- 1Department of Pharmacology, School of Pharmacy, Macau University of Science and Technology, Macau, China
- 2Shanghai Key Laboratory of Bioactive Small Molecules, Department of Pharmacology, School of Pharmacy, Fudan University, Shanghai, China
- 3Department of Oncology, School of Medicine, Fudan University, Shanghai, China
- 4Department of Medical Oncology, Fudan University Shanghai Cancer Center, Shanghai, China
Hydrogen sulfide (H2S), the third bio-active gasotransmitter, is produced endogenously and tightly involved in the pathogenesis and treatment for various diseases. Adenosine 5′-monophosphate-activated protein kinase (AMPK) plays a paramount role in maintaining cellular energetic balance. Increasing evidences have also suggested AMPK as a novel modulator in multiple pathological conditions. In this paper, we will review the biological principles of H2S and AMPK, and most importantly, the recent discoveries regarding AMPK-mediated pharmacological actions of H2S. Emphasis will be laid on AMPK/H2S interactions in the cardiovascular system, autophagy, diabetic complications, and inflammation. In most cases described in this article, by promoting AMPK activation, H2S exerts cytoprotective effects or therapeutic potentials, though there remain some controversies before we can fully understand the involved mechanisms. Further researches are in need to investigate more closely any relationship between H2S and AMPK, and to put forward the development of H2S donors for clinical application.
Introduction
Hydrogen sulfide (H2S), the gas with “rotten egg” smell, has been recognized as the third bio-active gasotransmitter after nitric oxide and carbon monoxide. Mounting studies have revealed its protective effects in the cardiovascular system, central nervous system as well as in diabetes and inflammation. Besides the prominent role in metabolic regulation, recent reports have suggested that adenosine 5′-monophosphate (AMP)-activated protein kinase (AMPK) also participates in various physiological and pathological processes and functions as a critical mediator in the effects of H2S. In this paper, we will review the latest and emerging evidences on AMPK-mediated therapeutic potentials of H2S.
Principles of H2S Biology
Biosynthesis of H2S
For 100s of years H2S was thought to be noxious and toxic. What’s interesting is that H2S and organosulfur compounds could be easily found in recipes, such as garlic products. Dietary garlic is well-known for its benefits in lowering blood pressure and lipid levels (Jung et al., 2014; Ried, 2016). In fact, Benavides et al. (2007) pointed out that garlic-derived organic polysulfides were converted rapidly by red blood cell into H2S, which was responsible for the subsequent vasorelaxation effects.
In addition to dietary consumption, H2S is endogenously produced as well. Physiological H2S production in mammal cells is mainly attributed to three enzymes, including cystathionine β-synthase (CBS), cystathionine γ-lyase (CSE), and 3-Mercaptopyruvate sulfurtransferase (3MST).
All these enzymes exhibit tissue-specific expression. CBS is expressed abundantly in the central nerves system, liver, kidney, and so on (Fiorucci et al., 2006; Kimura, 2013; Feliers et al., 2016). It was believed that CSE was mainly produced in the cardiovascular system (Polhemus and Lefer, 2014), but recent studies reported that CSE was also detected in the liver, lung, and kidney (Song et al., 2014; Wang et al., 2017). 3MST was originally discovered in the brain of Cbs knockout mice (Shibuya et al., 2009), and further confirmed as a ubiquitous enzyme expressed in the lung, kidney, liver, and vasculature (Ahmad et al., 2016).
Moreover, these enzymes differ in cellular localization and H2S metabolism. CBS and CSE are localized in the cytosol, while 3MST is produced in both the cytosol and mitochondrial (Kimura, 2011). In contrary to 3MST, CBS, and CSE are involved in multiple transsulfuration reactions, with pyridoxal 5′-phosphate as a cofactor and sulfur amino acids as substrates, including L-cysteine, L-cystine, and homocysteine (Li et al., 2011). The H2S metabolism has been reviewed in detail elsewhere by Wang (2012).
Pharmacological Actions of H2S
During the past decades, increasing studies have evidenced the functions of H2S in mammals, among which cardiovascular regulation is the most investigated (Polhemus and Lefer, 2014). Cse knockout leads to severe hypertension in mice, suggesting H2S as a potential vasodilator (Yang et al., 2008). Indeed, similar to the other two gasotransmitters, both endogenous and exogenous-applied H2S exhibits vasorelaxation effects in multiple types of blood vessels (Kiss et al., 2008). It was reported that the proliferation of vascular smooth muscle cell was inhibited by H2S, as opposed to vascular endothelial cells (Du et al., 2004; Papapetropoulos et al., 2009). Furthermore, H2S protects mice from myocardial infarction and ischemia-induced heart failure (Calvert et al., 2010; Miao et al., 2016).
Apart from the cardiovascular system, H2S also contributes to protective effects in inflammation, oxidative stress, and nervous system. Despite the controversy regarding the multiple roles of H2S in inflammatory disorders, it is generally accepted that H2S ameliorates neuroinflammation, suppresses inflammatory cytokine production, and inhibits activation of key transcriptional factors. More details about the physiological and pharmacological functions of H2S could be found in reviews by Li et al. (2011) and Wang (2012).
AMPK and its Biological Function
AMPK Regulation
Adenosine 5′-monophosphate-activated protein kinase is a conserved energetic sensor existed in almost all eukaryotes. Composed of a catalytic α-subunit and regulatory β- and γ-subunits, AMPK monitors intracellular AMP and adenosine triphosphate (ATP) levels (Kahn et al., 2005; Li et al., 2015). In mammals, APMKα catalytic subunits and β-subunits are encoded by two genes separately (α1, α2, and β1, β2), and γ-subunit by three genes (γ1, γ2, and γ3), making at least 12 possible heterotrimer combinations, of which the expression may be tissue restricted (Hardie et al., 2012). For example, γ3 isoform is dominantly expressed in skeletal muscle, and both the wild type and a mutation of arginine to glutamine at position 225 (R225Q) in the CBS domain results in increased glycogen concentrations in skeletal muscle of the transgenic mice (Yu et al., 2006).
During energy deprivation characterized by increased AMP/ATP ratio, AMPK is phosphorylated at Thr172 and allosteric activated with a 100-fold increase in kinase activity (Hawley et al., 1996). In mammals, the typical kinases involved in canonical AMPK activation include liver kinase B1 (LKB1) and Ca2+/calmodulin-activated protein kinase kinase β (CaMKKβ). LKB1, also known as a potent tumor suppressor, is vital for the basal phosphorylation level of AMPK (Hardie et al., 2012). Genetic knockout of LKB1 impairs AMPK activation by AMP, indicating its critical role during energy deprivation (Hardie and Alessi, 2013). AMPK is also activated in response to calcium flux, which relies on the intact function of CaMKKβ. It is noteworthy that this alternative AMPK activation pathway is independent of any change in cellular AMP level (Hardie et al., 2012), and is most highlighted in the brain (Green et al., 2011).
AMPK Activators
In addition to cellular metabolic signal, AMPK can be activated by a variety of compounds. AICAR (5-amino-4-imidazolecarboxamide riboside-1-β-D-ribofuranoside) is a potent AMPK activator. As an adenosine analog, AICAR is taken in by adenosine transporters and converted into ZMP (5-aminoimidazole-4-carboxamide-1-β-D-furanosyl 5′-monophosphate). Similar to AMP, ZMP binds directly to AMPK and induces its allosteric activation (Wong et al., 2009). Natural products and related derivatives represent another class of AMPK activators. Metformin, derived from French lilac, is widely administrated as the first-line medication for type 2 diabetes. Although the involved mechanisms remain partially understood, it is clear that AMPK plays an important role in the benefits of metformin (Zhou et al., 2001). Resveratrol, isolated from grapes and red wine, is reported to activator AMPK and SIRT1, a NAD+-dependent protein deacetylase sharing crosstalk with AMPK (Price et al., 2012). Other natural AMPK activators include epigallocatechin gallate, capsaicin, curcumin, berberine, and so on (Hwang et al., 2005; Ejaz et al., 2009; Jeong et al., 2009).
AMPK on Cellular Metabolism and More
As a master cellular energy gauge, AMPK regulates lipid and glucose metabolism by phosphorylating downstream targets. Activated AMPK not only suppresses fatty acid synthesis by impairing acetyl-CoA carboxylase (ACC), but also facilitates lipid oxidation by boosting malonyl-CoA decarboxylase (MCD) (Zang et al., 2004; Zhang et al., 2009). Similar regulating pattern is also observed in glucose metabolism. By promoting the translocation of glucose transporter 4 (GLUT4), AMPK activation stimulates glucose uptake in muscle tissue (Jäger et al., 2007). In parallel, hepatic gluconeogenesis is inhibited by AMPK through decreased phosphoenolpyruvate carboxykinase (PEPCK) and glucose-6-phosphatase (G6Pase) transcription (Zhang et al., 2009). In general, AMPK activation leads to metabolic changes toward relieving energy deprivation.
In addition to energy balance, recent studies have suggested the participation of AMPK in autophagy, atherosclerosis, inflammation, and cancer, which shares crosstalk with H2S (Motoshima et al., 2006; Kim et al., 2011; O’neill and Hardie, 2013). The advances in AMPK researches have shed light on novel therapeutic potentials of AMPK activators and H2S donors, and provided modern understanding of metabolism. More details will be discussed in the following sections.
AMPK in H2S Pharmacology
Great efforts have been made to elucidate the roles of AMPK in H2S pharmacology, in which AMPK usually serves as a key mediator. Despite the fact that AMPK was reported to be inhibited by H2S in some circumstances (Zhang et al., 2012), it is generally accepted that H2S exerts its biological activity by activating AMPK. In this part we will review the recent discoveries on AMPK-H2S pharmacology.
AMPK in Cardioprotective Effects of H2S
According to latest Global Burden of Disease Study, ischemic heart disease remained as the global leading cause for death in 2010, accounting for 13.3% of total death worldwide (Lozano et al., 2013). A great number of patients are suffering from related diseases including ischemic heart failure and stable arrhythmia (McMurray et al., 2005). The beneficial effects of H2S on survival after cardiac arrest in mice was first reported in 2009. By i.v. application of Na2S, an exogenous H2S donor, 7 min following cardiac arrest, significant increase in survival rate was achieved compared with vehicle (Minamishima et al., 2009). The authors pointed out that the treatment effects of Na2S was associated with activated AMPK prosurvival signals. In addition, AMPK is also attributed to the cardioprotective effects of H2S in high fat diet-induced cardiac dysfunction and impaired left ventricular function by smoking (Zhou et al., 2014a; Barr et al., 2015).
Myocardial ischemia/reperfusion (I/R) injury is a complication of inflammation and oxidative damage encountered when restoring blood supply of ischemic regions. Previous studies have demonstrated that H2S protects from I/R injury by multiple mechanisms, including ameliorating oxidative stress, inflammation, and apoptosis (Sivarajah et al., 2009; Meng et al., 2015). Xie et al. (2015) reported that AMPK is a critical mediator in the effects of H2S donor ADT. By maintaining autophagy flux impaired by I/R, as evidenced by reduced LC3-II/LC3-I ratio and beclin-1 expression, H2S-activated AMPK significantly relieved myocardial I/R injury.
Post-conditioning (PC), defined as brief repeated periods of ischemia performed at the onset of reperfusion, has been proved to reduce I/R injury in both cardiomyocytes and coronary vascular endothelium cells (Vinten-Johansen et al., 2005). Nevertheless PC only exerts cardioprotection in young but not old hearts (Boengler et al., 2009). Chen et al. (2016) suggested that by exogenous application of H2S, the treatment effects of PC could be restored in isolated aged rat hearts and aged cardiomyocytes. In contrast to the study on I/R, stimulated AMPK promoted autophagy, which subsequently decreased apoptosis, reduced myocardial injury, and improved cardiac function. More studies regarding AMPK-H2S and autophagy will be discussed in the next section.
AMPK-Regulated Autophagy in H2S Pharmacology
Autophagy is a lysosome-dependent “self-engulfment” process in which cells digest their own cytosolic components to maintain metabolic homeostasis during starvation. Moreover, autophagy is tightly involved in cancer, cardiac and liver diseases (Levine and Kroemer, 2008). The autophagy pathway was originally discovered with Atg1/UNC-51-like kinase (ULK) 1 complex as an essential initiator, which also senses cellular nutrient status from the mammalian target of rapamycin (mTOR). Previous studies have established the prominent role of AMPK in regulating autophagy. One of the most described mechanism involves the suppression of mTOR pathway by AMPK, which promotes the formation of autophagosomes (Kim and Guan, 2015). On the other hand, AMPK directly phosphorylates several critical sites in ULK1 and subsequently induces its activation in autophagy and mitochondrial homeostasis (Hardie, 2011). By inhibiting mTOR and activating ULK1, AMPK regulates autophagy in response to nutritional signal (Mihaylova and Shaw, 2011).
The AMPK-autophagy pathway has been identified in multiple pharmacological functions of H2S. As described in the last section, H2S mitigates cardiac I/R injury and restores PC protection by regulating AMPK-mediated autophagy. Similar results were also observed in high glucose conditions. Vascular endothelial dysfunction induced by hyperglycemia impairs vasodilation and angiogenic function, leading to diabetic complications (Hink et al., 2001). Exogenous H2S markedly preserved arterial endothelial cells by reducing AMPK phosphorylation and inhibiting excessive autophagy (Liu et al., 2016). Furthermore, Kundu et al. (2014) suggested that H2S relieved renal matrix accumulation during hyperglycemia by LKB1/AMPK cascade. Intriguingly, as opposed to the previous study, AMPK was activated by H2S, leading to protective autophagy in glomerular endothelial cells (Kundu et al., 2014).
Hypertriglyceridemia is among the most common metabolic diseases and is proved as an independent risk factor for cardiovascular and cerebrovascular events (Chapman et al., 2011). Lower plasma H2S content was reported to be associated with dyslipidemia, indicating the potential regulation of H2S on serum triglyceride (Liu et al., 2006). Indeed, Sun et al. (2015) claimed that H2S reduced triglyceride and relieved non-alcoholic fatty liver disease in mice by activating AMPK and inhibiting mTOR. Consistent regulation of AMPK and autophagy by H2S was reported in inhibiting colon epithelial cell proliferation (Wu et al., 2012).
AMPK in H2S Protection against Diabetic Complications
Chronic hyperglycemia of diabetes leads to damage and dysfunction of multiple organs, including the kidney, heart, and blood vessels (American Diabetes Association, 2014). The anti-diabetic effects of AMPK activators are well-documented, and numerous evidences have indicated the mitigation of hyperglycemia and related complications by H2S (Suzuki et al., 2011; Szabo, 2012). Lee et al. (2012, 2017) suggested that H2S decreased protein synthesis, cellular hypertrophy, and matrix protein accumulation of renal cells under high glucose condition. Moreover, H2S inhibited mTOR activity, mRNA initiation and elongation by activating AMPK through CaMKKβ. Corresponding AMPK/mTOR regulation by H2S was also reported in cardiomyocyte protection from high glucose (Wei et al., 2014).
Vascular inflammation induced by hyperglycemia is associated with impaired insulin sensitivity and accelerated atherosclerosis (Basta et al., 2004; Paneni et al., 2013). Both L-cysteine, the endogenous precursor of H2S, and exogenous H2S donors successfully diminished high glucose-stimulated inflammatory cytokine secretion from monocytic cells, indicating the link between H2S and vascular inflammation (Jain et al., 2010). It was further supported that H2S relieved vascular inflammation via multiple mechanisms including activating AMPK (Manna and Jain, 2013). These findings might bring light on the therapeutic potentials of H2S against diabetic complications.
AMPK in Anti-inflammatory and Anti-oxidant Stress Properties of H2S
There have been controversies over the exact role of H2S in inflammation (Whiteman and Winyard, 2011). Despite distinct results obtained, it appears that the inconsistency in the pro- and anti-inflammatory effects of H2S might be attributed to models, doses, and sampling time (Hegde and Bhatia, 2011). It is generally acknowledged that H2S shows remarkable suppression on lipopolysaccharide-induced production of inflammatory cytokines both in macrophages and microglia cells (Hu et al., 2007; Whiteman et al., 2010). In parallel, the inhibition of inflammation by AMPK activation has been also been reported (O’neill and Hardie, 2013). Zhou et al. (2014b) found that the suppression of microglia inflammation by H2S was largely dependent on AMPK activation via CaMKKβ pathway, which was evidenced by multiple H2S donors, CBS overexpression, and AMPK knockdown.
Anemia of inflammation (AI) is the second prevalent anemia and a common complication in patients with chronic diseases (Weiss and Goodnough, 2005). Lower hemoglobin is associated with increased mortality in diseases such as heart failure (Horwich et al., 2002; O’Meara et al., 2006), cancer (Caro et al., 2001), and chronic kidney disease (Foley et al., 1996; Vlagopoulos et al., 2005). The pathological changes of AI include iron disturbance and erythroid system dysfunction (Nemeth and Ganz, 2014), of which iron dysregulation is the hallmark. Mounting evidences have indicated that elevated hepatic and circulating hepcidin, a liver-derived iron-regulating peptide, is tightly involved in the progression of AI (Ganz, 2003), making it an ideal target for AI treatment. We first reported that during inflammation H2S inhibited hepatic hepcidin, a critical factor in AI pathogenesis (Xin et al., 2016). Our recent investigation revealed that the effects of H2S was partially mediated by AMPK (Wang et al., 2017). What’s more, both pharmacological and genetic activation of AMPK, as well as metformin, ameliorated chronic AI in mice, which was supported by our clinical samples and independent groups (Kim et al., 2016; Wang et al., 2017). Interestingly, metformin was reported to increase H2S content in various mouse tissues (Wiliński et al., 2013). Much more work will be needed to broaden our understanding about the interaction among H2S, AMPK and metformin.
5′-Monophosphate-activated protein kinase also contributes to the effects of H2S and garlic products against oxidant stress. Han et al. (2011) claimed that ajoene, a garlic by-product, reduced oxidative injury and hepatic steatosis by stimulating LKB1/AMPK pathway. Consistent results were observed in H2S donors. Sodium hydrosulfide, an inorganic H2S donor, ameliorated oxidative stress and apoptosis via activating CaMKKβ/AMPK signaling, finally attenuating experimental aging process (Chen et al., 2017). Furthermore, reactive oxygen species production was diminished by H2S-induced AMPK activation in osteoblast cells treated with dexamethasone (Yang et al., 2014).
Current Research Gaps
It is of great importance to understand the specific mechanisms within H2S-induced AMPK activation. However, few studies have looked into this topic. Kundu colleagues claimed that H2S activated AMPK by LKB1 (see section “AMPK in Cardioprotective Effects of H2S”), while Lee colleagues (see section “AMPK-Regulated Autophagy in H2S Pharmacology”) and Zhou colleagues (see section “AMPK in H2S Protection against Diabetic Complications”) suggested CaMKKβ was indispensable for AMPK activation by H2S.
It is worth noting that instead of fully elucidating the potential mechanisms, all these studies mainly pointed out several key mediators in the H2S-AMPK pathway. Moreover, recent studies have revealed that H2S might also regulate protein functions in a more “direct” manner, such as by sulfhydration (Paul and Snyder, 2012) and forming polysulfides (Greiner et al., 2013; Kimura et al., 2013). Future studies may help better understand the role of these novel modifications in H2S-AMPK interactions.
Conclusion
In summary, this minireview provides novel insights into latest AMPK-mediated H2S pharmacology in various tissues and diseases (Figure 1). Despite substantial progress, there remains a long road toward complete understanding of AMPK-H2S interactions and application of H2S donors in clinical settings.
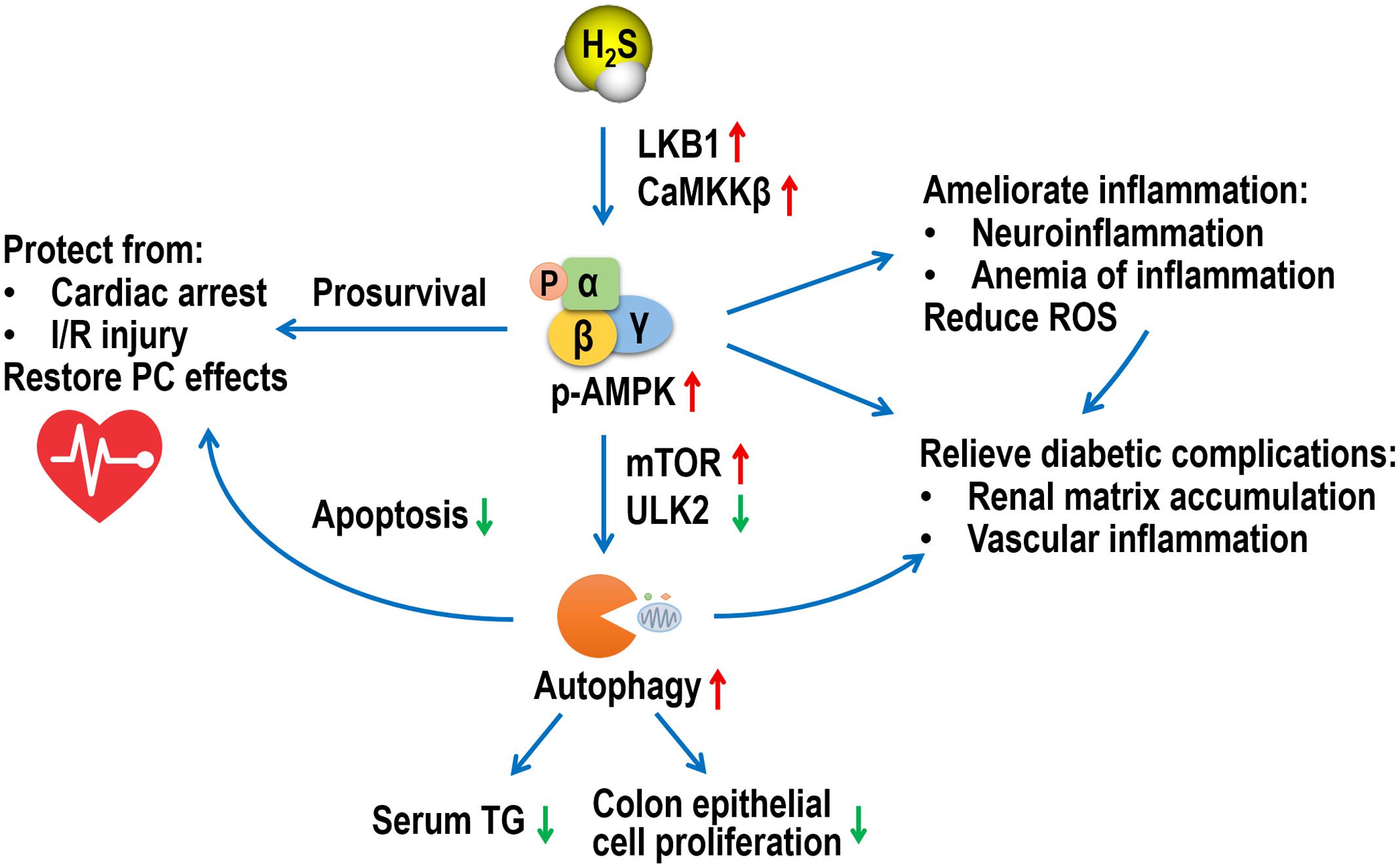
FIGURE 1. A schematic diagram of multiple roles of AMPK in the pharmacological functions of H2S. AMPK, adenosine 5′-monophosphate-activated protein kinase; H2S, hydrogen sulfide; LKB1, liver kinase B1; CaMKKβ, Ca2+/calmodulin-activated protein kinase kinase β; mTOR, mammalian target of rapamycin; ULK2, UNC-51-like kinase 2; TG, triglyceride; I/R, ischemia/reperfusion; PC, post-conditioning; ROS, reactive oxygen species.
Author Contributions
All authors listed have made substantial, direct and intellectual contributions to the work, and approved it for publication.
Funding
This work was supported by grants from Macao Science and Technology Development Fund (Grant No. FDCT/039/2016/A and FDCT/055/2016/A2).
Conflict of Interest Statement
The authors declare that the research was conducted in the absence of any commercial or financial relationships that could be construed as a potential conflict of interest.
References
Ahmad, A., Gerö, D., Olah, G., and Szabo, C. (2016). Effect of endotoxemia in mice genetically deficient in cystathionine-γ-lyase, cystathionine-β-synthase or 3-mercaptopyruvate sulfurtransferase. Int. J. Mol. Med. 38, 1683–1692. doi: 10.3892/ijmm.2016.2771
American Diabetes Association (2014). Diagnosis and classification of diabetes mellitus. Diabetes Care 37(Suppl. 1), S81–S90. doi: 10.2337/dc14-S081
Barr, L. A., Shimizu, Y., Lambert, J. P., Nicholson, C. K., and Calvert, J. W. (2015). Hydrogen sulfide attenuates high fat diet-induced cardiac dysfunction via the suppression of endoplasmic reticulum stress. Nitric Oxide 46, 145–156. doi: 10.1016/j.niox.2014.12.013
Basta, G., Schmidt, A. M., and De Caterina, R. (2004). Advanced glycation end products and vascular inflammation: implications for accelerated atherosclerosis in diabetes. Cardiovasc. Res. 63, 582–592. doi: 10.1016/j.cardiores.2004.05.001
Benavides, G. A., Squadrito, G. L., Mills, R. W., Patel, H. D., Isbell, T. S., Patel, R. P., et al. (2007). Hydrogen sulfide mediates the vasoactivity of garlic. Proc. Natl. Acad. Sci. U.S.A. 104, 17977–17982. doi: 10.1073/pnas.0705710104
Boengler, K., Schulz, R., and Heusch, G. (2009). Loss of cardioprotection with ageing. Cardiovasc. Res. 83, 247–261. doi: 10.1093/cvr/cvp033
Calvert, J. W., Elston, M., Nicholson, C. K., Gundewar, S., Jha, S., Elrod, J. W., et al. (2010). Genetic and pharmacologic hydrogen sulfide therapy attenuates ischemia-induced heart failure in mice. Circulation 122, 11–19. doi: 10.1161/CIRCULATIONAHA.109.920991
Caro, J. J., Salas, M., Ward, A., and Goss, G. (2001). Anemia as an independent prognostic factor for survival in patients with cancer. Cancer 91, 2214–2221. doi: 10.1002/1097-0142(20010615)91:12<2214::AID-CNCR1251>3.0.CO;2-P
Chapman, M. J., Ginsberg, H. N., Amarenco, P., Andreotti, F., Boren, J., Catapano, A. L., et al. (2011). Triglyceride-rich lipoproteins and high-density lipoprotein cholesterol in patients at high risk of cardiovascular disease: evidence and guidance for management. Eur. Heart J. 32, 1345–1361. doi: 10.1093/eurheartj/ehr112
Chen, J., Gao, J., Sun, W., Li, L., Wang, Y., Bai, S., et al. (2016). Involvement of exogenous H 2 S in recovery of cardioprotection from ischemic post-conditioning via increase of autophagy in the aged hearts. Int. J. Cardiol. 220, 681–692. doi: 10.1016/j.ijcard.2016.06.200
Chen, X., Zhao, X., Cai, H., Sun, H., Hu, Y., Huang, X., et al. (2017). The role of sodium hydrosulfide in attenuating the aging process via PI3K/AKT and CaMKKβ/AMPK pathways. Redox Biol. 12, 987–1003. doi: 10.1016/j.redox.2017.04.031
Du, J., Hui, Y., Cheung, Y., Bin, G., Jiang, H., Chen, X., et al. (2004). The possible role of hydrogen sulfide as a smooth muscle cell proliferation inhibitor in rat cultured cells. Heart Vessels 19, 75–80. doi: 10.1007/s00380-003-0743-7
Ejaz, A., Wu, D., Kwan, P., and Meydani, M. (2009). Curcumin inhibits adipogenesis in 3T3-L1 adipocytes and angiogenesis and obesity in C57/BL mice. J. Nutr. 139, 919–925. doi: 10.3945/jn.108.100966
Feliers, D., Lee, H. J., and Kasinath, B. S. (2016). Hydrogen sulfide in renal physiology and disease. Antioxid. Redox Signal. 25, 720–731. doi: 10.1089/ars.2015.6596
Fiorucci, S., Distrutti, E., Cirino, G., and Wallace, J. L. (2006). The emerging roles of hydrogen sulfide in the gastrointestinal tract and liver. Gastroenterology 131, 259–271. doi: 10.1053/j.gastro.2006.02.033
Foley, R. N., Parfrey, P. S., Harnett, J. D., Kent, G. M., Murray, D. C., and Barre, P. E. (1996). The impact of anemia on cardiomyopathy, morbidity, and mortality in end-stage renal disease. Am. J. Kidney Dis. 28, 53–61. doi: 10.1016/S0272-6386(96)90130-4
Ganz, T. (2003). Hepcidin, a key regulator of iron metabolism and mediator of anemia of inflammation. Blood 102, 783–788. doi: 10.1182/blood-2003-03-0672
Green, M. F., Anderson, K. A., and Means, A. R. (2011). Characterization of the CaMKKβ–AMPK signaling complex. Cell. Signal. 23, 2005–2012. doi: 10.1074/jbc.M115.684928
Greiner, R., Pálinkás, Z., Bäsell, K., Becher, D., Antelmann, H., Nagy, P., et al. (2013). Polysulfides link H2S to protein thiol oxidation. Antioxd. Redox Signal. 19, 1749–1765. doi: 10.1089/ars.2012.5041
Han, C. Y., Ki, S. H., Kim, Y. W., Noh, K., Lee, D. Y., Kang, B., et al. (2011). Ajoene, a stable garlic by-product, inhibits high fat diet-induced hepatic steatosis and oxidative injury through LKB1-dependent AMPK activation. Antioxid. Redox Signal. 14, 187–202. doi: 10.1089/ars.2010.3190
Hardie, D. G. (2011). AMPK and autophagy get connected. EMBO J. 30, 634–635. doi: 10.1038/emboj.2011.12
Hardie, D. G., and Alessi, D. R. (2013). LKB1 and AMPK and the cancer-metabolism link-ten years after. BMC Biol. 11:36. doi: 10.1186/1741-7007-11-36
Hardie, D. G., Ross, F. A., and Hawley, S. A. (2012). AMPK: a nutrient and energy sensor that maintains energy homeostasis. Nat. Rev. Mol. Cell Biol. 13, 251–262. doi: 10.1038/nrm3311
Hawley, S. A., Davison, M., Woods, A., Davies, S. P., Beri, R. K., Carling, D., et al. (1996). Characterization of the AMP-activated protein kinase kinase from rat liver and identification of threonine 172 as the major site at which it phosphorylates AMP-activated protein kinase. J. Biol. Chem. 271, 27879–27887. doi: 10.1074/jbc.271.44.27879
Hegde, A., and Bhatia, M. (2011). Hydrogen sulfide in inflammation: friend or foe? Inflamm. Allergy Drug Targets 10, 118–122. doi: 10.2174/187152811794776268
Hink, U., Li, H., Mollnau, H., Oelze, M., Matheis, E., Hartmann, M., et al. (2001). Mechanisms underlying endothelial dysfunction in diabetes mellitus. Circ. Res. 88, e14–e22. doi: 10.1161/01.RES.88.2.e14
Horwich, T. B., Fonarow, G. C., Hamilton, M. A., MacLellan, W. R., and Borenstein, J. (2002). Anemia is associated with worse symptoms, greater impairment in functional capacity and a significant increase in mortality in patients with advanced heart failure. J. Am. Coll. Cardiol. 39, 1780–1786. doi: 10.1016/S0735-1097(02)01854-5
Hu, L. F., Wong, P. T. H., Moore, P. K., and Bian, J. S. (2007). Hydrogen sulfide attenuates lipopolysaccharide-induced inflammation by inhibition of p38 mitogen-activated protein kinase in microglia. J. Neurochem. 100, 1121–1128. doi: 10.1111/j.1471-4159.2006.04283.x
Hwang, J.-T., Park, I.-J., Shin, J.-I., Lee, Y. K., Lee, S. K., Baik, H. W., et al. (2005). Genistein, EGCG, and capsaicin inhibit adipocyte differentiation process via activating AMP-activated protein kinase. Biochem. Biophys. Res. Commun. 338, 694–699. doi: 10.1016/j.bbrc.2005.09.195
Jäger, S., Handschin, C., Pierre, J. S., and Spiegelman, B. M. (2007). AMP-activated protein kinase (AMPK) action in skeletal muscle via direct phosphorylation of PGC-1α. Proc. Natl Acad. Sci. U.S.A. 104, 12017–12022. doi: 10.1073/pnas.0705070104
Jain, S. K., Bull, R., Rains, J. L., Bass, P. F., Levine, S. N., Reddy, S., et al. (2010). Low levels of hydrogen sulfide in the blood of diabetes patients and streptozotocin-treated rats causes vascular inflammation? Antioxid. Redox Signal. 12, 1333–1337. doi: 10.1089/ars.2009.2956
Jeong, H. W., Hsu, K. C., Lee, J.-W., Ham, M., Huh, J. Y., Shin, H. J., et al. (2009). Berberine suppresses proinflammatory responses through AMPK activation in macrophages. Am. J. Physiol. Endocrinol. Metab. 296, E955–E964. doi: 10.1152/ajpendo.90599.2008
Jung, E.-S., Park, S.-H., Choi, E.-K., Ryu, B.-H., Park, B.-H., Kim, D.-S., et al. (2014). Reduction of blood lipid parameters by a 12-wk supplementation of aged black garlic: a randomized controlled trial. Nutrition 30, 1034–1039. doi: 10.1016/j.nut.2014.02.014
Kahn, B. B., Alquier, T., Carling, D., and Hardie, D. G. (2005). AMP-activated protein kinase: ancient energy gauge provides clues to modern understanding of metabolism. Cell Metab. 1, 15–25. doi: 10.1016/j.cmet.2004.12.003
Kim, D.-K., Kim, Y.-H., Jung, Y. S., Kim, K.-S., Jeong, J.-H., Lee, Y.-S., et al. (2016). Orphan nuclear receptor SHP regulates iron metabolism through inhibition of BMP6-mediated hepcidin expression. Sci. Rep. 6:34630. doi: 10.1038/srep34630
Kim, J., Kundu, M., Viollet, B., and Guan, K.-L. (2011). AMPK and mTOR regulate autophagy through direct phosphorylation of Ulk1. Nat. Cell Biol. 13, 132–141. doi: 10.1038/ncb2152
Kim, Y. C., and Guan, K. L. (2015). mTOR: a pharmacologic target for autophagy regulation. J. Clin. Invest. 125, 25–32. doi: 10.1172/JCI73939
Kimura, H. (2011). Hydrogen sulfide: its production, release and functions. Amino Acids 41, 113–121. doi: 10.1007/s00726-010-0510-x
Kimura, H. (2013). Physiological role of hydrogen sulfide and polysulfide in the central nervous system. Neurochem. Int. 63, 492–497. doi: 10.1016/j.neuint.2013.09.003
Kimura, Y., Mikami, Y., Osumi, K., Tsugane, M., Oka, J., and Kimura, H. (2013). Polysulfides are possible H2S-derived signaling molecules in rat brain. FASEB J. 27, 2451–2457. doi: 10.1096/fj.12-226415
Kiss, L., Deitch, E. A., and Szabó, C. (2008). Hydrogen sulfide decreases adenosine triphosphate levels in aortic rings and leads to vasorelaxation via metabolic inhibition. Life Sci. 83, 589–594. doi: 10.1016/j.lfs.2008.08.006
Kundu, S., Pushpakumar, S., Khundmiri, S. J., and Sen, U. (2014). Hydrogen sulfide mitigates hyperglycemic remodeling via liver kinase B1-adenosine monophosphate-activated protein kinase signaling. Biochim. Biophys. Acta 1843, 2816–2826. doi: 10.1016/j.bbamcr.2014.08.005
Lee, H. J., Lee, D. Y., Mariappan, M. M., Feliers, D., Ghosh-Choudhury, G., Abboud, H. E., et al. (2017). Hydrogen sulfide inhibits high glucose-induced NADPH oxidase 4 expression and matrix increase by recruiting inducible nitric oxide synthase in kidney proximal tubular epithelial cells. J. Biol. Chem. 292, 5665–5675. doi: 10.1074/jbc.M116.766758
Lee, H. J., Mariappan, M. M., Feliers, D., Cavaglieri, R. C., Sataranatarajan, K., Abboud, H. E., et al. (2012). Hydrogen sulfide inhibits high glucose-induced matrix protein synthesis by activating AMP-activated protein kinase in renal epithelial cells. J. Biol. Chem. 287, 4451–4461. doi: 10.1074/jbc.M111.278325
Levine, B., and Kroemer, G. (2008). Autophagy in the pathogenesis of disease. Cell 132, 27–42. doi: 10.1016/j.cell.2007.12.018
Li, L., Rose, P., and Moore, P. K. (2011). Hydrogen sulfide and cell signaling. Annu. Rev. Pharmacol. Toxicol. 51, 169–187. doi: 10.1146/annurev-pharmtox-010510-100505
Li, X., Wang, L., Zhou, X. E., Ke, J., de Waal, P. W., Gu, X., et al. (2015). Structural basis of AMPK regulation by adenine nucleotides and glycogen. Cell Res. 25, 50–66. doi: 10.1038/cr.2014.150
Liu, J., Wu, J., Sun, A., Sun, Y., Yu, X., Liu, N., et al. (2016). Hydrogen sulfide decreases high glucose/palmitate-induced autophagy in endothelial cells by the Nrf2-ROS-AMPK signaling pathway. Cell Biosci. 6:33. doi: 10.1186/s13578-016-0099-1
Liu, Y., Mi, J., Ding, Y. G., and Du, J. B. (2006). Survey of plasma lipid value in children in Beijing area and the changes of gaseous molecule-hydrogen sulfide in the ones with dyslipidemia. Beijing Da Xue Xue Bao 38, 146–150.
Lozano, R., Naghavi, M., Foreman, K., Lim, S., Shibuya, K., Aboyans, V., et al. (2013). Global and regional mortality from 235 causes of death for 20 age groups in 1990 and 2010: a systematic analysis for the Global Burden of Disease Study 2010. Lancet 380, 2095–2128. doi: 10.1016/S0140-6736(12)61728-0
Manna, P., and Jain, S. K. (2013). L-cysteine and hydrogen sulfide increase PIP3 and AMPK/PPARγ expression and decrease ROS and vascular inflammation markers in high glucose treated human U937 monocytes. J. Cell. Biochem. 114, 2334–2345. doi: 10.1002/jcb.24578
McMurray, J., Køber, L., Robertson, M., Dargie, H., Colucci, W., Lopez-Sendon, J., et al. (2005). Antiarrhythmic effect of carvedilol after acute myocardial infarction: results of the Carvedilol Post-Infarct Survival Control in Left Ventricular Dysfunction (CAPRICORN) trial. J. Am. Coll. Cardiol. 45, 525–530. doi: 10.1016/j.jacc.2004.09.076
Meng, G., Wang, J., Xiao, Y., Bai, W., Xie, L., Shan, L., et al. (2015). GYY4137 protects against myocardial ischemia and reperfusion injury by attenuating oxidative stress and apoptosis in rats. J. Biomed. Res. 29, 203–213. doi: 10.7555/JBR.28.20140037
Miao, L., Shen, X., Whiteman, M., Xin, H., Shen, Y., Xin, X., et al. (2016). Hydrogen sulfide mitigates myocardial infarction via promotion of mitochondrial biogenesis-dependent M2 polarization of macrophages. Antioxid. Redox Signal. 25, 268–281. doi: 10.1089/ars.2015.6577
Mihaylova, M. M., and Shaw, R. J. (2011). The AMPK signalling pathway coordinates cell growth, autophagy and metabolism. Nat. Cell Biol. 13, 1016–1023. doi: 10.1038/ncb2329
Minamishima, S., Bougaki, M., Sips, P. Y., De Yu, J., Minamishima, Y. A., Elrod, J. W., et al. (2009). Hydrogen sulfide improves survival after cardiac arrest and cardiopulmonary resuscitation via a nitric oxide synthase 3–dependent mechanism in mice. Circulation 120, 888–896. doi: 10.1161/CIRCULATIONAHA.108.833491
Motoshima, H., Goldstein, B. J., Igata, M., and Araki, E. (2006). AMPK and cell proliferation–AMPK as a therapeutic target for atherosclerosis and cancer. J. Physiol. 574, 63–71. doi: 10.1113/jphysiol.2006.108324
Nemeth, E., and Ganz, T. (2014). Anemia of inflammation. Hematology 28, 671–681. doi: 10.1016/j.hoc.2014.04.005
O’Meara, E., Clayton, T., McEntegart, M. B., McMurray, J. J., Lang, C. C., Roger, S. D., et al. (2006). Clinical correlates and consequences of anemia in a broad spectrum of patients with heart failure: results of the Candesartan in heart failure: assessment of reduction in mortality and morbidity (CHARM) program. Circulation 113, 986–994. doi: 10.1161/CIRCULATIONAHA.105.582577
O’neill, L. A., and Hardie, D. G. (2013). Metabolism of inflammation limited by AMPK and pseudo-starvation. Nature 493, 346–355. doi: 10.1038/nature11862
Paneni, F., Beckman, J. A., Creager, M. A., and Cosentino, F. (2013). Diabetes and vascular disease: pathophysiology, clinical consequences, and medical therapy: part I. Eur. Heart J. 34, 2436–2443. doi: 10.1093/eurheartj/eht149
Papapetropoulos, A., Pyriochou, A., Altaany, Z., Yang, G., Marazioti, A., Zhou, Z., et al. (2009). Hydrogen sulfide is an endogenous stimulator of angiogenesis. Proc. Natl. Acad. Sci. U.S.A. 106, 21972–21977. doi: 10.1073/pnas.0908047106
Paul, B. D., and Snyder, S. H. (2012). H2S signalling through protein sulfhydration and beyond. Nat. Rev. Mol. Cell Biol. 13, 499–507. doi: 10.1038/nrm3391
Polhemus, D. J., and Lefer, D. J. (2014). Emergence of hydrogen sulfide as an endogenous gaseous signaling molecule in cardiovascular disease. Circ. Res. 114, 730–737. doi: 10.1161/CIRCRESAHA.114.300505
Price, N. L., Gomes, A. P., Ling, A. J., Duarte, F. V., Martin-Montalvo, A., North, B. J., et al. (2012). SIRT1 is required for AMPK activation and the beneficial effects of resveratrol on mitochondrial function. Cell Metab. 15, 675–690. doi: 10.1016/j.cmet.2012.04.003
Ried, K. (2016). Garlic lowers blood pressure in hypertensive individuals, regulates serum cholesterol, and stimulates immunity: an updated meta-analysis and review. J. Nutr. 146, 389S–396S. doi: 10.3945/jn.114.202192
Shibuya, N., Tanaka, M., Yoshida, M., Ogasawara, Y., Togawa, T., Ishii, K., et al. (2009). 3-Mercaptopyruvate sulfurtransferase produces hydrogen sulfide and bound sulfane sulfur in the brain. Antioxid. Redox Signal. 11, 703–714. doi: 10.1089/ars.2008.2253
Sivarajah, A., Collino, M., Yasin, M., Benetti, E., Gallicchio, M., Mazzon, E., et al. (2009). Anti-apoptotic and anti-inflammatory effects of hydrogen sulfide in a rat model of regional myocardial I/R. Shock 31, 267–274. doi: 10.1097/SHK.0b013e318180ff89
Song, K., Wang, F., Li, Q., Shi, Y.-B., Zheng, H.-F., Peng, H., et al. (2014). Hydrogen sulfide inhibits the renal fibrosis of obstructive nephropathy. Kidney Int. 85, 1318–1329. doi: 10.1038/ki.2013.449
Sun, L., Zhang, S., Yu, C., Pan, Z., Liu, Y., Zhao, J., et al. (2015). Hydrogen sulfide reduces serum triglyceride by activating liver autophagy via the AMPK-mTOR pathway. Am. J. Physiol. Endocrinol. Metab. 309, E925–E935. doi: 10.1152/ajpendo.00294.2015
Suzuki, K., Olah, G., Modis, K., Coletta, C., Kulp, G., Gerö, D., et al. (2011). Hydrogen sulfide replacement therapy protects the vascular endothelium in hyperglycemia by preserving mitochondrial function. Proc. Natl. Acad. Sci. U.S.A. 108, 13829–13834. doi: 10.1073/pnas.1105121108
Szabo, C. (2012). Roles of hydrogen sulfide in the pathogenesis of diabetes mellitus and its complications. Antioxid. Redox Signal. 17, 68–80. doi: 10.1089/ars.2011.4451
Vinten-Johansen, J., Yellon, D. M., and Opie, L. H. (2005). A simple, clinically applicable procedure to improve revascularization in acute myocardial infarction. Circulation 112, 2085–2088. doi: 10.1161/CIRCULATIONAHA.105.569798
Vlagopoulos, P. T., Tighiouart, H., Weiner, D. E., Griffith, J., Pettitt, D., Salem, D. N., et al. (2005). Anemia as a risk factor for cardiovascular disease and all-cause mortality in diabetes: the impact of chronic kidney disease. J. Am. Soc. Nephrol. 16, 3403–3410. doi: 10.1681/ASN.2005030226
Wang, M., Xin, H., Tang, W., Li, Y., Zhang, Z., Fan, L., et al. (2017). AMPK serves as a therapeutic target against anemia of inflammation. Antioxid. Redox Signal. 27, 251–268. doi: 10.1089/ars.2016.6846
Wang, R. (2012). Physiological implications of hydrogen sulfide: a whiff exploration that blossomed. Physiol. Rev. 92, 791–896. doi: 10.1152/physrev.00017.2011
Wei, W.-B., Hu, X., Zhuang, X.-D., Liao, L.-Z., and Li, W.-D. (2014). GYY4137, a novel hydrogen sulfide-releasing molecule, likely protects against high glucose-induced cytotoxicity by activation of the AMPK/mTOR signal pathway in H9c2 cells. Mol. Cell. Biochem. 389, 249–256. doi: 10.1007/s11010-013-1946-6
Weiss, G., and Goodnough, L. T. (2005). Anemia of chronic disease. N. Engl. J. Med. 352, 1011–1023. doi: 10.1056/NEJMra041809
Whiteman, M., Li, L., Rose, P., Tan, C.-H., Parkinson, D. B., and Moore, P. K. (2010). The effect of hydrogen sulfide donors on lipopolysaccharide-induced formation of inflammatory mediators in macrophages. Antioxid. Redox Signal. 12, 1147–1154. doi: 10.1089/ars.2009.2899
Whiteman, M., and Winyard, P. G. (2011). Hydrogen sulfide and inflammation: the good, the bad, the ugly and the promising. Expert Rev. Clin. Pharmacol. 4, 13–32. doi: 10.1586/ecp.10.134
Wiliński, B., Wiliński, J., Somogyi, E., Piotrowska, J., and Opoka, W. (2013). Metformin raises hydrogen sulfide tissue concentrations in various mouse organs. Pharmacol. Rep. 65, 737–742. doi: 10.1016/S1734-1140(13)71053-3
Wong, A. K., Howie, J., Petrie, J. R., and Lang, C. C. (2009). AMP-activated protein kinase pathway: a potential therapeutic target in cardiometabolic disease. Clin. Sci. 116, 607–620. doi: 10.1042/CS20080066
Wu, Y. C., Wang, X. J., Yu, L., Chan, F. K., Cheng, A. S., Yu, J., et al. (2012). Hydrogen sulfide lowers proliferation and induces protective autophagy in colon epithelial cells. PLOS ONE 7:e37572. doi: 10.1371/journal.pone.0037572
Xie, H., Xu, Q., Jia, J., Ao, G., Sun, Y., Hu, L., et al. (2015). Hydrogen sulfide protects against myocardial ischemia and reperfusion injury by activating AMP-activated protein kinase to restore autophagic flux. Biochem. Biophys. Res. Commun. 458, 632–638. doi: 10.1016/j.bbrc.2015.02.017
Xin, H., Wang, M., Tang, W., Shen, Z., Miao, L., Wu, W., et al. (2016). Hydrogen sulfide attenuates inflammatory hepcidin by reducing IL-6 secretion and promoting SIRT1-mediated STAT3 deacetylation. Antioxid. Redox Signal. 24, 70–83. doi: 10.1089/ars.2015.6315
Yang, G., Wu, L., Jiang, B., Yang, W., Qi, J., Cao, K., et al. (2008). H2S as a physiologic vasorelaxant: hypertension in mice with deletion of cystathionine γ-lyase. Science 322, 587–590. doi: 10.1126/science.1162667
Yang, M., Huang, Y., Chen, J., Chen, Y.-L., Ma, J.-J., and Shi, P.-H. (2014). Activation of AMPK participates hydrogen sulfide-induced cyto-protective effect against dexamethasone in osteoblastic MC3T3-E1 cells. Biochem. Biophys. Res. Commun. 454, 42–47. doi: 10.1016/j.bbrc.2014.10.033
Yu, H., Hirshman, M. F., Fujii, N., Pomerleau, J. M., Peter, L. E., and Goodyear, L. J. (2006). Muscle-specific overexpression of wild type and R225Q mutant AMP-activated protein kinase gamma3-subunit differentially regulates glycogen accumulation. Am. J. Physiol. Endocrinol. Metab. 291, E557–E565. doi: 10.1152/ajpendo.00073.2006
Zang, M., Zuccollo, A., Hou, X., Nagata, D., Walsh, K., Herscovitz, H., et al. (2004). AMP-activated protein kinase is required for the lipid-lowering effect of metformin in insulin-resistant human HepG2 cells. J. Biol. Chem. 279, 47898–47905. doi: 10.1074/jbc.M408149200
Zhang, B. B., Zhou, G., and Li, C. (2009). AMPK: an emerging drug target for diabetes and the metabolic syndrome. Cell Metab. 9, 407–416. doi: 10.1016/j.cmet.2009.03.012
Zhang, L., Yang, G., Untereiner, A., Ju, Y., Wu, L., and Wang, R. (2012). Hydrogen sulfide impairs glucose utilization and increases gluconeogenesis in hepatocytes. Endocrinology 154, 114–126. doi: 10.1210/en.2012-1658
Zhou, G., Myers, R., Li, Y., Chen, Y., Shen, X., Fenyk-Melody, J., et al. (2001). Role of AMP-activated protein kinase in mechanism of metformin action. J. Clin. Invest. 108, 1167–1174. doi: 10.1172/JCI13505
Zhou, X., An, G., and Chen, J. (2014a). Hydrogen sulfide improves left ventricular function in smoking rats via regulation of apoptosis and autophagy. Apoptosis 19, 998–1005.
Keywords: hydrogen sulfide, AMPK, pharmacology, cardiovascular, autophagy, inflammation, oxidative stress
Citation: Wang M, Tang W and Zhu YZ (2017) An Update on AMPK in Hydrogen Sulfide Pharmacology. Front. Pharmacol. 8:810. doi: 10.3389/fphar.2017.00810
Received: 23 May 2017; Accepted: 26 October 2017;
Published: 08 November 2017.
Edited by:
Junbao Du, Peking University First Hospital, ChinaCopyright © 2017 Wang, Tang and Zhu. This is an open-access article distributed under the terms of the Creative Commons Attribution License (CC BY). The use, distribution or reproduction in other forums is permitted, provided the original author(s) or licensor are credited and that the original publication in this journal is cited, in accordance with accepted academic practice. No use, distribution or reproduction is permitted which does not comply with these terms.
*Correspondence: Yi Zhun Zhu, eXp6aHVAbXVzdC5lZHUubW8=
†These authors have contributed equally to this work.