- CNRS, Institut des Neurosciences Cellulaires et Intégratives, UPR 3212, Strasbourg, France
G protein-coupled receptors (GPCRs) modulate most physiological functions but are also critically involved in numerous pathological states. Approximately a third of marketed drugs target GPCRs, which places this family of receptors in the main arena of pharmacological pre-clinical and clinical research. The complexity of GPCR function demands comprehensive appraisal in native environment to collect in-depth knowledge of receptor physiopathological roles and assess the potential of therapeutic molecules. Identifying neurons expressing endogenous GPCRs is therefore essential to locate them within functional circuits whereas GPCR visualization with subcellular resolution is required to get insight into agonist-induced trafficking. Both remain frequently poorly investigated because direct visualization of endogenous receptors is often hampered by the lack of appropriate tools. Also, monitoring intracellular trafficking requires real-time visualization to gather in-depth knowledge. In this context, knock-in mice expressing a fluorescent protein or a fluorescent version of a GPCR under the control of the endogenous promoter not only help to decipher neuroanatomical circuits but also enable real-time monitoring with subcellular resolution thus providing invaluable information on their trafficking in response to a physiological or a pharmacological challenge. This review will present the animal models and discuss their contribution to the understanding of the physiopathological role of GPCRs. We will also address the drawbacks associated with this methodological approach and browse future directions.
Introduction
G protein-coupled-receptors (GPCRs) are proteins composed of seven transmembrane alpha helices with an extracellular N-terminus and an intracellular C-terminus (Rosenbaum et al., 2009). They represent one of the largest gene families in mammals and humans (Lagerström and Schiöth, 2008, and references therein). GPCRs can respond to various stimuli such as photons, ions, lipids, peptides, odorants, nucleotides, hormones, or neurotransmitters (Congreve et al., 2014). There are five human GPCR families: Rhodopsin, Secretin, Adhesion, Glutamate, and Frizzled/Taste2 with the rhodopsin receptor family being the largest. More than half of the 800 human GPCRs are classified as chemosensory taste or olfactory receptors (Lagerström and Schiöth, 2008; Heng et al., 2013). The remaining human GPCRs -roughly 370- may be involved in pathophysiological processes and are therefore potentially drugable targets. Indeed, metabolic, inflammatory, infectious or neurodegenerative diseases as well as cancer all involve a plethora of GPCRs (Heng et al., 2013). As many GPCRs belong to neuromodulatory systems (van den Pol, 2012), a large number of them are targeted by drugs in the context of nervous system disorders such as pain, drug addiction, anxiety, depression, sleep disorders, and neuroendocrine deregulation (Heng et al., 2013). Altogether, GPCRs represent the targets of about one third of marketed drugs (Overington et al., 2006).
Understanding the roles of GPCRs requires both in depth small scale investigation and overview. Indeed, GPCR expression, function, modulation, and trafficking properties remain difficult to fathom and reflect the complex, highly regulated pathways in which they are involved. The study of GPCRs in physiology and disease therefore requires integrative and functional systems. This is especially true when considering the central nervous system (CNS) where neuronal networks are complex and intermingled. It is therefore of utmost importance to identify and delineate cells that express the GPCR of interest. In the majority of studies, mapping GPCR expression was overcast by poor antibody specificity. The measure of this limitation was only fully appreciated when genetically modified mice which were deficient for the GPCR of interest became available, emphasizing the insufficient specificity of the commonly used antibodies, thereby prompting the search for new technologies to monitor receptor trafficking, decipher activated intracellular signaling cascades or investigate functional outcomes of GPCR activation in integrated systems, and particularly in neuronal networks (Marder, 2012). Among the options which were being explored, fluorescent proteins (FPs) isolated from natural organisms attracted special interest as they appeared to be very promising tools to achieve these goals. There are many advantages to using fluorescent molecular tags; the inherent fluorescence is directly visible, chemically resistant to fixation and can be used in time-course studies in living cells for tracking receptor trafficking events (Kallal and Benovic, 2000).
The Green FP (GFP) was the first FP used in biology. This protein is composed of 238 amino acids (roughly 27 kDa) and was isolated from the jellyfish Aequorea victoria (Shimomura et al., 1962, for review see Tsien, 1998). A mutant form of GFP called enhanced GFP (eGFP) was later generated, with improved quantum yield efficiency and higher solubility, making eGFP a popular reporter molecule (Cormack et al., 1997). The additional mutants that were created offer a large palette of fluorescence, ranging from violet to far red, thus opening new perspectives, including the possibility of co-expressing two or more FP in the same cell, whereby protein interactions could be investigated (Heim and Tsien, 1996). Likewise, this can be achieved by simultaneously expressing eGFP and mcherry, a stable monomeric mutant derived from the red fluorescent protein (RFP) DsRed, the latter was isolated from the coral Discosoma sp. (Campbell et al., 2002; Shaner et al., 2004). Additional variants derived from the GFP or DsRed were also generated and possess fast maturation, improved pH stability and photostability (reviewed in Shaner et al., 2007; Subach et al., 2009). The development of these FPs has been paralleled by technological advances in the field of live cell imaging that have brought high quality approaches for analysis of biological processes in a time- and space-dependent manner (Nienhaus and Nienhaus, 2014).
Validation of drug targets and pharmacological mechanisms cannot be achieved without in vivo preclinical studies for which mouse models provide a mammalian background and genetic tools of great value (Doyle et al., 2012; Bradley et al., 2014). In order to address GPCR function in vivo, tracking endogenous receptors with FPs therefore represents indisputable added value. In the following sections, we will review and comment on the use of FPs that has helped to shed light on endogenous GPCR function in vivo.
In Vivo Expression of FP Under GPCR Promoter
From Transgenic to Knock-in Mouse Lines
Transgenic mouse lines expressing FPs under the control of promoters for a GPCR or an endogenous peptide were created. A number of reporter mice generated using bacterial artificial chromosomes (BACs) were part of a project called gene expression nervous system atlas (GENSAT) http://www.gensat.org/index.html (Gong et al., 2003) that produced an important set of data relative to gene expression which could be used for deciphering the developmental implications and network dynamics of selected genes of interest. On the account that specific CNS genes are most often expressed in a particular cell population or anatomically defined structure, tandem dimer Tomato (td-Tomato), a RFP, or eGFP-labeling of these cells renders analysis of the anatomical, physiological and biomolecular properties of a chosen subtype of neurons accessible. Overall, transgenic reporter mouse lines have proven to be extremely useful for the precise mapping of GPCR and endogenous ligands expression in the nervous system, and are suitable for analysis of cell populations (Heintz, 2001).
The shortcomings of the transgenic mouse models are, however, manifold (Haruyama et al., 2009). (1) Transgenic expression results in overexpression compared to wild type animals. (2) Low efficiency of transmission to offspring may be caused by mosaic expression of the transgene in founder animals. Indeed, high copy number insertion of transgenes is more vulnerable to epigenetic silencing, which reduces the transgene expression level in successive generations. (3) Expression in unexpected tissues or timeframes may result from transgene insertion in genomic regions containing an endogenous promoter or enhancer. (4) Silencing or ectopic expression can be caused by positional effects. Transgene insertion can take place into transcriptionally inactive regions of the genome, or can be affected by neighboring repressor sites. Transgene insertion being, in essence, random, the possibility of disrupting the normal genome is very high. As a consequence, the erratic nature of the transgene insertion may result in unpredicted and/or detrimental phenotypes and off-target effects. As an example, many groups used BAC transgenic mice expressing eGFP driven by the promoter for either D1 or D2 receptors, the dopamine receptor 1 or 2, respectively (Lee et al., 2006; Bertran-Gonzalez et al., 2008; Valjent et al., 2009; Tian et al., 2010; Kramer et al., 2011; Chan et al., 2012). Mainly, work published using these two BAC transgenic mice successfully identified neurons expressing dopamine receptors and delineated dopaminergic connectivity in the CNS. However, Kramer et al. (2011) brought evidence of molecular and behavioral alterations in Drd2-eGFP BAC transgenic mice comprising novel environment hyperactivity, reduced locomotor response to cocaine, and D2 receptor agonist hypersensitivity. These effects were presumably due to unfortunate insertion of the BAC, which caused receptor overexpression (Kramer et al., 2011).
Knock-in Mice: Toward More Specific Models
To overcome the limitations associated with the use of transgenic mice, efforts were made to generate knock-in animals in which a FP is introduced at the locus of interest by homologous recombination. Several strategies are used (see Figure 1). Models in which an FP is expressed either under the control of an endogenous GPCR promoter are valuable and reliable tools for localization and characterization of cell population which express the GPCR of interest. However, such strategies present a significant drawback since the GPCR is non-functional following partial or total replacement of its coding sequence by the FP coding one. The FP is thus expressed in appropriate cells, but the precise subcellular localization and function of the receptor cannot be examined and the final outcome, in the case of homozygous animals, is the absence of the functional GPCR, equivalent to a knock-out phenotype. This limitation can be circumvented by the introduction of an internal ribosomal entry site (IRES) sequence, whereby expression of the endogenous GPCR is maintained and the chosen FP is expressed under control of the endogenous promoter.
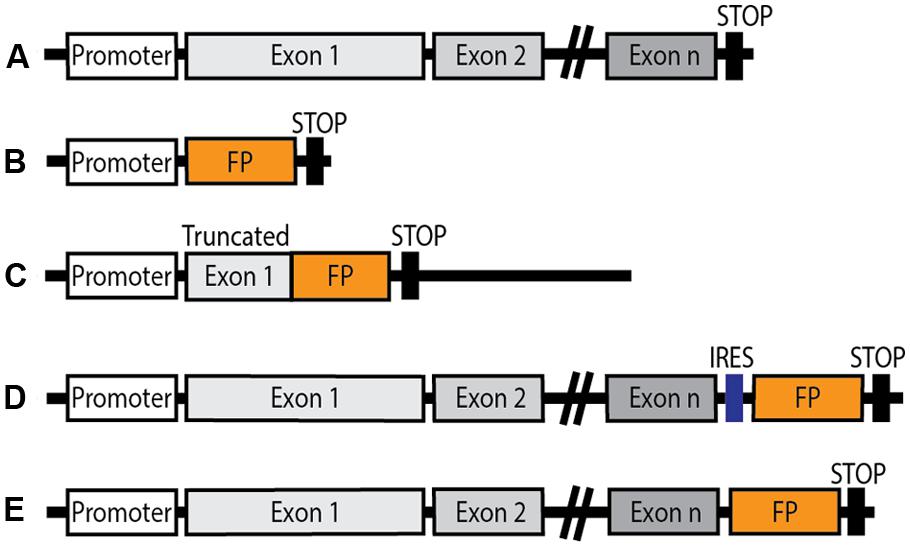
FIGURE 1. Schematic diagram of genetic constructions of knock-in mice expressing a fluorescent protein (FP) under the control of an endogenous GPCR promoter. (A) Endogenous GPCR gene layout. (B) Knock-in FP expressed under the control of the endogenous GPCR promoter: the endogenous GPCR gene is replaced by the FP coding sequence. (C) The FP coding sequence is knocked into the truncated gene coding for the native GPCR, resulting in genetic invalidation of the receptor. (D) Insertion of an internal ribosomal entry site (IRES) downstream of the endogenous GPCR gene, ahead of the FP coding sequence. Native GPCR expression is maintained, and the FP is also expressed under the control of the endogenous GPCR promoter. (E) The FP sequence is inserted in frame in place of the stop codon in the endogenous GPCR gene giving rise to a fluorescent fusion protein in which the FP is fused to the C-terminus of the functional GPCR in conditions of native expression.
Chemokine receptors
Jung et al. (2000) published the first knock-in mouse in which an FP was expressed under a GPCR promoter. The aim was to track cells which expressed the Fractalkin (CX3C) chemokine receptor CX3CR1, using a GFP knock-in strategy by replacing the first 390 bp of exon 2 of the CX3CR1 gene that encodes the receptor N-terminus by a eGFP-coding sequence, enabling direct identification of peripheral blood cells and brain microglia expressing CX3CR1 (see Table 1). In heterozygous animals, CX3CR1 expression remained detectable because these CX3CR1+/GFP heterozygous animals possess one allele for fluorescence visualization of cells expressing the GPCR of interest and one allele for expression of the functional receptor. Since CX3CR1 and its ligand Fractalkin play a role in immunological and inflammatory processes, this model was used to investigate microglia proliferation during early embryonic spinal cord invasion (Rigato et al., 2012) neuron-glia interactions in the context of nerve injury or neuroinflammation (Garcia et al., 2013) and in neurodegenerative diseases such as Alzheimer’s disease (Fuhrmann et al., 2010), or Parkinson’s disease (Virgone-Carlotta et al., 2013).
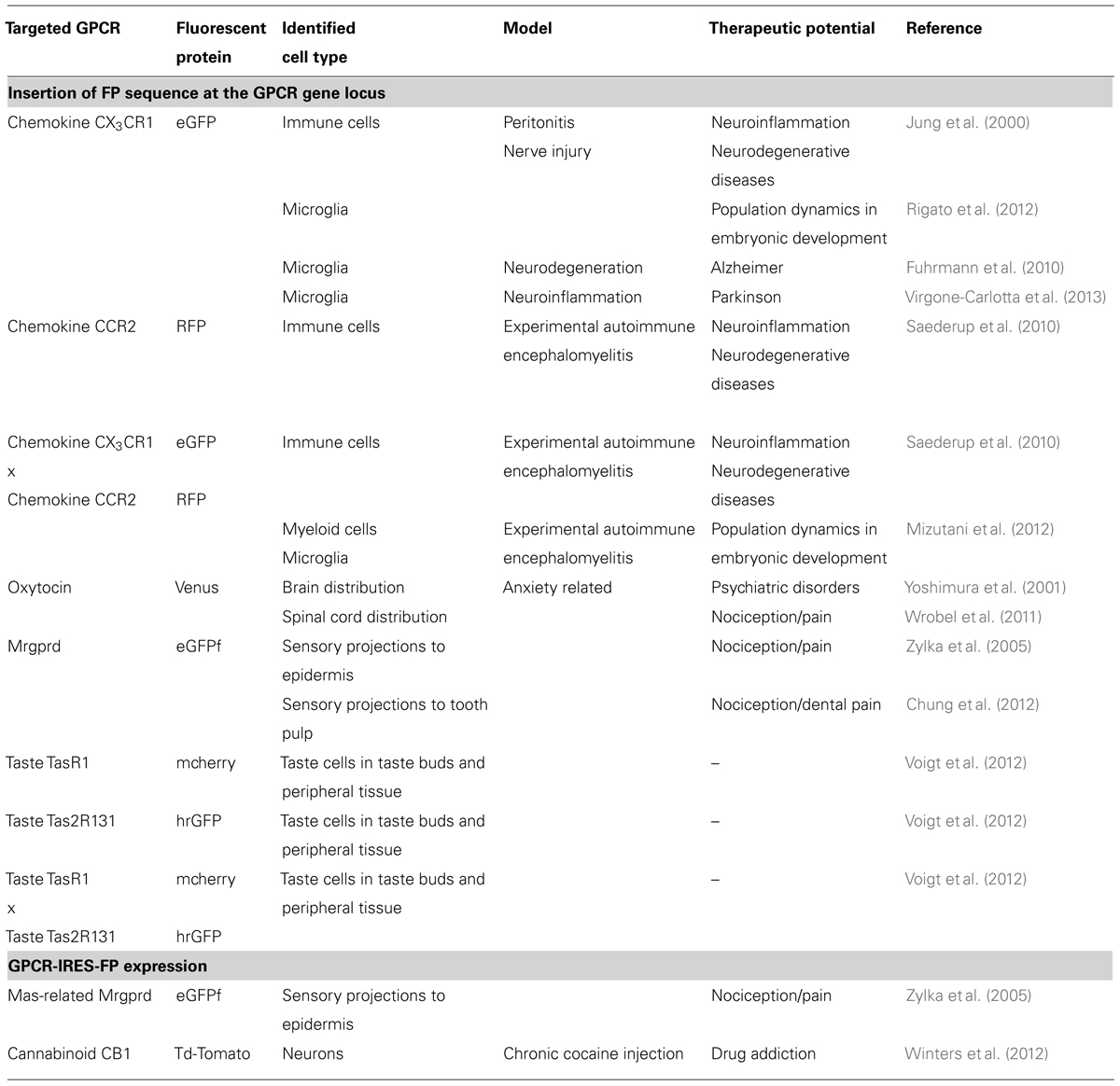
TABLE 1. Knock-in mice expressing fluorescent proteins under the control of G protein-coupled receptor (GPCR) endogenous promoters.
A follow-up to this knock-in mouse was published in 2010. In their paper, Saederup et al. (2010) designed a mouse with another single FP, RFP (a DsRed variant) replacing the first 279 base pairs of the open reading frame coding for the chemokine receptor type 2 (CCR2), and crossed the heterozygous CCR2+/RFP and homozygous CCR2RFP/RFP knock-in animals with the previously published CX3CR1GFP/GFP homozygous animals, in order to obtain heterozygous double knock-in animals CX3CR1+/GFPCCR2+/RFP. The two chemokine receptors are expressed by distinct monocyte populations, therefore the red and green FPs constitute an elegant “two-colored” mouse model which was ideally suited for immunological studies (see Table 1). Indeed, because the immune system is constituted of cells that circulate in blood and lymph vessels, mature cells do not constitute a solid organ and are not restricted by connective tissue, therefore immune cell tracking is essential. Both the double heterozygous knock-in animals and the first mouse line (CX3CR1+/GFP knock-in), were used to study and adequately quantify macrophage and monocyte population dynamics in a model of autoimmune tissue inflammation (experimental autoimmune encephalomyelitis), which recapitulates an animal model of multiple sclerosis (MS). In a subsequent study, the same group unveiled myeloid lineage and microglial chemokine receptor changes at embryonic stages 8.5–13.5, monitored CNS colonization by cells of interest, during development and in an MS model using adult mice (Mizutani et al., 2012). The knock-in models thus yielded exciting and fundamental results relative to the identification of cells expressing the designated GPCRs, and a fine description of cellular population changes in various disease paradigms.
Oxytocin receptors
Yoshida et al. (2009) engineered a mouse line in which a 5′ fragment of exon 3 of the oxytocin receptor (OTR) gene was replaced by a sequence coding for Venus FP, a yellow FP variant (Nagai et al., 2002). The recombined allele did not encode functional OTR but heterozygous animals retained radiolabelled oxytocin binding patterns through the intact allele, while enabling direct visualization of Venus in oxytocin expressing cells (Yoshida et al., 2009). Immunohistochemical analysis of brain sections from these animals revealed that there was a high expression of Venus (hence OTR) in monoaminergic areas of the brain in agreement with in situ hybridization (ISH) studies (Vaccari et al., 1998). However, the approach provided more sensitive detection of OTR expression by identifying additional areas and cells expressing Venus fluorescence among which serotoninergic ones. This study was the first to show evidence for interaction between oxytonergic and serotonergic systems in a pathway, which modulates anxiety. In a following study, these knock-in mice were used to map OTR expression in the spinal cord; shedding light on the modulatory role of oxytocinergic networks involved in spinal cord functions, such as nociception (Wrobel et al., 2011).
Taste receptors
Sensing of the chemical categories which are responsible for sweet, sour or umami taste is specifically encoded by GPCRs expressed on primary taste neurons (Liman et al., 2014). The taste receptor family 1 (Tas1r) belongs to class C GPCRs and function as obligatory heteromers, meaning that two GPCRs of different subtypes are associated and interact to form a functional entity. The taste receptor family 2 (Tas2r), on the other hand, are currently classified among class A GPCRs (Alexander, 2013).
In order to study the distribution of taste receptors in the mouse gustatory tissue, Voigt and collaborators engineered two knock-in mouse lines which they subsequently crossed in order to obtain double knock-in animals in which the open reading frame encoding the receptor was replaced by the sequence coding for the mcherry or humanized Renilla (hr)GFP under the control of Tas1r1 (umami taste receptor) or Tas2r131 (bitter taste receptor) promoters, respectively (Voigt et al., 2012). This approach permitted identification of cells expressing mcherry under the control of the Tas1r1 promoter in the lingual papillae, soft palate, fungiform and foliate papillae, confirming previous findings (Hoon et al., 1999; Stone et al., 2007) but also in extra-gustatory tissues (lung epithelium, testis, thymus) which had not been investigated before (Voigt et al., 2012). Expression of hrGFP under the control of Tas2r131 promoter was in accordance with previously findings describing taste receptor distributions (Behrens et al., 2007), showing abundant hrGFP expression in taste buds of the posterior tongue, vallate palate and foliate palate. In addition, it uncovered, for the first time, expression restricted to only half of the bitter sensor cells (Voigt et al., 2012). Double knock-in animals lacked both taste receptors, but expressed FPs in the targeted cells [verified by reverse transcription polymerase chain reaction (RT-PCR), ISH and immunohistochemistry]. This genetic labeling technique served for population distribution studies, which was until then unachievable, given the fact that Tasr expression is sparse in cells, and that the available antibodies lack specificity. The double knock-in animals yielded a valuable and detailed cartography of taste receptors in the mouse, and revealed that distinct chemosensory cell populations mediate specific and non-overlapping taste qualities.
Mas-related-G-protein coupled receptors
Mas-related-G-protein coupled receptor member D (Mrgprd) belongs to a GPCR family of approximately 50 members, related to Mas1 (oncogene-like MAS), called Mrgs. Mrgs are suspected to be involved in development, regulation and function of nociceptive neurons or nociceptors (Dong et al., 2001) and are expressed in a subset of nociceptors, which are small diameter primary sensory neurons in dorsal root ganglia (DRG) directly involved in processing nociceptive stimuli, especially itch (Liu et al., 2012).
Zylka et al. (2005) observed similar expression patterns of the eGFPf (a farnesylated form that anchors the FP to the cytoplasmic leaflet of the lipid bilayer) in nociceptors, and projections of the sensory neurons to the epidermis using knock-in mice in which the open reading frame coding for Mrgprd is replaced by the sequence encoding the eGFPf or knock-in animals in which the eGFPf sequence is inserted behind an IRES element downstream of the mouse Mrgprd gene (Zylka et al., 2005). This demonstrates that both strategies can be equally used for cellular mapping. In addition, similar projection profiles in the epidermis validated the eGFPf knock-in mouse for axonal tracing by comparison with the widely used human placental alkaline phosphatase tethered to the extracellular surface of the plasma membrane by a glycophosphatidylinositol linkage.
In a later study, the knock-in mouse model expressing eGFPf at the Mrgprd locus was used to identify non-peptidic nociceptive neurons of trigeminal ganglia innervating tooth pulp (Chung et al., 2012). This opens future application of this model to study the role and function of the targeted GPCR in dental pain.
Cannabinoid receptors
The endocannabinoid system plays roles in memory, appetite, stress and immune processes, as well as motivation and emotional responses and modulates the effects of some drugs of abuse (Pertwee, 2006; Tan et al., 2014). In the nucleus accumbens (NAc), a brain structure which has a crucial role in reward processing and a decisive influence on emotional and motivational responses, cannabinoid receptor 1 (CB1) expression is limited but nevertheless essential for cocaine-induced reward in mice (Marsicano and Lutz, 1999). In order to further identify and delineate the cellular and electrophysiological properties of CB1 receptor expressing cells in the NAc, Winters et al. (2012) designed a knock-in mouse line in which an IRES element ensures expression of both CB1 receptors and td-Tomato under the control of the CB1 promoter. Importantly, this mouse line still expressed functional CB1 receptors. Neurons expressing CB1 receptors were readily visualized in the NAc and their distribution was in accordance with previous data on CB1 receptor localization using ISH or immunohistochemistry (Mailleux and Vanderhaeghen, 1992; Tsou et al., 1997). This mouse line enabled to identify of cells and to explicitly demonstrate biochemical and signaling properties of a particular neuronal population of fast-spiking interneurons in the NAc which impacts on the NAc projections and connectivity. Results also revealed functional impact of cocaine on these neurons (Winters et al., 2012).
GPCR-FP Fusion for In Vivo Functional and Mapping Studies
Initial Validation of GPCR-FP Fusions in Heterologous Systems
Fusions between a GPCR and an FP as tools to monitor the GPCR subcellular localization and trafficking were first studied in heterologous systems. Two fusion options were considered: either the FP at the N-terminus or at the C-terminus. A vast majority of GPCRs do not have cleavable N-terminus signal sequences that target them to the plasma membrane. Introduction of a foreign sequence ahead of their N-terminus has been shown to disrupt surface addressing, and correct membrane targeting and insertion therefore requires introduction of an additional foreign signal sequence in front of the fusion construct (McDonald et al., 2007). If proper cell surface expression is indeed restored, introduction of such a signal sequence nonetheless strongly impacts on the relative ratio between surface expression and intracellular distribution by substantially increasing the amount of protein at the cell surface (Dunham and Hall, 2009, and references therein). Hence, such fusion proteins are not well suited to mimic the responses of endogenous GPCRs to agonist stimulation and were not used for in vivo studies.
Concerns have also been raised regarding in frame insertion of the FP at the C-terminus of the GPCR by substitution of the stop codon. The presence of a 27 kDa beta barrel at the intracellular extremity of the GPCR could indeed interfere with intracellular scaffold partners and modify signaling or internalization processes thus defeating the object when studying GPCR signaling properties. However, many studies performed in mammalian cells on a large number of GPCRs strongly suggest that addition of GFP at the C-terminus does not significantly affect subcellular distribution in the basal/unstimulated state, ligand binding or agonist-induced receptor phosphorylation and internalization, (for review Kallal and Benovic, 2000). McLean and Milligan (2000) expressed β1- and β2-adrenergic receptors fused to a C-terminal eGFP mutant in human embryonic kidney (HEK 293) cells. These authors concluded that the presence of the eGFP did not influence ligand binding but decreased the agonist-induced internalization kinetics without affecting the intracellular fate of the receptor. Trafficking of the fusion protein was qualitatively maintained, but was quantitatively slightly modified compared to native proteins. This study therefore supports the use of such fusions to monitor endogenous receptor subcellular localization. Similarly, the genetic construction encoding the delta opioid (DOP) receptor fused with eGFP protein at the C-terminus was expressed in transfected HEK 293 cells, and the fusion did not alter opioid ligand binding affinity or signaling (Scherrer et al., 2006). This construct was later successfully used to express a functional DOP-eGFP fusion in mice by knocking the modified sequence into the endogenous DOP receptor locus (Scherrer et al., 2006, see below).
In some cases, however, FP fusion at the GPCR C-terminus had deleterious effects. Defective targeting to the cell surface was reported for the melanocortin 2 receptor fused to the GFP in HEK 293 cells (Roy et al., 2007) and no recycling was observed for the muscarinic M4 receptor fused to a C-terminal red variant of GFP in neuroblastoma/glioma hybrid cells (NG108-15 cells; Madziva and Edwardson, 2001). In both cases, impairment was more likely to be due to gross overexpression rather than fusion of the FP to the C-terminus. High levels of expression of a GPCR in a non-native environment can indeed artificially elicit properties and interactions that would not occur in vivo. Moreover, cell lines used for heterologous expression may provide different intracellular machinery for complex protein folding or post-translational modifications compared to naturally producing cells. This represents an additional limitation to the study of GPCR functions and prompted to develop in vivo approaches.
From Transgenic to Knock-in Mouse Lines
Papay et al. (2004) reported a transgenic mouse model of a fluorescent tagged GPCR. The construct they described was composed of a 3.4 kb fragment of the mouse endogenous α1B adrenoceptor promoter, the human α1B adrenoceptor coding sequence with C-terminal fusion eGFP sequence. The resulting founder lines were characterized, and high expression levels were observed in all tissues that naturally express α1B adrenoceptors by fluorescence microscopy. Binding affinities and internalization profiles were similar to those of endogenous receptors. With this study, Papay et al. (2004) reported the first mouse model expressing a GPCR tagged with eGFP as a transgenic approach for in vivo GPCR localization studies. The generation of knock-in animals represented a further improvement by enabling for the first time to track down endogenous receptors, which has opened a new era for pharmacological research.
Knock-in Humanized Rhodopsin Fused With a Fluorescent Protein (hRho-eGFP)
Chan et al. (2004) mouse lines expressing human rhodopsin-eGFP were engineered using different knock-in strategies. All mouse lines showed decreased expression levels of the fusion protein relative to the endogenous mouse rhodopsin. Comparing the different homozygote mouse lines enabled to correlate the decrease in human rhodopsin–eGFP expression to the increased rate of retinal degeneration, providing a model of human diseases. More recently, using a human mutant rhodopsin allele [proline-to-histidine change at codon 23 (P23H) rhodopsin] which induces mislocalization and degradation of the human protein, the research group generated a knock-in mouse line which modeled a common cause of autosomal dominant retinitis pigmentosa (Price et al., 2011). In humans, mutation Q344X is responsible for a severe early onset form of retinitis pigmentosa. The Q344X mutation introduces a premature stop codon that prevents GFP expression in the human rhodopsin-eGFP construct. Knock-in animals expressing this mutant construct were used to monitor eGFP fluorescence recovery as an index of the frequency and timing of somatic mutations in the rhodopsin gene (Sandoval et al., 2014). These mouse lines provided substantial and valuable data concerning rhodopsin distribution in the retina (for references, also see Table 2), and were advantageously implemented for non-invasive measurement by illuminating the mouse retina in live animals with blue light (Wensel et al., 2005). They will provide a means to assess the impact of future gene-targeting treatment strategies for retinal degeneration (Gross et al., 2006; Sandoval et al., 2014).
Opioid Receptors
The opioid system modulates a wide range of physiological states, of which nociception, reward, mood, stress, neuroendocrine physiology, immunity, autonomic functions such as gastro-intestinal transit (Kieffer and Evans, 2009; Walwyn et al., 2010; Chu Sin Chung and Kieffer, 2013; Lutz and Kieffer, 2013). Opioid receptors are members of the class A GPCR family, mu (MOP), delta (DOP) and kappa (KOP) opioid receptors couple to inhibitory heterotrimeric inhibitory G protein, and have high sequence homology (Akil et al., 1998).
Mapping of receptor expression with neuronal resolution
Scherrer et al. (2006) generated a DOP-eGFP knock-in mouse line by homologous recombination in which the coding sequence for the DOP receptor fused to its C-terminus to the eGFP was inserted at the Oprd1 locus.
Delta opioid-eGFP knock-in mice proved very helpful to map DOP receptors in the nervous system and remedy the lack of highly specific antibodies (see Table 2). In the peripheral nervous system, DOP-eGFP receptors were detected in cell bodies of specific peripheral sensory neuronal populations which process sensory stimuli, namely mostly in large diameter myelinated (Neurofilament 200 positive), and in small diameter unmyelinated non-peptidergic (Isolectin B4 positive) neurons (Scherrer et al., 2009; Bardoni et al., 2014). The expression pattern of DOP-eGFP receptors was also reported in mechanosensory organs in the skin (Bardoni et al., 2014). Another study focused on the distribution of DOP-eGFP in enteric neurons with DOP-eGFP expression mainly in secretomotor neurons of the submucosal plexus of the digestive tract (Poole et al., 2011). The observed distribution reflects functional roles of DOP receptors in inhibition of intestinal motility and absorption.
In the CNS, DOP-eGFP mapping was performed in the brain and spinal cord (Erbs et al., 2014). Detailed DOP-eGFP expression was also reported in the hippocampus, where functional DOP-eGFP was found to be mainly expressed in GABAergic interneurons, mostly parvalbumin-positive ones (Erbs et al., 2012; Rezai et al., 2013). The DOP-eGFP knock-in mice also enabled to resolve the debate concerning the presence of DOP receptors in principal cells. The absence of colocalization with calbindin (Erbs et al., 2012) and presynaptic expression restricted to afferents to glutamatergic principal cells established that no functional DOP receptors are expressed under basal conditions in those cells (Rezai et al., 2012). These results are consistent with a modulation of principal cell activity in the hippocampus by DOP receptors, and therefore an impact of the receptors in learning and memory.
More recently, a knock-in mouse line expressing a MOP receptor fused with a RFP at the C-terminus, MOP-mcherry, was generated by Erbs et al. (2014). At the Oprm1 locus, mcherry cDNA was introduced into exon 4 of the MOP gene in frame and 5′ from the stop codon. This FP is monomeric and highly photostable, and the strong red signal of MOP-mcherry fusion protein enabled direct identification of neurons expressing MOP in the nervous system (Erbs et al., 2014). The authors compiled the DOP-eGFP and MOP-mcherry distributions in a neuroanatomical atlas available at http://mordor.ics-mci.fr
Several studies in heterologous systems or cell culture had suggested that MOP and DOP receptors may interact to form heteromers (van Rijn et al., 2010; Rozenfeld et al., 2012; Stockton and Devi, 2012) but their existence in vivo remains debated. Co-immunoprecipitation studies performed on tissue from spinal cord or DRGs also hinted at close physical proximity between the two receptors in these areas (Gomes et al., 2004; Xie et al., 2009). In addition, MOP-DOP heteromers had been detected in some brain areas using specific antibodies (Gupta et al., 2010). Recently, extensive mapping of MOP-DOP neuronal colocalization using double knock-in mice co-expressing DOP-eGFP and MOP-mcherry provided sound data to investigate MOP-DOP physical proximity and functional interactions. In the hippocampus, a brain area where the two receptors are highly co-expressed, co-immunoprecipitation experiments using antibodies raised against the FPs indeed confirmed physical proximity (Erbs et al., 2014). These animals will now be useful to address MOP-DOP specificities in ligand binding, signaling and trafficking as well as functional output and to investigate the potential of MOP-DOP heteromers as a novel therapeutic target.
In vivo trafficking, desensitization and behavioral output
The DOP-eGFP mouse line is the first example of the use of a knock-in line to study GPCR functions in vivo (Scherrer et al., 2006). DOP agonist-induced internalization was observed in vivo upon activation by the alkaloid [(+)-4-[(alphaR)-alpha-((2S,5R)-4-allyl-2,5- dimethyl-1-piperazinyl)-3-meth oxybenzyl]-N,N-diethylbenzamide] (SNC-80) and the endogenous peptide Met-enkephalin (Scherrer et al., 2006). The two agonists induce receptor internalization in heterologous systems with receptor phosphorylation as the first step of a cascade of events leading to termination of G protein dependent signaling, receptor removal from the cell membrane and trafficking to intracellular compartments (Ferguson et al., 1996; von Zastrow and Williams, 2012; Walther and Ferguson, 2013). DOP-eGFP mice revealed that these agonists also induce receptor phosphorylation, internalization via clathrin coated pits in vivo and degradation in the lysosomal compartment in the brain (Scherrer et al., 2006; Pradhan et al., 2009; Faget et al., 2012) and peripheral nervous system in the myenteric plexus (Poole et al., 2011) and DRGs (Scherrer et al., 2009). Moreover, these animals prove to be instrumental to decipher molecular mechanisms underlying receptor desensitization leading to a loss of responsiveness of the receptor upon stimulation by an agonist. Scherrer et al. (2006) were indeed able, for the first time, to establish the correlation between receptor trafficking in vivo and the behavioral response: namely that the receptor internalization induced by acute administration of the agonist SNC-80 was responsible for the observed locomotor desensitization. This paper was followed by additional studies exploring the consequences of receptor pharmacological stimulation in more detail, in particular the concept of biased agonism.
G protein-coupled receptors have a flexible and highly dynamic nature (Moreira, 2014) which enables a given ligand to show functional selectivity, that is, preferential activation of signal transduction pathways, otherwise termed biased agonism (Ostrom and Insel, 2004; Giguere et al., 2014; Kenakin, 2014). DOP-eGFP mice offer the possibility of addressing this concept in vivo and to link it to a functional response. DOP-eGFP mice were used to analyze the properties of two DOP receptor agonists possessing similar signaling potencies and efficacies but with different internalization profiles (Pradhan et al., 2009). SNC-80 and N,N-diethyl-4-(phenyl-piperidin-4-ylidenemethyl)-benzamide (AR-M100390), with high and low internalization properties respectively, were systemically administered to mice, and receptor trafficking was correlated to induced anti-allodynic effect in the context of inflammatory pain (Pradhan et al., 2009). As expected, acute SNC-80 administration resulted in receptor phosphorylation, decreased G protein coupling and receptor degradation in the lysosomal compartment, leading to desensitization with loss of anti-allodynic properties. On the other hand, acute injection of AR-M100390 did not result in receptor phosphorylation, did not reduce G protein coupling, did not induce receptor internalization or desensitization but retained analgesic properties. This study demonstrated that DOP receptor localization determines its function in vivo and highlights the importance of receptor tracking in order to extricate behavioral and cellular correlates of specific agonist properties (Pradhan et al., 2009).
In a following study, DOP-eGFP mice were used to assess the physiological impact of distinct signaling pathway recruitment and/or adaptive responses upon chronic administration of two DOP receptor agonists (Pradhan et al., 2010). Chronic administration of SNC-80, which has high internalization properties, led to marked receptor downregulation and degradation in SNC-80-tolerant animals. Receptor internalization prevented any additional activation through physical disappearance from the cell surface leading to general desensitization, as assessed by thermal and mechanical analgesia, locomotor activity and anxiety-related behavior. On the other hand, chronic administration of AR-M100390, with weak internalization properties, did not cause changes in DOP-eGFP localization and induced tolerance restricted to analgesia, with no effect on locomotor activity or anxiolytic responses. These data show that a selective internalization-independent tolerance was elicited and suggest the occurrence of adaptative mechanisms that are network dependent. These findings reinforce the importance of understanding agonist specific signaling underlying biased agonism and tolerance. Considering that drug design has focused on offering orthosteric or allosteric modulators of GPCRs (Bradley et al., 2014), research groups need to explore the downstream signaling cascades of these drugs in more detail in order to understand and target the molecular events which underlie their efficacy. This is an essential progress for the understanding of drug action and opens new possibilities for drug design.
Direct visualization of the receptor also permitted to decipher the functional role of delta receptors in neuronal networks and to understand the complex relation between behavior and receptor subcellular distribution. Of particular interest is the observation that DOP subcellular distribution is modified in two brain areas involved in the processing of information associated with emotional value or predicted outcome. The CA1 area of the hippocampus is known to operate as a coincidence detector that reflects association of the context with strong emotional stimuli of positive or aversive value (Duncan et al., 2012). Accordingly, increased c-Fos immunoreactivity revealed activation of this region in a drug-context association paradigm, and DOP-eGFP internalization in this area therefore suggested a modulatory role of the receptor in behavioral responses linked to context-induced withdrawal (Faget et al., 2012). Along the same line, persistent increase of DOP-eGFP expression at the cell surface of cholinergic interneurons was induced by conditioned training in the NAc shell, which is involved in decision making and predictive reward evaluation upon pavlovian conditioning (Bertran-Gonzalez et al., 2013; Laurent et al., 2014).
Finally, the knock-in strategy revealed that the DOP-eGFP internalization profile in response to endogenous opioid release is distinct from what is observed upon pharmacological stimulation (Faget et al., 2012). Indeed, only part of the receptor population present at the cell surface underwent internalization under physiological conditions. This observation further highlights the need to take into account the extent of changes that drug administration induces in receptor cellular distribution.
Methodological improvements
Interestingly, DOP-eGFP knock in mice also bring useful technical insight. During the process of acute brain slice preparation for electrophysiological recordings, DOP-eGFP revealed spontaneous receptor internalization (Rezai et al., 2013). This event was likely due to high glutamatergic activity in the hippocampus upon slicing that leads to exitoxicity. Direct visualization of the receptor therefore revealed a bias associated with previously unrecognized receptor trafficking that can now be addressed by initiating optimization of slice preparation conditions for electrophysiological recording (Rezai et al., 2013). This observation may be of particular relevance when addressing cellular responses elicited by drug application.
Concerns About the Use In Vivo of GPCR-FP Fusions for Functional Studies
Despite the undeniably wide advances which have been and will be brought by genetically engineered mice encoding fluorescent endogenous GPCRs, concerns were raised regarding the inherent consequences of genetic manipulation. The possibility that the observed localization does not entirely reflect the wild type receptor distribution appears irrelevant since both MOP-mcherry and DOP-eGFP receptor distributions in the brain are in full agreement with reports in mice and rats based on ligand binding (Kitchen et al., 1997; Slowe et al., 1999; Goody et al., 2002; Lesscher et al., 2003), GTPγS incorporation (Tempel and Zukin, 1987; Pradhan and Clarke, 2005) or mRNA detection [George et al., 1994; Mansour et al., 1995; Cahill et al., 2001; for a review see (LeMerrer et al., 2009)]. Also, in a more detailed study, DOP-eGFP expression in the hippocampus, mainly in parvalbumin-positive GABAergic interneurons (Erbs et al., 2012), was corroborated by ISH studies on DOP receptors (Stumm et al., 2004).
In the peripheral nervous system, despite previous reports suggesting SP-dependent trafficking of DOP receptors to the cell membrane (Guan et al., 2005), Scherrer et al. (2009) reported that DOP-eGFP almost never co-localized with substance P (SP) in peripheral sensory neurons (Scherrer et al., 2009), a finding that was debated by others (Wang et al., 2010). A more recent study addressed this discrepancy by comparing DOP-eGFP cellular distribution to that of the native DOP receptor using an ultrasensitive and specific ISH technique, which can detect single mRNA molecules (Bardoni et al., 2014). Patterns of DOP-eGFP distribution and Oprd1 mRNA expression were found to be very similar and detectable in the same neuronal populations, namely mostly in large diameter myelinated cells (Neurofilament 200 positive), and in small diameter unmyelinated non-peptidergic neurons (isolectin B4 positive; Bardoni et al., 2014). These data unambiguously confirm that the expression profile of the fluorescent constructs mimics the endogenous one and that fluorescent knock-in mice can be reliably used for mapping receptors in the central and peripheral nervous system.
Regarding functional aspects, there has been no evidence so far of any overt phenotypical or behavioral differences between the DOP receptor knock-in strain and wild type animals (Scherrer et al., 2006; Pradhan et al., 2009, 2010; Rezai et al., 2013), despite a twofold increase in mRNA and protein levels as well as increased G protein activation compared to wild type animals (Scherrer et al., 2006). However, the possibility that the subcellular distribution of the fluorescent fusion does not recapitulate that of the native untagged receptor is still debated. Indeed, high surface expression of DOP-eGFP is observed under basal conditions in several brain regions, particularly in the hippocampus (Scherrer et al., 2009; Erbs et al., 2012, 2014; Faget et al., 2012). This does not correlate with previous studies on wild type receptors using electron microscopy or fluorescent ligands that indicated a predominant intracellular localization under basal conditions and surface recruitment upon chronic morphine or chronic pain condition (Cahill et al., 2001; Morinville et al., 2004; Gendron et al., 2006; for review see Cahill et al., 2007; Gendron et al., 2014). Surface expression of DOP-eGFP, however, varies across CNS regions and neuronal type whereas high fluorescence is always visible within the cytoplasm (Erbs et al., 2014). Accordingly, high surface expression appears to be restricted to some neuronal types such as GABAergic interneurons in the hippocampus or large proprioceptors in DRGs (Scherrer et al., 2006; Erbs et al., 2014). In many areas where DOP receptors are highly expressed such as the striatum, the basal ganglia, the amygdala or the spinal cord, DOP-eGFP is not readily detected at the cell surface (Erbs et al., 2014) suggesting that DOP-eGFP intracellular localization is predominant in those neurons. Importantly, surface expression of DOP-eGFP can be augmented under physiological stimulation (Bertran-Gonzalez et al., 2013; Laurent et al., 2014; see above) or increased upon chronic morphine treatment as previously reported for wild type receptors (Erbs et al., unpublished data), strongly supporting that the fused FP does not impact on the native subcellular distribution of the receptor and that the latter can be modulated according to the physiological state or modified upon pharmacological treatment.
In the case of MOP-mcherry knock-in mice, the red fluorescent signal is stronger inside the cell than at the plasma membrane (Erbs et al., 2014). This distribution reflects actual receptor intracellular distribution, as evidenced by comparison with MOP-specific immunohistochemistry in heterozygous mice, which confirms that the fusion protein does not cause defective receptor localization or surface trafficking (Erbs et al., 2014). Importantly, MOP-mcherry retained unchanged receptor density as well as [D-Ala2, N-MePhe4, Gly-ol]-enkephalin (DAMGO) binding and efficacy and agonist-induced internalization compared to MOP. Moreover, behavioral effects of morphine in knock-in mice were similar to wild type animals: acute and chronic thermal analgesia, physical dependence, sensitization and rewarding properties revealed no significant differences with wild type animals (Erbs et al., 2014). These data suggest that predominant intracellular localization of MOP-mcherry receptors with low expression at the cell surface indeed reflect endogenous wild type receptor subcellular distribution under basal conditions, as observed in enteric neurons (Poole et al., 2011). In addition, internalization kinetics of MOP-mcherry upon activation by the agonist DAMGO in hippocampal primary neuronal cultures (Erbs et al., 2014) were similar to those reported for DAMGO promoted internalization of endogenous wild type receptors in the rat spinal cord (Trafton et al., 2000) and in organotypic cultures of guinea pig ileum (Minnis et al., 2003) or to Fluoro-dermorphin-induced sequestration in rat cortical primary neurons (Lee et al., 2002). This supports once again the use of fluorescent knock-in mice to study endogenous receptor trafficking. Of note, DAMGO promotes Flag-MOP receptor internalization with similar kinetics in transfected striatal primary neurons (Haberstock-Debic et al., 2005), in adenovirus infected primary cultures from DRG (Walwyn et al., 2006) or in neurons of the locus coeruleus in brain slices from transgenic FLAG-MOP receptor mice (Arttamangkul and Quillinan, 2008).
Conclusions and Impact for Drug Design
Fluorescent knock-in mice represent a substantial technical improvement in basic science. Precise identification and localization of the neurons expressing the GPCR of interest and reliable monitoring of receptor subcellular localization are both essential in understanding the physiopathological roles of endogenous GPCRs. This was greatly anticipated, given the difficulties encountered by many on the grounds of poor specificity of the available antibodies for GPCR targeting. The main surprising finding is maybe that the presence of the FP at the C-terminus of the GPCR does not significantly alter the behavioral output: this observation fully validates the technology. However, fluorescent knock-in animals available to date target a handful of class A GPCRs only. The potency of the model being now clearly established, one would expect rapid expansion to other receptors, in particular those with critical roles in human pathologies. Forefront candidates include class C GABAB and metabotropic glutamate receptors, both of which are involved in a wide range of neurological disorders such as schizophrenia, neuropathic pain, cerebral ischemia, mood disorders and substance abuse (Benes and Berretta, 2001; Delille et al., 2013; Kumar et al., 2013). Fluorescent knock-in animals would enable to revisit heterodimerization mechanisms, membrane targeting and cellular distribution patterns of these obligatory heterodimers in vivo. Furthermore, the relation between multimer scaffold composition, in particular GABAB auxiliary subunits, and neuronal or synaptic functions could also be readily examined to refine our current understanding of the variations in pharmacological and functional responses mediated by native receptors (Gassmann and Bettler, 2012).
The knock-in mice bearing GPCR-FP fusions already contributed to understanding the fundamental concepts of distinct signaling or regulatory responses recruited by different agonists of the same GPCR. These essential aspects of biased agonism are a growing central concern in drug discovery in the hope of developing strategies that ally high efficacy with low or no side effects. In addition, GPCR-FP fusions could bring considerable knowledge regarding functional aspects of receptor activity and internalization to evaluate the therapeutic potency of allosteric modulators. This very active field of research is mainly targeting class C GPCRs with well identified allosteric and orthosteric binding sites such as metabotropic glutamate or GABAB receptors but relevance for class A GPCRs is attracting increasing attention (Nickols and Conn, 2014). Direct visualization of the neurons of interest, either by FP under the control of a GPCR promoter or by expression of the GPCR fluorescent construct, also represents a significant breakthrough by making subsequent targeted investigations available. This includes electrophysiological recordings on previously identified cell, cell isolation by fluorescence-activated cell sorting for further biochemical (Western Blotting) and molecular (RT-PCR) downstream analysis or highly specific and efficient immunoprecipitation of the interacting partners. The presence of the FP also gives access to imaging techniques with which receptor population tracking within membranes can be achieved, by fluorescence recovery after photobleaching or fluorescence resonance energy transfer. The latter also opens ways to identify heteromer formation between GPCRs or between a GPCR and a ligand-gated channel and to investigate in vivo their intracellular fate and impact on signaling cascades. All these technological developments will undeniably contribute to deepening our current knowledge of GPCR controlled molecular and cellular processes and ultimately will benefit to drug design and screening.
Conflict of Interest Statement
The authors declare that the research was conducted in the absence of any commercial or financial relationships that could be construed as a potential conflict of interest.
Acknowledgments
The authors acknowledge the support of CNRS, the FRM grant (DPA20140629804).
References
Akil, H., Owens, C., Gutstein, H., Taylor, L., Curran, E., and Watson, S. (1998). Endogenous opioids: overview and current issues. Drug Alcohol Depend. 51, 127–140. doi: 10.1016/S0376-8716(98)00071-4
Pubmed Abstract | Pubmed Full Text | CrossRef Full Text | Google Scholar
Alexander, S. P. (2013). The concise guide to pharmacology 2013/14: G protein coupled receptors. Br. J. Pharmacol. 170, 1459–1581. doi: 10.1111/bph.12445
Pubmed Abstract | Pubmed Full Text | CrossRef Full Text | Google Scholar
Arttamangkul, S., and Quillinan, N. (2008). Differential activation and trafficking of μ-opioid receptors in brain slices. Mol. Pharmacol. 74, 972–979. doi: 10.1124/mol.108.048512
Pubmed Abstract | Pubmed Full Text | CrossRef Full Text | Google Scholar
Bardoni, R., Tawfik, V. L., Wang, D., François, A., Solorzano, C., Shuster, S. A.,et al. (2014). Delta opioid receptors presynaptically regulate cutaneous mechanosensory neuron input to the spinal cord dorsal horn. Neuron 81, 1312–1327. doi: 10.1016/j.neuron.2014.01.044
Pubmed Abstract | Pubmed Full Text | CrossRef Full Text | Google Scholar
Behrens, M., Foerster, S., Staehler, F., Raguse, J.-D., and Meyerhof, W. (2007). Gustatory expression pattern of the human TAS2R bitter receptor gene family reveals a heterogenous population of bitter responsive taste receptor cells. J. Neurosci. 27, 12630–12640. doi: 10.1523/JNEUROSCI.1168-07.2007
Pubmed Abstract | Pubmed Full Text | CrossRef Full Text | Google Scholar
Benes, F., and Berretta, S. (2001). GABAergic interneurons: implications for understanding schizophrenia and bipolar disorder. Neuropsychopharmacology 25, 1–27. doi: 10.1016/S0893-133X(01)00225-1
Pubmed Abstract | Pubmed Full Text | CrossRef Full Text | Google Scholar
Bertran-Gonzalez, J., Bosch, C., Maroteaux, M., Matamales, M., Hervé, D., Valjent, E.,et al. (2008). Opposing patterns of signaling activation in dopamine D1 and D2 receptor-expressing striatal neurons in response to cocaine and haloperidol. J. Neurosci. 28, 5671–5685. doi: 10.1523/JNEUROSCI.1039-08.2008
Pubmed Abstract | Pubmed Full Text | CrossRef Full Text | Google Scholar
Bertran-Gonzalez, J., Laurent, V., Chieng, B. C., Christie, M. J., and Balleine, B. W. (2013). Learning-related translocation of δ-opioid receptors on ventral striatal cholinergic interneurons mediates choice between goal-directed actions. J. Neurosci. 33, 16060–16071. doi: 10.1523/JNEUROSCI.1927-13.2013
Pubmed Abstract | Pubmed Full Text | CrossRef Full Text | Google Scholar
Bradley, S. J., Riaz, S. A., and Tobin, A. B. (2014). Employing novel animal models in the design of clinically efficacious GPCR ligands. Curr. Opin. Cell Biol. 27, 117–125. doi: 10.1016/j.ceb.2013.12.002
Pubmed Abstract | Pubmed Full Text | CrossRef Full Text | Google Scholar
Cahill, C. M., Holdridge, S. V., and Morinville, A. (2007). Trafficking of delta-opioid receptors and other G-protein-coupled receptors: implications for pain and analgesia. Trends Pharmacol. Sci. 28, 23–31. doi: 10.1016/j.tips.2006.11.003
Pubmed Abstract | Pubmed Full Text | CrossRef Full Text | Google Scholar
Cahill, C. M., Morinville, A., Lee, M., Vincent, J., Collier, B., and Beaudet, A. (2001). Prolonged morphine treatment targets delta opioid receptors to neuronal plasma membranes and enhances delta -mediated antinociception. J. Neurosci. 21, 7598–7607.
Campbell, R. E., Tour, O., Palmer, A. E., Steinbach, P. A., Baird, G. S., Zacharias, D. A.,et al. (2002). A monomeric red fluorescent protein. Proc. Natl. Acad. Sci. U.S.A. 99, 7877–7882. doi: 10.1073/pnas.082243699
Pubmed Abstract | Pubmed Full Text | CrossRef Full Text | Google Scholar
Chan, C. S., Peterson, J. D., Gertler, T. S., Glajch, K. E., Quintana, R. E., Cui, Q.,et al. (2012). Strain-specific regulation of striatal phenotype in Drd2-eGFP BAC transgenic mice. J. Neurosci. 32, 9124–9132. doi: 10.1523/JNEUROSCI.0229-12.2012
Pubmed Abstract | Pubmed Full Text | CrossRef Full Text | Google Scholar
Chan, F., Bradley, A., Wensel, T. G., and Wilson, J. H. (2004). Knock-in human rhodopsin-GFP fusions as mouse models for human disease and targets for gene therapy. Proc. Natl. Acad. Sci. U.S.A. 101, 9109–9114. doi: 10.1073/pnas.0403149101
Pubmed Abstract | Pubmed Full Text | CrossRef Full Text | Google Scholar
Chu Sin Chung, P., and Kieffer, B. L. (2013). Delta opioid receptors in brain function and diseases. Pharmacol. Ther. 140, 112–120. doi: 10.1016/j.pharmthera.2013.06.003
Pubmed Abstract | Pubmed Full Text | CrossRef Full Text | Google Scholar
Chung, M.-K., Jue, S. S., and Dong, X. (2012). Projection of non-peptidergic afferents to mouse tooth pulp. J. Dent. Res. 91, 777–782. doi: 10.1177/0022034512450298
Pubmed Abstract | Pubmed Full Text | CrossRef Full Text | Google Scholar
Congreve, M., Dias, J. M., and Marshall, F. H. (2014). Structure-based drug design for G protein-coupled receptors. Prog. Med. Chem. 53, 1–63 doi: 10.1016/B978-0-444-63380-4.00001-9
Pubmed Abstract | Pubmed Full Text | CrossRef Full Text | Google Scholar
Cormack, B. P., Bertram, G., Egerton, M., Gow, N. A. R., Falkow, S., and Brown, A. J. P. (1997). Yeast-enhanced green fluorescent protein (yEGFP): a reporter of gene expression in Candida albicans. Microbiology 143, 303–311. doi: 10.1099/00221287-143-2-303
Pubmed Abstract | Pubmed Full Text | CrossRef Full Text | Google Scholar
Delille, H. K., Mezler, M., and Marek, G. J. (2013). The two faces of the pharmacological interaction of mGlu2 and 5-HT2A – relevance of receptor heterocomplexes and interaction through functional brain pathways. Neuropharmacology 70, 296–305. doi: 10.1016/j.neuropharm.2013.02.005
Pubmed Abstract | Pubmed Full Text | CrossRef Full Text | Google Scholar
Dong, X., Han, S., Zylka, M. J., Simon, M. I., and Anderson, D. J. (2001). A diverse family of GPCRs expressed in specific subsets of nociceptive sensory neurons. Cell 106, 619–632. doi: 10.1016/S0092-8674(01)00483-4
Pubmed Abstract | Pubmed Full Text | CrossRef Full Text | Google Scholar
Doyle, A., McGarry, M. P., Lee, N. A., and Lee, J. J. (2012). The construction of transgenic and gene knockout/knockin mouse models of human disease. Transgenic Res. 21, 327–349. doi: 10.1007/s11248-011-9537-3
Pubmed Abstract | Pubmed Full Text | CrossRef Full Text | Google Scholar
Duncan, K., Ketz, N., and Davachi, L. (2012). Evidence for area CA1as a match/mismatch detector: a high-resolution fMRI study of the human hippocampus. Hippocampus 22, 389–398. doi: 10.1002/hipo.20933
Pubmed Abstract | Pubmed Full Text | CrossRef Full Text | Google Scholar
Dunham, J. H., and Hall, R. A. (2009). Enhancement of the surface expression of G protein-coupled receptors. Trends Biotechnol. 27, 541–545. doi: 10.1016/j.tibtech.2009.06.005
Pubmed Abstract | Pubmed Full Text | CrossRef Full Text | Google Scholar
Erbs, E., Faget, L., Scherrer, G., and Kessler, P. (2012). Distribution of delta opioid receptor-expressing neurons in the mouse hippocampus. Neuroscience 221, 203–213. doi: 10.1016/j.neuroscience.2012.06.023
Pubmed Abstract | Pubmed Full Text | CrossRef Full Text | Google Scholar
Erbs, E., Faget, L., Scherrer, G., Matifas, A., Filliol, D., Vonesch, J.-L.,et al. (2014). A mu-delta opioid receptor brain atlas reveals neuronal co-occurrence in subcortical networks. Brain Struct. Funct. doi: 10.1007/s00429-014-0717-9 [Epub ahead of print].
Pubmed Abstract | Pubmed Full Text | CrossRef Full Text | Google Scholar
Faget, L., Erbs, E., LeMerrer, J., Scherrer, G., Matifas, A., Benturquia, N.,et al. (2012). In vivo visualization of delta opioid receptors upon physiological activation uncovers a distinct internalization profile. J. Neurosci. 32, 7301–7310. doi: 10.1523/JNEUROSCI.0185-12.2012
Pubmed Abstract | Pubmed Full Text | CrossRef Full Text | Google Scholar
Ferguson, S. S. G., Ill, W. E. D., Colapietro, A., Barak, L. S., Menard, L., and Caron, M. G. (1996). Role of beta-arrestin in mediating agonist-promoted G protein-coupled receptor internalization. Science 271, 363–366. doi: 10.1126/science.271.5247.363
Pubmed Abstract | Pubmed Full Text | CrossRef Full Text | Google Scholar
Fuhrmann, M., Bittner, T., Jung, C. K. E., Burgold, S., Page, R. M., Mitteregger, G.,et al. (2010). Microglial Cx3cr1 knockout prevents neuron loss in a mouse model of Alzheimer’s disease. Nat. Neurosci. 13, 411–413. doi: 10.1038/nn.2511
Pubmed Abstract | Pubmed Full Text | CrossRef Full Text | Google Scholar
Garcia, J., Cardona, S., and Cardona, A. (2013). Analyses of microglia effector function using CX3CR1-GFP knock-in mice. Microglia 1041, 307–317. doi: 10.1007/978-1-62703-520-0_27
Pubmed Abstract | Pubmed Full Text | CrossRef Full Text | Google Scholar
Gassmann, M., and Bettler, B. (2012). Regulation of neuronal GABA(B) receptor functions by subunit composition. Nat. Rev. Neurosci. 13, 380–394. doi: 10.1038/nrn3249
Pubmed Abstract | Pubmed Full Text | CrossRef Full Text | Google Scholar
Gendron, L., Lucido, A. L., Mennicken, F., O’Donnell, D., Vincent, J.-P., Stroh, T.,et al. (2006). Morphine and pain-related stimuli enhance cell surface availability of somatic delta-opioid receptors in rat dorsal root ganglia. J. Neurosci. 26, 953–962. doi: 10.1523/JNEUROSCI.3598-05.2006
Pubmed Abstract | Pubmed Full Text | CrossRef Full Text | Google Scholar
Gendron, L., Mittal, N., Beaudry, H., and Walwyn, W. (2014). Recent advances on the δ opioid receptor: from trafficking to function. Br. J. Pharmacol. doi: 10.1111/bph.12706 [Epub ahead of print].
Pubmed Abstract | Pubmed Full Text | CrossRef Full Text | Google Scholar
George, S., Zastawny, R., Brionesurbina, R., Cheng, R., Nguyen, T., Heiber, M.,et al. (1994). Distinct distributions of mu, delta and kappa opioid receptor mRNA in rat brain. Biochem. Biophys. Res. Commun. 205, 1438–1444. doi: 10.1006/bbrc.1994.2826
Giguere, P. M., Kroeze, W. K., and Roth, B. L. (2014). Tuning up the right signal: chemical and genetic approaches to study GPCR functions. Curr. Opin. Cell Biol. 27, 51–55. doi: 10.1016/j.ceb.2013.11.006
Pubmed Abstract | Pubmed Full Text | CrossRef Full Text | Google Scholar
Gomes, I., Gupta, A., Filipovska, J., Szeto, H. H., Pintar, J. E., and Devi, L. A. (2004). A role for heterodimerization of mu and delta opiate receptors in enhancing morphine analgesia. Proc. Natl. Acad. Sci. U.S.A. 101, 5135–5139. doi: 10.1073/pnas.0307601101
Pubmed Abstract | Pubmed Full Text | CrossRef Full Text | Google Scholar
Gong, S., Zheng, C., Doughty, M. L., Losos, K., Didkovsky, N., Schambra, U. B.,et al. (2003). A gene expression atlas of the central nervous system based on bacterial artificial chromosomes. Nature 425, 917–925. doi: 10.1038/nature02033
Pubmed Abstract | Pubmed Full Text | CrossRef Full Text | Google Scholar
Goody, R. J., Oakley, S. M., Filliol, D., Kieffer, B. L., and Kitchen, I. (2002). Q uantitative autoradiographic mapping of opioid receptors in the brain of d -opioid receptor gene knockout mice. Brain Res. 945, 9–19. doi: 10.1016/S0006-8993(02)02452-6
Gross, A. K., Decker, G., Chan, F., Sandoval, I. M., Wilson, J. H., and Wensel, T. G. (2006). Defective development of photoreceptor membranes in a mouse model of recessive retinal degeneration. Vision Res. 46, 4510–4518. doi: 10.1016/j.visres.2006.07.012
Pubmed Abstract | Pubmed Full Text | CrossRef Full Text | Google Scholar
Guan, J.-S., Xu, Z.-Z., Gao, H., He, S.-Q., Ma, G.-Q., Sun, T.,et al. (2005). Interaction with vesicle luminal protachykinin regulates surface expression of delta-opioid receptors and opioid analgesia. Cell 122, 619–631. doi: 10.1016/j.cell.2005.06.010
Pubmed Abstract | Pubmed Full Text | CrossRef Full Text | Google Scholar
Gupta, A., Mulder, J., and Gomes, I. (2010). Increased abundance of opioid receptor heteromers after chronic morphine administration. Sci. Signal. 3:ra54. doi: 10.1126/scisignal.2000807
Pubmed Abstract | Pubmed Full Text | CrossRef Full Text | Google Scholar
Haberstock-Debic, H., Kim, K.-A., Yu, Y. J., and von Zastrow, M. (2005). Morphine promotes rapid, arrestin-dependent endocytosis of mu-opioid receptors in striatal neurons. J. Neurosci. 25, 7847–7857. doi: 10.1523/JNEUROSCI.5045-04.2005
Pubmed Abstract | Pubmed Full Text | CrossRef Full Text | Google Scholar
Haruyama, N., Cho, A., and Kulkarni, A. (2009). Overview: engineering transgenic constructs and mice. Curr. Protoc. Cell Biol. Unit 19.10(Suppl. 42), 1–12. doi: 10.1002/0471143030.cb1910s42
Pubmed Abstract | Pubmed Full Text | CrossRef Full Text | Google Scholar
Heim, R., and Tsien, R. Y. (1996). Engineering green fluorescent protein for improved brightness, longer wavelengths and fluorescence resonance energy transfer. Curr. Biol. 6, 178–182. doi: 10.1016/S0960-9822(02)00450-5
Pubmed Abstract | Pubmed Full Text | CrossRef Full Text | Google Scholar
Heintz, N. (2001). BAC to the future: the use of BAC transgenic mice. Nat. Rev. Neurosci: 2, 1–10. doi: 10.1038/35104049
Pubmed Abstract | Pubmed Full Text | CrossRef Full Text | Google Scholar
Heng, B. C., Aubel, D., and Fussenegger, M. (2013). An overview of the diverse roles of G-protein coupled receptors (GPCRs) in the pathophysiology of various human diseases. Biotechnol. Adv. 31, 1676–1694. doi: 10.1016/j.biotechadv.2013.08.017
Pubmed Abstract | Pubmed Full Text | CrossRef Full Text | Google Scholar
Hoon, M., Adler, E., Lindemeier, J., and Battey, J. (1999). Putative mammalian taste receptors: a class of taste-specific GPCRs with distinct topographic selectivity. Cell 96, 541–551. doi: 10.1016/S0092-8674(00)80658-3
Pubmed Abstract | Pubmed Full Text | CrossRef Full Text | Google Scholar
Jung, S., Aliberti, J., Graemmel, P., Sunshine, M. J., Kreutzberg, G. W., Sher, A.,et al. (2000). Analysis of fractalkine receptor CX(3)CR1 function by targeted deletion and green fluorescent protein reporter gene insertion. Mol. Cell. Biol. 20, 4106–4114. doi: 10.1128/MCB.20.11.4106-4114.2000
Pubmed Abstract | Pubmed Full Text | CrossRef Full Text | Google Scholar
Kallal, L., and Benovic, J. L. (2000). Using green fluorescent proteins to study G-protein-coupled receptor localization and trafficking. Trends Pharmacol. Sci. 21, 175–180. doi: 10.1016/S0165-6147(00)01477-2
Kenakin, T. (2014). What is pharmacological “affinity?” Relevance to biased agonism and antagonism. Trends Pharmacol. Sci. 35, 434–441. doi: 10.1016/j.tips.2014.06.003
Pubmed Abstract | Pubmed Full Text | CrossRef Full Text | Google Scholar
Kieffer, B. L., and Evans, C. J. (2009). Opioid receptors: from binding sites to visible molecules in vivo. Neuropharmacology 56(Suppl. 1), 205–212. doi: 10.1016/j.neuropharm.2008.07.033
Pubmed Abstract | Pubmed Full Text | CrossRef Full Text | Google Scholar
Kitchen, I., Slowe, S. J., Matthes, H. W. D., and Kieffer, B. L. (1997). Quantitative autoradiographic mapping of m -, d - and k -opioid receptors in knockout mice lacking the m -opioid receptor gene. Brain Res. 778, 73–88. doi: 10.1016/S0006-8993(97)00988-8
Kramer, P. F., Christensen, C. H., Hazelwood, L., Dobi, A., Bock, R., Sibley, D. R.,et al. (2011). Dopamine D2 receptor overexpression alters behavior and physiology in Drd2-EGFP mice. J. Neurosci. 31, 126–132. doi: 10.1523/JNEUROSCI.4287-10.2011
Pubmed Abstract | Pubmed Full Text | CrossRef Full Text | Google Scholar
Kumar, K., Sharma, S., Kumar, P., and Deshmukh, R. (2013). Therapeutic potential of GABA(B) receptor ligands in drug addiction, anxiety, depression and other CNS disorders. Pharmacol. Biochem. Behav. 110, 174–184. doi: 10.1016/j.pbb.2013.07.003
Pubmed Abstract | Pubmed Full Text | CrossRef Full Text | Google Scholar
Lagerström, M. C., and Schiöth, H. B. (2008). Structural diversity of G protein-coupled receptors and significance for drug discovery. Nat. Rev. Drug Discov. 7, 339–357. doi: 10.1038/nrd2518
Pubmed Abstract | Pubmed Full Text | CrossRef Full Text | Google Scholar
Laurent, V., Wong, F. L., and Balleine, B. W. (2014). δ-Opioid receptors in the accumbens shell mediate the influence of both excitatory and inhibitory predictions on choice. Br. J. Pharmacol. doi: 10.1111/bph.12731 [Epub ahead of print].
Pubmed Abstract | Pubmed Full Text | CrossRef Full Text | Google Scholar
Lee, K.-W., Kim, Y., Kim, A. M., Helmin, K., Nairn, A. C., and Greengard, P. (2006). Cocaine-induced dendritic spine formation in D1 and D2 dopamine receptor-containing medium spiny neurons in nucleus accumbens. Proc. Natl. Acad. Sci. U.S.A. 103, 3399–3404. doi: 10.1073/pnas.0511244103
Pubmed Abstract | Pubmed Full Text | CrossRef Full Text | Google Scholar
Lee, M., Cahill, C., Vincent, J., and Beaudet, A. (2002). Internalization and trafficking of opioid receptor ligands in rat cortical neurons. Synapse 111, 102–111. doi: 10.1002/syn.10014
Pubmed Abstract | Pubmed Full Text | CrossRef Full Text | Google Scholar
LeMerrer, J., Becker, J. A. J., Befort, K., and Kieffer, B. L. (2009). Reward processing by the opioid system in the brain. Physiol. Rev. 89, 1379–1412. doi: 10.1152/physrev.00005.2009
Pubmed Abstract | Pubmed Full Text | CrossRef Full Text | Google Scholar
Lesscher, H. M. B., Bailey, A., Burbach, J. P. H., van Ree, J. M., Kitchen, I., and Gerrits, M. A. F. M. (2003). Receptor-selective changes in u-, delta- and kappa-opioid receptors after chronic naltrexone treatment in mice. Eur. J. Neurosci. 17, 1006–1012. doi: 10.1046/j.1460-9568.2003.02502.x
Pubmed Abstract | Pubmed Full Text | CrossRef Full Text | Google Scholar
Liman, E. R., Zhang, Y. V., and Montell, C. (2014). Peripheral coding of taste. Neuron 81, 984–1000. doi: 10.1016/j.neuron.2014.02.022
Pubmed Abstract | Pubmed Full Text | CrossRef Full Text | Google Scholar
Liu, Q., Sikand, P., Ma, C., Tang, Z., Han, L., Li, Z.,et al. (2012). Mechanisms of itch evoked by β-alanine. J. Neurosci. 32, 14532–14537. doi: 10.1523/JNEUROSCI.3509-12.2012
Pubmed Abstract | Pubmed Full Text | CrossRef Full Text | Google Scholar
Lutz, P.-E., and Kieffer, B. L. (2013). The multiple facets of opioid receptor function: implications for addiction. Curr. Opin. Neurobiol. 23, 473–479. doi: 10.1016/j.conb.2013.02.005
Pubmed Abstract | Pubmed Full Text | CrossRef Full Text | Google Scholar
Madziva, M. T., and Edwardson, J. M. (2001). Trafficking of green fluorescent protein-tagged muscarinic M4 receptors in NG108-15 cells. Eur. J. Pharmacol. 428, 9–18. doi: 10.1016/S0014-2999(01)01266-3
Pubmed Abstract | Pubmed Full Text | CrossRef Full Text | Google Scholar
Mailleux, P., and Vanderhaeghen, J. J. (1992). Distribution of neuronal cannabinoid receptor in the adult rat brain: a comparative receptor binding radioautography and in situ hybridization histochemistry. Neuroscience 48, 655–668. doi: 10.1016/0306-4522(92)90409-U
Pubmed Abstract | Pubmed Full Text | CrossRef Full Text | Google Scholar
Mansour, A., Fox, C. A., Akil, H., and Watson, S. J. (1995). Opioid-receptor mRNA expression in the rat CNS: anatomical and functional implications. Trends Neurosci. 18, 22–29. doi: 10.1016/0166-2236(95)93946-U
Pubmed Abstract | Pubmed Full Text | CrossRef Full Text | Google Scholar
Marder, E. (2012). Neuromodulation of neuronal circuits: back to the future. Neuron 76, 1–11. doi: 10.1016/j.neuron.2012.09.010
Pubmed Abstract | Pubmed Full Text | CrossRef Full Text | Google Scholar
Marsicano, G., and Lutz, B. (1999). Expression of the cannabinoid receptor CB1 in distinct neuronal subpopulations in the adult mouse forebrain. Eur. J. Neurosci. 11, 4213–4225. doi: 10.1046/j.1460-9568.1999.00847.x
McDonald, N. A., Henstridge, C. M., Connolly, C. N., and Irving, A. J. (2007). Generation and functional characterization of fluorescent, N-terminally tagged CB1 receptor chimeras for live-cell imaging. Mol. Cell. Neurosci. 35, 237–248. doi: 10.1016/j.mcn.2007.02.016
Pubmed Abstract | Pubmed Full Text | CrossRef Full Text | Google Scholar
McLean, A. J., and Milligan, G. (2000). Ligand regulation of green fluorescent protein-tagged forms of the human beta(1)- and beta(2)-adrenoceptors; comparisons with the unmodified receptors. Br. J. Pharmacol. 130, 1825–1832. doi: 10.1038/sj.bjp.0703506
Pubmed Abstract | Pubmed Full Text | CrossRef Full Text | Google Scholar
Minnis, J. G., Patierno, S., Kohlmeier, S. E., Brecha, N. C., Tonini, M., and Sternini, C. (2003). Ligand-induced μ opioid receptor endocytosis and recycling in enteric neurons. Neuroscience 119, 33–42. doi: 10.1016/S0306-4522(03)00135-0
Mizutani, M., Pino, P. A., Saederup, N., Charo, I. F., Ransohoff, R. M., and Cardona, A. E. (2012). The fractalkine receptor but not CCR2 is present on microglia from embryonic development throughout adulthood. J. Immunol. 188, 29–36. doi: 10.4049/jimmunol.1100421
Moreira, I. S. (2014). Structural features of the G-protein/GPCR interactions. Biochim. Biophys. Acta 1840, 16–33. doi: 10.1016/j.bbagen.2013.08.027
Pubmed Abstract | Pubmed Full Text | CrossRef Full Text | Google Scholar
Morinville, A., Cahill, C. M., Kieffer, B., Collier, B., and Beaudet, A. (2004). Mu-opioid receptor knockout prevents changes in delta-opioid receptor trafficking induced by chronic inflammatory pain. Pain 109, 266–273. doi: 10.1016/j.pain.2004.01.011
Pubmed Abstract | Pubmed Full Text | CrossRef Full Text | Google Scholar
Nagai, T., Ibata, K., Park, E. S., Kubota, M., Mikoshiba, K., and Miyawaki, A. (2002). A variant of yellow fluorescent protein with fast and efficient maturation for cell-biological applications. Nat. Biotechnol. 20, 87–90. doi: 10.1038/nbt0102-87
Pubmed Abstract | Pubmed Full Text | CrossRef Full Text | Google Scholar
Nickols, H. H., and Conn, P. J. (2014). Development of allosteric modulators of GPCRs for treatment of CNS disorders. Neurobiol. Dis. 61, 55–71. doi: 10.1016/j.nbd.2013.09.013
Pubmed Abstract | Pubmed Full Text | CrossRef Full Text | Google Scholar
Nienhaus, K., and Nienhaus, G. U. (2014). Fluorescent proteins for live-cell imaging with super-resolution. Chem. Soc. Rev. 43, 1088–1106. doi: 10.1039/c3cs60171d
Pubmed Abstract | Pubmed Full Text | CrossRef Full Text | Google Scholar
Ostrom, R. S., and Insel, P. A. (2004). The evolving role of lipid rafts and caveolae in G protein-coupled receptor signaling: implications for molecular pharmacology. Br. J. Pharmacol. 143, 235–245. doi: 10.1038/sj.bjp.0705930
Pubmed Abstract | Pubmed Full Text | CrossRef Full Text | Google Scholar
Overington, J. P., Al-Lazikani, B., and Hopkins, A. L. (2006). How many drug targets are there? Nat.Rev. Drug Discov. 5, 993–996. doi: 10.1038/nrd2199
Pubmed Abstract | Pubmed Full Text | CrossRef Full Text | Google Scholar
Papay, R., Gaivin, R., McCune, D. F., Rorabaugh, B. R., Macklin, W. B., McGrath, J. C.,et al. (2004). Mouse alpha1B-adrenergic receptor is expressed in neurons and NG2 oligodendrocytes. J. Comp. Neurol. 478, 1–10. doi: 10.1002/cne.20215
Pubmed Abstract | Pubmed Full Text | CrossRef Full Text | Google Scholar
Pertwee, R. G. (2006). The pharmacology of cannabinoid receptors and their ligands: an overview. Int. J. Obes. (Lond.) 30(Suppl. 1), S13–8. doi: 10.1038/sj.ijo.0803272
Pubmed Abstract | Pubmed Full Text | CrossRef Full Text | Google Scholar
Poole, D. P., Pelayo, J.-C., Scherrer, G., Evans, C. J., Kieffer, B. L., and Bunnett, N. W. (2011). Localization and regulation of fluorescently labeled delta opioid receptor, expressed in enteric neurons of mice. Gastroenterology 141, 982–991.e1–8. doi: 10.1053/j.gastro.2011.05.042
Pubmed Abstract | Pubmed Full Text | CrossRef Full Text | Google Scholar
Pradhan, A. A. A., Becker, J. A. J., Scherrer, G., Tryoen-Toth, P., Filliol, D., Matifas, A.,et al. (2009). In vivo delta opioid receptor internalization controls behavioral effects of agonists. PLoS ONE 4:e5425. doi: 10.1371/journal.pone.0005425
Pubmed Abstract | Pubmed Full Text | CrossRef Full Text | Google Scholar
Pradhan, A. A. A., and Clarke, P. B. S. (2005). Comparison between delta-opioid receptor functional response and autoradiographic labeling in rat brain and spinal cord. J. Comp. Neurol. 481, 416–426. doi: 10.1002/cne.20378
Pubmed Abstract | Pubmed Full Text | CrossRef Full Text | Google Scholar
Pradhan, A. A. A., Walwyn, W., Nozaki, C., Filliol, D., Erbs, E., Matifas, A.,et al. (2010). Ligand-directed trafficking of the δ-opioid receptor in vivo: two paths toward analgesic tolerance. J. Neurosci. 30, 16459–16468. doi: 10.1523/JNEUROSCI.3748-10.2010
Pubmed Abstract | Pubmed Full Text | CrossRef Full Text | Google Scholar
Price, B. A., Sandoval, I. M., Chan, F., Simons, D. L., Wu, S. M., Wensel, T. G.,et al. (2011). Mislocalization and degradation of human P23H-rhodopsin-GFP in a knockin mouse model of retinitis pigmentosa. Invest. Ophthalmol. Vis. Sci. 52, 9728–9736. doi: 10.1167/iovs.11-8654
Pubmed Abstract | Pubmed Full Text | CrossRef Full Text | Google Scholar
Rezai, X., Faget, L., Bednarek, E., Schwab, Y., Kieffer, B. L., and Massotte, D. (2012). Mouse δ opioid receptors are located on presynaptic afferents to hippocampal pyramidal cells. Cell. Mol. Neurobiol. 32, 509–516. doi: 10.1007/s10571-011-9791-1
Pubmed Abstract | Pubmed Full Text | CrossRef Full Text | Google Scholar
Rezai, X., Kieffer, B. L., Roux, M. J., and Massotte, D. (2013). Delta opioid receptors regulate temporoammonic-activated feedforward inhibition to the mouse CA1 hippocampus. PLoS ONE 8:e79081. doi: 10.1371/journal.pone.0079081
Pubmed Abstract | Pubmed Full Text | CrossRef Full Text | Google Scholar
Rigato, C., Swinnen, N., Buckinx, R., Couillin, I., Mangin, J.-M., Rigo, J.-M.,et al. (2012). Microglia proliferation is controlled by P2X7 receptors in a Pannexin-1-independent manner during early embryonic spinal cord invasion. J. Neurosci. 32, 11559–11573. doi: 10.1523/JNEUROSCI.1042-12.2012
Pubmed Abstract | Pubmed Full Text | CrossRef Full Text | Google Scholar
Rosenbaum, D. M., Rasmussen, S. G. F., and Kobilka, B. K. (2009). The structure and function of G-protein-coupled receptors. Nature 459, 356–363. doi: 10.1038/nature08144
Pubmed Abstract | Pubmed Full Text | CrossRef Full Text | Google Scholar
Roy, S., Rached, M., and Gallo-Payet, N. (2007). Differential regulation of the human adrenocorticotropin receptor [melanocortin-2 receptor (MC2R)] by human MC2R accessory protein isoforms alpha and beta in isogenic human embryonic kidney 293 cells. Mol. Endocrinol. 21, 1656–1669. doi: 10.1210/me.2007-0041
Pubmed Abstract | Pubmed Full Text | CrossRef Full Text | Google Scholar
Rozenfeld, R., Bushlin, I., Gomes, I., Tzavaras, N., Gupta, A., Neves, S.,et al. (2012). Receptor heteromerization expands the repertoire of cannabinoid signaling in rodent neurons. PLoS ONE 7:e29239. doi: 10.1371/journal.pone.0029239
Pubmed Abstract | Pubmed Full Text | CrossRef Full Text | Google Scholar
Saederup, N., Cardona, A. E., Croft, K., Mizutani, M., Cotleur, A. C., Tsou, C.-L.,et al. (2010). Selective chemokine receptor usage by central nervous system myeloid cells in CCR2-red fluorescent protein knock-in mice. PLoS ONE 5:e13693. doi: 10.1371/journal.pone.0013693
Pubmed Abstract | Pubmed Full Text | CrossRef Full Text | Google Scholar
Sandoval, I. M., Price, B. A., Gross, A. K., Chan, F., Sammons, J. D., Wilson, J. H.,et al. (2014). Abrupt onset of mutations in a developmentally regulated gene during terminal differentiation of post-mitotic photoreceptor neurons in mice. PLoS ONE 9:e108135. doi: 10.1371/journal.pone.0108135
Pubmed Abstract | Pubmed Full Text | CrossRef Full Text | Google Scholar
Scherrer, G., Imamachi, N., Cao, Y.-Q., Contet, C., Mennicken, F., O’Donnell, D.,et al. (2009). Dissociation of the opioid receptor mechanisms that control mechanical and heat pain. Cell 137, 1148–1159. doi: 10.1016/j.cell.2009.04.019
Pubmed Abstract | Pubmed Full Text | CrossRef Full Text | Google Scholar
Scherrer, G., Tryoen-to, P., Filliol, D., Matifas, A., Laustriat, D., Cao, Y. Q.,et al. (2006). Knockin mice expressing fluorescent delta -opioid receptors uncover G protein-coupled receptor dynamics in vivo. Proc. Natl. Acad. Sci. U.S.A. 103, 9691–9696. doi: 10.1073/pnas.0603359103
Pubmed Abstract | Pubmed Full Text | CrossRef Full Text | Google Scholar
Shaner, N. C., Campbell, R. E., Steinbach, P. A., Giepmans, B. N. G., Palmer, A. E., and Tsien, R. Y. (2004). Improved monomeric red, orange and yellow fluorescent proteins derived from Discosoma sp. red fluorescent protein. Nat. Biotechnol. 22, 1567–1572. doi: 10.1038/nbt1037
Pubmed Abstract | Pubmed Full Text | CrossRef Full Text | Google Scholar
Shaner, N. C., Patterson, G. H., and Davidson, M. W. (2007). Advances in fluorescent protein technology. J. Cell Sci. 120, 4247–4260. doi: 10.1242/jcs.005801
Pubmed Abstract | Pubmed Full Text | CrossRef Full Text | Google Scholar
Shimomura, O., Johnson, F., and Saiga, Y. (1962). Extraction, purification and properties of aequorin, a bioluminescent protein from the luminous hydromedusan, Aequorea. J. Cell. Comp. Physiol. 59, 223–239. doi: 10.1002/jcp.1030590302
Pubmed Abstract | Pubmed Full Text | CrossRef Full Text | Google Scholar
Slowe, S. J., Simonin, F., Kieffer, B. L., and Kitchen, I. (1999). Quantitative autoradiography of mu, delta and kappa opioid receptors in kappa-opioid receptor knockout mice. Brain Res. 818, 335–345. doi: 10.1016/S0006-8993(98)01201-3
Stockton, S. D., and Devi, L. A. (2012). Functional relevance of μ-δ opioid receptor heteromerization: a role in novel signaling and implications for the treatment of addiction disorders: from a symposium on new concepts in mu-opioid pharmacology. Drug Alcohol Depend. 121, 167–172. doi: 10.1016/j.drugalcdep.2011.10.025
Pubmed Abstract | Pubmed Full Text | CrossRef Full Text | Google Scholar
Stone, L. M., Barrows, J., Finger, T. E., and Kinnamon, S. C. (2007). Expression of T1Rs and gustducin in palatal taste buds of mice. Chem. Senses 32, 255–262. doi: 10.1093/chemse/bjl053
Pubmed Abstract | Pubmed Full Text | CrossRef Full Text | Google Scholar
Stumm, R. K., Zhou, C., Schulz, S., and Höllt, V. (2004). Neuronal types expressing mu- and delta-opioid receptor mRNA in the rat hippocampal formation. J. Comp. Neurol. 469, 107–118. doi: 10.1002/cne.10997
Pubmed Abstract | Pubmed Full Text | CrossRef Full Text | Google Scholar
Subach, F. V, Patterson, G. H., Manley, S., Gillette, J. M., Lippincott-Schwartz, J., and Verkhusha, V. V. (2009). Photoactivatable mCherry for high-resolution two-color fluorescence microscopy. Nat. Methods 6, 153–159. doi: 10.1038/nmeth.1298
Pubmed Abstract | Pubmed Full Text | CrossRef Full Text | Google Scholar
Tan, H., Ahmad, T., Loureiro, M., Zunder, J., and Laviolette, S. R. (2014). The role of cannabinoid transmission in emotional memory formation: implications for addiction and schizophrenia. Front. Psychiatry 5:73. doi: 10.3389/fpsyt.2014.00073
Pubmed Abstract | Pubmed Full Text | CrossRef Full Text | Google Scholar
Tempel, A., and Zukin, R. (1987). Neuroanatomical patterns of the mu, delta, and kappa opioid receptors of rat brain as determined by quantitative in vitro autoradiography. Proc. Natl. Acad. Sci. U.S.A. 84, 4308–4312. doi: 10.1073/pnas.84.12.4308
Tian, X., Kai, L., Hockberger, P. E., Wokosin, D. L., and Surmeier, D. J. (2010). MEF-2 regulates activity-dependent spine loss in striatopallidal medium spiny neurons. Mol. Cell. Neurosci. 44, 94–108. doi: 10.1016/j.mcn.2010.01.012
Pubmed Abstract | Pubmed Full Text | CrossRef Full Text | Google Scholar
Trafton, J. A., Abbadie, C., Marek, K., and Basbaum, A. I. (2000). Postsynaptic signaling via the mu -opioid receptor: responses of dorsal horn neurons to exogenous opioids and noxious stimulation. J. Neurosci. 20, 8578–8584.
Tsien, R. (1998). The green fluorescent protein. Annu. Rev. Biochem. 67, 509–544. doi: 10.1146/annurev.biochem.67.1.509
Tsou, K., Brown, S., and Sanudo-Pena, M. (1997). Immunohistochemical distribution of cannabinoid CB1 receptors in the rat central nervous system. Neuroscience 83, 393–411. doi: 10.1016/S0306-4522(97)00436-3
Vaccari, C., Lolait, S. J., and Ostrowski, N. L. (1998). Comparative distribution of vasopressin V1b and oxytocin receptor messenger ribonucleic acids in brain. Endocrinology 139, 5015–5033. doi: 10.1210/endo.139.12.6382
Pubmed Abstract | Pubmed Full Text | CrossRef Full Text | Google Scholar
Valjent, E., Bertran-Gonzalez, J., Hervé, D., Fisone, G., and Girault, J.-A. (2009). Looking BAC at striatal signaling: cell-specific analysis in new transgenic mice. Trends Neurosci. 32, 538–547. doi: 10.1016/j.tins.2009.06.005
Pubmed Abstract | Pubmed Full Text | CrossRef Full Text | Google Scholar
van den Pol, A. N. (2012). Neuropeptide transmission in brain circuits. Neuron 76, 98–115. doi: 10.1016/j.neuron.2012.09.014
Pubmed Abstract | Pubmed Full Text | CrossRef Full Text | Google Scholar
van Rijn, R. M., Whistler, J. L., and Waldhoer, M. (2010). Opioid-receptor-heteromer-specific trafficking and pharmacology. Curr. Opin. Pharmacol. 10, 73–79. doi: 10.1016/j.coph.2009.09.007
Pubmed Abstract | Pubmed Full Text | CrossRef Full Text | Google Scholar
Virgone-Carlotta, A., Uhlrich, J., Akram, M. N., Ressnikoff, D., Chrétien, F., Domenget, C.,et al. (2013). Mapping and kinetics of microglia/neuron cell-to-cell contacts in the 6-OHDA murine model of Parkinson’s disease. Glia 61, 1645–1658. doi: 10.1002/glia.22546
Pubmed Abstract | Pubmed Full Text | CrossRef Full Text | Google Scholar
Voigt, A., Hübner, S., Lossow, K., Hermans-Borgmeyer, I., Boehm, U., and Meyerhof, W. (2012). Genetic labeling of Tas1r1 and Tas2r131 taste receptor cells in mice. Chem. Senses 37, 897–911. doi: 10.1093/chemse/bjs082
Pubmed Abstract | Pubmed Full Text | CrossRef Full Text | Google Scholar
von Zastrow, M., and Williams, J. T. (2012). Modulating neuromodulation by receptor membrane traffic in the endocytic pathway. Neuron 76, 22–32. doi: 10.1016/j.neuron.2012.09.022
Pubmed Abstract | Pubmed Full Text | CrossRef Full Text | Google Scholar
Walther, C., and Ferguson, S. S. G. (2013). Arrestins: role in the desensitization, sequestration, and vesicular trafficking of G protein-coupled receptors. Prog. Mol. Biol. Transl. Sci. 118, 93–113. doi: 10.1016/B978-0-12-394440-5.00004-8
Pubmed Abstract | Pubmed Full Text | CrossRef Full Text | Google Scholar
Walwyn, W. M., Miotto, K. A., and Evans, C. J. (2010). Opioid pharmaceuticals and addiction: the issues, and research directions seeking solutions. Drug Alcohol Depend. 108, 156–165. doi: 10.1016/j.drugalcdep.2010.01.001
Pubmed Abstract | Pubmed Full Text | CrossRef Full Text | Google Scholar
Walwyn, W. M., Wei, W., Xie, C.-W., Chiu, K., Kieffer, B. L., Evans, C. J.,et al. (2006). Mu opioid receptor-effector coupling and trafficking in dorsal root ganglia neurons. Neuroscience 142, 493–503. doi: 10.1016/j.neuroscience.2006.06.021
Pubmed Abstract | Pubmed Full Text | CrossRef Full Text | Google Scholar
Wang, H.-B., Zhao, B., Zhong, Y.-Q., Li, K.-C., Li, Z.-Y., Wang, Q.,et al. (2010). Coexpression of delta- and mu-opioid receptors in nociceptive sensory neurons. Proc. Natl. Acad. Sci. U.S.A. 107, 13117–13122. doi: 10.1073/pnas.1008382107
Pubmed Abstract | Pubmed Full Text | CrossRef Full Text | Google Scholar
Wensel, T. G., Gross, A. K., Chan, F., Sykoudis, K., and Wilson, J. H. (2005). Rhodopsin-EGFP knock-ins for imaging quantal gene alterations. Vision Res. 45, 3445–3453. doi: 10.1016/j.visres.2005.07.016
Pubmed Abstract | Pubmed Full Text | CrossRef Full Text | Google Scholar
Winters, B. D., Krüger, J. M., Huang, X., Gallaher, Z. R., Ishikawa, M., Czaja, K.,et al. (2012). Cannabinoid receptor 1-expressing neurons in the nucleus accumbens. Proc. Natl. Acad. Sci. U.S.A. 109, E2717–E2725. doi: 10.1073/pnas.1206303109
Pubmed Abstract | Pubmed Full Text | CrossRef Full Text | Google Scholar
Wrobel, L., Schorscher-Petcu, A., Dupré, A., Yoshida, M., Nishimori, K., and Tribollet, E. (2011). Distribution and identity of neurons expressing the oxytocin receptor in the mouse spinal cord. Neurosci. Lett. 495, 49–54. doi: 10.1016/j.neulet.2011.03.033
Pubmed Abstract | Pubmed Full Text | CrossRef Full Text | Google Scholar
Xie, W.-Y., He, Y., Yang, Y.-R., Li, Y.-F., Kang, K., Xing, B.-M.,et al. (2009). Disruption of Cdk5-associated phosphorylation of residue threonine-161 of the delta-opioid receptor: impaired receptor function and attenuated morphine antinociceptive tolerance. J. Neurosci. 29, 3551–3564. doi: 10.1523/JNEUROSCI.0415-09.2009
Pubmed Abstract | Pubmed Full Text | CrossRef Full Text | Google Scholar
Yoshida, M., Takayanagi, Y., Inoue, K., Kimura, T., Young, L. J., Onaka, T.,et al. (2009). Evidence that oxytocin exerts anxiolytic effects via oxytocin receptor expressed in serotonergic neurons in mice. J. Neurosci. 29, 2259–2271. doi: 10.1523/JNEUROSCI.5593-08.2009
Pubmed Abstract | Pubmed Full Text | CrossRef Full Text | Google Scholar
Yoshimura, N., Seki, S., Novakovic, S. D., Tzoumaka, E., Erickson, V. L., Erickson, K. A.,et al. (2001). The involvement of the tetrodotoxin-resistant sodium channel Na(v)1.8 (PN3/SNS) in a rat model of visceral pain. J. Neurosci. 21, 8690–8696.
Zylka, M. J., Rice, F. L., and Anderson, D. J. (2005). Topographically distinct epidermal nociceptive circuits revealed by axonal tracers targeted to Mrgprd. Neuron 45, 17–25. doi: 10.1016/j.neuron.2004.12.015
Pubmed Abstract | Pubmed Full Text | CrossRef Full Text | Google Scholar
Keywords: G protein-coupled receptors, fluorescent protein, knock-in, mouse model, drug design, biased agonism, receptor trafficking
Citation: Ceredig RA and Massotte D (2015) Fluorescent knock-in mice to decipher the physiopathological role of G protein-coupled receptors. Front. Pharmacol. 5:289. doi: 10.3389/fphar.2014.00289
Received: 03 November 2014; Accepted: 12 December 2014;
Published online: 06 January 2015.
Edited by:
Marianthi Papakosta, Pfizer, USAReviewed by:
Enrico Sanna, University of Cagliari, ItalyIoannis N. Charalampopoulos, University of Crete, Greece
Copyright © 2015 Ceredig and Massotte. This is an open-access article distributed under the terms of the Creative Commons Attribution License (CC BY). The use, distribution or reproduction in other forums is permitted, provided the original author(s) or licensor are credited and that the original publication in this journal is cited, in accordance with accepted academic practice. No use, distribution or reproduction is permitted which does not comply with these terms.
*Correspondence: Dominique Massotte, CNRS, Institut des Neurosciences Cellulaires et Intégratives, UPR 3212, 5 rue Blaise Pascal, 67084 Strasbourg Cedex 03, France e-mail:ZC5tYXNzb3R0ZUB1bmlzdHJhLmZy