- Joan and Sanford I. Weill Department of Medicine, Weill Cornell Medical Center, New York-Presbyterian Hospital – Weill Cornell Medical College, New York, NY, USA
Autophagy was originally described as a highly conserved system for the degradation of cytosol through a lysosome-dependent pathway. In response to starvation, autophagy degrades organelles and proteins to provide metabolites and energy for its pro-survival effects. Autophagy is recognized as playing a role in the pathogenesis of disease either directly or indirectly, through the regulation of vital processes such as programmed cell death, inflammation, and adaptive immune mechanisms. Recent studies have demonstrated that autophagy is not only a simple metabolite recycling system, but also has the ability to degrade specific cellular targets, such as mitochondria, cilia, and invading bacteria. In addition, selective autophagy has also been implicated in vesicle trafficking pathways, with potential roles in secretion and other intracellular transport processes. Selective autophagy has drawn the attention of researchers because of its potential importance in clinical diseases. Therapeutic strategies to target selective autophagy rather than general autophagy may maximize clinical benefit by enhancing selectivity. In this review, we outline the principle components of selective autophagy processes and their emerging importance in human disease, with an emphasis on pulmonary diseases.
Introduction
Autophagy is a lysosomal degradation system by which the cell can recycle its cytoplasmic components (Mizushima and Komatsu, 2011). At present, three different types of autophagic pathways have been reported, named as macroautophagy, microautophagy, and chaperone-mediated autophagy (Mizushima and Komatsu, 2011). Of these, macroautophagy is the best-characterized and most well-known form, often referred to simply as “autophagy.”
Autophagy proceeds through sequential steps, beginning with the generation of autophagosomes from an isolation membrane and followed by elongation to form a mature autophagosome which captures cytosolic cargo (Mizushima and Komatsu, 2011). Genetic studies in yeast have identified a series of autophagy-related genes (ATGs) shown to be essential for the autophagy process (Tsukada and Ohsumi, 1993; Klionsky et al., 2003). Among these, microtubule associated protein 1 light chain-3 (LC3), a homologue of yeast Atg8, is converted from a cytosolic form (LC3-I) to its phosphatidylethanolamine-conjugated form (LC3-II) which targets to autophagic membranes (Mizushima et al., 2010). Autophagosome formation is also regulated by the autophagy protein Beclin 1 (homolog of yeast Atg6; Liang et al., 1999).
The membrane origin of autophagosomes remains unclear. Although the endoplasmic reticulum (ER), mitochondria and plasma membrane have been reported as the membrane source, recent studies have also suggested that the ER-mitochondria contact site is important in autophagosome formation (Hailey et al., 2010; Ravikumar et al., 2010; Tooze and Yoshimori, 2010; Hamasaki et al., 2013). Subsequently, the autophagosome containing the cytosolic components and organelles fuses with the lysosome to form the autolysosome where the sequestered cargo is degraded (Mizushima and Komatsu, 2011). Initial studies on the molecular mechanisms of autophagy have largely focused on the early stage, however, precise mechanisms of the late stage where the autophagosome fuses with the lysosome have also been revealed (Shen and Mizushima, 2014). Recent studies have demonstrated that the transcription factor EB (TFEB), a master gene for lysosomal biogenesis, coordinates the autophagic process by driving expression of autophagy and lysosomal related genes (Settembre et al., 2011). An autophagosomal soluble N-ethylmaleimide-sensitive factor attachment protein receptor (SNARE) has been identified as the regulator of autophagosome–lysosome fusion (Itakura et al., 2012).
Once, autophagy was simply regarded as a non-specific degradation system, however, recent research shows that autophagy can selectively degrade specific targets in processes referred to as “selective autophagy” (Levine et al., 2011; Youle and Narendra, 2011). Each selective autophagy subtype was named after its specific targets, for example: aggregated proteins (aggrephagy; Yamamoto and Simonsen, 2011), mitochondria (mitophagy; Youle and Narendra, 2011), pathogens (xenophagy; Levine et al., 2011), and cilia (ciliophagy; Cloonan et al., 2014). Selective autophagy is also related to vesicle trafficking pathways, and its importance in secretion and other intracellular transport processes is rapidly increasing (Stolz et al., 2014).
Previous studies suggest that autophagy is relevant to human diseases, including pulmonary diseases (Levine and Kroemer, 2008; Choi et al., 2013). Furthermore, convincing evidence that selective autophagy may be implicated in human disease has been reported (Gomes and Dikic, 2014; Redmann et al., 2014). This led us to the hypothesis that selective autophagy would impact the pathogenesis of pulmonary diseases. In this review, we will examine the considerable evidence emerging for the role of selective autophagy in the pathogenesis of complex pulmonary diseases. A better understanding of the role(s) of selective autophagy in disease pathogenesis may help design more specific therapies for the treatment of pulmonary diseases, and other diseases where autophagy may contribute to pathogenesis.
Selective Autophagy
Selective autophagy can deliver a wide range of cargo to the lysosome, including protein aggregates, whole organelles (e.g., mitochondria), and intracellular pathogens (Stolz et al., 2014). Although the mechanisms of selective degradation remain incompletely understood, several reports suggest that ubiquitination of substrates may serve as general tag for selective autophagy in mammalian cells (Kirkin et al., 2009). Recent studies have described important functions of Atg8 family proteins in selective autophagy, including interactions with cargo receptors and components of the basal autophagy machinery, and in the regulation of autophagosome biogenesis (Kaufmann et al., 2014; Nath et al., 2014; Sawa-Makarska et al., 2014). To evaluate the inclusive list of selective autophagy processes currently in the literature is beyond the scope of this review; we will therefore focus on the three types of selective autophagy most related to pulmonary diseases; mitophagy, xenophagy, and ciliophagy.
Mitophagy
Mitophagy is a selective mechanism for the elimination of mitochondria through the autophagic machinery (Youle and Narendra, 2011). Two major mitophagy-related proteins, Parkin and PTEN-induced putative kinase protein 1 (PINK1), have been linked to the pathogenesis of Parkinson’s disease (Youle and Narendra, 2011). A proposal for the mechanism of mitophagy is that damaged and depolarized mitochondria stabilize PINK1 which in turn recruits the E3 ubiquitin ligase, Parkin. Parkin then ubiquitinylates various mitochondrial outer membrane proteins including mitofusins MFN1, MFN2 (Gegg et al., 2010), voltage dependent anion channel (VDAC; Geisler et al., 2010) and mitochondrial rho GTPase (MIRO; Wang et al., 2011); and induces mitophagy by the recruitment of autophagy receptors such as p62 (Geisler et al., 2010). However, several previous reports are suggestive of PINK1-dependent, but Parkin-independent, mitophagy. Gp78 E3 ubiquitin ligase overexpression causes mitophagy that is independent of Parkin (Fu et al., 2013). Mice genetically deficient in Pink1 were resistant to Staphylococcus aureus-induced acute lung injury (ALI). PINK1 was found to interact with an alternative ubiquitin E3 ligase component, F-box only protein 15 (Fbxo15), which promoted mitochondrial instability in this model (Chen et al., 2014a). Furthermore, although mitophagy was generally considered to serve as an intrinsic mitochondrial quality control system, it has been reported that mitophagy may trigger cell death (Sentelle et al., 2012; Mizumura et al., 2014). Now, mitophagy is generally recognized as a potential modulator of the pathogenesis of disease with either protective or harmful consequences.
Xenophagy
Autophagy can contribute to the immune response by providing a mechanism for the selective intracellular degradation of invading pathogens, a process termed “xenophagy.” Invading bacteria are tagged for removal with ubiquitin. Autophagy receptors including p62, nuclear domain 10 protein 52 (NDP52) and optineurin recognize ubiquitinated pathogens and target them to autophagosomes (Gomes and Dikic, 2014). Besides its direct role in pathogen clearance, xenophagy may also serve host defenses by enhancing immune recognition of infected cells via the generation of antigenic bacterial peptides (Yano and Kurata, 2011). Meanwhile, some bacteria, such as S. aureus and Anaplasma phagocytophilum, can use the host autophagosomes for replication (Schnaith et al., 2007; Niu et al., 2008). These bacteria can not only block autophagosomal maturation and acidification, but also can proliferate in LC3-positive compartments (Gomes and Dikic, 2014).
Ciliophagy
Recently, we have demonstrated that an autophagy-dependent pathway regulates cilia length (Lam et al., 2013), a process named “ciliophagy.” We have shown that the cytosolic deacetylase histone deacetylase 6 (HDAC6) mediates ciliophagy. Pampliega et al. (2013) also reported that autophagy negatively regulate ciliogenesis by degrading intraflagellar transport protein 20 homolog (IFT20). On the other hand, it has reported that autophagy removes oral-facial-digital syndrome 1 protein (OFD1) from centriolar satellites to promote ciliogenesis (Tang et al., 2013). However, the precise mechanisms by which autophagy can regulate these conflicting processes remains to be elucidated (Wrighton, 2013).
Selective Autophagy in COPD
Chronic obstructive pulmonary disease (COPD) contributes significantly to the global burden of disease as the fourth leading cause of mortality worldwide, however, the pathogenesis of this disease remains incompletely understood (Dal-Re, 2011; Vestbo et al., 2013). We previously reported increased autophagosome numbers and increased expression of LC3B-II, the active form of LC3B, in human lung specimens from patients with COPD (Chen et al., 2010). In an in vivo emphysema model, genetic deletion of specific autophagy proteins reduced airspace enlargement (Chen et al., 2010). More recently, we demonstrated that mitophagy regulates necroptosis, a form of programmed necrosis, which contributes to the pathogenesis of COPD (Mizumura et al., 2014). Cigarette smoke (CS) exposure induced mitophagy through the stabilization of the mitophagy regulator PINK1 in pulmonary epithelial cells. Mice genetically deficient in PINK1 were protected against mitochondrial dysfunction, airspace enlargement, and mucociliary clearance (MCC) disruption during CS exposure. The mitochondrial division/mitophagy inhibitor Mdivi-1 protected against CS-induced cell death and mitochondrial dysfunction, and reduced the phosphorylation of mixed lineage kinase domain-like protein (MLKL), a substrate for receptor-interacting serine/threonine-protein kinase 3 (RIP3) in the necroptosis pathway. In this study, we have shown that significant mitochondrial depolarization occurred in pulmonary epithelial cells in response to CS extract (CSE) exposure. Moreover, our results suggest that active mitophagy may alter mitochondrial membrane integrity, and lead to the induction of necroptosis. However, the precise mechanism by which mitophagy can serve to aggravate mitochondrial injury in the CS exposure model remains obscure. One possible hypothesis is that CS-induced aberrant mitophagy may cause an increase in the population of impaired mitochondria (Figure 1A). In addition, as dose-response effects of autophagy/mitophagy have been proposed, we cannot completely exclude the possibility that mitophagy may also contribute to mitochondrial quality control for its pro-survival role during mild CS exposure (Frank et al., 2012; Sureshbabu and Bhandari, 2013; Schiavi and Ventura, 2014). Further studies are necessary to improve the understanding of the role of mitophagy in the pathogenesis of COPD.
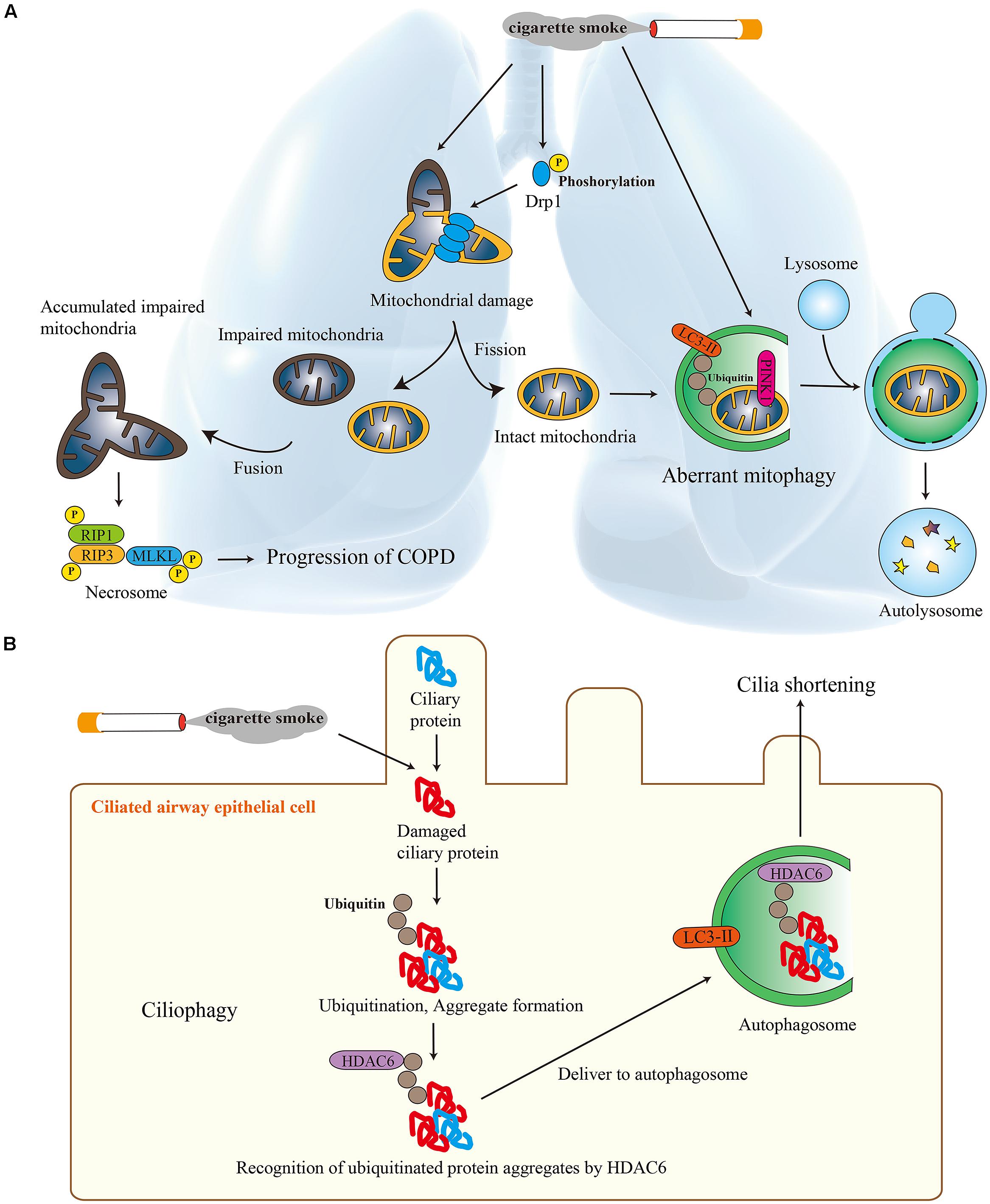
FIGURE 1. Selective autophagy in chronic obstructive pulmonary disease (COPD). (A) The role of mitophagy in COPD. Cigarette smoke (CS) induced mitochondrial fission and PINK1-dependent mitophagy in epithelial cells independently from mitochondrial damage. This aberrant mitophagy may cause the increase in the population of impaired mitochondria, which leads to the initiation of necroptosis. (B) The role of ciliophagy in COPD. CS induces oxidative stress, which causes cilia protein damage. Damaged cilia proteins are ubiquitinated which promotes aggregate formation. HDAC6 recognizes ubiquitinated protein aggregates and delivers them to autophagosomes. This degradation of cilia proteins, through an autophagy-dependent process termed “ciliophagy,” was associated with cilia shortening.
We also reported that ciliophagy, the consumption of cilia components by autophagy, regulates cilia length during CS exposure (Figure 1B; Lam et al., 2013; Cloonan et al., 2014). Impaired airway clearance caused by cilia shortening prevents the elimination of pathogens from the airways and may cause recurrent respiratory infections that exacerbate COPD. We demonstrated that autophagy-impaired (Becn1+/- or Map1lc3B-/-) mice, as well as tracheal epithelial cells isolated from these mice, resisted CS-induced cilia shortening. We identified the cytosolic deacetylase HDAC6 as a critical regulator of autophagy-mediated cilia shortening during CS exposure (Lam et al., 2013; Cloonan et al., 2014).
In contrast, previous studies have demonstrated defective autophagy in CS-exposed macrophages (Monick et al., 2010). Such a deficit in autophagy/xenophagy was observed in the alveolar macrophages of smokers and was proposed to lead to recurrent infections in smokers, since CS exposure impairs delivery of bacteria to the lysosomes.
Selective Autophagy in Respiratory Infection and Sepsis
The mechanism of antibacterial autophagy in Mycobacterium tuberculosis (Mtb) infection is well-characterized. The lungs are the major site for Mtb infection. Mtb employs a unique strategy for survival that interferes with the fusion between phagosomal compartments containing Mtb and lysosomes (Vergne et al., 2004). Despite the availability of anti-TB drugs, recent reports have identified cases of totally drug-resistant TB (Loewenberg, 2012; Udwadia et al., 2012). Since new therapeutic agents that have different mechanisms of action from conventional anti-TB drugs are needed to prevent the development of drug resistance, bacterial autophagy (xenophagy) has drawn attention as a candidate therapeutic target. Previous studies have demonstrated that polymorphisms in the immunity-related GTPase family M protein (IRGM) gene are linked to increased susceptibility to Mtb infection, and that IFN-γ induced IRGM regulates autophagy to eliminate mycobacteria in human macrophages (Figure 2A; Singh et al., 2006; Intemann et al., 2009). Recent studies have revealed that Mtb extracellular DNA activates ubiquitin-mediated selective autophagy through phagosomal permeabilization (Figure 2B; Watson et al., 2012). The bacterial early secretory antigenic target 6 (ESAT-6) system 1 (ESX-1) secretion system mediates phagosomal permeabilization to enable the ubiquitin-mediated autophagy pathway access to phagosomal Mtb. The stimulator of interferon genes (STING)-dependent cytosolic pathway recognizes extracellular bacterial DNA and tags bacteria with ubiquitin. Autophagy receptors, p62, and NDP52, recognize ubiquitinated Mtb and target them to autophagosomes. Several therapies that involve enhancing autophagy activity also have been proposed to be effective against Mtb infection. The antiprotozoal drug nitazoxanide and its active metabolite tizoxanide strongly stimulate autophagy through inhibition of mTORC1 signaling, which in turn prevents intracellular proliferation of Mtb (Lam et al., 2012). Vitamin D has revealed therapeutic benefits in persons with HIV and Mtb infection through the activation of autophagy (Campbell and Spector, 2012).
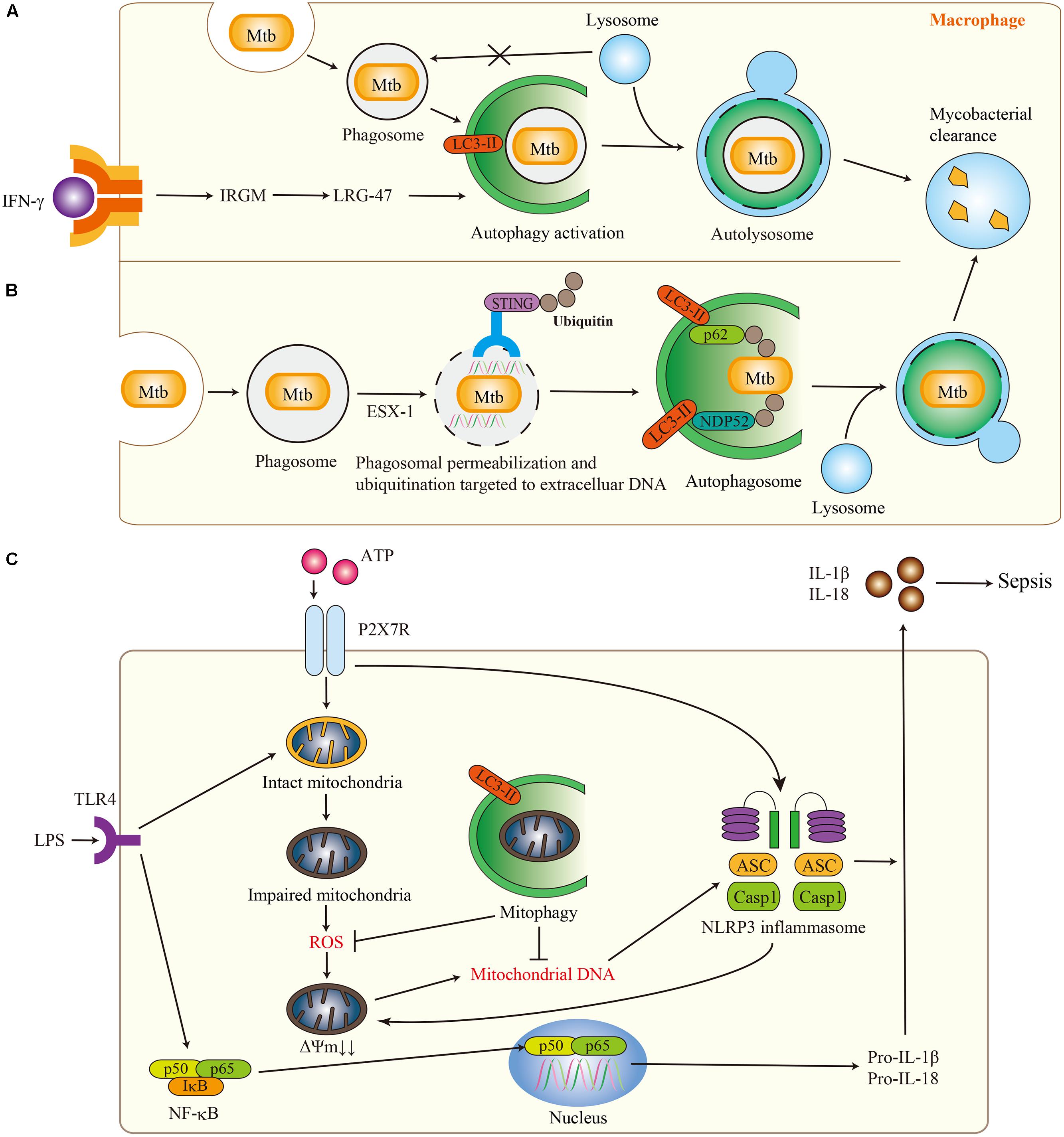
FIGURE 2. Selective autophagy in respiratory infection and sepsis. (A) The role of autophagy in Mycobacterium tuberculosis (Mtb) infection. IFN-γ induced IRGM activates autophagy and eliminates Mtb to outstrip the interference of fusion between phagosomal compartments containing Mtb and lysosomes. (B) The role of selective autophagy in Mtb infection. The bacterial ESX-1 secretion system mediates phagosomal permeabilization to enable the ubiquitin-mediated autophagy pathway to access phagosomal Mtb. Ubiquitinated Mtb is recognized by p62 and NDP52 as a target of selective autophagy. (C) The role of mitophagy in sepsis. The release of mitochondrial DNA (mtDNA) into the cytosol depends on the NLRP3 inflammasome and mitochondrial ROS. Cytosolic mtDNA contributes to the secretion of IL-1β and IL-18 in response to LPS and ATP. Mitophagy limits the secretion of IL-1β and IL-18 by targeting impaired mitochondria.
Autophagy has been implicated in the regulation of inflammation, particularly the regulation of the inflammasome pathway. Inflammasomes represent an inflammatory signaling platform activated by infection or stress that regulate the maturation and secretion of pro-inflammatory cytokines (e.g., IL-1β and IL-18; Schroder and Tschopp, 2010). Along with Zhou et al, we have demonstrated that suppression of autophagy causes the accumulation of damaged ROS-producing mitochondria, whereas activates the NLRP3 inflammasome (Figure 2C; Nakahira et al., 2011; Zhou et al., 2011). In this study, we also found that the NLRP3 inflammasome and mitochondrial ROS production regulate cytosolic translocation of mitochondrial DNA (mtDNA) in macrophages, which in turn contributed to the secretion of IL-1β and IL-18. Importantly, these findings are consistent with the observation that IL-1β and IL-18 are increased in patients with sepsis in the medical intensive care unit (ICU; Nakahira et al., 2011; Dolinay et al., 2012). Moreover, we demonstrated that increased mtDNA levels in plasma are associated with ICU mortality, and inclusion of mtDNA level improves risk prediction in medical ICU patients (Nakahira et al., 2013). Recently, we have also demonstrated that carbon monoxide (CO) confers protection in sepsis by enhancing Beclin 1-dependent autophagy and phagocytosis (Lee et al., 2014). CO enhanced bacterial phagocytosis in Becn1+/+ but not Becn1+/- mice in vivo and in corresponding cultured macrophages, which indicates that CO may induce xenophagy. These results suggest that CO gas may represent a novel therapy for patients with sepsis.
Selective Autophagy in Hyperoxia and Acute Lung Injury
We have demonstrated that autophagy is implicated in the pathogenesis of ALI (Tanaka et al., 2012). Although mechanical ventilation with high concentrations of oxygen is required to manage patients with severe respiratory failure, prolonged exposure to hyperoxia can result in lung injury. Hyperoxia can induce autophagy activity. Depletion of LC3B by RNA interference reduced cell viability under hyperoxic conditions (Tanaka et al., 2012). We also investigated the molecular mechanism by which autophagy can confer cytoprotection in lung epithelial cells after hyperoxia (Liang et al., 2013). Cellular homeostasis requires the constant formation of the p62/LC3B/truncated BH3-interacting domain death agonist (tBID) complex under normal conditions, however, hyperoxia leads to dissociation of the p62/LC3B/tBID complex, which stops the translocation of tBID into lysosome for degradation. Increased tBID causes cytochrome c release from the mitochondria and subsequent caspase-dependent cell death. These results suggest that the autophagy may have protective function during the pathogenesis of ALI, especially under hyperoxia.
It has been reported that mechanical ventilation and hyperoxia cause pulmonary mitochondrial dysfunction (Ratner et al., 2009, 2013; Waxman and Kolliputi, 2009). Given that mitochondrial damage can induce mitophagy, it is reasonable to presume that mitophagy may play a role in the pathogenesis of ALI (Youle and Narendra, 2011). Indeed, the role of mitophagy in hyperoxia has been reported. Genetic deletion of PINK1 or PINK1 silencing in the lung endothelium increased susceptibility to hyperoxia via alterations in autophagy/mitophagy (Zhang et al., 2014). NLRP3 may regulate autophagy/mitophagy via PINK1 during hyperoxia. Consistent with a role for autophagy, these results also suggest that mitophagy may have protective function during the pathogenesis of ALI.
Selective Autophagy in the Hypoxia Response and Pulmonary Hypertension
Hypoxia results in secondary pulmonary hypertension (PH). Hypoxic PH is a progressive and often fatal complication of chronic lung disease (Semenza, 2011). Chronic hypoxia induces pulmonary arterial vascular smooth muscle (PAVSM) cell proliferation, which is a major cause of PH (Stenmark et al., 2006). We previously demonstrated that elevated occurrences of autophagy have been observed in lung tissue from patients with PH. Mice genetically deficient in LC3B demonstrated increased indices of PH after chronic hypoxia (Lee et al., 2011). Chloroquine, the inhibitor of autophagy, has been reported to prevent progression of experimental PH (Long et al., 2013). Furthermore, as the inhibition of mTOR complex 1 (mTORC1) has been shown to induce autophagy, previous studies demonstrated that blockade of mTORC1 has anti-proliferative effects on pulmonary vascular cells (Krymskaya et al., 2011; Wang et al., 2014). These results suggest that autophagy may have a protective function during the pathogenesis of PH. LC3 and mTOR pathway are attracting attention as potential therapeutic targets in hypoxia-induced PH (Lahm and Petrache, 2012; Goncharova, 2013).
The mitochondrial outer-membrane protein FUN14 domain-containing protein 1 (FUNDC1) mediates hypoxia-induced mitophagy in mammalian cells (Liu et al., 2012). FUNDC1 interacted with LC3; and knockdown of endogenous FUNDC1 significantly prevented hypoxia-induced mitophagy, which could be reversed by the expression of wild-type FUNDC1. Hypoxia can dephosphorylate FUNDC1 at serine 13 through serine/threonine-protein phosphatase PGAM5 for the induction of mitophagy (Chen et al., 2014b; Wu et al., 2014). These results suggest that mitophagy may contribute to mitochondrial quality control in hypoxia. However, hypoxia-induced mitophagy has also been reported to cause apoptosis in cardiomyocytes (Yan et al., 2014). The role of mitophagy in the pathogenesis of PH remained incompletely understood.
Conclusion
Although autophagy originally was considered as a simple bulk degradation system for cellular components, accumulating evidence demonstrates that autophagy can selectively degrade specific targets. Selective autophagy plays a complex role in human diseases where it can have both protective and injurious effects. However, when viewed in the light of evidence that many cellular functions can have both protective and injurious effects, it is likely that selective autophagy may also act as a double-edged-sword in the pathogenesis of human diseases. For future clinical applications, rather than intervention strategies to target general autophagy, the specific targeting of selective autophagy pathways may enhance the efficacy of therapeutic strategies. Further research into selective autophagy in the lung and other organs will allow for the development new therapeutic interventions.
Conflict of Interest Statement
The authors declare that the research was conducted in the absence of any commercial or financial relationships that could be construed as a potential conflict of interest.
References
Campbell, G. R., and Spector, S. A. (2012). Vitamin D inhibits human immunodeficiency virus type 1 and Mycobacterium tuberculosis infection in macrophages through the induction of autophagy. PLoS Pathog. 8:e1002689. doi: 10.1371/journal.ppat.1002689
Pubmed Abstract | Pubmed Full Text | CrossRef Full Text | Google Scholar
Chen, B. B., Coon, T. A., Glasser, J. R., Zou, C., Ellis, B., Das, T.,et al. (2014a). E3 ligase subunit Fbxo15 and PINK1 kinase regulate cardiolipin synthase 1 stability and mitochondrial function in pneumonia. Cell Rep. 7, 476–487. doi: 10.1016/j.celrep.2014.02.048
Pubmed Abstract | Pubmed Full Text | CrossRef Full Text | Google Scholar
Chen, G., Han, Z., Feng, D., Chen, Y., Chen, L., Wu, H.,et al. (2014b). A regulatory signaling loop comprising the PGAM5 phosphatase and CK2 controls receptor-mediated mitophagy. Mol. Cell 54, 362–377. doi: 10.1016/j.molcel.2014.02.034
Pubmed Abstract | Pubmed Full Text | CrossRef Full Text | Google Scholar
Chen, Z. H., Lam, H. C., Jin, Y., Kim, H. P., Cao, J., Lee, S. J.,et al. (2010). Autophagy protein microtubule-associated protein 1 light chain-3B (LC3B) activates extrinsic apoptosis during cigarette smoke-induced emphysema. Proc. Natl. Acad. Sci. U.S.A. 107, 18880–18885. doi: 10.1073/pnas.1005574107
Pubmed Abstract | Pubmed Full Text | CrossRef Full Text | Google Scholar
Choi, A. M., Ryter, S. W., and Levine, B. (2013). Autophagy in human health and disease. N. Engl. J. Med. 368, 1845–1846. doi: 10.1056/NEJMra1205406
Pubmed Abstract | Pubmed Full Text | CrossRef Full Text | Google Scholar
Cloonan, S. M., Lam, H. C., Ryter, S. W., and Choi, A. M. (2014). “Ciliophagy”: the consumption of cilia components by autophagy. Autophagy 10, 532–534. doi: 10.4161/auto.27641
Pubmed Abstract | Pubmed Full Text | CrossRef Full Text | Google Scholar
Dal-Re, R. (2011). Worldwide behavioral research on major global causes of mortality. Health Educ. Behav. 38, 433–440. doi: 10.1177/1090198111402197
Pubmed Abstract | Pubmed Full Text | CrossRef Full Text | Google Scholar
Dolinay, T., Kim, Y. S., Howrylak, J., Hunninghake, G. M., An, C. H., Fredenburgh, L.,et al. (2012). Inflammasome-regulated cytokines are critical mediators of acute lung injury. Am. J. Respir. Crit. Care Med. 185, 1225–1234. doi: 10.1164/rccm.201201-0003OC
Pubmed Abstract | Pubmed Full Text | CrossRef Full Text | Google Scholar
Frank, M., Duvezin-Caubet, S., Koob, S., Occhipinti, A., Jagasia, R., Petcherski, A.,et al. (2012). Mitophagy is triggered by mild oxidative stress in a mitochondrial fission dependent manner. Biochim. Biophys. Acta 1823, 2297–2310. doi: 10.1016/j.bbamcr.2012.08.007
Pubmed Abstract | Pubmed Full Text | CrossRef Full Text | Google Scholar
Fu, M., St-Pierre, P., Shankar, J., Wang, P. T., Joshi, B., and Nabi, I. R. (2013). Regulation of mitophagy by the Gp78 E3 ubiquitin ligase. Mol. Biol. Cell 24, 1153–1162. doi: 10.1091/mbc.E12-08-0607
Pubmed Abstract | Pubmed Full Text | CrossRef Full Text | Google Scholar
Gegg, M. E., Cooper, J. M., Chau, K. Y., Rojo, M., Schapira, A. H., and Taanman, J. W. (2010). Mitofusin 1 and mitofusin 2 are ubiquitinated in a PINK1/parkin-dependent manner upon induction of mitophagy. Hum. Mol. Genet. 19, 4861–4870. doi: 10.1093/hmg/ddq419
Pubmed Abstract | Pubmed Full Text | CrossRef Full Text | Google Scholar
Geisler, S., Holmstrom, K. M., Skujat, D., Fiesel, F. C., Rothfuss, O. C., Kahle, P. J.,et al. (2010). PINK1/Parkin-mediated mitophagy is dependent on VDAC1 and p62/SQSTM1. Nat. Cell Biol. 12, 119–131. doi: 10.1038/ncb2012
Pubmed Abstract | Pubmed Full Text | CrossRef Full Text | Google Scholar
Gomes, L. C., and Dikic, I. (2014). Autophagy in antimicrobial immunity. Mol. Cell 54, 224–233. doi: 10.1016/j.molcel.2014.03.009
Pubmed Abstract | Pubmed Full Text | CrossRef Full Text | Google Scholar
Goncharova, E. A. (2013). mTOR and vascular remodeling in lung diseases: current challenges and therapeutic prospects. FASEB J. 27, 1796–1807. doi: 10.1096/fj.12-222224
Pubmed Abstract | Pubmed Full Text | CrossRef Full Text | Google Scholar
Hailey, D. W., Rambold, A. S., Satpute-Krishnan, P., Mitra, K., Sougrat, R., Kim, P. K.,et al. (2010). Mitochondria supply membranes for autophagosome biogenesis during starvation. Cell 141, 656–667. doi: 10.1016/j.cell.2010.04.009
Pubmed Abstract | Pubmed Full Text | CrossRef Full Text | Google Scholar
Hamasaki, M., Furuta, N., Matsuda, A., Nezu, A., Yamamoto, A., Fujita, N.,et al. (2013). Autophagosomes form at ER-mitochondria contact sites. Nature 495, 389–393. doi: 10.1038/nature11910
Pubmed Abstract | Pubmed Full Text | CrossRef Full Text | Google Scholar
Intemann, C. D., Thye, T., Niemann, S., Browne, E. N., Amanua Chinbuah, M., Enimil, A.,et al. (2009). Autophagy gene variant IRGM-261T contributes to protection from tuberculosis caused by Mycobacterium tuberculosis but not by M. africanum strains. PLoS Pathog. 5:e1000577. doi: 10.1371/journal.ppat.1000577
Pubmed Abstract | Pubmed Full Text | CrossRef Full Text | Google Scholar
Itakura, E., Kishi-Itakura, C., and Mizushima, N. (2012). The hairpin-type tail-anchored SNARE syntaxin 17 targets to autophagosomes for fusion with endosomes/lysosomes. Cell 151, 1256–1269. doi: 10.1016/j.cell.2012.11.001
Pubmed Abstract | Pubmed Full Text | CrossRef Full Text | Google Scholar
Kaufmann, A., Beier, V., Franquelim, H. G., and Wollert, T. (2014). Molecular mechanism of autophagic membrane-scaffold assembly and disassembly. Cell 156, 469–481. doi: 10.1016/j.cell.2013.12.022
Pubmed Abstract | Pubmed Full Text | CrossRef Full Text | Google Scholar
Kirkin, V., McEwan, D. G., Novak, I., and Dikic, I. (2009). A role for ubiquitin in selective autophagy. Mol. Cell 34, 259–269. doi: 10.1016/j.molcel.2009.04.026
Pubmed Abstract | Pubmed Full Text | CrossRef Full Text | Google Scholar
Klionsky, D. J., Cregg, J. M., Dunn, W. A. Jr., Emr, S. D., Sakai, Y., Sandoval, I. V.,et al. (2003). A unified nomenclature for yeast autophagy-related genes. Dev. Cell 5, 539–545. doi: 10.1016/S1534-5807(03)00296-X
Pubmed Abstract | Pubmed Full Text | CrossRef Full Text | Google Scholar
Krymskaya, V. P., Snow, J., Cesarone, G., Khavin, I., Goncharov, D. A., Lim, P. N.,et al. (2011). mTOR is required for pulmonary arterial vascular smooth muscle cell proliferation under chronic hypoxia. FASEB J. 25, 1922–1933. doi: 10.1096/fj.10-175018
Pubmed Abstract | Pubmed Full Text | CrossRef Full Text | Google Scholar
Lahm, T., and Petrache, I. (2012). LC3 as a potential therapeutic target in hypoxia-induced pulmonary hypertension. Autophagy 8, 1146–1147. doi: 10.4161/auto.20520
Pubmed Abstract | Pubmed Full Text | CrossRef Full Text | Google Scholar
Lam, H. C., Cloonan, S. M., Bhashyam, A. R., Haspel, J. A., Singh, A., Sathirapongsasuti, J. F.,et al. (2013). Histone deacetylase 6-mediated selective autophagy regulates COPD-associated cilia dysfunction. J. Clin. Invest. 123, 5212–5230. doi: 10.1172/JCI69636
Pubmed Abstract | Pubmed Full Text | CrossRef Full Text | Google Scholar
Lam, K. K., Zheng, X., Forestieri, R., Balgi, A. D., Nodwell, M., Vollett, S.,et al. (2012). Nitazoxanide stimulates autophagy and inhibits mTORC1 signaling and intracellular proliferation of Mycobacterium tuberculosis. PLoS Pathog. 8:e1002691. doi: 10.1371/journal.ppat.1002691
Pubmed Abstract | Pubmed Full Text | CrossRef Full Text | Google Scholar
Lee, S., Lee, S. J., Coronata, A. A., Fredenburgh, L. E., Chung, S. W., Perrella, M. A.,et al. (2014). Carbon monoxide confers protection in sepsis by enhancing beclin 1-dependent autophagy and phagocytosis. Antioxid. Redox Signal. 20, 432–442. doi: 10.1089/ars.2013.5368
Pubmed Abstract | Pubmed Full Text | CrossRef Full Text | Google Scholar
Lee, S. J., Smith, A., Guo, L., Alastalo, T. P., Li, M., Sawada, H.,et al. (2011). Autophagic protein LC3B confers resistance against hypoxia-induced pulmonary hypertension. Am. J. Respir. Crit. Care Med. 183, 649–658. doi: 10.1164/rccm.201005-0746OC
Pubmed Abstract | Pubmed Full Text | CrossRef Full Text | Google Scholar
Levine, B., and Kroemer, G. (2008). Autophagy in the pathogenesis of disease. Cell 132, 27–42. doi: 10.1016/j.cell.2007.12.018
Pubmed Abstract | Pubmed Full Text | CrossRef Full Text | Google Scholar
Levine, B., Mizushima, N., and Virgin, H. W. (2011). Autophagy in immunity and inflammation. Nature 469, 323–335. doi: 10.1038/nature09782
Pubmed Abstract | Pubmed Full Text | CrossRef Full Text | Google Scholar
Liang, X., Wei, S. Q., Lee, S. J., Fung, J. K., Zhang, M., Tanaka, A.,et al. (2013). p62 sequestosome 1/light chain 3b complex confers cytoprotection on lung epithelial cells after hyperoxia. Am. J. Respir. Cell Mol. Biol. 48, 489–496. doi: 10.1165/rcmb.2012-0017OC
Pubmed Abstract | Pubmed Full Text | CrossRef Full Text | Google Scholar
Liang, X. H., Jackson, S., Seaman, M., Brown, K., Kempkes, B., Hibshoosh, H.,et al. (1999). Induction of autophagy and inhibition of tumorigenesis by beclin 1. Nature 402, 672–676. doi: 10.1038/45257
Pubmed Abstract | Pubmed Full Text | CrossRef Full Text | Google Scholar
Liu, L., Feng, D., Chen, G., Chen, M., Zheng, Q., Song, P.,et al. (2012). Mitochondrial outer-membrane protein FUNDC1 mediates hypoxia-induced mitophagy in mammalian cells. Nat. Cell Biol. 14, 177–185. doi: 10.1038/ncb2422
Pubmed Abstract | Pubmed Full Text | CrossRef Full Text | Google Scholar
Loewenberg, S. (2012). India reports cases of totally drug-resistant tuberculosis. Lancet 379:205. doi: 10.1016/S0140-6736(12)60085-3
Long, L., Yang, X., Southwood, M., Lu, J., Marciniak, S. J., Dunmore, B. J.,et al. (2013). Chloroquine prevents progression of experimental pulmonary hypertension via inhibition of autophagy and lysosomal bone morphogenetic protein type II receptor degradation. Circ. Res. 112, 1159–1170. doi: 10.1161/CIRCRESAHA.111.300483
Pubmed Abstract | Pubmed Full Text | CrossRef Full Text | Google Scholar
Mizumura, K., Cloonan, S. M., Nakahira, K., Bhashyam, A. R., Cervo, M., Kitada, T.,et al. (2014). Mitophagy-dependent necroptosis contributes to the pathogenesis of COPD. J. Clin. Invest. 124, 3987–4003. doi: 10.1172/JCI74985
Pubmed Abstract | Pubmed Full Text | CrossRef Full Text | Google Scholar
Mizushima, N., and Komatsu, M. (2011). Autophagy: renovation of cells and tissues. Cell 147, 728–741. doi: 10.1016/j.cell.2011.10.026
Pubmed Abstract | Pubmed Full Text | CrossRef Full Text | Google Scholar
Mizushima, N., Yoshimori, T., and Levine, B. (2010). Methods in Mammalian autophagy research. Cell 140, 313–326. doi: 10.1016/j.cell.2010.01.028
Pubmed Abstract | Pubmed Full Text | CrossRef Full Text | Google Scholar
Monick, M. M., Powers, L. S., Walters, K., Lovan, N., Zhang, M., Gerke, A.,et al. (2010). Identification of an autophagy defect in smokers’ alveolar macrophages. J. Immunol. 185, 5425–5435. doi: 10.4049/jimmunol.1001603
Pubmed Abstract | Pubmed Full Text | CrossRef Full Text | Google Scholar
Nakahira, K., Haspel, J. A., Rathinam, V. A., Lee, S. J., Dolinay, T., Lam, H. C.,et al. (2011). Autophagy proteins regulate innate immune responses by inhibiting the release of mitochondrial DNA mediated by the NALP3 inflammasome. Nat. Immunol. 12, 222–230. doi: 10.1038/ni.1980
Pubmed Abstract | Pubmed Full Text | CrossRef Full Text | Google Scholar
Nakahira, K., Kyung, S. Y., Rogers, A. J., Gazourian, L., Youn, S., Massaro, A. F.,et al. (2013). Circulating mitochondrial DNA in patients in the ICU as a marker of mortality: derivation and validation. PLoS Med. 10:e1001577; discussion e1001577. doi: 10.1371/journal.pmed.1001577
Pubmed Abstract | Pubmed Full Text | CrossRef Full Text | Google Scholar
Nath, S., Dancourt, J., Shteyn, V., Puente, G., Fong, W. M., Nag, S.,et al. (2014). Lipidation of the LC3/GABARAP family of autophagy proteins relies on a membrane-curvature-sensing domain in Atg3. Nat. Cell Biol. 16, 415–424. doi: 10.1038/ncb2940
Pubmed Abstract | Pubmed Full Text | CrossRef Full Text | Google Scholar
Niu, H., Yamaguchi, M., and Rikihisa, Y. (2008). Subversion of cellular autophagy by Anaplasma phagocytophilum. Cell. Microbiol. 10, 593–605. doi: 10.1111/j.1462-5822.2007.01068.x
Pubmed Abstract | Pubmed Full Text | CrossRef Full Text | Google Scholar
Pampliega, O., Orhon, I., Patel, B., Sridhar, S., Diaz-Carretero, A., Beau, I.,et al. (2013). Functional interaction between autophagy and ciliogenesis. Nature 502, 194–200. doi: 10.1038/nature12639
Pubmed Abstract | Pubmed Full Text | CrossRef Full Text | Google Scholar
Ratner, V., Sosunov, S. A., Niatsetskaya, Z. V., Utkina-Sosunova, I. V., and Ten, V. S. (2013). Mechanical ventilation causes pulmonary mitochondrial dysfunction and delayed alveolarization in neonatal mice. Am. J. Respir. Cell Mol. Biol. 49, 943–950. doi: 10.1165/rcmb.2012-0172OC
Pubmed Abstract | Pubmed Full Text | CrossRef Full Text | Google Scholar
Ratner, V., Starkov, A., Matsiukevich, D., Polin, R. A., and Ten, V. S. (2009). Mitochondrial dysfunction contributes to alveolar developmental arrest in hyperoxia-exposed mice. Am. J. Respir. Cell Mol. Biol. 40, 511–518. doi: 10.1165/rcmb.2008-0341RC
Pubmed Abstract | Pubmed Full Text | CrossRef Full Text | Google Scholar
Ravikumar, B., Moreau, K., Jahreiss, L., Puri, C., and Rubinsztein, D. C. (2010). Plasma membrane contributes to the formation of pre-autophagosomal structures. Nat. Cell Biol. 12, 747–757. doi: 10.1038/ncb2078
Pubmed Abstract | Pubmed Full Text | CrossRef Full Text | Google Scholar
Redmann, M., Dodson, M., Boyer-Guittaut, M., Darley-Usmar, V., and Zhang, J. (2014). Mitophagy mechanisms and role in human diseases. Int. J. Biochem. Cell Biol. 53, 127–133. doi: 10.1016/j.biocel.2014.05.010
Pubmed Abstract | Pubmed Full Text | CrossRef Full Text | Google Scholar
Sawa-Makarska, J., Abert, C., Romanov, J., Zens, B., Ibiricu, I., and Martens, S. (2014). Cargo binding to Atg19 unmasks additional Atg8 binding sites to mediate membrane-cargo apposition during selective autophagy. Nat. Cell Biol. 16, 425–433. doi: 10.1038/ncb2935
Pubmed Abstract | Pubmed Full Text | CrossRef Full Text | Google Scholar
Schiavi, A., and Ventura, N. (2014). The interplay between mitochondria and autophagy and its role in the aging process. Exp. Gerontol. 56, 147–153. doi: 10.1016/j.exger.2014.02.015
Pubmed Abstract | Pubmed Full Text | CrossRef Full Text | Google Scholar
Schnaith, A., Kashkar, H., Leggio, S. A., Addicks, K., Kronke, M., and Krut, O. (2007). Staphylococcus aureus subvert autophagy for induction of caspase-independent host cell death. J. Biol. Chem. 282, 2695–2706. doi: 10.1074/jbc.M609784200
Pubmed Abstract | Pubmed Full Text | CrossRef Full Text | Google Scholar
Schroder, K., and Tschopp, J. (2010). The inflammasomes. Cell 140, 821–832. doi: 10.1016/j.cell.2010.01.040
Pubmed Abstract | Pubmed Full Text | CrossRef Full Text | Google Scholar
Semenza, G. L. (2011). Oxygen sensing, homeostasis, and disease. N. Engl. J. Med. 365, 537–547. doi: 10.1056/NEJMra1011165
Pubmed Abstract | Pubmed Full Text | CrossRef Full Text | Google Scholar
Sentelle, R. D., Senkal, C. E., Jiang, W., Ponnusamy, S., Gencer, S., Selvam, S. P.,et al. (2012). Ceramide targets autophagosomes to mitochondria and induces lethal mitophagy. Nat. Chem. Biol. 8, 831–838. doi: 10.1038/nchembio.1059
Pubmed Abstract | Pubmed Full Text | CrossRef Full Text | Google Scholar
Settembre, C., Di Malta, C., Polito, V. A., Garcia Arencibia, M., Vetrini, F., Erdin, S.,et al. (2011). TFEB links autophagy to lysosomal biogenesis. Science 332, 1429–1433. doi: 10.1126/science.1204592
Pubmed Abstract | Pubmed Full Text | CrossRef Full Text | Google Scholar
Shen, H. M., and Mizushima, N. (2014). At the end of the autophagic road: an emerging understanding of lysosomal functions in autophagy. Trends Biochem. Sci. 39, 61–71. doi: 10.1016/j.tibs.2013.12.001
Pubmed Abstract | Pubmed Full Text | CrossRef Full Text | Google Scholar
Singh, S. B., Davis, A. S., Taylor, G. A., and Deretic, V. (2006). Human IRGM induces autophagy to eliminate intracellular mycobacteria. Science 313, 1438–1441. doi: 10.1126/science.1129577
Pubmed Abstract | Pubmed Full Text | CrossRef Full Text | Google Scholar
Stenmark, K. R., Fagan, K. A., and Frid, M. G. (2006). Hypoxia-induced pulmonary vascular remodeling: cellular and molecular mechanisms. Circ. Res. 99, 675–691. doi: 10.1161/01.RES.0000243584.45145.3f
Pubmed Abstract | Pubmed Full Text | CrossRef Full Text | Google Scholar
Stolz, A., Ernst, A., and Dikic, I. (2014). Cargo recognition and trafficking in selective autophagy. Nat. Cell Biol. 16, 495–501. doi: 10.1038/ncb2979
Pubmed Abstract | Pubmed Full Text | CrossRef Full Text | Google Scholar
Sureshbabu, A., and Bhandari, V. (2013). Targeting mitochondrial dysfunction in lung diseases: emphasis on mitophagy. Front. Physiol. 4:384. doi: 10.3389/fphys.2013.00384
Pubmed Abstract | Pubmed Full Text | CrossRef Full Text | Google Scholar
Tanaka, A., Jin, Y., Lee, S. J., Zhang, M., Kim, H. P., Stolz, D. B.,et al. (2012). Hyperoxia-induced LC3B interacts with the Fas apoptotic pathway in epithelial cell death. Am. J. Respir. Cell Mol. Biol. 46, 507–514. doi: 10.1165/rcmb.2009-0415OC
Pubmed Abstract | Pubmed Full Text | CrossRef Full Text | Google Scholar
Tang, Z., Lin, M. G., Stowe, T. R., Chen, S., Zhu, M., Stearns, T.,et al. (2013). Autophagy promotes primary ciliogenesis by removing OFD1 from centriolar satellites. Nature 502, 254–257. doi: 10.1038/nature12606
Pubmed Abstract | Pubmed Full Text | CrossRef Full Text | Google Scholar
Tooze, S. A., and Yoshimori, T. (2010). The origin of the autophagosomal membrane. Nat. Cell Biol. 12, 831–835. doi: 10.1038/ncb0910-831
Pubmed Abstract | Pubmed Full Text | CrossRef Full Text | Google Scholar
Tsukada, M., and Ohsumi, Y. (1993). Isolation and characterization of autophagy-defective mutants of Saccharomyces cerevisiae. FEBS Lett. 333, 169–174. doi: 10.1016/0014-5793(93)80398-E
Udwadia, Z. F., Amale, R. A., Ajbani, K. K., and Rodrigues, C. (2012). Totally drug-resistant tuberculosis in India. Clin. Infect. Dis. 54, 579–581. doi: 10.1093/cid/cir889
Pubmed Abstract | Pubmed Full Text | CrossRef Full Text | Google Scholar
Vergne, I., Chua, J., Singh, S. B., and Deretic, V. (2004). Cell biology of Mycobacterium tuberculosis phagosome. Annu. Rev. Cell Dev. Biol. 20, 367–394. doi: 10.1146/annurev.cellbio.20.010403.114015
Pubmed Abstract | Pubmed Full Text | CrossRef Full Text | Google Scholar
Vestbo, J., Hurd, S. S., Agusti, A. G., Jones, P. W., Vogelmeier, C., Anzueto, A.,et al. (2013). Global strategy for the diagnosis, management, and prevention of chronic obstructive pulmonary disease: GOLD executive summary. Am. J. Respir. Crit. Care Med. 187, 347–365. doi: 10.1164/rccm.201204-0596PP
Pubmed Abstract | Pubmed Full Text | CrossRef Full Text | Google Scholar
Wang, W., Liu, J., Ma, A., Miao, R., Jin, Y., Zhang, H.,et al. (2014). mTORC1 Is Involved in hypoxia-induced pulmonary hypertension through the activation of Notch3. J. Cell. Physiol. 229, 2117–2125. doi: 10.1002/jcp.24670
Pubmed Abstract | Pubmed Full Text | CrossRef Full Text | Google Scholar
Wang, X., Winter, D., Ashrafi, G., Schlehe, J., Wong, Y. L., Selkoe, D.,et al. (2011). PINK1 and Parkin target Miro for phosphorylation and degradation to arrest mitochondrial motility. Cell 147, 893–906. doi: 10.1016/j.cell.2011.10.018
Pubmed Abstract | Pubmed Full Text | CrossRef Full Text | Google Scholar
Watson, R. O., Manzanillo, P. S., and Cox, J. S. (2012). Extracellular M. tuberculosis DNA targets bacteria for autophagy by activating the host DNA-sensing pathway. Cell 150, 803–815. doi: 10.1016/j.cell.2012.06.040
Pubmed Abstract | Pubmed Full Text | CrossRef Full Text | Google Scholar
Waxman, A. B., and Kolliputi, N. (2009). IL-6 protects against hyperoxia-induced mitochondrial damage via Bcl-2-induced Bak interactions with mitofusins. Am. J. Respir. Cell Mol. Biol. 41, 385–396. doi: 10.1165/rcmb.2008-0302OC
Pubmed Abstract | Pubmed Full Text | CrossRef Full Text | Google Scholar
Wrighton, K. H. (2013). Cytoskeleton: autophagy and ciliogenesis come together. Nat. Rev. Mol. Cell Biol. 14:687. doi: 10.1038/nrm3686
Pubmed Abstract | Pubmed Full Text | CrossRef Full Text | Google Scholar
Wu, H., Xue, D., Chen, G., Han, Z., Huang, L., Zhu, C.,et al. (2014). The BCL2L1 and PGAM5 axis defines hypoxia-induced receptor-mediated mitophagy. Autophagy 10, 1712–1725. doi: 10.4161/auto.29568
Pubmed Abstract | Pubmed Full Text | CrossRef Full Text | Google Scholar
Yamamoto, A., and Simonsen, A. (2011). The elimination of accumulated and aggregated proteins: a role for aggrephagy in neurodegeneration. Neurobiol. Dis. 43, 17–28. doi: 10.1016/j.nbd.2010.08.015
Pubmed Abstract | Pubmed Full Text | CrossRef Full Text | Google Scholar
Yan, L., Li, Y., Duan, H., Yang, H., Wu, J., Qian, P.,et al. (2014). Regulator of calcineurin 1-1L protects cardiomyocytes against hypoxia-induced apoptosis via mitophagy. J. Cardiovasc. Pharmacol. 64, 310–317. doi: 10.1097/FJC.0000000000000121
Pubmed Abstract | Pubmed Full Text | CrossRef Full Text | Google Scholar
Yano, T., and Kurata, S. (2011). Intracellular recognition of pathogens and autophagy as an innate immune host defence. J. Biochem. 150, 143–149. doi: 10.1093/jb/mvr083
Pubmed Abstract | Pubmed Full Text | CrossRef Full Text | Google Scholar
Youle, R. J., and Narendra, D. P. (2011). Mechanisms of mitophagy. Nat. Rev. Mol. Cell Biol. 12, 9–14. doi: 10.1038/nrm3028
Pubmed Abstract | Pubmed Full Text | CrossRef Full Text | Google Scholar
Zhang, Y., Sauler, M., Shinn, A. S., Gong, H., Haslip, M., Shan, P.,et al. (2014). Endothelial PINK1 mediates the protective effects of NLRP3 deficiency during lethal oxidant injury. J. Immunol. 192, 5296–5304. doi: 10.4049/jimmunol.1400653
Pubmed Abstract | Pubmed Full Text | CrossRef Full Text | Google Scholar
Zhou, R., Yazdi, A. S., Menu, P., and Tschopp, J. (2011). A role for mitochondria in NLRP3 inflammasome activation. Nature 469, 221–225. doi: 10.1038/nature09663
Pubmed Abstract | Pubmed Full Text | CrossRef Full Text | Google Scholar
Keywords: autophagy, mitophagy, lung diseases, ciliophagy, xenophagy
Citation: Mizumura K, Choi AMK and Ryter SW (2014) Emerging role of selective autophagy in human diseases. Front. Pharmacol. 5:244. doi: 10.3389/fphar.2014.00244
Received: 15 September 2014; Accepted: 23 October 2014;
Published online: 05 November 2014.
Edited by:
Frank Wagener, Radboud University Nijmegen Medical Centre, NetherlandsReviewed by:
Stephen Tait, University of Glasgow, UKDanhui Liu, Wenzhou Medical College, China
Frank Wagener, Radboud University Nijmegen Medical Centre, Netherlands
Copyright © 2014 Mizumura, Choi and Ryter. This is an open-access article distributed under the terms of the Creative Commons Attribution License (CC BY). The use, distribution or reproduction in other forums is permitted, provided the original author(s) or licensor are credited and that the original publication in this journal is cited, in accordance with accepted academic practice. No use, distribution or reproduction is permitted which does not comply with these terms.
*Correspondence: Stefan W. Ryter, Joan and Sanford I. Weill Department of Medicine, Weill Cornell Medical Center, New York-Presbyterian Hospital – Weill Cornell Medical College, 525 East 68th Street, Room M-522, P.O. Box 130, New York, NY 10065, USA e-mail:c3RyMjAyMEBtZWQuY29ybmVsbC5lZHU=