- 1Department of Anatomy, Physiology and Genetics, F. Edward Hebert School of Medicine, Uniformed Services University of the Health Sciences, Bethesda, MD, USA
- 2Developmental Therapeutic Branch, National Cancer Institute, National Institutes of Health, Bethesda, MD, USA
- 3Department of Medicine, F. Edward Hebert School of Medicine, Uniformed Services University of the Health Sciences, Bethesda, MD, USA
- 4Department of Biochemistry and Molecular Biology, LSU Health Sciences Center, New Orleans, LA, USA
- 5Department of Biochemistry, F. Edward Hebert School of Medicine, Uniformed Services University of the Health Sciences, Bethesda, MD, USA
Histone deacetylase inhibitors (HDIs) are under investigation for the treatment of a number of human health problems. HDIs have proven therapeutic value in refractory cases of cutaneous T-cell lymphoma. Electrocardiographic ST segment morphological changes associated with HDIs were observed during development. Because ST segment morphology is typically linked to changes in ATP sensitive potassium (KATP) channel activity, we tested the hypothesis that HDIs affect cardiac KATP channel subunit expression. Two different HDIs, romidepsin and trichostatin A, caused ~20-fold increase in SUR2 (Abcc9) subunit mRNA expression in HL-1 cardiomyocytes. The effect was specific for the SUR2 subunit as neither compound causes a marked change in SUR1 (Abcc8) expression. Moreover, the effect was cell specific as neither HDI markedly altered KATP subunit expression in MIN6 pancreatic β-cells. We observe significant enrichment of the H3K9Ac histone mark specifically at the SUR2 promoter consistent with the conclusion that chromatin remodeling at this locus plays a role in increasing SUR2 gene expression. Unexpectedly, however, we also discovered that HDI-dependent depletion of cellular cholesterol is required for the observed effects on SUR2 expression. Taken together, the data in the present study demonstrate that KATP subunit expression can be epigenetically regulated in cardiomyocytes, defines a role for cholesterol homeostasis in mediating epigenetic regulation and suggests a potential molecular basis for the cardiac effects of the HDIs.
Introduction
Histone deacetylase inhibitors (HDIs) have emerged as potential therapeutic agents for the treatment of cancer and heart disease (Ma et al., 2009; McKinsey, 2012). Histone deacetylases (HDACs) are known to be key co-factors of the machinery that regulates gene transcription. HDACs typically work in concert with DNA binding proteins to remove covalently attached acetyl groups from lysine residues contained within histone proteins, resulting in less accessible chromatin and reduced gene transcription (Kouzarides, 2007), however, this simple model of HDI action may belie the diverse effects of HDIs that can affect both normal and cancerous cell viability (Falkenberg and Johnstone, 2014).
To date, four HDIs—romidepsin, vorinostat, belinostat, and panobinostat—have been approved by the FDA for treating heamatologic malignancies including refractory cutaneous T-cell lymphoma or multiple myeloma (Mann et al., 2007; Piekarz et al., 2011; Coiffier et al., 2012; Lee et al., 2015; Richardson et al., 2015). While HDIs have proven successful, cardiac safety has been a concern. Non-specific ST segment changes, long QT, atrial fibrillation, and torsades de pointes have all been reported in clinical trials (Sandor et al., 2002; Shah et al., 2006; Stadler et al., 2006; Steele et al., 2008; Lynch et al., 2012; Noonan et al., 2013). In a recent trial with romidepsin, the most common ECG finding was ST segment morphological changes with no evidence of associated myocardial ischemia (Noonan et al., 2013). ST segment elevation and depression are typically associated with the activation of ATP-sensitive (KATP) channels during ischemia (Kubota et al., 1993; Li et al., 2000), suggesting that HDIs may specifically affect KATP channel subunit expression in cardiac myocytes.
HDIs have also shown promise in the treatment of cardiovascular disease (McKinsey, 2012). Trichostatin A (TSA) interferes with phenylephrine-induced hypertrophy of neonatal ventricular myocytes (Cao et al., 2011) and studies in animal models have shown that HDIs inhibit the development of cardiac hypertrophy due to angiotensin II infusion, aortic banding or pressure overload (Kee et al., 2006; Kong et al., 2006). HDIs have also been shown to inhibit the expression of hypertrophic genes, inflammatory markers, and fibrosis in the diseased heart (Liu et al., 2008; Cardinale et al., 2010; Cao et al., 2011). Interestingly, HDIs have also been shown to reduce infarct size and maintain contractile function following ischemia-reperfusion, similar to the effects of pharmacological or ischemic preconditioning (Zhao et al., 2007; Xie et al., 2014), which again is associated with activation of sarcolemmal KATP channels (Suzuki et al., 2002).
Based on these observations, we hypothesized that HDIs may specifically affect the expression of KATP channel subunits. A single KATP channel is a hetero-octomeric protein complex comprised of four pore-forming, inward rectifier (Kir6.1 or Kir6.2) and four sulfonylurea receptor (SUR1 or SUR2) subunits that are encoded by four separate genes (Inagaki et al., 1995a,b, 1996; Chutkow et al., 1999). Specific subunit combinations make up KATP channels in different tissues. For example, KATP channels in mouse atrial cardiomyocytes and pancreatic β-cells are composed of SUR1 and Kir6.2, while SUR2A and Kir6.2 form KATP in ventricular myocytes (Inagaki et al., 1995b, 1996; Flagg et al., 2008). There are relatively few studies to identify factors that regulate KATP channel subunit expression and many of these have been focused on pancreatic β-cell models rather than cardiomyocytes (Ashfield and Ashcroft, 1998; Hernandez-Sanchez et al., 1999; Kim et al., 2002). One study focused on myocardium demonstrated an important role for forkhead transcription factors in regulating KATP expression (Philip-Couderc et al., 2008), and we recently showed that DNA methylation can modulate SUR2 expression suggesting that epigenetic mechanisms may also regulate KATP subunit expression (Fatima et al., 2012). There have been no previous studies examining the effects of HDACs or HDIs on KATP subunit expression.
The results show that HDIs cause a specific increase in SUR2 expression in HL-1 cardiomyocytes but have no effect on MIN6 pancreatic β-cells indicating that HDIs have tissue specific mechanisms of action. Moreover, while HDAC-dependent histone acetylation likely contributes to the regulation of KATP expression, we made the unexpected discovery that TSA-dependent depletion of cholesterol was required for changes in SUR2 expression. Taken together the data demonstrate that HDIs specifically affect transcription of sarcolemmal KATP channel subunits and define a novel cholesterol-dependent mechanism that links HDIs with KATP subunit expression.
Materials and Methods
Cell Culture
Atrial myocyte derived HL-1 cell cultures were maintained in Claycomb medium (Sigma) (Claycomb et al., 1998). Cells were plated in tissue culture flasks coated overnight with gelatin (0.02% w/v) and fibronectin (0.5% v/v). MIN6 cells were maintained in DMEM containing 10% fetal bovine serum and 100 μg/mL penicillin/streptomycin. In all experiments to inhibit histone deacetylase (HDAC) activity, cells were incubated in standard culture medium supplemented with Trichostatin A (TSA) or Romidepsin at concentrations given in the text for 72 h. In some experiments, culture medium was also supplemented with cholesterol (250X cholesterol lipid concentrate, Gibco). Culture medium was changed daily.
Quantitative RT-PCR
Relative expression of KATP channel subunit mRNA was examined using quantitative RT-PCR, as described previously (Fatima et al., 2012). Total RNA was isolated using a silica-based column protocol (Qiagen, RNeasy) following the manufacturer's protocols. Isolated RNA was then treated with DNAseI to digest residual genomic DNA and repurified using a silica-based column protocol. RNA concentration was determined spectrophotometrically (Nanodrop Technologies, Inc). cDNA was synthesized from 1 μg RNA (Superscript III, Invitrogen). PCR was carried out using a CFX384 Real Time PCR Detection System (Bio-Rad, Inc.), using Taqman© probe and primer pairs (Applied Biosystems, Inc.) for monitoring reaction progress. Twenty nano gram of template cDNA was used in all reactions. Reactions with each primer/probe pair and template were performed in triplicate. Relative mRNA expression (compared to Hprt) is reported as 2−ΔCt * 1000 for untreated HL-1 and MIN6 cells. The fold change in expression in response to drug treatment is reported as 2−ΔΔCt, where ΔΔCt = ΔCt (drug)−ΔCt (notreatment).
Protein Isolation and Western Analysis
Cells were washed with PBS and disrupted with RIPA lysis buffer (Santa Cruz) supplemented with protease inhibitors (Roche). Protein concentration was determined by the BCA method (Pierce). For Western analysis, proteins (typically 50 μg) were separated by PAGE and transferred to PVDF membrane. Signal was visualized with the SuperSignal West Dura ECL substrate (Pierce).
Chromatin Immunoprecipitation (ChIP)
Chromatin immunoprecipitation (ChIP) assay was performed using the SimpleChip enzymatic chromatin IP kit (Cell Signaling Technology) according to manufacturer's protocol. Briefly, 1 × 108 cells were incubated with 1% formaldehyde in 1X PBS for 10 min at room temperature followed by quenching with 0.125 M glycine for 5 min. After cell lysis, cross-linked chromatin was digested with micrococcal nuclease, followed by sonication to yield fragments of 200–1000 bp. Soluble chromatin fragments were incubated with antibodies against AcH3 (Abcam, ab-10812), control IgG (Cell Signaling Technology, 2729) and H3 (Cell Signaling Technology, 4620). Bound chromatin was precipitated using MagnaChip protein A+G magnetic beads (Millipore). Precipitated complexes were eluted, decrosslinked and purified. Purified DNA was amplified by qPCR with primer set corresponding to the promoter regions (−1 kB) for SUR1 and SUR2 (Qaigen). The data was analyzed according to comparative Ct method, normalized to IgG control and reported as fold change in binding relative to control.
Genome-wide Expression Analysis
MouseRef-8 v2.0 Expression BeadChips (Illumina Inc., San Diego, CA, USA) were used to measure relative levels of mRNA expression for 24,854 well-established annotated coding transcripts. Preparation of labeled cDNA, hybridization, imaging, and data collection were carried out at the Cleveland Clinic Genome Core Facility. Background subtracted, quantile normalized data were generated and analyzed using GenomeStudio (Illumina Inc., San Diego, CA, USA), GenePattern 2.0 (Broad Institute, Cambridge, MA, USA) and GATHER (Chang and Nevins, 2006) software packages. Four paired samples (treated and control) of each group were tested. Cells were treated with either TSA (30 mg/mL) or Romidepsin (5 nM). Differentially expressed transcripts were filtered using strict criteria for, accurate detection in at least three out of four samples, transcripts with 4-fold expression level differences and were regulated by both TSA and Romidepsin.
Cholesterol Labeling with Filipin
The protocols were adapted from the protocols of Leventhal et al. (2001). After treatment for 72 h with TSA or TSA combined with cholesterol, HL-1 and MIN6 cells were fixed in formalin for 10 min at room temperature. Fixed cells were labeled with filipin (0.05 mg/mL in PBS, Sigma) and PicoGreen (1:1000, Molecular Probes) for 1 h at room temperature to label non-esterified cholesterol and DNA, respectively. Images were obtained using a Leica AF6000 using the same exposure between samples within an experiment.
Results
HDIs Increase SUR2 Subunit Expression in HL-1 Cardiomyocytes but not MIN6 Pancreatic β-cells
The objective of the present study was to test the hypothesis that histone deacetylase inhibitors (HDIs) cause changes in KATP channel subunit expression. For these studies, we made use of two cell lines that express KATP channel subunits—HL-1 atrial cardiomyocytes and MIN6 pancreatic β-cells. Figure 1 shows the distribution of SUR1 and SUR2 in HL-1 cells. Expression of SUR 1 is greater than SUR2 in both HL-1 and MIN6 cells, consistent with the distribution of subunits in primary tissue (Inagaki et al., 1995b, 1996; Flagg et al., 2008). In this study, we compared the responses of these two cell types to determine 1) if HDIs modulate KATP expression and 2) if the effects are tissue specific.
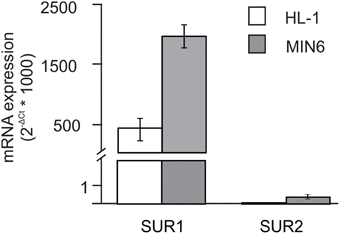
Figure 1. SUR1 and SUR2 expression in HL-1 and MIN6 cell lines. Relative mRNA expression of SUR1 and SUR2 obtained in atrial myocyte-derived HL-1 (n = 13) and pancreatic β-cell-derived MIN6 cells (n = 5). Expression (normalized to Hprt) was assessed by quantitative RT-PCR. Gene-specific Taqman primer and probes were obtained from Applied Biosystems. SUR1 expression was markedly higher in both cell lines when compared to SUR2.
Figure 2 shows the effects of HDAC inhibition on SUR1 and SUR2 mRNA expression in HL-1 cardiomyocytes. Treatment for 72 h with trichostatin A (TSA) induced a concentration-dependent increase in SUR2 mRNA expression with little or no change in SUR1. In contrast to the results with HL-1 cells, neither SUR1 nor SUR2 mRNA expression was markedly altered by HDAC inhibition in MIN6 pancreatic β-cells (Figure 2B). Taken together, these data demonstrate that HDIs do modulate KATP subunit expression and that this occurs in a cell or tissue specific manner.
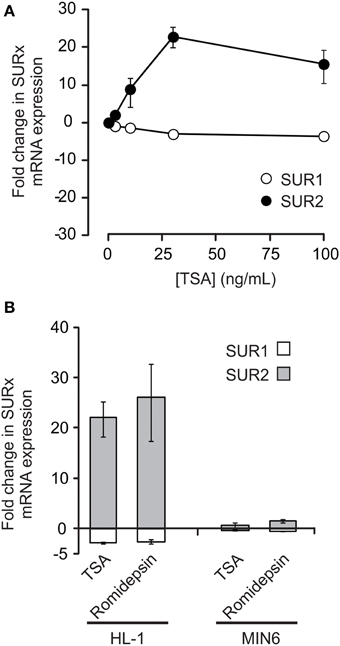
Figure 2. HDAC inhibitors (HDIs) significantly increase SUR2 expression in HL-1 cells but not MIN6 cells. (A) HDAC inhibition with trichostatin A (TSA) caused a marked, dose-dependent, increase in SUR2 expression, while SUR1 expression is essentially unchanged (n = 3–10). (B) Like TSA (30 ng/mL), another HDI, romidepsin (5 nM), caused an increase in SUR2 expression in HL-1 cardiomyocytes with little change in SUR1. In contrast, neither TSA nor romidepsin affected SUR subunit expression in MIN6 pancreatic β-cells (n = 5).
Expression of SUR2 Correlates with Histone Acetylation at the Promoter
To begin to elucidate the mechanism underlying the TSA-dependent increase in SUR2 expression, we confirmed that the HDAC inhibitors induced a global increase in histone acetylation in HL-1 cells. As expected, treatment with TSA significantly increased the amount of acetylated histone H3 and H4 without changes in the total histone protein (Figure 3A). Moreover, the increase in histone acetylation is concentration dependent and mirrors the concentration dependence of changes in SUR2 expression, suggesting that HDIs are influencing SUR2 expression through epigenetic histone modification. TSA also induces a similar increase in histone acetylation in MIN6 cells (Figure 3B). We examined the effects of TSA on gene specific histone acetylation using chromatin immunoprecipitation (ChIP). These results (Figure 3C) demonstrate a significant enrichment of the H3K9Ac histone mark at the SUR2, but not SUR1, promoter. Taken together, these data indicate that HDAC activity at the SUR2 promoter normally suppresses SUR2 transcription in HL-1 cardiomyocytes and that inhibition of this activity causes a specific increase in SUR2 expression.
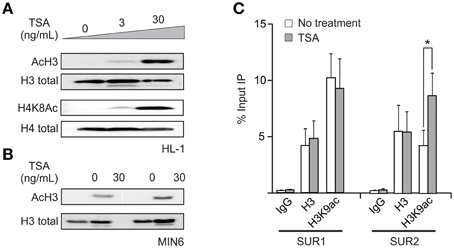
Figure 3. Changes in SUR2 expression correlate with increased histone acetylation at the promoter. (A) Representative western blot demonstrating the concentration-dependent increase in histone acetylation induced by TSA (30 ng/mL). A significant increase in both H3K9ac and H4K8ac activating histone marks, with no change in total histone protein, was observed. The concentration dependence of the TSA-dependent increase in SUR2 expression appears to correlate with the increase in histone acetylation. (B) Representative western blot showing that exposure of MIN6 cells to TSA causes a similar increase in histone acetylation. (C) Summary data from chromatin immunoprecipitation (ChIP) experiments demonstrating that the activating histone mark H3K9ac is enriched at the SUR2, but not SUR1, promoter following TSA treatment. *p < 0.05.
Genome-wide Expression Analysis Reveals Potential Role for Cholesterol Homeostasis
To gain further mechanistic insight, we next performed a comprehensive genome-wide expression BeadArray profiling to determine the transcripts that are up- and down-regulated by HDIs in HL-1 cells (Figure 4). Three hundred fifty five genes were found to be increased or decreased more than 4-fold in the presence of TSA. Similarly, 415 genes were changed more than 4-fold by romidepsin with 171 genes common to the two data sets. Analysis of the data set using the GATHER software (Chang and Nevins, 2006) revealed marked changes in genes associated with muscle contraction (GO: 0006936), including Myh6, Ttn, Casq2, Tnni3, Acta1, Nppb, and Tnni2. qRT-PCR analysis confirmed at least 4-fold differential expression in five of the seven transcripts that we tested (Figure 4C). One of these transcripts (Tnni2) was expressed at very low levels and the other (Ttn) was only decreeased 2.8-fold. We also annotated the 171 common gene dataset for enrichment of transcription factor binding sites (TRANSFAC v 8.2). Interestingly, this analysis demonstrated significant enrichment for sterol response element binding proteins (SREBPs) activity in response to HDIs. (60 of 171 transcripts, p < 0.0001). The enrichment of SREBP binding sites in the subset of transcripts affected by HDIs suggests that SREBP may play a role in mediating the effects of HDIs on KATP channel subunit expression. This possibility was wholly unexpected, however, it should be pointed out that a previous study noted the presence of SREBP binding sites in the promoter regions of the SUR2 and Kir6.2 genes (Philip-Couderc et al., 2008), and therefore we followed up this lead.
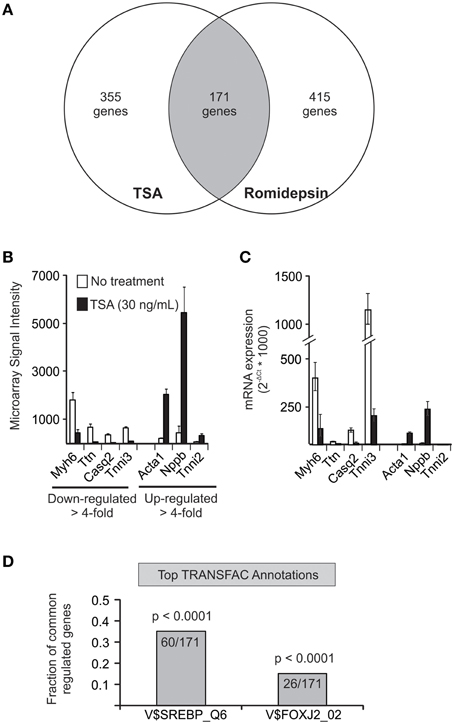
Figure 4. BeadArray profiling reveals a potential role for cholesterol and SREBP. (A) Venn diagram illustrating the number of genes specifically changed (≥4-fold increase or decrease). Analysis using GATHER software (Chang and Nevins, 2006) focused on the common gene set that was altered by both compounds. (B) Shown are the BeadArray signal intensities of seven transcripts that are included in the top gene ontology hit (GO0006936: muscle contraction). (C) Expression changes were confirmed in 5 of 7 genes tested by qRT-PCR. (D) Annotation of common gene set for transcription factor binding sites (TRANSFAC, v8.2) revealed a putative role for SREBP in 60 out of 171 genes annotated.
HDIs Specifically Deplete HL-1 Cells of Cholesterol
A major physiological regulator of SREBP activity is cellular cholesterol. Reduced cholesterol levels in the endoplasmic reticulum trigger a sequence of events leading to cleavage of full length SREBP, allowing translocation of the transcriptionally active SREBP cleavage product to the nucleus (Jeon and Osborne, 2012). Interestingly, recent evidence suggests that HDIs can interfere with cholesterol homeostasis (Meaney, 2014). Therefore, we investigated the effects of TSA treatment on cellular cholesterol in HL-1 and MIN6 cells cells using filipin, a fluorescent antibiotic that has been used as a qualitative measure of cellular cholesterol (Bittman and Fischkoff, 1972). TSA exposure markedly decreased filipin staining in HL-1 cells and cholesterol but did not affect filipin staining in MIN6 cells (Figure 5) suggesting that TSA-dependent cholesterol depletion might be required for TSA effects on gene expression.
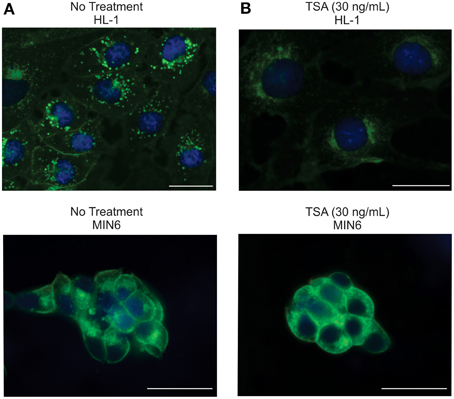
Figure 5. TSA depletes cholesterol in HL-1 cells, but not MIN6 cells. (A) Representative images of HL-1 or MIN6 cells labeled with filipin (Cholesterol, blue) and picoGreen (Nuclei, green). (B) Treatment with TSA (30 ng/mL) markedly reduces filipin staining in HL-1 cells but has little effect on filipin staining in MIN6 cells. Scale bar = 25 μm.
Cholesterol Suppresses the Effects of HDIs on KATP Subunit Expression
To determine whether TSA-dependent disruption of cholesterol homeostasis mediates the effects on KATP expression, we assessed SUR2 expression in HL-1 cells incubated in culture medium supplemented with increasing concentrations of cholesterol and a constant concentration of TSA. Addition of cholesterol to the media completely restored cellular cholesterol as evidenced by the restoration of filipin staining (Figure 6A). Cholesterol also abolished the effects of TSA on gene expression in a concentration-dependent manner (Figure 6B). Importantly, TSA continued to inhibit HDAC activity as evidenced by the increase in acetylated H3 in the presence or absence of cholesterol. Taken together, this data indicates that cholesterol depletion is required for HDI-dependent effects on KATP subunit expression in HL-1 cardiomyocytes.
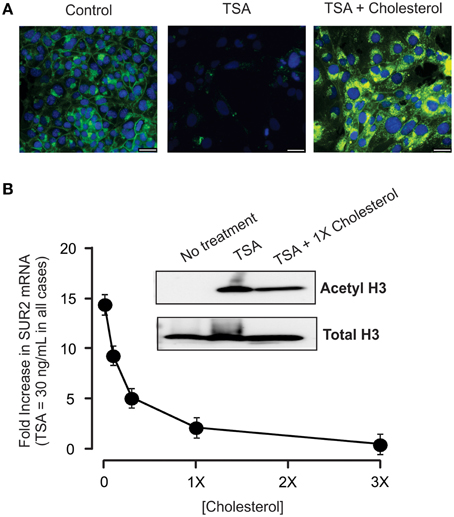
Figure 6. Cholesterol abolishes the effect of TSA. (A) Representative images of HL-1 cells treated with TSA alone or TSA + 1X cholesterol. Inclusion of cholesterol in the media prevents the marked reduction in cellular cholesterol caused by TSA. Scale bar = 25 μm. (B) Summary data from experiments (n = 5) in which HL-1 cells were treated with a constant concentration of TSA (30 ng/mL) together with increasing amounts of cholesterol. Importantly, cholesterol did not prevent the increase in histone acetylation (inset) indicating that cholesterol did not interfere with TSA access to the cell or enzymatic activity.
Discussion
The data in the present study demonstrate two novel aspects regarding the transcriptional control of KATP channel subunits. First, we show that inhibiting HDAC activity specifically increases SUR2 gene transcription in HL-1 cardiomyocytes. This result implies that under normal circumstances HDACs are recruited to the SUR2 gene locus in HL-1 cells contributing to the suppression of SUR2 expression in these cells under basal conditions. Second, we show that disruption of cellular cholesterol homeostasis is a critical mediator of HDI effects in HL-1 cells. This result implies that cholesterol homeostasis, in general, may be an important determinant of KATP expression in the cardiovascular system.
Specific Epigenetic Regulation of KATP Channel Subunit Expression by HDACs
Covalent histone modifications including acetylation, methylation, ubiquitylation, sumoylation, and phosphorylation are increasingly recognized as epigenetic regulators of when and where genes are actively expressed (Kouzarides, 2007). By promoting a more compact chromatin conformation, histone deacetylation is an important gene silencing factor. Here we show that inhibiting HDAC activity increases SUR2 gene transcription in HL-1 cardiomyocytes. This is the first demonstration that HDIs can influence KATP channel subunit expression providing evidence that KATP channel subunit expression is normally determined in part by HDAC-dependent epigenetic regulation.
Specificity is a key feature of epigenetic regulation of KATP channel expression. First, HDIs exhibit cell specific effects as evidenced by the observation that KATP subunit expression is markedly affected in HL-1 cardiomyocytes but unaffected in MIN6 cells. HL-1 cells appear to be primed to express SUR2, but tonic suppression by HDACs normally maintains a low level of SUR2 expression. In contrast, the appropriate transcriptional activators of SUR2 expression appear to be absent in MIN6 cells and in this case suppression of SUR2 gene expression by HDACs would be redundant and is therefore not present. In addition to cell specificity, epigenetic regulation of KATP channel expression is also subunit specific. HDIs specifically increase histone acetylation at the SUR2 gene but have no effect at the SUR1 locus. This demonstrates that HDACs are recruited specifically to the SUR2 locus in order to establish the pattern of KATP subunit expression observed in HL-1 cells.
HDI Therapy, Epigenetics, and Arrhythmia
Disruption of the appropriate KATP channel composition or function has been shown to cause arrhythmias or abnormal ECG responses to ischemia. Using transgenic models that overexpress KATP subunits, we have shown that disrupting normal KATP composition by overexpressing SUR1 is arrhythmogenic (Flagg et al., 2007), illustrating the importance of establishing the appropriate channel composition in the mouse heart (Flagg et al., 2008; Glukhov et al., 2010). Genetic or pharmacologic inhibition of KATP, for example, completely abolishes the ST segment elevation associated with ischemia (Kubota et al., 1993; Li et al., 2000) and KATP channel openers such as pinacidil have been shown to induce ST segment elevation independent of ischemia (Yan and Antzelevitch, 1999). KATP is a particularly potent means of modulating cardiac action potential duration. Activation of just 1% of the total KATP channels available has been shown to shorten the APD by 50% (Nichols et al., 1991; Weiss et al., 1992; Knopp et al., 1999). Moreover, in ischemia models it has been shown that shortening of APD by only about 20% is correlated with marked ST elevation of about 5–10 mV (Kingaby et al., 1986). Based on these observations, it is possible that even very small changes in KATP could be manifest as changes in the ECG.
The onset of cardiac arrhythmias has been observed in several clinical and pre-clinical studies of HDI therapy (Berry et al., 2008). Ventricular tachycardia, torsades de pointes, and atrial fibrillation have been reported as rare cardiovascular events in early phase trials of HDIs (Sandor et al., 2002; Kelly et al., 2005; Piekarz et al., 2006; Lynch et al., 2012). The major cardiac effect associated with romidepsin therapy is asymptomatic, non-specific, ischemia-independent ST segment elevation or depression (Sandor et al., 2002; Piekarz et al., 2006; Noonan et al., 2013). Because ST segment elevation is typically associated with activation of KATP channels (Kubota et al., 1993; Li et al., 2000), the cardio-specific effects of romidepsin and TSA on KATP channel subunit expression provide a potential molecular basis for the cardiac effects associated with HDIs. Similarly, re-entrant arrhythmias can be promoted by activation of KATP (Billman, 2008). Other mechanisms such as decreased connexin 43 expression (Xu et al., 2013) or non-specific HERG blockade have been suggested to account for the cardiac effects of HDI therapy. Here we suggest that epigenetic alterations in cardiac KATP channel expression may also contribute to the electrophysiological events observed during HDI-therapy.
HDIs, Cholesterol, and KATP Expression
The second major finding in this study is the discovery of a key role for cholesterol depletion in mediating the effect of HDIs on SUR2 expression. The key clue that focused attention on cholesterol was the association of SREBP with the gene changes induced by the HDIs (Figure 4). SREBPs are transcription factors that are typically associated with cellular lipid homeostasis (Jeon and Osborne, 2012). Previous studies have linked HDIs with a reduction of cholesterol. For example, Niemann-Pick C disease causes intracellular cholesterol accumulation as a result of mutations in the NPC1 or NPC2 gene product that are critical for cholesterol trafficking [Ory, 2004 and HDIs have been shown to largely correct the cholesterol accumulation phenotype both in fibroblasts cultured from human NPC patients (Pipalia et al., 2011) and neural stem cells from NPC−−∕−− mice (Kim et al., 2007). Similarly, others have shown that TSA causes a significant reduction in cellular cholesterol by increasing expression of genes that traffic and export cholesterol as well as inhibiting expression of genes in the mevalonate pathway of cholesterol synthesis (Nunes et al., 2013). The specific molecular mechanisms driving cholesterol reduction in HL-1 cells in the present study remain to be determined as does the cell-specific basis for the HDI effects on cholesterol homeostasis. Nevertheless, it is clear that HDIs significantly disrupt cellular lipid homeostasis in HL-1 cardiomyocytes, but not MIN6 pancreatic β-cells.
The data indicate that maintaining cellular cholesterol prevents the effect of HDIs on KATP subunit expression. This finding suggests the very intriguing conclusion that there is a link between cellular cholesterol homeostasis and KATP expression. There have been no previous studies that directly link cholesterol homeostasis with KATP channel expression. In silico analysis previously identified SREBP binding sites in the SUR2 and Kir6.2 promoters (Philip-Couderc et al., 2008), but the functional relevance of those putative binding sites has not specifically been explored. Interestingly, there have been studies that indirectly link cholesterol homeostasis with KATP expression. Hypercholesterolemia, which is expected to inhibit SREBP, has been associated with impaired ischemic preconditioning (Csonka et al., 2014) which requires functional sarcolemmal KATP channels (Suzuki et al., 2002). It should also be noted that there is a precedent for lipid homeostasis and SREBP in regulating the expression of an inward rectifying potassium channel. Park and colleagues demonstrated that lipid lowering leads to an increase in GIRK1 expression through activation of SREBP-1 (Park et al., 2008). The present results suggest that cholesterol homeostasis and lipid metabolism in general may have analogous effects on KATP subunit expression with consequent arrhythmias.
Conclusion
In summary, the results presented in this study demonstrate that HDIs such as TSA and romidepsin cause marked changes in the expression of KATP channel subunits. This defines a role for HDACs in determining which KATP channel subunits are expressed in cardiomyocytes but not pancreatic β-cells. Moreover, the data demonstrate a key role for cholesterol homeostasis in regulating this process. Taken together, these novel observations could lead to new strategies to modulate sarcolemmal KATP channel structure and function in the heart where it protects against stress and ischemia (Flagg et al., 2010). Moreover, our observations may explain the ST segment morphological changes that are associated with HDI therapy in cancer patients.
Conflict of Interest Statement
The authors declare that the research was conducted in the absence of any commercial or financial relationships that could be construed as a potential conflict of interest.
Acknowledgments
The authors thank the Biomedical Instrumentation Center at USUHS for providing advanced microscope technology and support for imaging experiments. This work was supported by awards from the American Heart Association Scientist Development Grant (11SDG7210070 to TF) and the Department of Defense (R0702O to TF).
Disclaimer
The views expressed are those of the authors and do not reflect the official policy or position of the Uniformed Services University of the Health Sciences, the Department of the Defense, or the United States government.
References
Ashfield, R., and Ashcroft, S. J. (1998). Cloning of the promoters for the beta-cell ATP-sensitive K-channel subunits Kir6.2 and SUR1. Diabetes 47, 1274–1280. doi: 10.2337/diabetes.47.8.1274
Berry, J. M., Cao, D. J., Rothermel, B. A., and Hill, J. A. (2008). Histone deacetylase inhibition in the treatment of heart disease. Expert Opin. Drug Saf. 7, 53–67. doi: 10.1517/14740338.7.1.53
Billman, G. E. (2008). The cardiac sarcolemmal ATP-sensitive potassium channel as a novel target for anti-arrhythmic therapy. Pharmacol. Ther. 120, 54–70. doi: 10.1016/j.pharmthera.2008.07.004
Bittman, R., and Fischkoff, S. A. (1972). Fluorescence studies of the binding of the polyene antibiotics filipin 3, amphotericin B, nystatin, and lagosin to cholesterol. Proc. Natl. Acad. Sci. U.S.A. 69, 3795–3799. doi: 10.1073/pnas.69.12.3795
Cao, D. J., Wang, Z. V., Battiprolu, P. K., Jiang, N., Morales, C. R., Kong, Y., et al. (2011). Histone deacetylase (HDAC) inhibitors attenuate cardiac hypertrophy by suppressing autophagy. Proc. Natl. Acad. Sci. U.S.A. 108, 4123–4128. doi: 10.1073/pnas.1015081108
Cardinale, J. P., Sriramula, S., Pariaut, R., Guggilam, A., Mariappan, N., Elks, C. M., et al. (2010). HDAC inhibition attenuates inflammatory, hypertrophic, and hypertensive responses in spontaneously hypertensive rats. Hypertension 56, 437–444. doi: 10.1161/HYPERTENSIONAHA.110.154567
Chang, J. T., and Nevins, J. R. (2006). GATHER: a systems approach to interpreting genomic signatures. Bioinformatics 22, 2926–2933. doi: 10.1093/bioinformatics/btl483
Chutkow, W. A., Makielski, J. C., Nelson, D. J., Burant, C. F., and Fan, Z. (1999). Alternative splicing of sur2 Exon 17 regulates nucleotide sensitivity of the ATP-sensitive potassium channel. J. Biol. Chem. 274, 13656–13665. doi: 10.1074/jbc.274.19.13656
Claycomb, W. C., Lanson, N. A. Jr. Stallworth, B. S., Egeland, D. B., Delcarpio, J. B., Bahinski, A., et al. (1998). HL-1 cells: a cardiac muscle cell line that contracts and retains phenotypic characteristics of the adult cardiomyocyte. Proc. Natl. Acad. Sci. U.S.A. 95, 2979–2984. doi: 10.1073/pnas.95.6.2979
Coiffier, B., Pro, B., Prince, H. M., Foss, F., Sokol, L., Greenwood, M., et al. (2012). Results From a pivotal, open-label, phase II study of romidepsin in relapsed or refractory peripheral T-cell lymphoma after prior systemic therapy. J. Clin. Oncol. 30, 631–636. doi: 10.1200/JCO.2011.37.4223
Csonka, C., Kupai, K., Bencsik, P., Görbe, A., Pálóczi, J., Zvara, Á., et al. (2014). Cholesterol-enriched diet inhibits cardioprotection by ATP-sensitive K+ channel activators cromakalim and diazoxide. Am. J. Physiol. Heart. Circ. Physiol. 306, H405–H413. doi: 10.1152/ajpheart.00257.2013
Falkenberg, K. J., and Johnstone, R. W. (2014). Histone deacetylases and their inhibitors in cancer, neurological diseases and immune disorders. Nat. Rev. Drug Discov. 13, 673–691. doi: 10.1038/nrd4360
Fatima, N., Schooley, J. F. Jr. Claycomb, W. C., and Flagg, T. P. (2012). Promoter DNA methylation regulates murine SUR1 (Abcc8) and SUR2 (Abcc9) expression in HL-1 cardiomyocytes. PLoS ONE 7:e41533. doi: 10.1371/journal.pone.0041533
Flagg, T. P., Enkvetchakul, D., Koster, J. C., and Nichols, C. G. (2010). Muscle KATP channels: recent insights to energy sensing and myoprotection. Physiol. Rev. 90, 799–829. doi: 10.1152/physrev.00027.2009
Flagg, T. P., Kurata, H. T., Masia, R., Caputa, G., Magnuson, M. A., Lefer, D. J., et al. (2008). Differential structure of atrial and ventricular KATP: atrial KATP channels require SUR1. Circ. Res. 103, 1458–1465. doi: 10.1161/CIRCRESAHA.108.178186
Flagg, T. P., Patton, B., Masia, R., Mansfield, C., Lopatin, A. N., Yamada, K. A., et al. (2007). Arrhythmia susceptibility and premature death in transgenic mice overexpressing both SUR1 and Kir6.2[{Delta}N30,K185Q] in the heart. Am. J. Physiol. Heart Circ. Physiol. 293, H836–H845. doi: 10.1152/ajpheart.00011.2007
Glukhov, A. V., Flagg, T. P., Fedorov, V. V., Efimov, I. R., and Nichols, C. G. (2010). Differential K(ATP) channel pharmacology in intact mouse heart. J. Mol. Cell Cardiol. 48, 152–160. doi: 10.1016/j.yjmcc.2009.08.026
Hernández-Sánchez, C., Ito, Y., Ferrer, J., Reitman, M., and LeRoith, D. (1999). Characterization of the Mouse Sulfonylurea Receptor 1áPromoter and its regulation. J. Biol. Chem. 274, 18261–18270. doi: 10.1074/jbc.274.26.18261
Inagaki, N., Gonoi, T., Clement, J. P., Namba, N., Inazawa, J., Gonzalez, G., et al. (1995b). Reconstitution of I-Katp - an inward rectifier subunit plus the sulfonylurea receptor. Science 270, 1166–1170. doi: 10.1126/science.270.5239.1166
Inagaki, N., Gonoi, T., Clement, J. P., Wang, C. Z., Aguilar-Bryan, L., Bryan, J., et al. (1996). A family of sulfonylurea receptors determines the pharmacological properties of ATP-sensitive K+ channels. Neuron 16, 1011–1017. doi: 10.1016/S0896-6273(00)80124-5
Inagaki, N., Inazawa, J., and Seino, S. (1995a). cDNA sequence, gene structure, and chromosomal localization of the human ATP-sensitive potassium channel, uKATP-1, gene (KCNJ8). Genomics 30, 102–104. doi: 10.1006/geno.1995.0018
Jeon, T. I., and Osborne, T. F. (2012). SREBPs: metabolic integrators in physiology and metabolism. Trends Endocrinol. Metab. 23, 65–72. doi: 10.1016/j.tem.2011.10.004
Kee, H. J., Sohn, I. S., Nam, K. I., Park, J. E., Qian, Y. R., Yin, Z., et al. (2006). Inhibition of Histone Deacetylation blocks cardiac hypertrophy induced by angiotensin ii infusion and aortic banding. Circulation 113, 51–59. doi: 10.1161/CIRCULATIONAHA.105.559724
Kelly, W. K., O'Connor, O. A., Krug, L. M., Chiao, J. H., Heaney, M., Curley, T., et al. (2005). Phase I study of an oral histone deacetylase inhibitor, suberoylanilide hydroxamic acid, in patients with advanced cancer. J. Clin. Oncol. 23, 3923–3931. doi: 10.1200/JCO.2005.14.167
Kim, J. W., Seghers, V., Cho, J. H., Kang, Y., Kim, S., Ryu, Y., et al. (2002). Transactivation of the mouse sulfonylurea receptor I gene by BETA2/NeuroD. Mol. Endocrinol. 16, 1097–1107. doi: 10.1210/mend.16.5.0934
Kim, S.-J., Lee, B.-H., Lee, Y.-S., and Kang, K.-S. (2007). Defective cholesterol traffic and neuronal differentiation in neural stem cells of Niemann–Pick type C disease improved by valproic acid, a histone deacetylase inhibitor. Biochem. Biophys. Res. Commun. 360, 593–599. doi: 10.1016/j.bbrc.2007.06.116
Kingaby, R. O., Lab, M. J., Cole, A. W. G., and Palmer, T. N. (1986). Relation between monophasic action potential duration, ST segment elevation, and regional myocardial blood flow after coronary occlusion in the pig. Cardiovasc. Res. 20, 740–751. doi: 10.1093/cvr/20.10.740
Knopp, A., Thierfelder, S., Koopmann, R., Biskup, C., Böhle, T., and Benndorf, K. (1999). Anoxia generates rapid and massive opening of KATP channels in ventricular cardiac myocytes. Cardiovasc. Res. 41, 629–640. doi: 10.1016/S0008-6363(98)00238-7
Kong, Y., Tannous, P., Lu, G., Berenji, K., Rothermel, B. A., Olson, E. N., et al. (2006). Suppression of Class I and II histone deacetylases blunts pressure-overload cardiac hypertrophy. Circulation 113, 2579–2588. doi: 10.1161/CIRCULATIONAHA.106.625467
Kouzarides, T. (2007). Chromatin modifications and their function. Cell 128, 693–705. doi: 10.1016/j.cell.2007.02.005
Kubota, I., Yamaki, M., Shibata, T., Ikeno, E., Hosoya, Y., and Tomoike, H. (1993). Role of ATP-sensitive K+ channel on ECG ST segment elevation during a bout of myocardial ischemia. A study on epicardial mapping in dogs. Circulation 88, 1845–1851. doi: 10.1161/01.CIR.88.4.1845
Lee, H.-Z., Kwitkowski, V. E., Del Valle, P. L., Ricci, M. S., Saber, H., Habtemariam, B. A., et al. (2015). FDA approval: belinostat for the treatment of patients with relapsed or refractory peripheral T-cell lymphoma. Clin. Cancer Res. 21, 2666–2670. doi: 10.1158/1078-0432.CCR-14-3119
Leventhal, A. R., Chen, W., Tall, A. R., and Tabas, I. (2001). Acid Sphingomyelinase-deficient Macrophages have defective cholesterol trafficking and efflux. J. Biol. Chem. 276, 44976–44983. doi: 10.1074/jbc.M106455200
Li, R. A., Leppo, M., Miki, T., Seino, S., and Marbán, E. (2000). Molecular basis of electrocardiographic ST-segment elevation. Circ. Res. 87, 837–839. doi: 10.1161/01.RES.87.10.837
Liu, F., Levin, M. D., Petrenko, N. B., Lu, M. M., Wang, T., Yuan, L. J., et al. (2008). Histone-deacetylase inhibition reverses atrial arrhythmia inducibility and fibrosis in cardiac hypertrophy independent of angiotensin. J. Mol. Cell. Cardiol. 45, 715–723. doi: 10.1016/j.yjmcc.2008.08.015
Lynch, D. R. Jr. Washam, J. B., and Newby, L. K. (2012). QT interval prolongation and torsades de pointes in a patient undergoing treatment with vorinostat: a case report and review of the literature. Cardiol. J. 19, 434–438. doi: 10.5603/CJ.2012.0078
Ma, X., Ezzeldin, H. H., and Diasio, R. B. (2009). Histone deacetylase inhibitors: current status and overview of recent clinical trials. Drugs 69, 1911–1934. doi: 10.2165/11315680-000000000-00000
Mann, B. S., Johnson, J. R., Cohen, M. H., Justice, R., and Pazdur, R. (2007). FDA approval summary: vorinostat for treatment of advanced primary cutaneous T-Cell lymphoma. Oncologist 12, 1247–1252. doi: 10.1634/theoncologist.12-10-1247
McKinsey, T. A. (2012). Therapeutic Potential for HDAC Inhibitors in the Heart. Annu. Rev. Pharmacol. Toxicol. 52, 303–319. doi: 10.1146/annurev-pharmtox-010611-134712
Meaney, S. (2014). Epigenetic regulation of cholesterol homeostasis. Front. Genet. 5:311. doi: 10.3389/fgene.2014.00311
Nichols, C. G., Ripoll, C., and Lederer, W. J. (1991). ATP-sensitive potassium channel modulation of the guinea pig ventricular action potential and contraction. Circ. Res. 68, 280–287. doi: 10.1161/01.RES.68.1.280
Noonan, A. M., Eisch, R. A., Liewehr, D. J., Sissung, T. M., Venzon, D. J., Flagg, T. P., et al. (2013). Electrocardiographic studies of romidepsin demonstrate its safety and identify a potential role for KATP channel. Clin. Cancer Res. 19, 3095–3104. doi: 10.1158/1078-0432.CCR-13-0109
Nunes, M. J., Moutinho, M., Gama, M. J., Rodrigues, C. M., and Rodrigues, E. (2013). Histone deacetylase inhibition decreases cholesterol levels in neuronal cells by modulating key genes in cholesterol synthesis, uptake and efflux. PLoS ONE 8:e53394. doi: 10.1371/journal.pone.0053394
Ory, D. S. (2004). The niemann-pick disease genes: regulators of cellular cholesterol homeostasis. Trends Cardiovasc. Med. 14, 66–72. doi: 10.1016/j.tcm.2003.12.003
Park, H. J., Georgescu, S. P., Du, C., Madias, C., Aronovitz, M. J., Welzig, C. M., et al. (2008). Parasympathetic response in chick myocytes and mouse heart is controlled by SREBP. J. Clin. Invest. 118, 259–271. doi: 10.1172/JCI32011
Philip-Couderc, P., Tavares, N. I., Roatti, A., Lerch, R., Montessuit, C., and Baertschi, A. J. (2008). Forkhead transcription factors coordinate expression of myocardial katp channel subunits and energy metabolism. Circ. Res. 102, e20–e35. doi: 10.1161/CIRCRESAHA.107.166744
Piekarz, R. L., Frye, A. R., Wright, J. J., Steinberg, S. M., Liewehr, D. J., Rosing, D. R., et al. (2006). Cardiac studies in patients treated with depsipeptide, FK228, in a phase II trial for T-cell lymphoma. Clin. Cancer Res. 12, 3762–3773. doi: 10.1158/1078-0432.CCR-05-2095
Piekarz, R. L., Frye, R., Prince, H. M., Kirschbaum, M. H., Zain, J., Allen, S. L., et al. (2011). Phase 2 trial of romidepsin in patients with peripheral T-cell lymphoma. Blood 117, 5827–5834. doi: 10.1182/blood-2010-10-312603
Pipalia, N. H., Cosner, C. C., Huang, A., Chartterjee, A., Bourbon, P., Farley, N., et al. (2011). Histone deacetylase inhibitor treatment dramatically reduces cholesterol accumulation in Niemann-Pick C1 mutant human fibroblasts. Proc. Natl. Acad. Sci. U.S.A. 108, 5620–5625. doi: 10.1073/pnas.1014890108
Richardson, P. G., Laubach, J. P., Lonial, S., Moreau, P., Yoon, S.-S., Hungria, V. T., et al. (2015). Panobinostat: a novel pan-deacetylase inhibitor for the treatment of relapsed or relapsed and refractory multiple myeloma. Expert Rev. Anticancer Ther. 15, 737–748. doi: 10.1586/14737140.2015.1047770
Sandor, V., Bakke, S., Robey, R. W., Kang, M. H., Blagosklonny, M. V., Bender, J., et al. (2002). Phase I trial of the histone deacetylase inhibitor, depsipeptide (FR901228, NSC 630176), in patients with refractory neoplasms. Clin. Cancer Res. 8, 718–728.
Shah, M. H., Binkley, P., Chan, K., Xiao, J., Arbogast, D., Collamore, M., et al. (2006). Cardiotoxicity of histone deacetylase inhibitor depsipeptide in patients with metastatic neuroendocrine tumors. Clin. Cancer Res. 12, 3997–4003. doi: 10.1158/1078-0432.CCR-05-2689
Stadler, W. M., Margolin, K., Ferber, S., McCulloch, W., and Thompson, J. A. (2006). A Phase II study of depsipeptide in refractory metastatic renal cell cancer. Clin. Genitourin. Cancer 5, 57–60. doi: 10.3816/CGC.2006.n.018
Steele, N. L., Plumb, J. A., Vidal, L., Tjørnelund, J., Knoblauch, P., Rasmussen, A., et al. (2008). A Phase 1 pharmacokinetic and pharmacodynamic study of the histone deacetylase inhibitor belinostat in patients with advanced solid tumors. Clin. Cancer Res. 14, 804–810. doi: 10.1158/1078-0432.CCR-07-1786
Suzuki, M., Sasaki, N., Miki, T., Sakamoto, N., Ohmoto-Sekine, Y., Tamagawa, M., et al. (2002). Role of sarcolemmal K(ATP) channels in cardioprotection against ischemia/reperfusion injury in mice. J. Clin. Invest. 109, 509–516. doi: 10.1172/JCI0214270
Weiss, J. N., Venkatesh, N., and Lamp, S. T. (1992). ATP-sensitive K+ channels and cellular K+ loss in hypoxic and ischaemic mammalian ventricle. J. Physiol. 447, 649–673. doi: 10.1113/jphysiol.1992.sp019022
Xie, M., Kong, Y., Tan, W., May, H., Battiprolu, P. K., Pedrozo, Z., et al. (2014). Histone deacetylase inhibition blunts ischemia/reperfusion injury by inducing cardiomyocyte autophagy. Circulation 129, 1139–1151. doi: 10.1161/CIRCULATIONAHA.113.002416
Xu, Q., Lin, X., Andrews, L., Patel, D., Lampe, P. D., and Veenstra, R. D. (2013). Histone deacetylase inhibition reduces cardiac connexin43 expression and gap junction communication. Front. Pharmacol. 4:44. doi: 10.3389/fphar.2013.00044
Yan, G. X., and Antzelevitch, C. (1999). Cellular basis for the Brugada syndrome and other mechanisms of arrhythmogenesis associated with ST-segment elevation. Circulation 100, 1660–1666. doi: 10.1161/01.CIR.100.15.1660
Keywords: epigenetics, Abcc8, Abcc9, romidepsin, cholesterol, SREBP
Citation: Fatima N, Cohen DC, Sukumar G, Sissung TM, Schooley JF Jr., Haigney MC, Claycomb WC, Cox RT, Dalgard CL, Bates SE and Flagg TP (2015) Histone deacetylase inhibitors modulate KATP subunit transcription in HL-1 cardiomyocytes through effects on cholesterol homeostasis. Front. Pharmacol. 6:168. doi: 10.3389/fphar.2015.00168
Received: 11 June 2015; Accepted: 27 July 2015;
Published: 13 August 2015.
Edited by:
Domenico Tricarico, University of Bari, ItalyReviewed by:
Hiroshi Hibino, Niigata University, JapanSaikat Chakraborty, Stony Brook Medicine, USA
Copyright © 2015 Fatima, Cohen, Sukumar, Sissung, Schooley, Haigney, Claycomb, Cox, Dalgard, Bates and Flagg. This is an open-access article distributed under the terms of the Creative Commons Attribution License (CC BY). The use, distribution or reproduction in other forums is permitted, provided the original author(s) or licensor are credited and that the original publication in this journal is cited, in accordance with accepted academic practice. No use, distribution or reproduction is permitted which does not comply with these terms.
*Correspondence: Thomas P. Flagg, Department of Anatomy, Physiology and Genetics, F. Edward Hebert School of Medicine, Uniformed Services University of the Health Sciences, 4301 Jones Bridge Rd., Rm. C-2114, Bethesda, MD 20814, USA,dGhvbWFzLmZsYWdnQHVzdWhzLmVkdQ==