- 1School of BioSciences, The University of Melbourne, Melbourne, VIC, Australia
- 2Heart Failure Pharmacology, Baker Heart and Diabetes Institute, Melbourne, VIC, Australia
- 3Department of Human and Molecular Genetics, Herbert Wertheim College of Medicine, Florida International University, Miami, FL, United States
- 4Science and Math Cluster, Singapore University of Technology and Design, Singapore, Singapore
- 5Department of Pharmacology & Therapeutics, The University of Melbourne, Melbourne, VIC, Australia
Cardiovascular complications are the major cause of mortality in patients with diabetes. This is closely associated with both macrovascular and microvascular complications of diabetes, which lead to organ injuries in diabetic patients. Previous studies have consistently demonstrated the beneficial effects of relaxin treatment for protection of the vasculature, with evidence of antioxidant and anti-remodeling actions. Relaxin enhances nitric oxide, prostacyclin and endothelium-derived hyperpolarization (EDH)-type-mediated relaxation in various vascular beds. These effects of relaxin on the systemic vasculature, coupled with its cardiac actions, reduce pulmonary capillary wedge pressure and pulmonary artery pressure. This results in an overall decrease in systemic and pulmonary vascular resistance in heart failure patients. The anti-fibrotic actions of relaxin are well established, a desirable property in the context of diabetes. Further, relaxin ameliorates diabetic wound healing, with accelerated angiogenesis and vasculogenesis. Relaxin-mediated stimulation of vascular endothelial growth factor (VEGF) and stromal cell-derived factor 1-α, as well as regulation of metalloproteinase expression, ameliorates cardiovascular fibrosis in diabetic mice. In the heart, relaxin is a cardioprotective molecule in several experimental animal models, exerting anti-fibrotic, anti-hypertrophy and anti-apoptotic effects in diabetic pathologies. Collectively, these studies provide a foundation to propose the therapeutic potential for relaxin as an adjunctive agent in the prevention or treatment of diabetes-induced cardiovascular complications. This review provides a comprehensive overview of the beneficial effects of relaxin, and identifies its therapeutic possibilities for alleviating diabetes-related cardiovascular injury.
Introduction
Diabetes mellitus is a major risk factor for the development of cardiovascular diseases. This disease affects an estimated 6.4% of adults worldwide, but this number is likely to increase to 7.7% by 2030 (Shaw et al., 2010). Diabetes leads to microvascular complications such as kidney failure, lower limb amputation, and blindness (Forbes and Fotheringham, 2017). Macrovascular complications of diabetes include peripheral vascular disease, myocardial infarction, stroke, and congestive heart failure. In the context of diabetic cardiomyopathy, left ventricular diastolic dysfunction at an early stage which subsequently progress to systolic dysfunction is closely associated with cardiac hypertrophy and fibrosis (Boudina and Abel, 2007). We and others have reviewed the key mechanisms and strategies to target diabetic cardiomyopathy (Boudina and Abel, 2010; Huynh et al., 2014; Ritchie et al., 2017; Tate et al., 2017b). It is however worth noting that there are still no effective therapies to limit the progression of diabetes-induced cardiovascular complications. Here, we propose a small peptide hormone, relaxin, which shows a promising role in protecting the cardiovascular system (Leo et al., 2016a; Sarwar et al., 2017). The possibilities of relaxin as a therapy for diabetes-related complications are also discussed.
Cardiovascular Complications of Diabetes
Diabetic cardiomyopathy is characterized by damage to the myocardium, in particular diastolic dysfunction (Pappachan et al., 2013), where the heart is unable to relax and undergo filling during the diastolic part of the cardiac cycle. Pathological processes underlying this include cardiac hypertrophy, neuronal abnormalities and myocardium stiffening as a result of collagen cross-linking and extracellular matrix deposition (Iimoto et al., 1988). Activation of the sympathetic nervous system as a result of insulin resistance leads to impaired kidney and vascular function, an effect that is associated with the onset of hypertension (Reaven et al., 1996). For example, an FDA-approved glucose-lowering drug for the treatment of type 2 diabetes, the sodium-glucose co-transporter 2 (SGLT2) antagonist empagliflozin, exhibits promising actions in reducing cardiovascular burden by limiting the sympathetic outflow to the kidneys (Sano, 2018). Diabetes-induced collagen cross-linking that accumulates within the myocardium can ultimately lead to structural remodeling of the heart tissue, including cardiac fibrosis. Mechanisms that contribute to the phenotype of the diabetic myocardium include (but are not limited to) impairments in function of type 2 ryanodine receptors (RyR2) (Santulli et al., 2015), increased oxidative stress, activation of the renin-angiotensin-aldosterone system (RAAS), endothelin-1 upregulation, with mitochondrial dysfunction, inflammation and endoplasmic reticulum stress (Huynh et al., 2014; Prakoso et al., 2017; Tate et al., 2017a,b). Reactive oxygen species (ROS) directly damage proteins, DNA and lipids, produce reactive lipid peroxidases, and can generate reactive nitrogen species, all which contribute to diabetic cardiomyopathy (Baynes and Thorpe, 1999). In diabetes, the over-activation of the RAAS increases angiotensin II (Ang II) production in cardiac fibroblasts and cardiomyocytes, to impair cardiac structure and function (Lavrentyev et al., 2007; Singh et al., 2008). Similarly, these pathological pathways also play a major role in diabetes-associated vasculopathy. The most well-known functional characteristic of diabetes-induced vascular complications is endothelial dysfunction, where there is an imbalance in vascular homeostasis, causing a shift toward vasoconstrictor state and reduced vasodilation. Hyperglycemia leads to the imbalance of vasoprotective molecules such as nitric oxide (NO), prostacyclin (PGI2), and endothelium-derived hyperpolarization (EDH) pathways, and causes overproduction of ROS or vasoconstrictor, thus leading to endothelial dysfunction (Johnstone et al., 1993; Guzik et al., 2002; Angulo et al., 2003; Vanhoutte et al., 2017). Both the cardiac (Bugger and Abel, 2014; Huynh et al., 2014; Tate et al., 2017b) and vascular complications (Forbes and Fotheringham, 2017; Shi and Vanhoutte, 2017) of diabetes have been extensively reviewed in detail elsewhere. Insulin resistance can be initiated by altered glucose metabolism and disturbance in fatty acid metabolism (McGarry, 1992), whereby there is an imbalance between fatty acid uptake and beta-oxidation in the skeletal muscle or heart, leading to impaired insulin signaling. Inhibition of both glucose metabolism and fatty acid uptake (Randle et al., 1963), and muscle glucose uptake via PKCζ activation (Samuel and Shulman, 2016) all contribute to the pathophysiology of diabetes.
Is Glucose-Lowering Sufficient?
Insulin therapy is the first line treatment for type 1 diabetic patients. One school of thought favors a role for insulin therapy in heart failure outcomes, particularly in the elderly (Sardu et al., 2014). However, this is countered by evidence from several studies in which diabetic patients on insulin therapy exhibit increased risk of developing heart failure (Nichols et al., 2005; Smooke et al., 2005; Mangiavacchi et al., 2008). Over the years, many approaches to safely treat type 2 diabetes have been developed. First-line glucose-lowering therapy for type 2 diabetes remains to be metformin (Holman, 2007). Recently, several new classes of drugs have been developed for treating type 2 diabetes, which include dipeptidyl peptidase-4 (DPP4) inhibitors, glucagon-like peptide-1 (GLP-1) receptor agonists and SGLT2 antagonists. These therapies can be added to first-line therapy if adequate glycemic control is not yet achieved, aiming to reduce and control blood glucose levels for as long as possible after diagnosis, and thereby potentially preventing the development and progression of cardiovascular complications. However, the majority of these conventional glucose-lowering drugs fail to prevent the progression of cardiovascular complications, despite their favorable effects on glycemic control. There has recently emerged the suggestion that GLP-1 receptor agonists may reduce cardiovascular complications, although this may be secondary to the reduction in glycated hemoglobin levels (Bethel et al., 2017). Excitingly however, the SGLT2 inhibitors empagliflozin and canagliflozin reduce heart failure hospitalization and may be the first glucose-lowering therapy to relieve some of the cardiovascular burden of diabetes as demonstrated in the EMPA-REG OUTCOME and CANVAS trials (Lytvyn et al., 2017). Hence, alternative therapies are needed to address the ever-increasing incidence of diabetes to prevent and/or treat diabetes-induced cardiovascular complications.
Protective Role for Relaxin in the Vasculature
An emerging molecule implicated in the protection of the cardiovascular system is the hormone relaxin. In rodents, the relaxin/insulin-like family peptide receptor 1 (RXFP1) is expressed in the aorta (Ng et al., 2015), small renal and mesenteric arteries (Novak et al., 2006). RXFP1 is predominantly localized in the endothelial cells of rat mesenteric artery and vein, vena cava and the aorta, but the distribution of this receptor is more abundant in the vascular smooth muscle cells of rat small pulmonary artery, femoral artery and vein (Jelinic et al., 2014). The differential distribution of RXFP1 in several vascular beds could serve as an important target for relaxin activity independent of any potential source of local relaxin production. The other members of the relaxin family peptide receptors comprise RXFP2, RXFP3, and RXFP4. Relaxin can weakly bind to and activate RXFP2 at higher concentrations, however, in human and rodents, relaxin has higher binding affinity to RXFP1. RXFP3, and RXFP4 on the other hand are structurally and functionally different to RXFP1 and RXFP2, with their corresponding ligands relaxin-3 and INSL5 (Bathgate et al., 2006). Traditionally, relaxin is known as a reproductive hormone that plays an important role in maternal adaptations to pregnancy, by elongating the pubic ligament at the end of pregnancy in rodents, as well as causing cervical ripening and nipple development (Sherwood, 2004). However, many actions of relaxin have also been extensively reported in several non-reproductive organs (Leo et al., 2016a; Samuel et al., 2016; Sarwar et al., 2017). Much of this has relied on the potent anti-fibrotic actions of relaxin (Samuel et al., 2016). Exogenous relaxin administration also has significant therapeutic benefits in animal models of hypertension, atherosclerosis and tobacco-related disease, often beyond its anti-fibrotic mechanisms, as detailed below.
In the vasculature, relaxin reduces myogenic reactivity and sensitivity to the α1-adrenergic vasoconstrictor, phenylephrine in Wistar Kyoto rats (WKY) and spontaneously hypertensive rats (SHR), accompanied by improved flow-mediated vasodilation (van Drongelen et al., 2013). This action is NO-dependent in normotensive, but not hypertensive rats (van Drongelen et al., 2013). In Ang II-induced hypertensive rats, relaxin reduces mean arterial pressure, albumin excretion and impairments in NO availability, effects attributed to reduced oxidative stress (Sasser et al., 2011). Furthermore, relaxin attenuates aortic remodeling to reduce wall thickness and collagen content (Xu et al., 2010). Inward remodeling of parenchymal arterioles is also ameliorated in hypertensive rats (Chan et al., 2013), thus increasing arterial compliance. These studies in hypertensive models clearly show the vasculoprotective effects of relaxin against hypertension-induced dysfunction and its potential role in reversing hypertension-associated fibrosis. More recently, relaxin treatment is reported to reverse cigarette smoke-induced endothelial dysfunction, an effect attributed to enhanced endothelial NO synthase (eNOS) expression (Pini et al., 2016). Relaxin infusion over 4 weeks also reverses the atherosclerosis-associated endothelial dysfunction in ApoE-/- mice, an effect attributed to reduction in angiotensin AT1 receptors and oxidative stress (Tiyerili et al., 2016). In addition, relaxin-mediated vasculoprotective effects are also evident in vascular preparations ex vivo, primarily through its action on endothelin B receptors (ETB), NO and PGI2 (Dschietzig et al., 2003; McGuane et al., 2011a; Dschietzig et al., 2012; Ng et al., 2016). In isolated small human gluteal (Fisher et al., 2002) and subcutaneous (McGuane et al., 2011b) arteries, relaxin induces rapid dilator actions. However, the rapid vasodilator effects of relaxin are not observed in all blood vessels such as the rat mesenteric arteries, despite the presence of RXFP1 (Leo et al., 2014b, 2016a, 2017). All of these studies provide an important insight into the protective mechanisms of relaxin against vascular dysfunction, where altered vasodilator and vasoconstrictor pathways are implicated, as well as modifying the vessel wall structure.
Cardiac Role for Relaxin
In addition to the above vasoprotective roles of relaxin, a plethora of literature has identified relaxin as a cardioprotective molecule, particularly through its anti-fibrotic and anti-hypertrophic actions. For example, relaxin ameliorates post-infarction fibrotic healing by reducing the density of mature scar tissue in the infarcted area (Samuel et al., 2011), and regulates cardiac fibroblast proliferation, differentiation and collagen deposition, thereby reversing cardiac fibrosis in vivo (Samuel et al., 2004). In SHR, relaxin administration for 2 weeks is sufficient to lower collagen content in the left ventricle, ameliorate left ventricular hypertrophy and dysfunction, as well as reverse cardiac fibrosis (Lekgabe et al., 2005). Furthermore, relaxin also prevents atrial fibrillation secondary to the amelioration of cardiac fibrosis and hypertrophy in SHR. This action is associated with changes in Na+ current density (Parikh et al., 2013; Henry et al., 2016). One of the well-reported mechanisms of relaxin as an anti-fibrotic molecule is its ability to up-regulate the Notch signaling pathway, whereby the transforming growth factor (TGF)-β/Smad 3 signaling is inhibited to prevent cardiac fibroblast-myofibroblast transition (Sassoli et al., 2013). In addition to the potent anti-fibrotic actions of relaxin in various disease animal models, relaxin has the ability to reduce oxidative stress, at least in part via protein kinase B (Akt) and extracellular signal-regulated kinase (ERK) signaling pathways, suppressing cardiomyocytes apoptosis and hypertrophy (Moore et al., 2007). Collectively, the actions of relaxin in pressure-overloaded animals may represent an attractive target of unloading the heart, via vasodilation and reducing systemic vascular resistance.
Role for Relaxin in the Context of Diabetes-Induced Vascular Complications
Further to the widely reported actions of relaxin in hypertensive animals, several lines of evidence suggest that relaxin may possess similar effects in diabetic animals. Relaxin ameliorates diabetic wound healing by accelerating angiogenesis and vasculogenesis through the stimulation of vascular endothelial growth factor (VEGF) and stromal cell-derived factor (SDF)1-α, as well as regulating metalloproteinase (MMP) expression to impede fibrosis in db/db mice (Bitto et al., 2013; Squadrito et al., 2013). These wound healing properties of relaxin are primarily attributed to induction of VEGF expression and subsequent angiogenesis that selectively target the wound site (Unemori et al., 2000). Relaxin also upregulates NO production in the vasculature, which may play an additional role in wound healing. Notably, relaxin treatment attenuates fibrosis in the heart of streptozotocin (STZ) Ren-2 diabetic rats by decreasing interstitial and total left ventricle collagen deposition, thereby reducing myocardial stiffness and improving overall left ventricular diastolic function (Samuel et al., 2008). Indeed, relaxin treatment inhibits both proliferation of cardiac fibroblasts and formation of type I and III fibrillar collagen induced by high glucose in vitro (Wang et al., 2009). Intriguingly, relaxin also been reported to attenuate skeletal muscle insulin resistance, accompanied by enhanced aortic endothelium-dependent relaxation in high fat-fed pre-diabetic mice (Bonner et al., 2013). The underlying mechanisms of relaxin actions in this context however are not further interrogated.
Our own studies have recently demonstrated that relaxin protects the mouse aorta and mesenteric arteries by restoring endothelial function under acute (high glucose) (Ng et al., 2016) and chronic hyperglycemia (Ng et al., 2017). Relaxin prevents high glucose-induced endothelial dysfunction in mouse aorta by suppressing oxidative stress and stimulating vasodilator PGI2 production, as well as changing the relative gene expression of the PGI2 receptor (IP) to thromboxane (TP) (Ng et al., 2016). Under high glucose conditions, PGI2, which normally acts as a vasodilator, reverts to a vasoconstrictor (Zhu et al., 2014), likely acting through the TP receptor. This ex vivo study on relaxin co-treatment under hyperglycemic conditions provided ‘proof-of-concept’ evidence that motivated us to further investigate the in vivo effects of relaxin utilizing chronic type 1 diabetic mice. It is well-established that chronic hyperglycemia induces endothelial dysfunction in an experimental model of diabetes (Pieper et al., 1995; Leo et al., 2011a), with downregulation of endothelium-derived NO. Consistent with the ex vivo data, relaxin treatment for 2 weeks following 10 weeks of untreated diabetes is sufficient to restore endothelial function in diabetic mouse aorta (Ng et al., 2017). This is underpinned by enhanced NO-mediated relaxation. Interestingly, relaxin treatment in this context failed to increase basal NO availability in both ex vivo and in vivo settings of hyperglycemia. This suggests that exogenous relaxin treatment selectively augments agonist stimulated NO-mediated relaxation pathways to preserve endothelial vasodilator function, without impacting on basal NO synthase activity in endothelial cells. In contrast to the findings in diabetic aortae, relaxin increases basal NOS activity and eNOS phosphorylation in healthy rat aortae following two days of continuous intravenous infusion (Leo et al., 2016b), leading to enhanced NO-mediated relaxation. The disparity of findings may be attributed to the difference between non-diabetic and diabetic settings, or deficits in the NOS co-factor, tetrahydrobiopterin (BH4), in diabetic aortae, as a result of oxidation by peroxynitrite produced from the interaction between superoxide and NO in the endothelium (Cai et al., 2005).
Diabetes also attenuates endothelial function in smaller resistance arteries (mesenteric arteries), by increasing the contribution of vasoconstrictor prostanoids to endothelial dysfunction and reducing EDH-type relaxation (Ng et al., 2017). It is worth noting that the role of EDH increases as the artery diameter decreases, thus the relaxation seen in mesenteric artery will be largely dependent on EDH, in contrast to larger blood vessel such as the aorta. Relaxin treatment for 2 weeks in diabetic mice reverses endothelial dysfunction (Ng et al., 2017). This is accompanied by a profound increase in the relative contribution of NO to endothelium-dependent relaxation. Although diabetes selectively impairs EDH-type relaxation in the mesenteric artery, relaxin treatment failed to restore EDH-type relaxation at least in the STZ C57BL/6 mouse model of diabetes. The mechanisms underpinning the impairment of EDH-type relaxation in diabetic mesenteric arteries are multi-factorial (Ding et al., 2005; Ding and Triggle, 2010; Leo et al., 2011b). One possible explanation is that relaxin is not able to restore the reduction in low-resistance electrical coupling between endothelial and vascular smooth muscle cells in the mouse small mesenteric arteries in the context of diabetes (Ding et al., 2005). Indeed, the mechanism of relaxin action is quite distinct to that of NO in reversing diabetes-induced endothelial dysfunction, possibly by reducing superoxide production and increasing eNOS dimerization. Relaxin treatment for two to five days in healthy rats significantly enhances NO-mediated relaxation in the mesenteric artery (van Drongelen et al., 2011, 2012, 2013; Jelinic et al., 2014; Leo et al., 2016b). Despite compelling evidence to suggest that relaxin treatment augments EDH-type relaxation in mesenteric arteries (Leo et al., 2014b) and cerebral parenchymal arterioles (Chan and Cipolla, 2011), with activation of intermediate conductance Ca2+-activated K+ channels, it is worth mentioning that these experiments were done in healthy male and non-pregnant female rats. Therefore, the discrepancies in animal species (mice vs. rats) and physiological conditions (diseased vs. healthy) may account for the differences in the mesenteric artery response to exogenous relaxin.
A key striking effect of relaxin treatment in diabetic mice is the normalization of vasoconstrictor prostanoids in the mesenteric artery. Consistent with this, our group previously shown that Rln-/- mice have altered prostanoid pathways in both the mesenteric artery (Leo et al., 2014a) and aorta (Ng et al., 2015). We suggest that relaxin likely restores diabetes-induced endothelial dysfunction by affecting the NO and prostanoid pathways, but not the EDH pathway, in both the aorta and mesenteric artery. In addition to these actions of relaxin in regulating vasodilator pathways, the hormone also suppresses the action of vasoconstrictors. Specifically, relaxin treatment markedly attenuates Ang II-induced contraction in the mesenteric artery of diabetic mice (Ng et al., 2017). It is well-established that diabetes leads to an imbalance of vasoactive factors with opposing actions, including NO and Ang II (Tousoulis et al., 2012), favoring vasoconstrictor actions, resulting in impaired vascular homeostasis. Relaxin may have a potential physiological antagonistic effect to the actions of AT-1 or AT-2 angiotensin receptor activation in the mesenteric artery (Marshall et al., 2016, 2017), counteracting diabetes-induced increased in Ang II contraction. It is feasible to speculate that relaxin counter-regulated the AT-1 mediated actions of Ang II by a mechanism which involved increased NO production. Indeed, relaxin treatment has been previously demonstrated to relieve Ang II-induced hypertension by increasing NO availability (Sasser et al., 2011), with possible concomitant reduction of oxidative stress (Sasser et al., 2014).
Roles of Relaxin in the Treatment of Diabetes-Induced Cardiac Remodeling
Left ventricular hypertrophy and fibrosis are associated with the progression of diabetic cardiomyopathy (Boudina and Abel, 2010). Relaxin treatment for the final 2 weeks of type 1 diabetes results in beneficial cardiovascular effects in diabetic mice, alleviating cardiomyocyte, and pro-hypertrophic gene expression hypertrophy (Ng et al., 2017). Interestingly, the cardioprotective effect of relaxin appears to selectively suppress cardiomyocyte hypertrophy, but not fibrosis, at least in the setting of a relatively short-term 2 weeks of relaxin administration, in direct contrast to the well-documented anti-fibrotic effects of relaxin in other animal models (Samuel et al., 2008, 2011, 2014). Relaxin is thought to inhibit the progression of fibrosis through suppression of TGF-β and downregulation of Smad2 (Samuel et al., 2014) and Smad3 phosphorylation (Sassoli et al., 2013). Given that left ventricular TGF-β gene expression was not elevated in placebo-treated type 1 diabetic mice in the above study (Ng et al., 2017), it is rational to speculate that the ability of relaxin to limit cardiac fibrosis may relate to its distinct action to restore physiological levels of TGF-β (Sassoli et al., 2013). Another possibility is that the anti-fibrotic properties of relaxin may be mediated by its ability to restore physiological levels of the inflammatory cytokine interleukin (IL)-6, as distinct to CTGF/TGF-β pathways, hence resulting in a moderate decrease in diabetes-induced interstitial cardiac collagen content as recently shown (Ng et al., 2017). Such speculation is consistent with that previously shown by Dschietzig et al. (2004), where relaxin suppressed endotoxin-induced increase in IL-6 in THP-1 cells in vitro (Dschietzig et al., 2004). Although relaxin fails to reverse diabetes-induced fibrosis in the left ventricle, it elicits potent anti-apoptotic actions in diabetic mouse myocardium (Ng et al., 2017). Consistent with this finding, Moore et al. (2007) also reported that relaxin treatment attenuated hydrogen peroxide-induced apoptosis in neonatal rat cardiomyocytes, an effect attributed to the actions of pro-survival proteins such as Akt and Bcl-2 (Moore et al., 2007). Taken together, relaxin treatment not only shows promising effects in the vasculature, but also in the heart in experimental settings of type 1 diabetes, by alleviating left ventricular hypertrophy and apoptosis.
Relaxin as an Adjunctive Treatment in Diabetes?
We and others have showed that relaxin has promising therapeutic effects to limit diabetes-induced cardiovascular complications with experimental evidence in in vitro and in vivo studies, as summarized in Table 1. Although the majority of rodent studies have utilized animal models that mimic type 1 diabetes, it remains necessary to determine whether or not relaxin exhibits similar actions in type 2 diabetes, utilizing spontaneously diabetic db/db mice or high fat-fed mice. This is important because type 2 diabetes accounts for 85% of patients affected by diabetes. The optimal relaxin treatment period in the context of type 2 diabetes also remains to be determined. In type 1 diabetes which tends to exhibit a juvenile onset, the majority of patients affected are still at a young age at time of diagnosis. Relaxin treatment in humans requires continuous intravenous infusion, hence it is not practical to start relaxin infusion in young children, where prolonged hospitalization might be required. In type 2 diabetes, the proportion of patients affected remain undiagnosed, or are considered pre-diabetic because blood glucose levels are not high enough to be classified as diabetic. Because these patients do not exhibit symptoms of severe diabetes when medical attention would be needed, it would not be feasible to treat with relaxin.
Although relaxin treatment exhibits fairly profound effects on the vasculature and heart in diabetic mice, its impact on metabolism remains controversial. Relaxin does not alter blood glucose levels, in either healthy rodents (Samuel et al., 2008; Bitto et al., 2013; Bonner et al., 2013; Wong et al., 2013) or in type 1 diabetic models (Samuel et al., 2008; Wong et al., 2013; Ng et al., 2017). However, in type 2 diabetic animal models, relaxin lowers blood glucose levels (Bitto et al., 2013; Bonner et al., 2013). Type 2 diabetes is closely associated with loss of insulin sensitivity and changes in peroxisome proliferator-activated receptor gamma coactivator-1α (PGC-1α) function, particularly at the level of both biogenesis of mitochondria and glucose metabolism (Liang and Ward, 2006). Relaxin modulates PPARγ and PCG-1α signaling pathways, downstream of RXFP1 (Singh et al., 2015). Hence, it is logical to speculate that relaxin may exert metabolic actions in type 2 diabetic mice, modulating their glucose metabolism and/or mitochondria biogenesis. Although there is promising evidence of relaxin blood glucose-lowering actions in mice with type 2 diabetes, it is however important to note that relaxin is ineffective in type 1 diabetes, where insulin administration is required for glycemic control. Hence, relaxin may be an adjunctive agent with glucose-lowering therapy for preventing or treating diabetes. Indeed, relaxin exhibits synergistic effects with the angiotensin converting enzyme inhibitor, enalapril, to abrogate cardiac fibrosis in isoprenaline-induced mouse model (Samuel et al., 2014). Thus, it is possible that relaxin might have similar actions in diabetic mice and could be used in combination with hypoglycemic drugs such as metformin, liraglutide or insulin, to then reduce cardiovascular complications associated with diabetes. However, this hypothesis remains to be tested. It is also yet to be determined whether or not relaxin exhibits adverse effects when used in combination with these conventional anti-diabetic therapies.
Conclusion
Figure 1 shows an overview of the therapeutic potential of relaxin in the pathogenesis of diabetes. Although the conventional glucose-lowering therapies such as metformin and insulin are widely prescribed for the treatment of diabetes, they may not be sufficient to limit the associated cardiovascular complications caused by hyperglycemia. Therefore, we now present the hypothesis that alternative adjunctive agents such as relaxin may offer a promising role in the prevention or treatment of diabetes-related cardiovascular complications, opening up new avenues in addressing the ever-increasing incidence of diabetes and its consequences. Although pre-clinical studies suggest a potential promising role for relaxin to protect against diabetes-associated cardiovascular complications, there remain many unresolved questions relating to the efficacy, dosage and duration of relaxin administration for clinical use in diabetic patients. Clinical trials are needed to specifically determine the translational effects of relaxin in the setting of diabetes, as there are no data available addressing this patient population. As chronic administration of relaxin is not feasible due to its pharmacokinetics and short half-life in vivo (Chen et al., 1993a,b), recent development of small molecule relaxin mimetics such as B7-33 (Hossain et al., 2016) and ML290 (Xiao et al., 2013) now warrants such enhanced translation of the vasoprotective effects of relaxin in this setting.
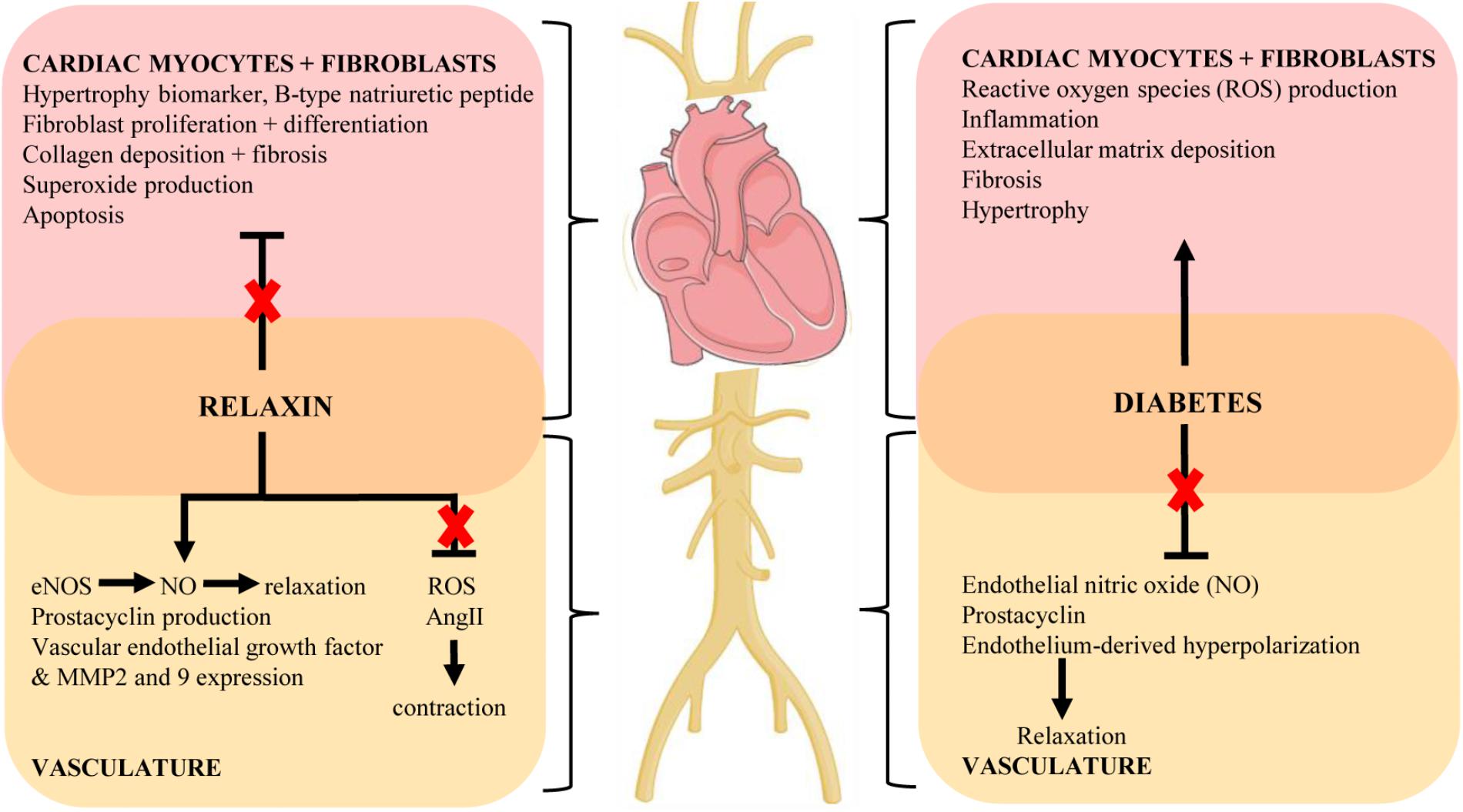
FIGURE 1. Key mechanisms of the cardiovascular complications of diabetes and proposed protective actions of relaxin to target these pathways (indicated by red cross). Diagram created using artwork provided by Servier Medical Art by Servier, licensed under a Creative Common License.
Author Contributions
HN and RR drafted the manuscript. All authors edited, revised, and approved the final version of this review.
Funding
HN received a Melbourne International Research Scholarship, a Melbourne International Fee Remission Scholarship and a grant from the Albert Shimmins Fund. RR is a National Health and Medical Research Council of Australia Senior Research Fellow (ID1059960).
Conflict of Interest Statement
The authors disclose that this research was partially funded by Novartis Pharma AG, who also provided the serelaxin as a condition of an Australian Research Council Linkage Grant (LP110200543). LP was also a paid consultant for Novartis Pharma AG.
Acknowledgments
This review forms part of HN’s Ph.D. thesis, and it is the only medium that first appeared online. The thesis can be accessed at http://hdl.handle.net/11343/123225 (Ng, 2016).
References
Angulo, J., Cuevas, P., Fernandez, A., Gabancho, S., Allona, A., Martin-Morales, A., et al. (2003). Diabetes impairs endothelium-dependent relaxation of human penile vascular tissues mediated by NO and EDHF. Biochem. Biophys. Res. Commun. 312, 1202–1208. doi: 10.1016/j.bbrc.2003.11.034
Bathgate, R. A., Ivell, R., Sanborn, B. M., Sherwood, O. D., and Summers, R. J. (2006). International union of pharmacology LVII: recommendations for the nomenclature of receptors for relaxin family peptides. Pharmacol. Rev. 58, 7–31. doi: 10.1124/pr.58.1.9
Baynes, J. W., and Thorpe, S. R. (1999). Role of oxidative stress in diabetic complications: a new perspective on an old paradigm. Diabetes Metab. Res. Rev. 48, 1–9. doi: 10.2337/diabetes.48.1.1
Bethel, M. A., Patel, R. A., Merrill, P., Lokhnygina, Y., Buse, J. B., Mentz, R. J., et al. (2017). Cardiovascular outcomes with glucagon-like peptide-1 receptor agonists in patients with type 2 diabetes: a meta-analysis. Lancet Diabetes Endocrinol. 6, 105–113. doi: 10.1016/S2213-8587(17)30412-6
Bitto, A., Irrera, N., Minutoli, L., Calo, M., Lo Cascio, P., Caccia, P., et al. (2013). Relaxin improves multiple markers of wound healing and ameliorates the disturbed healing pattern of genetically diabetic mice. Clin. Sci. (Lond.) 125, 575–585. doi: 10.1042/cs20130105
Bonner, J. S., Lantier, L., Hocking, K. M., Kang, L., Owolabi, M., James, F. D., et al. (2013). Relaxin treatment reverses insulin resistance in mice fed a high-fat diet. Diabetes Metab. Res. Rev. 62, 3251–3260. doi: 10.2337/db13-0033
Boudina, S., and Abel, E. D. (2007). Diabetic cardiomyopathy revisited. Circulation 115, 3213–3223. doi: 10.1161/circulationaha.106.679597
Boudina, S., and Abel, E. D. (2010). Diabetic cardiomyopathy, causes and effects. Rev. Endocr. Metab. Disord. 11, 31–39. doi: 10.1007/s11154-010-9131-7
Bugger, H., and Abel, E. D. (2014). Molecular mechanisms of diabetic cardiomyopathy. Diabetologia 57, 660–671. doi: 10.1007/s00125-014-3171-6
Cai, S., Khoo, J., Mussa, S., Alp, N. J., and Channon, K. M. (2005). Endothelial nitric oxide synthase dysfunction in diabetic mice: importance of tetrahydrobiopterin in eNOS dimerisation. Diabetologia 48, 1933–1940. doi: 10.1007/s00125-005-1857-5
Chan, S. L., and Cipolla, M. J. (2011). Relaxin causes selective outward remodeling of brain parenchymal arterioles via activation of peroxisome proliferator-activated receptor-gamma. FASEB J. 25, 3229–3239. doi: 10.1096/fj.10-175471
Chan, S. L., Sweet, J. G., and Cipolla, M. J. (2013). Treatment for cerebral small vessel disease: effect of relaxin on the function and structure of cerebral parenchymal arterioles during hypertension. FASEB J. 27, 3917–3927. doi: 10.1096/fj.13-230797
Chen, S. A., Perlman, A. J., Spanski, N., Peterson, C. M., Sanders, S. W., Jaffe, R., et al. (1993a). The pharmacokinetics of recombinant human relaxin in nonpregnant women after intravenous, intravaginal, and intracervical administration. Pharm. Res. 10, 834–838.
Chen, S. A., Reed, B., Nguyen, T., Gaylord, N., Fuller, G. B., and Mordenti, J. (1993b). The pharmacokinetics and absorption of recombinant human relaxin in nonpregnant rabbits and rhesus monkeys after intravenous and intravaginal administration. Pharm. Res. 10, 223–227.
Ding, H., Hashem, M., Wiehler, W. B., Lau, W., Martin, J., Reid, J., et al. (2005). Endothelial dysfunction in the streptozotocin-induced diabetic apoE-deficient mouse. Br. J. Pharmacol. 146, 1110–1118. doi: 10.1038/sj.bjp.0706417
Ding, H., and Triggle, C. R. (2010). Endothelial dysfunction in diabetes: multiple targets for treatment. Pflugers Arch. 459, 977–994. doi: 10.1007/s00424-010-0807-3
Dschietzig, T., Bartsch, C., Richter, C., Laule, M., Baumann, G., and Stangl, K. (2003). Relaxin, a pregnancy hormone, is a functional endothelin-1 antagonist: attenuation of endothelin-1-mediated vasoconstriction by stimulation of endothelin type-B receptor expression via ERK-1/2 and nuclear factor-kappaB. Circ. Res. 92, 32–40. doi: 10.1161/01.RES.0000051884.27117.7E
Dschietzig, T., Bartsch, C., Stangl, V., Baumann, G., and Stangl, K. (2004). Identification of the pregnancy hormone relaxin as glucocorticoid receptor agonist. FASEB J. 18, 1536–1538. doi: 10.1096/fj.03-1120fje
Dschietzig, T., Brecht, A., Bartsch, C., Baumann, G., Stangl, K., and Alexiou, K. (2012). Relaxin improves TNF-alpha-induced endothelial dysfunction: the role of glucocorticoid receptor and phosphatidylinositol 3-kinase signalling. Cardiovasc. Res. 95, 97–107. doi: 10.1093/cvr/cvs149
Fisher, C., MacLean, M., Morecroft, I., Seed, A., Johnston, F., Hillier, C., et al. (2002). Is the pregnancy hormone relaxin also a vasodilator peptide secreted by the heart? Circulation 106, 292–295.
Forbes, J. M., and Fotheringham, A. K. (2017). Vascular complications in diabetes: old messages, new thoughts. Diabetologia 60, 2129–2138. doi: 10.1007/s00125-017-4360-x
Guzik, T. J., Mussa, S., Gastaldi, D., Sadowski, J., Ratnatunga, C., Pillai, R., et al. (2002). Mechanisms of increased vascular superoxide production in human diabetes mellitus: role of NAD(P)H oxidase and endothelial nitric oxide synthase. Circulation 105, 1656–1662. doi: 10.1161/01.CIR.0000012748.58444.08
Henry, B. L., Gabris, B., Li, Q., Martin, B., Giannini, M., Parikh, A., et al. (2016). Relaxin suppresses atrial fibrillation in aged rats by reversing fibrosis and upregulating Na(+) channels. Heart Rhythm 13, 983–991. doi: 10.1016/j.hrthm.2015.12.030
Holman, R. (2007). Metformin as first choice in oral diabetes treatment: the UKPDS experience. Journ. Annu. Diabetol. Hotel Dieu 13–20.
Hossain, M. A., Kocan, M., Yao, S. T., Royce, S. G., Nair, V. B., Siwek, C., et al. (2016). A single-chain derivative of the relaxin hormone is a functionally selective agonist of the G protein-coupled receptor, RXFP1. Chem. Sci. 7, 3805–3819. doi: 10.1039/C5SC04754D
Huynh, K., Bernardo, B. C., McMullen, J. R., and Ritchie, R. H. (2014). Diabetic cardiomyopathy: mechanisms and new treatment strategies targeting antioxidant signaling pathways. Pharmacol. Ther. 142, 375–415. doi: 10.1016/j.pharmthera.2014.01.003
Iimoto, D. S., Covell, J. W., and Harper, E. (1988). Increase in cross-linking of type I and type III collagens associated with volume-overload hypertrophy. Circ. Res. 63, 399–408. doi: 10.1161/01.RES.63.2.399
Jelinic, M., Leo, C. H., Post Uiterweer, E. D., Sandow, S. L., Gooi, J. H., Wlodek, M. E., et al. (2014). Localization of relaxin receptors in arteries and veins, and region-specific increases in compliance and bradykinin-mediated relaxation after in vivo serelaxin treatment. FASEB J. 28, 275–287. doi: 10.1096/fj.13-233429
Johnstone, M. T., Creager, S. J., Scales, K. M., Cusco, J. A., Lee, B. K., and Creager, M. A. (1993). Impaired endothelium-dependent vasodilation in patients with insulin-dependent diabetes mellitus. Circulation 88, 2510–2516. doi: 10.1161/01.CIR.88.6.2510
Lavrentyev, E. N., Estes, A. M., and Malik, K. U. (2007). Mechanism of high glucose induced angiotensin II production in rat vascular smooth muscle cells. Circ. Res. 101, 455–464. doi: 10.1161/circresaha.107.151852
Lekgabe, E. D., Kiriazis, H., Zhao, C., Xu, Q., Moore, X. L., Su, Y., et al. (2005). Relaxin reverses cardiac and renal fibrosis in spontaneously hypertensive rats. Hypertension 46, 412–418. doi: 10.1161/01.HYP.0000171930.00697.2f
Leo, C. H., Hart, J. L., and Woodman, O. L. (2011a). 3’,4’-Dihydroxyflavonol reduces superoxide and improves nitric oxide function in diabetic rat mesenteric arteries. PLoS One 6:e20813. doi: 10.1371/journal.pone.0020813
Leo, C. H., Hart, J. L., and Woodman, O. L. (2011b). Impairment of both nitric oxide-mediated and EDHF-type relaxation in small mesenteric arteries from rats with streptozotocin-induced diabetes. Br. J. Pharmacol. 162, 365–377. doi: 10.1111/j.1476-5381.2010.01023.x
Leo, C. H., Jelinic, M., Gooi, J. H., Tare, M., and Parry, L. J. (2014a). A vasoactive role for endogenous relaxin in mesenteric arteries of male mice. PLoS One 9:e107382. doi: 10.1371/journal.pone.0107382
Leo, C. H., Jelinic, M., Parkington, H. C., Tare, M., and Parry, L. J. (2014b). Acute intravenous injection of serelaxin (recombinant human relaxin-2) causes rapid and sustained bradykinin-mediated vasorelaxation. J. Am. Heart Assoc. 3:e000493. doi: 10.1161/jaha.113.000493
Leo, C. H., Jelinic, M., Ng, H. H., Marshall, S. A., Novak, J., Tare, M., et al. (2017). Vascular actions of relaxin: nitric oxide and beyond. Br. J. Pharmacol. 174, 1002–1014. doi: 10.1111/bph.13614
Leo, C. H., Jelinic, M., Ng, H. H., Tare, M., and Parry, L. J. (2016a). Serelaxin: a novel therapeutic for vascular diseases. Trends Pharmacol. Sci. 37, 498–507. doi: 10.1016/j.tips.2016.04.001
Leo, C. H., Jelinic, M., Ng, H. H., Tare, M., and Parry, L. J. (2016b). Time-dependent activation of prostacyclin and nitric oxide pathways during continuous i.v. infusion of serelaxin (recombinant human H2 relaxin). Br. J. Pharmacol. 173, 1005–1017. doi: 10.1111/bph.13404
Liang, H., and Ward, W. F. (2006). PGC-1alpha: a key regulator of energy metabolism. Adv. Physiol. Educ. 30, 145–151. doi: 10.1152/advan.00052.2006
Lytvyn, Y., Bjornstad, P., Udell, J. A., Lovshin, J. A., and Cherney, D. Z. I. (2017). Sodium glucose cotransporter-2 inhibition in heart failure: potential mechanisms, clinical applications, and summary of clinical trials. Circulation 136, 1643–1658. doi: 10.1161/circulationaha.117.030012
Mangiavacchi, M., Gasparini, M., Genovese, S., Pini, D., Klersy, C., Bragato, R., et al. (2008). Insulin-treated type 2 diabetes is associated with a decreased survival in heart failure patients after cardiac resynchronization therapy. Pacing Clin. Electrophysiol. 31, 1425–1432. doi: 10.1111/j.1540-8159.2008.01206.x
Marshall, S. A., Leo, C. H., Girling, J. E., Tare, M., Beard, S., Hannan, N. J., et al. (2017). Relaxin treatment reduces angiotensin II-induced vasoconstriction in pregnancy and protects against endothelial dysfunctiondagger. Biol. Reprod. 96, 895–906. doi: 10.1093/biolre/iox023
Marshall, S. A., Leo, C. H., Senadheera, S. N., Girling, J. E., Tare, M., and Parry, L. J. (2016). Relaxin deficiency attenuates pregnancy-induced adaptation of the mesenteric artery to angiotensin II in mice. Am. J. Physiol. Regul. Integr. Comp. Physiol. 310, R847–R857. doi: 10.1152/ajpregu.00506.2015
McGarry, J. D. (1992). What if Minkowski had been ageusic? An alternative angle on diabetes. Science 258, 766–770. doi: 10.1126/science.1439783
McGuane, J. T., Danielson, L. A., Debrah, J. E., Rubin, J. P., Novak, J., and Conrad, K. P. (2011a). Angiogenic growth factors are new and essential players in the sustained relaxin vasodilatory pathway in rodents and humans. Hypertension 57, 1151–1160. doi: 10.1161/hypertensionaha.110.165027
McGuane, J. T., Debrah, J. E., Sautina, L., Jarajapu, Y. P., Novak, J., Rubin, J. P., et al. (2011b). Relaxin induces rapid dilation of rodent small renal and human subcutaneous arteries via PI3 kinase and nitric oxide. Endocrinology 152, 2786–2796. doi: 10.1210/en.2010-1126
Moore, X. L., Tan, S. L., Lo, C. Y., Fang, L., Su, Y. D., Gao, X. M., et al. (2007). Relaxin antagonizes hypertrophy and apoptosis in neonatal rat cardiomyocytes. Endocrinology 148, 1582–1589. doi: 10.1210/en.2006-1324
Ng, H. H. (2016). Vasoprotective Actions of Relaxin in the Vascular System. Ph.D. thesis, University of Melbourne, Parkville VIC.
Ng, H. H., Jelinic, M., Parry, L. J., and Leo, C. H. (2015). Increased superoxide production and altered nitric oxide-mediated relaxation in the aorta of young but not old male relaxin-deficient mice. Am. J. Physiol. Heart Circ. Physiol. 309, H285–H296. doi: 10.1152/ajpheart.00786.2014
Ng, H. H., Leo, C. H., and Parry, L. J. (2016). Serelaxin (recombinant human relaxin-2) prevents high glucose-induced endothelial dysfunction by ameliorating prostacyclin production in the mouse aorta. Pharmacol. Res. 107, 220–228. doi: 10.1016/j.phrs.2016.03.011
Ng, H. H., Leo, C. H., Prakoso, D., Qin, C., Ritchie, R. H., and Parry, L. J. (2017). Serelaxin treatment reverses vascular dysfunction and left ventricular hypertrophy in a mouse model of Type 1 diabetes. Sci. Rep. 7:39604. doi: 10.1038/srep39604
Nichols, G. A., Koro, C. E., Gullion, C. M., Ephross, S. A., and Brown, J. B. (2005). The incidence of congestive heart failure associated with antidiabetic therapies. Diabetes Metab. Res. Rev. 21, 51–57. doi: 10.1002/dmrr.480
Novak, J., Parry, L. J., Matthews, J. E., Kerchner, L. J., Indovina, K., Hanley-Yanez, K., et al. (2006). Evidence for local relaxin ligand-receptor expression and function in arteries. FASEB J. 20, 2352–2362. doi: 10.1096/fj.06-6263com
Pappachan, J. M., Varughese, G. I., Sriraman, R., and Arunagirinathan, G. (2013). Diabetic cardiomyopathy: pathophysiology, diagnostic evaluation and management. World J. Diabetes 4, 177–189. doi: 10.4239/wjd.v4.i5.177
Parikh, A., Patel, D., McTiernan, C. F., Xiang, W., Haney, J., Yang, L., et al. (2013). Relaxin suppresses atrial fibrillation by reversing fibrosis and myocyte hypertrophy and increasing conduction velocity and sodium current in spontaneously hypertensive rat hearts. Circ. Res. 113, 313–321. doi: 10.1161/circresaha.113.301646
Pieper, G. M., Meier, D. A., and Hager, S. R. (1995). Endothelial dysfunction in a model of hyperglycemia and hyperinsulinemia. Am. J. Physiol. 269(3 Pt 2), H845–H850. doi: 10.1152/ajpheart.1995.269.3.H845
Pini, A., Boccalini, G., Baccari, M. C., Becatti, M., Garella, R., Fiorillo, C., et al. (2016). Protection from cigarette smoke-induced vascular injury by recombinant human relaxin-2 (serelaxin). J. Cell Mol. Med. 20, 891–902. doi: 10.1111/jcmm.12802
Prakoso, D., De Blasio, M. J., Qin, C., Rosli, S., Kiriazis, H., Qian, H., et al. (2017). Phosphoinositide 3-kinase (p110alpha) gene delivery limits diabetes-induced cardiac NADPH oxidase and cardiomyopathy in a mouse model with established diastolic dysfunction. Clin. Sci. (Lond.) 131, 1345–1360. doi: 10.1042/cs20170063
Randle, P. J., Garland, P. B., Hales, C. N., and Newsholme, E. A. (1963). The glucose fatty-acid cycle. Its role in insulin sensitivity and the metabolic disturbances of diabetes mellitus. Lancet 1, 785–789. doi: 10.1016/S0140-6736(63)91500-9
Reaven, G. M., Lithell, H., and Landsberg, L. (1996). Hypertension and associated metabolic abnormalities–the role of insulin resistance and the sympathoadrenal system. N. Engl. J. Med. 334, 374–381. doi: 10.1056/nejm199602083340607
Ritchie, R. H., Zerenturk, E. J., Prakoso, D., and Calkin, A. C. (2017). Lipid metabolism and its implications for type 1 diabetes-associated cardiomyopathy. J. Mol. Endocrinol. 58, R225–R240. doi: 10.1530/JME-16-0249
Samuel, C. S., Bodaragama, H., Chew, J. Y., Widdop, R. E., Royce, S. G., and Hewitson, T. D. (2014). Serelaxin is a more efficacious antifibrotic than enalapril in an experimental model of heart disease. Hypertension 64, 315–322. doi: 10.1161/hypertensionaha.114.03594
Samuel, C. S., Cendrawan, S., Gao, X. M., Ming, Z., Zhao, C., Kiriazis, H., et al. (2011). Relaxin remodels fibrotic healing following myocardial infarction. Lab. Invest. 91, 675–690. doi: 10.1038/labinvest.2010.198
Samuel, C. S., Hewitson, T. D., Zhang, Y., and Kelly, D. J. (2008). Relaxin ameliorates fibrosis in experimental diabetic cardiomyopathy. Endocrinology 149, 3286–3293. doi: 10.1210/en.2008-0250
Samuel, C. S., Summers, R. J., and Hewitson, T. D. (2016). Antifibrotic actions of serelaxin - new roles for an old player. Trends Pharmacol. Sci. 37, 485–497. doi: 10.1016/j.tips.2016.02.007
Samuel, C. S., Unemori, E. N., Mookerjee, I., Bathgate, R. A., Layfield, S. L., Mak, J., et al. (2004). Relaxin modulates cardiac fibroblast proliferation, differentiation, and collagen production and reverses cardiac fibrosis in vivo. Endocrinology 145, 4125–4133. doi: 10.1210/en.2004-0209
Samuel, V. T., and Shulman, G. I. (2016). The pathogenesis of insulin resistance: integrating signaling pathways and substrate flux. J. Clin. Invest. 126, 12–22. doi: 10.1172/jci77812
Sano, M. (2018). A new class of drugs for heart failure: SGLT2 inhibitors reduce sympathetic overactivity. J. Cardiol. 71, 471–476. doi: 10.1016/j.jjcc.2017.12.004
Santulli, G., Pagano, G., Sardu, C., Xie, W., Reiken, S., D’Ascia, S. L., et al. (2015). Calcium release channel RyR2 regulates insulin release and glucose homeostasis. J. Clin. Invest. 125, 1968–1978. doi: 10.1172/jci79273
Sardu, C., Marfella, R., and Santulli, G. (2014). Impact of diabetes mellitus on the clinical response to cardiac resynchronization therapy in elderly people. J. Cardiovasc. Transl. Res. 7, 362–368. doi: 10.1007/s12265-014-9545-9
Sarwar, M., Du, X. J., Dschietzig, T. B., and Summers, R. J. (2017). The actions of relaxin on the human cardiovascular system. Br. J. Pharmacol. 174, 933–949. doi: 10.1111/bph.13523
Sasser, J. M., Cunningham, M. W. Jr., and Baylis, C. (2014). Serelaxin reduces oxidative stress and asymmetric dimethylarginine in angiotensin II-induced hypertension. Am. J. Physiol. Renal Physiol. 307, F1355–F1362. doi: 10.1152/ajprenal.00407.2014
Sasser, J. M., Molnar, M., and Baylis, C. (2011). Relaxin ameliorates hypertension and increases nitric oxide metabolite excretion in angiotensin II but not N(omega)-nitro-L-arginine methyl ester hypertensive rats. Hypertension 58, 197–204. doi: 10.1161/hypertensionaha.110.164392
Sassoli, C., Chellini, F., Pini, A., Tani, A., Nistri, S., Nosi, D., et al. (2013). Relaxin prevents cardiac fibroblast-myofibroblast transition via notch-1-mediated inhibition of TGF-beta/Smad3 signaling. PLoS One 8:e63896. doi: 10.1371/journal.pone.0063896
Shaw, J. E., Sicree, R. A., and Zimmet, P. Z. (2010). Global estimates of the prevalence of diabetes for 2010 and 2030. Diabetes Res. Clin. Pract. 87, 4–14. doi: 10.1016/j.diabres.2009.10.007
Sherwood, O. D. (2004). Relaxin’s physiological roles and other diverse actions. Endocr. Rev. 25, 205–234. doi: 10.1210/er.2003-0013
Shi, Y., and Vanhoutte, P. M. (2017). Macro- and microvascular endothelial dysfunction in diabetes. J. Diabetes 9, 434–449. doi: 10.1111/1753-0407.12521
Singh, S., Simpson, R. L., and Bennett, R. G. (2015). Relaxin activates peroxisome proliferator-activated receptor gamma (PPARgamma) through a pathway involving PPARgamma coactivator 1alpha (PGC1alpha). J. Biol. Chem. 290, 950–959. doi: 10.1074/jbc.M114.589325
Singh, V. P., Le, B., Khode, R., Baker, K. M., and Kumar, R. (2008). Intracellular angiotensin II production in diabetic rats is correlated with cardiomyocyte apoptosis, oxidative stress, and cardiac fibrosis. Diabetes Metab. Res. Rev. 57, 3297–3306. doi: 10.2337/db08-0805
Smooke, S., Horwich, T. B., and Fonarow, G. C. (2005). Insulin-treated diabetes is associated with a marked increase in mortality in patients with advanced heart failure. Am. Heart. J. 149, 168–174. doi: 10.1016/j.ahj.2004.07.005
Squadrito, F., Bitto, A., Irrera, N., Minutoli, L., Caccia, P., and Altavilla, D. (2013). Porcine derived relaxin stimulates new vessel formation and improves the disturbed wound healing of the genetically diabetic mice. Ital. J. Anat. Embryol. 118(1 Suppl.), 66–70.
Tate, M., Deo, M., Cao, A. H., Hood, S. G., Huynh, K., Kiriazis, H., et al. (2017a). Insulin replacement limits progression of diabetic cardiomyopathy in the low-dose streptozotocin-induced diabetic rat. Diab. Vasc. Dis. Res. 14, 423–433. doi: 10.1177/1479164117710390
Tate, M., Grieve, D. J., and Ritchie, R. H. (2017b). Are targeted therapies for diabetic cardiomyopathy on the horizon? Clin. Sci. (Lond.) 131, 897–915. doi: 10.1042/cs20160491
Tiyerili, V., Beiert, T., Schatten, H., Camara, B., Jehle, J., Schrickel, J. W., et al. (2016). Anti-atherosclerotic effects of serelaxin in apolipoprotein E-deficient mice. Atherosclerosis 251, 430–437. doi: 10.1016/j.atherosclerosis.2016.06.008
Tousoulis, D., Kampoli, A. M., and Stefanadis, C. (2012). Diabetes mellitus and vascular endothelial dysfunction: current perspectives. Curr. Vasc. Pharmacol. 10, 19–32. doi: 10.2174/157016112798829797
Unemori, E. N., Lewis, M., Constant, J., Arnold, G., Grove, B. H., Normand, J., et al. (2000). Relaxin induces vascular endothelial growth factor expression and angiogenesis selectively at wound sites. Wound Repair Regen. 8, 361–370. doi: 10.1111/j.1524-475X.2000.00361.x
van Drongelen, J., Ploemen, I. H., Pertijs, J., Gooi, J. H., Sweep, F. C., Lotgering, F. K., et al. (2011). Aging attenuates the vasodilator response to relaxin. Am. J. Physiol. Heart Circ. Physiol. 300, H1609–H1615. doi: 10.1152/ajpheart.00360.2010
van Drongelen, J., van Koppen, A., Pertijs, J., Gooi, J. H., Parry, L. J., Sweep, F. C., et al. (2012). Impaired vascular responses to relaxin in diet-induced overweight female rats. J. Appl. Physiol. 112, 962–969. doi: 10.1152/japplphysiol.00470.2011
van Drongelen, J., van Koppen, A., Pertijs, J., Gooi, J. H., Sweep, F. C., Lotgering, F. K., et al. (2013). Impaired effect of relaxin on vasoconstrictor reactivity in spontaneous hypertensive rats. Peptides 49, 41–48. doi: 10.1016/j.peptides.2013.08.020
Vanhoutte, P. M., Shimokawa, H., Feletou, M., and Tang, E. H. (2017). Endothelial dysfunction and vascular disease - a 30th anniversary update. Acta Physiol. 219, 22–96. doi: 10.1111/apha.12646
Wang, P., Li, H. W., Wang, Y. P., Chen, H., and Zhang, P. (2009). Effects of recombinant human relaxin upon proliferation of cardiac fibroblast and synthesis of collagen under high glucose condition. J. Endocrinol. Invest. 32, 242–247. doi: 10.1007/bf03346460
Wei, X., Yang, Y., Jiang, Y. J., Lei, J. M., Guo, J. W., and Xiao, H. (2018). Relaxin ameliorates high glucose-induced cardiomyocyte hypertrophy and apoptosis via the Notch1 pathway. Exp. Ther. Med. 15, 691–698. doi: 10.3892/etm.2017.5448
Wong, S. E., Samuel, C. S., Kelly, D. J., Zhang, Y., Becker, G. J., and Hewitson, T. D. (2013). The anti-fibrotic hormone relaxin is not reno-protective, despite being active, in an experimental model of type 1 diabetes. Protein Pept. Lett. 20, 1029–1038. doi: 10.2174/0929866511320090009
Xiao, J., Huang, Z., Chen, C. Z., Agoulnik, I. U., Southall, N., Hu, X., et al. (2013). Identification and optimization of small-molecule agonists of the human relaxin hormone receptor RXFP1. Nat. Commun. 4:1953. doi: 10.1038/ncomms2953
Xu, Q., Chakravorty, A., Bathgate, R. A., Dart, A. M., and Du, X. J. (2010). Relaxin therapy reverses large artery remodeling and improves arterial compliance in senescent spontaneously hypertensive rats. Hypertension 55, 1260–1266. doi: 10.1161/hypertensionaha.109.149369
Zhang, X., Fu, Y., Li, H., Shen, L., Chang, Q., Pan, L., et al. (2018). H3 relaxin inhibits the collagen synthesis via ROS- and P2X7R-mediated NLRP3 inflammasome activation in cardiac fibroblasts under high glucose. J. Cell Mol. Med. 22, 1816–1825. doi: 10.1111/jcmm.13464
Zhang, X., Ma, X., Zhao, M., Zhang, B., Chi, J., Liu, W., et al. (2015). H2 and H3 relaxin inhibit high glucose-induced apoptosis in neonatal rat ventricular myocytes. Biochimie 108, 59–67. doi: 10.1016/j.biochi.2014.11.004
Zhang, X., Pan, L., Yang, K., Fu, Y., Liu, Y., Chi, J., et al. (2017). H3 relaxin protects against myocardial injury in experimental diabetic cardiomyopathy by inhibiting myocardial apoptosis, fibrosis and inflammation. Cell Physiol. Biochem. 43, 1311–1324. doi: 10.1159/000481843
Zhu, N., Liu, B., Luo, W., Zhang, Y., Li, H., Li, S., et al. (2014). Vasoconstrictor role of cyclooxygenase-1-mediated prostacyclin synthesis in non-insulin-dependent diabetic mice induced by high-fat diet and streptozotocin. Am. J. Physiol. Heart Circ. Physiol. 307, H319–H327. doi: 10.1152/ajpheart.00022.2014
Keywords: relaxin, diabetes, vasculopathy, endothelial dysfunction, remodeling, cardiomyopathy
Citation: Ng HH, Leo CH, Parry LJ and Ritchie RH (2018) Relaxin as a Therapeutic Target for the Cardiovascular Complications of Diabetes. Front. Pharmacol. 9:501. doi: 10.3389/fphar.2018.00501
Received: 13 February 2018; Accepted: 26 April 2018;
Published: 15 May 2018.
Edited by:
Issy Laher, University of British Columbia, United StatesReviewed by:
Gaetano Santulli, Columbia University, United StatesJavier Angulo, Hospital Universitario Ramón y Cajal, Spain
Alberto Ferlin, University of Brescia, Italy
Copyright © 2018 Ng, Leo, Parry and Ritchie. This is an open-access article distributed under the terms of the Creative Commons Attribution License (CC BY). The use, distribution or reproduction in other forums is permitted, provided the original author(s) and the copyright owner are credited and that the original publication in this journal is cited, in accordance with accepted academic practice. No use, distribution or reproduction is permitted which does not comply with these terms.
*Correspondence: Hooi Hooi Ng, aG9vaWhvb2kubmdAZml1LmVkdQ==