- 1Department of Psychological and Brain Sciences, Indiana University, Bloomington, IN, USA
- 2Frank H. Netter MD School of Medicine, Quinnipiac University, North Haven, CT, USA
Microglial cells are extremely plastic and undergo a variety of CNS-prompted shape changes relative to their location and current role. Signaling molecules from neurons also regulate microglial cytokine production. Neurons are known to employ the endogenous cannabinoid system to communicate with other cells of the CNS. N-arachidonoyl glycine (NAGly) and Δ9-tetrahydrocannabinol (Δ9-THC) signaling via GPR18 has been introduced as an important new target in microglial–neuronal communication. Our hypothesis is that endogenous NAGly-GPR18 signaling regulates phenotypic shape and cytokine production in microglia, and is mimicked by Δ9-THC in the BV-2 microglia model system. BV-2 microglia were exposed to NAGly and Δ9-THC or Vh for 12 h, which resulted in significant differences in the cell morphologies expressed. Cannabidiol (CBD) was effective at antagonizing the effects of both NAGly and Δ9-THC. Using ELISA-based microarrays, BV-2 microglia were exposed to NAGly and Δ9-THC or Vh for 3 h and the presence of 40 cytokines in the culture media quantified. Production of Axl, CD40, IGF-I, OPN, and Pro-MMP-9 were significantly altered by NAGly and Δ9-THC, and antagonized by CBD. These data add to an emerging profile that emphasizes NAGly as a component of an endogenous system present in the CNS that tightly integrates microglial proliferation, recruitment, and adhesion with neuron–glia interactivity and tissue remodeling.
Introduction
Microglial cells are extremely plastic and undergo a variety of shape changes relative to their location and current role (Block et al., 2007; Colton and Wilcock, 2010). Ramified microglia possess a characteristic form composed of long branching processes that are very sensitive to alterations in the local microenvironment (Fetler and Amigorena, 2005; Raivich, 2005). Amoeboid microglia scavenge CNS plaque and foreign agents and mediate cytotoxic and inflammatory signaling (Gordon, 2003; Fetler and Amigorena, 2005; Raivich, 2005; Garden and Moller, 2006). Microglia have a significant impact on overall CNS stability and may act as an essential link in the interaction of cytokines with neurons (Zhang et al., 2003). In both developmental and post-developmental contexts, microglia discriminately engulf and eliminate dead or dying neurons. They are especially prevalent during rewiring of the brain when there are large amounts of extracellular apoptotic debris to remove.
These processes must be tightly controlled in order to sustain the least possible collateral damage to adjacent neurons. Indeed, microglial activity is not only orchestrated to exacting standards, but the coupling between the death of neurons and their degradation by microglia is both striking and swift. This remarkable relationship suggests a fast-acting communication between neurons and microglia, such that the microglia are forewarned of the specific task (i.e., apoptosis, infection, or damage). Once the phagocytic task of amoeboid microglia is complete a return to the resting (i.e., multi-branched, surveillant) form is usually observed. Yet, microglia are also implicated in virtually all CNS neuropathological processes, where they become highly reactive to dying neurons and provoke sustained secondary neurotoxicity (Carson, 2002; Eikelenboom et al., 2002; Streit, 2002; Danton and Dietrich, 2003; van Rossum and Hanisch, 2004). This extreme dichotomy of behavior underscores the importance of understanding the specific signaling systems that both recruit and instruct microglia to adapt their phenotype selectively in response to damaged/dying/apoptotic neurons, and which are foundational to our ability to monitor and manage dysregulated microglial activity.
Neurons are known to employ the endogenous cannabinoid system to communicate with other cells of the CNS. Walter et al. (2003) reported the involvement of the endogenous cannabinoid signaling in recruiting microglia toward dying neurons in vitro. Recently the pharmacology of N-arachidonoyl glycine (NAGly), various cannabinoids [most notably Δ9-tetrahydrocannabinol (Δ9-THC)] and other signaling ligands at GPR18 receptors has been described. During the same timeframe, NAGly-GPR18 signaling was also introduced as an important new target in microglial–neuronal communication (see McHugh, 2012; McHugh et al., 2012a,b for review). NAGly is synthesized in the CNS primarily from AEA via a fatty acid amide hydrolase (FAAH) dependent pathway and can be prevented by URB597, an irreversible inhibitor of FAAH (Bradshaw et al., 2009). It is ineffective as an agonist at either CB1 or CB2 receptors despite the obvious structural overlap with AEA (Járai et al., 1999; Begg et al., 2005; Mackie and Stella, 2006). Instead, NAGly acts as a high affinity ligand for the Gi/o-coupled GPCR GPR18 (Kohno et al., 2006; McHugh et al., 2010, 2012a,b; McHugh, 2012) and a partial agonist of Gq/11-coupled GPR92 receptors (Oh et al., 2008). Δ9-THC is a well-established agonist at Gi/o-coupled cannabinoid CB1 and CB2 receptors (for review, see Pertwee et al., 2010). More recent investigations have raised the prospect of Δ9-THC signaling via non-CB1 and non-CB2 GPCRs, including GPR55 and GPR18, although the current pharmacology of GPR55 is complicated and inconsistent (Pertwee et al., 2010). Here, we explore the hypothesis that NAGly–GPR18 signaling regulates morphological and cytokine signaling plasticity, which is mimicked by Δ9-THC in the BV-2 microglia model system.
Materials and Methods
Test Compounds
Cannabinoids and related lipids are practically stable during storage, with concentration changes attributed to oxidation, temperature effects, and lipophilic binding. The lipid compounds tested in this study were prepared in 100% DMSO to a stock concentration of 100 mM, aliquoted and kept at -20°C. Fresh aliquots were used for each independent experiment. Compounds were serially diluted to achieve the desired final working concentrations, where each contained 0.1% DMSO as vehicle. NAGly was purchased from Cayman Chemicals (Farmingdale, NY, USA), lipopolysaccharide (LPS), and phorbol 12- myristate 13- acetate (PMA) from Sigma-Aldritch (St. Louis, MO, USA). Δ9-THC and Cannabidiol (CBD) were provided by Dr. Ken Mackie, Indiana University.
Cells in Culture
The mouse microglial cell line BV-2 (a gift from Dr. N. Stella; University of Washington, Seattle), which was originally generated by immortalizing primary microglia (Blasi et al., 1990), were grown in high glucose DMEM (Gibco, USA) supplemented with FBS (5%), penicillin (100 units/ml), streptomycin (100 μg/ml), and L-glutamine (0.292 mg/ml). Cells were passaged every 2–3 days for a maximum of 30 passages. Media was replaced with serum-free Ham’s F-12 phenol red-free media (Gibco, USA), supplemented with penicillin (100 units/ml), streptomycin (100 μg/ml) and L-glutamine (0.292 mg/ml), 16 h prior to the start of experiments.
BV-2 Morphology Experiments
BV-2 microglia were plated using serum-free Ham’s F-12 phenol red-free media on 25 mm × 75 mm glass microscope slides that had been pre-coated for 1 h with 1 μg/ml poly-D-lysine. A coated microscope slide was placed in a 10 cm Petri dish then 1 ml of cell suspension (5 × 105 cells/ml) was placed onto the center of the slide using a Pasteur pipette. Then an additional 11 ml of the Ham’s F-12 media was added to the dish together with Vh or test compound. Cells were incubated for 12 h (5% CO2 atmosphere at 37°C) with Vh control (0.1% DMSO), 10 nM NAGly, 10 nM Δ9-THC, 100 nM CBD, 10 nM NAGly + 100 nM CBD, or 10 nM Δ9-THC + 100 nM CBD. 10 nM PMA was used as positive control. Each condition was tested in triplicate per individual experiment. The media was removed and replaced for 20 m with 12 ml of cytoskeleton buffer with sucrose and formaldehyde. This buffer consisted of 10 mM 2-N-morpholino ethanesulfonic acid (MES), 138 mM KCl, 3 mM MgCl2, 2 mM EGTA in 1 L of H2O, with its pH adjusted to 6.1. It was stored at 4°C in between use. 0.32 M sucrose was added to a 50 ml aliquot of the solution prior to each experiment and thoroughly vortexed. Next formaldehyde was added to the aliquot to achieve a concentration of 3.7%. Following the 20 m incubation, the slides were then washed with phosphate-buffered saline (PBS) three times. Next, permount was pipetted onto the slide and a glass coverslip placed on top. Slides were then put in a dark drawer, where they were protected from light, and left to dry for at least 24 h. Images from 10 random fields of view were collected at x20 magnification using a Nikon 80i light microscope from each microscope slide. Cell morphology was recorded and categorized by multiple investigators who were blind to the treatment condition into six categories: amoeboid, unipolar, bipolar, tripolar, multipolar, and flat. This amounted to 200+ cells per individual treatment replicate. Categorization was based on the number of processes that branched directly off the cell body. A cell was considered to be unipolar if it has one process, bipolar two, tripolar three, and multipolar four or more. The amoeboid and flat categories had zero processes. To distinguish between these, cells that were overtly three-dimensional and irregularly rounded were classed as amoeboid; while those with their cytoplasm spread over a larger area, with wavy edges and little three-dimensional depth were classed as flat (Figure 1).
BV-2 Cytokine Release Experiments
~ 90% confluent BV-2 cells were incubated for 3 h (5% CO2 atmosphere at 37°C) in 3 ml serum-free Ham’s F-12 phenol red-free media with Vh control (0.1% DMSO), 10 nM and 100 nM NAGly, 10 and 100 nM Δ9-THC, 100 nM CBD, 10 nM NAGly + 100 nM CBD, and 100 nM Δ9-THC + 100 nM CBD. 10 nM PMA was used as positive control. 1 μg/ml LPS was used as positive control. After the incubation, the culture media was carefully removed (to minimize transferring any cells) and placed in 15 ml centrifuge tubes. Tubes were left undisturbed on the bench for 10 min to allow any cell debris to settle to the bottom. 100 μl of culture media was then collected and the presence of 40 cytokines quantified using Quantibody Mouse Cytokine Array 4 (RayBiotech, Inc.), Odyssey blocking buffer (Li-Cor #927-40000), Li-Cor IRDye 800CW Streptavidin (#926-32230), and Odyssey infra-red imager (Li-Cor Biosciences). The Quantibody kit is a multiplexed, sandwich ELISA-based array that enables researchers to accurately determine the concentration of multiple cytokines simultaneously. Odyssey settings were: resolution, 21 μm; quality, highest; focal offset, 1.0 mm; and intensity, 10 for the 800 nm channel (the 700 nm channel was not required). The cytokines tested by the array were as follows: AR, Axl, CD27L, CD30T, CD40, CXCL16, EGF, E-selectin, Fractalkine, GITR,HGF, IGFBP-2, IGFBP-3, IGFBP-5, IGFBP-6, IGF-I, IL-12p70, IL-17E, IL-17F, IL-1ra, IL-2 Rα, IL-20, IL-23, IL-28, I-TAC, MDC, MIP-2, MIP-3α, OPN, OPG, Prolactin, Pro-matrix metallopeptidase-9 (Pro-MMP-9), P-selectin, Resistin, SCF, SDF-1α, TPO, VCAM-1, VEGF, and VEGF-D.
Analysis of Data
All data are expressed as means ± s.e.mean and n = number of independent experiments. Statistical analyses were performed with GraphPad Prism 4 or SPSS. One-way ANOVA (p < 0.05) with LSD post hoc test was run to determine significance between treatment conditions.
Results
Effects of Δ9-THC and NAGly on BV-2 Microglial Morphology
Microglial cells are extremely plastic and exist in a variety of morphologies, including: amoeboid, unipolar, bipolar, tripolar, multipolar, and flat, which are illustrated in Figure 1. At rest, BV2 microglia display primarily an amoeboid morphology (44%) with varying degrees of branched morphologies (Table 1). Data here replicated previous findings that 10 nM PMA induced a shift in morphology from one that was round and amoeboid to a non-round form with cytoplasmic extensions (Table 1). Likewise, 10 nM NAGly and 10 nM Δ9-THC significantly reduced the relative percentage of BV-2 microglia that manifest the amoeboid phenotype; instead, prompting them to adopt a non-round, branched morphology with cytoplasmic extensions, most of which was classified as being bipolar in shape (Table 1). Other than a small but significant decrease in the relative percentage of unipolar BV-2 microglia in the presence of 10 nM Δ9-THC + 100 nM CBD, Δ9-THC and NAGly produced non-significant fluctuations in the unipolar, tripolar, multipolar, or flat categories (Table 1). However, the morphologic phenotypic shift evoked by 10 nM NAGly and 10 nM Δ9-THC was antagonized by 100 nM CBD, which is in keeping with our previous reports of GPR18 pharmacology (McHugh et al., 2010, 2012a,b; McHugh, 2012). 100 nM CBD alone had no significant effects on BV-2 microglial shape (Table 1). When all branched morphologies are combined and compared to those cells in the amoeboid morphology, it is clear that there is an profound effect on the branched verses non-branched phenotype in the presence of Δ9-THC and NAGly and that this is reversed with CBD (Figure 2).
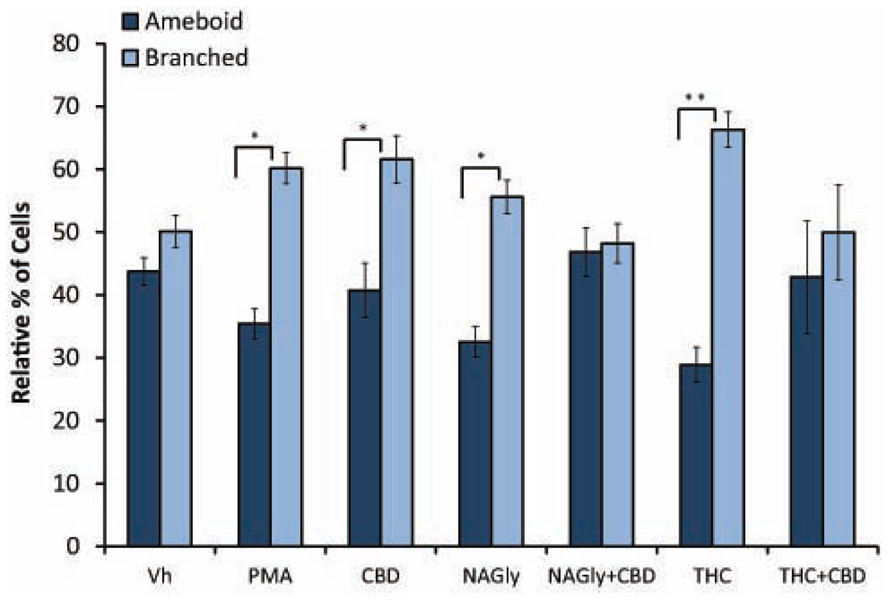
FIGURE 2. Amoeboid versus branched phenotypes. Vehicle (0.1% DMS0), 10 nM PMA, 10 nM NAGly, 100 nM CBD, 10 nM NAGly + 100 nM CBD, 10 nM Δ9-THC, 10 nM Δ9-THC + 100 nM CBD. n = 5–15. *p < 0.05, **p < 0.01 (One-way ANOVA).
Effects of Δ9-THC and NAGly on BV-2 Microglial Cytokine Release
Microglia signal and support numerous other cells by secreting a variety of signaling molecules and growth factors, including cytokines. Of the forty cytokines quantified by the Quantibody array, the production of five cytokines were significantly altered in BV-2 microglia in a concentration-dependent manner by NAGly and Δ9-THC. They were: Axl, CD40, IGF-I, OPN, and Pro-MMP-9 (Figure 3).
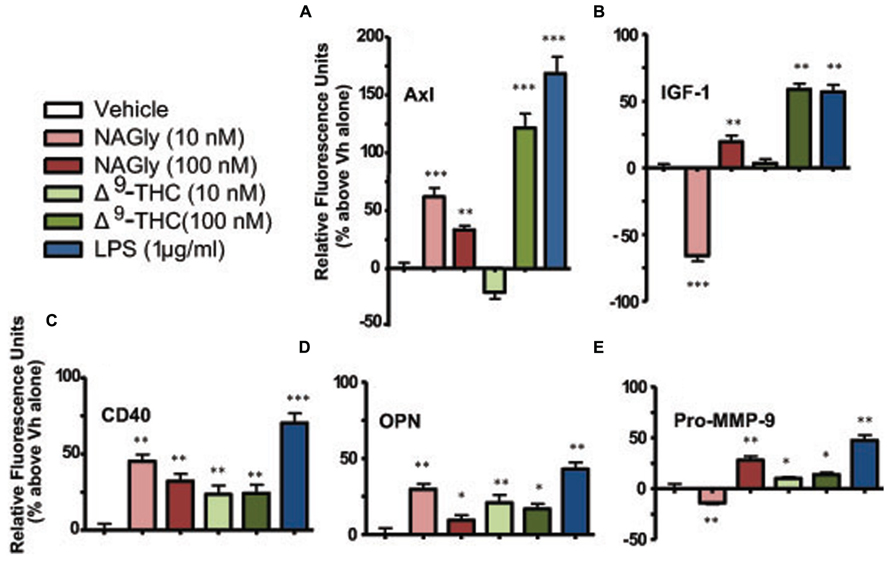
FIGURE 3. Levels of cytokine production after 3 h treatment in BV-2 microglia. (A) Axl, (B) IGF-1, (C) CD40, (D) OPN, (E) Pro-MMP-9. NAGly (10 nM and 100 nM) and Δ9-THC (10 nM and 100 nM). n = 4–5. *p < 0.05, **p < 0.01, ***p < 0.001 (One-way ANOVA).
Axl is a member of the tyrosine-protein kinase receptor (RTK) family and activated by the vitamin K-dependent protein, growth-arrest-specific 6 (Gas6; Mark et al., 1996). 10 nM and 100 nM concentrations of NAGly, 100 nM Δ9-THC and 1 μg/ml LPS caused a significant increase in the basal production of the cytokine Axl, while 10 nM Δ9-THC produced a significant reduction (Figure 3A). CD40 is a co-stimulatory protein and member of the TNF-receptor superfamily (Chatzigeorgious et al., 2009). 10 nM and 100 nM concentrations of NAGly and Δ9-THC together with 1 μg/ml LPS caused a significant increase in the basal production of the cytokine CD40 (Figure 3B). IGF-1 activates the Insulin-like growth factor 1 receptor (IGF1R), which is a receptor tyrosine kinase present on many cell types in many tissues (Jones and Clemmons, 1995). 100 nM Δ9-THC significantly increased basal IGF-1 levels, as did 1 μg/ml LPS, but 10 nM Δ9-THC had no effect. On the other hand, 10 nM NAGly produced a significant drop in IGF-1, while 100 nM NAGly produced a significant increase (Figure 3C). OPN is expressed in a range of immune cells, including macrophages, neutrophils, dendritic cells, and T and B cells (Mazzali et al., 2002). 10 nM and 100 nM concentrations of NAGly and Δ9-THC together with 1 μg/ml LPS caused a significant increase in the basal production of the cytokine OPN (Figure 3D). Pro-MMP-9 is the precursor form of MMP-9, which degrades type IV and V collagens (Toth et al., 2003). 10 nM and 100 nM Δ9-THC significantly increased basal Pro-MMP-9 levels, as did 1 μg/ml LPS and 100 nM NAGly. On the other hand, 10 nM NAGly produced a significant drop in Pro-MMP-9 (Figure 3E). Changes in cytokine production levels evoked by10 nM NAGly and 100 nM Δ9-THC for all five cytokines were significantly attenuated by 100 nM CBD (Figures 4A–E).
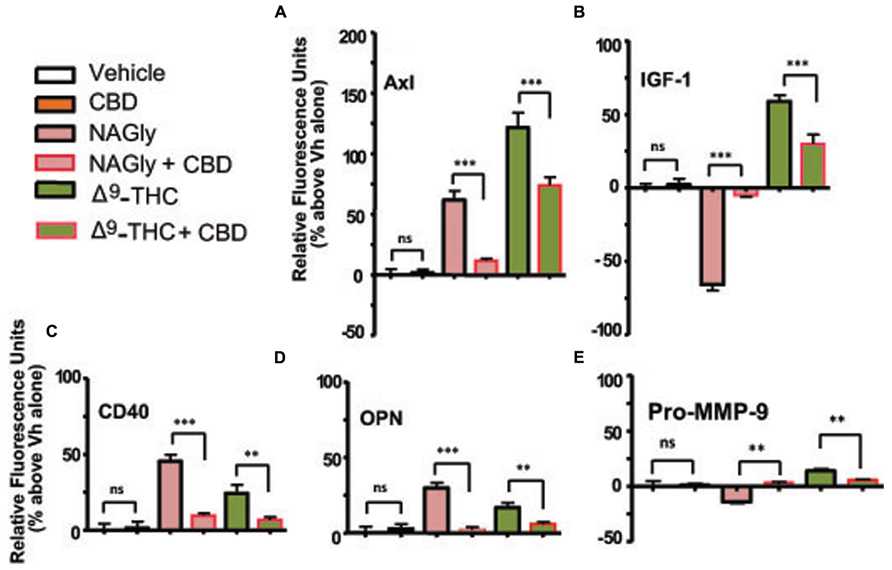
FIGURE 4. Levels of cytokine production after 3 h treatment co-incubation with CBD in BV-2 microglia. (A) Axl, (B) IGF-1, (C) CD40, (D) OPN, (E) Pro-MMP-9. Cytokine production by BV-2 microglia in response to NAGly (10 nM) and Δ9-THC (100 nM) ± CBD (100 nM) n = 4–5. **p < 0.01, ***p < 0.001 (One-way ANOVA).
Discussion
Microglia play crucial roles in the development, on-going activity and regeneration of the CNS. For example, during CNS injury, microglia participate in inflammation and wound healing by migrating into damaged tissue, where they proliferate and act as phagocytic scavengers (Zhang et al., 2003). Microglia communicate with and support other cells by secreting a variety of signaling molecules and growth factors (Zhang et al., 2003). Microglia actively influence the environment of the CNS and may act as an essential link in the interaction of cytokines with neurons (Zhang et al., 2003). They also actively regulate the number of functional synapses in the CNS and glutamate receptor expression (Ji et al., 2013). This does not merely take place during development, but rather constitutes a dynamic, reversible, and continuous event in the mature brain. Hence, they are directly involved in the modulation of synaptic activity (Ji et al., 2013). In short, microglia are highly complex cells found in the CNS that exhibit multiple forms and functional specializations, and it has long been appreciated that microglial cells exist in extremely different cell shapes ranging from an amoeboid to a ramified phenotype. Indeed, microglial cells adapt their cell morphology to the meet the functional demands of their microenvironment: amoeboid, when they have to eliminate cell debris; ramified, when they have to keep their microenvironment under surveillance; branched cytoplasmic extensions, when they make direct contact with synaptic elements to modulate activity; and non-polarized, when they secrete cytokines and reactive oxygen or nitrogen species. Moreover, the CNS microenvironment also prompts microglia to adapt their cytokine release profile to meet functional demands (i.e., pro- versus anti-inflammatory). The mechanisms regulating these adaptations are poorly understood and prompt the question of what triggers cytological plasticity in microglia. Our previous research has shown that Δ9-THC and NAGly are full agonists at GPR18 with EC50 values in the nanomolar range, and that NAGly-GPR18 signaling drives potent microglia migration, proliferation as well as mitogen-activated protein kinase (MAPK) activation. Given this, we explored the hypothesis that Δ9-THC and NAGly, putatively also through GPR18 receptors, regulates morphological and cytokine signaling plasticity in BV-2 microglia.
Δ9-THC and NAGLY: Morphological Plasticity
Our observations demonstrate that both Δ9-THC and NAGly influenced the shape of BV-2 microglia (Table 1). 10 nM concentrations of both molecules significantly reduced the proportion of cells that manifested the amoeboid phenotype by means of generally increasing the number of cytoplasmic processes branching off the microglial cell body. These morphological shifts were prevented by co-treatment with 100 nM CBD, which alone had no effects. (Pöttler et al., 2006) have reported that the addition of PMA decreased the number of amoeboid and increased the number of branched BV-2 microglia in vitro. Our results reproduced and confirmed their findings. A significant percentage of BV-2 microglia assumed a bipolar phenotype when exposed to Δ9-THC or NAGly. If the data is simplified by collapsing the shape categories into amoeboid versus branched, when exposed to Vh alone there is an almost equivalent amount of both phenotypes; however, the addition of PMA, NAGly, and Δ9-THC significantly shifted the percentage distribution in favor of branched, which was reversed by co-addition of CBD. This suggests that there may be a particular functional relevance to the branched morphology in the neuronal environment of the CNS. In support of this, recent reports state that non-amoeboid microglia contact active synapses, implying a cell-cell interaction between ramified microglia and neurons have also described how ramified microglia contribute to the protection of neurons from excitotoxicity (Tremblay et al., 2010; Fontainhas et al., 2011). Microglia are capable of adapting their cytoskeleton to adopt any of the six shapes categorized here. While this morphological plasticity is continuous in the sense that the changes are not binary or quantal, microglia do not necessarily have to progress from amoeboid to unipolar to bipolar to tripolar⋯ etc. The different morphological stages of microglial activation and their functional consequences have not been fully ascertained in detail; this is an emerging field of interest to which our data contribute.
Δ9-THC and NAGly: Cytokine Signaling Plasticity
Cytokines are varied substances (e.g., interferons, interleukins, growth factors, etc.) that are released by certain cells of the immune system and have an effect on other cells. The secretion profile of five cytokines (Axl, CD40, IGF-I, OPN, and Pro-MMP-9) was significantly altered in BV-2 microglia by Δ9-THC and NAGly; the changes were attenuated by CBD (100 nM). Axl is an enzyme of the RTK family and is involved in the transduction of signals from the extracellular matrix to the cytoplasm by binding growth factors like vitamin K-dependent protein growth-arrest-specific gene 6 (Gas6; Mark et al., 1996). Axl is involved in the mediation of cell aggregation by homotypic binding (Heide et al., 1998), which is an interaction between the same adhesion molecule expressed by different cell types (e.g., neurons and microglia). Axl signaling plays a role in cell growth and survival in normal and cancer cells, including colon cancer and melanoma (Sensi et al., 2011). Interestingly, the first report to describe GPR18 arose unanticipated from expression studies by Gantz et al. (1997), who inadvertently isolated the GPCR from the human colonic cancer Colo 320DM cell line. While, Qin et al. (2010) reported that expression levels of GPR18 were not only constitutively active but also ~ 24-fold higher in human metastatic melanoma compared with benign nevi.
CD40 is a co-stimulatory protein and member of the TNF-receptor superfamily; it is found on antigen presenting cells and has been found to be essential in mediating a broad variety of immune and inflammatory responses (Chatzigeorgious et al., 2009). CD40 helps mediate the induction of potent microbicidal substances in glial cells, including reactive oxygen species and nitric oxide, leading to the destruction of ingested microbes (Cao et al., 2009). The interaction of this cytokine with TNF receptors have been found to be necessary for amyloid beta (Aβ)-induced microglial activation (Tan et al., 2002), and thus is thought to be an early event in Alzheimer’s disease pathogenesis. IGF-1 activates the IGF1R, which is a receptor tyrosine kinase present on many cell types in many tissues (Jones and Clemmons, 1995). It is a primary mediator of the effects of growth hormone and one of the most potent natural activators of the AKT signaling pathway (Gallagher et al., 2010). IGF-1 has growth-promoting effects on almost every cell in the body; it regulates cellular growth, development and DNA synthesis, especially in neuronal cells (Ueno et al., 2013). OPN is expressed in a range of immune cells, including macrophages, neutrophils, dendritic cells, and T and B cells (Mazzali et al., 2002). This cytokine is a chemoattractant that promotes cell recruitment to inflammatory sites. OPN also functions as an adhesion protein, involved in cell attachment and wound healing, and mediates cell activation, cell survival (by regulating apoptosis) and cytokine production (Mazzali et al., 2002). Proteins of the matrix metalloproteinase (MMP) family are secreted as inactive pro-MMP proteins and subsequently cleaved by extracellular proteinases (Toth et al., 2003). They are involved in the breakdown of extracellular matrix in normal physiological processes, such as embryonic development, reproduction, and tissue remodeling, as well as in disease processes, such as arthritis, intracerebral hemorrhage, and metastasis (Wang and Tsirka, 2005). MMP-9 degrades type IV and V collagens (Kähäri and Saariaho-Kere, 1997).
While there is general interest in, as well as reports in the literature and ongoing investigations into, the influence of cannabinoids on cytokine signaling, this is the first data regarding these five specific cytokines in BV-2 microglia. A synopsis of the overall functional roles of these five cytokines would include growth, adhesion, tissue remodeling and apoptotic regulation. Their modulation implies an important role for the underlying mechanism of action exploited here by Δ9-THC and NAGly. The effects observed were concentration-dependent, where the most extreme differences in response between 10 nM and 100 nM of NAGly and Δ9-THC were: decreased versus increased IGF-1 and Pro-MMP-9 basal levels for NAGly; and decreased/no change versus increased IGF-1 and Axl basal levels for Δ9-THC. Antagonism of Δ9-THC and NAGly effects by 100 nM CBD is in keeping with our previous reports of GPR18 pharmacology (McHugh et al., 2010, 2012a,b). Further investigation is needed to fully ascertain the involvement of GPR18 and to rule in or rule out other potentially relevant receptors (i.e., CB1, CB2, GPR92). A time course study leading up to and continuing beyond the time points used here would be beneficial in determining when and to what extent does maximal cytoplasmic branching and cytokine release induced by Δ9-THC and NAGly occur in the BV-2 cells. This would also help clarify the apparent switching of some of the cytokines from below basal to above basal levels (e.g., IGF-1 at 10 nM and 100 nM Δ9-THC and NAGly), as would tests with additional concentrations. Cytokine signaling is known to be complex, and often includes opposing responses (e.g., pro- versus anti-inflammatory) that are considered to be physiologically routine. Alternatively, ligand-receptors interactions at GPCRs are often subject to desensitization, which may explain the differential effects observed. As mentioned above, it is also possible that Δ9-THC and NAGly are acting via multiple receptors to produce a form of physiological antagonism with respect to cytokine release.
The findings in this study support our hypothesis that Δ9-THC and NAGly, putatively through GPR18 receptors, regulate morphological and cytokine signaling plasticity in BV-2 microglia. These data add to an emerging profile that emphasizes NAGly as a component of an endogenous system present in the CNS that tightly integrates microglial proliferation, recruitment, and adhesion with neuron-glia interactivity and tissue remodeling. A greater understanding of this system is essential to improving our ability to therapeutically monitor and manage dysregulated microglial activity.
Author Contributions
Douglas McHugh: cytokine experiments; BV-2 microglia shape categorization; design and coordination of the studies; data interpretation; statistical analyses; and manuscript preparation. Daniel Roskowski: morphology experiments and BV-2 microglia shape categorization and quantification, data interpretation; statistical analyses. Sisi Xie: BV-2 microglia shape categorization and quantification. Heather B. Bradshaw: design and coordination of the studies; data interpretation; statistical analyses; and manuscript preparation. All authors read and approved the final manuscript.
Conflict of Interest Statement
The authors declare that the research was conducted in the absence of any commercial or financial relationships that could be construed as a potential conflict of interest.
Acknowledgments
This work was supported by NIH DA018224. We thank Anisa Curry-Vietze, Hannah Kasak-Gliboff, and Harpo Roeder for their work on validating the cell morphology phenotyping assay.
References
Begg, M., Pacher, P., Bátkai, S., Osei-Hyiaman, D., Offertáler, L., Mo, F. M., et al. (2005). Evidence for novel cannabinoid receptors. Pharmacol. Ther. 106,133–145. doi: 10.1016/j.pharmthera.2004.11.005
Blasi, E., Barluzzi, R., Bocchini, V., Mazzolla, R., and Bistoni, F. (1990). Immortalization of murine microglial cells by a v-raf/v-myc carrying retrovirus. J. Neuroimmunol. 27, 229–237. doi: 10.1016/0165-5728(90)90073-V
Block, M. L., Zecca, L., and Hong, J.-S. (2007). Microglia-mediated neurotoxicity: uncovering the molecular mechanisms. Nat. Rev. Neurosci. 8, 57–69. doi: 10.1038/nrn2038
Bradshaw, H., Rimmerman, N., Hu, S., Benton, V., Stuart, J., Masuda, K., et al. (2009). The endocannabinoid anandamide is a precursor for the signaling lipid N-arachidonoyl glycine by two distinct pathways. BMC Biochem. 10:14. doi: 10.1186/1471-2091-10-14
Cao, L., Plamer, C. D., Malon, J. T., and De Leo, J. A. (2009). Critical role of microglial CD40 in the maintenance of mechanical hypersensitivity in a murine model of neuropathic pain. Eur. J. Immunol. 39, 3562–3569. doi: 10.1002/eji.200939657
Carson, M. J. (2002). Microglia as liaisons between the immune and central nervous systems: functional implications for multiple sclerosis. Glia 40, 218–231. doi: 10.1002/glia.10145
Chatzigeorgious, A., Lyberi, M., Chatzilymperis, G., Nezos, A., and Kamper, E. (2009). CD40/CD40: signaling and its implications in health and disease. BioFactors 35, 474–483. doi: 10.1002/biof.62
Colton, C., and Wilcock, D. (2010). Assessing activation states in microglia. CNS Neurol. Disord. Drug. Targets 9, 174–191. doi: 10.2174/187152710791012053
Danton, G. H., and Dietrich, W. D. (2003). Inflammatory mechanisms after ischemia and stroke. J. Neuropathol. Exp. Neurol. 62,127–136.
Eikelenboom, P., Bate, C., Van Gool, W. A., Hoozemans, J. J., Rozemuller. J. M., Veerhuis, R., et al. (2002). Neuroinflammation in Alzheimer’s disease and prion disease. Glia 40, 232–239. doi: 10.1002/glia.10146
Fetler, L., and Amigorena, S. (2005). Neuroscience. Brain under surveillance: the microglia patrol. Science 309, 392–393. doi: 10.1126/science.1114852
Fontainhas, A. M., Wang, M., Liang, K. J., Chen, S., Mettu, P., Damani, M., et al. (2011). Microglial morphology and dynamic behavior is regulated by ionotropic glutamatergic and GABAergic neurotransmission. PLoS ONE 6:e15973. doi: 10.1371/journal.pone.0015973
Gallagher, E. J., Fierz, Y., Ferguson, R. D., and LeRoith, D. (2010). The pathway from diabetes and obesity to cancer, on the route to targeted therapy. Endocr. Pract. 16, 864–873. doi: 10.4158/EP10098.RA
Gantz, I., Muraoka, A., Yang, Y.-K., Samuelson, L. C., Zimmerman, E. M., and Cook, H. et al. (1997). Cloning and chromosomal localization of a gene (GPR18) encoding a novel seven transmembrane receptor highly expressed in spleen and testis. Genomics 42, 462–466. doi: 10.1006/geno.1997.4752
Garden, G. A., and Moller, T. (2006). Microglia biology in health and disease. J Neuroimmune Pharmacol. 1, 127–137. doi: 10.1007/s11481-006-9015-5
Gordon, S. (2003). Alternative activation of macrophages. Nat. Rev. Immunol. 3, 23–35. doi: 10.1038/nri978
Heide, I., Sokoll, A. C., Henz, B. M., Nagel, S., Kreissig, K., Grützkau, A., et al. (1998). Regulation and possible function of axl expression in immature human mast cells. Ann. Hematol. 77, 199–205. doi: 10.1007/s002770050443
Járai, Z., Wagner, J. A., Varga, K. R., Lake, K. D., Compton, D. R., Martin, B. R., et al. (1999). Cannabinoid-induced mesenteric vasodilation through an endothelial site distinct from CB1 or CB2 receptors. Proc. Natl. Acad. Sci. U.S.A. 96, 14136–14141. doi: 10.1073/pnas.96.24.14136
Ji, K., Akgul, G., Wollmuth, L. P., and Tsirka, S. E. (2013). Microglia actively regulate the number of functional synapses. PLoS ONE 8:e56293. doi:10.1371/journal.pone.0056293
Jones, J. I., and Clemmons, D. R. (1995). Insulin-like growth factors and their binding proteins: biological actions. Endocr. Rev. 16, 3–34.
Kähäri, V.-M., and Saariaho-Kere, U. (1997). Matrix metalloproteinases in skin. Exp. Dermatol. 6, 199–213. doi: 10.1111/j.1600-0625.1997.tb00164.x
Kohno, M., Hasegawa, H., Inoue, A., Muraoka, M., Miyazaki, T., Oka, K., et al. (2006). Identification of N-arachidonylglycine as the endogenous ligand for orphan G-protein-coupled receptor GPR18. Biochem. Biophyl. Res. Comm. 347, 827–832. doi: 10.1016/j.bbrc.2006.06.175
Mackie, K., and Stella, N. (2006). Cannabinoid receptors and endocannabinoids: Evidence for new players. AAPS J. 8, E298–E306.
Mark, M. R., Chen, J., Hammonds, R. G., Sadick, M., and Godowsk, P. J. (1996). Characterization of Gas6, a member of the superfamily of G domain-containing proteins, as a ligand for Rse and Axl. J. Biol. Chem. 271, 9785–9789. doi: 10.1074/jbc.271.16.9785
Mazzali, M., Kipari, T., Ophascharoensuk, V., Wesson, J. A., Johnson, R., and Hughes, J. (2002). Osteopontin – a molecule or all seasons. Q. J. Med. 95, 3–13. doi: 10.1093/qjmed/95.1.3
McHugh, D., Hu, S. S.-J., Rimmerman, N., Juknat, A., Vogel, Z., Walker, J. M., et al. (2010). N-arachidonoyl glycine, an abundant endogenous lipid, potently drives directed cellular migration through GPR18, the putative abnormal cannabidiol receptor. BMC Neurosci. 11:44. doi: 10.1186/1471-2202-11-44
McHugh, D. (2012). GPR18 in microglia: implications for the CNS and endocannabinoid system signalling. Br. J. Pharmacol. 167, 1575–1582. doi: 10.1111/j.1476-5381.2012.02019.x
McHugh, D., Page, J., Dunn, E., and Bradshaw, H. B. (2012a). (9-THC and N-arachidonoyl glycine are full agonists at GPR18 and cause migration in the human endometrial cell line, HEC-1B. Br. J. Pharmacol. 165, 2414–2424. doi: 10.1111/j.1476-5381.2011.01497.x
McHugh, D., Wager-Miller, J., Page, J., and Bradshaw, H. B. (2012b). siRNA knockdown of GPR18 receptors in BV-2 microglia attenuates the cell migration induced by N-arachidonyl glycine. J. Mol. Signal. 7, 10. doi: 10.1186/1750-2187-7-10
Oh, D. Y., Yoon, J. M., Moon, M. J., Hwang, J. I., Choe, H., Lee, J. Y., et al. (2008). Identification of farnesyl pyrophosphate and N-arachidonylglycine as endogenous ligands for GPR92. J. Biol. Chem. 283, 21054–21064. doi: 10.1074/jbc.M708908200
Pertwee, R. G., Howlett, A. C., Abood, M. E., Alexander, S. P. H., Di Marzo, V., Elphick, M. R. et al. (2010). International union of basic and clinical pharmacology. LXXIX. Cannabinoid receptors and their ligands: beyond CB1 and CB2. Pharmacol. Rev. 62, 588–631. doi: 10.1124/pr.110.003004
Pöttler, M., Zierler, S., and Kerschbaum, H. H. (2006). An artificial three-dimensional matrix promotes ramification in the microglial cell-line, BV-2. Neurosci. Lett. 410, 137–140. doi: 10.1016/j.neulet.2006.09.082
Qin, Y., Verdegaal, E. M. E., Siderius, M., Bebelman, J. P., Smit, M. J., and Leurs, R., et al. (2010). Quantitative expression profiling of G-protein-coupled receptors (GPCRs) in metastatic melanoma: the constitutively active orphan GPCR GPR18 as novel drug target. Pigment Cell Melanoma Res. 24, 207–218. doi: 10.1111/j.1755-148X.2010.00781.x
Raivich, G. (2005). Like cops on the beat: the active role of resting microglia. Trends Neurosci. 28, 571–573. doi: 10.1016/j.tins.2005.09.001
Sensi, M., Catani, M., Castellano, G., Nicolini, G., Alciato, F., Tragni, G., et al. (2011). Human cutaneous melanomas lacking MITF and melanocyte differentiation antigens express a functional Axl receptor kinase. J. Invest. Dermatol. 131, 2448–2457. doi: 10.1038/jid.2011.218
Streit, W. J. (2002). Microglia as neuroprotective, immunocompetent cells of the CNS. Glia 40, 133–139. doi: 10.1002/glia.10154
Tan, J., Town, T., Crawford, F., Mori, T., DelleDonne, A., Crescentini, R., et al. (2002). Role of CD40 ligand in amyloidosis in transgenic Alzheimer’s mice. Nat. Neurosci. 5, 1288–1293. doi: 10.1038/nn968
Toth, M., Chvyrkova, I., Bernardo, M. M., Hernandez-Barrantes, S., and Fridman, R. (2003). Pro-MMP-9 activation by the MT1-MMP/MMP-2 axis and MMP-3: role of TIMP-2 and plasma membranes. Biochem. Biophys. Res. Commun. 308, 386–395. doi: 10.1016/S0006-291X(03)01405-0
Tremblay, M. E., Lowery, R. L., and Majewska, A. K. (2010). Microglial interactions with synapses are modulated by visual experience. PLoS Biol. 8:e1000527. doi:10.1371/journal.pbio.1000527
Ueno, M., Fujita, Y., Tanaka, T., Nakamura, Y., Kikuta, J., Ishii, M., et al. (2013). Layer V cortical neurons require microglial support for survival during postnatal development. Nat. Neurosci. 16, 543–551. doi: 10.1038/nn.3358
van Rossum, D., and Hanisch, U-K. (2004). Microglia. Metab. Brain Dis. 19, 393–411. doi: 10.1023/B:MEBR.0000043984.73063.d8
Walter, L., Franklin, A., Witting, A., Wade, C,. Xie, Y., Kunos, G., Mackie, K., and Stella, N. (2003). Nonpsychotropic cannabinoid receptors regulate microglial cell migration. J. Neurosci. 23, 1398–1405.
Wang, J., and Tsirka, S. E. (2005). Neuroprotection by inhibition of matrix metalloproteinases in a mouse model of intracerebral haemorrhage. Brain 128, 1622–1633. doi: 10.1093/brain/awh489
Keywords: Δ9-THC, NAGly, microglia, BV-2, phenotype, cytokine, morphology, GPR18
Citation: McHugh D, Roskowski D, Xie S and Bradshaw HB (2014) Δ9-THC and N-arachidonoyl glycine regulate BV-2 microglial morphology and cytokine release plasticity: implications for signaling at GPR18. Front. Pharmacol. 4:162. doi: 10.3389/fphar.2013.00162
Received: 31 August 2013; Paper pending published: 31 October 2013;
Accepted: 09 December 2013; Published online: 02 January 2014.
Edited by:
Pietro Marini, University of Aberdeen, UKReviewed by:
Isabelle Reymond, Actelion Pharmaceuticals, SwitzerlandScott Smid, The University of Adelaide, Australia
Liu Xuebo, Northwest Agriculture and Forestry University, China
Copyright © 2014 McHugh, Roskowski, Xie and Bradshaw. This is an open-access article distributed under the terms of the Creative Commons Attribution License (CC BY). The use, distribution or reproduction in other forums is permitted, provided the original author(s) or licensor are credited and that the original publication in this journal is cited, in accordance with accepted academic practice. No use, distribution or reproduction is permitted which does not comply with these terms.
*Correspondence: Heather B. Bradshaw, Department of Psychological and Brain Sciences, Indiana University, 1101 10th Street, Bloomington, IN 47405, USA e-mail:aGJicmFkc2hAaW5kaWFuYS5lZHU=