- 1Institut National de la Santé et de la Recherche Médicale, UMR 1087, l'Institut du Thorax, Nantes, France
- 2Centre National de la Recherche Scientifique, UMR 6291, Nantes, France
- 3Université de Nantes, Nantes, France
- 4Institut National de la Santé et de la Recherche Médicale, U1127, Paris, France
- 5Sorbonne Universités, Université Pierre-et-Marie-Curie, UMR S1127, Paris, France
- 6Centre National de la Recherche Scientifique, UMR 7225, Paris, France
- 7Institut du Cerveau et de la Moelle Épinière, ICM, Paris, France
- 8Assistance Publique – Hôpitaux de Paris (AP-HP), Centres de Référence des Canalopathies Musculaires et des Maladies Neuro-musculaires Paris-Est, Paris, France
- 9Assistance Publique – Hôpitaux de Paris (AP-HP), Hôpital de la Pitié Salpêtrière, Service de Biochimie Métabolique, Unité de Cardiogénétique et Myogénétique, Paris, France
- 10Centre Hospitalier Universitaire de Nantes, Centre de Référence Maladies Neuromusculaires Nantes-Angers, Nantes, France
- 11Atlantic Gene Therapies - Biotherapy Institute for Rare Diseases, Nantes, France
- 12Centre Hospitalier Universitaire de Nantes, l'Institut du Thorax, Nantes, France
Mutations in Nav1.4 and Nav1.5 α-subunits have been associated with muscular and cardiac channelopathies, respectively. Despite intense research on the structure and function of these channels, a lot of information is still missing to delineate the various physiological and pathophysiological processes underlying their activity at the molecular level. Nav1.4 and Nav1.5 sequences are similar, suggesting structural and functional homologies between the two orthologous channels. This also suggests that any characteristics described for one channel subunit may shed light on the properties of the counterpart channel subunit. In this review article, after a brief clinical description of the muscular and cardiac channelopathies related to Nav1.4 and Nav1.5 mutations, respectively, we compare the knowledge accumulated in different aspects of the expression and function of Nav1.4 and Nav1.5 α-subunits: the regulation of the two encoding genes (SCN4A and SCN5A), the associated/regulatory proteins and at last, the functional effect of the same missense mutations detected in Nav1.4 and Nav1.5. First, it appears that more is known on Nav1.5 expression and accessory proteins. Because of the high homologies of Nav1.5 binding sites and equivalent Nav1.4 sites, Nav1.5-related results may guide future investigations on Nav1.4. Second, the analysis of the same missense mutations in Nav1.4 and Nav1.5 revealed intriguing similarities regarding their effects on membrane excitability and alteration in channel biophysics. We believe that such comparison may bring new cues to the physiopathology of cardiac and muscular diseases.
Voltage-gated sodium channels (Nav) constitute a family of 10 members in mammals, Nav1.1 to Nav1.9 and Nax, expressed in a large variety of tissues. In excitable cells such as striated myocytes, they initiate action potentials that, in heart as well as in skeletal muscles, trigger, and regulate the contraction. Because of their key role in this function, mutations impacting their activity have tremendous consequences. This review compares the knowledge accumulated in different aspects of the expression and function of Nav1.4 and Nav1.5 α-subunits, and focuses on “homologous” mutations i.e., in the same (aligned) amino acids of the skeletal muscle Nav1.4 and of the cardiac Nav1.5 leading to a large range of muscular and cardiac disorders also called channelopathies.
Clinical Description of the Main Nav1.4 and Nav1.5 Related Pathologies
Clinical Description of Nav1.4 Related Channelopathies
Nav1.4, which is encoded by the SCN4A gene, is the pore-forming subunit of the main sodium channel present in skeletal muscles. Nav1.4 related channelopathies that affect skeletal muscle excitability (Vicart et al., 2005; Jurkat-Rott et al., 2010; Nicole and Fontaine, 2015) are dominant diseases classified in two opposite groups as defined by the prevalent clinical symptoms: muscle stiffness and hypertonia (myotonia) episodes [non dystrophic myotonias (NDM)], and muscle weakness resulting in paralysis episodes (periodic paralyses; PP). It should be noted that similar clinical pattern are also associated with other channelopathies involving chloride channels (NDM) or calcium channels (PP). Table 1 summarizes the main classes of Nav1.4-related skeletal muscle channelopathies. Detailed clinical, electromyographic (Fournier et al., 2004, 2006), genetic and, in fine, pathophysiological analyses have led to distinguish several entities among skeletal muscle sodium channelopathies.
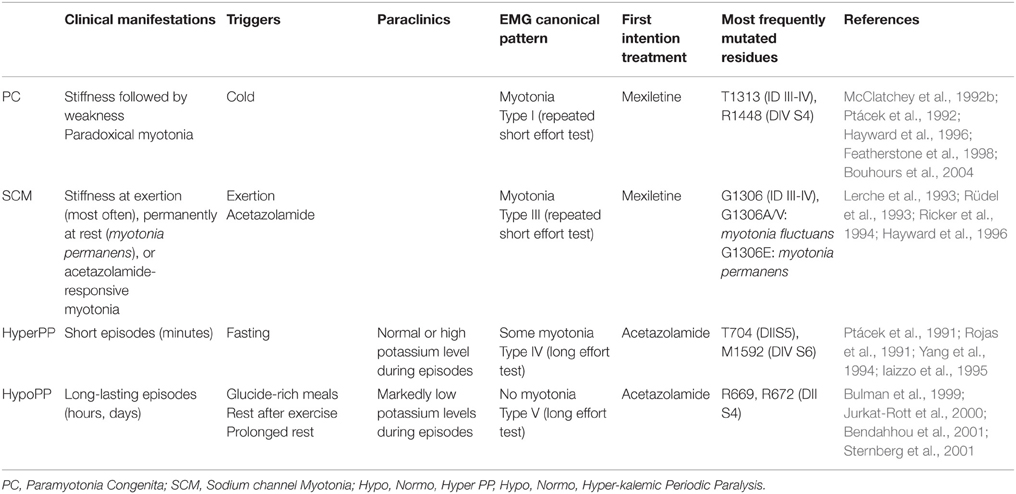
Table 1. Main classes of Nav1.4 skeletal muscle channelopathies (Trip et al., 2009; Raja Rayan and Hanna, 2010).
Nav1.4-Related non Dystrophic Myotonias
Myotonia may occur at the beginning of effort and be alleviated (myotonia, with warm-up effect), or aggravated (paradoxical myotonia, also named paramyotonia) by continuing effort. Those myotonic or paramyotonic symptoms are associated with myotonic discharges analyzed with electromyographic investigations. NDM are opposed to dystrophic myotonias as observed in Steinert (Myotonic Dystrophy type 1, DM1) and PROMM (PROximal Myotonic Myopathy or Myotonic Dystrophy type 2, DM2) diseases. Among NDM, at least two entities differ clinically and electromyographically (Trip et al., 2009; Raja Rayan and Hanna, 2010).
- Paramyotonia congenita (PC) consists of cold-induced stiffness often associated with some weakness of face and extremities muscles, and paradoxical myotonia; it is associated with a progressive decrease of compound muscle action potential (CMAP) amplitude during repetitive short efforts test at EMG (pattern I according to Fournier, Fournier et al., 2004).
- Sodium channel myotonias (SCM) regroup the remaining dominant sodium channel-related myotonias that are not significantly cold-sensitive or paradoxical, and do not exhibit any change of CMAP amplitude during repetitive short efforts test at EMG (pattern III according to Fournier); this SCM entity was initially termed “potassium-aggravated myotonia” as potassium load triggers myotonia in some cases. This group was further subdivided into three types: myotonia permanens, myotonia fluctuans, and acetazolamide-responsive myotonia. While this classification is not used in clinics, it has some relevance: myotonia permanens designates myotonia that is present permanently, even at rest; myotonia fluctuans designates myotonia that appears and disappears at some moment, with no systematic concomitance with exertion, a peculiar circumstance being exercise-induced delayed-onset myotonia, that occurs some time after exertion has stopped; acetazolamide-responsive myotonia is a treatment-related designation, that underlines the fact that some SCM are treatable by acetazolamide.
Nav1.4-Related Periodic Paralysis
Among PP, two distinct entities are recognized (Raja Rayan and Hanna, 2010): hypokalemic periodic paralysis (HypoPP) is characterized by a marked hypokalemia concomitant with paralysis episodes, and, on the opposite, hyperkalemic periodic paralysis (HyperPP) is associated with a tendency to high blood potassium levels during the paralysis episodes. From the electromyographic point of view, both are characterized by a marked decrease of CMAP amplitude after a 5 min-long effort (long effort test, also referred to as McManis test).
Overlap, borderline or mixed syndromes between PP and NDM or between their subtypes have been reported (McClatchey et al., 1992a; Sugiura et al., 2003; Webb and Cannon, 2008; Yoshinaga et al., 2012). The age at onset is usually in early to late childhood. Neonatal symptoms are not classically reported in the most frequent Nav1.4 channelopathies, but dominant de novo mutations are reported in moderate to severe neonatal clinical presentations such as severe neonatal episodic laryngospasm (SNEL) (Lion-Francois et al., 2010). In a general way, respiratory symptoms are not common in PP and NDM, however a small number of patients are exposed to laryngeal or diaphragmatic weakness or myotonia that may be symptomatic.
The minimal prevalence of skeletal muscle Nav1.4 channelopathies has been recently estimated to be 0.4:100,000 in England (Horga et al., 2013) and 1.4:100,000 in France. Mutations in Nav1.4 are mostly missense or rarely in-frame deletions or insertions, usually with a dominant effect. However exceptional recessive homozygosity (Arnold et al., 2015) and a possible recessive compound heterozygosity (Tsujino et al., 2003) have been reported in congenital myasthenic syndromes. A small number of canonical mutations account for a significant percentage of cases (Table 1), e.g., T1313M and R1448C/H for PC, T704M for HyperPP, V445M (Rosenfeld et al., 1997), V1293I (Koch et al., 1995), and G1306A/V/E for SCM, mutations of domains II and III S4 arginines (IIS4 and IIIS4) at position 669 (R>H), 672 (R>H/G/C/S), 1132 (R>Q) (Carle et al., 2006), 1135 (R>H) for HypoPP (Matthews et al., 2009). Mutations at IIS4 arginine 675 (R>Q/G/W) result in a special type of PP with both features of HyperPP and HypoPP (Vicart et al., 2004). However, beside those frequent canonical mutations, more than 70 different missense mutations at more than 55 different positions in different domains of the protein have been reported in the literature as causative mutations for Nav1.4 channelopathies. The penetrance of Nav1.4 dominant mutations is variable for each mutation: it is high for HyperPP (T704M), PC (T1313M/A and R1448C/H) and SCM (V445M and V1293I) mutations, and lower, with cases of gender-related non-penetrance in pedigrees, for some other mutations such as HypoPP mutations at position 669 or 672 (Ke et al., 2013).
Clinical Description of Nav1.5 Related Channelopathies
Nav1.5, which is encoded by the SCN5A gene, is the pore-forming subunit of the main cardiac sodium channel. Nav1.5 related channelopathies affecting cardiac excitability are dominant diseases that, similarly to Nav1.4 in the skeletal muscles, impact cardiac excitability through loss of function or gain of function effects on Nav1.5 activity. Table 2 summarizes the Nav1.5 related channelopathies that are discussed in this review, which only considers pathologies provoked by mutations in the same, i.e., aligned amino acids in Nav1.4 and Nav1.5 (cf. Part Comparison of Missense Mutations. Are there (dys-)Functional Homologies between Nav1.4 and Nav1.5?): the Brugada syndrome (BrS), the long QT syndrome (LQTS), and arrhythmic dilated cardiomyopathy. The latter includes a novel form of cardiac arrhythmia characterized by multifocal ectopic Purkinje-related premature contractions (MEPPCs), associated or not with atrial fibrillation and dilated cardiomyopathy. Consequently, Table 2 is not an exhaustive list of Nav1.5 related channelopathies.
The Brugada Syndrome
The BrS is a primary electrical disorder that is characterized by a specific ECG pattern consisting of ST-segment elevation followed by a negative T-wave in the right precordial leads (Brugada and Brugada, 1992), indicating abnormal electrical activity in the upper part of the right ventricle (right ventricular outflow tract). This ECG pattern is associated with an increased risk of sudden cardiac death (SCD) resulting from polymorphic ventricular tachyarrhythmias or ventricular fibrillation. The incidence of BrS in the general population is currently estimated at 1:2000 (Antzelevitch et al., 2005). This syndrome is 8–10 times more prevalent in males than in females and typically manifests during adulthood, with a mean age of SCD of 41 ± 15 years (Antzelevitch et al., 2005). BrS was first described as a monogenic disease, with autosomal dominant transmission. Although more than 20 genes have been proposed as causally related to BrS, mutations in these genes explain less than 30% of the cases (Crotti et al., 2012; Nielsen et al., 2013; Antzelevitch and Yan, 2015; Veerman et al., 2015). Around 25% of BrS patients possess a mutation in SCN5A. So far, ≈300 mutations in SCN5A have been reported as related to BrS (http://www.ncbi.nlm.nih.gov/clinvar). These mutations lead to a loss of Nav1.5 function and reduce Na+ current (INa). Besides BrS, loss-of-function mutations in SCN5A also cause isolated cardiac conduction disease and sinus node dysfunction (Remme et al., 2008). ECG signs of conduction defects are also a common feature of BrS. The other genes identified so far are coding for proteins that are involved in generating or regulating the sodium current (Antzelevitch and Yan, 2015), the L-type calcium current (Antzelevitch et al., 2007; Burashnikov et al., 2010; Béziau et al., 2014) or the transient outward potassium current (Delpón et al., 2008; Giudicessi et al., 2011).
If BrS was first described as a monogenic autosomal dominant disease, there is accumulating evidence suggesting that it follows a more complex genetic model. Concerning SCN5A, segregation studies performed in large affected pedigrees demonstrate that mutations in this gene are characterized by a low penetrance (47%). In some instances, a single SCN5A mutation can lead to different cardiac arrhythmia phenotypes in the same family or even in a single patient (Kyndt et al., 2001; Probst et al., 2009). Moreover, in some pedigrees, the absence of the familial SCN5A mutation is observed in some affected family members, suggesting other origins for the disease (Probst et al., 2009). Recently, a genome-wide association study in a large cohort of BrS patients has provided the proof of concept that common genetic variants outside the SCN5A gene, e.g., SCN10A and HEY2 loci in the reported study, may have a large effect on the development of the disease (Bezzina et al., 2013). Altogether, these data suggest that the BrS most probably involves combined contribution of different gene variants of variable impact.
The Long QT Syndrome
Congenital LQTS is defined by several criteria including a prolongation of the QT interval corrected for heart rate, i.e., QTc, to values above 440 ms in males and 460 ms in females, due to prolonged ventricular action potentials. LQTS patients are predisposed to ventricular polymorphic tachyarrhythmias (torsades de pointes) that may lead to syncope, seizure or SCD (Amin et al., 2013). The most common form of LQTS (also called Romano-Ward syndrome) is an autosomic dominant disease. Its incidence in the population worldwide is about 1:2000 (Schwartz et al., 2009). To date, genetic defects in 15 different genes have been found in 70% of the LQTS patients (Amin et al., 2013; Giudicessi and Ackerman, 2013). Similar to BrS, the disease penetrance is most often incomplete and highly variable, ranging from 25 to 100% (Priori et al., 1999; Viadero et al., 2011). This suggests that additional genetic and non-genetic factors may modify the clinical manifestations of a given LQTS-causing mutation. In recent years, numerous studies have shown that genetic variants play an important modulatory role in establishing the disease severity (Amin et al., 2013). Among non-genetic factors, hypokalemia, or treatment with drugs inhibiting KV11.1 (hERG) channels as side effect are well known to favor arrhythmic events. Sex is also a well-known modifier of QT interval duration in LQTS. Post-adolescence and pre-menopause women have a lower repolarization reserve than men and are therefore more prone to QT interval prolongation and cardiac events. This is partially explained by the effects of sex hormones on cardiac ion channel expression and function (Tanabe et al., 1999; Zicha et al., 2003; Bai et al., 2005; Gaborit et al., 2010). The most common types of LQTS are LQTS1 (30–35% of patients; Ackerman et al., 2011), LQTS2 (25–40%), and LQTS3 (5–10%), due to defects in KCNQ1 (KV7.1 channel), KCNH2 (KV11.1), and SCN5A (Nav1.5) genes, respectively. Approximately 80% of all LQTS causal mutations are found in these three genes. Clinically, LQTS3 is characterized by unusually increased duration of the ST segment with a late appearance of the T wave (Moss, 2002). It is often more lethal, although less frequent, than LQTS1 and LQTS2 (Priori et al., 2003). Bradycardia and pauses occurring at rest or more particularly during sleep are often at the origin of the arrhythmias, although fatal tachycardia-induced arrhythmias have also been reported for a third of the patients. Most of the SCN5A mutations that were reported to be related to LQTS3 (≈200; http://www.ncbi.nlm.nih.gov/clinvar) alter the fast inactivation process of the channel, leading to persistent inward sodium current causing prolonged membrane depolarizations (Wang et al., 1995; George, 2005).
Arrhythmic Dilated Cardiomyopathy
Dilated cardiomyopathy (DCM) is characterized by systolic dysfunction and, in most patients, left ventricular enlargement or dilatation. It has been associated with the mutations of more than 30 genes, including SCN5A (McNair et al., 2011; Hershberger et al., 2013). Sixteen SCN5A mutations are linked to familial or sporadic cases with DCM with various types of arrhythmias, for example, sinus node dysfunction, conduction delay, and atrial and/or ventricular tachy-arrhythmias (Amin, 2014). Among arrhythmic DCM, the MEPPC syndrome is a recently-described autosomal dominant form of cardiac arrhythmia (Laurent et al., 2012). It is characterized by frequent premature ventricular contractions (PVCs) originating from various ectopic foci along the fascicular-Purkinje system occasionally associated with dilated cardiomyopathy, non-sustained ventricular tachycardias (NSVTs), and sudden death. A similar phenotype was first reported in 2003 by Bezzina and collaborators in a newborn boy and his diseased sister, both genotyped with Nav1.5 W156X and R225W mutations (Bezzina et al., 2003). Both parents and an elder sibling, each one carrier of one or the other mutation, were asymptomatic. For the sister, arrhythmias being the cause of the DCM is unlikely because persistent arrhythmias were only present for a short period. Two other mutations in Nav1.5 (R222Q and R225P) have been linked to this MEPPC syndrome in several families (Laurent et al., 2012; Mann et al., 2012; Nair et al., 2012; Beckermann et al., 2014). In these families, dilated cardiomyopathy, when present, was suggested as a consequence of severe primary electrical dysfunctions.
Phenotypic and Genotypic Overlap between Cardiac and Skeletal Muscle Sodium Channelopathies?
A recently published study shows that patients carrying (or not) SCN4A causative mutations, present with mixed phenotype (BrS and myotonic features) (Bissay et al., 2015). Although SCN4A transcripts are present in human ventricles (Péréon et al., 2003), it is difficult to understand how the gain of function SCN4A mutations can be compared to the loss of function of SCN5A mutations classically associated with Brugada, as discussed in the study of Bissay and collaborators. Another study on a unique family described four patients carrying a SCN4A mutation and presenting with PC (Péréon et al., 2003), two of them having slightly prolonged QTc interval. Both PC and LQTS3 are associated with a gain of function of Nav1.4 and Nav1.5, respectively. In this case, it is tempting to hypothesize that the mutant Nav1.4 channels present in the heart are responsible for the QT prolongation. Identifying more families with such overlap phenotypes would help to confirm the potential mutual influence of both channels on the pathogenesis of cardiac and muscular diseases.
Channel Molecular Bases and Gene Expression
Voltage-gated sodium channels consist of an α-subunit, constituting the pore, and accessory β-subunits controlling the expression and activity of the pore-forming subunit. Nav1.4, the most frequent Nav α-subunit expressed in the skeletal muscle is a glycosylated transmembrane protein of 1836 amino acids and has an apparent molecular weight of approximately 260 kDa (George et al., 1992a,b). Nav1.5, the most frequent Nav cardiac α-subunit is 2015–2016 amino acid long, depending on the splice variants, and has a similar apparent molecular weight (Gellens et al., 1992; Makielski et al., 2003; Balasuriya et al., 2012).
The SCN4A gene which encodes Nav1.4 is composed of 24 exons, all containing coding sequence. No alternative splicing events have been reported in the literature. Nav1.5 is encoded by the SCN5A gene, composed of 28 exons, among which exons 2–28 contain the coding sequence. Exon 1 and part of exon 2 encode the 5′ untranslated region (UTR) while exon 28 contains the 3′-UTR (Wang et al., 1996). Intron 2 of SCN4A and intron 3 of SCN5A are AT-AC type I introns. Intron 21 of SCN4A and intron 25 of SCN5A are AT-AC type II introns (Wu and Krainer, 1999). All other introns are canonical GT-AG introns. Unlike Nav1.4, mRNA variants of Nav1.5 are detected in the heart of mammals, resulting from alternative splicing. In human and murine hearts, 3′-UTRs present two different splicing variants, generating short or long poly-adenine tails (Shang and Dudley, 2005). In addition, three rare variants were identified only in human, corresponding to alternative splicing of exon 28A by exons 28B–28D coding for truncated and non-functional forms of Nav1.5 (Shang et al., 2007). To date, only the mechanisms of this splice site are understood. They involve interactions with two splicing factors, the RBM25 and LUC7F3 proteins (Gao et al., 2011; Gao and Dudley, 2013). Four and three splice variants, which differ from the canonical non-coding sequence, were described for the 5′UTR of human and mouse SCN5A mRNAs, respectively. These transcripts originate from the alternative splicing encompassing exons 1 (designated 1A, 1B, 1C, and 1D) and 2, and are preferentially expressed in the heart as compared with other tissues. Also, a neonatal isoform containing a neonatal exon 6A of 31 nucleotides has been reported. This form presents a difference of seven amino acids in the S3–S4 loop of domain I, in comparison with exon 6 of the adult form (Rook et al., 2012). Ventricular myocardial analysis displayed abnormal splicing of SCN5A exon 6, characterized by over-expression of this neonatal isoform, in one patient who present DCM with conduction system disease (Wahbi et al., 2013). These findings suggest a potential implication of mis-splicing of SCN5A in the cardiac defect observed in this patient.
Two distinct sodium currents and channels were historically described in skeletal muscle depending upon the developmental and innervation status of the myofiber. SkM1, the TTX-sensitive sodium channel expressed in innervated adult myofibers, corresponds to Nav1.4 and is the main skeletal muscle sodium channel (Trimmer et al., 1989; Kallen et al., 1990). SkM2, the TTX resistant sodium channel expressed in immature and denervated myofibers, corresponds to Nav1.5. In rodents, SCN4A expression increases just after birth concomitantly with the decrease of SCN5A gene expression (Stocksley et al., 2005). SCN4A expression is not sensitive to myofiber denervation by contrast to SCN5A gene expression, which was found to be upregulated in response to denervation (Awad et al., 2001).
The SCN4A promoter contains distinct positive-acting promoter E-box and negative-acting repressor E-box that cooperate to yield specific gene expression in differentiated skeletal myofibers (Kraner et al., 1998, 1999). It is suggested that the muscle specificity of SCN4A expression result from the binding of two basic helix-loop-helix transcription factors (bHLH) of the muscle-specific MyoD family, myogenin and MRF4 for initiation and maintenance, respectively, to the positive-acting promoter E-box located upstream the translation initiation site. NFI would be another major regulator of SCN4A gene expression acting in concert with bHLH factors, especially MRF4 (Hebert et al., 2007). The density of Nav1.4 is around 20 times higher at the neuromuscular junction (NMJ), in part as a result of local mRNA accumulation (Stocksley et al., 2005). Although the promoter element responsible for the transcriptional regulation of subsynaptic genes in response to neuronal factors at the NMJ is the N-box (TTCCGG) (Méjat et al., 2003), no N-box is present within the promoter of SCN4A, suggesting the involvement of other regulatory elements.
Similarly to alternative splicing, more is known concerning the regulation of the SCN5A promoter, compared with SCN4A. After the identification of a first promoter region for human SCN5A which includes multiple positive and negative cis-acting elements extending into intron 1 (Yang et al., 2004), two other promoter regions for murine SCN5A (designated P2 and P3) containing two distinct cardiac-specific enhancer regions were identified and functionally characterized (Shang and Dudley, 2005). In human and rat, the segment immediately upstream of the major transcription start site contains three GC boxes that could serve as binding sites for the Sp1 transcription factor, which are homologous to the CACC boxes recognized in promoters of muscle restricted genes, and an E-box binding site for bHLH factors (Yang et al., 2004). The human sequence also includes an additional C-rich motif which is recognized as a major regulator of expression in myocytes. Further, Yang and collaborators have characterized a binding site for GATA in intron 1, which is also known as a key regulator of gene expression in the heart. Surprisingly, variants in SCN10A (encoding Nav1.8 of which expression is extremely low in heart and undetectable in atrioventricular bundle) are associated with alterations of cardiac conduction parameters and BrS (van den Boogaard et al., 2014). Van den Boogaard and collaborators have shown that the SCN10A variants act more likely through an alteration SCN5A gene expression level. They have demonstrated that a cis-regulatory element located in SCN10A gene -which is immediately located next to SCN5A- was able to interact with both SCN5A and SCN10A promoters. Furthermore, they described, using healthy human heart samples, a direct correlation between the SCN5A (but not SCN10A) expression and the presence of the rs6801957 risk-associated SNP in the SCN10A intronic enhancer. Together, their data provided a genomic mechanism explaining how a common genetic variant at SCN10A locus influences cardiac physiology and predispose to Brs.
Associated/Regulatory Proteins
Although expression of Nav1.5 or Nav1.4 α-subunits alone results in the generation of functional channels in heterologous expression systems, it is now quite clear that the regulation of gating and/or expression of the Nav subunits substantially relies on a variety of other accessory/regulatory proteins (Abriel, 2010; Rook et al., 2012). Interestingly, the alignment of Nav1.5 and Nav1.4 amino acid sequences could facilitate the identification of novel associated/regulatory proteins of the counterpart channel subunit. In addition, this direct sequence comparison has contributed, as for Navβ1 (Makita et al., 1996), and will certainly continue to contribute to localizing the structural determinants involved in the channel regulation. In this respect, Table 3 and Figure 1 recapitulate the Nav1.5 or Nav1.4 amino acid sequences previously identified to mediate interaction with associated/regulatory proteins, and indicates the corresponding sequences in the other Nav α-subunit. Whereas, a number of Nav1.5 interacting proteins, with their binding sites in the channel subunit, have been described in the literature (see references in Table 3), very little is known for Nav1.4 (Figure 1). Nevertheless, it is interesting to note that the amino acid sequence similarity obtained for some binding sites is high, suggesting the possibility that both channel subunits share the same associated/regulatory proteins. This is the case for example of calmodulin, which associates with the very well conserved (100% sequence similarity) IQ-motif on both Nav1.5 and Nav1.4 C-terminal domains (Tan et al., 2002; Young and Caldwell, 2005). Most of the proteins shown to interact with Nav1.5 on a site conserved in Nav1.4 are ubiquitously expressed (dynamitin, 14-3-3, CaMKII, MOG-1, calmodulin, FGF, PTPH1/PTPN3, SAP97), suggesting that an interaction with Nav1.4 may take place in the skeletal muscle cells (Marfatia et al., 2001; Blair et al., 2006). When not ubiquitously expressed, proteins known to interact with Nav1.5 are also expressed in skeletal muscle (α actinin2) that argue for a possible interaction with Nav1.4 (Foley and Young, 2014). Conversely, weaker sequence similarity may suggest different affinities, sites or absence of interaction/regulation. This is the case for example of Navβ1 for which the region within D1/S5-S6 that confers regulation of Nav1.4 in Xenopus oocytes (Makita et al., 1996) is not very well conserved in Nav1.5 (63.1% sequence similarity) which is also regulated by Navβ1, suggesting that the structural determinants of the interaction of Nav1.5 or Nav1.4 with Navβ1 are different. Finally, it is striking to note the complete absence of the PY-motif from the C-terminus of Nav1.4. This suggests that the regulation of Nav1.4 channel internalization and/or degradation is achieved through different mechanisms as compared to Nav1.5 for which cell surface expression is regulated through the ubiquitin-proteasome pathway (van Bemmelen et al., 2004; Rougier et al., 2005). These mechanisms remain to be identified.
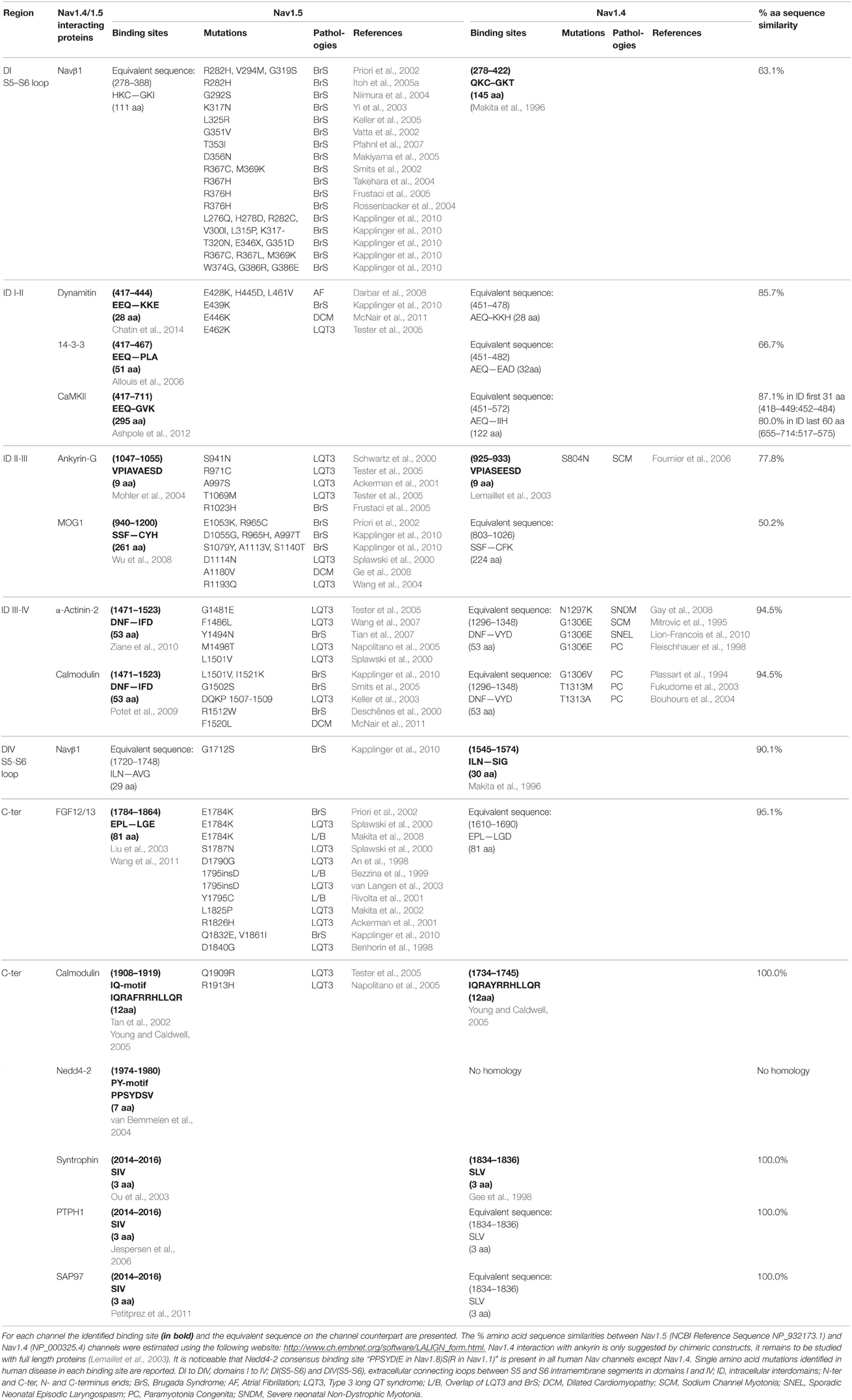
Table 3. Comparison of Nav1.5 and Nav1.4 channel associated/regulatory proteins and corresponding binding sites.
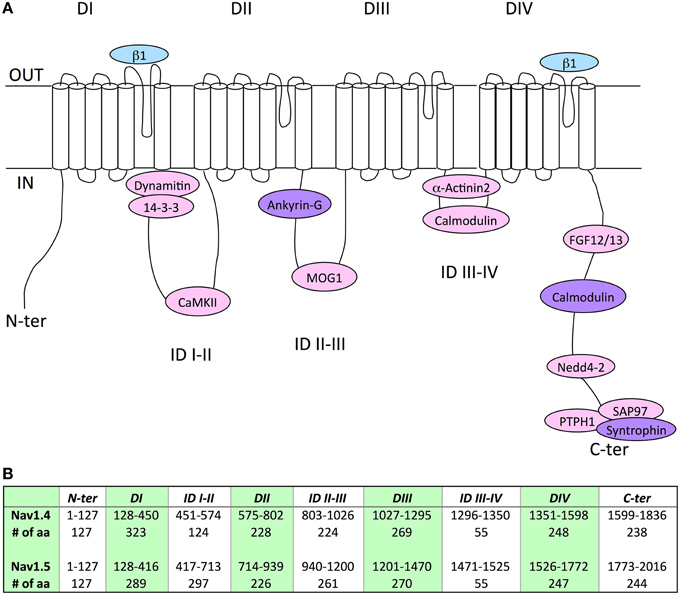
Figure 1. (A) Schematic representation of the localization of the binding sites of associated/regulatory proteins identified for Nav1.4 (blue), Nav1.5 (pink), or both (purple). Nav1.4 interaction with ankyrin is only suggested by chimeric constructs, it remains to be studied with full length proteins (Lemaillet et al., 2003). DI to DIV, domains I to IV; ID, intracellular interdomains; N-ter and C-ter, N-and C-terminus ends. (B) Table presenting the amino-acid (aa) numbering and length of specific regions and domains in Nav1.4 and Nav1.5 proteins. It is noticeable that Nav1.4 IDI-II total amino-acid length is shorter than Nav1.5 IDI-II.
Comparison of Missense Mutations. are there (dys-) Functional Homologies Between Nav1.4 and Nav1.5?
Around 300 mutations in SCN5A have been identified in patients presenting with BrS, 31% being frameshift, nonsense or splice-site mutations, and 69% being missense or rarely in-frame deletions/insertions (Kapplinger et al., 2010). When studied in patch-clamp in heterologous expression systems, mutated Nav1.5 channels are showing different types of loss of function, such as a decrease in current density, a positive shift in the activation curve, a negative shift in the inactivation curve, or a loss of regulation by PKA (Tarradas et al., 2013; Zeng et al., 2013; Aiba et al., 2014). Mutations in SCN5A have also been found in patients presenting with LQT syndrome (named LQTS3 when SCN5A is mutated). As opposed to BrS, mutated channels in LQTS3 patients show a gain a function, mainly through an increase in a persistent Na+ current (cf. Part Clinical Description of the Main Nav1.4 and Nav1.5 Related Pathologies). As a result, BrS mutations are associated to membrane hypo-excitability, whereas LQTS3 mutations are associated to prolonged action potential, referred here as membrane hyper-activity.
In the skeletal muscle, a similar binary classification is observable among the 70 mutations identified so far, that are nearly exclusively missense or rarely in-frame deletions/insertions. NDM are linked to membrane hyper-excitability, often due to defective inactivation and hence a gain of function of Nav1.4 channel activity (Clarke et al., 2011). On the contrary, hypoPP is linked to membrane hypo-excitability, and is often due to the apparition of an aberrant current through the gating pore that can be a proton or a monovalent cation current (Sokolov et al., 2007). This so-called “omega” current (or gating pore current) causes paradoxical depolarization of myofibers in low K+, which inactivates Nav1.4 and renders myofibers non excitable. Seemingly paradoxical, hyperPP is associated with gain of function of Nav1.4 (as observed for myotonia) but loss of function on skeletal muscles (paralysis). As for myotonia, defective inactivation of Nav1.4 is often observed and favors membrane depolarization. The paradox is resolved if we consider that wild type Nav1.4 channels will be more inactivated due to a slightly more depolarized membrane, thus causing a loss of sarcolemmal excitability and myofiber paralysis (Cannon, 2015). The development of myotonia or hyperPP may depend on the degree of membrane excitability. This has been suggested for instance when in the same family, females carrying the M1370V mutation develop only a myotonia (PC) whereas males are presenting with both myotonia and hyperPP (Okuda et al., 2001).
Nav1.4 and Nav1.5 are similar. If we consider the aligned region between Nav1.5 and Nav1.4, which represents 95% of Nav1.4 sequence, 67% of the amino-acids are identical. Knowing that, one can wonder whether mutations have been identified at equivalent positions in both channels, and whether, in this case, the new amino-acid is the same, such as Q270K in both Nav1.4 and Nav1.5 or V445M in Nav1.4 and V411M in Nav1.5 (V445 is aligned with V411). It is possible to use an online compilation that has been proposed using a paralog annotation approach in order to retrieve homologous or nearly homologous variants in both genes (Ware et al., 2012; Walsh et al., 2014). If the same mutations of homologous residues exist, do they give rise to similar dysfunction on both channels? If yes, we can expect that both mutations give rise to the same change in membrane excitability. For instance, if a Nav1.5 mutation leads to hyper-activity of cardiac cells (LQTS3), the corresponding mutation in Nav1.4 may also give rise to a hyper-excitability phenotype of the skeletal muscle cells such as HyperPP, PC, or SCM. Tables 4, 5 present all the corresponding amino acids found to be mutated in patients with cardiac (Nav1.5) or neuromuscular (Nav1.4) pathologies. Table 4 and Figure 2A list the mutations for which the amino acid substitutions are the same, and Table 5 and Figure 2B those for which they are divergent.
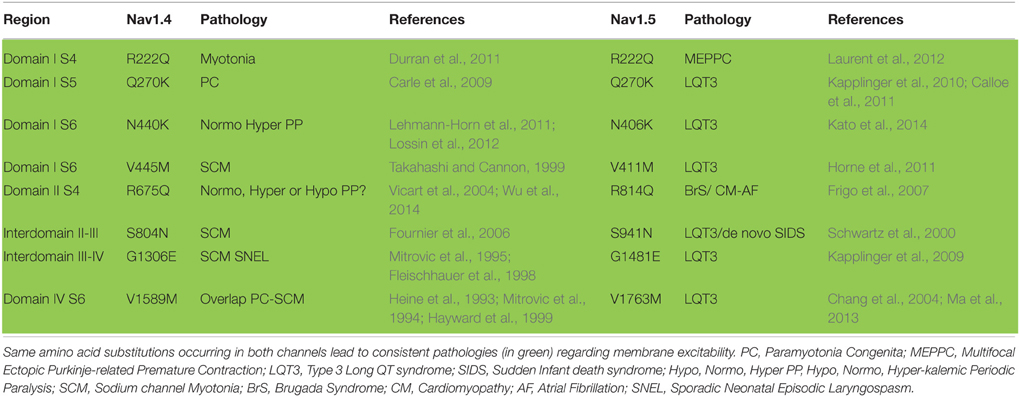
Table 4. List of equivalent amino acids found to be similarly mutated in patients with cardiac (Nav1.5) or skeletal (Nav1.4) pathologies.
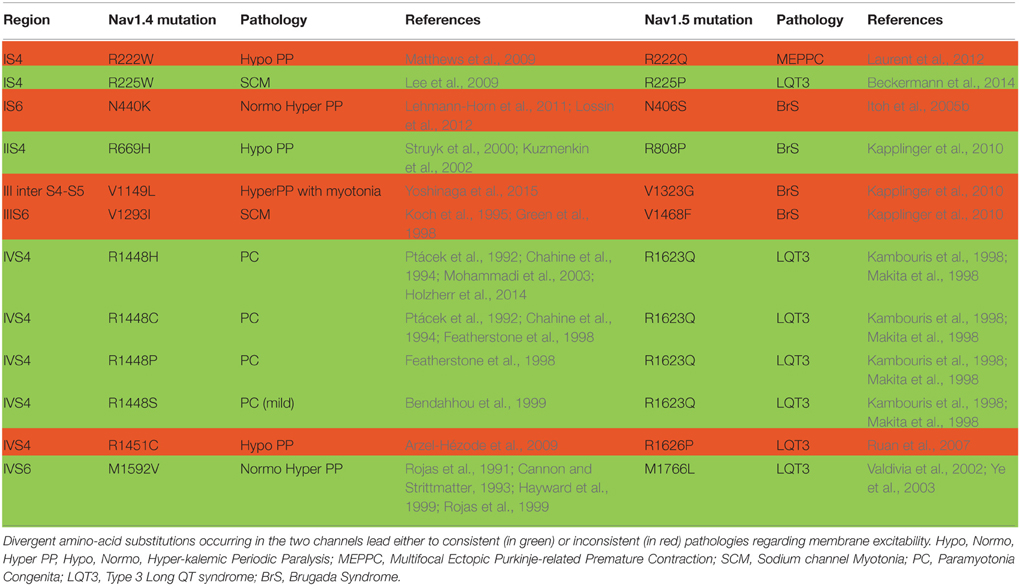
Table 5. List of equivalent amino acids found to be differently mutated in patients with cardiac (for Nav1.5) or neuromuscular (for Nav1.4) pathologies.
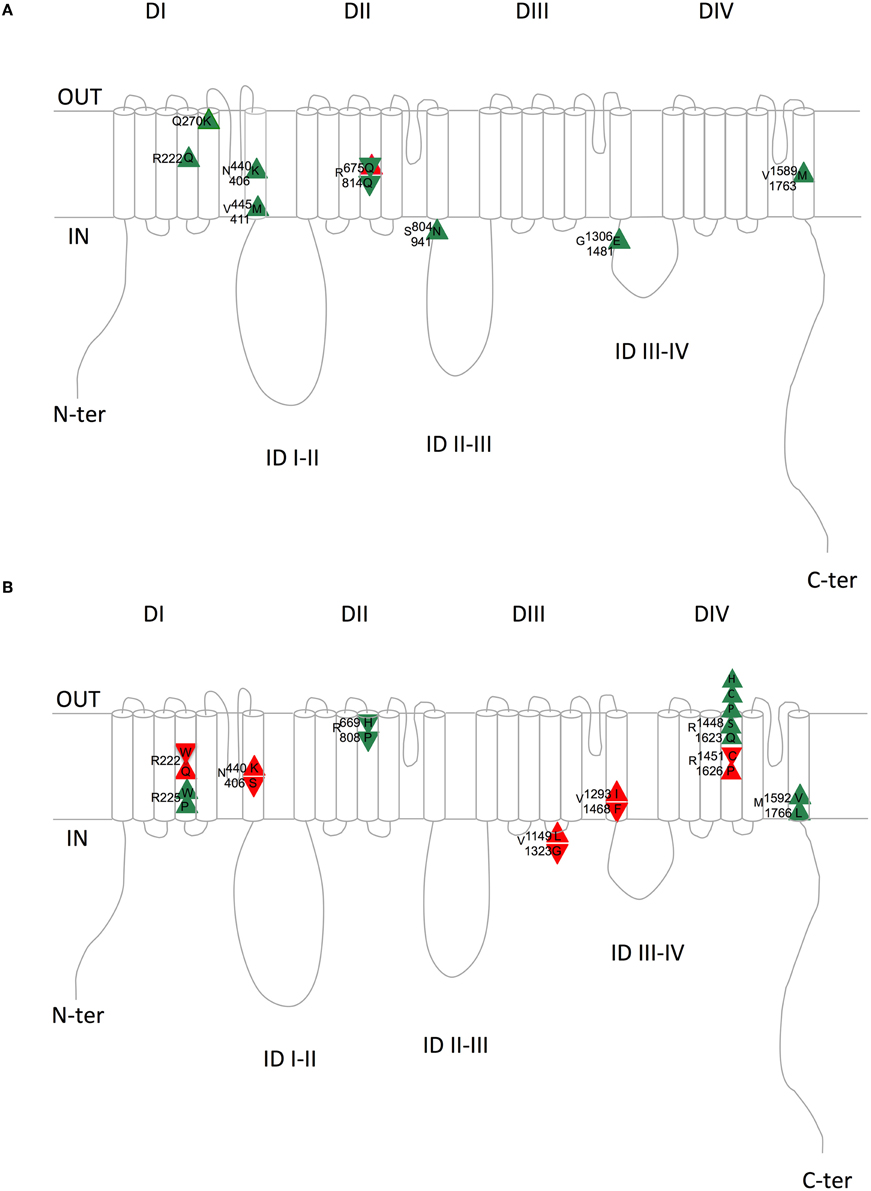
Figure 2. Schematic representation of the equivalent Nav1.4/Nav1.5 amino acids with similar (A) or divergent (B) mutations in patients with skeletal (Nav1.4) or cardiac (Nav1.5) pathologies. Upward triangles indicate a gain of function on membrane excitability and downward triangles a loss of function on membrane excitability. Green and red triangles indicate consistent and inconsistent effects on Nav1.4 and Nav1.5 regarding membrane excitability, respectively. Upper amino acid number/letter, Nav1.4; Lower amino acid number/letter, Nav1.5. DI to DIV, domains I to IV; ID, intracellular interdomains; N-ter and C-ter, N-and C-terminus ends.
When looking at Table 4 and Figure 2A, it is striking to observe that all paralog mutations give rise to clearly consistent functional effects, except one which is at first unclear, as detailed below. Indeed, all Nav1.4 mutations linked to membrane hyper-excitability (PC, SCM, and HyperPP) correspond to Nav1.5 mutations linked to membrane hyper-activity (LQTS3), except R675Q (R814Q in Nav1.5). The comparison between Nav1.4 R675Q and Nav1.5 R814Q is not obvious because the pathology induced by Nav1.4 R675Q mutation is difficult to classify as Normo/Hyper PP or Hypo PP (Vicart et al., 2004). Indeed, patients experienced normal as well as decreased potassium levels concomitant to attacks. The rat ortholog of human Nav1.4 R675Q generates an omega current activated by depolarization when expressed in Xenopus oocytes (Sokolov et al., 2008) (cf. above). The omega current represents less than 1% of the peak pore current but it remains constant after slow inactivation of the pore current and requires high hyperpolarizations to deactivate. Therefore, it is suspected that this current, carried by Na+ and K+ ions, maintained during trains of action potentials and with a residual non-deactivated activity at resting potential could lead to sodium accumulation and a decrease in membrane excitability. It will be interesting to test whether the corresponding mutation in Nav1.5 is also responsible for an omega current. Moreover, the R675Q Nav1.4 mutation gives rise to a hyperpolarizing shift of the inactivation curve and a slower recovery from inactivation when expressed in HEK293 cells. (Vicart et al., 2004; Wu et al., 2014). Altogether, these observations suggested us to rank it as a hypo-excitability causing mutation, consistent with the BrS phenotype (loss of function) induced by the homologous Nav1.5 mutation R814Q.
Table 4 and Figure 2A summarize the (dys-)functional homology between the equivalent mutant in Nav1.4 and Nav1.5. On the contrary, Table 5 and Figure 2B show that divergent amino acid substitution at the equivalent position leads to some inconsistencies (in red, 5/12). This suggests that the nature of the amino acid substitution is determinant for the direction of the functional net effect (loss or gain of function).
At last, we focused on five equivalent mutations that have been studied extensively in patch-clamp in both Nav1.4 and Nav1.5. Table 6 shows changes in each biophysical parameter for these mutations. When looking at the direction of the functional effects (gain or loss of function), we observe two major points. First, a strikingly similar functional effect of the same mutations in both channels. Second, all the gain of function mutations leading to hyper-activity/excitability provoke an increase in the persistent current, when measured, suggesting that this mechanism plays a major role in the pathogenesis of Nav channelopathies.
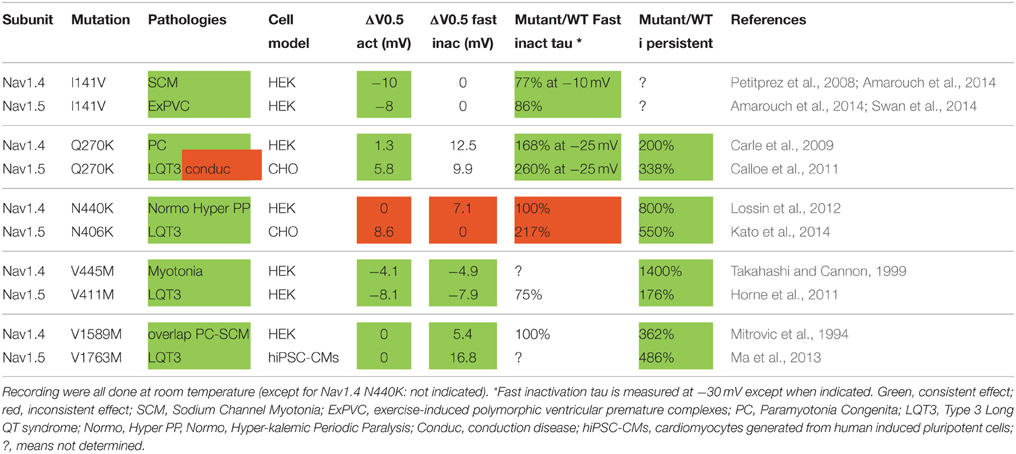
Table 6. Variations of biophysical parameters compared to wild type channels for five equivalent mutations identified in Nav1.4 and Nav1.5 that have been studied extensively in patch clamp.
Recently, an omega current has been observed in Nav1.5 mutant channels identified in patients presenting with arrhythmic DCM or MEPPC (Gosselin-Badaroudine et al., 2012b; Moreau et al., 2015b). This omega current, due to mutations of arginine in the S4 of domain I, is similar to the one observed in Nav1.4 (Sokolov et al., 2007; Struyk et al., 2008; Francis et al., 2011; Gosselin-Badaroudine et al., 2012a; Groome et al., 2014). This further strengthens the functional similarity between Nav1.4 and Nav1.5 in pathophysiological situations. A common feature of MEPPC, is an increase in window current provoked by the Nav1.5 R225W, R222Q, and R225P mutations, increasing cardiac excitability of the fascicular-Purkinje system (Laurent et al., 2012; Mann et al., 2012). Another common feature of two of these mutations: R222Q and R225W is the presence of an omega current. This Nav1.5 omega current may be responsible for the peculiar cardiac phenotype (Moreau et al., 2015a), similar to the omega current of Nav1.4 being responsible for the hypoPP phenotype, through sodium accumulation and a decrease in membrane excitability (Sokolov et al., 2008). Indeed, most of the SCN5A mutations linked to DCM are located in the voltage sensor domain (VSD) as pointed by McNair et al. (2011). However, in some cases DCM may be secondary to arrhythmias and window current increase. For instance, preventing arrhythmias by quinidine improved the ventricular function (ejection fraction) in patients with the Nav1.5 R222Q mutation, via a decrease in the window sodium current (Laurent et al., 2012). The use of specific inhibitor of the alpha pore and the omega (or gating pore) current would allow to test for the respective role of the altered gating (activation, inactivation) and the omega current on the development of the pathology. Noteworthy, the various localization of the Nav1.4 mutations giving rise to omega current (in domains I, II, and III) strongly suggests that similar mutations in Nav1.5 will be identified in domain II and III in addition to the ones already identified in domain I (Moreau et al., 2015b).
To conclude, given the sequence similarity between Nav1.4 and Nav1.5, any characteristics described for one channel subunit may shed light on the properties of the counterpart channel subunit, such as the presence of specific protein partners, or the effects of a specific amino acid substitution. One can argue that the effect of a mutation on Nav1.4 is difficult to compare with Nav1.5 since the different molecular and cellular environment may drastically modify the effect of the mutation. Nevertheless, we noticed that the same mutation lead to comparable effect regarding membrane hypo or hyper-excitability (Table 4 and Figure 2A). This suggests that the cellular environment is usually not able to invert the effect of a mutation from gain to loss of function phenotypes and reciprocally. Such comparison between Nav1.4 and Nav1.5 will probably draw more and more interest, to address the challenge of interpreting and understanding pathogenicity of rare SCN4A or SCN5A variants revealed by next-generation sequencing studies (Arnold et al., 2015; Bergareche et al., 2015; Coll et al., 2015).
Author Contributions
Parts were written by: Part I : DS, YP, VF (Nav1.4), FC (Nav1.5). Part II: SN (Nav1.4), GT (Nav1.5). Part III: FL, CM. Part IV: DS (Nav1.4), JB, OM, IB, GL (Nav1.5). DS and GL initiated the project. IB, FC, JB, YP critically read the entire Manuscript. GL supervised the Ms.
Funding
This work was supported by INSERM, CNRS, the Fondation d'entreprise Génavie, the Fondation pour la Recherche Médicale (PLP20141031304), the Association Française contre les Myopathies - Téléthon (16495), the 7th European Community Framework Programme (PIOF-GA-2011-298280, PIRG06-GA-2009-256397, HEALTH-F2-2009-241526), the ANR (ANR-12-BSV1-0013-01), Investissements d'avenir (ANR-10-IAIHU-06), and Nantes and Sorbonne universities, UPMC-Paris 06.
Conflict of Interest Statement
The authors declare that the research was conducted in the absence of any commercial or financial relationships that could be construed as a potential conflict of interest.
References
Abriel, H. (2010). Cardiac sodium channel Na(v)1.5 and interacting proteins: physiology and pathophysiology. J. Mol. Cell. Cardiol. 48, 2–11. doi: 10.1016/j.yjmcc.2009.08.025
Ackerman, M. J., Priori, S. G., Willems, S., Berul, C., Brugada, R., Calkins, H., et al. (2011). HRS/EHRA expert consensus statement on the state of genetic testing for the channelopathies and cardiomyopathies this document was developed as a partnership between the Heart Rhythm Society (HRS) and the European Heart Rhythm Association (EHRA). Heart Rhythm 8, 1308–1339. doi: 10.1016/j.hrthm.2011.05.020
Ackerman, M. J., Siu, B. L., Sturner, W. Q., Tester, D. J., Valdivia, C. R., Makielski, J. C., et al. (2001). Postmortem molecular analysis of SCN5A defects in sudden infant death syndrome. JAMA 286, 2264–2269. doi: 10.1001/jama.286.18.2264
Aiba, T., Farinelli, F., Kostecki, G., Hesketh, G. G., Edwards, D., Biswas, S., et al. (2014). A mutation causing Brugada syndrome identifies a mechanism for altered autonomic and oxidant regulation of cardiac sodium currents. Circ. Cardiovasc. Genet. 7, 249–256. doi: 10.1161/CIRCGENETICS.113.000480
Allouis, M., Le Bouffant, F., Wilders, R., Péroz, D., Schott, J. J., Noireaud, J., et al. (2006). 14-3-3 is a regulator of the cardiac voltage-gated sodium channel Nav1.5. Circ. Res. 98, 1538–1546. doi: 10.1161/01.RES.0000229244.97497.2c
Amarouch, M. Y., Kasimova, M. A., Tarek, M., and Abriel, H. (2014). Functional interaction between S1 and S4 segments in voltage-gated sodium channels revealed by human channelopathies. Channels (Austin.) 8, 414–420. doi: 10.4161/19336950.2014.958922
Amin, A. S. (2014). SCN5A-related dilated cardiomyopathy: what do we know? Heart Rhythm 11, 1454–1455. doi: 10.1016/j.hrthm.2014.05.031
Amin, A. S., Pinto, Y. M., and Wilde, A. A. (2013). Long QT syndrome: beyond the causal mutation. J. Physiol. 591, 4125–4139. doi: 10.1113/jphysiol.2013.254920
An, R. H., Wang, X. L., Kerem, B., Benhorin, J., Medina, A., Goldmit, M., et al. (1998). Novel LQT-3 mutation affects Na+ channel activity through interactions between alpha- and beta1-subunits. Circ. Res. 83, 141–146. doi: 10.1161/01.RES.83.2.141
Antzelevitch, C., Brugada, P., Borggrefe, M., Brugada, J., Brugada, R., Corrado, D., et al. (2005). Brugada syndrome: report of the second consensus conference. Heart Rhythm 2, 429–440. doi: 10.1016/j.hrthm.2005.01.005
Antzelevitch, C., Pollevick, G. D., Cordeiro, J. M., Casis, O., Sanguinetti, M. C., Aizawa, Y., et al. (2007). Loss-of-function mutations in the cardiac calcium channel underlie a new clinical entity characterized by ST-segment elevation, short QT intervals, and sudden cardiac death. Circulation 115, 442–449. doi: 10.1161/CIRCULATIONAHA.106.668392
Antzelevitch, C., and Yan, G. X. (2015). J-wave syndromes: brugada and early repolarization syndromes. Heart Rhythm 12, 1852–1866. doi: 10.1016/j.hrthm.2015.04.014
Arnold, W. D., Feldman, D. H., Ramirez, S., He, L., Kassar, D., Quick, A., et al. (2015). Defective fast inactivation recovery of Nav 1.4 in congenital myasthenic syndrome. Ann. Neurol. 77, 840–850. doi: 10.1002/ana.24389
Arzel-Hézode, M., McGoey, S., Sternberg, D., Vicart, S., Eymard, B., and Fontaine, B. (2009). Glucocorticoids may trigger attacks in several types of periodic paralysis. Neuromuscul. Disord. 19, 217–219. doi: 10.1016/j.nmd.2008.12.008
Ashpole, N. M., Herren, A. W., Ginsburg, K. S., Brogan, J. D., Johnson, D. E., Cummins, T. R., et al. (2012). Ca2+/calmodulin-dependent protein kinase II (CaMKII) regulates cardiac sodium channel NaV1.5 gating by multiple phosphorylation sites. J. Biol. Chem. 287, 19856–19869. doi: 10.1074/jbc.M111.322537
Awad, S. S., Lightowlers, R. N., Young, C., Chrzanowska-Lightowlers, Z. M., Lomo, T., and Slater, C. R. (2001). Sodium channel mRNAs at the neuromuscular junction: distinct patterns of accumulation and effects of muscle activity. J. Neurosci. 21, 8456–8463.
Bai, C. X., Kurokawa, J., Tamagawa, M., Nakaya, H., and Furukawa, T. (2005). Nontranscriptional regulation of cardiac repolarization currents by testosterone. Circulation 112, 1701–1710. doi: 10.1161/CIRCULATIONAHA.104.523217
Balasuriya, D., Stewart, A. P., Crottès, D., Borgese, F., Soriani, O., and Edwardson, J. M. (2012). The sigma-1 receptor binds to the Nav1.5 voltage-gated Na+ channel with 4-fold symmetry. J. Biol. Chem. 287, 37021–37029. doi: 10.1074/jbc.M112.382077
Beckermann, T. M., McLeod, K., Murday, V., Potet, F., and George, A. L. Jr. (2014). Novel SCN5A mutation in amiodarone-responsive multifocal ventricular ectopy-associated cardiomyopathy. Heart Rhythm 11, 1446–1453. doi: 10.1016/j.hrthm.2014.04.042
Bendahhou, S., Cummins, T. R., Griggs, R. C., Fu, Y. H., and Ptácek, L. J. (2001). Sodium channel inactivation defects are associated with acetazolamide-exacerbated hypokalemic periodic paralysis. Ann. Neurol. 50, 417–420. doi: 10.1002/ana.1144
Bendahhou, S., Cummins, T. R., Kwiecinski, H., Waxman, S. G., and Ptácek, L. J. (1999). Characterization of a new sodium channel mutation at arginine 1448 associated with moderate Paramyotonia congenita in humans. J. Physiol. 518(Pt 2), 337–344. doi: 10.1111/j.1469-7793.1999.0337p.x
Benhorin, J., Goldmit, M., MacCluer, J. W., Blangero, J., Goffen, R., Leibovitch, A., et al. (1998). Identification of a new SCN5A mutation, D1840G, associated with the long QT syndrome. Mutations in brief no. 153. Online. Hum. Mutat. 12, 72.
Bergareche, A., Bednarz, M., Sánchez, E., Krebs, C. E., Ruiz-Martinez, J., De La Riva, P., et al. (2015). SCN4A pore mutation pathogenetically contributes to autosomal dominant essential tremor and may increase susceptibility to epilepsy. Hum. Mol. Genet. 24, 7111–7120. doi: 10.1093/hmg/ddv410
Béziau, D. M., Barc, J., O'Hara, T., Le, G. L., Amarouch, M. Y., Solnon, A., et al. (2014). Complex Brugada syndrome inheritance in a family harbouring compound SCN5A and CACNA1C mutations. Basic Res. Cardiol. 109, 446. doi: 10.1007/s00395-014-0446-5
Bezzina, C., Veldkamp, M. W., van Den Berg, M. P., Postma, A. V., Rook, M. B., Viersma, J. W., et al. (1999). A single Na(+) channel mutation causing both long-QT and Brugada syndromes. Circ. Res. 85, 1206–1213. doi: 10.1161/01.RES.85.12.1206
Bezzina, C. R., Barc, J., Mizusawa, Y., Remme, C. A., Gourraud, J. B., Simonet, F., et al. (2013). Common variants at SCN5A-SCN10A and HEY2 are associated with Brugada syndrome, a rare disease with high risk of sudden cardiac death. Nat. Genet. 45, 1044–1049. doi: 10.1038/ng.2712
Bezzina, C. R., Rook, M. B., Groenewegen, W. A., Herfst, L. J., van der Wal, A. C., Lam, J., et al. (2003). Compound heterozygosity for mutations (W156X and R225W) in SCN5A associated with severe cardiac conduction disturbances and degenerative changes in the conduction system. Circ. Res. 92, 159–168. doi: 10.1161/01.RES.0000052672.97759.36
Bissay, V., Van Malderen, S. C., Keymolen, K., Lissens, W., Peeters, U., Daneels, D., et al. (2015). SCN4A variants and Brugada syndrome: phenotypic and genotypic overlap between cardiac and skeletal muscle sodium channelopathies. Eur. J. Hum. Genet. doi: 10.1038/ejhg.2015.125. [Epub ahead of print].
Blair, E. R., Hoffman, H. E., and Bishop, A. C. (2006). Engineering non-natural inhibitor sensitivity in protein tyrosine phosphatase H1. Bioorg. Med. Chem. 14, 464–471. doi: 10.1016/j.bmc.2005.08.025
Bouhours, M., Sternberg, D., Davoine, C. S., Ferrer, X., Willer, J. C., Fontaine, B., et al. (2004). Functional characterization and cold sensitivity of T1313A, a new mutation of the skeletal muscle sodium channel causing paramyotonia congenita in humans. J. Physiol. 554, 635–647. doi: 10.1113/jphysiol.2003.053082
Brugada, P., and Brugada, J. (1992). Right bundle branch block, persistent ST segment elevation and sudden cardiac death: a distinct clinical and electrocardiographic syndrome. A multicenter report. J. Am. Coll. Cardiol. 20, 1391–1396. doi: 10.1016/0735-1097(92)90253-J
Bulman, D. E., Scoggan, K. A., van Oene, M. D., Nicolle, M. W., Hahn, A. F., Tollar, L. L., et al. (1999). A novel sodium channel mutation in a family with hypokalemic periodic paralysis. Neurology 53, 1932–1936. doi: 10.1212/WNL.53.9.1932
Burashnikov, E., Pfeiffer, R., Barajas-Martinez, H., Delpón, E., Hu, D., Desai, M., et al. (2010). Mutations in the cardiac L-type calcium channel associated with inherited J-wave syndromes and sudden cardiac death. Heart Rhythm 7, 1872–1882. doi: 10.1016/j.hrthm.2010.08.026
Calloe, K., Schmitt, N., Grubb, S., Pfeiffer, R., David, J. P., Kanter, R., et al. (2011). Multiple arrhythmic syndromes in a newborn, owing to a novel mutation in SCN5A. Can. J. Physiol. Pharmacol. 89, 723–736. doi: 10.1139/y11-070
Cannon, S. C. (2015). Channelopathies of skeletal muscle excitability. Compr. Physiol. 5, 761–790. doi: 10.1002/cphy.c140062
Cannon, S. C., and Strittmatter, S. M. (1993). Functional expression of sodium channel mutations identified in families with periodic paralysis. Neuron 10, 317–326. doi: 10.1016/0896-6273(93)90321-H
Carle, T., Fournier, E., Sternberg, D., Fontaine, B., and Tabti, N. (2009). Cold-induced disruption of Na+ channel slow inactivation underlies paralysis in highly thermosensitive paramyotonia. J. Physiol. 587, 1705–1714. doi: 10.1113/jphysiol.2008.165787
Carle, T., Lhuillier, L., Luce, S., Sternberg, D., Devuyst, O., Fontaine, B., et al. (2006). Gating defects of a novel Na+ channel mutant causing hypokalemic periodic paralysis. Biochem. Biophys. Res. Commun. 348, 653–661. doi: 10.1016/j.bbrc.2006.07.101
Chahine, M., George, A. L. Jr., Zhou, M., Ji, S., Sun, W., Barchi, R. L., et al. (1994). Sodium channel mutations in paramyotonia congenita uncouple inactivation from activation. Neuron 12, 281–294. doi: 10.1016/0896-6273(94)90271-2
Chang, C. C., Acharfi, S., Wu, M. H., Chiang, F. T., Wang, J. K., Sung, T. C., et al. (2004). A novel SCN5A mutation manifests as a malignant form of long QT syndrome with perinatal onset of tachycardia/bradycardia. Cardiovasc. Res. 64, 268–278. doi: 10.1016/j.cardiores.2004.07.007
Clarke, C., Howard, R., Rossor, M., and Shorvon, S. D. (2011). Neurology: A Queen Square Textbook. Hoboken, NJ: Wiley Blackwell Publishing Ltd.
Chatin, B., Colombier, P., Gamblin, A. L., Allouis, M., and Le Bouffant, F. (2014). Dynamitin affects cell-surface expression of voltage-gated sodium channel Nav1.5. Biochem. J. 463, 339–349. doi: 10.1042/BJ20140604
Coll, M., Allegue, C., Partemi, S., Mates, J., Del, O. B., Campuzano, O., et al. (2015). Genetic investigation of sudden unexpected death in epilepsy cohort by panel target resequencing. Int. J. Legal Med. doi: 10.1007/s00414-015-1269-0. [Epub ahead of print].
Crotti, L., Marcou, C. A., Tester, D. J., Castelletti, S., Giudicessi, J. R., Torchio, M., et al. (2012). Spectrum and prevalence of mutations involving BrS1- through BrS12-susceptibility genes in a cohort of unrelated patients referred for Brugada syndrome genetic testing: implications for genetic testing. J. Am. Coll. Cardiol. 60, 1410–1418. doi: 10.1016/j.jacc.2012.04.037
Darbar, D., Kannankeril, P. J., Donahue, B. S., Kucera, G., Stubblefield, T., Haines, J. L., et al. (2008). Cardiac sodium channel (SCN5A) variants associated with atrial fibrillation. Circulation 117, 1927–1935. doi: 10.1161/CIRCULATIONAHA.107.757955
Delpón, E., Cordeiro, J. M., Nunez, L., Thomsen, P. E., Guerchicoff, A., Pollevick, G. D., et al. (2008). Functional effects of KCNE3 mutation and its role in the development of Brugada syndrome. Circ. Arrhythm. Electrophysiol. 1, 209–218. doi: 10.1161/CIRCEP.107.748103
Deschênes, I., Baroudi, G., Berthet, M., Barde, I., Chalvidan, T., Denjoy, I., et al. (2000). Electrophysiological characterization of SCN5A mutations causing long QT (E1784K) and Brugada (R1512W and R1432G) syndromes. Cardiovasc. Res. 46, 55–65. doi: 10.1016/S0008-6363(00)00006-7
Durran, S. C., Matthews, E., Raja Rayan, D. L., Sud, R., Polke, J., Haworth, A., et al. (2011). “Genetic heterogeneity and mechanisms of phenotypic variability in human skeletal muscle channelopathies,” in UK Neuromuscular Translational Research Conference 2011 (London).
Featherstone, D. E., Fujimoto, E., and Ruben, P. C. (1998). A defect in skeletal muscle sodium channel deactivation exacerbates hyperexcitability in human paramyotonia congenita. J. Physiol. 506(Pt 3), 627–638. doi: 10.1111/j.1469-7793.1998.627bv.x
Fleischhauer, R., Mitrovic, N., Deymeer, F., Lehmann-Horn, F., and Lerche, H. (1998). Effects of temperature and mexiletine on the F1473S Na+ channel mutation causing paramyotonia congenita. Pflugers Arch. 436, 757–765. doi: 10.1007/s004240050699
Foley, K. S., and Young, P. W. (2014). The non-muscle functions of actinins: an update. Biochem. J. 459, 1–13. doi: 10.1042/BJ20131511
Fournier, E., Arzel, M., Sternberg, D., Vicart, S., Laforet, P., Eymard, B., et al. (2004). Electromyography guides toward subgroups of mutations in muscle channelopathies. Ann. Neurol. 56, 650–661. doi: 10.1002/ana.20241
Fournier, E., Viala, K., Gervais, H., Sternberg, D., Arzel-Hézode, M., Laforet, P., et al. (2006). Cold extends electromyography distinction between ion channel mutations causing myotonia. Ann. Neurol. 60, 356–365. doi: 10.1002/ana.20905
Francis, D. G., Rybalchenko, V., Struyk, A., and Cannon, S. C. (2011). Leaky sodium channels from voltage sensor mutations in periodic paralysis, but not paramyotonia. Neurology 76, 1635–1641. doi: 10.1212/WNL.0b013e318219fb57
Frigo, G., Rampazzo, A., Bauce, B., Pilichou, K., Beffagna, G., Danieli, G. A., et al. (2007). Homozygous SCN5A mutation in Brugada syndrome with monomorphic ventricular tachycardia and structural heart abnormalities. Europace 9, 391–397. doi: 10.1093/europace/eum053
Frustaci, A., Priori, S. G., Pieroni, M., Chimenti, C., Napolitano, C., Rivolta, I., et al. (2005). Cardiac histological substrate in patients with clinical phenotype of Brugada syndrome. Circulation 112, 3680–3687. doi: 10.1161/CIRCULATIONAHA.105.520999
Fukudome, T., Izumoto, H., Goto, H., Matsuo, H., Yoshimura, T., Sakoda, S., et al. (2003). Paramyotonia congenita due to a de novo mutation: a case report. Muscle Nerve 28, 232–235. doi: 10.1002/mus.10396
Gaborit, N., Varro, A., Le, B. S., Szuts, V., Escande, D., Nattel, S., et al. (2010). Gender-related differences in ion-channel and transporter subunit expression in non-diseased human hearts. J. Mol. Cell Cardiol. 49, 639–646. doi: 10.1016/j.yjmcc.2010.06.005
Gao, G., and Dudley, S. C. Jr. (2013). RBM25/LUC7L3 function in cardiac sodium channel splicing regulation of human heart failure. Trends Cardiovasc. Med. 23, 5–8. doi: 10.1016/j.tcm.2012.08.003
Gao, G., Xie, A., Huang, S. C., Zhou, A., Zhang, J., Herman, A. M., et al. (2011). Role of RBM25/LUC7L3 in abnormal cardiac sodium channel splicing regulation in human heart failure. Circulation 124, 1124–1131. doi: 10.1161/CIRCULATIONAHA.111.044495
Gay, S., Dupuis, D., Faivre, L., Masurel-Paulet, A., Labenne, M., Colombani, M., et al. (2008). Severe neonatal non-dystrophic myotonia secondary to a novel mutation of the voltage-gated sodium channel (SCN4A) gene. Am. J. Med. Genet. A 146A, 380–383. doi: 10.1002/ajmg.a.32141
Ge, J., Sun, A., Paajanen, V., Wang, S., Su, C., Yang, Z., et al. (2008). Molecular and clinical characterization of a novel SCN5A mutation associated with atrioventricular block and dilated cardiomyopathy. Circ. Arrhythm. Electrophysiol. 1, 83–92. doi: 10.1161/CIRCEP.107.750752
Gee, S. H., Madhavan, R., Levinson, S. R., Caldwell, J. H., Sealock, R., and Froehner, S. C. (1998). Interaction of muscle and brain sodium channels with multiple members of the syntrophin family of dystrophin-associated proteins. J. Neurosci. 18, 128–137.
Gellens, M. E., George, A. L. Jr., Chen, L. Q., Chahine, M., Horn, R., Barchi, R. L., et al. (1992). Primary structure and functional expression of the human cardiac tetrodotoxin-insensitive voltage-dependent sodium channel. Proc. Natl. Acad. Sci. U.S.A. 89, 554–558. doi: 10.1073/pnas.89.2.554
George, A. L. Jr. (2005). Inherited disorders of voltage-gated sodium channels. J. Clin. Invest. 115, 1990–1999. doi: 10.1172/JCI25505
George, A. L. Jr., Knittle, T. J., and Tamkun, M. M. (1992a). Molecular cloning of an atypical voltage-gated sodium channel expressed in human heart and uterus: evidence for a distinct gene family. Proc. Natl. Acad. Sci. U.S.A. 89, 4893–4897.
George, A. L. Jr., Komisarof, J., Kallen, R. G., and Barchi, R. L. (1992b). Primary structure of the adult human skeletal muscle voltage-dependent sodium channel. Ann. Neurol. 31, 131–137.
Giudicessi, J. R., and Ackerman, M. J. (2013). Genotype- and phenotype-guided management of congenital long QT syndrome. Curr. Probl. Cardiol. 38, 417–455. doi: 10.1016/j.cpcardiol.2013.08.001
Giudicessi, J. R., Ye, D., Tester, D. J., Crotti, L., Mugione, A., Nesterenko, V. V., et al. (2011). Transient outward current (I(to)) gain-of-function mutations in the KCND3-encoded Kv4.3 potassium channel and Brugada syndrome. Heart Rhythm 8, 1024–1032. doi: 10.1016/j.hrthm.2011.02.021
Gosselin-Badaroudine, P., Delemotte, L., Moreau, A., Klein, M. L., and Chahine, M. (2012a). Gating pore currents and the resting state of Nav1.4 voltage sensor domains. Proc. Natl. Acad. Sci. U.S.A. 109, 19250–19255. doi: 10.1073/pnas.1217990109
Gosselin-Badaroudine, P., Keller, D. I., Huang, H., Pouliot, V., Chatelier, A., Osswald, S., et al. (2012b). A proton leak current through the cardiac sodium channel is linked to mixed arrhythmia and the dilated cardiomyopathy phenotype. PLoS ONE. 7:e38331. doi: 10.1371/journal.pone.0038331
Green, D. S., George, A. L. Jr., and Cannon, S. C. (1998). Human sodium channel gating defects caused by missense mutations in S6 segments associated with myotonia: S804F and V1293I. J. Physiol. 510(Pt 3), 685–694. doi: 10.1111/j.1469-7793.1998.685bj.x
Groome, J. R., Jurkat-Rott, K., and Lehmann-Horn, F. (2014). Domain III S4 in closed-state fast inactivation: insights from a periodic paralysis mutation. Channels (Austin.) 8, 467–471. doi: 10.4161/19336950.2014.958924
Hayward, L. J., Brown, R. H. Jr., and Cannon, S. C. (1996). Inactivation defects caused by myotonia-associated mutations in the sodium channel III-IV linker. J. Gen. Physiol. 107, 559–576. doi: 10.1085/jgp.107.5.559
Hayward, L. J., Sandoval, G. M., and Cannon, S. C. (1999). Defective slow inactivation of sodium channels contributes to familial periodic paralysis. Neurology 52, 1447–1453. doi: 10.1212/WNL.52.7.1447
Hebert, S. L., Simmons, C., Thompson, A. L., Zorc, C. S., Blalock, E. M., and Kraner, S. D. (2007). Basic helix-loop-helix factors recruit nuclear factor I to enhance expression of the NaV 1.4 Na+ channel gene. Biochim. Biophys. Acta 1769, 649–658. doi: 10.1016/j.bbaexp.2007.08.004
Heine, R., Pika, U., and Lehmann-Horn, F. (1993). A novel SCN4A mutation causing myotonia aggravated by cold and potassium. Hum. Mol. Genet. 2, 1349–1353. doi: 10.1093/hmg/2.9.1349
Hershberger, R. E., Hedges, D. J., and Morales, A. (2013). Dilated cardiomyopathy: the complexity of a diverse genetic architecture. Nat. Rev. Cardiol. 10, 531–547. doi: 10.1038/nrcardio.2013.105
Holzherr, B., Lehmann-Horn, F., Kuzmenkina, E., Fan, C., and Jurkat-Rott, K. (2014). A gating model for wildtype and R1448H Nav1.4 channels in paramyotonia. Acta Myol. 33, 22–33.
Horga, A., Raja Rayan, D. L., Matthews, E., Sud, R., Fialho, D., Durran, S. C., et al. (2013). Prevalence study of genetically defined skeletal muscle channelopathies in England. Neurology 80, 1472–1475. doi: 10.1212/WNL.0b013e31828cf8d0
Horne, A. J., Eldstrom, J., Sanatani, S., and Fedida, D. (2011). A novel mechanism for LQT3 with 2:1 block: a pore-lining mutation in Nav1.5 significantly affects voltage-dependence of activation. Heart Rhythm 8, 770–777. doi: 10.1016/j.hrthm.2010.12.041
Iaizzo, P. A., Quasthoff, S., and Lehmann-Horn, F. (1995). Differential diagnosis of periodic paralysis aided by in vitro myography. Neuromuscul. Disord. 5, 115–124. doi: 10.1016/0960-8966(94)00036-9
Itoh, H., Shimizu, M., Mabuchi, H., and Imoto, K. (2005a). Clinical and electrophysiological characteristics of Brugada syndrome caused by a missense mutation in the S5-pore site of SCN5A. J. Cardiovasc. Electrophysiol. 16, 378–383. doi: 10.1046/j.1540-8167.2005.40606.x
Itoh, H., Shimizu, M., Takata, S., Mabuchi, H., and Imoto, K. (2005b). A novel missense mutation in the SCN5A gene associated with Brugada syndrome bidirectionally affecting blocking actions of antiarrhythmic drugs. J. Cardiovasc. Electrophysiol. 16, 486–493. doi: 10.1111/j.1540-8167.2005.40711.x
Jespersen, T., Gavillet, B., van Bemmelen, M. X., Cordonier, S., Thomas, M. A., Staub, O., et al. (2006). Cardiac sodium channel Na(v)1.5 interacts with and is regulated by the protein tyrosine phosphatase PTPH1. Biochem. Biophys. Res. Commun. 348, 1455–1462. doi: 10.1016/j.bbrc.2006.08.014
Jurkat-Rott, K., Holzherr, B., Fauler, M., and Lehmann-Horn, F. (2010). Sodium channelopathies of skeletal muscle result from gain or loss of function. Pflugers Arch. 460, 239–248. doi: 10.1007/s00424-010-0814-4
Jurkat-Rott, K., Mitrovic, N., Hang, C., Kouzmekine, A., Iaizzo, P., Herzog, J., et al. (2000). Voltage-sensor sodium channel mutations cause hypokalemic periodic paralysis type 2 by enhanced inactivation and reduced current. Proc. Natl. Acad. Sci. U.S.A. 97, 9549–9554. doi: 10.1073/pnas.97.17.9549
Kallen, R. G., Sheng, Z. H., Yang, J., Chen, L. Q., Rogart, R. B., and Barchi, R. L. (1990). Primary structure and expression of a sodium channel characteristic of denervated and immature rat skeletal muscle. Neuron 4, 233–242. doi: 10.1016/0896-6273(90)90098-Z
Kambouris, N. G., Nuss, H. B., Johns, D. C., Tomaselli, G. F., Marban, E., and Balser, J. R. (1998). Phenotypic characterization of a novel long-QT syndrome mutation (R1623Q) in the cardiac sodium channel. Circulation 97, 640–644. doi: 10.1161/01.CIR.97.7.640
Kapplinger, J. D., Tester, D. J., Alders, M., Benito, B., Berthet, M., Brugada, J., et al. (2010). An international compendium of mutations in the SCN5A-encoded cardiac sodium channel in patients referred for Brugada syndrome genetic testing. Heart Rhythm 7, 33–46. doi: 10.1016/j.hrthm.2009.09.069
Kapplinger, J. D., Tester, D. J., Salisbury, B. A., Carr, J. L., Harris-Kerr, C., Pollevick, G. D., et al. (2009). Spectrum and prevalence of mutations from the first 2,500 consecutive unrelated patients referred for the FAMILION long QT syndrome genetic test. Heart Rhythm 6, 1297–1303. doi: 10.1016/j.hrthm.2009.05.021
Kato, K., Makiyama, T., Wu, J., Ding, W. G., Kimura, H., Naiki, N., et al. (2014). Cardiac channelopathies associated with infantile fatal ventricular arrhythmias: from the cradle to the bench. J. Cardiovasc. Electrophysiol. 25, 66–73. doi: 10.1111/jce.12270
Ke, Q., Luo, B., Qi, M., Du, Y., and Wu, W. (2013). Gender differences in penetrance and phenotype in hypokalemic periodic paralysis. Muscle Nerve 47, 41–45. doi: 10.1002/mus.23460
Keller, D. I., Acharfi, S., Delacrétaz, E., Benammar, N., Rotter, M., Pfammatter, J. P., et al. (2003). A novel mutation in SCN5A, delQKP 1507-1509, causing long QT syndrome: role of Q1507 residue in sodium channel inactivation. J. Mol. Cell. Cardiol. 35, 1513–1521. doi: 10.1016/j.yjmcc.2003.08.007
Keller, D. I., Rougier, J. S., Kucera, J. P., Benammar, N., Fressart, V., Guicheney, P., et al. (2005). Brugada syndrome and fever: genetic and molecular characterization of patients carrying SCN5A mutations. Cardiovasc. Res. 67, 510–519. doi: 10.1016/j.cardiores.2005.03.024
Koch, M. C., Baumbach, K., George, A. L., and Ricker, K. (1995). Paramyotonia congenita without paralysis on exposure to cold: a novel mutation in the SCN4A gene (Val1293Ile). Neuroreport 6, 2001–2004. doi: 10.1097/00001756-199510010-00012
Kraner, S. D., Rich, M. M., Kallen, R. G., and Barchi, R. L. (1998). Two E-boxes are the focal point of muscle-specific skeletal muscle type 1 Na+ channel gene expression. J. Biol. Chem. 273, 11327–11334. doi: 10.1074/jbc.273.18.11327
Kraner, S. D., Rich, M. M., Sholl, M. A., Zhou, H., Zorc, C. S., Kallen, R. G., et al. (1999). Interaction between the skeletal muscle type 1 Na+ channel promoter E-box and an upstream repressor element. Release of repression by myogenin. J. Biol. Chem. 274, 8129–8136. doi: 10.1074/jbc.274.12.8129
Kuzmenkin, A., Muncan, V., Jurkat-Rott, K., Hang, C., Lerche, H., Lehmann-Horn, F., et al. (2002). Enhanced inactivation and pH sensitivity of Na(+) channel mutations causing hypokalaemic periodic paralysis type II. Brain 125, 835–843. doi: 10.1093/brain/awf071
Kyndt, F., Probst, V., Potet, F., Demolombe, S., Chevallier, J. C., Baro, I., et al. (2001). Novel SCN5A mutation leading either to isolated cardiac conduction defect or Brugada syndrome in a large French family. Circulation 104, 3081–3086. doi: 10.1161/hc5001.100834
Laurent, G., Saal, S., Amarouch, M. Y., Béziau, D. M., Marsman, R. F., Faivre, L., et al. (2012). Multifocal ectopic Purkinje-related premature contractions: a new SCN5A-related cardiac channelopathy. J. Am. Coll. Cardiol. 60, 144–156. doi: 10.1016/j.jacc.2012.02.052
Lee, S. C., Kim, H. S., Park, Y. E., Choi, Y. C., Park, K. H., and Kim, D. S. (2009). Clinical diversity of SCN4A-mutation-associated skeletal muscle sodium channelopathy. J. Clin. Neurol. 5, 186–191. doi: 10.3988/jcn.2009.5.4.186
Lehmann-Horn, F., Orth, M., Kuhn, M., and Jurkat-Rott, K. (2011). A novel N440K sodium channel mutation causes myotonia with exercise-induced weakness–exclusion of CLCN1 exon deletion/duplication by MLPA. Acta Myol. 30, 133–137.
Lemaillet, G., Walker, B., and Lambert, S. (2003). Identification of a conserved ankyrin-binding motif in the family of sodium channel alpha subunits. J. Biol. Chem. 278, 27333–27339. doi: 10.1074/jbc.M303327200
Lerche, H., Heine, R., Pika, U., George, A. L. Jr., Mitrovic, N., Browatzki, M., et al. (1993). Human sodium channel myotonia: slowed channel inactivation due to substitutions for a glycine within the III-IV linker. J. Physiol. 470, 13–22. doi: 10.1113/jphysiol.1993.sp019843
Lion-Francois, L., Mignot, C., Vicart, S., Manel, V., Sternberg, D., Landrieu, P., et al. (2010). Severe neonatal episodic laryngospasm due to de novo SCN4A mutations: a new treatable disorder. Neurology 75, 641–645. doi: 10.1212/WNL.0b013e3181ed9e96
Liu, C. J., Dib-Hajj, S. D., Renganathan, M., Cummins, T. R., and Waxman, S. G. (2003). Modulation of the cardiac sodium channel Nav1.5 by fibroblast growth factor homologous factor 1B. J. Biol. Chem. 278, 1029–1036. doi: 10.1074/jbc.M207074200
Lossin, C., Nam, T. S., Shahangian, S., Rogawski, M. A., Choi, S. Y., Kim, M. K., et al. (2012). Altered fast and slow inactivation of the N440K Nav1.4 mutant in a periodic paralysis syndrome. Neurology 79, 1033–1040. doi: 10.1212/WNL.0b013e3182684683
Ma, D., Wei, H., Zhao, Y., Lu, J., Li, G., Sahib, N. B., et al. (2013). Modeling type 3 long QT syndrome with cardiomyocytes derived from patient-specific induced pluripotent stem cells. Int. J. Cardiol. 168, 5277–5286. doi: 10.1016/j.ijcard.2013.08.015
Makielski, J. C., Ye, B., Valdivia, C. R., Pagel, M. D., Pu, J., Tester, D. J., et al. (2003). A ubiquitous splice variant and a common polymorphism affect heterologous expression of recombinant human SCN5A heart sodium channels. Circ. Res. 93, 821–828. doi: 10.1161/01.RES.0000096652.14509.96
Makita, N., Behr, E., Shimizu, W., Horie, M., Sunami, A., Crotti, L., et al. (2008). The E1784K mutation in SCN5A is associated with mixed clinical phenotype of type 3 long QT syndrome. J. Clin. Invest. 118, 2219–2229. doi: 10.1172/jci34057
Makita, N., Bennett, P. B., and George, A. L. Jr. (1996). Molecular determinants of beta 1 subunit-induced gating modulation in voltage-dependent Na+ channels. J. Neurosci. 16, 7117–7127.
Makita, N., Horie, M., Nakamura, T., Ai, T., Sasaki, K., Yokoi, H., et al. (2002). Drug-induced long-QT syndrome associated with a subclinical SCN5A mutation. Circulation 106, 1269–1274. doi: 10.1161/01.CIR.0000027139.42087.B6
Makita, N., Shirai, N., Nagashima, M., Matsuoka, R., Yamada, Y., Tohse, N., et al. (1998). A de novo missense mutation of human cardiac Na+ channel exhibiting novel molecular mechanisms of long QT syndrome. FEBS Lett. 423, 5–9. doi: 10.1016/S0014-5793(98)00033-7
Makiyama, T., Akao, M., Tsuji, K., Doi, T., Ohno, S., Takenaka, K., et al. (2005). High risk for bradyarrhythmic complications in patients with Brugada syndrome caused by SCN5A gene mutations. J. Am. Coll. Cardiol. 46, 2100–2106. doi: 10.1016/j.jacc.2005.08.043
Mann, S. A., Castro, M. L., Ohanian, M., Guo, G., Zodgekar, P., Sheu, A., et al. (2012). R222Q SCN5A mutation is associated with reversible ventricular ectopy and dilated cardiomyopathy. J. Am. Coll. Cardiol. 60, 1566–1573. doi: 10.1016/j.jacc.2012.05.050
Marfatia, K. A., Harreman, M. T., Fanara, P., Vertino, P. M., and Corbett, A. H. (2001). Identification and characterization of the human MOG1 gene. Gene 266, 45–56. doi: 10.1016/S0378-1119(01)00364-X
Matthews, E., Labrum, R., Sweeney, M. G., Sud, R., Haworth, A., Chinnery, P. F., et al. (2009). Voltage sensor charge loss accounts for most cases of hypokalemic periodic paralysis. Neurology 72, 1544–1547. doi: 10.1212/01.wnl.0000342387.65477.46
McClatchey, A. I., McKenna-Yasek, D., Cros, D., Worthen, H. G., Kuncl, R. W., DeSilva, S. M., et al. (1992a). Novel mutations in families with unusual and variable disorders of the skeletal muscle sodium channel. Nat. Genet. 2, 148–152.
McClatchey, A. I., Van den Bergh, P., Pericak-Vance, M. A., Raskind, W., Verellen, C., McKenna-Yasek, D., et al. (1992b). Temperature-sensitive mutations in the III-IV cytoplasmic loop region of the skeletal muscle sodium channel gene in paramyotonia congenita. Cell 68, 769–774.
McNair, W. P., Sinagra, G., Taylor, M. R., Di, L. A., Ferguson, D. A., Salcedo, E. E., et al. (2011). SCN5A mutations associate with arrhythmic dilated cardiomyopathy and commonly localize to the voltage-sensing mechanism. J. Am. Coll. Cardiol. 57, 2160–2168. doi: 10.1016/j.jacc.2010.09.084
Méjat, A., Ravel-Chapuis, A., Vandromme, M., and Schaeffer, L. (2003). Synapse-specific gene expression at the neuromuscular junction. Ann. N.Y. Acad. Sci. 998, 53–65. doi: 10.1196/annals.1254.008
Mitrovic, N., George, A. L. Jr., Heine, R., Wagner, S., Pika, U., Hartlaub, U., et al. (1994). K(+)-aggravated myotonia: destabilization of the inactivated state of the human muscle Na+ channel by the V1589M mutation. J. Physiol. 478(Pt 3), 395–402. doi: 10.1113/jphysiol.1994.sp020260
Mitrovic, N., George, A. L. Jr., Lerche, H., Wagner, S., Fahlke, C., and Lehmann-Horn, F. (1995). Different effects on gating of three myotonia-causing mutations in the inactivation gate of the human muscle sodium channel. J. Physiol. 487(Pt 1), 107–114.
Mohammadi, B., Mitrovic, N., Lehmann-Horn, F., Dengler, R., and Bufler, J. (2003). Mechanisms of cold sensitivity of paramyotonia congenita mutation R1448H and overlap syndrome mutation M1360V. J. Physiol. 547, 691–698. doi: 10.1113/jphysiol.2002.033928
Mohler, P. J., Rivolta, I., Napolitano, C., LeMaillet, G., Lambert, S., Priori, S. G., et al. (2004). Nav1.5 E1053K mutation causing Brugada syndrome blocks binding to ankyrin-G and expression of Nav1.5 on the surface of cardiomyocytes. Proc. Natl. Acad. Sci. U.S.A. 101, 17533–17538. doi: 10.1073/pnas.0403711101
Moreau, A., Gosselin-Badaroudine, P., and Chahine, M. (2015a). Gating pore currents, a new pathological mechanism underlying cardiac arrhythmias associated with dilated cardiomyopathy. Channels (Austin.) 9, 139–144. doi: 10.1080/19336950.2015.1031937
Moreau, A., Gosselin-Badaroudine, P., Delemotte, L., Klein, M. L., and Chahine, M. (2015b). Gating pore currents are defects in common with two Nav1.5 mutations in patients with mixed arrhythmias and dilated cardiomyopathy. J. Gen. Physiol. 145, 93–106. doi: 10.1085/jgp.201411304
Moss, A. J. (2002). T-wave patterns associated with the hereditary long QT syndrome. Card. Electrophysiol. Rev. 6, 311–315. doi: 10.1023/A:1016301730302
Nair, K., Pekhletski, R., Harris, L., Care, M., Morel, C., Farid, T., et al. (2012). Escape capture bigeminy: phenotypic marker of cardiac sodium channel voltage sensor mutation R222Q. Heart Rhythm 9, 1681–1688. doi: 10.1016/j.hrthm.2012.06.029
Napolitano, C., Priori, S. G., Schwartz, P. J., Bloise, R., Ronchetti, E., Nastoli, J., et al. (2005). Genetic testing in the long QT syndrome: development and validation of an efficient approach to genotyping in clinical practice. JAMA 294, 2975–2980. doi: 10.1001/jama.294.23.2975
Nicole, S., and Fontaine, B. (2015). Skeletal muscle sodium channelopathies. Curr. Opin. Neurol. 28, 508–514. doi: 10.1097/wco.0000000000000238
Nielsen, M. W., Holst, A. G., Olesen, S. P., and Olesen, M. S. (2013). The genetic component of Brugada syndrome. Front. Physiol. 4:179. doi: 10.3389/fphys.2013.00179
Niimura, H., Matsunaga, A., Kumagai, K., Ohwaki, K., Ogawa, M., Noguchi, H., et al. (2004). Genetic analysis of Brugada syndrome in Western Japan: two novel mutations. Circ. J. 68, 740–746. doi: 10.1253/circj.68.740
Okuda, S., Kanda, F., Nishimoto, K., Sasaki, R., and Chihara, K. (2001). Hyperkalemic periodic paralysis and paramyotonia congenita—a novel sodium channel mutation. J. Neurol. 248, 1003–1004. doi: 10.1007/s004150170059
Ou, Y., Strege, P., Miller, S. M., Makielski, J., Ackerman, M., Gibbons, S. J., et al. (2003). Syntrophin gamma 2 regulates SCN5A gating by a PDZ domain-mediated interaction. J. Biol. Chem. 278, 1915–1923. doi: 10.1074/jbc.M209938200
Péréon, Y., Lande, G., Demolombe, S., Nguyen The Tich, S., Sternberg, D., Le, M. H., et al. (2003). Paramyotonia congenita with an SCN4A mutation affecting cardiac repolarization. Neurology 60, 340–342. doi: 10.1212/01.WNL.0000042093.96309.5A
Petitprez, S., Tiab, L., Chen, L., Kappeler, L., Rösler, K. M., Schorderet, D., et al. (2008). A novel dominant mutation of the Nav1.4 alpha-subunit domain I leading to sodium channel myotonia. Neurology 71, 1669–1675. doi: 10.1212/01.wnl.0000335168.86248.55
Petitprez, S., Zmoos, A. F., Ogrodnik, J., Balse, E., Raad, N., El-Haou, S., et al. (2011). SAP97 and dystrophin macromolecular complexes determine two pools of cardiac sodium channels Nav1.5 in cardiomyocytes. Circ. Res. 108, 294–304. doi: 10.1161/CIRCRESAHA.110.228312
Pfahnl, A. E., Viswanathan, P. C., Weiss, R., Shang, L. L., Sanyal, S., Shusterman, V., et al. (2007). A sodium channel pore mutation causing Brugada syndrome. Heart Rhythm 4, 46–53. doi: 10.1016/j.hrthm.2006.09.031
Plassart, E., Reboul, J., Rime, C. S., Recan, D., Millasseau, P., Eymard, B., et al. (1994). Mutations in the muscle sodium channel gene (SCN4A) in 13 French families with hyperkalemic periodic paralysis and paramyotonia congenita: phenotype to genotype correlations and demonstration of the predominance of two mutations. Eur. J. Hum. Genet. 2, 110–124.
Potet, F., Chagot, B., Anghelescu, M., Viswanathan, P. C., Stepanovic, S. Z., Kupershmidt, S., et al. (2009). Functional Interactions between distinct sodium channel cytoplasmic domains through the action of calmodulin. J. Biol. Chem. 284, 8846–8854. doi: 10.1074/jbc.M806871200
Priori, S. G., Napolitano, C., and Schwartz, P. J. (1999). Low penetrance in the long-QT syndrome: clinical impact. Circulation 99, 529–533. doi: 10.1161/01.CIR.99.4.529
Priori, S. G., Napolitano, C., Gasparini, M., Pappone, C., Della, B. P., Giordano, U., et al. (2002). Natural history of Brugada syndrome: insights for risk stratification and management. Circulation 105, 1342–1347. doi: 10.1161/hc1102.105288
Priori, S. G., Schwartz, P. J., Napolitano, C., Bloise, R., Ronchetti, E., Grillo, M., et al. (2003). Risk stratification in the long-QT syndrome. N. Engl. J. Med. 348, 1866–1874. doi: 10.1056/NEJMoa022147
Probst, V., Wilde, A. A., Barc, J., Sacher, F., Babuty, D., Mabo, P., et al. (2009). SCN5A mutations and the role of genetic background in the pathophysiology of Brugada syndrome. Circ. Cardiovasc. Genet. 2, 552–557. doi: 10.1161/CIRCGENETICS.109.853374
Ptácek, L. J., George, A. L. Jr., Barchi, R. L., Griggs, R. C., Riggs, J. E., Robertson, M., et al. (1992). Mutations in an S4 segment of the adult skeletal muscle sodium channel cause paramyotonia congenita. Neuron 8, 891–897. doi: 10.1016/0896-6273(92)90203-P
Ptácek, L. J., George, A. L. Jr., Griggs, R. C., Tawil, R., Kallen, R. G., Barchi, R. L., et al. (1991). Identification of a mutation in the gene causing hyperkalemic periodic paralysis. Cell 67, 1021–1027. doi: 10.1016/0092-8674(91)90374-8
Raja Rayan, D. L., and Hanna, M. G. (2010). Skeletal muscle channelopathies: nondystrophic myotonias and periodic paralysis. Curr. Opin. Neurol. 23, 466–476. doi: 10.1097/WCO.0b013e32833cc97e
Remme, C. A., Wilde, A. A., and Bezzina, C. R. (2008). Cardiac sodium channel overlap syndromes: different faces of SCN5A mutations. Trends Cardiovasc. Med. 18, 78–87. doi: 10.1016/j.tcm.2008.01.002
Ricker, K., Moxley, R. T. III, Heine, R., and Lehmann-Horn, F. (1994). Myotonia fluctuans. A third type of muscle sodium channel disease. Arch Neurol. 51, 1095–1102. doi: 10.1001/archneur.1994.00540230033009
Rivolta, I., Abriel, H., Tateyama, M., Liu, H., Memmi, M., Vardas, P., et al. (2001). Inherited Brugada and long QT-3 syndrome mutations of a single residue of the cardiac sodium channel confer distinct channel and clinical phenotypes. J. Biol. Chem. 276, 30623–30630. doi: 10.1074/jbc.M104471200
Rojas, C. V., Neely, A., Velasco-Loyden, G., Palma, V., and Kukuljan, M. (1999). Hyperkalemic periodic paralysis M1592V mutation modifies activation in human skeletal muscle Na+ channel. Am. J. Physiol. 276, C259–C266.
Rojas, C. V., Wang, J. Z., Schwartz, L. S., Hoffman, E. P., Powell, B. R., and Brown, R. H. Jr. (1991). A Met-to-Val mutation in the skeletal muscle Na+ channel alpha-subunit in hyperkalaemic periodic paralysis. Nature 354, 387–389. doi: 10.1038/354387a0
Rook, M. B., Evers, M. M., Vos, M. A., and Bierhuizen, M. F. (2012). Biology of cardiac sodium channel Nav1.5 expression. Cardiovasc. Res. 93, 12–23. doi: 10.1093/cvr/cvr252
Rosenfeld, J., Sloan-Brown, K., and George, A. L. Jr. (1997). A novel muscle sodium channel mutation causes painful congenital myotonia. Ann. Neurol. 42, 811–814. doi: 10.1002/ana.410420520
Rossenbacker, T., Carroll, S. J., Liu, H., Kuipéri, C., de Ravel, T. J., Devriendt, K., et al. (2004). Novel pore mutation in SCN5A manifests as a spectrum of phenotypes ranging from atrial flutter, conduction disease, and Brugada syndrome to sudden cardiac death. Heart Rhythm 1, 610–615. doi: 10.1016/j.hrthm.2004.07.001
Rougier, J. S., van Bemmelen, M. X., Bruce, M. C., Jespersen, T., Gavillet, B., Apothéloz, F., et al. (2005). Molecular determinants of voltage-gated sodium channel regulation by the Nedd4/Nedd4-like proteins. Am. J. Physiol. Cell Physiol. 288, C692–C701. doi: 10.1152/ajpcell.00460.2004
Ruan, Y., Liu, N., Bloise, R., Napolitano, C., and Priori, S. G. (2007). Gating properties of SCN5A mutations and the response to mexiletine in long-QT syndrome type 3 patients. Circulation 116, 1137–1144. doi: 10.1161/CIRCULATIONAHA.107.707877
Rüdel, R., Ricker, K., and Lehmann-Horn, F. (1993). Genotype-phenotype correlations in human skeletal muscle sodium channel diseases. Arch Neurol. 50, 1241–1248. doi: 10.1001/archneur.1993.00540110113011
Schwartz, P. J., Priori, S. G., Dumaine, R., Napolitano, C., Antzelevitch, C., Stramba-Badiale, M., et al. (2000). A molecular link between the sudden infant death syndrome and the long-QT syndrome. N. Engl. J. Med. 343, 262–267. doi: 10.1056/NEJM200007273430405
Schwartz, P. J., Stramba-Badiale, M., Crotti, L., Pedrazzini, M., Besana, A., Bosi, G., et al. (2009). Prevalence of the congenital long-QT syndrome. Circulation 120, 1761–1767. doi: 10.1161/CIRCULATIONAHA.109.863209
Shang, L. L., and Dudley, S. C. Jr. (2005). Tandem promoters and developmentally regulated 5′- and 3′-mRNA untranslated regions of the mouse Scn5a cardiac sodium channel. J. Biol. Chem. 280, 933–940. doi: 10.1074/jbc.M409977200
Shang, L. L., Pfahnl, A. E., Sanyal, S., Jiao, Z., Allen, J., Banach, K., et al. (2007). Human heart failure is associated with abnormal C-terminal splicing variants in the cardiac sodium channel. Circ. Res. 101, 1146–1154. doi: 10.1161/CIRCRESAHA.107.152918
Smits, J. P., Eckardt, L., Probst, V., Bezzina, C. R., Schott, J. J., Remme, C. A., et al. (2002). Genotype-phenotype relationship in Brugada syndrome: electrocardiographic features differentiate SCN5A-related patients from non-SCN5A-related patients. J. Am. Coll. Cardiol. 40, 350–356. doi: 10.1016/S0735-1097(02)01962-9
Smits, J. P., Veldkamp, M. W., Bezzina, C. R., Bhuiyan, Z. A., Wedekind, H., Schulze-Bahr, E., et al. (2005). Substitution of a conserved alanine in the domain IIIS4-S5 linker of the cardiac sodium channel causes long QT syndrome. Cardiovasc. Res. 67, 459–466. doi: 10.1016/j.cardiores.2005.01.017
Sokolov, S., Scheuer, T., and Catterall, W. A. (2007). Gating pore current in an inherited ion channelopathy. Nature 446, 76–78. doi: 10.1038/nature05598
Sokolov, S., Scheuer, T., and Catterall, W. A. (2008). Depolarization-activated gating pore current conducted by mutant sodium channels in potassium-sensitive normokalemic periodic paralysis. Proc. Natl. Acad. Sci. U.S.A. 105, 19980–19985. doi: 10.1073/pnas.0810562105
Splawski, I., Shen, J., Timothy, K. W., Lehmann, M. H., Priori, S., Robinson, J. L., et al. (2000). Spectrum of mutations in long-QT syndrome genes. KVLQT1, HERG, SCN5A, KCNE1, and KCNE2. Circulation 102, 1178–1185. doi: 10.1161/01.CIR.102.10.1178
Sternberg, D., Maisonobe, T., Jurkat-Rott, K., Nicole, S., Launay, E., Chauveau, D., et al. (2001). Hypokalaemic periodic paralysis type 2 caused by mutations at codon 672 in the muscle sodium channel gene SCN4A. Brain 124, 1091–1099. doi: 10.1093/brain/124.6.1091
Stocksley, M. A., Awad, S. S., Young, C., Lightowlers, R. N., Brenner, H. R., and Slater, C. R. (2005). Accumulation of Nav1 mRNAs at differentiating postsynaptic sites in rat soleus muscles. Mol. Cell Neurosci. 28, 694–702. doi: 10.1016/j.mcn.2004.11.015
Struyk, A. F., Markin, V. S., Francis, D., and Cannon, S. C. (2008). Gating pore currents in DIIS4 mutations of NaV1.4 associated with periodic paralysis: saturation of ion flux and implications for disease pathogenesis. J. Gen. Physiol. 132, 447–464. doi: 10.1085/jgp.200809967
Struyk, A. F., Scoggan, K. A., Bulman, D. E., and Cannon, S. C. (2000). The human skeletal muscle Na channel mutation R669H associated with hypokalemic periodic paralysis enhances slow inactivation. J. Neurosci. 20, 8610–8617.
Sugiura, Y., Makita, N., Li, L., Noble, P. J., Kimura, J., Kumagai, Y., et al. (2003). Cold induces shifts of voltage dependence in mutant SCN4A, causing hypokalemic periodic paralysis. Neurology 61, 914–918. doi: 10.1212/01.WNL.0000086820.54065.A0
Swan, H., Amarouch, M. Y., Leinonen, J., Marjamaa, A., Kucera, J. P., Laitinen-Forsblom, P. J., et al. (2014). Gain-of-function mutation of the SCN5A gene causes exercise-induced polymorphic ventricular arrhythmias. Circ. Cardiovasc. Genet. 7, 771–781. doi: 10.1161/CIRCGENETICS.114.000703
Takahashi, M. P., and Cannon, S. C. (1999). Enhanced slow inactivation by V445M: a sodium channel mutation associated with myotonia. Biophys. J. 76, 861–868. doi: 10.1016/S0006-3495(99)77249-8
Takehara, N., Makita, N., Kawabe, J., Sato, N., Kawamura, Y., Kitabatake, A., et al. (2004). A cardiac sodium channel mutation identified in Brugada syndrome associated with atrial standstill. J. Intern. Med. 255, 137–142. doi: 10.1046/j.0954-6820.2003.01247.x
Tan, H. L., Kupershmidt, S., Zhang, R., Stepanovic, S., Roden, D. M., Wilde, A. A., et al. (2002). A calcium sensor in the sodium channel modulates cardiac excitability. Nature 415, 442–447. doi: 10.1038/415442a
Tanabe, S., Hata, T., and Hiraoka, M. (1999). Effects of estrogen on action potential and membrane currents in guinea pig ventricular myocytes. Am. J. Physiol. 277, H826–H833.
Tarradas, A., Selga, E., Beltran-Alvarez, P., Pérez-Serra, A., Riuro, H., Pico, F., et al. (2013). A novel missense mutation, I890T, in the pore region of cardiac sodium channel causes Brugada syndrome. PLoS ONE 8:e53220. doi: 10.1371/journal.pone.0053220
Tester, D. J., Will, M. L., Haglund, C. M., and Ackerman, M. J. (2005). Compendium of cardiac channel mutations in 541 consecutive unrelated patients referred for long QT syndrome genetic testing. Heart Rhythm 2, 507–517. doi: 10.1016/j.hrthm.2005.01.020
Tian, L., Zhu, J. F., and Yang, J. G. (2007). [Gene (SCN5A) mutation analysis of a Chinese family with Brugada syndrome]. Zhonghua Xin. Xue. Guan. Bing. Za Zhi. 35, 1122–1125.
Trimmer, J. S., Cooperman, S. S., Tomiko, S. A., Zhou, J. Y., Crean, S. M., Boyle, M. B., et al. (1989). Primary structure and functional expression of a mammalian skeletal muscle sodium channel. Neuron 3, 33–49. doi: 10.1016/0896-6273(89)90113-X
Trip, J., Drost, G., Ginjaar, H. B., Nieman, F. H., van der Kooi, A. J., de Visser, M., et al. (2009). Redefining the clinical phenotypes of non-dystrophic myotonic syndromes. J. Neurol. Neurosurg. Psychiatry 80, 647–652. doi: 10.1136/jnnp.2008.162396
Tsujino, A., Maertens, C., Ohno, K., Shen, X. M., Fukuda, T., Harper, C. M., et al. (2003). Myasthenic syndrome caused by mutation of the SCN4A sodium channel. Proc. Natl. Acad. Sci. U.S.A. 100, 7377–7382. doi: 10.1073/pnas.1230273100
Valdivia, C. R., Ackerman, M. J., Tester, D. J., Wada, T., McCormack, J., Ye, B., et al. (2002). A novel SCN5A arrhythmia mutation, M1766L, with expression defect rescued by mexiletine. Cardiovasc. Res. 55, 279–289. doi: 10.1016/S0008-6363(02)00445-5
van Bemmelen, M. X., Rougier, J. S., Gavillet, B., Apothéloz, F., Daidie, D., Tateyama, M., et al. (2004). Cardiac voltage-gated sodium channel Nav1.5 is regulated by Nedd4-2 mediated ubiquitination. Circ. Res. 95, 284–291. doi: 10.1161/01.RES.0000136816.05109.89
van den Boogaard, M., Smemo, S., Burnicka-Turek, O., Arnolds, D. E., van de Werken, H. J., Klous, P., et al. (2014). A common genetic variant within SCN10A modulates cardiac SCN5A expression. J. Clin. Invest. 124, 1844–1852. doi: 10.1172/JCI73140
van Langen, I. M., Birnie, E., Alders, M., Jongbloed, R. J., Le, M. H., and Wilde, A. A. (2003). The use of genotype-phenotype correlations in mutation analysis for the long QT syndrome. J. Med. Genet. 40, 141–145. doi: 10.1136/jmg.40.2.141
Vatta, M., Dumaine, R., Antzelevitch, C., Brugada, R., Li, H., Bowles, N. E., et al. (2002). Novel mutations in domain I of SCN5A cause Brugada syndrome. Mol. Genet. Metab. 75, 317–324. doi: 10.1016/S1096-7192(02)00006-9
Veerman, C. C., Wilde, A. A., and Lodder, E. M. (2015). The cardiac sodium channel gene SCN5A and its gene product NaV1.5: Role in physiology and pathophysiology. Gene 573, 177–187. doi: 10.1016/j.gene.2015.08.062
Viadero, M. T., Rubín, E., Amigo, T., and González-Lamuño, D. (2011). Three generations of hereditary long-QT syndrome with complete penetrance caused by the p.G316E KCNQ1 mutation. Pediatr. Cardiol. 32, 102–104. doi: 10.1007/s00246-010-9821-7
Vicart, S., Sternberg, D., Fontaine, B., and Meola, G. (2005). Human skeletal muscle sodium channelopathies. Neurol. Sci. 26, 194–202. doi: 10.1007/s10072-005-0461-x
Vicart, S., Sternberg, D., Fournier, E., Ochsner, F., Laforet, P., Kuntzer, T., et al. (2004). New mutations of SCN4A cause a potassium-sensitive normokalemic periodic paralysis. Neurology 63, 2120–2127. doi: 10.1212/01.WNL.0000145768.09934.EC
Wahbi, K., Algalarrondo, V., Bécane, H. M., Fressart, V., Beldjord, C., Azibi, K., et al. (2013). Brugada syndrome and abnormal splicing of SCN5A in myotonic dystrophy type 1. Arch. Cardiovasc. Dis. 106, 635–643. doi: 10.1016/j.acvd.2013.08.003
Walsh, R., Peters, N. S., Cook, S. A., and Ware, J. S. (2014). Paralogue annotation identifies novel pathogenic variants in patients with Brugada syndrome and catecholaminergic polymorphic ventricular tachycardia. J. Med. Genet. 51, 35–44. doi: 10.1136/jmedgenet-2013-101917
Wang, C., Wang, C., Hoch, E. G., and Pitt, G. S. (2011). Identification of novel interaction sites that determine specificity between fibroblast growth factor homologous factors and voltage-gated sodium channels. J. Biol. Chem. 286, 24253–24263. doi: 10.1074/jbc.M111.245803
Wang, D. W., Desai, R. R., Crotti, L., Arnestad, M., Insolia, R., Pedrazzini, M., et al. (2007). Cardiac sodium channel dysfunction in sudden infant death syndrome. Circulation 115, 368–376. doi: 10.1161/CIRCULATIONAHA.106.646513
Wang, Q., Chen, S., Chen, Q., Wan, X., Shen, J., Hoeltge, G. A., et al. (2004). The common SCN5A mutation R1193Q causes LQTS-type electrophysiological alterations of the cardiac sodium channel. J. Med. Genet. 41, e66. doi: 10.1136/jmg.2003.013300
Wang, Q., Li, Z., Shen, J., and Keating, M. T. (1996). Genomic organization of the human SCN5A gene encoding the cardiac sodium channel. Genomics 34, 9–16. doi: 10.1006/geno.1996.0236
Wang, Q., Shen, J., Splawski, I., Atkinson, D., Li, Z., Robinson, J. L., et al. (1995). SCN5A mutations associated with an inherited cardiac arrhythmia, long QT syndrome. Cell 80, 805–811. doi: 10.1016/0092-8674(95)90359-3
Ware, J. S., Walsh, R., Cunningham, F., Birney, E., and Cook, S. A. (2012). Paralogous annotation of disease-causing variants in long QT syndrome genes. Hum. Mutat. 33, 1188–1191. doi: 10.1002/humu.22114
Webb, J., and Cannon, S. C. (2008). Cold-induced defects of sodium channel gating in atypical periodic paralysis plus myotonia. Neurology 70, 755–761. doi: 10.1212/01.wnl.0000265397.70057.d8
Wu, L., Yong, S. L., Fan, C., Ni, Y., Yoo, S., Zhang, T., et al. (2008). Identification of a new co-factor, MOG1, required for the full function of cardiac sodium channel Nav 1.5. J. Biol. Chem. 283, 6968–6978. doi: 10.1074/jbc.M709721200
Wu, L., Zhang, B., Kang, Y., and Wu, W. (2014). Enhanced slow inactivation of the human skeletal muscle sodium channel causing normokalemic periodic paralysis. Cell Mol. Neurobiol. 34, 707–714. doi: 10.1007/s10571-014-0052-y
Wu, Q., and Krainer, A. R. (1999). AT-AC pre-mRNA splicing mechanisms and conservation of minor introns in voltage-gated ion channel genes. Mol. Cell Biol. 19, 3225–3236. doi: 10.1128/MCB.19.5.3225
Yang, N., Ji, S., Zhou, M., Ptácek, L. J., Barchi, R. L., Horn, R., et al. (1994). Sodium channel mutations in paramyotonia congenita exhibit similar biophysical phenotypes in vitro. Proc. Natl. Acad. Sci. U.S.A. 91, 12785–12789. doi: 10.1073/pnas.91.26.12785
Yang, P., Kupershmidt, S., and Roden, D. M. (2004). Cloning and initial characterization of the human cardiac sodium channel (SCN5A) promoter. Cardiovasc. Res. 61, 56–65. doi: 10.1016/j.cardiores.2003.09.030
Ye, B., Valdivia, C. R., Ackerman, M. J., and Makielski, J. C. (2003). A common human SCN5A polymorphism modifies expression of an arrhythmia causing mutation. Physiol. Genomics 12, 187–193. doi: 10.1152/physiolgenomics.00117.2002
Yi, S. D., Meng, S. R., Cui, Y. K., Chen, Z. M., and Peng, J. (2003). [PCR-based site-directed mutagenesis and recombinant expression plasmid construction of a SCN5A mutation (K317N) identified in a Chinese family with Brugada syndrome]. Di Yi Jun Yi Da Xue Xue Bao 23, 1139–1142.
Yoshinaga, H., Sakoda, S., Good, J. M., Takahashi, M. P., Kubota, T., Arikawa-Hirasawa, E., et al. (2012). A novel mutation in SCN4A causes severe myotonia and school-age-onset paralytic episodes. J. Neurol. Sci. 315, 15–19. doi: 10.1016/j.jns.2011.12.015
Yoshinaga, H., Sakoda, S., Shibata, T., Akiyama, T., Oka, M., Yuan, J. H., et al. (2015). Phenotypic variability in childhood of skeletal muscle sodium channelopathies. Pediatr. Neurol. 52, 504–508. doi: 10.1016/j.pediatrneurol.2015.01.014
Young, K. A., and Caldwell, J. H. (2005). Modulation of skeletal and cardiac voltage-gated sodium channels by calmodulin. J. Physiol. 565, 349–370. doi: 10.1113/jphysiol.2004.081422
Zeng, Z., Zhou, J., Hou, Y., Liang, X., Zhang, Z., Xu, X., et al. (2013). Electrophysiological characteristics of a SCN5A voltage sensors mutation R1629Q associated with Brugada syndrome. PLoS ONE 8:e78382. doi: 10.1371/journal.pone.0078382
Ziane, R., Huang, H., Moghadaszadeh, B., Beggs, A. H., Levesque, G., and Chahine, M. (2010). Cell membrane expression of cardiac sodium channel Na(v)1.5 is modulated by alpha-actinin-2 interaction. Biochemistry 49, 166–178. doi: 10.1021/bi901086v
Keywords: Nav1.4, Nav1.5, physiopathology, associated/regulatory proteins, missense mutations
Citation: Loussouarn G, Sternberg D, Nicole S, Marionneau C, Le Bouffant F, Toumaniantz G, Barc J, Malak OA, Fressart V, Péréon Y, Baró I and Charpentier F (2016) Physiological and Pathophysiological Insights of Nav1.4 and Nav1.5 Comparison. Front. Pharmacol. 6:314. doi: 10.3389/fphar.2015.00314
Received: 05 November 2015; Accepted: 21 December 2015;
Published: 14 January 2016.
Edited by:
Mohamed Chahine, Laval University, CanadaReviewed by:
Anselm Zdebik, University College London, UKAdrien Moreau, Centre de Recherche de l'Institut Universitaire en Santé, Canada
Copyright © 2016 Loussouarn, Sternberg, Nicole, Marionneau, Le Bouffant, Toumaniantz, Barc, Malak, Fressart, Péréon, Baró and Charpentier. This is an open-access article distributed under the terms of the Creative Commons Attribution License (CC BY). The use, distribution or reproduction in other forums is permitted, provided the original author(s) or licensor are credited and that the original publication in this journal is cited, in accordance with accepted academic practice. No use, distribution or reproduction is permitted which does not comply with these terms.
*Correspondence: Gildas Loussouarn, Z2lsZGFzLmxvdXNzb3Vhcm5AaW5zZXJtLmZy