- 1Department of Clinical Pharmacy and Pharmacology, University of Dhaka, Dhaka, Bangladesh
- 2Department of Pharmaceutical Chemistry, University of Dhaka, Dhaka, Bangladesh
Thymoquinone (TQ), the main bioactive component of Nigella sativa, has been found to exhibit anticancer effects in numerous preclinical studies. Due to its multitargeting nature, TQ interferes in a wide range of tumorigenic processes and counteracts carcinogenesis, malignant growth, invasion, migration, and angiogenesis. Moreover, TQ can specifically sensitize tumor cells toward conventional cancer treatments (e.g., radiotherapy, chemotherapy, and immunotherapy) and simultaneously minimize therapy-associated toxic effects in normal cells. In this review, we summarized the adjuvant potential of TQ as observed in various in vitro and in vivo animal models and discussed the pharmacological properties of TQ to rationalize its supplementary role in potentiating the efficacy of standard therapeutic modalities namely surgery, radiotherapy, chemotherapy, and immunotherapy. Altogether, we suggest further comprehensive evaluation of TQ in preclinical and clinical levels to delineate its implied utility as a novel complementary adjuvant therapy for cancer treatment.
Introduction
For the last five decades, large-scale “war on cancer” leads to significant progress in the development of new therapeutic options and more in-depth insights about various malignancies. Despite the emergence of numerous treatment strategies such as chemotherapeutic agents, small molecule inhibitors, specific gene, or protein targeting so-called smart drugs, and immunotherapies that are found to be effective in the treatment of some form of cancers (e.g., childhood leukemia, human epidermal growth factor receptor 2-positive breast cancer), cancer death rate, in general, has not changed substantially (only 5% since 1950; Sung et al., 2012). According to Centers for Disease control and Prevention (CDC), cancer is the second leading cause of death in US (591,699 deaths registered in 2015–2016), almost catching up heart diseases (https://www.cdc.gov/nchs/fastats/leading-causes-of-death.htm). Several underlying factors have been identified for failures of current cancer therapies, and among these factors, intratumoral heterogeneity is the most prominent. Targeting a particular gene, gene product, or signaling pathway can only eliminate a specific group of cells from the tumor, other genetically distinct variants can easily escape from that treatment and start developing tumor at surrounding area or may metastasize to distant sites. Due to this fact, most cancer treatment involves combination therapies where each drug works by different mechanisms and thus diminishing the chance of developing resistance.
Thymoquinone (2-methyl-5-isopropyl-1, 4-benzoquinone; TQ), a monoterpene, is the main active ingredient of the volatile oil of Nigella sativa L. (NS) (family Renunculaceae) which is familiar as black cumin or black seed (Salomi et al., 1991). Black seed is traditionally used both as condiment and natural medicine in many societies including Indian subcontinents and Arabian countries. Ayurvedic and Unani medicinal systems have been recommending to use black seed oil for the treatment of various human diseases such as bronchial asthma, dysentery, headache, gastrointestinal problems, eczema, hypertension, and obesity. Some recent clinical studies have found potent anti-inflammatory and antioxidant effects of oral NS extracts (Dehkordi and Kamkhah, 2008; Al-Jenoobi et al., 2010). Hence, the usefulness of black cumin seed in cancer prevention and treatment is now more than a speculation. TQ is first isolated from NS extracts in 1963 by El-Dakhakhany, since then diverse pharmacological properties of TQ have been reported from various studies (Salomi et al., 1991; Dajani et al., 2016). Numerous preclinical studies have been performed to determine the anticancer effects of TQ where it has exhibited selective cytotoxicity for human cancer cells (Gali-Muhtasib et al., 2004). In addition to its cell death and tumor growth inhibitory activities, TQ is found to interfere with other tumorigenic processes including angiogenesis, invasion, and metastasis (Peng et al., 2013; Khan et al., 2015). Furthermore, TQ can sensitize cancer cells to conventional chemotherapy and radiotherapy by modulating the resistance mechanisms (Velho-Pereira et al., 2011; Zhang et al., 2016). Multiple targets (e.g., carcinogen metabolizing enzymes, transcription factors, cell cycle regulatory proteins, etc.) of TQ have been identified that are somehow involved in tumorigenesis or development of drug resistance (Kundu et al., 2014b) (Figure 1). Though treatment with TQ alone has shown antitumor efficacy in several in vitro and in vivo studies as mentioned in details in a recent review (Majdalawieh et al., 2017), the lower efficacy (Effenberger et al., 2010) and poor bioavailability (Ganea et al., 2010; Elmowafy et al., 2016) of TQ is the primary bottleneck for considering it as the primary therapeutic agent. Therefore, in this review, we proposed the potential role of TQ as an adjuvant therapy with different types of conventional cancer treatments namely surgery, radiotherapy, chemotherapy, and immunotherapy either to prevent carcinogenesis or to potentiate the efficiency of conventional therapeutic modalities.
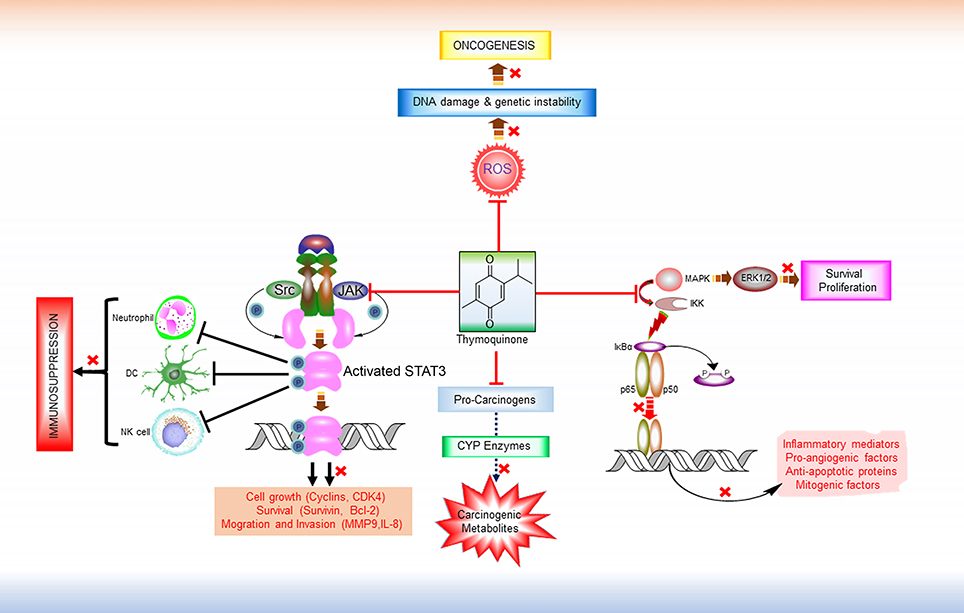
Figure 1. Major Anti-tumorigenic pharmacological properties of thymoquinone. TQ is a multi-targeting anticancer molecule. It prevents the formation of carcinogenic metabolites from pro-carcinogens by inhibiting CYP enzymes. By virtue of its free radical scavenging properties, TQ can inhibit ROS-mediated DNA damage induction and genetic instability and thereby prevent tumorigenesis. TQ also exhibits anti-proliferative and anti-survival effects by interfering in the MAPK/ERK pathway. In addition, TQ is found to modulate the activity of various transcription factors like NF-κB and STAT3. IKKs activates NF-κB by inducing the phosphorylation and proteasomal degradation of NF-κB inhibitor IκBa. The resulting free NF-κB (e.g., heterodimer of p65 and p50 subunits) then translocates to the nucleus and activate the transcription of various target genes that encodes numerous inflammatory mediators, pro-angiogenic factors, anti-apoptotic proteins. By targeting IKKs and thus inhibiting NF-κB activation, TQ shows anti-inflammatory, anti-angiogenic, and pro-apoptotic effects. Persistent STAT3 activity is a common feature in various malignancies. Overactive STAT3 leads to the dysregulation of immune response in tumor microenvironment by interfering in the proliferation and activation of various immune cells (e.g., NK cells, Neutrophils), maturation of DC, activation of tumor-antigen-specific CD8+ T cells, and differentiation of plasma cells. TQ has shown inhibitory effect on both the constitutive and ligand-induced activation of these transcription factors by disrupting their upstream signaling pathways, and thereby might reverse immune suppression and potentiate the efficacy of immunotherapeutic agents. ROS, Reactive oxygen species; MAPK, Mitogen-activated protein kinase; ERK 1/2, extracellular signal-regulated protein kinase 1 and 2; IKK, IκB kinases; Src, Non-receptor tyrosine kinase (Sarcoma-family kinases); JAK, Janus activated kinase; STAT3, Signal transducer and activator of transcription 3; DC, Dendritic cell; NK Cell, Natural killer cells.
Pharmacological Properties of TQ Indicates its Potential Role as an Adjuvant Therapy for Surgery
Though surgical tumor removal remains the first option for most malignancies (~60% of all cancer patients have to go through surgical procedures; Demicheli et al., 2008), many cancers reappear after surgery and start proliferating more aggressively. Another unavoidable consequence of surgical trauma is induction of metastatic potential in the microscopic tumors that are not eliminated through tumor resection (Ceelen et al., 2007, 2014). Numerous mitogenic and pro-angiogenic factors, such as transforming growth factor beta (TGF-β), fibroblast growth factor (FGF), vascular endothelial growth factor (VEGF), epidermal growth factor (EGF)-like growth factors, endostatin etc., were found in the wound fluids which explains the stimulation of cancer cell proliferation and neoangiogenesis during post-surgery wound healing period (Maniwa et al., 1998; Abramovitch et al., 1999; Wu et al., 2003). Therefore, several pre-operative and post-operative adjuvant therapies are administered in order to shrink the tumor into an operable stage and to prevent spreading of cancer cells from primary tumor sites. Post-operative therapies are important to eradicate the residual cancer cells (microscopic circulating tumor cells escaped from surgery) and to counteract the surgical trauma-induced pro-angiogenic and metastatic signaling pathways. Unfortunately, the current neoadjuvant therapies (mostly radiation and chemotherapy) are unable to elicit such ideal therapeutic efficacies and in many cases deteriorate patient quality of life due to unavoidable adverse effects. Therefore, novel multi-targeting, safe, and effective neoadjuvant treatments are urgently needed to improve the present standard of surgical tumor resection.
Treatment with TQ showed significant attenuation of tumorigenic signaling, including those mediated by TGF-β, VEGF, FGF, EGF, and several other pro-mitogenic, angiogenic, and metastatic factors, with a consequent dose-dependent inhibition of cancer cell growth, migration, and invasion (Yi et al., 2008; Ahmad et al., 2013; Khan et al., 2015; Rajput et al., 2015). It has also been reported that TQ can counteract the trauma-induced chemotaxis of circulating malignant cells and their epithelial to mesenchymal transition (EMT; Badr et al., 2011b; Ahmad et al., 2013; Khan et al., 2015). Moreover, TQ is found to interfere in the activation of various transcription factors including nuclear factor erythroid-related factor-2 (Nrf-2), nuclear factor-kappaB (NF-κB), signal transducer and activator of transcription-3 (STAT-3) that are responsible for the transcriptional activation of genes encoding proteins involved in cell proliferation, angiogenesis, and metastasis (Kundu et al., 2014c; Zhang et al., 2016). Overall, TQ has favorable pharmacological properties to counteract surgery-associated tumor invasion and metastasis. Although no direct study has been conducted to determine the usefulness of TQ along with cancer surgery, emerging evidences warrant preclinical and clinical evaluation of TQ as a pre-operative and/or post-operative neoadjuvant therapy.
TQ with a Dual Mode of Action: Radioprotection and Radiosensitization
Radiotherapy is the most widely utilized therapeutic modality in cancer management, almost 50% of all cancer patients receive this therapy in one form or another during their course of illness (Delaney et al., 2005). In many cases when tumors are inoperable, radiation is the main alternative to restrain the disease progression. Though radiation therapy is highly effective in tumor cell eradication, its success in cancer treatment is restricted by some inherent limitations, particularly the deleterious effect to surrounding normal tissues and stimulation of cancer cells adaptive responses to counteract the damage process. Since radiotherapy is generally applied in a course of multiple fractions, cancer cells that survived after initial cycles acquire resistance through multiple cellular mechanisms including activation of NF-κB, phosphatidylinositol 3-kinase (PI3K), protein kinase B (Akt), mammalian target of rapamycin (mTOR), and become less responsive to the later cycles of radiotherapy and/or chemotherapy (Baskar et al., 2014). Higher doses of ionizing radiation can effectively kill all tumor cells irrespective of cancer types and resistance status; however, application of such radiation beam may severely impact on normal cells. Therefore, current efforts in radiation research have aimed to devise strategies that will make tumor tissue more sensitive to ionizing radiation while protecting normal cells.
To maximize the benefit, radiotherapy is often supplemented with either radiosensitizers to intensify the radiation-induced cytotoxicity through augmenting biomolecular damage (e.g., DNA damaging agents) or radioprotectors to mitigate the deleterious effect of ionizing radiation in normal tissues mostly by scavenging highly reactive free radicals (e.g., antioxidants). Currently available radiosensitizers are unable to exhibit tumor cell specific radiation sensitization unless administered through targeted delivery. Similarly, radioprotectors inextricably scavenge the ionizing radiation-induced reactive free radicals and interfere in the efficacy of radiotherapy. Therefore, we need an adjuvant therapy that can simultaneously exert radiosensitization in cancer cells and radioprotection in healthy cells. In this regard, TQ can be an ideal candidate. Reelma et al. confirmed that TQ can enhance the cytotoxic efficacy of radiation through modulation of cell cycle and apoptosis (Velho-Pereira et al., 2011). Another study reported the prevention of radiation-induced metastatic progression of breast cancer cells by TQ through restoration of TGF-β (Rajput et al., 2015). Moreover, it has been found that TQ can directly modulate the activation of several signal transduction pathways including PI3K-Akt-mTOR that are frequently upregulated in various cancers and confer resistance to radiotherapy (Baskar et al., 2014; Kundu et al., 2014b). Radio-protective effect of TQ or Nigella sativa seed extracts was also observed in some in vivo studies (Velho-Pereira et al., 2012; Orhon et al., 2016). Results from those studies showed that animal treated with macerated extracts of Nigella sativa L. seeds (contains TQ along with other components) experienced less damage in their liver, spleen, brain, and intestines upon exposure to radiation. Another recently published work demonstrated the rescue of T-lymphocytes by TQ from gamma irradiation-induced apoptosis (Guida et al., 2016). From mechanistic point of view, these radioprotective effect of TQ is mainly mediated through its free radical scavenging ability and anti-oxidant properties (Velho-Pereira et al., 2012). Collectively, TQ can be a suitable adjuvant therapy for radiation treatment of cancer. Further studies are required to determine the differential dose and treatment schedule of TQ and radiation to achieve the best therapeutic outcome.
Chemo-Potentiating Role of TQ
A wide variety of chemotherapeutic drugs (more than 100) have been used in the treatment of different malignancies since 1940s. Despite the advent of numerous highly efficient cytotoxic agents, the overall rate of cancer-related death barely reduced in these 70 years of extensive researches and development. Some major drawbacks of currently used anticancer chemotherapy include non-specific cytotoxicity which results in bone marrow suppression and other organ toxicity and development of drug resistance by cancer cells. An augmented drug efflux, alteration of the molecular target, increased repair of drug-induced DNA damage, activation/suppression of signaling pathways leading to an upregulation of survival molecules and avoidance of apoptosis have been reported to be the most prominent reasons of tumor cell recalcitrance against chemotherapy (Baguley, 2010; Zahreddine and Borden, 2013; Alfarouk et al., 2015). Furthermore, heterogeneous nature of cancer cells is another hurdle that curbs the efficacy of targeted drug delivery or aiming a particular cancer-specific tumorigenic pathways. Hence, a suitable adjuvant along with the novel treatment approaches is obviously the Holy Grail for cancer patients.
There are ample evidences (mostly preclinical studies) showing that TQ, alone or in combination with other main course chemotherapeutic agents, can significantly impede cancer progression and synergistically reduce tumor burden in various malignancies through alteration of multiple tumorigenic pathways (Table 1). The administration of Nigella sativa seed extract enriched with TQ suppressed mice skin papillomagenesis when applied topicall (Salomi et al., 1991). In a similar fashion, 7,12-dimethylbenz[a]anthracene DMBA-induced hamster buccal squamous cell carcinoma burden was lessened when TQ was administered by gavage (Rajkamal et al., 2010). A significant attenuation has been reported in breast, gastric, and colon cancer xenografts after intra peritoneal administration of TQ (Gali-Muhtasib et al., 2008; Lei et al., 2012; Woo et al., 2013). TQ, when administered intraperitoneally suppressed the formation of aberrant crypt foci and colon adenoma burden in Balb/c mice (Gali-Muhtasib et al., 2008) and also elicited a reduction in the incidence and multiplicity of colon tumors in Wister rats both before or after treatment with 1,2-dimethyl hydrazine (Jrah-Harzallah et al., 2013). A decrease in the number of large polyps in the intestine of adenomatous polyposis coli (APC)min+ mice was reported by Lang et al. after oral feeding of TQ (Lang et al., 2013). The key liver enzymes namely, such as aspartate amino- transferase, alanine aminotransaminase, alkaline phosphatase, and lactate dehydrogenase exhibited reduced activity and the average number of hepatic nodules in rats treated with N-nitrosodiethylamine (NDEA) was decreased after both pre- and post-treatment with TQ. The expression of several cell proliferation markers, such as cyclin D1, cyclin E, proliferating cell nuclear antigen (PCNA), and Ki67 was also declined (Raghunandhakumar et al., 2013). A considerable decrease in the incidence and multiplicity of forestomach tumors and fibrosarcoma development in Swiss albino mice followed by treatment with benzo[α]pyrene (B[α]P) and 20-methylcholanthrene, respectively, was reported after addition of TQ in drinking water (Badary et al., 1999; Badary and Gamal El-Din, 2001). Intratumoral injection of TQ produced a marked regression in fibrosarcoma (FsaR) and squamous cell carcinomas xenograft tumors in mice (Ivankovic et al., 2006). A similar decrease was reported in prostate and lung cancer xenograft models in nude mice after subcutaneous injection of TQ (Kaseb et al., 2007; Yi et al., 2008; Jafri et al., 2010).
Pazhouhi et al. demonstrated that TQ synergistically augmented the anti-cancer activity of temozolomide (TMZ) in the glioblastoma (GBM) cell line U87MG through the inhibition of autophagy (Pazhouhi et al., 2016). Another recent study by Khazaei et al. reported synergistic apoptotic cell death of glioblastoma cells upon combination treatment with TQ and TMZ (Khazaei and Pazhouhi, 2017). Similar finding was also reported by Gurung et al. where TQ was shown to inhibit proliferation and induce DNA damage, cell cycle arrest and apoptosis in the glioblastoma cells (Gurung et al., 2010). This group of researchers demonstrated that through the inhibition of telomerase activity TQ was able to promote telomere attrition in GBM cells and the effect was more pronounced in GBM cells that abundantly expressed DNA-PKcs (Gurung et al., 2010). There is also a report where TQ was found to restrain the tumor growth and enhance the chemopreventive effect of 5-fluorouracil in an early colorectal tumor model in rat (Kensara et al., 2016). In this model azoxymethane (AOM) was used to induce colorectal neoplasia and treatment with 5-FU/TQ combination produced a greater reduction of AOM-induced colorectal tumors and large aberrant crypts foci compared to either agents alone. TQ collaborated with 5-FU to inhibit the expression of procancerous NF-κB, iNOS, VEGF, Wnt, β-catenin, COX-2, and TBRAS with a concomitant increase in anti-tumorigenic TGF-β1, TGF-βRII, DKK-1, CDNK-1A, Smad4, and GPx expression (Kensara et al., 2016). They also concluded with no significant differences in the liver function enzymes and renal function parameters between the control and treatment groups, demonstrating an excellent safety profile of the TQ/5-FU combination therapy (Kensara et al., 2016). A recent study by Fröhlich et al. demonstrated enhanced level of ROS generation and concomitant DNA damage induction in human colon cancer cells treated with a novel hybrid of TQ and artemisinin (Frohlich et al., 2017). In another study, conducted by Lei et al., TQ was found to chemosensitize 5-FU in gastric cancer treatment through increased apoptosis induction and growth inhibition (Lei et al., 2012).
A study conducted by Siveen et al. demonstrated that TQ was able to inhibit multiple myeloma (MM) cell proliferation along with induction of chemosensitization in xenograft mouse model (Siveen et al., 2014). TQ itself inhibited the proliferation of MM cells and CD138+ cells isolated from MM patient samples in a concentration dependent manner. TQ treatment elicited a potentiation of apoptosis exerted by bortezomib as evidenced by the activation of caspase-3 and cleaved PARP (Siveen et al., 2014). Most importantly, the antitumor efficacy of bortezomib in a xenograft model was potentiated by TQ through the modulation of different survival and angiogenesis markers such as Bcl-2, p65, Ki-67, and VEGF (Siveen et al., 2014). A similar study was done by Li et al. on MM model with constitutively activated signal transducer and activator of transcription 3 (STAT3) pathway, where TQ treatment resulted in suppression of STAT3 phosphorylation/activation followed by significant potentiation of thalidomide and bortezomib efficacy, in terms of apoptosis induction and growth arrest in MM cells (Li et al., 2010).
TQ also displayed promising results both in vitro and in vivo in an orthotopic model of pancreatic cancer. There was a significant growth inhibition of tumor cells when TQ was added to gemcitabine or oxaliplatin (Banerjee et al., 2009; Mu et al., 2015). This was accompanied by an increase in tumor cell killing through the down-regulation of NF-κB, Bcl-2, survivin, and cyclooxygenase-2. Concomitantly, an enhanced apoptosis and diminished proliferation of the tumor tissues were supportive of strong chemosensitization potential of TQ in the orthotopic pancreatic mouse model. Synergistic combinations of TQ and oxaliplatin were also investigated by some other groups where a third chemotherapy (5-FU or cisplatin) was used along with TQ and oxaliplatin, resulted in reversal of chemo resistance and improved cytotoxic effects at sub-therapeutic doses of chemo agents (Nessa et al., 2011; Sarman et al., 2016). Moreover, there combinations were found to exhibit chemo-preventive effects and reduced chemotherapy-induced toxicities and side effects. Several other preclinical studies have reported synergistic effect of TQ and cisplatin on multiple in vitro and in vivo cancer models including colon, ovarian, cervical, and lung cancer (Jafri et al., 2010; Nessa et al., 2011; Al-Malki and Sayed, 2014; Hafiza and Latifah, 2014; Wilson et al., 2015). In these studies, TQ was found to downregulate pro-angiogenic factors (e.g., VEGF), growth promoting oncogenic proteins (e.g., cMyc), and anti-apoptotic proteins (e.g., Bcl-2) through inhibition of NF-κB activation. Combination treatment with TQ and cisplatin showed hindrance in tumorigenesis evident from diminished expression of proliferation markers, a concomitant enhancement of double strand DNA break and apoptotic cell death were also observed (Jafri et al., 2010; Wilson et al., 2015).
TQ treatment also showed promising results in doxorubicin-resistant human breast cancer cells (Arafa el et al., 2011). After doxorubicin-resistant MCF-7/DOX cells were exposed to TQ, there was an extensive decrease of the cell survival regulators, phosphorylated Akt and Bcl2 along with an increased expression of PTEN and apoptotic markers such as Bax, cleaved caspases, and cleaved PARP. TQ also produced an augmented expression of p53 and p21 proteins with a concomitant G2/M arrest in the same cell line (Arafa el et al., 2011). TQ-mediated chemopotentiation of doxorubicin was also observed in several other cancer models including acute lymphoblastic leukemia, melanoma, colon cancer, and cervical cancer (Effenberger-Neidnicht and Schobert, 2011; Brown et al., 2014). In addition to the induction of synergistic cytotoxic effects through interference in tumor growth and survival signaling, combination treatment with TQ also exhibited chemo-protective effects and limited organ toxicity (Brown et al., 2014). Numerous other studies have investigated the potential chemo-potentiating effects of TQ with diverse classes of chemotherapeutic drugs (summarized in Table 1) including anti-estrogen (e.g., tamoxifen), topoisomerase-I inhibitor (e.g., topotecan), and microtubule disrupting agents (Rajput et al., 2013; Khalife et al., 2014, 2016; Dirican et al., 2015; Ganji-Harsini et al., 2016; Sakalar et al., 2016). Rajput et al. recently reported that TQ can cause reversal of tamoxifen resistance in triple negative breast cancer cells by interfering in Akt-mediated induction of apoptosis inhibitory protein such as X-linked inhibitor of apoptosis protein (XIAP; Rajput et al., 2013). Chemo-potentiation of microtubule disrupting agents (e.g., docetaxel and paclitaxel) by TQ was found to be mediated by upregulation of tumor suppressor genes such as p21 and Brca1, induction of pro-apoptotic factors, and inhibition of cancer cell growth and survival promoting signaling pathways such as PI3K/Akt and MAPK/ERK (Dirican et al., 2015; Sakalar et al., 2016).
Most of the above mentioned studies have observed synergistic cytotoxic and other anti-tumorigenic effects (e.g., angiogenesis, migration, invasion, and metastasis) of TQ against cancer cells with simultaneous protection of non-cancerous cells from chemotherapy induced hazardous effects, through preferential modulation of a complex array of tumorigenic, and deregulated signaling pathways. In addition to these chemopotentiating effect, TQ is recently found to synergize with miR-34a, a microRNA which targets various epithelial to mesenchymal transition-inducing transcription factors (EMT-TFs) including twist-related protein 1 (TWIST1), SLUG, and NOTCH1 (Imani et al., 2017). Overall, TQ was found to show almost all the favorable characteristics of an ideal adjuvant agents that can be used along with a wide variety of main course anticancer chemotherapeutic drugs. These emerging evidences justify further in-depth evaluation of TQ's chemopotentiating role in preclinical and clinical level.
Immunomodulatory Effects of TQ and Its Prospective Use with Immunotherapies
Possible immunomodulatory role of TQ is evident from numerous in vitro and in vivo studies (Gholamnezhad et al., 2015; Majdalawieh and Fayyad, 2015), where TQ was found to regulate the growth and cellular response of various immune cells such as T cells, B cells, macrophages, neutrophils, NK cells, and dendritic cells. Beside other inflammatory mediators, these immune cells are essential components of the tumor microenvironment and frequently release different cytokines and growth factors that lead to the generation of immunosuppressive milieu at the tumor sites along with promotion of cancer cell proliferation, survival, migration, invasion, and metastasis (Lin and Karin, 2007). During tumorigenesis, a pre-existing chronic inflammatory conditions elicit higher level of immune inhibitory cytokines and other immunosuppressive factors. Likewise, the immune cells infiltrated in the tumor microenvironment such as cytotoxic T cells and natural killer (NK) cells, rather showing tumoricidal activity, feed into the tumor associated inflammation (Zou, 2005). Due to this dysregulated immune response, immunomodulatory drugs are now considered as potential supplementary agents with various immunotherapies including cancer vaccines, immune checkpoint blocking antibodies, adoptive T cell therapy, and dendritic cell (DC) based immunotherapy (Mahoney et al., 2015). Moreover, immunomodulatory agents can functionalize cancer related flawed innate and adaptive immune systems to enhance anticancer immune activity.
Several lines of studies have confirmed that TQ can exert anti-inflammatory effect through inhibition of eicosanoids and prostaglandine synthesis (Houghton et al., 1995; El Mezayen et al., 2006) and intervention in the production and release of pro-inflammatory cytokines and reactive oxygen and nitrogen species (Mansour et al., 2002; Sankaranarayanan and Pari, 2011; Umar et al., 2012). A direct inhibitory effect of TQ treatment on NF-κB activation was also reported in multiple occasions (Mohamed et al., 2005; El Gazzar et al., 2007; Sethi et al., 2008; Zhang et al., 2016), which might explain the downregulation of pro-inflammatory mediators upon TQ administration. Although, NF-κB-mediated gene transcription is required for normal cellular activities, most of the cancers are associated with aberrant NF-κB signaling that lead to the release of different inflammatory cytokines followed by activation of diverse arrays of tumorigenic signaling pathways (Karin, 2006, 2009). Therefore, by attenuating NF-κB-mediated gene transcriptions, TQ might alter inflammation-induced immunosuppression in tumor microenvironment, as well as can restrict the tumorigenesis processes. Signal transducer and activator of transcription 3 (STAT3) is a key player in the mechanism of tumor-induced immune deregulation characterized by the paucity of immunological danger signals essential for immune activation and escaping of cancer cells from natural immune surveillance (Yu et al., 2007). Constitutive activation of STAT3 has been observed in many cancers which results in the generation of immature myeloid and dendritic cells. The subsequent prevention of DC maturation leads to immune tolerance due to T cells deletion or their differentiation into regulatory or suppressor T cells. In addition, persistent activation of STAT3 in NK cells and neutrophils inhibit the tumor killing activity of those effector cells. A study by Li et al. reported that TQ can interfere in both the constitutive and IL-6-inducible STAT3 phosphorylation through inhibition of upstream signaling kinases (Li et al., 2010). Similar observations were stated by couple of other groups where TQ treatment resulted in decease phosphorylation and subsequent activation of STAT3 (Badr et al., 2011c; Kundu et al., 2014a). Though, there are no reports showing the correlation of TQ-mediated suppression of STAT3 activation and reversal of immune dysregulation, several studies have investigated the effect of TQ treatment on DC maturation (Xuan et al., 2010), cytotoxic T cell activation (Badr et al., 2011a; Salem et al., 2011), and enhancement of NK cytotoxic functions (Salim et al., 2014).
Treating cancer with immunotherapies have gained immense interest, mostly due its unique feature of re-weoponizing body's own immune systems to identify and destroy tumor cells. After long history of failure of immunotherapy, time has now changed and progress has been made for effective cancer immunotherapy against certain cancers especially after successful introduction of immune checkpoint blocking antibodies, e.g., cytotoxic T lymphocyte associated protein-4 (CTLA-4) and program cell death protein-1 (PD-1) blocking antibodies. However, there are some other strategies which can be utilized as successful cancer immunotherapies including cancer vaccines, adoptive T cell therapy and DC based immunotherapy. Effect of TQ in some of the cases has been shown by some research groups whereas there are still lots of research yet to be done in this specific field. Cancer vaccination is a promising approach toward cancer immunotherapy. An effective anti-tumor response is obtained if antigen is captured and processed by dendritic cells, presented through its MHC molecule to CD4/CD8 T cells, and subsequently activation and proliferation of T cells occurred until it eliminates the cancer cell (Mellman et al., 2011). Suppression at any point can cause immune tolerance. In order to make a successful cancer vaccine, it needs to break the tolerance obtained against tumor cells (Mellman et al., 2011; Topalian et al., 2011; Farkona et al., 2016). Among different categories of cancer vaccines, peptide vaccines, tumor and immune cell vaccines are very crucial in cancer immunotherapy (Subramaniam et al., 2016). Tacemotide, a peptide vaccine that target MUC1 glycoprotein is one of the prominent vaccine in this group whereas Sipuleucel-T is an immune cell vaccine in prostate cancer (Anassi and Ndefo, 2011; Wurz et al., 2014; Hossain and Wall, 2016).
Dendritic cells, because of its antigen presenting abilities and role in correlation between innate and adaptive immunity, can be utilized to target large number of antigens and activate in order to break the tolerance (Palucka and Banchereau, 2012). The target of vaccination with DC is to see if it can induce cytotoxic CD8+ effector T cells which are tumor specific (Palucka and Banchereau, 2012). While immature dendritic cells are able to capture and process antigen, it needs to be matured in order to present antigenic peptide to naïve T cells in the lymphoid organ. The most common method to mature DC is cytokine cocktail that includes TNFα with combination of other cytokine, e.g., IL6, PGE2 (Bol et al., 2016). Other factors include bacterial components such as lipopolysaccharides (LPS), CD40 ligand, IFNα and IFNγ (Castiello et al., 2011). One group has investigated if TQ has any role on LPS induced maturation of DC and cytokine release (Xuan et al., 2010). They found out that TQ inhibits maturation and impairs cytokine release by LPS stimulated DCs. Instead, it stimulates DC apoptosis by caspase activation and impairing the phosphorylation of LPS induced Akt and ERK1/2. However, there are still research needs to be done to see if TQ helps in DC maturation in presence of different cytokines, an important aspects for DC based immunotherapy.
T cell based immunotherapy mostly includes adoptive T cell transfer and immune checkpoint inhibitor antibodies (Houot et al., 2015). Adoptive cell therapy emerged as one of the most successful cancer immunotherapy in metastatic melanoma cancer patients (Rosenberg et al., 2008). It is being used to artificially enrich the quality and quantity of T lymphocytes that can detect tumor specific antigen and kill tumor. This process requires to harvest patients own T lymphocytes either from peripheral blood or draining lymph node, expand in-vitro with the stimulation of anti-CD3 and anti-CD28 monoclonal antibodies in presence of IL-2 and reintroduce back into the patient's body (Houot et al., 2015). Those adoptively transferred T cells, because of their specificity to tumor antigen, can recognize the tumor antigen that is being presented through MHC1 complex and improve cell engraftment and survival (Salem et al., 2011). Those functionally significant anti-tumor T cells can be recognized by the co expression of lymphoid homing molecule L-selectin CD62L+ and chemokine receptor CCR7+ (Rosenberg et al., 2008; Klebanoff et al., 2012). It has been shown that TQ can increase the survival rate of CD62L at a lower dose. It also increased CD8+ T cell proliferation and the production of CD8+ released cytokine IFN-γ, meaning it can enhance the survival of antigen stimulated CD8+ T cells. However, high dose of TQ might cause toxic effect and leads to apoptosis of T cells (Salem et al., 2011).
Over-all, immunomodulatory activity of TQ, pointed by experimental evidences, can be exploited by combining it with various immunotherapies such as monoclonal antibodies, immune checkpoint inhibitors, and cancer vaccines (1). More studies are warranted to investigate the feasibility of priming the innate and adaptive immune systems by TQ and its potential to improve immunotherapeutic efficacy.
Summary and Future Perspective
Though considerable advancements have been accomplished in cancer pathogenesis and thereby in treatment strategies, the overall survival rates still remain poor. Targeting a particular molecule or signaling pathway, involved in one of the singular aspects of the multistep complex tumorigenesis processes, has recently been deemed as extravagant attempt to curtail malignant progression. Due to the inherent heterogeneous nature, some cancer cells can always evade a particular therapeutic modality and continue to survive on alternative pathways followed by recurrence of tumor at a far more aggressive form. Therefore, the paradigm in cancer treatment strategy is now shifting from targeted therapy to combination or multi-targeted approaches.
TQ, immediately after its isolation, has been investigated in numerous disease models including cancer. Those studies have identified some important and useful pharmacological properties of TQ that can be exploited to devise novel and more effective therapeutic interventions against cancer. It was found to exhibit a wide range of biochemical functions through its modulatory interactions with diversified molecular targets. Reportedly, TQ interferes in the phosphorylation and subsequent activation of several upstream tyrosine kinases (e.g., MAPK, Akt, mTOR, PIP3) that are involved in tumor cell proliferation signaling pathways (Yi et al., 2008; Kundu et al., 2014b). Transcriptional factors (e.g., Nrf2, NF-κB, and STAT-3), key players in various oncogenesis process, are other crucial molecular targets of TQ (Kundu et al., 2014b; Darakhshan et al., 2015; Majdalawieh et al., 2017). By regulating the activation of these transcription factors, TQ can counteract different tumorigenic processes including inflammation, cell proliferation, cell survival, angiogenesis, cell invasions, and metastasis. Moreover, TQ shows chemopreventive properties by downregulating carcinogen metabolizing enzymes (e.g., CYP 1A2, CYP 3A4), upregulating cytoprotective enzymes (e.g., glutathione S-transferase, superoxide dismutase, and oxidoreductase), attenuated production of pro-inflammatory mediators (e.g., cytokines, chemokines, and prostaglandins; Kundu et al., 2014b).
By virtue of its multi-targeting nature, TQ can be considered as a promising therapeutic moiety for cancer treatment. But the main focus of this review was to show how TQ can improve the therapeutic efficacy and safety profile of the main course cancer therapies, namely surgery, radiotherapy, chemotherapy, and immunotherapy through induction of selective cytotoxicity in cancer cells and cytoprotection in healthy cells. This was primarily because of the fact that TQ has low potency and poor bioavailability. These issues can be resolved by synthesizing various analogs of TQ and formulating those into different delivery systems. We have to also address some other critical questions about TQ regarding its opposite biochemical functions, such as it can act both as an antioxidant (at low concentration) and ROS inducer (at relatively high concentration). To better understand these differential cellular effects of TQ, more in vitro, in vivo, and in silico studies should be conducted at both proteomic and genomic level. Furthermore, upcoming studies should be concentrated on finding better derivatives of TQ along with detailed and accurate characterizations thereof. We have to also come up with suitable dosage form and delivery system for those analogs along with determination of their pharmacokinetic behavior, efficacy, and toxicity in multiple in vivo cancer models. Findings from such studies will enable us to devise clinically effective combination therapeutics where TQ or its derivatives can potentiate the anti-tumorigenic potential of various conventional and established anti-cancer agents.
Author Contributions
AM wrote the major portion of the manuscript and coordinated the manuscript writing, MH wrote the “Immunomodulatory effects of TQ and its prospective use with immunotherapies” portion of the manuscript; DB wrote the “Chemo-potentiating role of TQ” portion of the manuscript and MB conceived the idea and contributed writing and coordinating the manuscript.
Conflict of Interest Statement
The authors declare that the research was conducted in the absence of any commercial or financial relationships that could be construed as a potential conflict of interest.
References
Abramovitch, R., Marikovsky, M., Meir, G., and Neeman, M. (1999). Stimulation of tumour growth by wound-derived growth factors. Br. J. Cancer 79, 1392–1398. doi: 10.1038/sj.bjc.6690223
Ahmad, I., Muneer, K. M., Tamimi, I. A., Chang, M. E., Ata, M. O., and Yusuf, N. (2013). Thymoquinone suppresses metastasis of melanoma cells by inhibition of NLRP3 inflammasome. Toxicol. Appl. Pharmacol. 270, 70–76. doi: 10.1016/j.taap.2013.03.027
Alfarouk, K. O., Stock, C. M., Taylor, S., Walsh, M., Muddathir, A. K., Verduzco, D., et al. (2015). Resistance to cancer chemotherapy: failure in drug response from ADME to P-gp. Cancer Cell Int. 15:71. doi: 10.1186/s12935-015-0221-1
Al-Jenoobi, F. I., Al-Thukair, A. A., Abbas, F. A., Ansari, M. J., Alkharfy, K. M., Al-Mohizea, A. M., et al. (2010). Effect of black seed on dextromethorphan O- and N-demethylation in human liver microsomes and healthy human subjects. Drug Metab. Lett. 4, 51–55. doi: 10.2174/187231210790980435
Al-Malki, A. L., and Sayed, A. A. (2014). Thymoquinone attenuates cisplatin-induced hepatotoxicity via nuclear factor kappa-beta. BMC Complement. Altern. Med. 14:282. doi: 10.1186/1472-6882-14-282
Anassi, E., and Ndefo, U. A. (2011). Sipuleucel-T (provenge) injection: the first immunotherapy agent (vaccine) for hormone-refractory prostate cancer. P T 36, 197–202.
Arafa el, S. A., Zhu, Q., Shah, Z. I., Wani, G., Barakat, B. M., Racoma, I., et al. (2011). Thymoquinone up-regulates PTEN expression and induces apoptosis in doxorubicin-resistant human breast cancer cells. Mutat. Res. 706, 28–35. doi: 10.1016/j.mrfmmm.2010.10.007
Badary, O. A., Al-Shabanah, O. A., Nagi, M. N., Al-Rikabi, A. C., and Elmazar, M. M. (1999). Inhibition of benzo(a)pyrene-induced forestomach carcinogenesis in mice by thymoquinone. Eur. J. Cancer Prev. 8, 435–440. doi: 10.1097/00008469-199910000-00009
Badary, O. A., and Gamal El-Din, A. M. (2001). Inhibitory effects of thymoquinone against 20-methylcholanthrene-induced fibrosarcoma tumorigenesis. Cancer Detect. Prev. 25, 362–368.
Badr, G., Alwasel, S., Ebaid, H., Mohany, M., and Alhazza, I. (2011a). Perinatal supplementation with thymoquinone improves diabetic complications and T cell immune responses in rat offspring. Cell. Immunol. 267, 133–140. doi: 10.1016/j.cellimm.2011.01.002
Badr, G., Lefevre, E. A., and Mohany, M. (2011b). Thymoquinone inhibits the CXCL12-induced chemotaxis of multiple myeloma cells and increases their susceptibility to Fas-mediated apoptosis. PLoS ONE 6:e23741. doi: 10.1371/journal.pone.0023741
Badr, G., Mohany, M., and Abu-Tarboush, F. (2011c). Thymoquinone decreases F-actin polymerization and the proliferation of human multiple myeloma cells by suppressing STAT3 phosphorylation and Bcl2/Bcl-XL expression. Lipids Health Dis. 10:236. doi: 10.1186/1476-511X-10-236
Baguley, B. C. (2010). Multiple drug resistance mechanisms in cancer. Mol. Biotechnol. 46, 308–316. doi: 10.1007/s12033-010-9321-2
Banerjee, S., Kaseb, A. O., Wang, Z., Kong, D., Mohammad, M., Padhye, S., et al. (2009). Antitumor activity of gemcitabine and oxaliplatin is augmented by thymoquinone in pancreatic cancer. Cancer Res. 69, 5575–5583. doi: 10.1158/0008-5472.CAN-08-4235
Baskar, R., Dai, J., Wenlong, N., Yeo, R., and Yeoh, K. W. (2014). Biological response of cancer cells to radiation treatment. Front. Mol. Biosci. 1:24. doi: 10.3389/fmolb.2014.00024
Bol, K. F., Schreibelt, G., Gerritsen, W. R., de Vries, I. J., and Figdor, C. G. (2016). Dendritic cell-based immunotherapy: state of the art and beyond. Clin. Cancer Res. 22, 1897–1906. doi: 10.1158/1078-0432.CCR-15-1399
Brown, R. K., Wilson, G., Tucci, M. A., and Benghuzzi, H. A. (2014). The effects of thymoquinone and Doxorubicin on leukemia and cardiomyocyte cell lines. Biomed. Sci. Instrum. 50, 391–396.
Castiello, L., Sabatino, M., Jin, P., Clayberger, C., Marincola, F. M., Krensky, A. M., et al. (2011). Monocyte-derived DC maturation strategies and related pathways: a transcriptional view. Cancer Immunol. Immunother. 60, 457–466. doi: 10.1007/s00262-010-0954-6
Ceelen, W., Pattyn, P., and Mareel, M. (2014). Surgery, wound healing, and metastasis: recent insights and clinical implications. Crit. Rev. Oncol. Hematol. 89, 16–26. doi: 10.1016/j.critrevonc.2013.07.008
Ceelen, W. P., Morris, S., Paraskeva, P., and Pattyn, P. (2007). Surgical trauma, minimal residual disease and locoregional cancer recurrence. Cancer Treat. Res. 134, 51–69. doi: 10.1007/978-0-387-48993-3_4
Dajani, E. Z., Shahwan, T. G., and Dajani, N. E. (2016). Overview of the preclinical pharmacological properties of Nigella sativa (black seeds): a complementary drug with historical and clinical significance. J. Physiol. Pharmacol. 67, 801–817.
Darakhshan, S., Bidmeshki Pour, A., Hosseinzadeh Colagar, A., and Sisakhtnezhad, S. (2015). Thymoquinone and its therapeutic potentials. Pharmacol. Res. 95–96, 138–158. doi: 10.1016/j.phrs.2015.03.011
Dehkordi, F. R., and Kamkhah, A. F. (2008). Antihypertensive effect of Nigella sativa seed extract in patients with mild hypertension. Fundam. Clin. Pharmacol. 22, 447–452. doi: 10.1111/j.1472-8206.2008.00607.x
Delaney, G., Jacob, S., Featherstone, C., and Barton, M. (2005). The role of radiotherapy in cancer treatment: estimating optimal utilization from a review of evidence-based clinical guidelines. Cancer 104, 1129–1137. doi: 10.1002/cncr.21324
Demicheli, R., Retsky, M. W., Hrushesky, W. J., Baum, M., and Gukas, I. D. (2008). The effects of surgery on tumor growth: a century of investigations. Ann. Oncol. 19, 1821–1828. doi: 10.1093/annonc/mdn386
Dirican, A., Atmaca, H., Bozkurt, E., Erten, C., Karaca, B., and Uslu, R. (2015). Novel combination of docetaxel and thymoquinone induces synergistic cytotoxicity and apoptosis in DU-145 human prostate cancer cells by modulating PI3K-AKT pathway. Clin. Transl. Oncol. 17, 145–151. doi: 10.1007/s12094-014-1206-6
Effenberger, K., Breyer, S., and Schobert, R. (2010). Terpene conjugates of the Nigella sativa seed-oil constituent thymoquinone with enhanced efficacy in cancer cells. Chem. Biodivers. 7, 129–139. doi: 10.1002/cbdv.200900328
Effenberger-Neidnicht, K., and Schobert, R. (2011). Combinatorial effects of thymoquinone on the anti-cancer activity of doxorubicin. Cancer Chemother. Pharmacol. 67, 867–874. doi: 10.1007/s00280-010-1386-x
El Gazzar, M. A., El Mezayen, R., Nicolls, M. R., and Dreskin, S. C. (2007). Thymoquinone attenuates proinflammatory responses in lipopolysaccharide-activated mast cells by modulating NF-kappaB nuclear transactivation. Biochim. Biophys. Acta 1770, 556–564. doi: 10.1016/j.bbagen.2007.01.002
El Mezayen, R., El Gazzar, M., Nicolls, M. R., Marecki, J. C., Dreskin, S. C., and Nomiyama, H. (2006). Effect of thymoquinone on cyclooxygenase expression and prostaglandin production in a mouse model of allergic airway inflammation. Immunol. Lett. 106, 72–81. doi: 10.1016/j.imlet.2006.04.012
Elmowafy, M., Samy, A., Raslan, M. A., Salama, A., Said, R. A., Abdelaziz, A. E., et al. (2016). Enhancement of bioavailability and pharmacodynamic effects of thymoquinone via Nanostructured Lipid Carrier (NLC) Formulation. AAPSPharmSciTech 17, 663–672. doi: 10.1208/s12249-015-0391-0
Farkona, S., Diamandis, E. P., and Blasutig, I. M. (2016). Cancer immunotherapy: the beginning of the end of cancer? BMC Med. 14:73. doi: 10.1186/s12916-016-0623-5
Frohlich, T., Ndreshkjana, B., Muenzner, J. K., Reiter, C., Hofmeister, E., Mederer, S., et al. (2017). Synthesis of novel hybrids of thymoquinone and artemisinin with high activity and selectivity against colon cancer. ChemMedChem 12, 226–234. doi: 10.1002/cmdc.201600594
Gali-Muhtasib, H., Ocker, M., Kuester, D., Krueger, S., El-Hajj, Z., Diestel, A., et al. (2008). Thymoquinone reduces mouse colon tumor cell invasion and inhibits tumor growth in murine colon cancer models. J. Cell. Mol. Med. 12, 330–342. doi: 10.1111/j.1582-4934.2007.00095.x
Gali-Muhtasib, H. U., Abou Kheir, W. G., Kheir, L. A., Darwiche, N., and Crooks, P. A. (2004). Molecular pathway for thymoquinone-induced cell-cycle arrest and apoptosis in neoplastic keratinocytes. Anticancer Drugs 15, 389–399. doi: 10.1097/00001813-200404000-00012
Ganea, G. M., Fakayode, S. O., Losso, J. N., van Nostrum, C. F., Sabliov, C. M., and Warner, I. M. (2010). Delivery of phytochemical thymoquinone using molecular micelle modified poly(D, L lactide-co-glycolide) (PLGA) nanoparticles. Nanotechnology 21:285104. doi: 10.1088/0957-4484/21/28/285104
Ganji-Harsini, S., Khazaei, M., Rashidi, Z., and Ghanbari, A. (2016). Thymoquinone could increase the efficacy of Tamoxifen induced apoptosis in human breast cancer cells: an in vitro study. Cell J. 18, 245–254. doi: 10.22074/cellj.2016.4320
Gholamnezhad, Z., Rafatpanah, H., Sadeghnia, H. R., and Boskabady, M. H. (2015). Immunomodulatory and cytotoxic effects of Nigella sativa and thymoquinone on rat splenocytes. Food Chem. Toxicol. 86, 72–80. doi: 10.1016/j.fct.2015.08.028
Guida, M. S., Abd El-Aal, A., Kafafy, Y., Salama, S. F., Badr, B. M., and Badr, G. (2016). Thymoquinone rescues T lymphocytes from gamma irradiation-induced apoptosis and exhaustion by modulating pro-inflammatory cytokine levels and PD-1, Bax, and Bcl-2 signaling. Cell. Physiol. Biochem. 38, 786–800. doi: 10.1159/000443034
Gurung, R. L., Lim, S. N., Khaw, A. K., Soon, J. F., Shenoy, K., Mohamed Ali, S., et al. (2010). Thymoquinone induces telomere shortening, DNA damage and apoptosis in human glioblastoma cells. PLoS ONE 5:e12124. doi: 10.1371/journal.pone.0012124
Hafiza, W. A., and Latifah, S. Y. (2014). Potential implications of GRP58 expression and susceptibility of cervical cancer to cisplatin and thymoquinone-based therapy. Onco. Targets. Ther. 7, 1375–1387. doi: 10.2147/OTT.S62928
Hossain, M. K., and Wall, K. A. (2016). Immunological Evaluation of Recent MUC1 Glycopeptide Cancer Vaccines. Vaccines (Basel) 4:25. doi: 10.3390/vaccines4030025
Houghton, P. J., Zarka, R., de las Heras, B., and Hoult, J. R. (1995). Fixed oil of Nigella sativa and derived thymoquinone inhibit eicosanoid generation in leukocytes and membrane lipid peroxidation. Planta Med. 61, 33–36. doi: 10.1055/s-2006-957994
Houot, R., Schultz, L. M., Marabelle, A., and Kohrt, H. (2015). T-cell-based immunotherapy: adoptive cell transfer and checkpoint inhibition. Cancer Immunol. Res. 3, 1115–1122. doi: 10.1158/2326-6066.CIR-15-0190
Imani, S., Wei, C., Cheng, J., Khan, M. A., Fu, S., Yang, L., et al. (2017). MicroRNA-34a targets epithelial to mesenchymal transition-inducing transcription factors (EMT-TFs) and inhibits breast cancer cell migration and invasion. Oncotarget 8, 21362–21379. doi: 10.18632/oncotarget.15214
Ivankovic, S., Stojkovic, R., Jukic, M., Milos, M., Milos, M., and Jurin, M. (2006). The antitumor activity of thymoquinone and thymohydroquinone in vitro and in vivo. Exp. Oncol. 28, 220–224.
Jafri, S. H., Glass, J., Shi, R., Zhang, S., Prince, M., and Kleiner-Hancock, H. (2010). Thymoquinone and cisplatin as a therapeutic combination in lung cancer: in vitro and in vivo. J. Exp. Clin. Cancer Res. 29:87. doi: 10.1186/1756-9966-29-87
Jrah-Harzallah, H., Ben-Hadj-Khalifa, S., Almawi, W. Y., Maaloul, A., Houas, Z., and Mahjoub, T. (2013). Effect of thymoquinone on 1,2-dimethyl-hydrazine-induced oxidative stress during initiation and promotion of colon carcinogenesis. Eur. J. Cancer 49, 1127–1135. doi: 10.1016/j.ejca.2012.10.007
Karin, M. (2006). Nuclear factor-kappaB in cancer development and progression. Nature 441, 431–436. doi: 10.1038/nature04870
Karin, M. (2009). NF-kappaB as a critical link between inflammation and cancer. Cold Spring Harb. Perspect. Biol. 1:a000141. doi: 10.1101/cshperspect.a000141
Kaseb, A. O., Chinnakannu, K., Chen, D., Sivanandam, A., Tejwani, S., Menon, M., et al. (2007). Androgen receptor and E2F-1 targeted thymoquinone therapy for hormone-refractory prostate cancer. Cancer Res. 67, 7782–7788. doi: 10.1158/0008-5472.CAN-07-1483
Kensara, O. A., El-Shemi, A. G., Mohamed, A. M., Refaat, B., Idris, S., and Ahmad, J. (2016). Thymoquinone subdues tumor growth and potentiates the chemopreventive effect of 5-fluorouracil on the early stages of colorectal carcinogenesis in rats. Drug Des. Devel. Ther. 10, 2239–2253. doi: 10.2147/DDDT.S109721
Khalife, R., El-Hayek, S., Tarras, O., Hodroj, M. H., and Rizk, S. (2014). Antiproliferative and proapoptotic effects of topotecan in combination with thymoquinone on acute myelogenous leukemia. Clin. Lymphoma Myeloma Leuk. 14(Suppl.), S46–S55. doi: 10.1016/j.clml.2014.04.014
Khalife, R., Hodroj, M. H., Fakhoury, R., and Rizk, S. (2016). Thymoquinone from Nigella Sativa seeds promotes the antitumor activity of noncytotoxic doses of Topotecan in human colorectal cancer cells in vitro. Planta Med. 82, 312–321. doi: 10.1055/s-0035-1558289
Khan, M. A., Tania, M., Wei, C., Mei, Z., Fu, S., Cheng, J., et al. (2015). Thymoquinone inhibits cancer metastasis by downregulating TWIST1 expression to reduce epithelial to mesenchymal transition. Oncotarget 6, 19580–19591. doi: 10.18632/oncotarget.3973
Khazaei, M., and Pazhouhi, M. (2017). Temozolomide-Mediated apoptotic death is improved by Thymoquinone in U87MG cell line. Cancer Invest. 35, 225–236. doi: 10.1080/07357907.2017.1289383
Klebanoff, C. A., Gattinoni, L., and Restifo, N. P. (2012). Sorting through subsets: which T-cell populations mediate highly effective adoptive immunotherapy? J. Immunother. 35, 651–660. doi: 10.1097/CJI.0b013e31827806e6
Kundu, J., Choi, B. Y., Jeong, C. H., Kundu, J. K., and Chun, K. S. (2014a). Thymoquinone induces apoptosis in human colon cancer HCT116 cells through inactivation of STAT3 by blocking JAK2- and Srcmediated phosphorylation of EGF receptor tyrosine kinase. Oncol. Rep. 32, 821–828. doi: 10.3892/or.2014.3223
Kundu, J., Chun, K. S., Aruoma, O. I., and Kundu, J. K. (2014b). Mechanistic perspectives on cancer chemoprevention/chemotherapeutic effects of thymoquinone. Mutat. Res. 768, 22–34. doi: 10.1016/j.mrfmmm.2014.05.003
Kundu, J., Kim, D. H., Kundu, J. K., and Chun, K. S. (2014c). Thymoquinone induces heme oxygenase-1 expression in HaCaT cells via Nrf2/ARE activation: Akt and AMPKalpha as upstream targets. Food Chem. Toxicol. 65, 18–26. doi: 10.1016/j.fct.2013.12.015
Lang, M., Borgmann, M., Oberhuber, G., Evstatiev, R., Jimenez, K., Dammann, K. W., et al. (2013). Thymoquinone attenuates tumor growth in ApcMin mice by interference with Wnt-signaling. Mol. Cancer 12:41. doi: 10.1186/1476-4598-12-41
Lei, X., Lv, X., Liu, M., Yang, Z., Ji, M., Guo, X., et al. (2012). Thymoquinone inhibits growth and augments 5-fluorouracil-induced apoptosis in gastric cancer cells both in vitro and in vivo. Biochem. Biophys. Res. Commun. 417, 864–868. doi: 10.1016/j.bbrc.2011.12.063
Li, F., Rajendran, P., and Sethi, G. (2010). Thymoquinone inhibits proliferation, induces apoptosis and chemosensitizes human multiple myeloma cells through suppression of signal transducer and activator of transcription 3 activation pathway. Br. J. Pharmacol. 161, 541–554. doi: 10.1111/j.1476-5381.2010.00874.x
Lin, W. W., and Karin, M. (2007). A cytokine-mediated link between innate immunity, inflammation, and cancer. J. Clin. Invest. 117, 1175–1183. doi: 10.1172/JCI31537
Mahoney, K. M., Rennert, P. D., and Freeman, G. J. (2015). Combination cancer immunotherapy and new immunomodulatory targets. Nat. Rev. Drug Discov. 14, 561–584. doi: 10.1038/nrd4591
Majdalawieh, A. F., and Fayyad, M. W. (2015). Immunomodulatory and anti-inflammatory action of Nigella sativa and thymoquinone: a comprehensive review. Int. Immunopharmacol. 28, 295–304. doi: 10.1016/j.intimp.2015.06.023
Majdalawieh, A. F., Fayyad, M. W., and Nasrallah, G. K. (2017). Anti-cancer properties and mechanisms of action of thymoquinone, the major active ingredient of Nigella sativa. Crit. Rev. Food Sci. Nutr. doi: 10.1080/10408398.2016.1277971. [Epub ahead of print].
Maniwa, Y., Okada, M., Ishii, N., and Kiyooka, K. (1998). Vascular endothelial growth factor increased by pulmonary surgery accelerates the growth of micrometastases in metastatic lung cancer. Chest 114, 1668–1675. doi: 10.1378/chest.114.6.1668
Mansour, M. A., Nagi, M. N., El-Khatib, A. S., and Al-Bekairi, A. M. (2002). Effects of thymoquinone on antioxidant enzyme activities, lipid peroxidation and DT-diaphorase in different tissues of mice: a possible mechanism of action. Cell Biochem. Funct. 20, 143–151. doi: 10.1002/cbf.968
Mellman, I., Coukos, G., and Dranoff, G. (2011). Cancer immunotherapy comes of age. Nature 480, 480–489. doi: 10.1038/nature10673
Mohamed, A., Afridi, D. M., Garani, O., and Tucci, M. (2005). Thymoquinone inhibits the activation of NF-kappaB in the brain and spinal cord of experimental autoimmune encephalomyelitis. Biomed. Sci. Instrum. 41, 388–393.
Mu, G. G., Zhang, L. L., Li, H. Y., Liao, Y., and Yu, H. G. (2015). Thymoquinone pretreatment overcomes the insensitivity and potentiates the antitumor effect of gemcitabine through abrogation of Notch1, PI3K/Akt/mTOR regulated signaling pathways in pancreatic cancer. Dig. Dis. Sci. 60, 1067–1080. doi: 10.1007/s10620-014-3394-x
Nessa, M. U., Beale, P., Chan, C., Yu, J. Q., and Huq, F. (2011). Synergism from combinations of cisplatin and oxaliplatin with quercetin and thymoquinone in human ovarian tumour models. Anticancer Res. 31, 3789–3797.
Orhon, Z. N., Uzal, C., Kanter, M., Erboga, M., and Demiroglu, M. (2016). Protective effects of Nigella sativa on gamma radiation-induced jejunal mucosal damage in rats. Pathol. Res. Pract. 212, 437–443. doi: 10.1016/j.prp.2016.02.017
Palucka, K., and Banchereau, J. (2012). Cancer immunotherapy via dendritic cells. Nat. Rev. Cancer 12, 265–277. doi: 10.1038/nrc3258
Pazhouhi, M., Sariri, R., Rabzia, A., and Khazaei, M. (2016). Thymoquinone synergistically potentiates temozolomide cytotoxicity through the inhibition of autophagy in U87MG cell line. Iran. J. Basic Med. Sci. 19, 890–898.
Peng, L., Liu, A., Shen, Y., Xu, H. Z., Yang, S. Z., Ying, X. Z., et al. (2013). Antitumor and anti-angiogenesis effects of thymoquinone on osteosarcoma through the NF-kappaB pathway. Oncol. Rep. 29, 571–578. doi: 10.3892/or.2012.2165
Raghunandhakumar, S., Paramasivam, A., Senthilraja, S., Naveenkumar, C., Asokkumar, S., Binuclara, J., et al. (2013). Thymoquinone inhibits cell proliferation through regulation of G1/S phase cell cycle transition in N-nitrosodiethylamine-induced experimental rat hepatocellular carcinoma. Toxicol. Lett. 223, 60–72. doi: 10.1016/j.toxlet.2013.08.018
Rajkamal, G., Suresh, K., Sugunadevi, G., Vijayaanand, M. A., and Rajalingam, K. (2010). Evaluation of chemopreventive effects of Thymoquinone on cell surface glycoconjugates and cytokeratin expression during DMBA induced hamster buccal pouch carcinogenesis. BMB Rep. 43, 664–669. doi: 10.5483/BMBRep.2010.43.10.664
Rajput, S., Kumar, B. N., Banik, P., Parida, S., and Mandal, M. (2015). Thymoquinone restores radiation-induced TGF-beta expression and abrogates EMT in chemoradiotherapy of breast cancer cells. J. Cell. Physiol. 230, 620–629. doi: 10.1002/jcp.24780
Rajput, S., Kumar, B. N., Sarkar, S., Das, S., Azab, B., Santhekadur, P. K., et al. (2013). Targeted apoptotic effects of thymoquinone and tamoxifen on XIAP mediated Akt regulation in breast cancer. PLoS ONE 8:e61342. doi: 10.1371/journal.pone.0061342
Rosenberg, S. A., Restifo, N. P., Yang, J. C., Morgan, R. A., and Dudley, M. E. (2008). Adoptive cell transfer: a clinical path to effective cancer immunotherapy. Nat. Rev. Cancer 8, 299–308. doi: 10.1038/nrc2355
Sakalar, C., Izgi, K., Iskender, B., Sezen, S., Aksu, H., Cakir, M., et al. (2016). The combination of thymoquinone and paclitaxel shows anti-tumor activity through the interplay with apoptosis network in triple-negative breast cancer. Tumour Biol. 37, 4467–4477. doi: 10.1007/s13277-015-4307-0
Salem, M. L., Alenzi, F. Q., and Attia, W. Y. (2011). Thymoquinone, the active ingredient of Nigella sativa seeds, enhances survival and activity of antigen-specific CD8-positive T cells in vitro. Br. J. Biomed. Sci. 68, 131–137. doi: 10.1080/09674845.2011.11730340
Salim, L. Z., Othman, R., Abdulla, M. A., Al-Jashamy, K., Ali, H. M., Hassandarvish, P., et al. (2014). Thymoquinone inhibits murine leukemia WEHI-3 cells in vivo and in vitro. PLoS ONE 9:e115340. doi: 10.1371/journal.pone.0115340
Salomi, M. J., Nair, S. C., and Panikkar, K. R. (1991). Inhibitory effects of Nigella sativa and saffron (Crocus sativus) on chemical carcinogenesis in mice. Nutr. Cancer 16, 67–72. doi: 10.1080/01635589109514142
Sankaranarayanan, C., and Pari, L. (2011). Thymoquinone ameliorates chemical induced oxidative stress and beta-cell damage in experimental hyperglycemic rats. Chem. Biol. Interact. 190, 148–154. doi: 10.1016/j.cbi.2011.02.029
Sarman, H., Bayram, R., and Benek, S. B. (2016). Anticancer drugs with chemotherapeutic interactions with thymoquinone in osteosarcoma cells. Eur. Rev. Med. Pharmacol. Sci. 20, 1263–1270.
Sethi, G., Ahn, K. S., and Aggarwal, B. B. (2008). Targeting nuclear factor-kappa B activation pathway by thymoquinone: role in suppression of antiapoptotic gene products and enhancement of apoptosis. Mol. Cancer Res. 6, 1059–1070. doi: 10.1158/1541-7786.MCR-07-2088
Siveen, K. S., Mustafa, N., Li, F., Kannaiyan, R., Ahn, K. S., Kumar, A. P., et al. (2014). Thymoquinone overcomes chemoresistance and enhances the anticancer effects of bortezomib through abrogation of NF-kappaB regulated gene products in multiple myeloma xenograft mouse model. Oncotarget 5, 634–648. doi: 10.18632/oncotarget.1596
Subramaniam, D. S., Liu, S. V., and Giaccone, G. (2016). Novel approaches in cancer immunotherapy. Discov. Med. 21, 267–274.
Sung, B., Prasad, S., Yadav, V. R., and Aggarwal, B. B. (2012). Cancer cell signaling pathways targeted by spice-derived nutraceuticals. Nutr. Cancer 64, 173–197. doi: 10.1080/01635581.2012.630551
Topalian, S. L., Weiner, G. J., and Pardoll, D. M. (2011). Cancer immunotherapy comes of age. J. Clin. Oncol. 29, 4828–4836. doi: 10.1200/JCO.2011.38.0899
Umar, S., Zargan, J., Umar, K., Ahmad, S., Katiyar, C. K., and Khan, H. A. (2012). Modulation of the oxidative stress and inflammatory cytokine response by thymoquinone in the collagen induced arthritis in Wistar rats. Chem. Biol. Interact. 197, 40–46. doi: 10.1016/j.cbi.2012.03.003
Velho-Pereira, R., Kumar, A., Pandey, B. N., Jagtap, A. G., and Mishra, K. P. (2011). Radiosensitization in human breast carcinoma cells by thymoquinone: role of cell cycle and apoptosis. Cell Biol. Int. 35, 1025–1029. doi: 10.1042/CBI20100701
Velho-Pereira, R., Kumar, A., Pandey, B. N., Mishra, K. P., and Jagtap, A. G. (2012). Radioprotection by macerated extract of Nigella sativa in normal tissues of fibrosarcoma bearing mice. Indian J. Pharm. Sci. 74, 403–414. doi: 10.4103/0250-474X.108415
Wilson, A. J., Saskowski, J., Barham, W., Yull, F., and Khabele, D. (2015). Thymoquinone enhances cisplatin-response through direct tumor effects in a syngeneic mouse model of ovarian cancer. J. Ovarian Res. 8:46. doi: 10.1186/s13048-015-0177-8
Woo, C. C., Hsu, A., Kumar, A. P., Sethi, G., and Tan, K. H. (2013). Thymoquinone inhibits tumor growth and induces apoptosis in a breast cancer xenograft mouse model: the role of p38 MAPK and ROS. PLoS ONE 8:e75356. doi: 10.1371/journal.pone.0075356
Wu, F. P., Hoekman, K., Meijer, S., and Cuesta, M. A. (2003). VEGF and endostatin levels in wound fluid and plasma after breast surgery. Angiogenesis 6, 255–257. doi: 10.1023/B:AGEN.0000029410.32264.b0
Wurz, G. T., Kao, C. J., Wolf, M., and DeGregorio, M. W. (2014). Tecemotide: an antigen-specific cancer immunotherapy. Hum. Vaccin. Immunother. 10, 3383–3393. doi: 10.4161/hv.29836
Xuan, N. T., Shumilina, E., Qadri, S. M., Gotz, F., and Lang, F. (2010). Effect of thymoquinone on mouse dendritic cells. Cell. Physiol. Biochem. 25, 307–314. doi: 10.1159/000276563
Yi, T., Cho, S. G., Yi, Z., Pang, X., Rodriguez, M., Wang, Y., et al. (2008). Thymoquinone inhibits tumor angiogenesis and tumor growth through suppressing AKT and extracellular signal-regulated kinase signaling pathways. Mol. Cancer Ther. 7, 1789–1796. doi: 10.1158/1535-7163.MCT-08-0124
Yu, H., Kortylewski, M., and Pardoll, D. (2007). Crosstalk between cancer and immune cells: role of STAT3 in the tumour microenvironment. Nat. Rev. Immunol. 7, 41–51. doi: 10.1038/nri1995
Zahreddine, H., and Borden, K. L. (2013). Mechanisms and insights into drug resistance in cancer. Front. Pharmacol. 4:28. doi: 10.3389/fphar.2013.00028
Zhang, L., Bai, Y., and Yang, Y. (2016). Thymoquinone chemosensitizes colon cancer cells through inhibition of NF-kappaB. Oncol. Lett. 12, 2840–2845. doi: 10.3892/ol.2016.4971
Keywords: thymoquinone, cancer treatment, adjuvant therapy, preclinical studies
Citation: Mostofa AGM, Hossain MK, Basak D and Bin Sayeed MS (2017) Thymoquinone as a Potential Adjuvant Therapy for Cancer Treatment: Evidence from Preclinical Studies. Front. Pharmacol. 8:295. doi: 10.3389/fphar.2017.00295
Received: 28 March 2017; Accepted: 08 May 2017;
Published: 12 June 2017.
Edited by:
Thomas Efferth, Johannes Gutenberg-Universität Mainz, GermanyReviewed by:
Esam Z. Dajani, International Drug Development Consultants Corp, United StatesJunjiang Fu, Affiliated Hospital of Southwest Medical University, China
Copyright © 2017 Mostofa, Hossain, Basak and Bin Sayeed. This is an open-access article distributed under the terms of the Creative Commons Attribution License (CC BY). The use, distribution or reproduction in other forums is permitted, provided the original author(s) or licensor are credited and that the original publication in this journal is cited, in accordance with accepted academic practice. No use, distribution or reproduction is permitted which does not comply with these terms.
*Correspondence: Muhammad Shahdaat Bin Sayeed, bXVoYW1tYWQtc2hhaGRhYXQuYmluLXNheWVlZEBmdWxicmlnaHRtYWlsLm9yZw==