- Institut für Allgemeine Pharmakologie und Toxikologie, Klinikum der Johann Wolfgang Goethe-Universität, Frankfurt am Main, Germany
The sphingosine-1-phosphate (S1P) signaling system with its specific G-protein-coupled S1P receptors, the enzymes of S1P metabolism and the S1P transporters, offers a multitude of promising targets for drug development. Until today, drug development in this area has nearly exclusively focused on (functional) antagonists at the S1P1 receptor, which cause a unique phenotype of immunomodulation. Accordingly, the first-in class S1P1 receptor modulator, fingolimod, has been approved for the treatment of relapsing-remitting multiple sclerosis, and novel S1P1 receptor (functional) antagonists are being developed for autoimmune and inflammatory diseases such as psoriasis, inflammatory bowel disease, lupus erythematodes, or polymyositis. Besides the S1P1 receptor, also S1P2 and S1P3 are widely expressed and regulate many diverse functions throughout the body. The S1P2 receptor, in particular, often exerts cellular functions which are opposed to the functions of the S1P1 receptor. As a consequence, antagonists at the S1P2 receptor have the potential to be useful in a contrasting context and different areas of indication compared to S1P1 antagonists. The present review will focus on the therapeutic potential of S1P2 receptor antagonists and discuss their opportunities as well as their potential risks. Open questions and areas which require further investigations will be emphasized in particular.
Introduction
Sphingosine-1-phosphate (S1P), initially considered as an intermediate breakdown product of complex sphingolipid catabolism, is meanwhile acknowledged as important bioactive lipid (Maceyka et al., 2012; Kunkel et al., 2013; Blaho and Hla, 2014; Proia and Hla, 2015). S1P regulates crucial cellular functions such as proliferation and survival, migration, and adhesion (Maceyka et al., 2012; Blaho and Hla, 2014). Systemic and local gradients of S1P provide direction for migration and trafficking of various cell types (Olivera et al., 2013a; Nishi et al., 2014). Metabolically, S1P resides in a dynamically balanced equilibrium with sphingosine and ceramide (Hannun and Obeid, 2008; Newton et al., 2015). Ceramide, derived from de novo sphingolipid synthesis, from breakdown of glycosphingolipids or via the sphingomyelin cycle, can be converted reversibly into sphingosine, which in turn can be phosphorylated to S1P by the sphingosine kinases, SphK1 and SphK2 (Hannun and Obeid, 2008). S1P is a substrate of specific S1P phosphatases and non-specific lipid phosphate phosphatases which direct the equilibrium into the direction of sphingosine/ceramide (Hannun and Obeid, 2008). S1P can also be cleaved by S1P lyase, which generates hexadecenal and phosphoethanolamine and thus catalyzes an irreversible step of sphingolipid breakdown (Aguilar and Saba, 2012).
With regard to its complexity and to the multitude of options for pharmacological interventions, the S1P signaling system might well be compared to the adrenergic system. First of all, there are five specific G-protein-coupled S1P receptors, which are widely expressed and can act both in a redundant and in an antagonistic manner by coupling to distinct G-proteins (Blaho and Hla, 2014; Kihara et al., 2014), reminiscent of α1, α2, and β adrenergic receptors (Bylund et al., 1994; Alexander et al., 2015). Furthermore, the enzymes which catalyze the formation and degradation of S1P, as well as the specific and non-specific S1P transporters, represent promising drug targets (Meyer zu Heringdorf et al., 2013; Orr Gandy and Obeid, 2013; Nishi et al., 2014), reminiscent of the enzymes of catecholamine synthesis and degradation as well as the catecholamine transporters (Alexander et al., 2013). However, drug development targeting the S1P signaling system has focused almost exclusively on the S1P1 receptor until now (Roberts et al., 2013; Bigaud et al., 2014; Gonzalez-Cabrera et al., 2014). This is not least due to the success of the non-specific S1P receptor agonist, fingolimod, which has been approved for the treatment of multiple sclerosis in 2010/11 by the FDA and the EMA, respectively (Kihara et al., 2015). In brief, phosphorylated fingolimod, acting as a superagonist or functional antagonist at S1P1, causes internalization and degradation of the receptor, thereby rendering lymphocytes insensitive to the tissue-blood S1P gradient with the consequence of lymphopenia (Bigaud et al., 2014). Subsequent to the approval of fingolimod, novel S1P1 functional or competitive antagonists with improved properties are presently being developed (Meyer zu Heringdorf et al., 2013; Roberts et al., 2013; Bigaud et al., 2014; Gonzalez-Cabrera et al., 2014). While the development of sphingosine kinase and S1P lyase inhibitors has turned out to be not that straightforward (see e.g., Schnute et al., 2012; Deniz et al., 2015, and discussion in Meyer zu Heringdorf et al., 2013), the focus on G-protein-coupled S1P receptors other than S1P1 might be promising. Aside from causing immunosuppression, the effects of S1P1 receptor antagonism are rather undesirable: elevation of blood pressure, bronchial constriction, and on the long term a disturbance of the vascular endothelial barrier (Bigaud et al., 2014). Since the S1P2 receptor often acts contrarily to S1P1, S1P2 antagonists might turn up as promising tools for improving local blood flow in combination with tightening of the endothelial barrier, as anti-inflammatory and anti-fibrotic treatment options, and as potentially beneficial to treat the metabolic syndrome. The current review will discuss this therapeutic potential of S1P2 receptor antagonists and potential risks.
Overview of G-Protein-Coupled S1P Receptors and Specific Features of S1P2
There are five specific G-protein-coupled receptors with a high affinity for S1P. According to the International Union of Basic and Clinical Pharmacology (IUPHAR) receptor nomenclature, they are named S1P1–S1P5 (human gene names S1PR1–S1PR5; Kihara et al., 2014). S1P1-3 are expressed nearly ubiquitously, whereas S1P4 is preferentially expressed in the hematopoietic system, and S1P5 is found in the white matter of the brain as well as in some other tissues (Blaho and Hla, 2014; Kihara et al., 2014, 2015; Pyne et al., 2015). S1P1 couples exclusively to Gi, while S1P2 and S1P3 couple to Gi, Gq, and G12/13. S1P4 and S1P5 couple to Gi and G12/13. These receptors regulate cell growth and survival, migration, and adhesion. Thereby they orchestrate for example the circulation of lymphocytes, the formation and maturation of blood vessels, vascular tone and permeability, as well as heart rate (Blaho and Hla, 2014; Kihara et al., 2014). Furthermore, they play a role in inflammation, fibrosis, and cancer (Maceyka et al., 2012; Schwalm et al., 2013; Newton et al., 2015; Pyne et al., 2015).
The S1P2 receptor, also referred to as EDG-5, H218, AGR16, or lpB2, was originally cloned from rat aortic vascular smooth muscle cells (Okazaki et al., 1993) and later identified as high-affinity S1P receptor (Gonda et al., 1999). With a Kd of 27 nM and an EC50 in GTPγS binding of 3.8–8.9 nM, the affinity of S1P to S1P2 appears marginally lower than its affinity to S1P1 (Kd of 8 nM and EC50 in GTPγS binding 0.4–79; for review, see Spiegel, 2000; Kihara et al., 2014). S1P2 is coupled via Gi, Gq, and G12/13 to phospholipase C, [Ca2+]i increases, activation of mitogen-activated protein kinases, and activation of Rho and/or Rho kinase (reviewed in Skoura and Hla, 2009; Adada et al., 2013; Blaho and Hla, 2014). Similar to S1P1, S1P2 can be internalized upon stimulation with agonists (see e.g., Ter Braak et al., 2011; Imeri et al., 2014). Mechanistically, this has been analyzed in zebrafish. The zebrafish miles apart mutant, S1P2 R150H, which impedes the migration of cardiac precursor cells to the midline, was shown to be constitutively desensitized and internalized (Burczyk et al., 2015). As this involved β-arrestin-2 and G-protein-coupled receptor kinase (GRK)-2, S1P2 R150H receptor functionality could be restored by reduced GRK2/3 expression (Burczyk et al., 2015).
S1P2 can activate Akt (Means et al., 2007), but in most cells, it inhibits Akt (Schüppel et al., 2008; Du et al., 2010; Michaud et al., 2010; Green et al., 2011; Japtok et al., 2015, and many other reports), and it can act pro- as well as anti-proliferative. Similarly, S1P2 can both activate and inhibit Rac (Okamoto et al., 2000; Sugimoto et al., 2003; Takashima et al., 2008; Rapizzi et al., 2009). S1P2 inhibited the phosphatidylinositol-3-kinase/Akt pathway by mediating Rho-dependent activation of the phosphoinositide phosphatase and tensin homolog deleted on chromosome 10 (PTEN) in fibroblasts and endothelial cells (Sanchez et al., 2005, 2007), but in macrophages, S1P2-mediated inhibition of Akt and migration were independent of PTEN (Michaud et al., 2010). Via G12/13 or Gq and activation of Rho/Rho kinase, in conjunction with inhibition of Rac, S1P2 exerts its eminent anti-migratory effect, important, e.g., for retention of B cells and follicular T helper cells in germinal centers (Green et al., 2011; Moriyama et al., 2014). The S1P2 receptor can furthermore regulate cytoskeleton organization via phosphorylation of ezrin/radixin/moesin (ERM) proteins (Gandy et al., 2013). This pathway appears to be involved in control of the endothelial barrier function and cancer cell invasion (Adyshev et al., 2011; Adada et al., 2015). Another signaling pathway recently discovered to be regulated by G-protein-coupled receptors including S1P2 is the Hippo pathway (Yu et al., 2012). S1P2, again via G12/13 and Rho, activated the transcription coactivators, YAP and TAZ (Miller et al., 2012; Yu et al., 2012). This occurred independently of the core Hippo pathway kinases (Miller et al., 2012), or via inhibition of LATS1/2 (Yu et al., 2012). The Hippo pathway regulates tissue proliferation and organ size, and plays a role in tumorigenesis (Zhang et al., 2015; Ye and Eisinger-Mathason, 2016).
The S1P2 receptor itself might be regulated by signals other than S1P. Thus, a recent report suggests that the well-known effect of S1P2 on neurite retraction (MacLennan et al., 2000; Toman et al., 2004) might be involved in neuronal Nogo-A signaling (Kempf et al., 2014). It was shown that Nogo-A-Δ20 directly activated S1P2 by binding to its extracellular loops 2 and 3 independently of the presence of S1P, and via S1P2/G13/RhoA inhibited neurite outgrowth and repressed synaptic plasticity (Kempf et al., 2014). The authors suggested that the S1P2 receptor is a multi-ligand receptor, regulated by both lipid and protein ligands (Kempf et al., 2014). Yet other potential agonists at S1P2 might be conjugated bile acids such as taurocholate (Studer et al., 2012). Several reports show that effects of conjugated bile acids, such as activation of ERK and Akt in primary rodent hepatocytes, and cell growth, survival, induction of cyclooxygenase (COX)-2 and formation of prostaglandin (PG)E2 in cholangiocarcinoma cells, are dependent on the S1P2 receptor, as they are blocked by the antagonist JTE-013 and by knocking down S1P2 (Studer et al., 2012; Liu et al., 2014, 2015). While these observations do not preclude that preparations of conjugated bile acids contain traces of S1P, taurocholate specifically induced ERK phosphorylation in S1P2 but not S1P1 overexpressing HEK-293 cells (data obtained with the other S1P receptors were not shown; Studer et al., 2012), thereby rebutting the contamination hypothesis. A direct interaction is furthermore supported by molecular modeling of taurocholate into S1P2 (Studer et al., 2012).
S1P2 Receptor Pharmacology
S1P2 receptor pharmacology is still in its infancy as only few S1P2 selective compounds have been identified so far. These are still experimental tool compounds. Until recently, JTE-013 (Osada et al., 2002) was the only available S1P2 antagonist. Many functional studies have made use of this compound and thus, besides the results obtained with genetic approaches, the effects of JTE-013 have shaped our present view of the physiological roles of S1P2. However, JTE-013 has a low potency and lacks selectivity. This is illustrated by the fact that JTE-013 inhibited responses to S1P in S1P2 knockout mice, and it had effects in cells that had no S1P2 mRNA transcripts (Salomone et al., 2008; Li et al., 2012). Later, it was found that JTE-013 inhibited not only S1P2 but also the S1P4 receptor (Long et al., 2010). Furthermore, JTE-013 lacks stability in vivo (Li et al., 2015). Recently, AB1 has been developed as derivative of JTE-013 with improved stability in vivo, higher potency and better efficacy, but the selectivity of the compound is not well defined so far (Li et al., 2015). Other recently synthesized S1P2 antagonists require further characterization (Kusumi et al., 2015). Interestingly, a series of S1P2 agonists has also been described, of which at least CYM-5520 acted at an allosteric site (Satsu et al., 2013). While not much is known so far concerning the biological effects of CYM-5520, the related compound CYM-5478 (Satsu et al., 2013) reduced cisplatin-mediated cell death by reducing reactive oxygen species (ROS) in C6 glioma cells (Herr et al., 2016). Taken together, further development is required in the area of S1P2 receptor pharmacology.
Anti-Migratory Effect of S1P2 and Consequences for Angiogenesis and B Cell Homing
One of the main effects of the S1P1 receptor is induction of migration and chemotaxis within gradients of S1P (Spiegel et al., 2002). In contrast to S1P1, S1P2 inhibits migration in many cell types, including vascular endothelial and smooth muscle cells as well as tumor cells, via activation of Rho and inhibition of Rac (Okamoto et al., 2000; Arikawa et al., 2003; Goparaju et al., 2005; Lepley et al., 2005; Tamama et al., 2005; Takashima et al., 2008). The S1P2 antagonist JTE-013 augmented S1P-induced angiogenesis in vivo in a Matrigel implant assay (Inoki et al., 2006). The S1P2 receptor appears to be upregulated in senescent endothelial cells, such as pulmonary microvascular endothelial cells from aged rats, and senescence-associated impairment in chemotaxis and migration was attenuated by down-regulation of S1P2 (Lu et al., 2012). In human umbilical vein endothelial cells, the S1P2 receptor suppressed angiogenic sprouting via a Gα12/13/leukemia-associated RhoGEF (LARG)/RhoC-signaling cascade (Del Galdo et al., 2013). Nevertheless, both pro- and anti-migratory S1P receptors, i.e., S1P1-3, are required for proper development of the vasculature during embryonic development (Kono et al., 2004). In the mouse retina, S1P2 was not required for normal angiogenesis (Skoura et al., 2007). Interestingly, S1P2 was induced by hypoxia, and its knockout reduced the hypoxia-associated pathologic neovascularization in the vitreous chamber, while it augmented the revascularization into the avascular zones of the retina (Skoura et al., 2007). While these observations suggest that antagonism at S1P2 might be useful for treatment of pathologic neovascularization, S1P2 knockout in fact went along with enhanced tumor angiogenesis (Du et al., 2010), suggesting that this indication should be regarded with caution.
Interestingly, similar to the pro-migratory effect of the S1P1 receptor, which is required for lymphocyte egress from secondary lymphatic tissues, the anti-migratory effect of the S1P2 receptor plays an eminent role in the regulation of lymphocyte localization. By inhibition of migration and proliferation, S1P2 mediates the confinement of B cells and follicular T helper cells to lymph node germinal centers (Green et al., 2011; Moriyama et al., 2014). It is likely a consequence thereof that mice lacking the S1P2 receptor develop clonal B cell lymphomas with age (Cattoretti et al., 2009). Furthermore, somatic mutations in the 5′ sequence of the S1PR2 gene were detected in about 25% of human germinal center-derived diffuse large B cell lymphomas (Cattoretti et al., 2009). Importantly, mice which were heterozygous for the S1P2 receptor did not develop lymphomas (Cattoretti et al., 2009), suggesting that treatment with a pharmacological inhibitor would probably not cause this devastating condition, although this is clearly one of the critical facets in S1P2 antagonist development.
Contractile Effect of S1P2 in Smooth Muscle and Consequences for Vessel Tone, Blood Flow, and Inner Ear Function
The S1P2 receptor induces contraction of diverse types of smooth muscle, including vascular, bronchial, intestinal, and bladder smooth muscle, by inducing increases in intracellular Ca2+ concentration and activation of Rho/Rho kinase (Ohmori et al., 2003; Hu et al., 2006; Kendig et al., 2013). In the vascular system, endothelial S1P1 and S1P3 receptors mediate NO-dependent vasodilation, while smooth muscle S1P2 and S1P3 receptors mediate vasoconstriction, with differential responses in different vascular beds (Igarashi and Michel, 2009; Kerage et al., 2014). S1P2 knockout mice had normal mean arterial pressure and left ventricular function, but reduced renal and mesenteric vascular resistance which went along with an enhanced blood flow in the affected areas (Lorenz et al., 2007). Furthermore, the S1P2 receptor contributed to blood pressure elevation and vasoconstriction after α-adrenergic stimulation (Lorenz et al., 2007). In a mouse model of myocardial infarction-induced heart failure, S1P2 knockout mice did not develop enhanced myogenic responses (Hoefer et al., 2010). While blood flow in cerebral arteries was dependent on S1P3 (Salomone et al., 2008), S1P2 appears to play a role in perfusion of lung, liver, and kidney. Thus, S1P2 activation increases pulmonary vascular resistance in isolated perfused mouse lung (Szczepaniak et al., 2010). Another study suggested that S1P-induced pulmonary vasoconstriction was mediated by S1P4 (Ota et al., 2011), but it is a contradiction that JTE-013, as a S1P2/S1P4 antagonist (Long et al., 2010), had no effect in that study. Interestingly, hypoxia-induced pulmonary hypertension in rodents was reduced by JTE-013 (Chen et al., 2014). In agreement, S1P2 antagonists have been suggested for the treatment of pulmonary hypertension (Xing et al., 2015). In isolated perfused rat liver, S1P2 activation increased portal pressure (Ikeda et al., 2004), and S1P2 antagonism reduced portal vein pressure in cirrhotic rats after bile duct ligation (Kageyama et al., 2012). Finally, S1P2-mediated vasoconstriction in the isolated perfused kidney was enhanced in diabetic rats (Bautista-Pérez et al., 2011).
While these results suggest that S1P2 antagonism could be helpful to increase organ blood flow, there is also a caveat: S1P2 knockout mice are deaf and have a progressive loss of vestibular function (MacLennan et al., 2006). Among the earliest lesions in the cochlea of S1P2-deficient mice, there were defects in the stria vascularis (Kono et al., 2007). These data suggest that the absence of S1P2 caused a dysregulation of the spiral modiolar artery tone, and subsequently an abnormal perfusion of the inner ear, which is accountable for the phenotype (Kono et al., 2007). In addition, the S1P2 receptor also contributes to cochlear hair cell maintenance and survival (Herr et al., 2007, 2016). Thus, it was shown recently that ROS accumulated in the cochlea of S1P2 knockout mice (Herr et al., 2016). Furthermore, S1P2 inhibited NADPH oxidase-3 activity in a recombinant system, and the S1P2 agonist, CYM-5478, reduced cisplatin-induced ROS formation in C6 glioma cells (Herr et al., 2016). Also very recently, the S1P2 receptor gene has been allocated to the autosomal-recessive non-syndromic hearing impairment locus DFNB68 on chromosome 19, and two pathogenic S1P2 variants have been identified (Santos-Cortez et al., 2016). In conclusion, S1P2 receptor agonists might be a treatment option for cisplatin- or gentamicin-induced ototoxicity (Nakayama et al., 2014; Herr et al., 2016), while S1P2 antagonists will have to be analyzed for potential ototoxic effects.
Vascular Permeability and Vascular Inflammation
While early studies reported that functional antagonists at S1P1 improved the vascular endothelial barrier, e.g., in lipopolysaccharide (LPS)-induced edema, it soon turned out that this was due to the initial agonistic activity of the compounds, which was however followed by an increase in vascular permeability on the long term (Xiong and Hla, 2014). Here again, the S1P2 receptor plays an opposite role, as activation of S1P2 causes a disruption of endothelial adherens junctions and increased paracellular permeability. Consequently, JTE-013 improved barrier integrity (Sanchez et al., 2007). An improvement of the vascular barrier by knockout or inhibition of S1P2 has been shown in endothelial cell cultures and in diverse in vivo models including stroke (Kim et al., 2015), experimental autoimmune encephalitis (EAE; Cruz-Orengo et al., 2014), and anaphylactic shock (see below). Thus, JTE-013 attenuated the H2O2-evoked vascular leak in the isolated perfused rat lung (Sanchez et al., 2007). LPS and tumor necrosis factor-α (TNF-α) upregulated S1P2 in endothelial cells. Importantly, vascular inflammation induced by LPS or TNF-α, with NF-κB activation and upregulation of vascular cell adhesion molecule (VCAM)-1, intercellular adhesion molecule (ICAM)-1, E-selectin, and monocyte chemoattractant protein (MCP)-1, was reduced by inhibition and/or knockout of S1P2 (Du et al., 2012; Zhang W. et al., 2013). In models of LPS-induced acute lung injury or endotoxemia, both vascular and alveolar leakage were reduced by these pharmacological or genetic approaches (Sammani et al., 2010; Zhang G. et al., 2013). Taken together, a barrier disruptive role of the S1P2 receptor, and thus a therapeutic potential for S1P2 antagonists in diverse conditions of vascular barrier breakdown, is supported by a broad range of studies. However, it was also shown that S1P2 might be protective against acute vascular barrier disruption in active anaphylaxis and after injection of platelet-activating factor (Cui et al., 2013; see below). These authors observed that the activities of Akt and endothelial NO synthase were enhanced in S1P2 knockout aorta, lung, and endothelial cells, and the enhanced NO release in turn compromised vascular barrier function (Cui et al., 2013). Furthermore, S1P2 contributed to maintenance of adherens junctions in this study, in contrast to previous reports (Sanchez et al., 2007). The reason for this, however, remains unclear.
Atherosclerosis
S1P occurs at high concentrations in blood plasma and serum, where it is bound mostly to high-density lipoproteins and albumin, and both pro- and anti-atherosclerotic activities of S1P have been described (Levkau, 2015). The S1P2 receptor, in particular, is significantly upregulated by inflammatory mediators in the endothelium, and it mediates vascular inflammation (see above), which might be a key factor for promoting atherosclerosis. In fact, S1P2 is upregulated in atherosclerotic endothelium (Estrada et al., 2008). However, after carotid artery ligation, S1P2-deficient mice developed large neointimal lesions, which went along with medial and intimal smooth muscle proliferation (Shimizu et al., 2007), suggesting that S1P2 on vascular smooth muscle cells was atheroprotective. In agreement, S1P2, by inducing serum response-factor enrichment of CArG box promotor regions, induced phenotypic modulation of smooth muscle cells in response to S1P (Wamhoff et al., 2008). On the other hand, atherosclerosis in the mouse aorta was decreased by S1P2 knockout in apolipoprotein E-deficient mice (Skoura et al., 2011). This study showed that body weight, plasma cholesterol, plasma triglyceride, and lipoprotein profiles were not altered in atherosclerotic S1P2 knockout mice, but that the number of macrophages and foam cells infiltrating the vessel wall was decreased, and that S1P2 in the myeloid cells was responsible for the effect. It was concluded that S1P2 signaling retained macrophages in the plaques, where they promoted inflammation (Skoura et al., 2011). Recently, it was shown that a knockout of both Gα12 and Gα13 in myeloid cells protected LDL receptor-deficient mice from atherosclerosis, which is of importance here, since the S1P2 receptor was identified as the major G12/13 activator in the relevant cells, which were identified as peritoneal macrophages (Grimm et al., 2016). In brief, the authors showed that the alternative, anti-inflammatory polarization of aortic macrophages in those mice was an indirect effect, mediated by activation of atheroprotective B cells through classical, pro-inflammatory peritoneal macrophages. Importantly, in both bone marrow-derived and peritoneal macrophages from myeloid G12/13 knockout mice, pro-inflammatory cytokines were strongly upregulated, and this was mimicked by JTE-013 in wild-type macrophages (Grimm et al., 2016). Thus, the study suggested that G12/13, and S1P2 as major G12/13 activator in macrophages, decreased rather than increased pro-inflammatory cytokine production, but nevertheless, atherosclerosis was attenuated by inhibition of this pathway (Grimm et al., 2016). Taken together, inhibition of S1P2 might be atheroprotective, but further studies on the underlying mechanisms are clearly required.
Mast Cells and Anaphylaxis
Early reports have shown that in mast cells, the S1P2 receptor is involved in an autocrine loop that augments degranulation induced by Fc𝜀 receptor-I crosslinking (Jolly et al., 2004). However, recent studies on the role of S1P2 in anaphylaxis yielded contradictory results (Table 1): One group observed that JTE-013 and S1P2 knockout attenuated the severity of anaphylaxis and reduced circulating histamine levels as well as pulmonary edema and allergic lung infiltration in a mouse model of immunoglobulin E (IgE)/antigen-triggered anaphylaxis (Oskeritzian et al., 2010, 2015). Interestingly, after histamine injection to shortcut mast cell degranulation, JTE-013 and S1P2 knockout did not attenuate the anaphylactic response, suggesting that S1P2 acted primarily by augmenting histamine release from mast cells during the onset of anaphylaxis (Oskeritzian et al., 2010). Another group observed that the symptoms of histamine-induced anaphylaxis were more severe in S1P2 knockout mice (Olivera et al., 2010). Most importantly, they observed a poor recovery after both histamine and IgE/antigen challenge in these mice, which went along with a severe hypotension and a delay in plasma histamine clearance (Olivera et al., 2010, 2013b). Yet another group even questioned that S1P2 knockout improved the vascular barrier breakdown, as deletion of this receptor aggravated the vascular leak and strongly impaired survival in mice challenged with antigen or platelet-activating factor (Cui et al., 2013). As possible explanations for the discrepant findings, differential responses of mast cell populations, the respective activity of the IgE preparations, active versus passive anaphylaxis as well as a role of the mouse strains were considered (Cui et al., 2013; Olivera et al., 2013b; Oskeritzian et al., 2015). Taken together, it remains open whether the potential beneficial effects of S1P2 antagonists, i.e., inhibition of mast cell degranulation, improvement of the vascular barrier, and attenuation of airway constriction, would outweigh their potential deleterious effect, i.e., aggravation of hypotension, in human anaphylaxis.
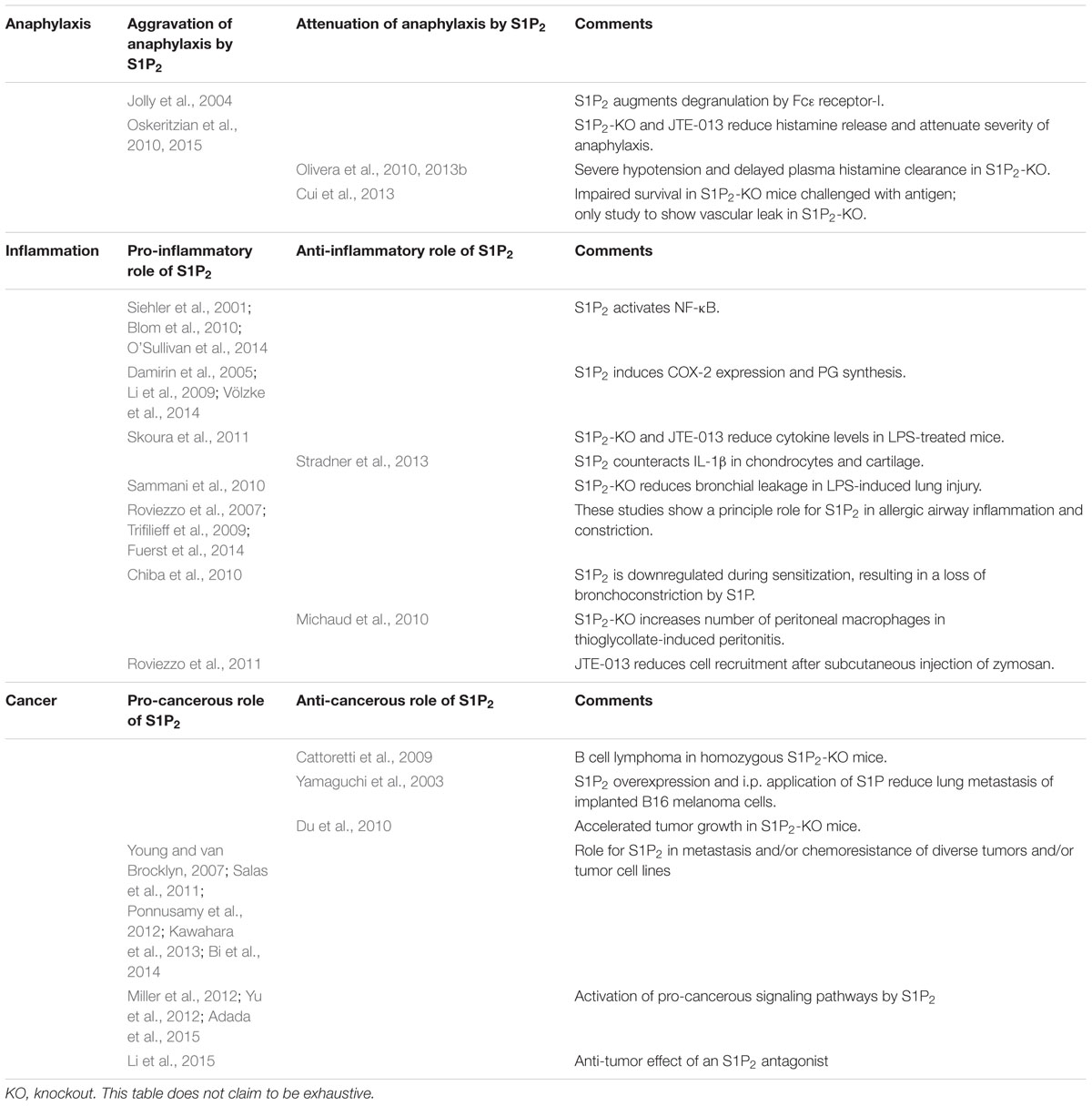
TABLE 1. Overview of controversial publications on the role of the S1P2 receptor in anaphylaxis, inflammation and cancer.
Inflammation and Fibrosis
As mentioned above, the S1P2 receptor plays a role in vascular permeability and inflammation, atherosclerosis, mast cell function, and anaphylaxis. Further studies suggest that the S1P2 receptor acts pro-inflammatory also in other inflammatory settings. In LPS-treated mice, elevated serum levels of interleukin (IL)-1β and IL-18 were reduced by S1P2 knockout and JTE-013 (Skoura et al., 2011). In diverse cell types, S1P2 activated NF-κB (Siehler et al., 2001; Blom et al., 2010; O’Sullivan et al., 2014) or induced COX-2 expression and PG synthesis (Damirin et al., 2005; Li et al., 2009; Völzke et al., 2014). As mentioned above, in a mouse model of LPS-induced lung injury, knockout of S1P2 reduced protein leakage into the bronchoalveolar lavage fluid (Sammani et al., 2010). On the other hand, in primary human chondrocytes and osteoarthritis cartilage, S1P2 counteracted the pro-inflammatory signaling of IL-1β, including IL-1β-induced upregulation of inducible NO synthase (Stradner et al., 2013).
S1P generally induces airway smooth muscle contraction (Rosenfeldt et al., 2003) and plays a role in airway hyper-responsiveness and asthma (Kume et al., 2007; Roviezzo et al., 2010, 2015). Recently, it was shown that S1P repressed β2 adrenergic activity in airway smooth muscle cells by increasing COX-2-mediated PGE2 production (Rumzhum et al., 2016). S1P2 and S1P3 appear to be involved in these effects (for review, see Lai et al., 2011). Thus, JTE-013 inhibited ovalbumin-induced contraction of lung parenchymal strips from ovalbumin-sensitized rats (Trifilieff et al., 2009). However, in isolated bronchial rings, S1P augmented KCl-induced contraction in a JTE-013-sensitive manner only in bronchi from control mice, not from antigen-challenged mice, which was traced back to a down-regulation of S1P2 during sensitization (Chiba et al., 2010). In contrast, S1P2 and S1P3 were upregulated during ovalbumin-sensitization in the study of Roviezzo et al. (2007). Importantly, S1P2 and S1P3 receptors induced a steroid-resistant pro-remodeling response in airway smooth muscle cells (Fuerst et al., 2014). S1P2 furthermore had a pro-inflammatory influence on the bronchial epithelium, as S1P-induced IL-8 release via NF-κB was inhibited by JTE-013 in human bronchial epithelial cells (O’Sullivan et al., 2014). The data suggest that S1P2 antagonists might have a beneficial effect on airway inflammation and remodeling in asthma.
Notably, the inhibitory influence of S1P2 on migration plays an important role in the recruitment of inflammatory cells to sites of inflammation. Thus, in thioglycollate-induced peritonitis, knockout of the S1P2 receptor led to an increase in the number of macrophages in the peritoneal cavity (Michaud et al., 2010). On the other hand, cell recruitment after subcutaneous injection of zymosan was reduced by JTE-013 (Roviezzo et al., 2011). These seemingly contradictory observations suggest that the underlying migratory events of the different cell types are regulated in a complex manner by S1P2.
Some additional activities of the S1P2 receptor might be important in inflammation. Thus, S1P2 reduced antigen capture by murine Langerhans cells, thereby potentially alleviating cutaneous contact hypersensitivity (Japtok et al., 2012). S1P2 furthermore suppressed macrophage phagocytosis, thereby impairing host defense in sepsis (Hou et al., 2015). Finally, while knockout and blockade of S1P2 improved the tightness of blood–brain barrier and ameliorated EAE, the receptor was found to be more abundant in female EAE mice and female patients with multiple sclerosis compared to their male counterparts, and it was suggested that this underlies the enhanced female CNS autoimmune susceptibility (Cruz-Orengo et al., 2014). Taken together, we are just beginning to understand the complex role of S1P2 in infection, inflammation, and autoimmunity.
With regard to fibrosis, it has been shown that intracellular S1P acts anti-fibrotic, while extracellular S1P has a pro-fibrotic activity. However, the respective role of the different S1P receptor subtypes appears to be less clear (Schwalm et al., 2013). In bleomycin-induced lung injury, a prolonged exposure to S1P1 functional antagonists including fingolimod not only worsened the vascular barrier function but also increased the fibrotic response (Shea et al., 2010), suggesting that S1P1 acts anti-fibrotic in pulmonary fibrosis. On the contrary, pro-fibrotic events such as Smad activation, induction of connective tissue growth factor (CTGF), and enhanced synthesis of extracellular matrix components, were dependent on signaling pathways involving G12/13 and/or Rho/Rho kinase, thereby pointing toward S1P2 and S1P3 as the main pro-fibrotic S1P receptors (Schwalm et al., 2013). Indeed, in carbon tetrachloride-induced liver fibrosis, S1P2 knockout mice showed reduced accumulation of hepatic myofibroblasts and decreased induction of fibrotic markers (Serriere-Lanneau et al., 2007; Ikeda et al., 2009). Considering also the role of S1P2 in portal hypertension (Kageyama et al., 2012; see above), S1P2 antagonists may turn out to be helpful in liver fibrosis. Other reports suggest a role for S1P2 and/or S1P3 in pulmonary, renal, or cardiac fibrosis (Schwalm et al., 2013). Of note, also the S1P5 receptor appears to be involved in fibrosis, as it has recently been shown to induce CTGF expression in renal mesangial cells (Wünsche et al., 2015).
Diabetes Mellitus and Insulin Resistance
Function and survival of pancreatic β-cells, as well as the responsiveness of insulin-sensitive tissues to insulin, play an important role in diabetes mellitus, and both are regulated by the SphK/S1P axis (Jessup et al., 2011; Fayyaz et al., 2014b). While earlier work showed that S1P itself contributed to glucose-stimulated insulin secretion and generally improved β-cell survival (Fayyaz et al., 2014b), it was shown recently that the S1P2 receptor had a rather negative impact on pancreatic β-cells. In streptozotocin-induced diabetes mellitus, S1P2 knockout mice had lower blood glucose levels, higher insulin/glucose ratios, less β-cell apoptosis, and a better survival rate (Imasawa et al., 2010). Furthermore, JTE-013 attenuated the development of diabetes in streptozotocin-treated wild type mice (Imasawa et al., 2010). In New Zealand obese mice, plasma concentrations of S1P increased over 28 days of high-fat diet, and treatment with JTE-013 reduced the concomitant β-cell loss (Japtok et al., 2015). Mechanistically, S1P2 counteracted the anti-apoptotic and proliferative effects of insulin by inhibiting Akt (Japtok et al., 2015).
Of the insulin-sensitive tissues, both skeletal muscle and hepatocytes were regulated by S1P2 (Rapizzi et al., 2009; Fayyaz et al., 2014a). In C2C12 myoblasts, S1P caused a ROS-dependent transphosphorylation of the insulin receptor, thereby increasing glucose uptake into the cells. S1P-induced ROS production was inhibited by JTE-013, suggesting that it was the S1P2 receptor which mimicked the activity of insulin in myoblasts (Rapizzi et al., 2009). In hepatocytes, however, S1P induced insulin resistance as it attenuated insulin-stimulated Akt phosphorylation in primary rat and human hepatocytes. This effect was sensitive to JTE-013, indicating an involvement of S1P2 which was the prevailing S1P receptor subtype on the mRNA level in hepatocytes. Importantly, in New Zealand obese mice, JTE-013 attenuated the continuous increase in blood glucose levels under high-fat diet, and partially reversed the high-fat diet-mediated loss in phospho-Akt in the liver of these mice (Fayyaz et al., 2014a). Thus, insulin sensitivity was regulated by S1P2 in a different manner in skeletal muscle and hepatocytes, respectively. Finally, it has to be mentioned that not much is known about S1P2 receptor activity in adipocytes, but a recent study suggests that the receptor had an anti-adipogenic effect in 3T3-L1 preadipocytes, as it decreased the expression of peroxisome proliferator-activated receptor-γ (PPAR-γ), CCAAT/enhancer binding protein-α and adiponectin, and decreased the triglyceride content of the cells (Moon et al., 2015). Consequently, the authors concluded that activation of S1P2 might be helpful against obesity (Moon et al., 2015). However, one has to keep in mind, that activation of PPAR-γ is well-known to improve insulin sensitivity and plasma lipid profiles, and to inhibit inflammation (Yki-Jarvinen, 2004; Cariou et al., 2012). Thiazolidinediones, which are PPAR-γ agonists, have been used for years as insulin sensitizers in diabetes mellitus type 2, but they have been gradually removed from the market because of hepatotoxicity, increased cardiovascular risk, or increased occurrence of bladder cancer (Yki-Jarvinen, 2004; Cariou et al., 2012). Presently, pharmacologists aim at next-generation PPAR-γ agonists with improved safety profiles (Sauer, 2015). In this context, it will be interesting to study the potential of S1P2 antagonists as inducers of PPAR-γ in relation to the reported negative effects of thiazolidinediones.
Skeletal Muscle
The SphK/S1P axis plays an important role in skeletal muscle (patho)physiology, and the S1P2 receptor, in particular, is involved in myogenic differentiation and muscle regeneration (Donati et al., 2013). Thus, by antisense, silencing or overexpression approaches, it was shown that S1P2 induced differentiation of C2C12 myoblasts and contributed to myogenesis by low doses of TNF-α (Donati et al., 2005, 2007). Importantly, S1P2 knockout mice had no alteration in the soleus muscle mass and morphology, however, as their body weight increased during aging stronger than that of wild-type mice, their muscle/body weight ratio decreased more strongly (Germinario et al., 2012). In a model of soleus muscle degeneration by the myotoxic compound notexin, knockout of or antagonism at S1P2 delayed muscle regeneration and decreased the expression of the promyogenic marker myogenin (Germinario et al., 2012). It was finally shown that S1P2 activated quiescent satellite cells and facilitated muscle regeneration via STAT3 (signal transducer and activator of transcription-3; Saba and de la Garza-Rodea, 2013). Taken together, it will be important to analyze the impact of a long-term treatment with an S1P2 antagonist on skeletal muscle homeostasis.
Cancer
It appears obvious that inhibition of a receptor which acts anti-migratory and often anti-proliferative would result in enhanced tumorigenesis and particularly in metastasis. Indeed, lack or mutation of S1P2 is linked to B cell lymphoma, as explained above (Cattoretti et al., 2009). In an early study with B16 melanoma cells which endogenously express only S1P2, pretreatment of the cells with S1P before implantation as well as daily i.p. application of S1P reduced lung metastasis, and stable overexpression of S1P2 enhanced this effect (Yamaguchi et al., 2003). Remarkably, this study not only shows an anti-metastatic role of S1P2, but also contradicts the anti-tumor effect of anti-S1P-antibody treatment reported in many studies (e.g., Ader et al., 2015, for review see Sabbadini, 2011). Furthermore, lung carcinoma and melanoma cells implanted into S1P2 knockout mice showed accelerated tumor growth, enhanced angiogenesis and more efficient recruitment of CD11b-positive bone marrow-derived cells into the tumors (Du et al., 2010). However, several reports underline a rather pro-cancerous role of S1P2 (Table 1): although S1P2 inhibited migration of glioma cells, it enhanced the expression of the matricellular protein CCN1/Cyr61 and stimulated glioma cell invasiveness and adhesion (Young and van Brocklyn, 2007). In chronic myeloid leukemia, S1P2 enhanced Bcr–Abl1 stability via inhibition of protein phosphatase-2a, and inhibition of SphK1/S1P2 restored the chemosensitivity of leukemia allografts in mice (Salas et al., 2011). S1P2 also increased the chemoresistance of colon carcinoma cells (Kawahara et al., 2013). Inhibition of S1P2 upregulated breast carcinoma metastasis suppressor-1, which in turn suppressed lung metastasis of bladder carcinoma cells (Ponnusamy et al., 2012). Furthermore, pancreatic cancer cells implanted together with S1P2 receptor-deficient pancreatic stellate cells showed less cancer growth and metastasis in vivo (Bi et al., 2014). Moreover, the above-mentioned phosphorylation of ERM proteins mediated by S1P2 was suggested to take part in cancer cell invasion (Adada et al., 2015). Finally, S1P2-mediated activation of the Hippo pathway might be pro-cancerous (Miller et al., 2012; Yu et al., 2012; see above). As metastasis of epithelium-derived tumors has been linked to basal rather than apical epithelial extrusion, it is interesting to note that the S1P2 antagonist, JTE-013, preferentially inhibited apical extrusion (Gu et al., 2011), although the proposed role of reduced S1P production in basal extrusion of oncogenic K-Ras-expressing cells remains unclear as S1P production by these cells has not been quantified (Slattum et al., 2014). Taken together, apart from the specific situation with S1P2 in B-cell lymphoma, the presently available studies suggest that the S1P2 receptor controls mechanisms which both promote and prevent tumor growth, invasion, and metastasis. Therefore, for development of S1P2 antagonists, thorough studies with the development candidates are required to address their potential for promotion of tumor growth and metastasis.
Conclusion
As described in the present review, the S1P2 receptor plays an important role in many tissues and organs, and therefore, a therapeutic application of S1P2 antagonists will necessarily cause unintended effects. Here, an attempt is made to suggest potential therapeutic indications for S1P2 antagonists, and to point out the potential risks that have to be taken into account during drug development. First of all, the available data suggest that selective S1P2 receptor antagonists might be helpful in pulmonary hypertension, chronic obstructive pulmonary disease, and lung fibrosis. Similarly, portal hypertension and liver fibrosis, which often occur in conjunction, might be an indication. Although S1P2 antagonists will probably improve the vascular endothelial barrier, they are likely to cause problems in conditions with critical systemic arterial hypotension, such as sepsis or anaphylaxis. Finally, an interesting approach will be to test the therapeutic potential of S1P2 antagonists in diabetes mellitus type 2 with insulin resistance and progressive β-cell loss, and to see whether atherosclerosis is also attenuated in these patients. As the proposed indications require a chronic treatment, the potential long-term adverse effects need to be carefully addressed. Potentially devastating effects in the central nervous system, such as neuronal hyperexcitability, seizures, memory deficits, and increased anxiety (MacLennan et al., 2001; Akahoshi et al., 2011), suggested by observations with S1P2 knockout mice or JTE-013 treatment, must be avoided by appropriate chemical–pharmaceutical engineering, i.e., by designing compounds that do not cross the blood brain barrier. Other critical points are inner ear functionality and B cell lymphoma. However, a full receptor knockout cannot be compared to the activity that can be reached with a (competitive) receptor antagonist. In this regard, it is important that mice that were heterozygous for the S1P2 receptor did not develop B cell lymphoma, and that hearing impairment due to S1P2 missense mutations in humans was autosomal-recessive. While the potential of S1P2 antagonists to induce or exacerbate tumor growth and metastasis has to be addressed carefully, these agents might also act protective in cancer. For instance, in a recent study with a novel S1P2 antagonist, which is a derivative of JTE-013 with improved properties, the compound inhibited the growth of neuroblastoma xenografts (Li et al., 2015).
Indeed, a major problem in this research area is the lack of appropriate tools. As discussed above, the widely used S1P2 antagonist, JTE-013 (Osada et al., 2002), has a low potency and lacks selectivity and stability in vivo (Long et al., 2010; Li et al., 2015). Other more recently synthesized S1P2 agonists and antagonists still await further characterization (Satsu et al., 2013; Kusumi et al., 2015; Li et al., 2015). In conclusion, the discovery of novel highly selective antagonists with high affinity at S1P2 is urgently required to address pending questions regarding the roles and effects of S1P2, and to enable definite conclusions with regard to the therapeutic suitability of these drugs.
Author Contributions
All authors listed, have made substantial, direct and intellectual contribution to the work, and approved it for publication.
Funding
The authors are supported by the Deutsche Forschungsgemeinschaft (SFB 1039), and Fondation Leducq (JP).
Conflict of Interest Statement
The authors declare that the research was conducted in the absence of any commercial or financial relationships that could be construed as a potential conflict of interest.
References
Adada, M., Canals, D., Hannun, Y. A., and Obeid, L. M. (2013). Sphingosine-1-phosphate receptor 2. FEBS J. 280, 6354–6366. doi: 10.1111/febs.12446
Adada, M. M., Canals, D., Jeong, N., Kelkar, A. D., Hernandez-Corbacho, M., Pulkoski-Gross, M. J., et al. (2015). Intracellular sphingosine kinase 2-derived sphingosine-1-phosphate mediates epidermal growth factor-induced ezrin-radixin-moesin phosphorylation and cancer cell invasion. FASEB J. 29, 4654–4669. doi: 10.1096/fj.15-274340
Ader, I., Gstalder, C., Bouquerel, P., Golzio, M., Andrieu, G., Zalvidea, S., et al. (2015). Neutralizing S1P inhibits intratumoral hypoxia, induces vascular remodelling and sensitizes to chemotherapy in prostate cancer. Oncotarget 6, 13803–13821. doi: 10.18632/oncotarget.3144
Adyshev, D. M., Moldobaeva, N. K., Elangovan, V. R., Garcia, J. G. N., and Dudek, S. M. (2011). Differential involvement of ezrin/radixin/moesin proteins in sphingosine 1-phosphate-induced human pulmonary endothelial cell barrier enhancement. Cell. Signal. 23, 2086–2096. doi: 10.1016/j.cellsig.2011.08.003
Aguilar, A., and Saba, J. D. (2012). Truth and consequences of sphingosine-1-phosphate lyase. Adv. Biol. Regul. 52, 17–30. doi: 10.1016/j.advenzreg.2011.09.015
Akahoshi, N., Ishizaki, Y., Yasuda, H., Murashima, Y. L., Shinba, T., Goto, K., et al. (2011). Frequent spontaneous seizures followed by spatial working memory/anxiety deficits in mice lacking sphingosine 1-phosphate receptor 2. Epilepsy Behav. 22, 659–665. doi: 10.1016/j.yebeh.2011.09.002
Alexander, S. P., Davenport, A. P., Kelly, E., Marrion, N., Peters, J. A., Benson, H. E., et al. (2015). The concise guide to pharmacology 2015/16: G protein-coupled receptors. Br. J. Pharmacol. 172, 5744–5869. doi: 10.1111/bph.13348
Alexander, S. P. H., Benson, H. E., Faccenda, E., Pawson, A. J., Sharman, J. L., Spedding, M., et al. (2013). The concise guide to pharmacology 2013/14: transporters. Br. J. Pharmacol. 170, 1706–1796. doi: 10.1111/bph.12450
Arikawa, K., Takuwa, N., Yamaguchi, H., Sugimoto, N., Kitayama, J., Nagawa, H., et al. (2003). Ligand-dependent inhibition of B16 melanoma cell migration and invasion via endogenous S1P2 G protein-coupled receptor. Requirement of inhibition of cellular RAC activity. J. Biol. Chem. 278, 32841–32851. doi: 10.1074/jbc.M305024200
Bautista-Pérez, R., Arellano, A., Franco, M., Osorio, H., and Coronel, I. (2011). Sphingosine-1-phosphate induced vasoconstriction is increased in the isolated perfused kidneys of diabetic rats. Diabetes Res. Clin. Pract. 94, e8–e11. doi: 10.1016/j.diabres.2011.06.023
Bi, Y., Li, J., Ji, B., Kang, N., Yang, L., Simonetto, D. A., et al. (2014). Sphingosine-1-phosphate mediates a reciprocal signaling pathway between stellate cells and cancer cells that promotes pancreatic cancer growth. Am. J. Pathol. 184, 2791–2802. doi: 10.1016/j.ajpath.2014.06.023
Bigaud, M., Guerini, D., Billich, A., Bassilana, F., and Brinkmann, V. (2014). Second generation S1P pathway modulators: research strategies and clinical developments. Biochim. Biophys. Acta 1841, 745–758. doi: 10.1016/j.bbalip.2013.11.001
Blaho, V. A., and Hla, T. (2014). An update on the biology of sphingosine 1-phosphate receptors. J. Lipid Res. 55, 1596–1608. doi: 10.1194/jlr.R046300
Blom, T., Bergelin, N., Meinander, A., Löf, C., Slotte, J. P., Eriksson, J. E., et al. (2010). An autocrine sphingosine-1-phosphate signaling loop enhances NF-kappaB-activation and survival. BMC Cell Biol. 11:45. doi: 10.1186/1471-2121-11-45
Burczyk, M., Burkhalter, M. D., Blätte, T., Matysik, S., Caron, M. G., Barak, L. S., et al. (2015). Phenotypic regulation of the sphingosine 1-Phosphate receptor miles apart by G protein-coupled receptor kinase 2. Biochemistry 54, 765–775. doi: 10.1021/bi501061h
Bylund, D. B., Eikenberg, D. C., Hieble, J. P., Langer, S. Z., Lefkowitz, R. J., Minneman, K. P., et al. (1994). International union of pharmacology nomenclature of adrenoceptors. Pharmacol. Rev. 46, 121–136.
Cariou, B., Charbonnel, B., and Staels, B. (2012). Thiazolidinediones and PPARγ agonists: time for a reassessment. Trends Endocrinol. Metab. 23, 205–215. doi: 10.1016/j.tem.2012.03.001
Cattoretti, G., Mandelbaum, J., Lee, N., Chaves, A. H., Mahler, A. M., Chadburn, A., et al. (2009). Targeted disruption of the S1P2 sphingosine 1-phosphate receptor gene leads to diffuse large B-cell lymphoma formation. Cancer Res. 69, 8686–8692. doi: 10.1158/0008-5472.CAN-09-1110
Chen, J., Tang, H., Sysol, J. R., Moreno-Vinasco, L., Shioura, K. M., Chen, T., et al. (2014). The sphingosine kinase 1/sphingosine-1-phosphate pathway in pulmonary arterial hypertension. Am. J. Respir. Crit. Care Med. 190, 1032–1043. doi: 10.1164/rccm.201401-0121OC
Chiba, Y., Suzuki, K., Uechi, M., Kurihara, E., Goto, K., Sakai, H., et al. (2010). Downregulation of sphingosine-1-phosphate receptors in bronchial smooth muscle of mouse experimental asthma. Pharmacol. Res. 62, 357–363. doi: 10.1016/j.phrs.2010.05.005
Cruz-Orengo, L., Daniels, B. P., Dorsey, D., Basak, S. A., Grajales-Reyes, J. G., McCandless, E. E., et al. (2014). Enhanced sphingosine-1-phosphate receptor 2 expression underlies female CNS autoimmunity susceptibility. J. Clin. Invest. 124, 2571–2584. doi: 10.1172/JCI73408
Cui, H., Okamoto, Y., Yoshioka, K., Du, W., Takuwa, N., Zhang, W., et al. (2013). Sphingosine-1-phosphate receptor 2 protects against anaphylactic shock through suppression of endothelial nitric oxide synthase in mice. J. Aller. Clin. Immunol. 132, 1205–1214. doi: 10.1016/j.jaci.2013.07.026
Damirin, A., Tomura, H., Komachi, M., Tobo, M., Sato, K., Mogi, C., et al. (2005). Sphingosine 1-phosphate receptors mediate the lipid-induced cAMP accumulation through cyclooxygenase-2/prostaglandin I2 pathway in human coronary artery smooth muscle cells. Mol. Pharmacol. 67, 1177–1185. doi: 10.1124/mol.104.004317
Del Galdo, S., Vettel, C., Meyer zu Heringdorf, D., and Wieland, T. (2013). The activation of RhoC in vascular endothelial cells is required for the S1P receptor type 2-induced inhibition of angiogenesis. Cell. Signal. 25, 2478–2484. doi: 10.1016/j.cellsig.2013.08.017
Deniz, U., Ozkirimli, E., and Ulgen, K. O. (2015). A systematic methodology for large scale compound screening: a case study on the discovery of novel S1PL inhibitors. J. Mol. Graph. Model. 63, 110–124. doi: 10.1016/j.jmgm.2015.11.004
Donati, C., Cencetti, F., and Bruni, P. (2013). Sphingosine 1-phosphate axis: a new leader actor in skeletal muscle biology. Front. Physiol. 4:338. doi: 10.3389/fphys.2013.00338
Donati, C., Meacci, E., Nuti, F., Becciolini, L., Farnararo, M., and Bruni, P. (2005). Sphingosine 1-phosphate regulates myogenic differentiation: a major role for S1P2 receptor. FASEB J. 19, 449–451. doi: 10.1096/fj.04-1780fje
Donati, C., Nincheri, P., Cencetti, F., Rapizzi, E., Farnararo, M., and Bruni, P. (2007). Tumor necrosis factor-alpha exerts pro-myogenic action in C2C12 myoblasts via sphingosine kinase/S1P2 signaling. FEBS Lett. 581, 4384–4388. doi: 10.1016/j.febslet.2007.08.007
Du, J., Zeng, C., Li, Q., Chen, B., Liu, H., Huang, X., et al. (2012). LPS and TNF-α induce expression of sphingosine-1-phosphate receptor-2 in human microvascular endothelial cells. Pathol. Res. Pract. 208, 82–88. doi: 10.1016/j.prp.2011.11.008
Du, W., Takuwa, N., Yoshioka, K., Okamoto, Y., Gonda, K., Sugihara, K., et al. (2010). S1P(2), the G protein-coupled receptor for sphingosine-1-phosphate, negatively regulates tumor angiogenesis and tumor growth in vivo in mice. Cancer Res. 70, 772–781. doi: 10.1158/0008-5472.CAN-09-2722
Estrada, R., Zeng, Q., Lu, H., Sarojini, H., Lee, J.-F., Mathis, S. P., et al. (2008). Up-regulating sphingosine 1-phosphate receptor-2 signaling impairs chemotactic, wound-healing, and morphogenetic responses in senescent endothelial cells. J. Biol. Chem. 283, 30363–30375. doi: 10.1074/jbc.M804392200
Fayyaz, S., Henkel, J., Japtok, L., Krämer, S., Damm, G., Seehofer, D., et al. (2014a). Involvement of sphingosine 1-phosphate in palmitate-induced insulin resistance of hepatocytes via the S1P2 receptor subtype. Diabetologia 57, 373–382. doi: 10.1007/s00125-013-3123-6
Fayyaz, S., Japtok, L., and Kleuser, B. (2014b). Divergent role of sphingosine 1-phosphate on insulin resistance. Cell. Physiol. Biochem. 34, 134–147. doi: 10.1159/000362990
Fuerst, E., Foster, H. R., Ward, J. P. T., Corrigan, C. J., Cousins, D. J., and Woszczek, G. (2014). Sphingosine-1-phosphate induces pro-remodelling response in airway smooth muscle cells. Allergy 69, 1531–1539. doi: 10.1111/all.12489
Gandy, K. A. O., Canals, D., Adada, M., Wada, M., Roddy, P., Snider, A. J., et al. (2013). Sphingosine 1-phosphate induces filopodia formation through S1PR2 activation of ERM proteins. Biochem. J. 449, 661–672. doi: 10.1042/BJ20120213
Germinario, E., Peron, S., Toniolo, L., Betto, R., Cencetti, F., Donati, C., et al. (2012). S1P2 receptor promotes mouse skeletal muscle regeneration. J. Appl. Physiol. 113, 707–713. doi: 10.1152/japplphysiol.00300.2012
Gonda, K., Okamoto, H., Takuwa, N., Yatomi, Y., Okazaki, H., Sakurai, T., et al. (1999). The novel sphingosine 1-phosphate receptor AGR16 is coupled via pertussis toxin-sensitive and -insensitive G-proteins to multiple signalling pathways. Biochem. J. 337(Pt 1), 67–75. doi: 10.1042/bj3370067
Gonzalez-Cabrera, P. J., Brown, S., Studer, S. M., and Rosen, H. (2014). S1P signaling: new therapies and opportunities. F1000Prime Rep. 6:109. doi: 10.12703/P6-109
Goparaju, S. K., Jolly, P. S., Watterson, K. R., Bektas, M., Alvarez, S., Sarkar, S., et al. (2005). The S1P2 receptor negatively regulates platelet-derived growth factor-induced motility and proliferation. Mol. Cell. Biol. 25, 4237–4249. doi: 10.1128/MCB.25.10.4237-4249.2005
Green, J. A., Suzuki, K., Cho, B., Willison, L. D., Palmer, D., Allen, C. D. C., et al. (2011). The sphingosine 1-phosphate receptor S1P2 maintains the homeostasis of germinal center B cells and promotes niche confinement. Nat. Immunol. 12, 672–680. doi: 10.1038/ni.2047
Grimm, M., Tischner, D., Troidl, K., Albarrán Juárez, J., Sivaraj, K. K., Ferreirós Bouzas, N., et al. (2016). S1P2/G12/13 signaling negatively regulates macrophage activation and indirectly shapes the atheroprotective B1-Cell population. Arterioscler. Thromb. Vasc. Biol. 36, 37–48. doi: 10.1161/ATVBAHA.115.306066
Gu, Y., Forostyan, T., Sabbadini, R., and Rosenblatt, J. (2011). Epithelial cell extrusion requires the sphingosine-1-phosphate receptor 2 pathway. J. Cell Biol. 193, 667–676. doi: 10.1083/jcb.201010075
Hannun, Y. A., and Obeid, L. M. (2008). Principles of bioactive lipid signalling: lessons from sphingolipids. Nature Rev. Mol. Cell Biol. 9, 139–150. doi: 10.1038/nrm2329
Herr, D. R., Grillet, N., Schwander, M., Rivera, R., Müller, U., and Chun, J. (2007). Sphingosine 1-phosphate (S1P) signaling is required for maintenance of hair cells mainly via activation of S1P2. J. Neurosci. 27, 1474–1478. doi: 10.1523/JNEUROSCI.4245-06.2007
Herr, D. R., Reolo, M. J. Y., Peh, Y. X., Wang, W., Lee, C.-W., Rivera, R., et al. (2016). Sphingosine 1-phosphate receptor 2 (S1P2) attenuates reactive oxygen species formation and inhibits cell death. Implications for otoprotective therapy. Sci. Rep. 6:24541. doi: 10.1038/srep24541
Hoefer, J., Azam, M. A., Kroetsch, J. T. E., Leong-Poi, H., Momen, M. A., Voigtlaender-Bolz, J., et al. (2010). Sphingosine-1-phosphate-dependent activation of p38 MAPK maintains elevated peripheral resistance in heart failure through increased myogenic vasoconstriction. Circ. Res. 107, 923–933. doi: 10.1161/CIRCRESAHA.110.226464
Hou, J., Chen, Q., Zhang, K., Cheng, B., Xie, G., Wu, X., et al. (2015). Sphingosine 1-phosphate receptor 2 signaling suppresses macrophage phagocytosis and impairs host defense against sepsis. Anesthesiology 123, 409–422. doi: 10.1097/ALN.0000000000000725
Hu, W., Mahavadi, S., Huang, J., Li, F., and Murthy, K. S. (2006). Characterization of S1P1 and S1P2 receptor function in smooth muscle by receptor silencing and receptor protection. Am. J. Physiol. Gastrointest. Liver Physiol. 291, G605–G610. doi: 10.1152/ajpgi.00147.2006
Igarashi, J., and Michel, T. (2009). Sphingosine-1-phosphate and modulation of vascular tone. Cardiovasc. Res. 82, 212–220. doi: 10.1093/cvr/cvp064
Ikeda, H., Nagashima, K., Yanase, M., Tomiya, T., Arai, M., Inoue, Y., et al. (2004). Sphingosine 1-phosphate enhances portal pressure in isolated perfused liver via S1P2 with Rho activation. Biochem. Biophys. Res. Commun. 320, 754–759. doi: 10.1016/j.bbrc.2004.04.207
Ikeda, H., Watanabe, N., Ishii, I., Shimosawa, T., Kume, Y., Tomiya, T., et al. (2009). Sphingosine 1-phosphate regulates regeneration and fibrosis after liver injury via sphingosine 1-phosphate receptor 2. J. Lipid Res. 50, 556–564. doi: 10.1194/jlr.M800496-JLR200
Imasawa, T., Koike, K., Ishii, I., Chun, J., and Yatomi, Y. (2010). Blockade of sphingosine 1-phosphate receptor 2 signaling attenuates streptozotocin-induced apoptosis of pancreatic beta-cells. Biochem. Biophys. Res. Commun. 392, 207–211. doi: 10.1016/j.bbrc.2010.01.016
Imeri, F., Fallegger, D., Zivkovic, A., Schwalm, S., Enzmann, G., Blankenbach, K., et al. (2014). Novel oxazolo-oxazole derivatives of FTY720 reduce endothelial cell permeability, immune cell chemotaxis and symptoms of experimental autoimmune encephalomyelitis in mice. Neuropharmacology 85, 314–327. doi: 10.1016/j.neuropharm.2014.05.012
Inoki, I., Takuwa, N., Sugimoto, N., Yoshioka, K., Takata, S., Kaneko, S., et al. (2006). Negative regulation of endothelial morphogenesis and angiogenesis by S1P2 receptor. Biochem. Biophys. Res. Commun. 346, 293–300. doi: 10.1016/j.bbrc.2006.05.119
Japtok, L., Schaper, K., Bäumer, W., Radeke, H. H., Jeong, S. K., and Kleuser, B. (2012). Sphingosine 1-phosphate modulates antigen capture by murine Langerhans cells via the S1P2 receptor subtype. PLoS ONE 7:e49427. doi: 10.1371/journal.pone.0049427
Japtok, L., Schmitz, E. I., Fayyaz, S., Krämer, S., Hsu, L. J., and Kleuser, B. (2015). Sphingosine 1-phosphate counteracts insulin signaling in pancreatic β-cells via the sphingosine 1-phosphate receptor subtype 2. FASEB J. 29, 3357–3369. doi: 10.1096/fj.14-263194
Jessup, C. F., Bonder, C. S., Pitson, S. M., and Coates, P. T. H. (2011). The sphingolipid rheostat: a potential target for improving pancreatic islet survival and function. Endocr. Metab. Immune Disord. Drug Targets 11, 262–272. doi: 10.2174/187153011797881201
Jolly, P. S., Bektas, M., Olivera, A., Gonzalez-Espinosa, C., Proia, R. L., Rivera, J., et al. (2004). Transactivation of sphingosine-1-phosphate receptors by FcepsilonRI triggering is required for normal mast cell degranulation and chemotaxis. J. Exp. Med. 199, 959–970. doi: 10.1084/jem.20030680
Kageyama, Y., Ikeda, H., Watanabe, N., Nagamine, M., Kusumoto, Y., Yashiro, M., et al. (2012). Antagonism of sphingosine 1-phosphate receptor 2 causes a selective reduction of portal vein pressure in bile duct-ligated rodents. Hepatology 56, 1427–1438. doi: 10.1002/hep.25780
Kawahara, S., Otsuji, Y., Nakamura, M., Murakami, M., Murate, T., Matsunaga, T., et al. (2013). Sphingosine kinase 1 plays a role in the upregulation of CD44 expression through extracellular signal-regulated kinase signaling in human colon cancer cells. Anticancer. Drugs 24, 473–483. doi: 10.1097/CAD.0b013e32835f705f
Kempf, A., Tews, B., Arzt, M. E., Weinmann, O., Obermair, F. J., Pernet, V., et al. (2014). The sphingolipid receptor S1PR2 is a receptor for Nogo-a repressing synaptic plasticity. PLoS Biol. 12:e1001763. doi: 10.1371/journal.pbio.1001763
Kendig, D. M., Matsumoto, A. K., and Moreland, R. S. (2013). Sphingosine-1-phosphate induced contraction of bladder smooth muscle. Eur. J. Pharmacol. 720, 355–362. doi: 10.1016/j.ejphar.2013.10.004
Kerage, D., Brindley, D. N., and Hemmings, D. G. (2014). Review: novel insights into the regulation of vascular tone by sphingosine 1-phosphate. Placenta 35(Suppl.), S86–S92. doi: 10.1016/j.placenta.2013.12.006
Kihara, Y., Maceyka, M., Spiegel, S., and Chun, J. (2014). Lysophospholipid receptor nomenclature review: IUPHAR Review 8. Br. J. Pharmacol. 171, 3575–3594. doi: 10.1111/bph.12678
Kihara, Y., Mizuno, H., and Chun, J. (2015). Lysophospholipid receptors in drug discovery. Exp. Cell Res. 333, 171–177. doi: 10.1016/j.yexcr.2014.11.020
Kim, G. S., Yang, L., Zhang, G., Zhao, H., Selim, M., McCullough, L. D., et al. (2015). Critical role of sphingosine-1-phosphate receptor-2 in the disruption of cerebrovascular integrity in experimental stroke. Nat. Commun. 6:7893. doi: 10.1038/ncomms8893
Kono, M., Belyantseva, I. A., Skoura, A., Frolenkov, G. I., Starost, M. F., Dreier, J. L., et al. (2007). Deafness and stria vascularis defects in S1P2 receptor-null mice. J. Biol. Chem. 282, 10690–10696. doi: 10.1074/jbc.M700370200
Kono, M., Mi, Y., Liu, Y., Sasaki, T., Allende, M. L., Wu, Y.-P., et al. (2004). The sphingosine-1-phosphate receptors S1P1, S1P2, and S1P3 function coordinately during embryonic angiogenesis. J. Biol. Chem. 279, 29367–29373. doi: 10.1074/jbc.M403937200
Kume, H., Takeda, N., Oguma, T., Ito, S., Kondo, M., Ito, Y., et al. (2007). Sphingosine 1-phosphate causes airway hyper-reactivity by rho-mediated myosin phosphatase inactivation. J. Pharmacol. Exp. Ther. 320, 766–773. doi: 10.1124/jpet.106.110718
Kunkel, G. T., Maceyka, M., Milstien, S., and Spiegel, S. (2013). Targeting the sphingosine-1-phosphate axis in cancer, inflammation and beyond. Nat. Rev. Drug Discov. 12, 688–702. doi: 10.1038/nrd4099
Kusumi, K., Shinozaki, K., Yamaura, Y., Hashimoto, A., Kurata, H., Naganawa, A., et al. (2015). Discovery of novel S1P2 antagonists. Part 2: improving the profile of a series of 1,3-bis(aryloxy)benzene derivatives. Bioorg. Med. Chem. Lett. 25, 4387–4392. doi: 10.1016/j.bmcl.2015.09.022
Lai, W.-Q., Wong, W. S. F., and Leung, B. P. (2011). Sphingosine kinase and sphingosine 1-phosphate in asthma. Biosci. Rep. 31, 145–150. doi: 10.1042/BSR20100087
Lepley, D., Paik, J.-H., Hla, T., and Ferrer, F. (2005). The G protein-coupled receptor S1P2 regulates Rho/Rho kinase pathway to inhibit tumor cell migration. Cancer Res. 65, 3788–3795. doi: 10.1158/0008-5472.CAN-04-2311
Levkau, B. (2015). HDL-S1P: cardiovascular functions, disease-associated alterations, and therapeutic applications. Front. Pharmacol. 6:243. doi: 10.3389/fphar.2015.00243
Li, C., Chi, X. X., Xie, W., Strong, J. A., Zhang, J.-M., and Nicol, G. D. (2012). Sphingosine 1-phosphate receptor 2 antagonist JTE-013 increases the excitability of sensory neurons independently of the receptor. J. Neurophysiol. 108, 1473–1483. doi: 10.1152/jn.00825.2011
Li, M.-H., Sanchez, T., Milne, G. L., Morrow, J. D., Hla, T., and Ferrer, F. (2009). S1P/S1P2 signaling induces cyclooxygenase-2 expression in Wilms tumor. J. Urol. 181, 1347–1352. doi: 10.1016/j.juro.2008.10.140
Li, M.-H., Swenson, R., Harel, M., Jana, S., Stolarzewicz, E., Hla, T., et al. (2015). Antitumor activity of a novel sphingosine-1-Phosphate 2 antagonist, AB1, in Neuroblastoma. J. Pharmacol. Exp. Ther. 354, 261–268. doi: 10.1124/jpet.115.224519
Liu, R., Li, X., Luo, L., Qiang, X., Hylemon, P. B., Jiang, Z., et al. (2015). Taurocholate induces Cyclooxygenase-2 expression via the sphingosine 1-phosphate receptor 2 in a human cholangiocarcinoma cell line. J. Biol. Chem. 290, 30988–31002. doi: 10.1074/jbc.M115.668277
Liu, R., Zhao, R., Zhou, X., Liang, X., Campbell, D. J. W., Zhang, X., et al. (2014). Conjugated bile acids promote cholangiocarcinoma cell invasive growth through activation of sphingosine 1-phosphate receptor 2. Hepatology 60, 908–918. doi: 10.1002/hep.27085
Long, J. S., Fujiwara, Y., Edwards, J., Tannahill, C. L., Tigyi, G., Pyne, S., et al. (2010). Sphingosine 1-phosphate receptor 4 uses HER2 (ERBB2) to regulate extracellular signal regulated kinase-1/2 in MDA-MB-453 breast cancer cells. J. Biol. Chem. 285, 35957–35966. doi: 10.1074/jbc.M110.117945
Lorenz, J. N., Arend, L. J., Robitz, R., Paul, R. J., and MacLennan, A. J. (2007). Vascular dysfunction in S1P2 sphingosine 1-phosphate receptor knockout mice. Am. J. Physiol. Regul. Integr. Comp. Physiol. 292, R440–R446. doi: 10.1152/ajpregu.00085.2006
Lu, H., Yuan, H., Chen, S., Huang, L., Xiang, H., Yang, G., et al. (2012). Senescent endothelial dysfunction is attributed to the up-regulation of sphingosine-1-phosphate receptor-2 in aged rats. Mol. Cell. Biochem. 363, 217–224. doi: 10.1007/s11010-011-1173-y
Maceyka, M., Harikumar, K. B., Milstien, S., and Spiegel, S. (2012). Sphingosine-1-phosphate signaling and its role in disease. Trends Cell Biol. 22, 50–60. doi: 10.1016/j.tcb.2011.09.003
MacLennan, A. J., Benner, S. J., Andringa, A., Chaves, A. H., Rosing, J. L., Vesey, R., et al. (2006). The S1P2 sphingosine 1-phosphate receptor is essential for auditory and vestibular function. Hear. Res. 220, 38–48. doi: 10.1016/j.heares.2006.06.016
MacLennan, A. J., Carney, P. R., Zhu, W. J., Chaves, A. H., Garcia, J., Grimes, J. R., et al. (2001). An essential role for the H218/AGR16/Edg-5/LP(B2) sphingosine 1-phosphate receptor in neuronal excitability. Eur. J. Neurosci. 14, 203–209. doi: 10.1046/j.0953-816x.2001.01634.x
MacLennan, A. J., Devlin, B. K., Marks, L., Gaskin, A. A., Neitzel, K. L., and Lee, N. (2000). Antisense studies in PC12 cells suggest a role for H218, a sphingosine 1-phosphate receptor, in growth-factor-induced cell-cell interaction and neurite outgrowth. Dev. Neurosci. 22, 283–295. doi: 10.1159/000017452
Means, C. K., Xiao, C.-Y., Li, Z., Zhang, T., Omens, J. H., Ishii, I., et al. (2007). Sphingosine 1-phosphate S1P2 and S1P3 receptor-mediated Akt activation protects against in vivo myocardial ischemia-reperfusion injury. Am. J. Physiol. Heart Circ. Physiol. 292, H2944–H2951. doi: 10.1152/ajpheart.01331.2006
Meyer zu Heringdorf, D., Ihlefeld, K., and Pfeilschifter, J. (2013). Pharmacology of the sphingosine-1-phosphate signalling system. Handb. Exp. Pharmacol. 215, 239–253. doi: 10.1007/978-3-7091-1368-4_13
Michaud, J., Im, D.-S., and Hla, T. (2010). Inhibitory role of sphingosine 1-phosphate receptor 2 in macrophage recruitment during inflammation. J. Immunol. 184, 1475–1483. doi: 10.4049/jimmunol.0901586
Miller, E., Yang, J., DeRan, M., Wu, C., Su, A. I., Bonamy, G. M. C., et al. (2012). Identification of serum-derived sphingosine-1-phosphate as a small molecule regulator of YAP. Chem. Biol. 19, 955–962. doi: 10.1016/j.chembiol.2012.07.005
Moon, M.-H., Jeong, J.-K., and Park, S.-Y. (2015). Activation of S1P2 receptor, a possible mechanism of inhibition of adipogenic differentiation by sphingosine 1-phosphate. Mol. Med. Rep. 11, 1031–1036. doi: 10.3892/mmr.2014.2810
Moriyama, S., Takahashi, N., Green, J. A., Hori, S., Kubo, M., Cyster, J. G., et al. (2014). Sphingosine-1-phosphate receptor 2 is critical for follicular helper T cell retention in germinal centers. J. Exp. Med. 211, 1297–1305. doi: 10.1084/jem.20131666
Nakayama, M., Tabuchi, K., Hoshino, T., Nakamagoe, M., Nishimura, B., and Hara, A. (2014). The influence of sphingosine-1-phosphate receptor antagonists on gentamicin-induced hair cell loss of the rat cochlea. Neurosci. Lett. 561, 91–95. doi: 10.1016/j.neulet.2013.12.063
Newton, J., Lima, S., Maceyka, M., and Spiegel, S. (2015). Revisiting the sphingolipid rheostat: evolving concepts in cancer therapy. Exp. Cell Res. 333, 195–200. doi: 10.1016/j.yexcr.2015.02.025
Nishi, T., Kobayashi, N., Hisano, Y., Kawahara, A., and Yamaguchi, A. (2014). Molecular and physiological functions of sphingosine 1-phosphate transporters. Biochim. Biophys. Acta 1841, 759–765. doi: 10.1016/j.bbalip.2013.07.012
Ohmori, T., Yatomi, Y., Osada, M., Kazama, F., Takafuta, T., Ikeda, H., et al. (2003). Sphingosine 1-phosphate induces contraction of coronary artery smooth muscle cells via S1P2. Cardiovasc. Res. 58, 170–177. doi: 10.1016/S0008-6363(03)00260-8
Okamoto, H., Takuwa, N., Yokomizo, T., Sugimoto, N., Sakurada, S., Shigematsu, H., et al. (2000). Inhibitory regulation of Rac activation, membrane ruffling, and cell migration by the G protein-coupled sphingosine-1-phosphate receptor EDG5 but not EDG1 or EDG3. Mol. Cell. Biol. 20, 9247–9261. doi: 10.1128/MCB.20.24.9247-9261.2000
Okazaki, H., Ishizaka, N., Sakurai, T., Kurokawa, K., Goto, K., Kumada, M., et al. (1993). Molecular cloning of a novel putative G protein-coupled receptor expressed in the cardiovascular system. Biochem. Biophys. Res. Commun. 190, 1104–1109. doi: 10.1006/bbrc.1993.1163
Olivera, A., Allende, M. L., and Proia, R. L. (2013a). Shaping the landscape: metabolic regulation of S1P gradients. Biochim. Biophys. Acta 1831, 193–202. doi: 10.1016/j.bbalip.2012.06.007
Olivera, A., Dillahunt, S. E., and Rivera, J. (2013b). Interrogation of sphingosine-1-phosphate receptor 2 function in vivo reveals a prominent role in the recovery from IgE and IgG-mediated anaphylaxis with minimal effect on its onset. Immunol. Lett. 150, 89–96. doi: 10.1016/j.imlet.2013.01.005
Olivera, A., Eisner, C., Kitamura, Y., Dillahunt, S., Allende, L., Tuymetova, G., et al. (2010). Sphingosine kinase 1 and sphingosine-1-phosphate receptor 2 are vital to recovery from anaphylactic shock in mice. J. Clin. Invest. 120, 1429–1440. doi: 10.1172/JCI40659
Orr Gandy, K. A., and Obeid, L. M. (2013). Targeting the sphingosine kinase/sphingosine 1-phosphate pathway in disease: review of sphingosine kinase inhibitors. Biochim. Biophys. Acta 1831, 157–166. doi: 10.1016/j.bbalip.2012.07.002
Osada, M., Yatomi, Y., Ohmori, T., Ikeda, H., and Ozaki, Y. (2002). Enhancement of sphingosine 1-phosphate-induced migration of vascular endothelial cells and smooth muscle cells by an EDG-5 antagonist. Biochem. Biophys. Res. Commun. 299, 483–487. doi: 10.1016/S0006-291X(02)02671-2
Oskeritzian, C. A., Hait, N. C., Wedman, P., Chumanevich, A., Kolawole, E. M., Price, M. M., et al. (2015). The sphingosine-1-phosphate/sphingosine-1-phosphate receptor 2 axis regulates early airway T-cell infiltration in murine mast cell-dependent acute allergic responses. J. Allergy Clin. Immunol. 135, 1008-18.e1. doi: 10.1016/j.jaci.2014.10.044
Oskeritzian, C. A., Price, M. M., Hait, N. C., Kapitonov, D., Falanga, Y. T., Morales, J. K., et al. (2010). Essential roles of sphingosine-1-phosphate receptor 2 in human mast cell activation, anaphylaxis, and pulmonary edema. J. Exp. Med. 207, 465–474. doi: 10.1084/jem.20091513
O’Sullivan, M. J., Hirota, N., and Martin, J. G. (2014). Sphingosine 1-phosphate (S1P) induced interleukin-8 (IL-8) release is mediated by S1P receptor 2 and nuclear factor κB in BEAS-2B cells. PLoS ONE 9:e95566. doi: 10.1371/journal.pone.0095566
Ota, H., Beutz, M. A., Ito, M., Abe, K., Oka, M., and McMurtry, I. F. (2011). S1P(4) receptor mediates S1P-induced vasoconstriction in normotensive and hypertensive rat lungs. Pulm. Circ. 1, 399–404. doi: 10.4103/2045-8932.87309
Ponnusamy, S., Selvam, S. P., Mehrotra, S., Kawamori, T., Snider, A. J., Obeid, L. M., et al. (2012). Communication between host organism and cancer cells is transduced by systemic sphingosine kinase 1/sphingosine 1-phosphate signalling to regulate tumour metastasis. EMBO Mol. Med. 4, 761–775. doi: 10.1002/emmm.201200244
Proia, R. L., and Hla, T. (2015). Emerging biology of sphingosine-1-phosphate: its role in pathogenesis and therapy. J. Clin. Invest. 125, 1379–1387. doi: 10.1172/JCI76369
Pyne, N. J., McNaughton, M., Boomkamp, S., MacRitchie, N., Evangelisti, C., Martelli, A. M., et al. (2015). Role of sphingosine 1-phosphate receptors, sphingosine kinases and sphingosine in cancer and inflammation. Adv. Biol. Regul. 60, 151–159. doi: 10.1016/j.jbior.2015.09.001
Rapizzi, E., Taddei, M. L., Fiaschi, T., Donati, C., Bruni, P., and Chiarugi, P. (2009). Sphingosine 1-phosphate increases glucose uptake through trans-activation of insulin receptor. Cell. Mol. Life Sci. 66, 3207–3218. doi: 10.1007/s00018-009-0106-3
Roberts, E., Guerrero, M., Urbano, M., and Rosen, H. (2013). Sphingosine 1-phosphate receptor agonists: a patent review (2010-2012). Expert Opin. Ther. Pat. 23, 817–841. doi: 10.1517/13543776.2013.783022
Rosenfeldt, H. M., Amrani, Y., Watterson, K. R., Murthy, K. S., Panettieri, R. A., and Spiegel, S. (2003). Sphingosine-1-phosphate stimulates contraction of human airway smooth muscle cells. FASEB J. 17, 1789–1799. doi: 10.1096/fj.02-0836com
Roviezzo, F., Brancaleone, V., Gruttola, L., De Vellecco, V., Bucci, M., D’Agostino, B., et al. (2011). Sphingosine-1-phosphate modulates vascular permeability and cell recruitment in acute inflammation in vivo. J. Pharmacol. Exp. Ther. 337, 830–837. doi: 10.1124/jpet.111.179168
Roviezzo, F., D’Agostino, B., Brancaleone, V., Gruttola, L., de Bucci, M., De Dominicis, G., et al. (2010). Systemic administration of sphingosine-1-phosphate increases bronchial hyperresponsiveness in the mouse. Am. J. Respir. Cell Mol. Biol. 42, 572–577. doi: 10.1165/rcmb.2009-0108OC
Roviezzo, F., Di Lorenzo, A., Bucci, M., Brancaleone, V., Vellecco, V., De Nardo, M., et al. (2007). Sphingosine-1-phosphate/sphingosine kinase pathway is involved in mouse airway hyperresponsiveness. Am. J. Respir. Cell Mol. Biol. 36, 757–762. doi: 10.1165/rcmb.2006-0383OC
Roviezzo, F., Sorrentino, R., Bertolino, A., Gruttola, L., de Terlizzi, M., and Pinto, A. (2015). S1P-induced airway smooth muscle hyperresponsiveness and lung inflammation in vivo: molecular and cellular mechanisms. Br. J. Pharmacol. 172, 1882–1893. doi: 10.1111/bph.13033
Rumzhum, N. N., Rahman, M. M., Oliver, B. G., and Ammit, A. J. (2016). Effect of Sphingosine 1-Phosphate on Cyclo-Oxygenase-2 Expression, Prostaglandin E2 Secretion, and β2-Adrenergic Receptor Desensitization. Am. J. Respir. Cell Mol. Biol. 54, 128–135. doi: 10.1165/rcmb.2014-0443OC
Saba, J. D., and de la Garza-Rodea, A. S. (2013). S1P lyase in skeletal muscle regeneration and satellite cell activation: exposing the hidden lyase. Biochim. Biophys. Acta 1831, 167–175. doi: 10.1016/j.bbalip.2012.06.009
Sabbadini, R. A. (2011). Sphingosine-1-phosphate antibodies as potential agents in the treatment of cancer and age-related macular degeneration. Br. J. Pharmacol. 162, 1225–1238. doi: 10.1111/j.1476-5381.2010.01118.x
Salas, A., Ponnusamy, S., Senkal, C. E., Meyers-Needham, M., Selvam, S. P., Saddoughi, S. A., et al. (2011). Sphingosine kinase-1 and sphingosine 1-phosphate receptor 2 mediate Bcr-Abl1 stability and drug resistance by modulation of protein phosphatase 2A. Blood 117, 5941–5952. doi: 10.1182/blood-2010-08-300772
Salomone, S., Potts, E. M., Tyndall, S., Ip, P. C., Chun, J., Brinkmann, V., et al. (2008). Analysis of sphingosine 1-phosphate receptors involved in constriction of isolated cerebral arteries with receptor null mice and pharmacological tools. Br. J. Pharmacol. 153, 140–147. doi: 10.1038/sj.bjp.0707581
Sammani, S., Moreno-Vinasco, L., Mirzapoiazova, T., Singleton, P. A., Chiang, E. T., Evenoski, C. L., et al. (2010). Differential effects of sphingosine 1-phosphate receptors on airway and vascular barrier function in the murine lung. Am. J. Respir. Cell Mol. Biol. 43, 394–402. doi: 10.1165/rcmb.2009-0223OC
Sanchez, T., Skoura, A., Wu, M. T., Casserly, B., Harrington, E. O., and Hla, T. (2007). Induction of vascular permeability by the sphingosine-1-phosphate receptor-2 (S1P2R) and its downstream effectors ROCK and PTEN. Arterioscler. Thromb. Vasc. Biol. 27, 1312–1318. doi: 10.1161/ATVBAHA.107.143735
Sanchez, T., Thangada, S., Wu, M.-T., Kontos, C. D., Wu, D., Wu, H., et al. (2005). PTEN as an effector in the signaling of antimigratory G protein-coupled receptor. Proc. Natl. Acad. Sci. U.S.A. 102, 4312–4317. doi: 10.1073/pnas.0409784102
Santos-Cortez, R. L., Faridi, R., Rehman, A. U., Lee, K., Ansar, M., Wang, X., et al. (2016). Autosomal-recessive hearing impairment due to rare missense variants within S1PR2. Am. J. Hum. Genet. 98, 331–338. doi: 10.1016/j.ajhg.2015.12.004
Satsu, H., Schaeffer, M.-T., Guerrero, M., Saldana, A., Eberhart, C., Hodder, P., et al. (2013). A sphingosine 1-phosphate receptor 2 selective allosteric agonist. Bioorg. Med. Chem. 21, 5373–5382. doi: 10.1016/j.bmc.2013.06.012
Sauer, S. (2015). Ligands for the nuclear peroxisome proliferator-activated receptor gamma. Trends Pharmacol. Sci. 36, 688–704. doi: 10.1016/j.tips.2015.06.010
Schnute, M. E., McReynolds, M. D., Kasten, T., Yates, M., Jerome, G., Rains, J. W., et al. (2012). Modulation of cellular S1P levels with a novel, potent and specific inhibitor of sphingosine kinase-1. Biochem. J. 444, 79–88. doi: 10.1042/BJ20111929
Schüppel, M., Kürschner, U., Kleuser, U., Schäfer-Korting, M., and Kleuser, B. (2008). Sphingosine 1-phosphate restrains insulin-mediated keratinocyte proliferation via inhibition of Akt through the S1P2 receptor subtype. J. Invest. Dermatol. 128, 1747–1756. doi: 10.1038/sj.jid.5701259
Schwalm, S., Pfeilschifter, J., and Huwiler, A. (2013). Sphingosine-1-phosphate: a Janus-faced mediator of fibrotic diseases. Biochim. Biophys. Acta 1831, 239–250. doi: 10.1016/j.bbalip.2012.07.022
Serriere-Lanneau, V., Teixeira-Clerc, F., Li, L., Schippers, M., Wries, W., and de Julien, B. (2007). The sphingosine 1-phosphate receptor S1P2 triggers hepatic wound healing. FASEB J. 21, 2005–2013. doi: 10.1096/fj.06-6889com
Shea, B. S., Brooks, S. F., Fontaine, B. A., Chun, J., Luster, A. D., and Tager, A. M. (2010). Prolonged exposure to sphingosine 1-phosphate receptor-1 agonists exacerbates vascular leak, fibrosis, and mortality after lung injury. Am. J. Respir. Cell Mol. Biol. 43, 662–673. doi: 10.1165/rcmb.2009-0345OC
Shimizu, T., Nakazawa, T., Cho, A., Dastvan, F., Shilling, D., Daum, G., et al. (2007). Sphingosine 1-phosphate receptor 2 negatively regulates neointimal formation in mouse arteries. Circ. Res. 101, 995–1000. doi: 10.1161/CIRCRESAHA.107.159228
Siehler, S., Wang, Y., Fan, X., Windh, R. T., and Manning, D. R. (2001). Sphingosine 1-phosphate activates nuclear factor-kappa B through Edg receptors. Activation through Edg-3 and Edg-5, but not Edg-1, in human embryonic kidney 293 cells. J. Biol. Chem. 276, 48733–48739. doi: 10.1074/jbc.M011072200
Skoura, A., and Hla, T. (2009). Regulation of vascular physiology and pathology by the S1P2 receptor subtype. Cardiovasc. Res. 82, 221–228. doi: 10.1093/cvr/cvp088
Skoura, A., Michaud, J., Im, D.-S., Thangada, S., Xiong, Y., Smith, J. D., et al. (2011). Sphingosine-1-phosphate receptor-2 function in myeloid cells regulates vascular inflammation and atherosclerosis. Arterioscler. Thromb. Vasc. Biol. 31, 81–85. doi: 10.1161/ATVBAHA.110.213496
Skoura, A., Sanchez, T., Claffey, K., Mandala, S. M., Proia, R. L., and Hla, T. (2007). Essential role of sphingosine 1-phosphate receptor 2 in pathological angiogenesis of the mouse retina. J. Clin. Invest. 117, 2506–2516. doi: 10.1172/JCI31123
Slattum, G., Gu, Y., Sabbadini, R., and Rosenblatt, J. (2014). Autophagy in oncogenic K-Ras promotes basal extrusion of epithelial cells by degrading S1P. Curr. Biol. 24, 19–28. doi: 10.1016/j.cub.2013.11.029
Spiegel, S. (2000). Sphingosine 1-Phosphate. A Ligand for the EDG-1 Family of G-Protein-Coupled Receptors. Ann. N. Y. Acad. Sci. 905, 54–60. doi: 10.1111/j.1749-6632.2000.tb06537.x
Spiegel, S., English, D., and Milstien, S. (2002). Sphingosine 1-phosphate signaling: providing cells with a sense of direction. Trends Cell Biol. 12, 236–242. doi: 10.1016/S0962-8924(02)02277-8
Stradner, M. H., Gruber, G., Angerer, H., Huber, V., Setznagl, D., Kremser, M.-L., et al. (2013). Sphingosine 1-phosphate counteracts the effects of interleukin-1β in human chondrocytes. Arthritis Rheum. 65, 2113–2122. doi: 10.1002/art.37989
Studer, E., Zhou, X., Zhao, R., Wang, Y., Takabe, K., Nagahashi, M., et al. (2012). Conjugated bile acids activate the sphingosine-1-phosphate receptor 2 in primary rodent hepatocytes. Hepatology 55, 267–276. doi: 10.1002/hep.24681
Sugimoto, N., Takuwa, N., Okamoto, H., Sakurada, S., and Takuwa, Y. (2003). Inhibitory and stimulatory regulation of Rac and cell motility by the G12/13-Rho and Gi pathways integrated downstream of a single G protein-coupled sphingosine-1-phosphate receptor isoform. Mol. Cell. Biol. 23, 1534–1545. doi: 10.1128/MCB.23.5.1534-1545.2003
Szczepaniak, W. S., Pitt, B. R., and McVerry, B. J. (2010). S1P2 receptor-dependent Rho-kinase activation mediates vasoconstriction in the murine pulmonary circulation induced by sphingosine 1-phosphate. Am. J. Physiol. Lung Cell. Mol. Physiol. 299, L137–L145. doi: 10.1152/ajplung.00233.2009
Takashima, S.-I., Sugimoto, N., Takuwa, N., Okamoto, Y., Yoshioka, K., Takamura, M., et al. (2008). G12/13 and Gq mediate S1P2-induced inhibition of Rac and migration in vascular smooth muscle in a manner dependent on Rho but not Rho kinase. Cardiovasc. Res. 79, 689–697. doi: 10.1093/cvr/cvn118
Tamama, K., Tomura, H., Sato, K., Malchinkhuu, E., Damirin, A., Kimura, T., et al. (2005). High-density lipoprotein inhibits migration of vascular smooth muscle cells through its sphingosine 1-phosphate component. Atherosclerosis 178, 19–23. doi: 10.1016/j.atherosclerosis.2004.07.032
Ter Braak, M., Claas, R. F., Hegen, B., Labocha, S., Ferreirós, N., Pfeilschifter, J., et al. (2011). Cis-4-methylsphingosine is a sphingosine-1-phosphate receptor modulator. Biochem. Pharmacol. 81, 617–625. doi: 10.1016/j.bcp.2010.12.002
Toman, R. E., Payne, S. G., Watterson, K. R., Maceyka, M., Lee, N. H., Milstien, S., et al. (2004). Differential transactivation of sphingosine-1-phosphate receptors modulates NGF-induced neurite extension. J. Cell Biol. 166, 381–392. doi: 10.1083/jcb.200402016
Trifilieff, A., Baur, F., and Fozard, J. R. (2009). Role of sphingosine-1-phosphate (S1P) and the S1P(2) receptor in allergen-induced, mast cell-dependent contraction of rat lung parenchymal strips. Naunyn-Schmiedeberg’s Arch. Pharmacol. 380, 303–309. doi: 10.1007/s00210-009-0438-4
Völzke, A., Koch, A., Meyer zu Heringdorf, D., Huwiler, A., and Pfeilschifter, J. (2014). Sphingosine 1-phosphate (S1P) induces COX-2 expression and PGE2 formation via S1P receptor 2 in renal mesangial cells. Biochim. Biophys. Acta 1841, 11–21. doi: 10.1016/j.bbalip.2013.09.009
Wamhoff, B. R., Lynch, K. R., Macdonald, T. L., and Owens, G. K. (2008). Sphingosine-1-phosphate receptor subtypes differentially regulate smooth muscle cell phenotype. Arterioscler. Thromb. Vasc. Biol. 28, 1454–1461. doi: 10.1161/ATVBAHA.107.159392
Wünsche, C., Koch, A., Goldschmeding, R., Schwalm, S., Meyer zu Heringdorf, D., Huwiler, A., et al. (2015). Transforming growth factor β2 (TGF-β2)-induced connective tissue growth factor (CTGF) expression requires sphingosine 1-phosphate receptor 5 (S1P5) in human mesangial cells. Biochim. Biophys. Acta 1851, 519–526. doi: 10.1016/j.bbalip.2015.01.003
Xing, X.-Q., Li, Y.-L., Zhang, Y.-X., Xiao, Y., Li, Z.-D., Liu, L.-Q., et al. (2015). Sphingosine kinase 1/sphingosine 1-phosphate signalling pathway as a potential therapeutic target of pulmonary hypertension. Int. J. Clin. Exp. Med. 8, 11930–11935.
Xiong, Y., and Hla, T. (2014). S1P control of endothelial integrity. Curr. Top. Microbiol. Immunol. 378, 85–105. doi: 10.1007/978-3-319-05879-5_4
Yamaguchi, H., Kitayama, J., Takuwa, N., Arikawa, K., Inoki, I., Takehara, K., et al. (2003). Sphingosine-1-phosphate receptor subtype-specific positive and negative regulation of Rac and haematogenous metastasis of melanoma cells. Biochem. J. 374, 715–722. doi: 10.1042/BJ20030381
Ye, S., and Eisinger-Mathason, T. S. K. (2016). Targeting the hippo pathway: clinical implications and therapeutics. Pharmacol. Res. 103, 270–278. doi: 10.1016/j.phrs.2015.11.025
Yki-Jarvinen, H. (2004). Thiazolidinediones. N. Engl. J. Med. 351, 1106–1118. doi: 10.1056/NEJMra041001
Young, N., and van Brocklyn, J. R. (2007). Roles of sphingosine-1-phosphate (S1P) receptors in malignant behavior of glioma cells. Differential effects of S1P2 on cell migration and invasiveness. Exp. Cell Res. 313, 1615–1627. doi: 10.1016/j.yexcr.2007.02.009
Yu, F.-X., Zhao, B., Panupinthu, N., Jewell, J. L., Lian, I., Wang, L. H., et al. (2012). Regulation of the Hippo-YAP pathway by G-protein-coupled receptor signaling. Cell 150, 780–791. doi: 10.1016/j.cell.2012.06.037
Zhang, G., Yang, L., Kim, G. S., Ryan, K., Lu, S., O’Donnell, R. K., et al. (2013). Critical role of sphingosine-1-phosphate receptor 2 (S1PR2) in acute vascular inflammation. Blood 122, 443–455. doi: 10.1182/blood-2012-11-467191
Zhang, K., Qi, H.-X., Hu, Z.-M., Chang, Y.-N., Shi, Z.-M., Han, X.-H., et al. (2015). YAP and TAZ take center stage in cancer. Biochemistry 54, 6555–6566. doi: 10.1021/acs.biochem.5b01014
Zhang, W., An, J., Jawadi, H., Siow, D. L., Lee, J.-F., and Zhao, J. (2013). Sphingosine-1-phosphate receptor-2 mediated NFκB activation contributes to tumor necrosis factor-α induced VCAM-1 and ICAM-1 expression in endothelial cells. Prostaglandins Other Lipid Mediat. 106, 62–71. doi: 10.1016/j.prostaglandins.2013.06.001
Keywords: sphingosine-1-phosphate, G-protein-coupled receptors, vascular tone, endothelial barrier, insulin sensitivity
Citation: Blankenbach KV, Schwalm S, Pfeilschifter J and Meyer zu Heringdorf D (2016) Sphingosine-1-Phosphate Receptor-2 Antagonists: Therapeutic Potential and Potential Risks. Front. Pharmacol. 7:167. doi: 10.3389/fphar.2016.00167
Received: 04 April 2016; Accepted: 03 June 2016;
Published: 21 June 2016.
Edited by:
Christian Waeber, Harvard Medical School, USAReviewed by:
Paola Bruni, Università degli studi di Firenze, ItalyBodo Levkau, University of Duisburg-Essen, Germany
Copyright © 2016 Blankenbach, Schwalm, Pfeilschifter and Meyer zu Heringdorf. This is an open-access article distributed under the terms of the Creative Commons Attribution License (CC BY). The use, distribution or reproduction in other forums is permitted, provided the original author(s) or licensor are credited and that the original publication in this journal is cited, in accordance with accepted academic practice. No use, distribution or reproduction is permitted which does not comply with these terms.
*Correspondence: Dagmar Meyer zu Heringdorf, aGVyaW5nZG9yZkBtZWQudW5pLWZyYW5rZnVydC5kZQ==