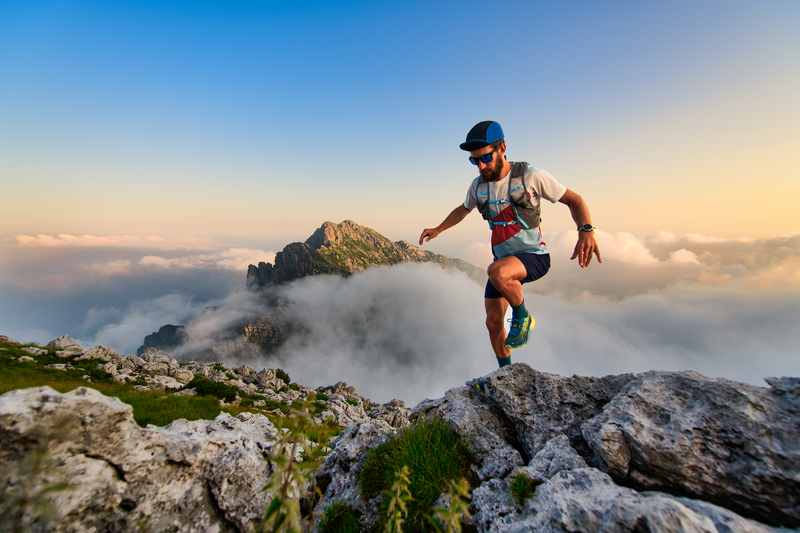
95% of researchers rate our articles as excellent or good
Learn more about the work of our research integrity team to safeguard the quality of each article we publish.
Find out more
ORIGINAL RESEARCH article
Front. Pharmacol. , 20 March 2018
Sec. Predictive Toxicology
Volume 9 - 2018 | https://doi.org/10.3389/fphar.2018.00253
Alumina nanoparticles (AlNP) have been shown to accumulate in organs and penetrate biological barriers which lead to toxic effects in many organ systems. However, it is not known whether AlNP exposure to female mice during pregnancy can affect the development of the central nervous system or induce neurodevelopmental toxicity in the offspring. The present study aims to examine the effect of AlNP on neurodevelopment and associated underlying mechanism. ICR strain adult female mice were randomly divided into four groups, which were treated with normal saline (control), 10 μm particle size of alumina (bulk-Al), and 50 and 13 nm AlNP during entire pregnancy period. Aluminum contents in the hippocampus of newborns were measured and neurodevelopmental behaviors were tracked in the offspring from birth to 1 month of age. Furthermore, oxidative stress and neurotransmitter levels were measured in the cerebral cortex of the adolescents. Our results showed that aluminum contents in the hippocampus of newborns in AlNP-treated groups were significantly higher than those in bulk-Al and controls. Moreover, the offspring delivered by AlNP-treated female mice displayed stunted neurodevelopmental behaviors. Finally, the offspring of AlNP-treated mice demonstrated significantly increased anxiety-like behavior with impaired learning and memory performance at 1 month of age. The underlying mechanism could be related to increased oxidative stress and decreased neurotransmitter levels in the cerebral cortex. We therefore conclude that AlNP exposure of female mice during pregnancy can induce neurodevelopmental toxicity in offspring.
Neurodevelopmental disabilities affect millions of children worldwide (Grandjean and Landrigan, 2014); it is mainly because the placenta does not block the transmission of many environmental toxicants from the maternal to the fetal circulation (Needham et al., 2011). It is known that nanoparticles (NPs), unlike bulk materials, are able to pass through biological barriers, such as the blood–brain barrier (BBB), blood–testis barrier, and placental barrier (Keelan, 2011; Zoroddu et al., 2014; Bakand and Hayes, 2016; Muoth et al., 2016). Therefore, NPs can localize in various organs and tissues and generate toxicological effects (Kagan et al., 2005; Nel et al., 2006; Oesterling et al., 2008; Almeida et al., 2011). Although the safety issues are of high importance, related toxicological studies are far behind the development of nanotechnologies (Chen et al., 2008). Alumina nanoparticles (AlNP) have unique physical and chemical characteristics compared to bulk alumina (bulk-Al); they are widely used in industries of pharmacy, water treatment, and manufacturing (Kagan et al., 2005), raising concern of the biological safety regarding public health.
Studies have shown that AlNP can penetrate biological barriers and accumulate in multiple organs (Chen et al., 2008; Oesterling et al., 2008; Almeida et al., 2011; Morsy et al., 2016b), leading to neurotoxicity (Oesterling et al., 2008; Dong et al., 2011; Zhang et al., 2011; Chen et al., 2013; Shah et al., 2015; Morsy et al., 2016a), immunotoxicity (Braydich-Stolle et al., 2010), genotoxicity (Balasubramanyam et al., 2009a,b), as well as toxicity in the lung (Balasubramanyam et al., 2009a; Li et al., 2016), liver (Shrivastava et al., 2014; Morsy et al., 2016a), kidney (Morsy et al., 2016a), and skin (Dey et al., 2008). Our previous work indicated that AlNP can cause neurotoxicity both in vivo and in vitro (Zhang et al., 2011, 2013). However, no studies have addressed whether AlNP induces developmental toxicity, and in particular, neurodevelopmental toxicity.
Previous research on metal oxidative NPs found developmental toxicity induced by nano-silver (Xin et al., 2015; Charehsaz et al., 2016; Ema et al., 2017; Pecoraro et al., 2017b), nano-silica (Yi et al., 2016), graphene oxide (Buccheri et al., 2016; Pecoraro et al., 2017a), zinc oxide (Bonfanti et al., 2015; Zhou et al., 2015; Lee et al., 2016; Spence et al., 2016), iron oxide (Di Bona et al., 2015), copper oxide (Maisano et al., 2015; Ganesan et al., 2016; Torres-Duarte et al., 2016), magnesium oxide (Ghobadian et al., 2015), titanium dioxide (Scuderi et al., 2014; Parivar et al., 2015; Rollerova et al., 2015; Brundo et al., 2016), and cerium oxide (Andersen et al., 2016). Moreover, studies have shown that bulk-Al, once entering the brain, persists for a very long time with a half-life ranging from 20% of the lifespan to greater than the entire lifespan (Yokel, 2000, 2002; Priest, 2004; Exley and House, 2011). Due to the continuing accumulation in brain, bulk-Al compound can lead to both neurotoxicity (Nel et al., 2006) and developmental toxicity (Poirier et al., 2011; Abu-Taweel et al., 2012). In particular, bulk-Al can incorporate into the brain of the fetus following gestational exposure of female mice (Cranmer et al., 1986; Yumoto et al., 2001). Since AlNP belong to the group of metal oxidative NPs and have Al compound as its basic chemical component, we hypothesized that AlNP could induce neurodevelopmental toxicity.
Given the high susceptibility of the central nervous system (CNS) to external insults during the developmental period, we address specific concerns on neurodevelopmental toxicity effects of AlNP in the present study. Female mice were treated with AlNP at 13 or 50 nm particle sizes via nasal drip delivery prior to and throughout the entire pregnancy. Physical developmental and motor maturation levels of the pups were tracked as indicators of neurotoxicity, anxiety, and cognitive performance of adolescents and were used to confirm the neurotoxic effect; the underlying mechanism was explored as well. To our best knowledge, this study is the first investigation regarding neurodevelopmental toxicity of AlNP and warrants further studies to address potential neurodevelopmental toxicity associated with NPs.
Bulk-Al (10 μm particle size) and AlNP (13 and 50 nm particle sizes) were all purchased from Sigma-Aldrich Corporation (St. Louis, MO, United States). NP stock suspensions were freshly prepared in double distilled (dd) water. Ultrasonic vibration (100 W, 30 kHz) was performed for 30 min before use. AlNP were characterized using a transmission electron microscope (TEM) and the diameters were analyzed by Image-pro plus software. Zeta potential of AlNP was analyzed with a Nano Zetasizer (Malvern Instrument, Ltd., Malvern, United Kingdom).
For the TEM, a droplet of AlNP suspension was placed on a 200-mesh Cu-lacy carbon TEM grid (EM Sciences, Hatfield, PA, United States), and was allowed to dry overnight in a vacuum oven at room temperature. After evaporation, the AlNP were dispersed on the TEM grid. Observation and imaging were performed using a JEOL 2010f instrument (JEOL, Tokyo, Japan) with an operating voltage of 200 kV.
Zeta potential of NPs was measured by a Zetasizer (Malvern Instruments, Ltd.) at 25°C. NP stock was diluted 1:4 in double distilled water and measured in triplicates with 20 sub-runs. The samples were stored for 2 h to achieve equilibrium, and put into an ultrasound bath to disperse any aggregates before the zeta potential measurements.
Thirty-six adult ICR mice (25–30 g, 12 males and 24 females) were purchased from Chinese Science Academy Animal Research Institute, and acclimatized for 1 week before the start of the experiment. The animal experiments were performed in accordance with the “Policies on the Use of Animals and Humans in Neuroscience Research” published by the Society for Neuroscience in 1995. The protocol was approved by the Animal Administration and Ethics Committee of Shanxi Medical University. All the mice were fed with homemade standard laboratory food (Shanxi Medical University, China) and water ad libitum at (23 ± 3)°C, relative humidity of (50 ± 10)%, a 12 h light/dark cycle, and ventilation of (10–20) times/h. Temperature and relative humidity were monitored daily. After acclimation, 24 female mice were randomly divided into four groups, six for each group, which were treated with 25–35 μL of saline control, bulk-Al, or AlNP (50 or 13 nm particle sizes) by nasal drip three times/day at the concentration of 50 mg/kg of body weight. The female mice were mated with healthy untreated male mice overnight after a 2-week treatment period. The male mice were removed after successful mating and the female mice were continually exposed to the treatments until birth of offspring. Pups were randomly chosen from each litter group for tests, two pups for detection of aluminum content, eight pups for body weight and neuromotor maturation tests, and two adolescents (one male and one female) for Openfield and Morris water maze (MWM) task. In total, 12 pups from each treatment group were used for aluminum content measurement, 48 pups for body weight and neuromotor maturation tests, and 12 adolescents (six males and six females) for Openfield and MWM task followed by oxidative stress and neurotransmitter levels’ measurement (Figure 1).
FIGURE 1. Flowchart of experimental design. Twenty-four female mice were randomly divided into four groups and were treated with saline control, bulk-Al, or AlNP (50 or 13 nm particle sizes) during pregnancy, respectively. Aluminum content and body weight were measured in newborns, neuromotor maturation tests were tracked in pups, and Openfield and Morris water maze task were performed in adolescents followed by oxidative stress and neurotransmitter levels measurement.
Body weight (BW) used as an indicator of development was tracked in newborn pups every 3 days from postnatal day (PD) 1 through PD28.
Newborns were anesthetized with 75% ice-cold ethanol for 2 min. Next, brains were carefully removed, and the hippocampus was dissected on an ice-cold glass tube. Then, the hippocampus was digested with a multi-wave 3000 sample preparation system (PerkinElmer, Inc., Shelton, WA, United States) according to the manufacturer’s instruction. In the presence of 5% HNO3, aluminum content of samples was analyzed using graphite furnace atomic absorption spectrometry as previously described with minor modifications (Gorsky and Dietz, 1978).
Physical developmental landmarks are morphological indicators of development. Examples of such landmarks, including ear pinna open, eye opening, and teeth appearance, were observed in the developing offspring starting from PD1 through the entire developing period until PD28. The exact day in which the neonatal ear pinna completely detached from the head, both eyes opened, and the emergence of teeth through the gum line was recorded.
To assess neuromotor maturation and general behavioral profiles in offspring of AlNP-treated female mice, a routine testing battery was performed on each pup.
A pup was placed in a supine position on a rough horizontal board and righting reflex was determined to be present if the pup was able to flip itself to the normal dorso-ventral position within 2 min. The test was performed twice for each pup on both PD4 and PD7.
A pup was placed on a platform (30 cm height) with its forepaws touching the edge and head facing outwards from the center of the platform. Cliff avoidance was considered positive if the pup moved its body away from the “cliff” within a 1 min.
It is also referred to as the forelimb grip strength test. Endurance was measured on PD12 and PD14. The forelimbs of pups were positioned on a wooden pole with a diameter of 0.5 cm. The amount of time that the pup was able to grasp the pole was recorded.
The Openfield and MWM tests were used to evaluate anxiety and cognitive performance in adolescent mice.
The Openfield test was conducted on PD28. General motion in a grid was measured in an Openfield apparatus, consisting of a square wooden arena with surrounding walls (80 cm × 80 cm × 30 cm). The floor of the arena was divided into 64 squares of equal size. The visual observations in the arena lasted 300 s for each mouse. The path of mouse was recorded by a video camera (Sony CCD-IRIS model) that was placed above the arena and was connected to a VHS videocassette recorder (Panasonic AG-5700 model). The video tracking program Etho-Vision (Noldus Information Technologies, Wageningen, Netherlands) was used to record the total distance traveled in 16 central grids and the total duration that the mouse stayed in the center grids. If the mouse reared (forelimbs upright against the wall) or groomed for longer than 3 s, these behaviors were also recorded.
Learning and memory performance of the adolescent mice were tested using MWM task. The water maze consists of a galvanized white circular water tank (90 cm diameter, 50 cm height) filled with clear tap water (22 ± 1°C) to a depth of 15 cm. A 6 cm × 6 cm invisible escape platform was placed 1 cm below the water level and 13 cm from the rim. The water was made opaque by adding white non-toxic paint. The MWM task was performed as previously described (Zhang et al., 2014). Briefly, each mouse underwent daily sessions of four trials for four consecutive days. For each trial, the mouse was released into the water facing the wall at one of the four standard start locations selected at random (N, S, W, or E). When it succeeded in locating the platform, the mouse was allowed to remain on the platform for 15 s. If the mouse failed to find the platform within 60 s, it was assisted by the experimenter and allowed to remain on the platform for the same amount of time. The interval between trials was 20 min. A probe trial was performed 24 h after the last training session. For the probe trial, the platform was removed from the tank and the mouse was allowed to swim freely for 60 s. The experimenters conducted the trials while blind to the group assignments. All of the trials were videotaped using a camera located 2 m above the water surface. The time required to locate the hidden platform in the training trial (latency) and the time spent in as well as the number of entries into the target quadrant during the probe trial were recorded.
Superoxide dismutase (SOD) and malondialdehyde (MDA) were measured to assess oxidative stress and damage. Six samples of cerebral cortex were dissected from adolescents 24 h after the last behavioral test. Tissue samples were homogenized in 100× volumes of ice-cold 0.1 mol/L phosphate buffer solution (containing 300 mmol/L sucrose and 0.1 mmol/L ethylenediamine tetra acetic acid and adjusted to pH 7.8) to yield a 1% (w/v) homogenate. This was then centrifuged at 4°C at 10,000 ×g for 10 min. Protein concentration was determined by the dye binding in a Bradford assay using bovine serum albumin as a standard. SOD and MDA contents were assayed using commercially available kits according to the manufacturer’s protocol (Nanjing Jiancheng Bioengineering Institute, China).
Choline acetyltransferase (ChAT) and total cholinesterase (TChE) were assessed as potential mechanism underlying cognitive dysfunction in adolescent mice, since ChAT and TChE have been shown to be directly associated with acetylcholinergic content in rodents (Tayebati et al., 2004). Biochemical tests for cholinergic enzymes were conducted in adolescents 24 h after the last behavioral test. Half of the cerebral cortex from each mouse was dissected and homogenized with ice-cold 150 mM KCl. The homogenate (10% w/v) was centrifuged at 10,000 ×g for 15 min, and the supernatant was used for analysis. TChE activity was measured by the modified Ellman’s colorimetric method (Ellman et al., 1961; Ingkaninan et al., 2000), and ChAT activity was measured using the chemiluminescence assay described previously (Vijayaraghavan et al., 2013).
For quantitative data, the data are expressed as mean ± standard deviation ( ± s). One-way analysis of variance (ANOVA) is used to test the difference between means of the four subgroups of a variable (multiple testing) following by Dunnett’s post hoc test to evaluate the statistical difference between the experimental groups and the controls, and Student–Newman–Keuls (SNK) test to assess the difference between experimental groups. For categorical data, the data are expressed as numbers of categorical counting, and the difference among groups and between any other two groups is analyzed by χ2 test. All data were analyzed with SPSS 12.0 (SPSS, Chicago, IL, United States). A p-value less than 0.05 is considered to be statistically significant.
The primary characterization of particles was observed under TEM (Figure 2). Since NPs often form agglomerates in a solution, the size of the NPs and their agglomerates were estimated by Image-pro plus software. The TEM images in Figure 2 display AlNP at 13 nm (Figure 2A), 50 nm (Figure 2B), and bulk-Al (Figure 2C) size in both agglomerated and non-agglomerated states. The particle size was distributed over a wide range due to differences in characteristics such as aggregation, mean diameters, and zeta potentials (Table 1).
FIGURE 2. Morphology of AlNP and bulk-Al under a transmission electron microscope (TEM). To observe the morphology of and measure their particle size, AlNP and bulk-Al particles were characterized under a TEM. (A) AlNP of 13 nm particle size (bar = 100 nm). (B) AlNP of 50 nm particle size (bar = 100 nm). (C) Bulk-Al of 10 μm particle size (bar = 10 μm).
The consumption of food and water appeared normal for all treated female mice. However, the newborn pups delivered by AlNP-treated mice had significantly lower BW on PD1 compared with controls [F(3,188) = 30.14, p < 0.0001; Figure 3A]. Al contents in the hippocampus of PD1 pups delivered by AlNP-treated mice were significantly higher compared with those by controls [F(3,44)= 106.1, p < 0.0001]. There was a significant decrease in the birth weight of pups delivered by female mice treated with both 50 and 13 nm AlNP compared with those treated with bulk-Al and normal saline controls (bulk-Al vs. control, p = 0.0109; 13 and 50 nm AlNP vs. bulk-Al and control, p < 0.0001; 13 vs. 50 nm AlNP, p < 0.0001; Figure 3B). Weight gain of pups in bulk-Al and AlNP groups significantly increased from PD4 to PD7 to PD14 and to PD28 [F(4,187)= 116.9, p < 0.0001], but comparison of the actual body weight of pups at various weight days had no significant difference among groups [F(3,188)= 0.5364, p = 0.6587; Figure 3C].
FIGURE 3. Body weight (BW) and Al contents in the newborn pups delivered by AlNP-treated female mice. To evaluate the accumulation of Al in the newborn brains, Al contents were measured using graphite furnace atomic absorption spectrometry (GFAAS). BW of newborns was used to assess the general impact of AlNP and bulk-Al particles on their next generations. The BW of PD1 newborns was significantly lower in AlNP-treated groups compared with the controls (A). Al contents in the hippocampus of PD1 newborns significantly increased in bulk-Al vs. control, AlNP vs. bulk-Al and control, and 13 vs. 50 nm AlNP-treated mice (B). The difference of BW in treated mice was not significant among groups though BW within each group increased by days (C). a: p < 0.05, compared with controls; b: p < 0.05, compared with bulk-Al group; and c: p < 0.05, compared with 50 nm AlNP group.
Ear pinna structure, appearance of teeth, and eye opening of the newborn pups were recorded in Table 2. The time needed for ear pinna opening among groups was significantly different [F(3,188)= 17.69, p < 0.0001]; ear pinna opening was delayed significantly in bulk-Al and AlNP (13 and 50 nm) groups compared with controls (p < 0.01). Further, AlNP (13 or 50 nm) groups were significantly delayed compared to bulk-Al group (p < 0.01). Similarly, the time needed for teeth appearance among groups was significantly different [F(3,188)= 85.64, p < 0.0001]; teeth appearance was delayed significantly in bulk-Al and AlNP (13 or 50 nm) groups compared with controls (p < 0.01). Furthermore, pups delivered by female mice treated with 13 nm AlNP were significantly delayed compared with bulk-Al and 50 nm AlNP groups (p < 0.01). Additionally, the time needed for eye opening was significantly different among groups [F(3,188)= 7.42, p < 0.0001], which was significantly delayed in AlNP (13 or 50 nm) groups compared with control and bulk-Al groups (p < 0.01), the 13 nm AlNP group displayed a greater delay in eye opening compared with 50 nm AlNP group (p < 0.01).
Neuromotor maturation of pups was evaluated and the results are shown in Table 3. The number of righting reflex positive pups was significantly different among groups at PD4 (χ2= 2.856, p = 0.048), but no significance was found at PD7 (χ2= 1.644, p = 0.067). Compared with controls, the 13 nm AlNP group demonstrated lower righting reflexes (p < 0.05), while no significance was found in bulk-Al and 50 nm AlNP groups (p > 0.05). The number of cliff avoidance positive pups was significantly different among treated groups on PD4 (χ2= 4.397, p = 0.028) and PD7 (χ2= 6.12, p < 0.001). Compared with controls, the bulk-Al (p < 0.01, p < 0.05), 50 nm (p < 0.01, p < 0.05), and 13 nm (p < 0.05, p < 0.05) AlNP groups had fewer cliff avoidance positive mice on PD4 and PD7.
The effect of AlNP treatment on neuromotor development was evaluated on PD12 and PD14 by testing forelimb grip strength and endurance (Table 4). Effects of particle sizes were observed with significant differences in forelimb grip strength among groups [F(3,188)= 36.59, p < 0.0001]. On PD12, 50 and 13 nm AlNP groups had significantly lower forelimb grip strength compared with control and bulk-Al groups (p < 0.01), but no significant difference was found between 50 and 13 nm AlNP groups (p > 0.05). On PD14, bulk-Al, 50 nm, and 13 nm AlNP-treated mice had significantly lower forelimb grip strength compared with controls (p < 0.01). Furthermore, the 13 nm AlNP group demonstrated significantly lower forelimb grip strength compared with bulk-Al and 50 nm AlNP groups (p < 0.01), but no significant difference between bulk-Al and 50 nm AlNP groups was detected (p > 0.05).
Neurobehavioral performance was evaluated by anxiety, learning, and memory in 1-month-old adolescents by Openfield and MWM tests (Figure 4). For distance in the central grids of Openfield test (Figure 4A), significant difference was found among groups [F(3,44) = 4.554, p = 0.0061]. The distance in central grids traveled by pups in the AlNP (50 and 13 nm) groups was significantly higher than that in control and bulk-Al treated groups (p = 0.047 and 0.0116), but no statistical significance was found between bulk-Al and controls (p = 0.8425). For duration in central grid (Figure 4B), no significant difference was found among groups [F(3,44) = 0.4194, p = 0.7396]. For grooming times (Figure 4C), a significant difference was found among groups [F(3,44) = 35.83, p < 0.0001], though no significant difference was found between bulk-Al treated group and controls (p = 0.9891). However, there was a significant decrease in the 50 and 13 nm AlNP-treated groups (both p < 0.0001) compared with bulk-Al and controls. In addition, no significant difference was found between 50 and 13 nm AlNP-treated groups (p = 0.8607). For rearing times (Figure 4D), a significant difference was found among groups [F(3,44)= 27.70, p < 0.0001], though no significant difference was found between bulk-Al treated group and controls (p = 0.9329). However, there was a significant decrease in rearing times of 50 and 13 nm AlNP-treated groups (both p < 0.0001) when compared with bulk-Al group and controls, but no significance between the AlNP-treated groups (50 and 13 nm; p = 0.1816).
FIGURE 4. Anxiety scale of the adolescents delivered by AlNP-treated female mice. Openfield test was used to determine anxiety in the adolescents delivered by AlNP-treated female mice. The results are shown as distance in central grid (A), duration in central grid (B), grooming times (C), and rearing times (D). a: p < 0.05, compared with controls and b: p < 0.05, compared with bulk-Al group.
Morris water maze task was used to determine learning and memory performance in adolescents delivered by AlNP-treated female mice (Figure 5). The adolescents demonstrated a significant decrease (Figure 5A) in learning performance within 5-day training [F(4,235)= 42.93, p < 0.0001] with particle-size effects among groups [F(4,235)= 4.452, p = 0.0044]. No significant difference was found between bulk-Al treaded group and controls (p = 0.6223), but a significant decrease in learning was found in 50 (p = 0.0410) and 13 nm (p = 0.0053) AlNP-treated groups compared with controls. However, no significant difference was found between bulk-Al group and 50 (p = 0.4691) and 13 nm (p = 0.1473) AlNP-treated groups, nor between the 50 and 13 nm AlNP-treated groups (p = 0.9064).
FIGURE 5. Learning and memory performance in the adolescents delivered by AlNP-treated female mice. Morris water maze was used to assess learning and memory performance in the offspring delivered by AlNP-treated female mice. Learning (A) and memory (B,C) performance of the offspring delivered by AlNP-treated female mice was displayed. a: p < 0.01 compared with controls and b: p < 0.01, compared with bulk-Al group.
Memory profiles also differed in the amount of time spent in the target arm (Figure 5B) among groups [F(3,44)= 14.92, p < 0.0001]. No significant difference was found compared bulk-Al (p = 0.0942) and 50 nm AlNP-treated groups (p = 0.4299), but adolescents in 13 nm AlNP-treated group spent less time in the target arm (p = 0.0006) compared with controls. Compared with bulk-Al, adolescents in 50 (p = 0.0.0014) and 13 nm (p < 0.0001) AlNP-treated groups spent less time in target arm but no significance was found between 50 and 13 nm AlNP-treated groups (p = 0.0529). There was also a significant decrease in the number of entries into the target arm (Figure 5C) among groups [F(3,44) = 60.29, p < 0.0001]. Compared with controls, adolescents in bulk-Al (p < 0.0001) and 50 and 13 nm AlNP-treated groups (both p < 0.0001) all had less entries to the target arm, while those in 50 and 13 nm AlNP-treated groups had much less entries compared with that in bulk-Al group (Figure 5, both p < 0.0001), though no significance was found between 50 and 13 nm AlNP-treated groups (p = 0.9997).
Superoxide dismutase activity and MDA products in the cerebral cortex were measured to evaluate oxidative stress in adolescents delivered by AlNP-treated female mice (Figure 6). SOD activity was significantly different among groups [Figure 6A, F(3,44) = 38.5, p < 0.0001]. Compared to controls, SOD activity in bulk-Al and AlNP (50 and 13 nm) treated groups was significantly lower (p < 0.0001 for all). No significance was found between bulk-Al and 50 nm AlNP-treated groups (p = 0.4365), while SOD activity in 13 nm AlNP-treated mice was significantly lower compared with those in bulk-Al and 50 nm AlNP-treated groups (p = 0.0387 and 0.0008). For MDA products, though a significant difference in MDA products in the cerebral cortex among groups was found [Figure 6B, F(3,44)= 3.121, p = 0.0417], no significant difference (p > 0.05) was found between any two groups (p > 0.05).
FIGURE 6. Oxidative stress in the cerebral cortex of the adolescents delivered by AlNP-treated female mice. To explore the mechanism involved in the neurobehavioral deficits, oxidative stress levels in the cerebral cortex were quantified. SOD activity (A) and MDA products (B) as measurements of oxidative stress were examined. There was a significant difference in both SOD activity and MDA products among groups. a: p < 0.05, compared with controls; b: p < 0.05, compared with bulk-Al group; and c: p < 0.05, compared with 50 nm AlNP group.
Neurotransmitter enzyme levels of ChAT and TChE were measured in the cerebral cortex of adolescents delivered by AlNP-treated female mice (Figure 7). ChAT activity was significantly different among groups [Figure 7A, F(3,44) = 368.3, p < 0.0001]. Compared to controls, ChAT activity in bulk-Al and AlNP-treated groups (50 and 13 nm) was significantly decreased (p < 0.0001 for all). Although ChAT activity in bulk-Al vs. 50 nm AlNP-treated mice did not differ (p = 0.9653), mice in 13 nm AlNP-treated group had significantly lower levels of ChAT compared to bulk-Al and 50 nm AlNP-treated mice (p < 0.0001 both). For TchE activity, a significant difference among groups was found [Figure 7B, F(3,44) = 34.6, p < 0.0001]. Though no significance was found between bulk-Al treated group and controls (p = 0.5440), the TChE activity in AlNP (50 and 13 nm) treated groups was significantly higher compared with bulk-Al treated group and controls (p = 0.0001 and p < 0.0001). There was no significance found between 50 and 13 nm AlNP groups on TChE activity (p = 0.1460).
FIGURE 7. Neurotransmitter levels in cerebral cortex of adolescents delivered by AlNP-treated female mice. To explore the mechanism involved in the neurobehavioral deficits, neurotransmitter levels in the cerebral cortex were quantified. Choline acetyltransferase (A) and total cholinesterase activities (B) were examined. a: p < 0.05, compared with controls; b: p < 0.05, compared with bulk-Al group; and c: p < 0.05, compared with 50 nm AlNP group.
The developing nervous system is especially vulnerable to toxic chemical exposures (Rodier, 1995; Bondy and Campbell, 2005), and major windows of developmental vulnerability occur in utero and during infancy and early childhood (Rice and Barone, 2000). During these sensitive life stages, exposure to chemicals, even at low concentrations, can cause permanent brain injury that can manifest as functional impairments or disease at any point in the human lifespan—from early infancy to old age. With the rapid development of nanotechnology, the likelihood of environmental exposure to NPs has significantly increased either actively or passively (Service, 2004). Although BBB and blood–placental barrier can provide some protection against the entry of external products into the CNS during fetal development and early infancy, exposure to untested NPs should not be presumed safe. As new products with distinguished chemical and physical characteristics differ from their bulk counterparts, NPs in existing use and all new products must therefore be tested for developmental neurotoxicity (Grandjean and Landrigan, 2014).
The placenta is a multifunctional organ constituting the barrier between maternal and fetal tissues. Research has shown that NPs can cross the placental barrier (Keelan, 2011; Muoth et al., 2016), and there is increasing evidence that the extent of transfer is dependent on particle characteristics and functionalization (Yang et al., 2012; Tian et al., 2013). It is reported that exposure of pregnant mice to different NPs induces pregnancy complications or damage to the fetus (e.g., to silver NPs, titanium dioxide, silicon dioxide, and carbon nanotubes) (Shimizu et al., 2009; Hougaard et al., 2010; Pietroiusti et al., 2011; Yamashita et al., 2011; Campagnolo et al., 2013, 2017; Huang et al., 2014; Qi et al., 2014). Given the high susceptibility of the CNS to external insults during the developmental period, we address specific concerns on neurodevelopmental toxicity effects of AlNP in the present study.
Since developmental events are intertwined in the reproductive process, effects on developmental toxicity may be detected in developmental combined reproductive studies. Evaluation of the developmental effects in the present study is based on the National Toxicity Program Criteria from the U.S. Department of Health and Human Services for Levels of Evidence for Developmental Toxicity (Foster, 2009). A standard Reproductive/Developmental Toxicity Screening Assay (Organization for Economic Cooperation and Development [OECD], 2016) is used in female mice dosed with AlNP for 2 weeks prior to mating, during mating, and throughout gestation until the pups were born. Compared with developmental toxicological experiments that treatments are designed to start from the first day of mating, our experimental design of treatment starting from 2 weeks before mating considers the potential toxicity in utero to the female and toxicological consequences in the offspring.
First, the BW of newborn developing pups delivered by AlNP-treated female mice decreased significantly while Al contents in the hippocampus of PD1 newborns were increased (Figure 3). It was found that BW of the newborns on PD1 was significantly lower in pups delivered by AlNP-treated female mice. Moreover, PD1 newborns in AlNP-treated groups had significant higher contents of Al element in the hippocampus, indicating that AlNP can enter the hippocampus of the embryos and result in greater neuronal damage due to known Al neurotoxicity. Furthermore, neurodevelopmental deficits persisted into adolescents even though their lower BW was reconciled on the following PD7–PD28.
Second, AlNP administration via nasal drip in pregnant female mice affected the physical developmental landmarks in their offspring (Table 2). Development of morphological indicators including ear pinna structure, appearance of teeth, and eye opening of the newborn pups delivered by AlNP-treated female mice was significantly delayed compared to the controls. In particular, the smaller sized AlNP (13 nm) caused a greater delay in developmental characteristics compared to 50 nm AlNP-treated group, suggesting possible interference in molecular pathways involved in physical growth and development.
Third, AlNP suppressed CNS maturation in the offspring. Normal neuromotor responses, such as righting reflex and cliff avoidance, were reduced significantly (Table 3) and the endurance test demonstrated worse neuromuscular skeletal development in the offspring delivered by AlNP-treated female mice (Table 4). These findings suggest AlNP-induced defects in maturation of the offspring’s CNS when AlNP are prenatally exposed to the developing fetus in utero. It is possible that continuous exposure to AlNP during fetal development in utero has a cumulative toxic effect in the developing brains. Persisting neurodevelopmental deficits are indicative of potentially irreversible neural damage or improper neurodevelopment.
Finally, adolescent offspring in AlNP groups demonstrated greater anxiety-like behavior (Figure 4) and decreased learning and memory performance (Figure 5), indicating that prenatal exposure of AlNP in female mice during pregnancy can have long-lasting changes in neurocognitive function of the offspring which continues through adolescence. Evidence of elevated oxidative stress (Figure 6) and decreased neurotransmitter levels (Figure 7) in cerebral cortex of the adolescents suggests that prenatal AlNP exposure can induce brain dysfunction in adolescents by promoting oxidative stress accumulation while suppressing availability of neurotransmitters. In fact, studies have demonstrated repeatedly that AlNP can induce ROS and reduce neurotransmitter content in brain (Sanchez-Iglesias et al., 2009; Fu et al., 2014; Simko and Mattsson, 2014; Shah et al., 2015).
Therefore, it is suggested in the present study that AlNP exposure of female mice during pregnancy can jeopardize the proper development of the brain in offspring, which is one of the most susceptible target organs during early perinatal period. It also appears that AlNP-induced neurodevelopmental deficits can persist into adulthood, as shown by altered anxiety-like behavior and cognitive function. An important line of evidence in this study was that oxidative stress and neurotransmitter enzyme levels in offspring were altered by AlNP exposure of female mice during pregnancy, although further work is necessary to determine whether these findings precede neurobehavioral outcomes and lead to neurodevelopmental deficits or whether altered neurophysiology brought by perinatal exposure to AlNP leads to elevated oxidative stress and reduced neurotransmitter contents. Further studies to examine the relationship between AlNP and neurodevelopment are needed to investigate associated mechanisms and potential therapeutic strategies during the perinatal period, before permanent changes to the brain have occurred.
QZ takes charge of experimental design, implementation, results, discussion, manuscript revision, and agrees to be accountable for all aspects of the work. YD, HL, and XW take charge of experimental data acquisition, analysis, manuscript drafting, and agree to be accountable for the animal experiments. KH takes charge of experimental data interpretation, manuscript drafting, and agrees to be accountable for the data interpretation. FG takes charge of experimental data acquisition of nanoparticles, analysis, manuscript revision, and agrees to be accountable for the nanoparticle characteristics experiments. TM and JD take charge of experimental data interpretation, manuscript drafting and revision, and agree to be accountable for the accuracy of interpreting and discussion of the experiments. QN takes charge of experimental design, revision of the manuscript, and agrees to be accountable for the aspects of experiments design and concepts. All authors approved the final version of the manuscript to be published.
This study was supported by the National Nature Science Foundation of China (Grant Nos. 81241098 and 81110108003) and the Scientific and Technological Programs in Shanxi Province (Grant No. 20130313020-1).
The authors declare that the research was conducted in the absence of any commercial or financial relationships that could be construed as a potential conflict of interest.
Abu-Taweel, G. M., Ajarem, J. S., and Ahmad, M. (2012). Neurobehavioral toxic effects of perinatal oral exposure to aluminum on the developmental motor reflexes, learning, memory and brain neurotransmitters of mice offspring. Pharmacol. Biochem. Behav. 101, 49–56. doi: 10.1016/j.pbb.2011.11.003
Almeida, J. P., Chen, A. L., Foster, A., and Drezek, R. (2011). In vivo biodistribution of nanoparticles. Nanomedicine 6, 815–835. doi: 10.2217/nnm.11.79
Andersen, C. P., King, G., Plocher, M., Storm, M., Pokhrel, L. R., Johnson, M. G., et al. (2016). Germination and early plant development of ten plant species exposed to titanium dioxide and cerium oxide nanoparticles. Environ. Toxicol. Chem. 35, 2223–2229. doi: 10.1002/etc.3374
Bakand, S., and Hayes, A. (2016). Toxicological considerations, toxicity assessment, and risk management of inhaled nanoparticles. Int. J. Mol. Sci. 17:E929. doi: 10.3390/ijms17060929
Balasubramanyam, A., Sailaja, N., Mahboob, M., Rahman, M. F., Hussain, S. M., and Grover, P. (2009a). In vivo genotoxicity assessment of aluminium oxide nanomaterials in rat peripheral blood cells using the comet assay and micronucleus test. Mutagenesis 24, 245–251. doi: 10.1093/mutage/gep003
Balasubramanyam, A., Sailaja, N., Mahboob, M., Rahman, M. F., Misra, S., Hussain, S. M., et al. (2009b). Evaluation of genotoxic effects of oral exposure to aluminum oxide nanomaterials in rat bone marrow. Mutat. Res. 676, 41–47. doi: 10.1016/j.mrgentox.2009.03.004
Bondy, S. C., and Campbell, A. (2005). Developmental neurotoxicology. J. Neurosci. Res. 81, 605–612. doi: 10.1002/jnr.20589
Bonfanti, P., Moschini, E., Saibene, M., Bacchetta, R., Rettighieri, L., Calabri, L., et al. (2015). Do nanoparticle physico-chemical properties and developmental exposure window influence nano ZnO Embryotoxicity in Xenopus laevis? Int. J. Environ. Res. Public Health 12, 8828–8848. doi: 10.3390/ijerph120808828
Braydich-Stolle, L. K., Speshock, J. L., Castle, A., Smith, M., Murdock, R. C., and Hussain, S. M. (2010). Nanosized aluminum altered immune function. ACS Nano 4, 3661–3670. doi: 10.1021/nn9016789
Brundo, M. V., Pecoraro, R., Marino, F., Salvaggio, A., Tibullo, D., Saccone, S., et al. (2016). Toxicity evaluation of new engineered nanomaterials in zebrafish. Front. Physiol. 7:130. doi: 10.3389/fphys.2016.00130
Buccheri, M. A., D’Angelo, D., Scalese, S., Spano, S. F., Filice, S., Fazio, E., et al. (2016). Modification of graphene oxide by laser irradiation: a new route to enhance antibacterial activity. Nanotechnology 27:245704. doi: 10.1088/0957-4484/27/24/245704
Campagnolo, L., Massimiani, M., Palmieri, G., Bernardini, R., Sacchetti, C., Bergamaschi, A., et al. (2013). Biodistribution and toxicity of pegylated single wall carbon nanotubes in pregnant mice. Part. Fibre Toxicol. 10:21. doi: 10.1186/1743-8977-10-21
Campagnolo, L., Massimiani, M., Vecchione, L., Piccirilli, D., Toschi, N., Magrini, A., et al. (2017). Silver nanoparticles inhaled during pregnancy reach and affect the placenta and the foetus. Nanotoxicology 11, 687–698. doi: 10.1080/17435390.2017.1343875
Charehsaz, M., Hougaard, K. S., Sipahi, H., Ekici, A. I., Kaspar, C., Culha, M., et al. (2016). Effects of developmental exposure to silver in ionic and nanoparticle form: a study in rats. Daru 24:24. doi: 10.1186/s40199-016-0162-9
Chen, L., Yokel, R. A., Hennig, B., and Toborek, M. (2008). Manufactured aluminum oxide nanoparticles decrease expression of tight junction proteins in brain vasculature. J. Neuroimmune Pharmacol. 3, 286–295. doi: 10.1007/s11481-008-9131-5
Chen, L., Zhang, B., and Toborek, M. (2013). Autophagy is involved in nanoalumina-induced cerebrovascular toxicity. Nanomedicine 9, 212–221. doi: 10.1016/j.nano.2012.05.017
Cranmer, J. M., Wilkins, J. D., Cannon, D. J., and Smith, L. (1986). Fetal-placental-maternal uptake of aluminum in mice following gestational exposure: effect of dose and route of administration. Neurotoxicology 7, 601–608.
Dey, S., Bakthavatchalu, V., Tseng, M. T., Wu, P., Florence, R. L., Grulke, E. A., et al. (2008). Interactions between SIRT1 and AP-1 reveal a mechanistic insight into the growth promoting properties of alumina (Al2O3) nanoparticles in mouse skin epithelial cells. Carcinogenesis 29, 1920–1929. doi: 10.1093/carcin/bgn175
Di Bona, K. R., Xu, Y., Gray, M., Fair, D., Hayles, H., Milad, L., et al. (2015). Short- and long-term effects of prenatal exposure to iron oxide nanoparticles: influence of surface charge and dose on developmental and reproductive toxicity. Int. J. Mol. Sci. 16, 30251–30268. doi: 10.3390/ijms161226231
Dong, E., Wang, Y., Yang, S. T., Yuan, Y., Nie, H., Chang, Y., et al. (2011). Toxicity of nano gamma alumina to neural stem cells. J. Nanosci. Nanotechnol. 11, 7848–7856. doi: 10.1166/jnn.2011.4748
Ellman, G. L., Courtney, K. D., Andres, V. Jr., and Feather-Stone, R. M. (1961). A new and rapid colorimetric determination of acetylcholinesterase activity. Biochem. Pharmacol. 7, 88–95. doi: 10.1016/0006-2952(61)90145-9
Ema, M., Okuda, H., Gamo, M., and Honda, K. (2017). A review of reproductive and developmental toxicity of silver nanoparticles in laboratory animals. Reprod. Toxicol. 67, 149–164. doi: 10.1016/j.reprotox.2017.01.005
Exley, C., and House, E. (2011). Aluminium in the human brain. Monatsh. Chem. 142, 357–363. doi: 10.1007/s00706-010-0417-y
Foster, P. M. (2009). Explanation of Levels of Evidence for Developmental Toxicity. Research Triangle Park, NC: National Toxicology Program.
Fu, B., Zhang, J., Zhang, X., Zhang, C., Li, Y., Zhang, Y., et al. (2014). Alpha-lipoic acid upregulates SIRT1-dependent PGC-1α expression and protects mouse brain against focal ischemia. Neuroscience 281, 251–257. doi: 10.1016/j.neuroscience.2014.09.058
Ganesan, S., Anaimalai Thirumurthi, N., Raghunath, A., Vijayakumar, S., and Perumal, E. (2016). Acute and sub-lethal exposure to copper oxide nanoparticles causes oxidative stress and teratogenicity in zebrafish embryos. J. Appl. Toxicol. 36, 554–567. doi: 10.1002/jat.3224
Ghobadian, M., Nabiuni, M., Parivar, K., Fathi, M., and Pazooki, J. (2015). Toxic effects of magnesium oxide nanoparticles on early developmental and larval stages of zebrafish (Danio rerio). Ecotoxicol. Environ. Saf. 122, 260–267. doi: 10.1016/j.ecoenv.2015.08.009
Gorsky, J. E., and Dietz, A. A. (1978). Determination of aluminum in biological samples by atomic absorption spectrophotometry with a graphite furnace. Clin. Chem. 24, 1485–1490.
Grandjean, P., and Landrigan, P. J. (2014). Neurobehavioural effects of developmental toxicity. Lancet Neurol. 13, 330–338. doi: 10.1016/S1474-4422(13)70278-3
Hougaard, K. S., Jackson, P., Jensen, K. A., Sloth, J. J., Loschner, K., Larsen, E. H., et al. (2010). Effects of prenatal exposure to surface-coated nanosized titanium dioxide (UV-Titan). A study in mice. Part. Fibre Toxicol. 7:16. doi: 10.1186/1743-8977-7-16
Huang, X., Zhang, F., Sun, X., Choi, K. Y., Niu, G., Zhang, G., et al. (2014). The genotype-dependent influence of functionalized multiwalled carbon nanotubes on fetal development. Biomaterials 35, 856–865. doi: 10.1016/j.biomaterials.2013.10.027
Ingkaninan, K., de Best, C. M., van der Heijden, R., Hofte, A. J., Karabatak, B., Irth, H., et al. (2000). High-performance liquid chromatography with on-line coupled UV, mass spectrometric and biochemical detection for identification of acetylcholinesterase inhibitors from natural products. J. Chromatogr. A 872, 61–73. doi: 10.1016/S0021-9673(99)01292-3
Kagan, V. E., Bayir, H., and Shvedova, A. A. (2005). Nanomedicine and nanotoxicology: two sides of the same coin. Nanomedicine 1, 313–316. doi: 10.1016/j.nano.2005.10.003
Keelan, J. A. (2011). Nanotoxicology: nanoparticles versus the placenta. Nat. Nanotechnol. 6, 263–264. doi: 10.1038/nnano.2011.65
Lee, J., Yu, W. J., Song, J., Sung, C., Jeong, E. J., Han, J. S., et al. (2016). Developmental toxicity of intravenously injected zinc oxide nanoparticles in rats. Arch. Pharm. Res. 39, 1682–1692. doi: 10.1007/s12272-016-0767-z
Li, X., Zhang, C., Zhang, X., Wang, S., Meng, Q., Wu, S., et al. (2016). An acetyl-L-carnitine switch on mitochondrial dysfunction and rescue in the metabolomics study on aluminum oxide nanoparticles. Part. Fibre Toxicol. 13:4. doi: 10.1186/s12989-016-0115-y
Maisano, M., Cappello, T., Catanese, E., Vitale, V., Natalotto, A., Giannetto, A., et al. (2015). Developmental abnormalities and neurotoxicological effects of CuO NPs on the black sea urchin Arbacia lixula by embryotoxicity assay. Mar. Environ. Res. 111, 121–127. doi: 10.1016/j.marenvres.2015.05.010
Morsy, G. M., Abou El-Ala, K. S., and Ali, A. A. (2016a). Studies on fate and toxicity of nanoalumina in male albino rats: oxidative stress in the brain, liver and kidney. Toxicol. Ind. Health 32, 200–214. doi: 10.1177/0748233713498462
Morsy, G. M., El-Ala, K. S., and Ali, A. A. (2016b). Studies on fate and toxicity of nanoalumina in male albino rats: lethality, bioaccumulation and genotoxicity. Toxicol. Ind. Health 32, 344–359. doi: 10.1177/0748233713498449
Muoth, C., Aengenheister, L., Kucki, M., Wick, P., and Buerki-Thurnherr, T. (2016). Nanoparticle transport across the placental barrier: pushing the field forward! Nanomedicine 11, 941–957. doi: 10.2217/nnm-2015-0012
Needham, L. L., Grandjean, P., Heinzow, B., Jorgensen, P. J., Nielsen, F., Patterson, D. G., et al. (2011). Partition of environmental chemicals between maternal and fetal blood and tissues. Environ. Sci. Technol. 45, 1121–1126. doi: 10.1021/es1019614
Nel, A., Xia, T., Madler, L., and Li, N. (2006). Toxic potential of materials at the nanolevel. Science 311, 622–627. doi: 10.1126/science.1114397
Organization for Economic Cooperation and Development [OECD] (ed.). (2016). “Reproduction/developmental toxicity screening test,” in OECD Guideline for Testing of Chemicals. Paris: OECD.
Oesterling, E., Chopra, N., Gavalas, V., Arzuaga, X., Lim, E. J., Sultana, R., et al. (2008). Alumina nanoparticles induce expression of endothelial cell adhesion molecules. Toxicol. Lett. 178, 160–166. doi: 10.1016/j.toxlet.2008.03.011
Parivar, K., Hayati Rudbari, N., Khanbabaee, R., and Khaleghi, M. (2015). The effect of nano-titanium dioxide on limb bud development of NMRI mouse embryo In Vivo. Cell J. 17, 296–303.
Pecoraro, R., D’Angelo, D., Filice, S., Scalese, S., Capparucci, F., Marino, F., et al. (2017a). Toxicity evaluation of graphene oxide and titania loaded nafion membranes in zebrafish. Front. Physiol. 8:1039. doi: 10.3389/fphys.2017.01039
Pecoraro, R., Marino, F., Salvaggio, A., Capparucci, F., Di Caro, G., Iaria, C., et al. (2017b). Evaluation of chronic nanosilver toxicity to adult zebrafish. Front. Physiol. 8:1011. doi: 10.3389/fphys.2017.01011
Pietroiusti, A., Massimiani, M., Fenoglio, I., Colonna, M., Valentini, F., Palleschi, G., et al. (2011). Low doses of pristine and oxidized single-wall carbon nanotubes affect mammalian embryonic development. ACS Nano 5, 4624–4633. doi: 10.1021/nn200372g
Poirier, J., Semple, H., Davies, J., Lapointe, R., Dziwenka, M., Hiltz, M., et al. (2011). Double-blind, vehicle-controlled randomized twelve-month neurodevelopmental toxicity study of common aluminum salts in the rat. Neuroscience 193, 338–362. doi: 10.1016/j.neuroscience.2011.05.008
Priest, N. D. (2004). The biological behaviour and bioavailability of aluminium in man, with special reference to studies employing aluminium-26 as a tracer: review and study update. J. Environ. Monit. 6, 375–403. doi: 10.1039/b314329p
Qi, W., Bi, J., Zhang, X., Wang, J., Wang, J., Liu, P., et al. (2014). Damaging effects of multi-walled carbon nanotubes on pregnant mice with different pregnancy times. Sci. Rep. 4:4352. doi: 10.1038/srep04352
Rice, D., and Barone, S. Jr. (2000). Critical periods of vulnerability for the developing nervous system: evidence from humans and animal models. Environ. Health Perspect. 108(Suppl. 3), 511–533. doi: 10.1289/ehp.00108s3511
Rodier, P. M. (1995). Developing brain as a target of toxicity. Environ. Health Perspect. 103(Suppl. 6), 73–76. doi: 10.2307/3432351
Rollerova, E., Tulinska, J., Liskova, A., Kuricova, M., Kovriznych, J., Mlynarcikova, A., et al. (2015). Titanium dioxide nanoparticles: some aspects of toxicity/focus on the development. Endocr. Regul. 49, 97–112. doi: 10.4149/endo_2015_02_97
Sanchez-Iglesias, S., Mendez-Alvarez, E., Iglesias-Gonzalez, J., Munoz-Patino, A., Sanchez-Sellero, I., Labandeira-Garcia, J. L., et al. (2009). Brain oxidative stress and selective behaviour of aluminium in specific areas of rat brain: potential effects in a 6-OHDA-induced model of Parkinson’s disease. J. Neurochem. 109, 879–888. doi: 10.1111/j.1471-4159.2009.06019.x
Scuderi, V., Impellizzeri, G., Romano, L., Scuderi, M., Brundo, M. V., Bergum, K., et al. (2014). An enhanced photocatalytic response of nanometric TiO2 wrapping of Au nanoparticles for eco-friendly water applications. Nanoscale 6, 11189–11195. doi: 10.1039/c4nr02820a
Service, R. F. (2004). Nanotoxicology: nanotechnology grows up. Science 304, 1732–1734. doi: 10.1126/science.304.5678.1732
Shah, S. A., Yoon, G. H., Ahmad, A., Ullah, F., Ul Amin, F., and Kim, M. O. (2015). Nanoscale-alumina induces oxidative stress and accelerates amyloid beta (Abeta) production in ICR female mice. Nanoscale 7, 15225–15237. doi: 10.1039/c5nr03598h
Shimizu, M., Tainaka, H., Oba, T., Mizuo, K., Umezawa, M., and Takeda, K. (2009). Maternal exposure to nanoparticulate titanium dioxide during the prenatal period alters gene expression related to brain development in the mouse. Part. Fibre Toxicol. 6:20. doi: 10.1186/1743-8977-6-20
Shrivastava, R., Raza, S., Yadav, A., Kushwaha, P., and Flora, S. J. (2014). Effects of sub-acute exposure to TiO2, ZnO and Al2O3 nanoparticles on oxidative stress and histological changes in mouse liver and brain. Drug Chem. Toxicol. 37, 336–347. doi: 10.3109/01480545.2013.866134
Simko, M., and Mattsson, M. O. (2014). Interactions between nanosized materials and the brain. Curr. Med. Chem. 21, 4200–4214. doi: 10.2174/0929867321666140716100449
Spence, A. R., Hopkins, G. R., Neuman-Lee, L. A., Smith, G. D., Brodie, E. D. Jr., and French, S. S. (2016). Detrimental effects of zinc oxide nanoparticles on amphibian life stages. J. Exp. Zool. A Ecol. Genet. Physiol. 325, 415–424. doi: 10.1002/jez.2026
Tayebati, S. K., Di Tullio, M. A., and Amenta, F. (2004). Effect of treatment with the cholinesterase inhibitor rivastigmine on vesicular acetylcholine transporter and choline acetyltransferase in rat brain. Clin. Exp. Hypertens. 26, 363–373. doi: 10.1081/CEH-120034140
Tian, X., Zhu, M., Du, L., Wang, J., Fan, Z., Liu, J., et al. (2013). Intrauterine inflammation increases materno-fetal transfer of gold nanoparticles in a size-dependent manner in murine pregnancy. Small 9, 2432–2439. doi: 10.1002/smll.201300817
Torres-Duarte, C., Adeleye, A. S., Pokhrel, S., Madler, L., Keller, A. A., and Cherr, G. N. (2016). Developmental effects of two different copper oxide nanomaterials in sea urchin (Lytechinus pictus) embryos. Nanotoxicology 10, 671–679. doi: 10.3109/17435390.2015.1107145
Vijayaraghavan, S., Karami, A., Aeinehband, S., Behbahani, H., Grandien, A., Nilsson, B., et al. (2013). Regulated extracellular choline acetyltransferase activity- The plausible missing link of the distant action of acetylcholine in the cholinergic anti-inflammatory pathway. PLoS One 8:e65936. doi: 10.1371/journal.pone.0065936
Xin, Q., Rotchell, J. M., Cheng, J., Yi, J., and Zhang, Q. (2015). Silver nanoparticles affect the neural development of zebrafish embryos. J. Appl. Toxicol. 35, 1481–1492. doi: 10.1002/jat.3164
Yamashita, K., Yoshioka, Y., Higashisaka, K., Mimura, K., Morishita, Y., Nozaki, M., et al. (2011). Silica and titanium dioxide nanoparticles cause pregnancy complications in mice. Nat. Nanotechnol. 6, 321–328. doi: 10.1038/nnano.2011.41
Yang, H., Sun, C., Fan, Z., Tian, X., Yan, L., Du, L., et al. (2012). Effects of gestational age and surface modification on materno-fetal transfer of nanoparticles in murine pregnancy. Sci. Rep. 2:847. doi: 10.1038/srep00847
Yi, H., Wang, Z., Li, X., Yin, M., Wang, L., Aldalbahi, A., et al. (2016). Silica nanoparticles target a Wnt signal transducer for degradation and impair embryonic development in zebrafish. Theranostics 6, 1810–1820. doi: 10.7150/thno.16127
Yokel, R. A. (2000). The toxicology of aluminum in the brain: a review. Neurotoxicology 21, 813–828.
Yokel, R. A. (2002). Brain uptake, retention, and efflux of aluminum and manganese. Environ. Health Perspect. 110(Suppl. 5), 699–704. doi: 10.1289/ehp.02110s5699
Yumoto, S., Nagai, H., Matsuzaki, H., Matsumura, H., Tada, W., Nagatsuma, E., et al. (2001). Aluminium incorporation into the brain of rat fetuses and sucklings. Brain Res. Bull. 55, 229–234. doi: 10.1016/S0361-9230(01)00509-3
Zhang, Q., Li, N., Jiao, X., Qin, X., Kaur, R., Lu, X., et al. (2014). Caspase-3 short hairpin RNAs: a potential therapeutic agent in neurodegeneration of aluminum-exposed animal model. Curr. Alzheimer Res. 11, 961–970. doi: 10.2174/1567205011666141107150938
Zhang, Q., Xu, L., Wang, J., Sabbioni, E., Piao, L., Di Gioacchino, M., et al. (2013). Lysosomes involved in the cellular toxicity of nano-alumina: combined effects of particle size and chemical composition. J. Biol. Regul. Homeost. Agents 27, 365–375.
Zhang, Q. L., Li, M. Q., Ji, J. W., Gao, F. P., Bai, R., Chen, C. Y., et al. (2011). In vivo toxicity of nano-alumina on mice neurobehavioral profiles and the potential mechanisms. Int. J. Immunopathol. Pharmacol. 24(Suppl. 1), 23S–29S.
Zhou, Z., Son, J., Harper, B., Zhou, Z., and Harper, S. (2015). Influence of surface chemical properties on the toxicity of engineered zinc oxide nanoparticles to embryonic zebrafish. Beilstein J. Nanotechnol. 6, 1568–1579. doi: 10.3762/bjnano.6.160
Keywords: alumina nanoparticles, developmental neurotoxicity, neurodevelopmental toxicity, oxidative stress, neurotransmitter
Citation: Zhang Q, Ding Y, He K, Li H, Gao F, Moehling TJ, Wu X, Duncan J and Niu Q (2018) Exposure to Alumina Nanoparticles in Female Mice During Pregnancy Induces Neurodevelopmental Toxicity in the Offspring. Front. Pharmacol. 9:253. doi: 10.3389/fphar.2018.00253
Received: 21 September 2017; Accepted: 06 March 2018;
Published: 20 March 2018.
Edited by:
Eleonore Fröhlich, Medizinische Universität Graz, AustriaReviewed by:
Catherine Thornton, Swansea University, United KingdomCopyright © 2018 Zhang, Ding, He, Li, Gao, Moehling, Wu, Duncan and Niu. This is an open-access article distributed under the terms of the Creative Commons Attribution License (CC BY). The use, distribution or reproduction in other forums is permitted, provided the original author(s) and the copyright owner are credited and that the original publication in this journal is cited, in accordance with accepted academic practice. No use, distribution or reproduction is permitted which does not comply with these terms.
*Correspondence: Qinli Zhang, emhhbmdxbDkzMDYxMTFAZ21haWwuY29t
† Present address: Yong Ding, Shaanxi Provincial Center for Disease Control and Prevention, Xi’an, China
Disclaimer: All claims expressed in this article are solely those of the authors and do not necessarily represent those of their affiliated organizations, or those of the publisher, the editors and the reviewers. Any product that may be evaluated in this article or claim that may be made by its manufacturer is not guaranteed or endorsed by the publisher.
Research integrity at Frontiers
Learn more about the work of our research integrity team to safeguard the quality of each article we publish.