- State Key Laboratory of Quality Research in Chinese Medicine and Institute of Chinese Medical Sciences, University of Macau, Macau, China
Triptolide and celastrol are predominantly active natural products isolated from the medicinal plant Tripterygium wilfordii Hook F. These compounds exhibit similar pharmacological activities, including anti-cancer, anti-inflammation, anti-obesity, and anti-diabetic activities. Triptolide and celastrol also provide neuroprotection and prevent cardiovascular and metabolic diseases. However, toxicity restricts the further development of triptolide and celastrol. In this review, we comprehensively review therapeutic targets and mechanisms of action, and translational study of triptolide and celastrol. We systemically discuss the structure-activity-relationship of triptolide, celastrol, and their derivatives. Furthermore, we propose the use of structural derivatives, targeted therapy, and combination treatment as possible solutions to reduce toxicity and increase therapeutic window of these potent natural products from T. wilfordii Hook F.
Natural Products Originating From Tripterygium wilfordii Hook F Exhibit Distinct Pharmacological Activities
Tripterygium wilfordii Hook F (TWHF), also known as Lei Gong Teng (Thunder God Vine), has a long history of improving symptoms of RA (Peterson and Schreiber, 1998; Bao and Dai, 2011; Law et al., 2011). The root bark of TWHF exhibits pharmacological activities against inflammation (Liu et al., 2010; Xue et al., 2012), autoimmune disorders (Chen Y.Z. et al., 2010), fibrosis, atherosclerosis, neurodegeneration (Choi et al., 2010), and kidney diseases (Wan Y.G. et al., 2014). TWHF extracts are used for treating autoimmune and inflammatory diseases in clinical practice (Marks, 2011). In China, the Lei Gong Teng tablet and Lei Gong Teng multiglycoside tablet have been developed for RA treatment since the 1970s (Tao et al., 2001). The chloroform/methanol extract of TWHF attenuates inflammation in patients with Crohn’s disease by inducing the differentiation of Foxp3+ T regulatory (Treg) cells and suppressing the serum levels of pro-inflammation cytokines, such as interleukin-10 and TGF-β (Li et al., 2014).
Several classes of bioactive substances have been isolated and characterized from TWHF, including sesquiterpenes, diterpenes (triptolide, tripdiolide, and triptonide), triterpenes (celastrol, pristimerin, and wilforlide A), lignans, glycosides, and alkaloids (Bao and Dai, 2011; Li et al., 2012b; Wang et al., 2013). Triptolide and celastrol are considered as the most active and promising components of TWHF. Thus, their pharmacological activities and mechanisms of action have been extensively investigated in many disease models (Table 1).
Pharmacological Activities of Triptolide
Triptolide, a diterpenoid triepoxide, was first isolated and characterized from TWHF in Kupchan et al. (1972). Subsequent studies revealed that triptolide exhibit potent pharmacological activities against inflammation, fibrosis, cancer, viral infection, oxidative stress, and osteoporosis (Chugh et al., 2012; Guo et al., 2016; Long et al., 2016). Results from molecular docking and dynamics simulation study suggest that triptolide has similar structures as hormones and thus can also bind to nuclear receptors (Liu X. et al., 2015). Triptolide selectively inhibits the chaperone activity of peroxiredoxin I, an antioxidant enzyme and molecular chaperone that plays essential functions in the development of cancer and inflammation (Zhao Q. et al., 2015). The XBP1 subunit of the transcription factor TFIIH core complex is identified as one of the molecular targets of triptolide, which was critical for the inhibitory activity of triptolide to RNA polymerase II-mediated transcription (Titov et al., 2011). This unique feature is the reason that triptolide is active to most type of diseases in preclinical investigation, such as inflammation and cancer (Han et al., 2012; Yi et al., 2016).
Anti-inflammation Activities of Triptolide
Triptolide exhibits anti-inflammation activity in T helper cell-mediated immunity especially against RA and inflammatory bowel diseases. It primarily attenuates inflammatory response in RA by inhibiting NF-κB, NF-κB/TNF-α/vascular cell adhesion molecule-1, and TGF-β1/α-smooth muscle/vimentin signaling pathways induced by TNFs and TLR4 (Huang J. et al., 2015; Gong et al., 2017). In RA, triptolide down-regulates the expression levels of myeloid cells-1 and DNAX-associated protein 12 (Fan et al., 2016). In IL-10 deficient mice colitis model, triptolide ameliorates post-surgical intestine inflammation by suppressing the miRNA-155/inositol polyphosphate-5-phosphatease D signaling pathway and producing of inflammatory cytokines (Wu et al., 2013). Triptolide also suppresses IL-6/signal transducers, the activators of transcription (STAT)3/suppressor of the cytokine signaling (SOCS)3 signaling pathway, and promotes apoptotic cell death of lamina propria mononuclear cells (Li et al., 2013).
Triptolide suppresses abnormally activated innate immune response and attenuates LPS-induced acute lung injury by decreasing leukocyte numbers, myeloperoxidase activity, and secretion of pro-inflammatory cytokines such as TNF-α, IL-1β, and IL-6 (Wang et al., 2014). The suppression of prostaglandin E2 receptor 2 signaling pathway leads to the inhibitory activity of triptolide on LPS-induced inflammation and expression of inducible nitric oxide synthase in microglia (Zhang et al., 2015). Treatment with triptolide prevents kidney damage by reducing the expression level of malondialdehyde and increasing superoxide dismutase activity by modulating the NF-κB signaling pathway in rats (Zhou Y. et al., 2016). The formation of the NLRP3 inflammasomes is abrogated by triptolide in transverse aortic constriction in mice (Li et al., 2017). Triptolide diminishes neuroinflammation through the downregulation of p38 MAPK and NF-κB signaling pathways in rat models of depression or middle cerebral artery occlusion, respectively (Wan B. et al., 2014; Xu et al., 2015; Bai et al., 2016; Zhang B. et al., 2016; Hu G. et al., 2017; Hu X. et al., 2017).
Anticancer Activities of Triptolide
Triptolide suppresses the proliferation and promotes apoptotic cell death in various cancers, especially hard-to-treat types, including prostate and pancreatic cancers. Prostate and pancreatic cancers are the third and fourth leading causes of cancer death in western countries, respectively (Isharwal et al., 2015). Treatment with triptolide induces the nuclear accumulation of p53 and apoptotic cell death in primary cultures of human prostatic epithelial cells (Kiviharju et al., 2002). The growth of prostate cancer xenograft in nude mice is inhibited after triptolide decreases the expression levels of SUMO-specific protease 1 and androgen receptor (Huang et al., 2012). Furthermore, the sensitivity of gemcitabine-resistant pancreatic cancer cells to cisplatin treatment is enhanced by triptolide through activation of mitochondria-initiated cell death pathway and suppression of HSP27 expression (Zhu W. et al., 2012).
The generally recognized molecular target of triptolide is the XBP1 subunit of transcription factor TFIIH (Titov et al., 2011). Triptolide covalently binds to the Cys342 of XBP1 by the 12,13-epoxide group (He et al., 2015), and thus inhibits transcription and nucleotide excision repair activity of RNA polymerase II in an ATP-dependent manner (Pan, 2010; Titov et al., 2011). In addition, triptolide induces phosphorylation of RPB1 subunit of RNA polymerase II on Ser1878 by activating cyclin-dependent kinase (CDK)7, which promotes degradation of RPB1 and subsequently induced cancer cell death (Manzo et al., 2012).
Developing MDR is one of the biggest challenges for cancer therapy (Joshi et al., 2017). Triptolide suppresses the expression of MDR protein, and induces apoptotic cell death of drug-sensitive parental KB cells and multidrug-resistant KB-7D and KB-tax cells (Chen Y.W. et al., 2010). The inhibitory activity on MDR cell lines is mediated through the suppression of overall transcription mediated by RNA polymerase II in a CDK7-dependent manner. In addition, triptolide alters the activity of p-glycoprotein drug efflux and mRNA level of MDR genes (Yi et al., 2016).
Pharmacological Activities of Celastrol
Pharmacological Activities of Celastrol Mediated through HSP90
Celastrol was first reported to inhibit HSP90 by promoting the nuclear translocation of HSF1, a transcription factor regulating HSP genes by binding to the heat shock elements in yeast and mammalian cells (Trott et al., 2008; Akerfelt et al., 2010; Der Sarkissian et al., 2014). Thus celastrol was defined as a HSP90 inhibitor.
Classical HSP90 inhibitors suppress the ATPase activity of HSP90 (Fontaine et al., 2015). Celastrol induces the degradation of HSP90 and its client proteins without interfering the bonds between ATP and HSP90 in pancreatic cancers and NSCLC in micromolar concentration (Zhang et al., 2008; Fan et al., 2014). Treatment with celastrol also induces the dephosphorylation and degradation of HSP90/CDC37 client protein kinases, including Raf family proteins, AKT, MEK1/2, CDK4, and epidermal growth factor receptor (EGFR) in HCC cells, leading to the inhibition of proliferation and induction of apoptotic cell death (Wei et al., 2014). Suppressing the expression of HSP90 by celastrol also sensitizes glioblastoma cells to celastrol treatment (Boridy et al., 2014).
HSP90 activity can be modulated through celastrol treatment. Celastrol restores the disrupted association between HSP90 and its co-chaperone CDC37/HSP90-HSP70 complex, and thus reduces HSP90-mediated degradation of glucocerebrosidase (GCase) (Yang et al., 2014; Van Rossum and Holsopple, 2016). The resulting increased quantity and catalytic activity of GCase compensates for glucosidase mutation, which is the primary cause of Gaucher disease (Irun et al., 2013; Yang et al., 2014). Treatment with celastrol also induces expression of HSP72, phosphorylation, and nucleus accumulation of HSF1, which abrogates proteasome activity in dexamethasone-induced atrophy (Gwag et al., 2013).
Anticancer Activities of Celastrol Independent of HSP90
Celastrol effectively inhibits the growth of melanoma xenograft in mouse by triggering ROS-mediated caspase-dependent and caspase-independent apoptotic cell death, and inactivating the PI3K/AKT signaling pathway with the dosage of 1 mg/kg (Lee et al., 2012). Treatment with celastrol also suppresses the proliferation of osteosarcoma and bladder cancer cells via ROS/c-JNK and mitochondrial apoptotic pathways, and induces apoptosis and autophagy both in vivo and in vitro (Li et al., 2015; Yu et al., 2015).
Celastrol treatment initiates programmed cell death by activating glycogen synthase kinase-3β (Feng et al., 2013). The treatment induces cell cycle arrest, activation of caspase 3/7, and apoptosis in c-Met-deficient Huh7 cells, Bel 7402 cells, and diethylnitrosamine-induced liver cancer in rats (Chang et al., 2016). It inhibits the viability of HepG2 cells by suppressing the TLR4-NF-κB signaling pathway when used alone (Shen et al., 2016), and suppresses EGFR expression when combined with lapatinib (Yan et al., 2014). Celastrol also inhibits cancer growth by activating TNF-α-induced NF-κB signaling pathway (Kang et al., 2013), inhibiting the mTOR/ribosomal protein S6 kinase/eIF4E/AKT and ERK signaling pathway, and down-regulating hypoxia-inducible factor-1α (HIF-1α) (Ma et al., 2014). Celastrol inhibits the growth of MCF-7 breast cancer cells through AMPK (Kim et al., 2013), and induces apoptosis in HT-29 colon adenocarcinoma cells through the canonical WNT/β-catenin pathway (Lu et al., 2012). Furthermore, celastrol suppresses the proliferation and invasion of ulcerative colitis-related colorectal cancer and NSCLC by inhibiting epithelial–mesenchymal transition, increasing E-cadherin expression, down-regulating N-cadherin, vimentin, and snail (Lin et al., 2015; Lo Iacono et al., 2015).
Treatment with celastrol inhibits the proliferation, migration, and invasion of chondrosarcomas cells by suppressing the inhibitor of the protein phosphatase 2A-Akt signaling pathway in vivo (Liu Z. et al., 2014). Celastrol rapidly reduces the expression level of c-MYC protein and stimulates an energy crisis by depleting ATP, inducing accumulation of neutral lipid, and activating AMPK, thereby ultimately induces cell cycle arrest and apoptotic cell death in human cancer cells (Wang et al., 2015). In addition, celastrol inhibits the growth of head and neck cancer cells by activating C/EBP-homologous protein and XBP1s, which increase the transcription of ER stress target genes and subsequently induces ER stress (Fribley et al., 2015).
Anti-inflammation Activities of Celastrol Independent of HSP90
Treatment with celastrol reduces inflammation in several disease models independent of HSP90. Celastrol significantly suppresses inflammation by reducing the secretion of IL-1β and IL-18, and inactivating the NLRP3 inflammasomes and caspase-1 in LPS/ATP-primed macrophage cells (Xin et al., 2017; Yu X. et al., 2017). Celastrol ameliorates colitis in IL-10-deficient mice by reducing colon myeloperoxidase concentration and inhibiting colonic pro-inflammatory cytokines via PI3K/Akt/mTOR signaling pathway (Zhao J. et al., 2015). It also ameliorates dextran sulfate sodium-induced colitis in caspase-1-/- mice by inhibiting the activation of NLRP3 inflammasomes and the subsequent secretion of IL-1β (Yu X. et al., 2017). Celastrol potentiates mitochondrial damage and inflammation in palmitate-induced insulin resistance in C3A hepatocytes (Abu Bakar et al., 2017). Treatment with celastrol also inhibits acute liver inflammation through the Nur77-dependent pathway and activation of autophagy in LPS and D-galactosamine-induced inflammation in mice (Greenhill, 2015). Although it inhibits the differentiation of Th17 cells, celastrol enhances the production of Treg cells by restricting the activation of STAT3 and its downstream target genes. In adjuvant arthritis rats, these processes suppress joint inflammation (Astry et al., 2015).
Activities of Celastrol in Metabolic Diseases
Metabolic diseases are often associated with over-activated inflammatory response, for example obesity and diabetes (Straub, 2017). Delaminating pathogenic factors of the disease progression lead to reduced inflammation. Celastrol inhibits obesity and metabolic dysfunction by increasing energy expenditure, activation of brown adipose tissue, and expression of mitochondrial genes in vivo. Treatment with celastrol activates the transcription of HSF1 and expression of peroxisome proliferative activated receptor coactivator-1α in mouse adipose tissues and muscle (Ma et al., 2015), promoting thermogenesis and the remodeling of white adipose tissues. In addition, celastrol inhibits high fat diet-induced obesity in Nur77-/- mice by activating autophagy and promoting the interaction between Nur77 and TRAF2 (Hu M. et al., 2017). Celastrol treatment reduces body weight gain and food intake in both high fat-fed obese in diabetes (db/db) and leptin-deficient (ob/ob) mice by potentiating STAT3-dependent leptin signaling and inhibiting ER stress (Liu J. et al., 2015).
Translational Development of Triptolide and Celastrol
Despite the various activities offered, clinical application of triptolide and celastrol remains limited due to their narrow therapeutic window and poor solubility (Wang et al., 2016). The major toxicity of triptolide includes hepatic and cardiac toxicity. Triptolide induces hepatotoxicity by suppressing the transcription of four major cytochromes P450 (CYP) isoforms, namely CYP3A, CYP2C9, CYP2C19, and CYP2E1, thus decreasing the substrate affinities and activities of these CYP enzymes, which are vital to normal metabolism regulation (Lu et al., 2017). Decrease in CYP enzymes antagonizes the activity of other drugs metabolized through these CYP isoforms (Lu et al., 2017). Treatment with triptolide leads to cardiac cytotoxicity through induction of oxidative stress, mitochondrial dysfunction, and apoptotic damage regulated by the mitochondria-mediated apoptotic signaling pathway in cardiomyocytes (Zhou et al., 2014). Considerable effort has been exerted for the reduction of toxicity of triptolide and celastrol, including combination therapy, nanoparticle coating for enhancement of water solubility and targeted delivery, and development of water-soluble analogs of triptolide and celastrol. The structural basis of the toxicity of TWHF compounds can be determined through in-depth understanding of the relationship between structures and activities of their functional analogs.
Combination Treatment for Toxicity Reduction
Lowering the dosage used of TWHF compounds by combining them with agent(s) effectively reduces their cytotoxicity and related adverse effects. Combination treatment offer new opportunities for the translational development of triptolide and celastrol. Active components from the same herb can synergize with other compounds isolated from the same herb (Zhou X. et al., 2016). For example, combination treatment of triptolide and celastrol synergistically inhibits cell growth, induces cell cycle arrest at G2/M phase and apoptosis, and increases intracellular ROS accumulation in many types of cancer cells, including H1299 and H157 lung cancer cells (Jiang et al., 2015).
Triptolide has been used for the reduction of resistance against chemotherapeutic agents. Low doses of triptolide reverses resistance to cytarabine and doxorubicin in acute lymphoblastic leukemia cell line NALM-6/R and primary cells isolated from patients with relapsed or refractory acute lymphoblastic leukemia in the mouse xenograft model (Zhao et al., 2016a). The combination treatment of triptolide and oxaliplatin significantly inhibits the proliferation of colon cancer cell lines by regulating the Wnt/β-catenin pathway in nanomolar concentration (Liu Y. et al., 2014). Triptolide together with hydroxycamptothecin exhibits broad-spectrum anticancer activities by regulating MAPK and Akt signaling pathways (Meng et al., 2015). Combination treatment with farnesoid X receptor activator GW4064 relieves triptolide-induced hepatic toxicity (Jin et al., 2015). The treatment reduces incidence of spontaneous lung cancer from 70 to 10% by potentiating the NF-κB signaling pathway-mediated production of proinflammatory cytokines in vivo when used in a low dose (1 mg/kg body weight) and in combination with aspirin (Zheng et al., 2017).
The combined treatment of celastrol and histone deacetylases inhibitor suberoylanilide hydroxamic acid simultaneously activate the NF-κB and E-cadherin signaling pathway, thus substantially inhibit growth of human cancer cells in vitro and in vivo (Zheng et al., 2014). When combined with ABT-737, a BH3 mimetic inhibitor of Bcl-xL, Bcl-2, and Bcl-w, celastrol synergistically suppresses the proliferation of HCC cells and induces apoptotic cell death (Zhu H. et al., 2012). The combination treatment with triptolide and celastrol exhibits synergistic anticancer activity in H1299 human NSCLC cells and H157 human oral carcinoma xenografts by inhibiting HSP90 activity and reducing ROS level (Jiang et al., 2015).
Nanoparticle Coating to Enhance Solubility and Organ Targeting
Nanoparticle is used as drug delivery system to improve therapeutic efficacy by increasing solubility and organ targeting through electrostatic interactions or receptor and/or membrane binding (Zhou et al., 2017).
Follicle-stimulating hormone (FSH)-β-peptide modified nanoparticle is used to increase water solubility and reduce cytotoxicity of triptolide in ovarian cancer mouse model (Chen X.Y. et al., 2015). Administration of FSH-β-peptide nanoparticle containing triptolide suppresses the serum level of antimullerian hormone, reduces prominent ovarian fibrosis, vacuolar changes, and follicle numbers (Chen X.Y. et al., 2015). A nanoparticle formed with PF-A299-585 (amino acid 299–585) peptide fragment of human serum albumin increases the kidney targeting property of triptolide, and exhibits comparable anti-inflammatory activity with less cytotoxicity in LPS-stimulated Madin-Darby canine kidney cells and in membranous nephropathic rodent model (Yuan et al., 2015). Triptolide packed with the reduction-sensitive lipid-polymer hybrid nanoparticles inhibits proliferation of human oral cavity squamous cell carcinoma cells more effectively with a low combination index with doxorubicin and reduced cytotoxicity (Wu et al., 2017). Coating with pH-sensitive floating nanoparticles allows specific uptake of triptolide by liver cancer cells, results in improved efficacy as well as reduced cytotoxicity compared with triptolide alone in vivo and in vitro (Ling et al., 2014). Disodium phosphonooxymethyl nanoparticle extends the shelf life of triptolide, reduces the dosage required to inhibit growth of human ovarian cancer xenograft compared with that of triptolide (Patil et al., 2015).
PEGylated distearoyl phosphatidylcholine liposomes packed with celastrol exhibits higher efficiency in inducing apoptotic cell death in prostate cancer cells, compared with celastrol dissolved in dimethyl sulfoxide (Wolfram et al., 2014). This formula prolongs the blood circulation time of celastrol, increasing bioavailability and reducing the frequency of dosing (Wolfram et al., 2014). Celastrol nanoparticles significantly inhibits suture-induced corneal neovascularization by reducing macrophage infiltration and decreasing the expression of vascular endothelial growth factor and matrix metalloproteinase 9 in rat cornea (Li et al., 2012c; Sanna et al., 2015). Mesoporous silica nanoparticle and axitinib in PEGylate lipid bilayers increases the inhibitory activity of celastrol on angiogenesis and mitochondrial function, and enhances its anticancer activity in SCC-7, BT-474, and SH-SY5Y cells (Choi et al., 2016). Glucose-functionalized mesoporous silica nanoparticles significantly enhances tissue targeting and inhibitory activity of celastrol toward Hela and A549 cancer cells (Niemela et al., 2015). Celastrol-albumin nanoparticles exhibits tissue targeting property to mesangial cells, and attenuates proteinuria, inflammation, and glomerular hypercellularity in mesangial cell-mediated glomerulonephritis (Guo et al., 2017). Celastrol-albumin nanoparticles reduces the accumulation of free celastrol in off-target organs and tissues, thus successfully reducing the systemic toxicity of celastrol (Guo et al., 2017).
Development of Water-Soluble Analogs of Triptolide and Celastrol
Several water-soluble analogs of triptolide and celastrol were synthesized and evaluated in laboratory animal models and clinical trials. PG490-88, as a water-soluble succinate salt analog of triptolide, more specifically and effectively blocks pulmonary fibrosis in intratracheal bleomycin mouse model than triptolide (Krishna et al., 2001). PG490-88 also inhibits the growth of cancer-derived primary cultures of human prostatic epithelial cells in a p53-dependent manner (Kiviharju et al., 2002). MRx 102, a triptolide derivative with C-14-hydroxyl modification of amine ester group, differentially regulates the expression of retinoid X receptor-α (RXRα) but not the level of full length of RXRα, and inhibits cancer cell growth through the inhibition of AKT signaling pathway (Wang et al., 2017).
(5R)-5-hydroxytriptolide (LLDT-8) is a triptolide analog with a favorable safety profile, the efficacy of which will be examined for the treatment of RA and cancer in phase II clinical trials (Wang L. et al., 2012; Qi et al., 2017). LLDT-8 maintains the immune suppressive activity of triptolide (Zhang et al., 2017). Treatment with LLDT-8 ameliorates anti-GBM glomerulonephritis by regulating the Fcγ receptor signaling (Qi et al., 2017). LLDT-8 exhibits anti-inflammation activity and inhibits transcription in a similar fashion as triptolide (Chen et al., 2016). However, testis toxicity is associated with long-term LLDT-8 treatment due to its upregulation of TGF-β activated kinase 1 (Yu C. et al., 2017).
Minnelide, a phosphonooxymethytriptolide disodium salt, was synthesized by reacting triptolide with acetic anhydride in dimethyl sulfoxide at room temperature for 5 days (Chugh et al., 2012). Minnelide inhibits the growth of multiple cancers in pre-clinical studies (Chugh et al., 2012; Jacobson et al., 2015; Oliveira et al., 2015; Arora et al., 2017; Isharwal et al., 2017), for example, colon cancer and metastasis to liver (Oliveira et al., 2015). The growth of pancreatic cancer in KRas and TP53 mutant mouse model (KRasG12D; Trp53R172H; Pdx-1Cre) is also attenuated by minnelide (Chugh et al., 2012). No overt signs of toxicity is observed during more than 1 year’s treatment of minnelide in athymic nude mice bearing human pancreatic cancer xenograft (Chugh et al., 2012); thus the therapeutic window of minnelide is greatly enhanced than that of triptolide. The efficacy of minnelide in patients with refractory pancreatic cancer is currently evaluated in phase II clinical trial (NCT03117920).
Few C6-indole modified water soluble analogs of celastrol were synthesized. NST001A, a sodium salt of celastrol, inhibits the growth of human colon cancer cell-Colo 205 colon cells in vitro and in vivo (Tang et al., 2014). Two celastrol derivatives (NST001 and NST001B) also exhibits enhanced potency against the growth of HCC cells than celastrol (Tang et al., 2014). CEL20 disrupts the interaction of HSP90-CDC37 more efficiently than celastrol in A549, MCF7, and pancreatic Panc-1 human cancer cell lines (Jiang et al., 2016).
Structure-Activity-Relationship of Compounds Isolated from TWHF
The structural-activity-relationship of major compounds isolated from TWHF against key signaling pathways regulating inflammation have been studied by many groups, aiming to evaluate SAR for the future modifications of TWHF compounds.
Tripterygium wilfordii Hook F compounds including triptolide with epoxide are attacked by a well-positioned -SH, which is one of the determinant factors for their pharmacological activities (He et al., 2015). Triptolide binds to the cysteine residues of target proteins through covalent bond; and thus modifies the properties and activities of the target proteins. However, no epoxide moiety is observed on other TWHF compounds such as withaferin A and celastrol, although they exhibits the same activities as triptolide (Zhao Q. et al., 2015). In withaferin A and celastrol, 1,4-dipolar structure constructed by the carbonyl and adjacent double carbon-carbon bond that binds to the cysteine residues of the target proteins. The epoxide and 1,4-dipolar structure are both electrophilic groups that can be attacked by -SH via ring-opening and Michael reactions, respectively. Therefore, the covalent bond between the electrophilic structure of TWHF compounds and cysteine residues on target proteins could be considered as determinant factor for the SAR of TWHF compounds.
The difference in the Michael addition and ring-opening reactions of celastrol, pristimerin, and triptolide may have led to the huge difference among the biological activities of these TWHF compounds. TWHF compounds with the electrophilic structure, including triptolide, celastrol, and pristimerin exhibit similar inhibitory activities against NF-κB, STAT3, and SMAD2/3 signaling pathways (Table 2). Comparatively, the potency of celastrol and pristimerin against key signaling pathways regulating inflammation is lower than that of triptolide (Table 2). Especially that the concentration of pristimerin required to suppresses NF-κB activation already reduces cell viability, suggesting that the immune suppressive activity of pristimerin is due to the inhibition of cell growth. This is in line with other reports that pristimerin minimally inhibits the proliferation of cancer cells (Huang S. et al., 2015; Zhao et al., 2016b).
Inspired by the epoxide’s effect on protein proposed by Zhao Q. et al. (2015), the epoxide moieties on the C-6a and C-7a positions of triptolide could act as acceptors for cysteine (Figure 1). Triptolide directly binds to cysteine via the ring-opening interaction between epoxide and -SH moiety of cysteine, which could lead to the dissociation of these proteins to their downstream target elements. Instead of epoxide, the 1,4-dipolar structure constructed by the carbonyl on C-2 and its adjacent alkenyl on celastrol and pristimerin acts as an acceptor of cysteine (Figure 1). This structure can combine with -SH by the Michael reaction. The activities of the Michael addition and ring-opening reaction might have caused the differences among the pharmacological activities of celastrol, pristimerin, and triptolide. Wilforlide A without epoxide or 1,4-dipolar structure is inactive to all these signaling pathways, and only exhibits marginal potency against inflammation in RA rats (Xue et al., 2010). Optimized derivatives with reduced toxicity may be used for further translational development.
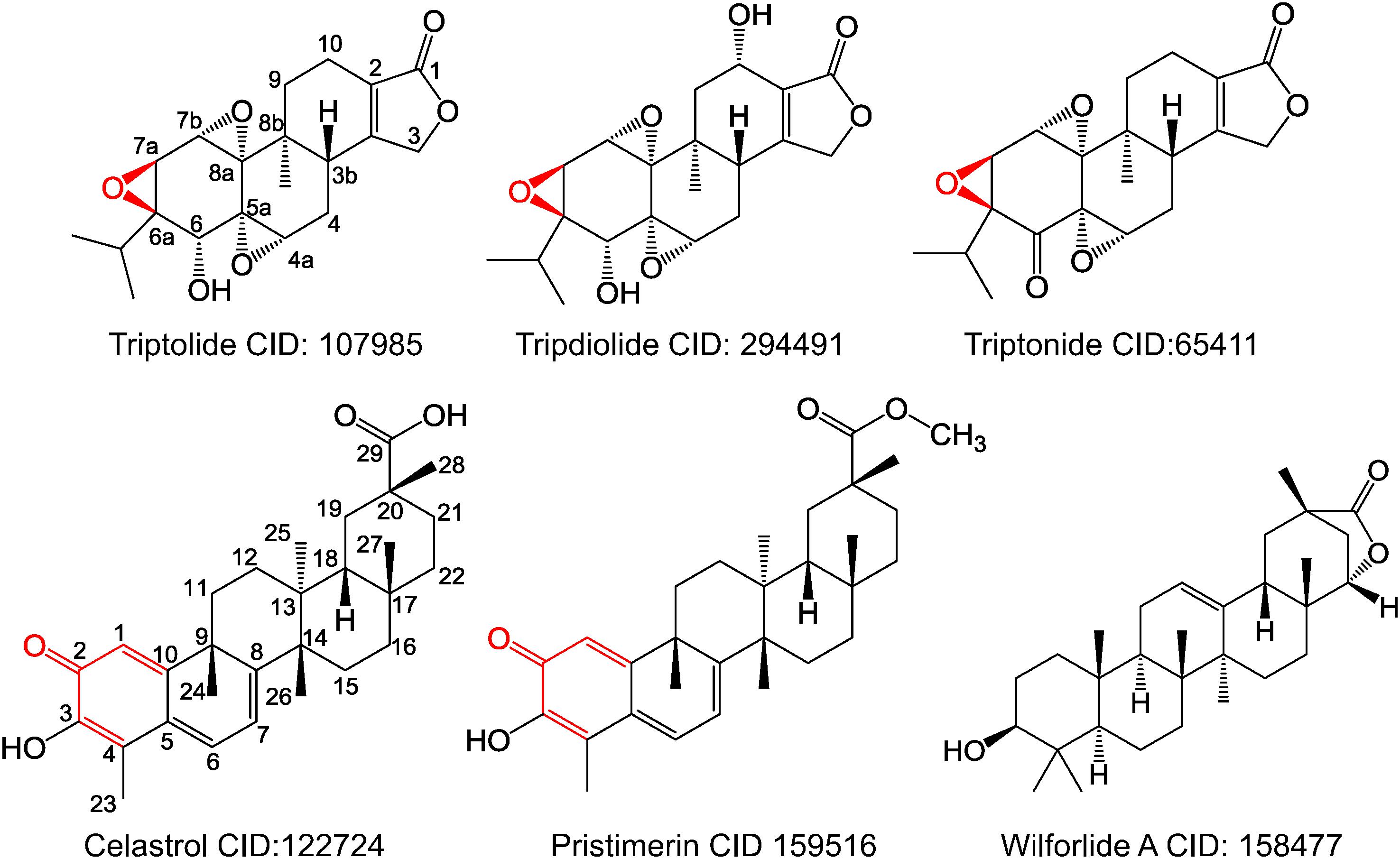
FIGURE 1. Chemical structures of compounds isolated from Tripterygium wilfordii Hook F. Red color labeled moieties essential to maintain biological activities.
Summary
On the basis of the pharmacological activities of compounds isolated from THWF, especially triptolide and celastrol, we conclude that the systemic evaluation of the in vivo activities of these compounds is still needed. Targeted delivery systems, structure modifications, and the mechanisms of action of these compounds are essential in the design of novel derivatives with reduced cytotoxicity, improved efficacy, and increased therapeutic index.
Author Contributions
S-RC, YtW, and YW mainly drafted the work critical for important intellectual content; YD, JZ, and LL finished the SAR discussion; all authors approved the version to be published.
Funding
This work was partially supported by Macao Science and Technology Development Fund 041/2014/A1, Research Fund of the University of Macau MYRG 2016-00105-ICMS-QRCM, and MYRG 2017-00116-ICMS-QRCM to YW, and MYRG2015-00098-ICMS-QRCM to YtW.
Conflict of Interest Statement
The authors declare that the research was conducted in the absence of any commercial or financial relationships that could be construed as a potential conflict of interest.
Abbreviations
AMPK, AMP-activated protein kinase; ATP, adenosine triphosphate; BMP, bone morphogenetic protein; CDC, cell division cycle; CDK, cyclin dependent kinase; CYP, cytochrome protein; DEN, diethylnitrosamine; ER, endoplasmic reticulum; GCase, glucocerebrosidase; HCC, hepatocellular carcinoma; HSF, heat shock factor; HSP, heat shock protein; IFN, interferon; IL, interleukin; LPS, lipopolysaccharide; MDR, multi-drug resistance; mTOR, mammalian target of rapamycin; NF, nuclear factor; NLRP3, NLR family pyrin domain containing 3; NSCLC, non-small cell lung carcinoma; RA, rheumatoid arthritis; ROS, reactive oxygen species; RXRα, retinoid X receptor-α; SHIP, inositol polyphosphate-5-phosphatease; STAT, signal transducer and activator of transcription; TGF, transforming growth factor; TLR, toll-like receptor; TNF, tumor necrosis factor; Treg, T regulatory; TWHF, Tripterygium wilfordii Hook F; XBP1, X box binding protein 1.
References
Abu Bakar, M. H., Sarmidi, M. R., Tan, J. S., and Mohamad Rosdi, M. N. (2017). Celastrol attenuates mitochondrial dysfunction and inflammation in palmitate-mediated insulin resistance in C3A hepatocytes. Eur. J. Pharmacol. 799, 73–83. doi: 10.1016/j.ejphar.2017.01.043
Akerfelt, M., Morimoto, R. I., and Sistonen, L. (2010). Heat shock factors: integrators of cell stress, development and lifespan. Nat. Rev. Mol. Cell Biol. 11, 545–555. doi: 10.1038/nrm2938
Alsaied, O. A., Sangwan, V., Banerjee, S., Krosch, T. C., Chugh, R., Saluja, A., et al. (2014). Sorafenib and triptolide as combination therapy for hepatocellular carcinoma. Surgery 156, 270–279. doi: 10.1016/j.surg.2014.04.055
Arora, N., Alsaied, O., Dauer, P., Majumder, K., Modi, S., Giri, B., et al. (2017). Downregulation of Sp1 by Minnelide leads to decrease in HSP70 and decrease in tumor burden of gastric cancer. PLOS ONE 12:e0171827. doi: 10.1371/journal.pone.0171827
Astry, B., Venkatesha, S. H., Laurence, A., Christensen-Quick, A., Garzino-Demo, A., Frieman, M. B., et al. (2015). Celastrol, a Chinese herbal compound, controls autoimmune inflammation by altering the balance of pathogenic and regulatory T cells in the target organ. Clin. Immunol. 157, 228–238. doi: 10.1016/j.clim.2015.01.011
Bai, S., Hu, Z., Yang, Y., Yin, Y., Li, W., Wu, L., et al. (2016). Anti-inflammatory and neuroprotective effects of triptolide via the NF-kappaB signaling pathway in a rat MCAO model. Anat. Rec. 299, 256–266. doi: 10.1002/ar.23293
Bao, J., and Dai, S. M. (2011). A Chinese herb Tripterygium wilfordii Hook F in the treatment of rheumatoid arthritis: mechanism, efficacy, and safety. Rheumatol. Int. 31, 1123–1129. doi: 10.1007/s00296-011-1841-y
Boridy, S., Le, P. U., Petrecca, K., and Maysinger, D. (2014). Celastrol targets proteostasis and acts synergistically with a heat-shock protein 90 inhibitor to kill human glioblastoma cells. Cell Death Dis. 5:e1216. doi: 10.1038/cddis.2014.182
Brand, D. D., Latham, K. A., and Rosloniec, E. F. (2007). Collagen-induced arthritis. Nat. Protoc. 2, 1269–1275. doi: 10.1038/nprot.2007.173
Chang, W., He, W., Li, P. P., Song, S. S., Yuan, P. F., Lu, J. T., et al. (2016). Protective effects of Celastrol on diethylnitrosamine-induced hepatocellular carcinoma in rats and its mechanisms. Eur. J. Pharmacol. 784, 173–180. doi: 10.1016/j.ejphar.2016.04.045
Chen, M., Lv, Z., Huang, L., Zhang, W., Lin, X., Shi, J., et al. (2015). Triptolide inhibits TGF-beta1-induced cell proliferation in rat airway smooth muscle cells by suppressing Smad signaling. Exp. Cell Res. 331, 362–368. doi: 10.1016/j.yexcr.2014.10.016
Chen, X. Y., Chen, W. L., Ma, M., Gu, C., Xiao, X. R., and Li, B. (2015). The potential of follicle-stimulating hormone peptide-modified triptolide-loaded nanoparticles to induce a mouse model of premature ovarian insufficiency. Int. J. Nanomedicine 10, 2765–2774. doi: 10.2147/IJN.S72593
Chen, Y., Zhang, L., Ni, J., Wang, X., Cheng, J., Li, Y., et al. (2016). LLDT-8 protects against cerebral ischemia/reperfusion injury by suppressing post-stroke inflammation. J. Pharmacol. Sci. 131, 131–137. doi: 10.1016/j.jphs.2016.05.003
Chen, Y. W., Lin, G. J., Chuang, Y. P., Chia, W. T., Hueng, D. Y., Lin, C. K., et al. (2010). Triptolide circumvents drug-resistant effect and enhances 5-fluorouracil antitumor effect on KB cells. Anticancer Drugs 21, 502–513. doi: 10.1097/CAD.0b013e328337337c
Chen, Y. Z., Gao, Q., Zhao, X. Z., Chen, X. M., Zhang, F., Chen, J., et al. (2010). Meta-analysis of Tripterygium wilfordii Hook F in the immunosuppressive treatment of IgA nephropathy. Intern. Med. 49, 2049–2055. doi: 10.2169/internalmedicine.49.3704
Choi, B. S., Sapkota, K., Kim, S., Lee, H. J., Choi, H. S., and Kim, S. J. (2010). Antioxidant activity and protective effects of Tripterygium regelii extract on hydrogen peroxide-induced injury in human dopaminergic cells, SH-SY5Y. Neurochem. Res. 35, 1269–1280. doi: 10.1007/s11064-010-0185-4
Choi, J. Y., Ramasamy, T., Kim, S. Y., Kim, J., Ku, S. K., Youn, Y. S., et al. (2016). PEGylated lipid bilayer-supported mesoporous silica nanoparticle composite for synergistic co-delivery of axitinib and celastrol in multi-targeted cancer therapy. Acta Biomater. 39, 94–105. doi: 10.1016/j.actbio.2016.05.012
Chu, C., He, W., Kuang, Y., Ren, K., and Gou, X. (2014). Celastrol protects kidney against ischemia-reperfusion-induced injury in rats. J. Surg. Res. 186, 398–407. doi: 10.1016/j.jss.2013.07.048
Chugh, R., Sangwan, V., Patil, S. P., Dudeja, V., Dawra, R. K., Banerjee, S., et al. (2012). A preclinical evaluation of Minnelide as a therapeutic agent against pancreatic cancer. Sci. Transl. Med. 4:156ra139. doi: 10.1126/scitranslmed.3004334
Deeb, D., Gao, X., Liu, Y., Pindolia, K., and Gautam, S. C. (2015). Inhibition of hTERT/telomerase contributes to the antitumor activity of pristimerin in pancreatic ductal adenocarcinoma cells. Oncol. Rep. 34, 518–524. doi: 10.3892/or.2015.3989
Der Sarkissian, S., Cailhier, J. F., Borie, M., Stevens, L. M., Gaboury, L., Mansour, S., et al. (2014). Celastrol protects ischaemic myocardium through a heat shock response with up-regulation of haeme oxygenase-1. Br. J. Pharmacol. 171, 5265–5279. doi: 10.1111/bph.12838
Ding, X., Zhou, X., Jiang, B., Zhao, Q., and Zhou, G. (2015). Triptolide suppresses proliferation, hypoxia-inducible factor-1alpha and c-Myc expression in pancreatic cancer cells. Mol. Med. Rep. 12, 4508–4513. doi: 10.3892/mmr.2015.3960
Fan, D., He, X., Bian, Y., Guo, Q., Zheng, K., Zhao, Y., et al. (2016). Triptolide modulates TREM-1 signal pathway to inhibit the inflammatory response in rheumatoid arthritis. Int. J. Mol. Sci. 17:498. doi: 10.3390/ijms17040498
Fan, X. X., Li, N., Wu, J. L., Zhou, Y. L., He, J. X., Liu, L., et al. (2014). Celastrol induces apoptosis in gefitinib-resistant non-small cell lung cancer cells via caspases-dependent pathways and Hsp90 client protein degradation. Molecules 19, 3508–3522. doi: 10.3390/molecules19033508
Feng, L., Zhang, D., Fan, C., Ma, C., Yang, W., Meng, Y., et al. (2013). ER stress-mediated apoptosis induced by celastrol in cancer cells and important role of glycogen synthase kinase-3beta in the signal network. Cell Death Dis. 4:e715. doi: 10.1038/cddis.2013.222
Fontaine, S. N., Rauch, J. N., Nordhues, B. A., Assimon, V. A., Stothert, A. R., Jinwal, U. K., et al. (2015). Isoform-selective genetic inhibition of constitutive cytosolic Hsp70 activity promotes client tau degradation using an altered co-chaperone complement. J. Biol. Chem. 290, 13115–13127. doi: 10.1074/jbc.M115.637595
Freudlsperger, C., Bian, Y., Contag Wise, S., Burnett, J., Coupar, J., Yang, X., et al. (2013). TGF-beta and NF-kappaB signal pathway cross-talk is mediated through TAK1 and SMAD7 in a subset of head and neck cancers. Oncogene 32, 1549–1559. doi: 10.1038/onc.2012.171
Fribley, A. M., Miller, J. R., Brownell, A. L., Garshott, D. M., Zeng, Q., Reist, T. E., et al. (2015). Celastrol induces unfolded protein response-dependent cell death in head and neck cancer. Exp. Cell Res. 330, 412–422. doi: 10.1016/j.yexcr.2014.08.014
Fu, Y., Lin, Q., Gong, T., Sun, X., and Zhang, Z. R. (2016). Renal-targeting triptolide-glucosamine conjugate exhibits lower toxicity and superior efficacy in attenuation of ischemia/reperfusion renal injury in rats. Acta Pharmacol. Sin. 37, 1467–1480. doi: 10.1038/aps.2016.44
Gao, Q., Shen, W., Qin, W., Zheng, C., Zhang, M., Zeng, C., et al. (2010). Treatment of db/db diabetic mice with triptolide: a novel therapy for diabetic nephropathy. Nephrol. Dial. Transplant. 25, 3539–3547. doi: 10.1093/ndt/gfq245
Gong, Y., Huang, X., Wang, D., Li, M., and Liu, Z. (2017). Triptolide protects bone against destruction by targeting RANKL-mediated ERK/AKT signalling pathway in the collagen-induced rheumatoid arthritis. Biomed. Res. 28, 4111–4116.
Greenhill, C. (2015). Celastrol identified as a leptin sensitizer and potential novel treatment for obesity. Nat. Rev. Endocrinol. 11:444. doi: 10.1038/nrendo.2015.94
Gu, L., Bai, W., Li, S., Zhang, Y., Han, Y., Gu, Y., et al. (2013). Celastrol prevents atherosclerosis via inhibiting LOX-1 and oxidative stress. PLOS ONE 8:e65477. doi: 10.1371/journal.pone.0065477
Guan, Y., Cui, Z. J., Sun, B., Han, L. P., Li, C. J., and Chen, L. M. (2016). Celastrol attenuates oxidative stress in the skeletal muscle of diabetic rats by regulating the AMPK-PGC1alpha-SIRT3 signaling pathway. Int. J. Mol. Med. 37, 1229–1238. doi: 10.3892/ijmm.2016.2549
Guo, L., Luo, S., Du, Z., Zhou, M., Li, P., Fu, Y., et al. (2017). Targeted delivery of celastrol to mesangial cells is effective against mesangioproliferative glomerulonephritis. Nat. Commun. 8:878. doi: 10.1038/s41467-017-00834-8
Guo, X., Xue, M., Li, C. J., Yang, W., Wang, S. S., Ma, Z. J., et al. (2016). Protective effects of triptolide on TLR4 mediated autoimmune and inflammatory response induced myocardial fibrosis in diabetic cardiomyopathy. J. Ethnopharmacol. 193, 333–344. doi: 10.1016/j.jep.2016.08.029
Gwag, T., Park, K., Kim, E., Son, C., Park, J., Nikawa, T., et al. (2013). Inhibition of C2C12 myotube atrophy by a novel HSP70 inducer, celastrol, via activation of Akt1 and ERK1/2 pathways. Arch. Biochem. Biophys. 537, 21–30. doi: 10.1016/j.abb.2013.06.006
Han, R., Rostami-Yazdi, M., Gerdes, S., and Mrowietz, U. (2012). Triptolide in the treatment of psoriasis and other immune-mediated inflammatory diseases. Br. J. Clin. Pharmacol. 74, 424–436. doi: 10.1111/j.1365-2125.2012.04221.x
He, Q. L., Titov, D. V., Li, J., Tan, M., Ye, Z., Zhao, Y., et al. (2015). Covalent modification of a cysteine residue in the XPB subunit of the general transcription factor TFIIH through single epoxide cleavage of the transcription inhibitor triptolide. Angew. Chem. Int. Ed. Engl. 54, 1859–1863. doi: 10.1002/anie.201408817
Hu, G., Gong, X., Wang, L., Liu, M., Liu, Y., Fu, X., et al. (2017). Triptolide promotes the clearance of alpha-synuclein by enhancing autophagy in neuronal cells. Mol. Neurobiol. 54, 2361–2372. doi: 10.1007/s12035-016-9808-3
Hu, M., Luo, Q., Alitongbieke, G., Chong, S., Xu, C., Xie, L., et al. (2017). Celastrol-induced Nur77 interaction with TRAF2 alleviates inflammation by promoting mitochondrial ubiquitination and autophagy. Mol. Cell 66, 141.e6–153.e6. doi: 10.1016/j.molcel.2017.03.008
Hu, X., Dong, Y., Jin, X., Zhang, C., Zhang, T., Zhao, J., et al. (2017). The novel and potent anti-depressive action of triptolide and its influences on hippocampal neuroinflammation in a rat model of depression comorbidity of chronic pain. Brain Behav. Immun. 64, 180–194. doi: 10.1016/j.bbi.2017.03.005
Huang, J., Zhou, L., Wu, H., Pavlos, N., Chim, S. M., Liu, Q., et al. (2015). Triptolide inhibits osteoclast formation, bone resorption, RANKL-mediated NF-B activation and titanium particle-induced osteolysis in a mouse model. Mol. Cell. Endocrinol. 399, 346–353. doi: 10.1016/j.mce.2014.10.016
Huang, S., He, P., Peng, X., Li, J., Xu, D., and Tang, Y. (2015). Pristimerin inhibits prostate cancer bone metastasis by targeting PC-3 stem cell characteristics and VEGF-induced vasculogenesis of BM-EPCs. Cell Physiol. Biochem. 37, 253–268. doi: 10.1159/000430350
Huang, W., He, T., Chai, C., Yang, Y., Zheng, Y., Zhou, P., et al. (2012). Triptolide inhibits the proliferation of prostate cancer cells and down-regulates SUMO-specific protease 1 expression. PLOS ONE 7:e37693. doi: 10.1371/journal.pone.0037693
Hui, B., Zhang, L., Zhou, Q., and Hui, L. (2018). Pristimerin inhibits LPS-triggered neurotoxicity in BV-2 microglia cells through modulating IRAK1/TRAF6/TAK1-mediated NF-kappaB and AP-1 signaling pathways in vitro. Neurotox. Res. 33, 268–283. doi: 10.1007/s12640-017-9837-3
Irun, P., Alfonso, P., Aznarez, S., Giraldo, P., and Pocovi, M. (2013). Chitotriosidase variants in patients with Gaucher disease. Implications for diagnosis and therapeutic monitoring. Clin. Biochem. 46, 1804–1807. doi: 10.1016/j.clinbiochem.2013.09.006
Isharwal, S., Modi, S., Arora, N., Uhlrich, C. III, Giri, B., Barlass, U., et al. (2017). Minnelide inhibits androgen dependent, castration resistant prostate cancer growth by decreasing expression of androgen receptor full length and splice variants. Prostate 77, 584–596. doi: 10.1002/pros.23298
Isharwal, S., Modi, S., Barlass, U., Dudeja, V., Saluja, A., Banerjee, S., et al. (2015). “Abstract LB-017: Minnelide reduces castration-resistant and enzalutamide-resistant prostate cancer via downregulation of androgen receptor-mediated signaling,” in Proceedings of the AACR 106th Annual Meeting 2015, April 18–22, 2015, Philadelphia, PA. doi: 10.1158/1538-7445.AM2015-LB-017
Jacobson, B. A., Chen, E. Z., Tang, S., Belgum, H. S., McCauley, J. A., Evenson, K. A., et al. (2015). Triptolide and its prodrug Minnelide suppress Hsp70 and inhibit in vivo growth in a xenograft model of mesothelioma. Genes Cancer 6, 144–152. doi: 10.18632/genesandcancer.55
Jia, Z., Xu, C., Shen, J., Xia, T., Yang, J., and He, Y. (2015). The natural compound celastrol inhibits necroptosis and alleviates ulcerative colitis in mice. Int. Immunopharmacol. 29, 552–559. doi: 10.1016/j.intimp.2015.09.029
Jiang, C., Fang, X., Zhang, H., Wang, X., Li, M., Jiang, W., et al. (2017). Triptolide inhibits the growth of osteosarcoma by regulating microRNA-181a via targeting PTEN gene in vivo and vitro. Tumour Biol. 39:1010428317697556. doi: 10.1177/1010428317697556
Jiang, F., Wang, H. J., Bao, Q. C., Wang, L., Jin, Y. H., Zhang, Q., et al. (2016). Optimization and biological evaluation of celastrol derivatives as Hsp90-Cdc37 interaction disruptors with improved druglike properties. Bioorg. Med. Chem. 24, 5431–5439. doi: 10.1016/j.bmc.2016.08.070
Jiang, Q. W., Cheng, K. J., Mei, X. L., Qiu, J. G., Zhang, W. J., Xue, Y. Q., et al. (2015). Synergistic anticancer effects of triptolide and celastrol, two main compounds from thunder god vine. Oncotarget 6, 32790–32804. doi: 10.18632/oncotarget.5411
Jin, J., Sun, X., Zhao, Z., Wang, W., Qiu, Y., Fu, X., et al. (2015). Activation of the farnesoid X receptor attenuates triptolide-induced liver toxicity. Phytomedicine 22, 894–901. doi: 10.1016/j.phymed.2015.06.007
Joshi, P., Vishwakarma, R. A., and Bharate, S. B. (2017). Natural alkaloids as P-gp inhibitors for multidrug resistance reversal in cancer. Eur. J. Med. Chem. 138, 273–292. doi: 10.1016/j.ejmech.2017.06.047
Kang, H., Lee, M., and Jang, S. W. (2013). Celastrol inhibits TGF-beta1-induced epithelial-mesenchymal transition by inhibiting Snail and regulating E-cadherin expression. Biochem. Biophys. Res. Commun. 437, 550–556. doi: 10.1016/j.bbrc.2013.06.113
Kannaiyan, R., Hay, H. S., Rajendran, P., Li, F., Shanmugam, M. K., Vali, S., et al. (2011). Celastrol inhibits proliferation and induces chemosensitization through down-regulation of NF-kappaB and STAT3 regulated gene products in multiple myeloma cells. Br. J. Pharmacol. 164, 1506–1521. doi: 10.1111/j.1476-5381.2011.01449.x
Kim, J. H., Lee, J. O., Lee, S. K., Kim, N., You, G. Y., Moon, J. W., et al. (2013). Celastrol suppresses breast cancer MCF-7 cell viability via the AMP-activated protein kinase (AMPK)-induced p53-polo like kinase 2 (PLK-2) pathway. Cell. Signal. 25, 805–813. doi: 10.1016/j.cellsig.2012.12.005
Kiviharju, T. M., Lecane, P. S., Sellers, R. G., and Peehl, D. M. (2002). Antiproliferative and proapoptotic activities of triptolide (PG490), a natural product entering clinical trials, on primary cultures of human prostatic epithelial cells. Clin. Cancer Res. 8, 2666–2674.
Krishna, G., Liu, K., Shigemitsu, H., Gao, M., Raffin, T. A., and Rosen, G. D. (2001). PG490-88, a derivative of triptolide, blocks bleomycin-induced lung fibrosis. Am. J. Pathol. 158, 997–1004. doi: 10.1016/S0002-9440(10)64046-1
Kupchan, S. M., Court, W. A., Dailey, R. G. Jr., Gilmore, C. J., and Bryan, R. F. (1972). Triptolide and tripdiolide, novel antileukemic diterpenoid triepoxides from Tripterygium wilfordii. J. Am. Chem. Soc. 94, 7194–7195. doi: 10.1021/ja00775a078
Kyung, H., Kwong, J. M., Bekerman, V., Gu, L., Yadegari, D., Caprioli, J., et al. (2015). Celastrol supports survival of retinal ganglion cells injured by optic nerve crush. Brain Res. 1609, 21–30. doi: 10.1016/j.brainres.2015.03.032
Law, S. K., Simmons, M. P., Techen, N., Khan, I. A., He, M. F., Shaw, P. C., et al. (2011). Molecular analyses of the Chinese herb Leigongteng (Tripterygium wilfordii Hook.f.). Phytochemistry 72, 21–26. doi: 10.1016/j.phytochem.2010.10.015
Lee, H. W., Jang, K. S., Choi, H. J., Jo, A., Cheong, J. H., and Chun, K. H. (2014). Celastrol inhibits gastric cancer growth by induction of apoptosis and autophagy. BMB Rep. 47, 697–702. doi: 10.5483/BMBRep.2014.47.12.069
Lee, J. H., Won, Y. S., Park, K. H., Lee, M. K., Tachibana, H., Yamada, K., et al. (2012). Celastrol inhibits growth and induces apoptotic cell death in melanoma cells via the activation ROS-dependent mitochondrial pathway and the suppression of PI3K/AKT signaling. Apoptosis 17, 1275–1286. doi: 10.1007/s10495-012-0767-5
Lee, K. Y., Chang, W., Qiu, D., Kao, P. N., and Rosen, G. D. (1999). PG490 (triptolide) cooperates with tumor necrosis factor-alpha to induce apoptosis in tumor cells. J. Biol. Chem. 274, 13451–13455. doi: 10.1074/jbc.274.19.13451
Lee, K. Y., Park, J. S., Jee, Y. K., and Rosen, G. D. (2002). Triptolide sensitizes lung cancer cells to TNF-related apoptosis-inducing ligand (TRAIL)-induced apoptosis by inhibition of NF-kappaB activation. Exp. Mol. Med. 34, 462–468. doi: 10.1038/emm.2002.64
Li, C. J., Chu, C. Y., Huang, L. H., Wang, M. H., Sheu, L. F., Yeh, J. I., et al. (2012a). Synergistic anticancer activity of triptolide combined with cisplatin enhances apoptosis in gastric cancer in vitro and in vivo. Cancer Lett. 319, 203–213. doi: 10.1016/j.canlet.2012.01.006
Li, C. J., Xie, F. G., Yang, J. Z., Luo, Y. M., Chen, X. G., and Zhang, D. M. (2012b). Two sesquiterpene pyridine alkaloids and a triterpenoid saponin from the root barks of Tripterygium hypoglaucum. J. Asian Nat. Prod. Res. 14, 973–980. doi: 10.1080/10286020.2012.729049
Li, G., Ren, J., Wang, G., Gu, G., Hu, D., Ren, H., et al. (2014). T2 enhances in situ level of Foxp3+ regulatory cells and modulates inflammatory cytokines in Crohn’s disease. Int. Immunopharmacol. 18, 244–248. doi: 10.1016/j.intimp.2013.12.014
Li, H. Y., Zhang, J., Sun, L. L., Li, B. H., Gao, H. L., Xie, T., et al. (2015). Celastrol induces apoptosis and autophagy via the ROS/JNK signaling pathway in human osteosarcoma cells: an in vitro and in vivo study. Cell Death Dis. 6:e1604. doi: 10.1038/cddis.2014.543
Li, R., Lu, K., Wang, Y., Chen, M., Zhang, F., Shen, H., et al. (2017). Triptolide attenuates pressure overload-induced myocardial remodeling in mice via the inhibition of NLRP3 inflammasome expression. Biochem. Biophys. Res. Commun. 485, 69–75. doi: 10.1016/j.bbrc.2017.02.021
Li, Y., Tian, Y., Zhu, W., Gong, J., Zhang, W., Yu, C., et al. (2013). Triptolide induces suppressor of cytokine signaling-3 expression and promotes lamina propria mononuclear cells apoptosis in Crohn’s colitis. Int. Immunopharmacol. 16, 268–274. doi: 10.1016/j.intimp.2013.04.018
Li, Z., Yao, L., Li, J., Zhang, W., Wu, X., Liu, Y., et al. (2012c). Celastrol nanoparticles inhibit corneal neovascularization induced by suturing in rats. Int. J. Nanomedicine 7, 1163–1173. doi: 10.2147/IJN.S27860
Lin, L., Sun, Y., Wang, D., Zheng, S., Zhang, J., and Zheng, C. (2015). Celastrol ameliorates ulcerative colitis-related colorectal cancer in mice via suppressing inflammatory responses and epithelial-mesenchymal transition. Front. Pharmacol. 6:320. doi: 10.3389/fphar.2015.00320
Ling, D., Xia, H., Park, W., Hackett, M. J., Song, C., Na, K., et al. (2014). pH-sensitive nanoformulated triptolide as a targeted therapeutic strategy for hepatocellular carcinoma. ACS Nano 8, 8027–8039. doi: 10.1021/nn502074x
Liu, B., Zhang, H., Li, J., Lu, C., Chen, G., Zhang, G., et al. (2013). Triptolide downregulates Treg cells and the level of IL-10, TGF-β, and VEGF in melanoma-bearing mice. Planta Med. 79, 1401–1407. doi: 10.1055/s-0033-1350708
Liu, J., Lee, J., Salazar Hernandez, M. A., Mazitschek, R., and Ozcan, U. (2015). Treatment of obesity with celastrol. Cell 161, 999–1011. doi: 10.1016/j.cell.2015.05.011
Liu, X., Wang, K., Duan, N., Lan, Y., Ma, P., Zheng, H., et al. (2015). Computational prediction and experimental validation of low-affinity target of triptolide and its analogues. RSC Adv. 5, 34572–34579. doi: 10.1039/C4RA17009A
Liu, Y., Chen, H. L., and Yang, G. (2010). Extract of Tripterygium wilfordii Hook F protect dopaminergic neurons against lipopolysaccharide-induced inflammatory damage. Am. J. Chin. Med. 38, 801–814. doi: 10.1142/S0192415X10008251
Liu, Y., Xiao, E., Yuan, L., and Li, G. (2014). Triptolide synergistically enhances antitumor activity of oxaliplatin in colon carcinoma in vitro and in vivo. DNA Cell Biol. 33, 418–425. doi: 10.1089/dna.2014.2356
Liu, Z., Ma, L., Wen, Z. S., Hu, Z., Wu, F. Q., Li, W., et al. (2014). Cancerous inhibitor of PP2A is targeted by natural compound celastrol for degradation in non-small-cell lung cancer. Carcinogenesis 35, 905–914. doi: 10.1093/carcin/bgt395
Lo Iacono, M., Monica, V., Vavala, T., Gisabella, M., Saviozzi, S., Bracco, E., et al. (2015). ATF2 contributes to cisplatin resistance in non-small cell lung cancer and celastrol induces cisplatin resensitization through inhibition of JNK/ATF2 pathway. Int. J. Cancer 136, 2598–2609. doi: 10.1002/ijc.29302
Long, C., Guo, W., Zhou, H., Wang, J., Wang, H., and Sun, X. (2016). Triptolide decreases expression of latency-associated nuclear antigen 1 and reduces viral titers in Kaposi’s sarcoma-associated and herpesvirus-related primary effusion lymphoma cells. Int. J. Oncol. 48, 1519–1530. doi: 10.3892/ijo.2016.3353
Lu, W., Jia, G., Meng, X., Zhao, C., Zhang, L., Ren, Y., et al. (2012). Beta-catenin mediates the apoptosis induction effect of celastrol in HT29 cells. Life Sci. 91, 279–283. doi: 10.1016/j.lfs.2012.07.032
Lu, Y., Xie, T., Zhang, Y., Zhou, F., Ruan, J., Zhu, W., et al. (2017). Triptolide induces hepatotoxicity via inhibition of CYP450s in Rat liver microsomes. BMC Complement. Altern. Med. 17:15. doi: 10.1186/s12906-016-1504-3
Ma, J., Han, L. Z., Liang, H., Mi, C., Shi, H., Lee, J. J., et al. (2014). Celastrol inhibits the HIF-1alpha pathway by inhibition of mTOR/p70S6K/eIF4E and ERK1/2 phosphorylation in human hepatoma cells. Oncol. Rep. 32, 235–242. doi: 10.3892/or.2014.3211
Ma, R., Liu, L., Liu, X., Wang, Y., Jiang, W., and Xu, L. (2013). Triptolide markedly attenuates albuminuria and podocyte injury in an animal model of diabetic nephropathy. Exp. Ther. Med. 6, 649–656. doi: 10.3892/etm.2013.1226
Ma, X., Xu, L., Alberobello, A. T., Gavrilova, O., Bagattin, A., Skarulis, M., et al. (2015). Celastrol protects against obesity and metabolic dysfunction through activation of a HSF1-PGC1alpha transcriptional axis. Cell Metab. 22, 695–708. doi: 10.1016/j.cmet.2015.08.005
Manzo, S. G., Zhou, Z. L., Wang, Y. Q., Marinello, J., He, J. X., Li, Y. C., et al. (2012). Natural product triptolide mediates cancer cell death by triggering CDK7-dependent degradation of RNA polymerase II. Cancer Res. 72, 5363–5373. doi: 10.1158/0008-5472.CAN-12-1006
Marks, W. H. (2011). Tripterygium wilfordii Hook F. versus Sulfasalazine in the treatment of rheumatoid arthritis: a well-designed clinical trial of a botanical demonstrating effectiveness. Fitoterapia 82, 85–87. doi: 10.1016/j.fitote.2010.11.024
Meng, G., Wang, W., Chai, K., Yang, S., Li, F., and Jiang, K. (2015). Combination treatment with triptolide and hydroxycamptothecin synergistically enhances apoptosis in A549 lung adenocarcinoma cells through PP2A-regulated ERK, p38 MAPKs and Akt signaling pathways. Int. J. Oncol. 46, 1007–1017. doi: 10.3892/ijo.2015.2814
Niemela, E., Desai, D., Nkizinkiko, Y., Eriksson, J. E., and Rosenholm, J. M. (2015). Sugar-decorated mesoporous silica nanoparticles as delivery vehicles for the poorly soluble drug celastrol enables targeted induction of apoptosis in cancer cells. Eur. J. Pharm. Biopharm. 96, 11–21. doi: 10.1016/j.ejpb.2015.07.009
Oliveira, A. R., Beyer, G., Chugh, R., Skube, S. J., Majumder, K., Banerjee, S., et al. (2015). Triptolide abrogates growth of colon cancer and induces cell cycle arrest by inhibiting transcriptional activation of E2F. Lab. Invest. 95, 648–659. doi: 10.1038/labinvest.2015.46
Pan, J. (2010). RNA polymerase - an important molecular target of triptolide in cancer cells. Cancer Lett. 292, 149–152. doi: 10.1016/j.canlet.2009.11.018
Pan, Y., Meng, M., Zheng, N., Cao, Z., Yang, P., Xi, X., et al. (2017). Targeting of multiple senescence-promoting genes and signaling pathways by triptonide induces complete senescence of acute myeloid leukemia cells. Biochem. Pharmacol. 126, 34–50. doi: 10.1016/j.bcp.2016.11.024
Patil, S., Lis, L. G., Schumacher, R. J., Norris, B. J., Morgan, M. L., Cuellar, R. A., et al. (2015). Phosphonooxymethyl prodrug of triptolide: synthesis, physicochemical characterization, and efficacy in human colon adenocarcinoma and ovarian cancer xenografts. J. Med. Chem. 58, 9334–9344. doi: 10.1021/acs.jmedchem.5b01329
Peterson, R. T., and Schreiber, S. L. (1998). Translation control: connecting mitogens and the ribosome. Curr. Biol. 8, R248–R250. doi: 10.1016/S0960-9822(98)70152-6
Qi, Q., Li, H., Lin, Z. M., Yang, X. Q., Zhu, F. H., Liu, Y. T., et al. (2017). (5R)-5-hydroxytriptolide ameliorates anti-glomerular basement membrane glomerulonephritis in NZW mice by regulating Fcgamma receptor signaling. Acta Pharmacol. Sin. 39, 107–116. doi: 10.1038/aps.2017.88
Sanna, V., Chamcheu, J. C., Pala, N., Mukhtar, H., Sechi, M., and Siddiqui, I. A. (2015). Nanoencapsulation of natural triterpenoid celastrol for prostate cancer treatment. Int. J. Nanomedicine 10, 6835–6846. doi: 10.2147/IJN.S93752
Sethi, G., Ahn, K. S., Pandey, M. K., and Aggarwal, B. B. (2007). Celastrol, a novel triterpene, potentiates TNF-induced apoptosis and suppresses invasion of tumor cells by inhibiting NF-kappaB-regulated gene products and TAK1-mediated NF-kappaB activation. Blood 109, 2727–2735. doi: 10.1182/blood-2006-10-050807
Shen, Y. F., Zhang, X., Wang, Y., Cao, F. F., Uzan, G., Peng, B., et al. (2016). Celastrol targets IRAKs to block Toll-like receptor 4-mediated nuclear factor-kappaB activation. J. Integr. Med. 14, 203–208. doi: 10.1016/S2095-4964(16)60257-1
Shi, Q. W., Li, S. G., Li, J., and Ling, C. Q. (2013). Anti-tumor effects of triptolide on osteosarcoma cells in vitro and in vivo: an experimental research. Zhongguo Zhong Xi Yi Jie He Za Zhi 33, 659–663.
Song, J. M., Molla, K., Anandharaj, A., Cornax, O. I, Sullivan, M. G., Kirtane, A. R., et al. (2017). Triptolide suppresses the in vitro and in vivo growth of lung cancer cells by targeting hyaluronan-CD44/RHAMM signaling. Oncotarget 8, 26927–26940. doi: 10.18632/oncotarget.15879
Straub, R. H. (2017). The brain and immune system prompt energy shortage in chronic inflammation and ageing. Nat. Rev. Rheumatol. 13, 743–751. doi: 10.1038/nrrheum.2017.172
Tang, K., Huang, Q., Zeng, J., Wu, G., Huang, J., Pan, J., et al. (2014). Design, synthesis and biological evaluation of C6-modified celastrol derivatives as potential antitumor agents. Molecules 19, 10177–10188. doi: 10.3390/molecules190710177
Tao, X., Cush, J. J., Garret, M., and Lipsky, P. E. (2001). A phase I study of ethyl acetate extract of the Chinese antirheumatic herb Tripterygium wilfordii Hook F in rheumatoid arthritis. J. Rheumatol. 28, 2160–2167.
Titov, D. V., Gilman, B., He, Q. L., Bhat, S., Low, W. K., Dang, Y., et al. (2011). XPB, a subunit of TFIIH, is a target of the natural product triptolide. Nat. Chem. Biol. 7, 182–188. doi: 10.1038/nchembio.522
Trott, A., West, J. D., Klaic, L., Westerheide, S. D., Silverman, R. B., Morimoto, R. I., et al. (2008). Activation of heat shock and antioxidant responses by the natural product celastrol: transcriptional signatures of a thiol-targeted molecule. Mol. Biol. Cell 19, 1104–1112. doi: 10.1091/mbc.E07-10-1004
Van Rossum, A., and Holsopple, M. (2016). Enzyme replacement or substrate reduction? A review of Gaucher disease treatment options. Hosp. Pharm. 51, 553–563. doi: 10.1310/hpj5107-553
Wan, B., Hu, X., Nie, J., Zhou, M., Yang, B., Li, Y., et al. (2014). Effects of triptolide on degeneration of dendritic spines induced by Abeta1-40 injection in rat hippocampus. Neurol. Sci. 35, 35–40. doi: 10.1007/s10072-013-1463-0
Wan, Y. G., Che, X. Y., Sun, W., Huang, Y. R., Meng, X. J., Chen, H. L., et al. (2014). Low-dose of multi-glycoside of Tripterygium wilfordii Hook. f., a natural regulator of TGF-beta1/Smad signaling activity improves adriamycin-induced glomerulosclerosis in vivo. J. Ethnopharmacol. 151, 1079–1089. doi: 10.1016/j.jep.2013.12.005
Wang, C., Li, C. J., Yang, J. Z., Ma, J., Chen, X. G., Hou, Q., et al. (2013). Anti-inflammatory sesquiterpene derivatives from the leaves of Tripterygium wilfordii. J. Nat. Prod. 76, 85–90. doi: 10.1021/np300759u
Wang, H., Teriete, P., Hu, A., Raveendra-Panickar, D., Pendelton, K., Lazo, J. S., et al. (2015). Direct inhibition of c-Myc-Max heterodimers by celastrol and celastrol-inspired triterpenoids. Oncotarget 6, 32380–32395. doi: 10.18632/oncotarget.6116
Wang, L., Xu, Y., Fu, L., Li, Y., and Lou, L. (2012). (5R)-5-hydroxytriptolide (LLDT-8), a novel immunosuppressant in clinical trials, exhibits potent antitumor activity via transcription inhibition. Cancer Lett. 324, 75–82. doi: 10.1016/j.canlet.2012.05.004
Wang, P. Y., Zeng, W. J., Liu, J., Wu, Y. L., Ma, Y., Zeng, Z., et al. (2017). TRC4, an improved triptolide derivative, specifically targets to truncated form of retinoid X receptor-alpha in cancer cells. Biochem. Pharmacol. 124, 19–28. doi: 10.1016/j.bcp.2016.10.014
Wang, X., Zhang, L., Duan, W., Liu, B., Gong, P., Ding, Y., et al. (2014). Anti-inflammatory effects of triptolide by inhibiting the NF-kappaB signalling pathway in LPS-induced acute lung injury in a murine model. Mol. Med. Rep. 10, 447–452. doi: 10.3892/mmr.2014.2191
Wang, X., Zhao, F., Lv, Z. M., Shi, W. Q., Zhang, L. Y., and Yan, M. (2016). Triptolide disrupts the actin-based Sertoli-germ cells adherens junctions by inhibiting Rho GTPases expression. Toxicol. Appl. Pharmacol. 310, 32–40. doi: 10.1016/j.taap.2016.08.017
Wang, Y., Zhou, Y., Zhou, H., Jia, G., Liu, J., Han, B., et al. (2012). Pristimerin causes G1 arrest, induces apoptosis, and enhances the chemosensitivity to gemcitabine in pancreatic cancer cells. PLOS ONE 7:e43826. doi: 10.1371/journal.pone.0043826
Wang, Z., Jin, H., Xu, R., Mei, Q., and Fan, D. (2009). Triptolide downregulates Rac1 and the JAK/STAT3 pathway and inhibits colitis-related colon cancer progression. Exp. Mol. Med. 41, 717–727. doi: 10.3858/emm.2009.41.10.078
Wei, W., Wu, S., Wang, X., Sun, C. K., Yang, X., Yan, X., et al. (2014). Novel celastrol derivatives inhibit the growth of hepatocellular carcinoma patient-derived xenografts. Oncotarget 5, 5819–5831. doi: 10.18632/oncotarget.2171
Wolfram, J., Suri, K., Huang, Y., Molinaro, R., Borsoi, C., Scott, B., et al. (2014). Evaluation of anticancer activity of celastrol liposomes in prostate cancer cells. J. Microencapsul. 31, 501–507. doi: 10.3109/02652048.2013.879932
Wu, B., Lu, S. T., Zhang, L. J., Zhuo, R. X., Xu, H. B., and Huang, S. W. (2017). Codelivery of doxorubicin and triptolide with reduction-sensitive lipid-polymer hybrid nanoparticles for in vitro and in vivo synergistic cancer treatment. Int. J. Nanomedicine 12, 1853–1862. doi: 10.2147/IJN.S131235
Wu, R., Li, Y., Guo, Z., Gong, J., Zhu, W., Li, N., et al. (2013). Triptolide ameliorates ileocolonic anastomosis inflammation in IL-10 deficient mice by mechanism involving suppression of miR-155/SHIP-1 signaling pathway. Mol. Immunol. 56, 340–346. doi: 10.1016/j.molimm.2013.05.006
Xin, W., Wang, Q., Zhang, D., and Wang, C. (2017). A new mechanism of inhibition of IL-1beta secretion by celastrol through the NLRP3 inflammasome pathway. Eur. J. Pharmacol. 814, 240–247. doi: 10.1016/j.ejphar.2017.08.036
Xu, L.-M., Zheng, Y.-J., Wang, Y., Yang, Y., Cao, F.-F., Peng, B., et al. (2014). Celastrol inhibits lung infiltration in differential syndrome animal models by reducing TNF-α and ICAM-1 levels while preserving differentiation in ATRA-induced acute promyelocytic leukemia cells. PLOS ONE 9:e105131. doi: 10.1371/journal.pone.0105131
Xu, P., Li, Z., Wang, H., Zhang, X., and Yang, Z. (2015). Triptolide inhibited cytotoxicity of differentiated PC12 cells induced by amyloid-Beta25-35 via the autophagy pathway. PLOS ONE 10:e0142719. doi: 10.1371/journal.pone.0142719
Xue, M., Jiang, Z. Z., Liu, J. P., Zhang, L. Y., Wang, T., Wang, H., et al. (2010). Comparative study on the anti-inflammatory and immune suppressive effect of Wilforlide A. Fitoterapia 81, 1109–1112. doi: 10.1016/j.fitote.2010.07.007
Xue, M., Jiang, Z. Z., Wu, T., Li, J., Zhang, L., Zhao, Y., et al. (2012). Anti-inflammatory effects and hepatotoxicity of Tripterygium-loaded solid lipid nanoparticles on adjuvant-induced arthritis in rats. Phytomedicine 19, 998–1006. doi: 10.1016/j.phymed.2012.06.006
Yan, Y. Y., Guo, Y., Zhang, W., Ma, C. G., Zhang, Y. X., Wang, C., et al. (2014). Celastrol enhanced the anticancer effect of lapatinib in human hepatocellular carcinoma cells in vitro. J. BUON 19, 412–418.
Yang, C., Swallows, C. L., Zhang, C., Lu, J., Xiao, H., Brady, R. O., et al. (2014). Celastrol increases glucocerebrosidase activity in Gaucher disease by modulating molecular chaperones. Proc. Natl. Acad. Sci. U.S.A. 111, 249–254. doi: 10.1073/pnas.1321341111
Yi, J. M., Huan, X. J., Song, S. S., Zhou, H., Wang, Y. Q., and Miao, Z. H. (2016). Triptolide induces cell killing in multidrug-resistant tumor cells via CDK7/RPB1 rather than XPB or p44. Mol. Cancer Ther. 15, 1495–1503. doi: 10.1158/1535-7163.MCT-15-0753
Yu, C., Li, Y., Liu, M., Gao, M., Li, C., Yan, H., et al. (2017). Critical role of hepatic Cyp450s in the testis-specific toxicity of (5R)-5-hydroxytriptolide in C57BL/6 mice. Front. Pharmacol. 8:832. doi: 10.3389/fphar.2017.00832
Yu, H., Shi, L., Zhao, S., Sun, Y., Gao, Y., Sun, Y., et al. (2016). Triptolide attenuates myocardial ischemia/reperfusion injuries in rats by inducing the activation of Nrf2/HO-1 defense pathway. Cardiovasc. Toxicol. 16, 325–335. doi: 10.1007/s12012-015-9342-y
Yu, X., Zhao, Q., Zhang, X., Zhang, H., Liu, Y., Wu, X., et al. (2017). Celastrol ameliorates inflammation through inhibition of NLRP3 inflammasome activation. Oncotarget 8, 67300–67314. doi: 10.18632/oncotarget.18619
Yu, X., Zhou, X., Fu, C., Wang, Q., Nie, T., Zou, F., et al. (2015). Celastrol induces apoptosis of human osteosarcoma cells via the mitochondrial apoptotic pathway. Oncol. Rep. 34, 1129–1136. doi: 10.3892/or.2015.4124
Yuan, K., Huang, G., Zhang, S., Zhu, Q., Yu, R., Sheng, H., et al. (2017). Celastrol alleviates arthritis by modulating the inflammatory activities of neutrophils. J. Tradit. Chin. Med. Sci. 4, 50–58. doi: 10.1016/j.jtcms.2017.05.007
Yuan, Z. X., Wu, X. J., Mo, J., Wang, Y. L., Xu, C. Q., and Lim, L. Y. (2015). Renal targeted delivery of triptolide by conjugation to the fragment peptide of human serum albumin. Eur. J. Pharm. Biopharm. 94, 363–371. doi: 10.1016/j.ejpb.2015.06.012
Zhang, B., Song, C., Feng, B., and Fan, W. (2016). Neuroprotection by triptolide against cerebral ischemia/reperfusion injury through the inhibition of NF-kappaB/PUMA signal in rats. Ther. Clin. Risk Manag. 12, 817–824. doi: 10.2147/TCRM.S106012
Zhang, L. Y., Li, H., Wu, Y. W., Cheng, L., Yan, Y. X., Yang, X. Q., et al. (2017). (5R)-5-hydroxytriptolide ameliorates lupus nephritis in MRL/lpr mice by preventing infiltration of immune cells. Am. J. Physiol. Renal Physiol. 312, F769–F777. doi: 10.1152/ajprenal.00649.2016
Zhang, T., Gong, X., Hu, G., and Wang, X. (2015). EP2-PKA signaling is suppressed by triptolide in lipopolysaccharide-induced microglia activation. J. Neuroinflammation 12:50. doi: 10.1186/s12974-015-0275-y
Zhang, T., Hamza, A., Cao, X., Wang, B., Yu, S., Zhan, C. G., et al. (2008). A novel Hsp90 inhibitor to disrupt Hsp90/Cdc37 complex against pancreatic cancer cells. Mol. Cancer Ther. 7, 162–170. doi: 10.1158/1535-7163.MCT-07-0484
Zhang, X., Yang, J., Chen, M., Li, L., Huan, F., Li, A., et al. (2016). Metabolomics profiles delineate uridine deficiency contributes to mitochondria-mediated apoptosis induced by celastrol in human acute promyelocytic leukemia cells. Oncotarget 7, 46557–46572. doi: 10.18632/oncotarget.10286
Zhao, H., Shi, P., Deng, M., Jiang, Z., Li, Y., Kannappan, V., et al. (2016a). Low dose triptolide reverses chemoresistance in adult acute lymphoblastic leukemia cells via reactive oxygen species generation and DNA damage response disruption. Oncotarget 7, 85515–85528. doi: 10.18632/oncotarget.13454
Zhao, H., Wang, C., Lu, B., Zhou, Z., Jin, Y., Wang, Z., et al. (2016b). Pristimerin triggers AIF-dependent programmed necrosis in glioma cells via activation of JNK. Cancer Lett. 374, 136–148. doi: 10.1016/j.canlet.2016.01.055
Zhao, J., Sun, Y., Shi, P., Dong, J. N., Zuo, L. G., Wang, H. G., et al. (2015). Celastrol ameliorates experimental colitis in IL-10 deficient mice via the up-regulation of autophagy. Int. Immunopharmacol. 26, 221–228. doi: 10.1016/j.intimp.2015.03.033
Zhao, Q., Ding, Y., Deng, Z., Lee, O. Y., Gao, P., Chen, P., et al. (2015). Natural products triptolide, celastrol, and withaferin A inhibit the chaperone activity of peroxiredoxin I. Chem. Sci. 6, 4124–4130. doi: 10.1039/c5sc00633c
Zheng, L., Fu, Y., Zhuang, L., Gai, R., Ma, J., Lou, J., et al. (2014). Simultaneous NF-kappaB inhibition and E-cadherin upregulation mediate mutually synergistic anticancer activity of celastrol and SAHA in vitro and in vivo. Int. J. Cancer 135, 1721–1732. doi: 10.1002/ijc.28810
Zheng, L., Jia, J., Dai, H., Wan, L., Liu, J., Hu, L., et al. (2017). Triptolide-assisted phosphorylation of p53 suppresses inflammation-induced NF-kappaB survival pathways in cancer cells. Mol. Cell. Biol. 37:e00149-17. doi: 10.1128/MCB.00149-17
Zhou, J., Xi, C., Wang, W., Fu, X., Jinqiang, L., Qiu, Y., et al. (2014). Triptolide-induced oxidative stress involved with Nrf2 contribute to cardiomyocyte apoptosis through mitochondrial dependent pathways. Toxicol. Lett. 230, 454–466. doi: 10.1016/j.toxlet.2014.08.017
Zhou, W., Yu, H., Zhang, L. J., Wu, B., Wang, C. X., Wang, Q., et al. (2017). Redox-triggered activation of nanocarriers for mitochondria-targeting cancer chemotherapy. Nanoscale 9, 17044–17053. doi: 10.1039/c7nr06130g
Zhou, X., Seto, S. W., Chang, D., Kiat, H., Razmovski-Naumovski, V., Chan, K., et al. (2016). Synergistic effects of Chinese herbal medicine: a comprehensive review of methodology and current research. Front. Pharmacol. 7:201. doi: 10.3389/fphar.2016.00201
Zhou, Y., Hong, Y., and Huang, H. (2016). Triptolide attenuates inflammatory response in membranous glomerulo-nephritis rat via downregulation of NF-kappaB signaling pathway. Kidney Blood Press. Res. 41, 901–910. doi: 10.1159/000452591
Zhu, H., Yang, W., He, L. J., Ding, W. J., Zheng, L., Liao, S. D., et al. (2012). Upregulating Noxa by ER stress, celastrol exerts synergistic anti-cancer activity in combination with ABT-737 in human hepatocellular carcinoma cells. PLOS ONE 7:e52333. doi: 10.1371/journal.pone.0052333
Keywords: Tripterygium wilfordii Hook F, triptolide, celastrol, mechanisms of action, structure-activity-relationship
Citation: Chen S-R, Dai Y, Zhao J, Lin L, Wang Y and Wang Y (2018) A Mechanistic Overview of Triptolide and Celastrol, Natural Products from Tripterygium wilfordii Hook F. Front. Pharmacol. 9:104. doi: 10.3389/fphar.2018.00104
Received: 16 November 2017; Accepted: 30 January 2018;
Published: 14 February 2018.
Edited by:
Ruiwen Zhang, University of Houston, United StatesReviewed by:
Liselotte Krenn, University of Vienna, AustriaPinarosa Avato, Università degli Studi di Bari Aldo Moro, Italy
Copyright © 2018 Chen, Dai, Zhao, Lin, Wang and Wang. This is an open-access article distributed under the terms of the Creative Commons Attribution License (CC BY). The use, distribution or reproduction in other forums is permitted, provided the original author(s) and the copyright owner are credited and that the original publication in this journal is cited, in accordance with accepted academic practice. No use, distribution or reproduction is permitted which does not comply with these terms.
*Correspondence: Ying Wang, ZW1pbHl5d2FuZ0B1bWFjLm1v; ZW1pbHl3eUBnbWFpbC5jb20= Yitao Wang, eXR3YW5nQHVtYWMubW8=