E., Petronilli, V., Blachly-Dyson, E., Di Lisa, F., et al. (2006). The mitochondrial permeability transition from in vitro artifact to disease target. FEBS J. 273, 2077–2099. doi: 10.1111/j.1742-4658.2006.05213.x
Boyette-Davis, J. A., Walters, E. T., and Dougherty, P. M. (2015). Mechanisms involved in the development of chemotherapy-induced neuropathy. Pain Manag. 5, 285–296. doi: 10.2217/pmt.15.19
Brandolini, L., Benedetti, E., Ruffini, P. A., Russo, R., Cristiano, L., Antonosante, A., et al. (2017). CXCR1/2 pathways in paclitaxel-induced neuropathic pain. Oncotarget 8, 23188–23201. doi: 10.18632/oncotarget.15533
Cao, H., Zhang, A., Zhang, H., Sun, H., and Wang, X. (2015). The application of metabolomics in traditional Chinese medicine opens up a dialogue between Chinese and Western medicine. Phytother. Res. 29, 159–166. doi: 10.1002/ptr.5240
Carozzi, V. A., Canta, A., and Chiorazzi, A. (2015). Chemotherapy-induced peripheral neuropathy: what do we know about mechanisms? Neurosci. Lett. 596, 90–107. doi: 10.1016/j.neulet.2014.10.014
Cata, J. P., Weng, H. R., Chen, J. H., and Dougherty, P. M. (2006). Altered discharges of spinal wide dynamic range neurons and down-regulation of glutamate transporter expression in rats with paclitaxel-induced hyperalgesia. Neuroscience 138, 329–338. doi: 10.1016/j.neuroscience.2005.11.009
Cavaletti, G., Cavalletti, E., Oggioni, N., Sottani, C., Minoia, C., D'Incalci, M., et al. (2000). Distribution of paclitaxel within the nervous system of the rat after repeated intravenous administration. Neurotoxicology 21, 389–393.
Chaplan, S. R., Bach, F. W., Pogrel, J. W., Chung, J. M., and Yaksh, T. L. (1994). Quantitative assessment of tactile allodynia in the rat paw. J. Neurosci. Methods 53, 55–63. doi: 10.1016/0165-0270(94)90144-9
Chen, Y., Yang, C., and Wang, Z. J. (2011). Proteinase-activated receptor 2 sensitizes transient receptor potential vanilloid 1, transient receptor potential vanilloid 4, and transient receptor potential ankyrin 1 in paclitaxel-induced neuropathic pain. Neuroscience 193, 440–451. doi: 10.1016/j.neuroscience.2011.06.085
Dai, D., Zeldin, D. C., Blaisdell, J. A., Chanas, B., Coulter, S. J., Ghanayem, B. I., et al. (2001). Polymorphisms in human CYP2C8 decrease metabolism of the anticancer drug paclitaxel and arachidonic acid. Pharmacogenetics 11, 597–607. doi: 10.1097/00008571-200110000-00006
Deng, B., Jia, L., Pan, L., Song, A., Wang, Y., Tan, H., et al. (2016). Wen-Luo-Tong prevents glial activation and nociceptive sensitization in a rat model of oxaliplatin-induced neuropathic pain. Evid. Based Complement Alternat. Med. 2016:3629489. doi: 10.1155/2016/3629489
Deng, C., Deng, B., Jia, L., Tan, H., Zhang, P., Liu, S., et al. (2017). Preventive effects of a chinese herbal formula, shengjiang xiexin decoction, on irinotecan-induced delayed-onset diarrhea in rats. Evid. Based Complement Alternat. Med. 2017:7350251. doi: 10.1155/2017/7350251
Dixon, W. J. (1980). Efficient analysis of experimental observations. Annu. Rev. Pharmacol. Toxicol 20, 441–462. doi: 10.1146/annurev.pa.20.040180.002301
Dutra, R. C., Bicca, M. A., Segat, G. C., Silva, K. A., Motta, E. M., Pianowski, L. F., et al. (2015). The antinociceptive effects of the tetracyclic triterpene euphol in inflammatory and neuropathic pain models: the potential role of PKCepsilon. Neuroscience 303, 126–137. doi: 10.1016/j.neuroscience.2015.06.051
Fang, Z. Z., and Gonzalez, F. J. (2014). LC-MS-based metabolomics: an update. Arch. Toxicol. 88, 1491–1502. doi: 10.1007/s00204-014-1234-6
Flatters, S. J., and Bennett, G. J. (2006). Studies of peripheral sensory nerves in paclitaxel-induced painful peripheral neuropathy: evidence for mitochondrial dysfunction. Pain 122, 245–257. doi: 10.1016/j.pain.2006.01.037
Gan, R. Y., Kuang, L., Xu, X. R., Zhang, Y., Xia, E. Q., Song, F. L., et al. (2010). Screening of natural antioxidants from traditional Chinese medicinal plants associated with treatment of rheumatic disease. Molecules 15, 5988–5997. doi: 10.3390/molecules15095988
Glinski, M., and Weckwerth, W. (2006). The role of mass spectrometry in plant systems biology. Mass Spectrom. Rev. 25, 173–214. doi: 10.1002/mas.20063
Glynn, P. (2013). Neuronal phospholipid deacylation is essential for axonal and synaptic integrity. Biochim. Biophys. Acta 1831, 633–641. doi: 10.1016/j.bbalip.2012.07.023
Gui, Y., Zhang, J., Chen, L., Duan, S., Tang, J., Xu, W., et al. (2018). Icariin, a flavonoid with anti-cancer effects, alleviated paclitaxel-induced neuropathic pain in a SIRT1-dependent manner. Mol. Pain 14:1744806918768970. doi: 10.1177/1744806918768970
Gunawardena, D., Karunaweera, N., Lee, S., van Der Kooy, F., Harman, D. G., Raju, R., et al. (2015). Anti-inflammatory activity of cinnamon (C. zeylanicum and C. cassia) extracts - identification of E-cinnamaldehyde and o-methoxy cinnamaldehyde as the most potent bioactive compounds. Food Funct. 6, 910–919. doi: 10.1039/C4FO00680A
Haefner, B. (2006). Targeting NF-kappaB in anticancer adjunctive chemotherapy. Cancer Treat Res. 130, 219–245. doi: 10.1007/0-387-26283-0_10
Hara, T., Chiba, T., Abe, K., Makabe, A., Ikeno, S., Kawakami, K., et al. (2013). Effect of paclitaxel on transient receptor potential vanilloid 1 in rat dorsal root ganglion. Pain 154, 882–889. doi: 10.1016/j.pain.2013.02.023
Hershman, D. L., Lacchetti, C., Dworkin, R. H., Lavoie Smith, E. M., Bleeker, J., Cavaletti, G., et al. (2014). Prevention and management of chemotherapy-induced peripheral neuropathy in survivors of adult cancers: American Society of Clinical Oncology Clinical Practice Guideline. J. Clin. Oncol. 32, 1941–1967. doi: 10.1200/JCO.2013.54.0914
Hershman, D. L., Weimer, L. H., Wang, A., Kranwinkel, G., Brafman, L., Fuentes, D., et al. (2011). Association between patient reported outcomes and quantitative sensory tests for measuring long-term neurotoxicity in breast cancer survivors treated with adjuvant paclitaxel chemotherapy. Breast Cancer Res. Treat 125, 767–774. doi: 10.1007/s10549-010-1278-0
Huang, M., Yao, P. W., Chang, M. D., Ng, S. K., Yu, C. H., Zhang, Y. F., et al. (2015). Identification of anti-inflammatory fractions of Geranium wilfordii using tumor necrosis factor-alpha as a drug target on Herbochip(R) - an array-based high throughput screening platform. BMC Complement Altern. Med. 15, 146. doi: 10.1186/s12906-015-0665-9
Huang, Z. Z., Li, D., Liu, C. C., Cui, Y., Zhu, H. Q., Zhang, W. W., et al. (2014). CX3CL1-mediated macrophage activation contributed to paclitaxel-induced DRG neuronal apoptosis and painful peripheral neuropathy. Brain Behav. Immun. 40, 155–165. doi: 10.1016/j.bbi.2014.03.014
Jang, Y., Jung, J., Kim, H., Oh, J., Jeon, J. H., Jung, S., et al. (2012). Axonal neuropathy-associated TRPV4 regulates neurotrophic factor-derived axonal growth. J. Biol. Chem. 287, 6014–6024. doi: 10.1074/jbc.M111.316315
Jo, A. R., Han, H. S., Seo, S., Shin, J. S., Lee, J. Y., Kim, H. J., et al. (2017). Inhibitory effect of moschamine isolated from Carthamus tinctorius on LPS-induced inflammatory mediators via AP-1 and STAT1/3 inactivation in RAW 264.7 macrophages. Bioorg. Med. Chem. Lett. 27, 5245–5251. doi: 10.1016/j.bmcl.2017.10.035
Kou, Y., Wang, Z., Wu, Z., Zhang, P., Zhang, Y., Yin, X., et al. (2013). Epimedium extract promotes peripheral nerve regeneration in rats. Evid. Based Complement Alternat. Med. 2013:954798. doi: 10.1155/2013/954798
Kuruvilla, R., and Eichberg, J. (1998). Depletion of phospholipid arachidonoyl-containing molecular species in a human Schwann cell line grown in elevated glucose and their restoration by an aldose reductase inhibitor. J. Neurochem. 71, 775–783. doi: 10.1046/j.1471-4159.1998.71020775.x
Ledeboer, A., Jekich, B. M., Sloane, E. M., Mahoney, J. H., Langer, S. J., Milligan, E. D., et al. (2007). Intrathecal interleukin-10 gene therapy attenuates paclitaxel-induced mechanical allodynia and proinflammatory cytokine expression in dorsal root ganglia in rats. Brain Behav. Immun. 21, 686–698. doi: 10.1016/j.bbi.2006.10.012
Lee, J. J., and Swain, S. M. (2006). Peripheral neuropathy induced by microtubule-stabilizing agents. J. Clin. Oncol. 24, 1633–1642. doi: 10.1200/JCO.2005.04.0543
Li, D., Huang, Z. Z., Ling, Y. Z., Wei, J. Y., Cui, Y., Zhang, X. Z., et al. (2015). Up-regulation of CX3CL1 via Nuclear Factor-kappaB-dependent Histone Acetylation Is Involved in Paclitaxel-induced Peripheral Neuropathy. Anesthesiology 122, 1142–1151. doi: 10.1097/ALN.0000000000000560
Li, S., and Zhang, B. (2013). Traditional Chinese medicine network pharmacology: theory, methodology and application. Chin. J. Nat. Med. 11, 110–120. doi: 10.1016/S1875-5364(13)60037-0
Li, S., Zhang, B., and Zhang, N. (2011). Network target for screening synergistic drug combinations with application to traditional Chinese medicine. BMC Syst. Biol. 5 (Suppl. 1):S10. doi: 10.1186/1752-0509-5-S.1-S.10
Li, Y., Adamek, P., Zhang, H., Tatsui, C. E., Rhines, L. D., Mrozkova, P., et al. (2015). The cancer chemotherapeutic paclitaxel increases human and rodent sensory neuron responses to TRPV1 by activation of TLR4. J. Neurosci. 35, 13487–13500. doi: 10.1523/JNEUROSCI.1956-15.2015
Lin, H. M., Lin, L. F., Xia, Z. Z., Mao, Y., Liu, J., Xu, L. Y., et al. (2017). Neuroprotective effects and UPLC-Q-TOF/MS-based active components identification of external applied a novel Wen-Luo-Tong microemulsion. Artif. Cells Nanomed. Biotechnol. doi: 10.1080/21691401.2017.1397002. [Epub ahead of print].
Lou, Y. N., Jia, L. Q., Deng, H. Y., Li, L. Y., Li, X., and Wan, D. J. (2008). Clinical study on the effects of tong luo san tropical application on oxaliplatin iduced peripheral neuropathy. Beijing J. Trad. Chin. Med. 27, 258–260. doi: 10.16025/j.1674-1307.2008.04.028
Lou, Y. N., Tian, A. P., Zhang, X., Feng, L., Tan, H. Y., Zhu, S. J., et al. (2014). Randomized, multi-center, double-blinded, controlled clinical trial of external treatment of TCM on peripheral neuropathy caused by chemotherapy. Chin. Tradit. Chin. Med. Pharm. 29, 2682–2685.
Lu, C., Deng, J., Li, L., Wang, D., and Li, G. (2014). Application of metabolomics on diagnosis and treatment of patients with psoriasis in traditional Chinese medicine. Biochim. Biophys. Acta 1844, 280–288. doi: 10.1016/j.bbapap.2013.05.019
Martin, L. J., Gertz, B., Pan, Y., Price, A. C., Molkentin, J. D., and Chang, Q. (2009). The mitochondrial permeability transition pore in motor neurons: involvement in the pathobiology of ALS mice. Exp. Neurol. 218, 333–346. doi: 10.1016/j.expneurol.2009.02.015
Materazzi, S., Fusi, C., Benemei, S., Pedretti, P., Patacchini, R., Nilius, B., et al. (2012). TRPA1 and TRPV4 mediate paclitaxel-induced peripheral neuropathy in mice via a glutathione-sensitive mechanism. Pflug. Arch. 463, 561–569. doi: 10.1007/s00424-011-1071-x
Nicholson, J. K., and Lindon, J. C. (2008). Systems biology: metabonomics. Nature 455, 1054–1056. doi: 10.1038/4551054a
Nieto, F. R., Entrena, J. M., Cendán, C. M., Pozo, E. D., Vela, J. M., and Baeyens, J. M. (2008). Tetrodotoxin inhibits the development and expression of neuropathic pain induced by paclitaxel in mice. Pain 137, 520–531. doi: 10.1016/j.pain.2007.10.012
Oh, Y. C., Jeong, Y. H., Cho, W. K., Ha, J. H., Lee, S. J., and Ma, J. Y. (2015). Inhibitory effects of epimedium herb on the inflammatory response in vitro and in vivo. Am. J. Chin. Med. 43, 953–968. doi: 10.1142/S0192415X1550055X
Park, S. J., Wu, C. H., Gordon, J. D., Zhong, X., Emami, A., and Safa, A. R. (2004). Taxol induces caspase-10-dependent apoptosis. J. Biol. Chem. 279, 51057–51067. doi: 10.1074/jbc.M406543200
Patwardhan, A. M., Akopian, A. N., Ruparel, N. B., Diogenes, A., Weintraub, S. T., Uhlson, C., et al. (2010). Heat generates oxidized linoleic acid metabolites that activate TRPV1 and produce pain in rodents. J. Clin. Invest. 120, 1617–1626. doi: 10.1172/JCI41678
Pei, J. P., Fan, L. H., Nan, K., Li, J., Dang, X. Q., and Wang, K. Z. (2017). HSYA alleviates secondary neuronal death through attenuating oxidative stress, inflammatory response, and neural apoptosis in SD rat spinal cord compression injury. J. Neuroinflammation 14:97. doi: 10.1186/s12974-017-0870-1
Reyes-Gibby, C. C., Morrow, P. K., Buzdar, A., and Shete, S. (2009). Chemotherapy-induced peripheral neuropathy as a predictor of neuropathic pain in breast cancer patients previously treated with paclitaxel. J. Pain 10, 1146–1150. doi: 10.1016/j.jpain.2009.04.006
Ruiz, M., Jové, M., Schlüter, A., Casasnovas, C., Villarroya, F., Guilera, C., et al. (2015). Altered glycolipid and glycerophospholipid signaling drive inflammatory cascades in adrenomyeloneuropathy. Hum. Mol. Genet. 24, 6861–6876. doi: 10.1093/hmg/ddv375
Shindel, A. W., Xin, Z. C., Lin, G., Fandel, T. M., Huang, Y. C., Banie, L., et al. (2010). Erectogenic and neurotrophic effects of icariin, a purified extract of horny goat weed (Epimedium spp.) in vitro and in vivo. J. Sex. Med. 7, 1518–1528. doi: 10.1111/j.1743-6109.2009.01699.x
Sisignano, M., Angioni, C., Park, C. K., Meyer, D. S. S., Jordan, H., Kuzikov, M., et al. (2016). Targeting CYP2J to reduce paclitaxel-induced peripheral neuropathic pain. Proc. Natl. Acad. Sci. U.S.A. 113, 12544–12549. doi: 10.1073/pnas.1613246113
Sun, L., Zong, S. B., Li, J. C., Lv, Y. Z., Liu, L. N., Wang, Z. Z., et al. (2016). The essential oil from the twigs of Cinnamomum cassia Presl alleviates pain and inflammation in mice. J. Ethnopharmacol. 194, 904–912. doi: 10.1016/j.jep.2016.10.064
Wang, H. Y., Tsai, Y. J., Chen, S. H., Lin, C. T., and Lue, J. H. (2013). Lysophosphatidylcholine causes neuropathic pain via the increase of neuronal nitric oxide synthase in the dorsal root ganglion and cuneate nucleus. Pharmacol. Biochem. Behav. 106, 47–56. doi: 10.1016/j.pbb.2013.03.002
Wang, P., Wang, Q., Yang, B., Zhao, S., and Kuang, H. (2015). The progress of metabolomics study in traditional chinese medicine research. Am. J. Chin. Med. 43, 1281–1310. doi: 10.1142/S0192415X15500731
Wang, X., Cui, D. N., Dai, X. M., Wang, J., Zhang, W., Zhang, Z. J., et al. (2017). HuangQin decoction attenuates cpt-11-induced gastrointestinal toxicity by regulating bile acids metabolism homeostasis. Front. Pharmacol. 8:156. doi: 10.3389/fphar.2017.00156
Wang, Y., Chen, P., Tang, C., Wang, Y., Li, Y., and Zhang, H. (2014). Antinociceptive and anti-inflammatory activities of extract and two isolated flavonoids of Carthamus tinctorius L. J. Ethnopharmacol. 151, 944–950. doi: 10.1016/j.jep.2013.12.003
Weckwerth, W. (2003). Metabolomics in systems biology. Annu. Rev. Plant Biol. 54, 669–689. doi: 10.1146/annurev.arplant.54.031902.135014
Weckwerth, W. (2010). Metabolomics: an integral technique in systems biology. Bioanalysis 2, 829–836. doi: 10.4155/bio.09.192
Wolf, S., Barton, D., Kottschade, L., Grothey, A., and Loprinzi, C. (2008). Chemotherapy-induced peripheral neuropathy: prevention and treatment strategies. Eur. J. Cancer 44, 1507–1515. doi: 10.1016/j.ejca.2008.04.018
Xia, J., Sinelnikov, I. V., Han, B., and Wishart, D. S. (2015). MetaboAnalyst 3.0–making metabolomics more meaningful. Nucleic Acids Res. 43, W251–W257. doi: 10.1093/nar/gkv380
Xia, J., and Wishart, D. S. (2016). Using metaboanalyst 3.0 for comprehensive metabolomics data analysis. Curr. Protoc. Bioinformatics 55, 14.10.1–14.10.91. doi: 10.1002/cpbi.11
Xiao, W., Boroujerdi, A., Bennett, G. J., and Luo, Z. D. (2007). Chemotherapy-evoked painful peripheral neuropathy: analgesic effects of gabapentin and effects on expression of the alpha-2-delta type-1 calcium channel subunit. Neuroscience 144, 714–720. doi: 10.1016/j.neuroscience.2006.09.044
Yue, S. J., Tang, Y. P., Wang, L. Y., Tang, H., Li, S. J., Liu, P., et al. (2014). Separation and evaluation of antioxidant constituents from Carthamus tinctorius. Zhongguo Zhong Yao Za Zhi 39, 3295–3300. doi: 10.4268/cjcmm20141717
Zhang, H., Nei, H., and Dougherty, P. M. (2010). A p38 mitogen-activated protein kinase-dependent mechanism of disinhibition in spinal synaptic transmission induced by tumor necrosis factor-alpha. J. Neurosci. 30, 12844–12855. doi: 10.1523/JNEUROSCI.2437-10.2010
Keywords: paclitaxel, peripheral neuropathy, metabolomics, Wen-Luo-Tong (WLT), TCM, UHPLC-MS/MS
Citation: Wu F, Xu W, Deng B, Liu S, Deng C, Wu M, Gao Y and Jia L (2018) Wen-Luo-Tong Decoction Attenuates Paclitaxel-Induced Peripheral Neuropathy by Regulating Linoleic Acid and Glycerophospholipid Metabolism Pathways. Front. Pharmacol. 9:956. doi: 10.3389/fphar.2018.00956
Received: 13 March 2018; Accepted: 03 August 2018;
Published: 28 August 2018.
Edited by:
Zhiling Yu, Hong Kong Baptist University, Hong KongReviewed by:
Hongjie Zhang, Hong Kong Baptist University, Hong KongJianxin Chen, Beijing University of Chinese Medicine, China
Rongbiao Pi, Sun Yat-sen University, China
Copyright © 2018 Wu, Xu, Deng, Liu, Deng, Wu, Gao and Jia. This is an open-access article distributed under the terms of the Creative Commons Attribution License (CC BY). The use, distribution or reproduction in other forums is permitted, provided the original author(s) and the copyright owner(s) are credited and that the original publication in this journal is cited, in accordance with accepted academic practice. No use, distribution or reproduction is permitted which does not comply with these terms.
*Correspondence: Li-qun Jia, bGlxdW4tamlhQGhvdG1haWwuY29t