- 1INSERM 930, Faculté de Sciences et Techniques, Université François Rabelais, Tours, France
- 2Centre Hospitalier Régional Universitaire de Tours, Centre Expert Dépression Résistante, Fondation FondaMental, Tours, France
Depression is one of the most frequent and severe mental disorder. Since the discovery of antidepressant (AD) properties of the imipramine and then after of other tricyclic compounds, several classes of psychotropic drugs have shown be effective in treating major depressive disorder (MDD). However, there is a wide range of variability in response to ADs that might lead to non response or partial response or in increased rate of relapse or recurrence. The mechanisms of response to AD therapy are poorly understood, and few biomarkers are available than can predict response to pharmacotherapy. Here, we will first review markers that can be used to predict response to pharmacotherapy, such as markers of drug metabolism or blood-brain barrier (BBB) function, the activity of specific brain areas or neurotransmitter systems, hormonal dysregulations or plasticity, and related molecular targets. We will describe both clinical and preclinical studies and describe factors that might affect the expression of these markers, including environmental or genetic factors and comorbidities. This information will permit us to suggest practical recommendations and innovative treatment strategies to improve therapeutic outcomes.
Introduction
Major depressive disorder (MDD) is among the most frequent mental disorders, with an estimated lifetime prevalence of 1–16%, depending on the country (Andrade et al., 2003; Kessler and Ustun, 2004; Kessler et al., 2010). Recovering from MDD is a major challenge because the disease dramatically increases the risk of suicide (Cheng et al., 2000) and non-suicide mortality (Schulz et al., 2002). Practical guidelines recommend treating MDD with antidepressant (AD) therapy (NICE, 2004; Bauer et al., 2007; Ramasubbu et al., 2012). Since the initial serendipitous discovery of the AD effect of monoamine oxidase inhibitors (MAOIs) and tricyclics (TCAs), most ADs have been pharmacological agents that act on monoamine function, including serotonin (selective serotonin reuptake inhibitors, SSRIs), noradrenaline (noradrenaline reuptake inhibitors, NRIs), dopamine (such as bupropion), and melatonin (agomelatine). Some drugs act on several of these targets (serotonin and noradrenaline reuptake inhibitors, SNRIs) (Krishnan and Nestler, 2008). The main goals of treating MDD are to achieve remission and to maintain these therapeutic effects over time. In the absence of any reliable biomarker of MDD, the response to treatment is still based on clinical assessment as evidenced by changes on scores on standardized rating instruments, such as the Hamilton Depression Rating Scale (HDRS) (Hamilton, 1960) or the Montgomery Asberg Depression Rating Scale (Montgomery and Asberg, 1979). The response to ADs is typically characterized as “non-response” when only minimum improvement is achieved, “partial response” when the score on the standardized instrument decreases by 25–50%, “response” when a decrease of at least 50% is obtained, and “remission” when only residual clinical symptoms are reported, with a level of psychopathology under the typical threshold score currently correlated to MDD diagnosis (Nierenberg and DeCecco, 2001). Using these criteria, several studies, including the naturalistic STAR*D study, have shown that only one third of MDD patients receiving ADs achieve complete remission after a single AD trial (Trivedi et al., 2006). The remission rate reaches up to 60% after four trials, but the probability of remission drops significantly after the failure of two consecutive AD trials (Rush et al., 2006). Moreover, early improvement predicts sustained response and remission (Lam, 2012). Consequently, the concept of treatment-resistant depression (TRD) was proposed to describe depressive conditions that did not reach sufficient remission after treatment (Lehmann, 1974; Sartorius, 1974). Even several criteria have been proposed to define TRD-including non-response to one AD for at least 4 weeks or failure to respond to multiple trials of different classes of ADs-there is now an emerging consensus to consider any MDD patient that did not respond to two or more adequate (in terms of duration and dosage) AD trials of different classes as TRD (Berlim and Turecki, 2007). The characterization of TRD has been improved by considering the level of resistance (severity and duration) through staging classifications, such as the Antidepressant Treatment History Form (ATHF) (Sackeim et al., 1990), the Thase and Rush Model (Thase and Rush, 1997), the European Staging Model (Souery et al., 1999), the Massachusetts General Hospital Staging Model (MGH-s) (Fava, 2003), and the Maudsley Staging Model (MSM) (Fekadu et al., 2009) (for a review, see Ruhé et al., 2012).
Translational research enables to study the mechanisms of non response to ADs in animal models. Indeed, this involves invasive protocols, as particular proteins, brain areas or process have to be suppressed to elucidate their causal involvement in response to AD. Therefore, bioassays (forced swim test, tail suspension tests…) and more generally animal models have been designed that enable to induce a behavioral deficit after experimental manipulations (stressors during the developmental period, social defeat or unpredictable chronic stress during adulthood, chronic corticosterone). The induced modifications are then assessed via behavioral testing such as scoring of sucrose preference, of coat state, of grooming behavior, of anxiety-related behavior. It is usually observed that chronic ADs reverse the behavioral alterations that have been induced by the experimental manipulations and this enables to assess AD response (depending on the magnitude of the reversal that has been observed, and on the number of behavioral dimensions that have been counteracted). It is pivotal here to assess several different behaviors, as it can succeed that only a specific phenotype is reversed, and not the others as seen for example in David et al. (2009).
The main aim of this paper is to review the different potential predictors of response/non response to AD and discuss their clinical and practical implications. We will successively discuss the potential mechanisms and correlates of response to ADs with regard to some general clinical and pharmacological considerations and at the different levels of neurobiological understanding of AD mechanisms of action (Kupfer et al., 2012), with a particular consideration of the neuroanatomical, neurotransmission, molecular, and genetic levels, as well as to potential hormonal and neuroplasticity aspects (Table 1).
Poor Response to Antidepressant Therapy: Clinical Correlates
The efficacy of ADs has been strongly debated recently because of negative randomized controlled studies in which the response rates in the placebo control group were as high as 30–40% (Iovieno and Papakostas, 2012). Rather than arguing against the efficacy of ADs in MDD, these results indicated some methodological limitations of studies recently submitted to the European or US authorities (Khin et al., 2011). In particular, an inappropriately low baseline disease severity is likely the most problematic methodological flaw that eliminates the statistical significance of the difference in the response rate between the active and placebo groups (Kirsch et al., 2008; Fournier et al., 2010).
Another important methodological consideration is that even if the rating scales (such as the HDRS) on which the treatment response is assessed are robust and reliable, not all items of the scale (e.g., anxiety, somatic, early insomnia, hypochondriasis, and somatic symptoms) represent equal proportions of the observed change in the global score (Nelson et al., 2005). This could explain why a high level of anxiety symptoms could result in an underestimation of the response to treatment and why anxiety disorders are frequently associated with TRD (Souery et al., 2007). Other clinical characteristics are associated with a higher risk of low response to ADs. In particular, some MDD patients who meet the criteria for TRD are later revealed to suffer from bipolar disorder (Fekadu et al., 2012). Bipolar depression is less prone to respond to ADs than MDD (Gijsman et al., 2004), even though recent findings suggest that ADs may be as effective against bipolar depression as against MDD (Tondo et al., 2013; Vázquez et al., 2013).
Among clinical characteristics, older age has also been shown to be associated with a lower response rate to ADs. In a meta-analysis conducted on 15 late life MMD trials and 59 adult MDD trials, it was found that the response rate drops from 53.9% in adults to 45.2% in older patients (Tedeschini et al., 2011). In adolescents, response rate has been shown to be much higher, several studies reporting response rate at week 36 from 65 (Tao et al., 2009) to 80% (March et al., 2006), with a mean remission rate of 67% (Cox et al., 2012). Whether age itself may explain the difference remains unclear because somatic comorbidities may have a role in increasing the risk of non-response or partial response to ADs in older patients, particularly cardiac (Scherrer et al., 2012), cerebrovascular (Miller et al., 2002), and neurodegenerative disorders (Price et al., 2011).
Sex also constitutes a clinical characteristic associated with difference in AD treatment response. Indeed, a study suggested that men respond more favorably to imipramine than women, and premenopausal women more frequently to fluvoxamine than men (Vermeiden et al., 2010). In animal studies, Goel et al. (2011) showed that acute citalopram induced higher neuronal activation in male brain than in females or gonadectomized males. This suggests a gonadal hormone influence on complex interactions between serotonin and neural circuits that mediate the stress axis (see section HPA Axis Regulation below) and could therefore explain some of the sex differences in the response to AD.
The lack of response to ADs may also be the consequence of non-adherence to the treatment as the rate of adherence to ADs has been estimated to be particularly low, varying over a period of six months from 12.4% for patients taking older MAOIs and TCAs to 29.3% for those taking SSRIs and 33.6% for those taking SNRIs (Sheehan et al., 2008). In a 9-week follow-up period, up to 20% of patients missed taking their treatment for at least four consecutive days (Demyttenaere et al., 2001). This poor compliance has been shown to alter the estimation of response and remission rates (Akerblad et al., 2006). According to Jin et al. (2008), various factors contribute to non-compliance. Jeon-Slaughter (2012) found that low income level, combined with health insurance status and race/ethnicity, predicted non-adherence to ADs. This was confirmed in a recent review (Rivero-Santana et al., 2013) that demonstrated that younger people were less compliant than older patients and minority ethnic patients were less compliant than white patients. Non-pharmacological interventions can improve the adherence to AD treatments. Vergouwen et al. (2003) found that collaborative interventions in primary care were associated with clinical benefit, particularly in patients suffering from MDD who were prescribed adequate dosages of ADs.
Predictors of Poor Response to Antidepressant Therapy: Pharmacological Component
Drug Metabolism
Availability of the drug to its brain targets is one of the first requisite conditions of its effect and clinical impact. However, various conditions affect drug delivery such as its metabolism. Drug metabolism is altered by a wide range of factors such as age, rate of expression of drug metabolism systems, comorbid disease, sex, pregnancy, environment, drug dose, enzyme induction/inhibition, diet, genetics… For instance, younger people metabolize drugs faster than elderly people, men faster than women, and smokers faster than non-smokers. AD medications are metabolized mainly in the liver into compounds that are typically pharmacologically active but with different properties than the parent drug. Drug metabolism may result in poor response. Hepatic metabolism mainly occurs via cytochrome P450 (CYP) enzymes, which comprise more than 200 isoenzymes (mainly CYP1A2, CYP2B6, CYP2D6, CYP2C9, CYP2C19, and CYP3A4/5): they account for 75% of drug metabolism (Guengerich, 2008), particularly oxidative metabolism.
Variations in CYP genes have been shown to be associated with modified pharmacokinetic clearance of ADs. In humans, CYP is encoded by 18 families and 43 subfamilies of genes, corresponding to 57 genes and more than 59 pseudogenes. Thus, many different genes may alter CYP, some patients being poor and others ultrarapid metabolizers. When ultrarapid metabolizers are treated with typical doses of ADs, they have low plasma concentrations and do not respond. Polymorphisms in genes for crucial CYP enzymes, such as CYP2D6 or CYP2C19, alter the metabolism of ADs and thus their plasma concentrations (Brosen, 2004). Carriers of the non-functional allele of CYP2C19 exhibit a 42% decrease in clearance of the SSRI citalopram compared to carriers of the functional allele (Yin et al., 2006). Bondolfi et al. (1996) investigated seven non-responders to citalopram; six were extensive CYP2D6 metabolizers, and all seven were extensive CYP2C19 metabolizers. Furthermore, when administered an inhibitor of these two enzymes, citalopram serum levels rose in all subjects, as well as the therapeutic response. However, Grasmäder et al. (2004) found that plasma concentrations of several ADs were altered depending on the CYP2D6 and CYP2C19 genotype, even if this genotype was unrelated to clinical response. Peters et al. (2008) using subjects from the STAR*D study found no association between 15 polymorphisms of four P450 genes (CYP2D6, CYP2C19, CYP3A4, and CYP3A5) and citalopram response. More recently, Mrazek et al. (2011) examined data from the white non-Hispanic subjects who were treated with citalopram in the same STAR*D sample. They found a modest association between CYP2C19 variation and remission following citalopram, particularly in a subset of patients able to tolerate the medication. Thus, evidence on the association between genetic variations in CYP and AD response is inconsistent and likely depends on the patients and drug used.
Among the environmental factors that influence pharmacokinetics, smoking, treatment adherence, and concurrent medications are particularly important. There are numerous drug interactions with cigarette smoking. Suzuki et al. (2011) found that smoking status significantly affected fluvoxamine concentration (only in the low 50 mg/d dose group). Together, CYP2D6 genotype and smoking status explained 23% of the variance in fluvoxamine concentration in this group.
Failure to respond to or tolerate a drug may be related to comorbid medical conditions (hepatic or renal insufficiency, cardiovascular disease) and/or to its related polypharmacy. Comorbid medical conditions that alter hepatic function are likely to decrease the rate of drug metabolism. In addition, co-prescriptions increase the risk of drug-drug interactions with ADs in the treatment of comorbid illness. Drug interactions are more likely to occur with high-risk drugs, such as fluvoxamine, fluoxetine, paroxetine, and nefazodone (Richelson, 1998). Coelho and Brum Cde (2009) investigated the interactions between ADs and antihypertensive and glucose-lowering drugs at two primary care units and found that 19 of 29 patients were exposed to 47 interactions involving pharmacokinetic and pharmacodynamic mechanisms. When initiating a new prescription, the physician should select an AD while considering comorbid medical conditions, including dosage adjustment, possible drug interactions, adverse effects, and tolerability issues. The physician should also inform the patient about the influence of co-administered drugs and simultaneous intake of beverages and food on the bioavailability of drugs. For instance, grapefruit juice consumption increases the mean peak plasma concentrations and the concentration-time curve of sertraline (Ueda et al., 2009) and fluvoxamine (Hori et al., 2003).
In case of TRD, therapeutic drug monitoring is a valuable tool for tailoring the dosage of the prescribed medication to the individual characteristics. Dose titration is strongly recommended to achieve therapeutic plasma concentrations that allow for the highest probability of response or remission. In addition to drug concentration measurements, symptom rating by the treating physician at baseline and at week 2 is recommended (Hiemke et al., 2011). In certain situations, AD monitoring could be combined with pharmacogenetic metabolism tests (Hiemke et al., 2011). For instance, when the concentrations are outside the reference range, pharmacogenetic tests could be recommended to detect polymorphisms that give rise to slow/rapid metabolizers. Winner et al. (2013) recently demonstrated that pharmacogenomic-directed prescribing reduced the incidence of adverse drug reactions and improved the efficacy of AD medication regimens. Thus, pharmacogenomic testing to determine metabolic capacity may be a valuable strategy to recognize individuals who will obtain a therapeutic benefit from a drug.
Blood-Brain Barrier
Among the mechanisms of poor response to ADs, the drug efflux transporters that are expressed at the blood-brain barrier (BBB) and enable drugs to access the brain play a major role. The BBB is composed of brain capillary endothelial cells in association with pericytes and smooth muscle cells that delineate the circulating blood from glial cells and the neuronal terminals of the central nervous system. The BBB limits the traffic of substances to trans-cellular transport rather through the intercellular spaces because the tight junction system of the trans-membrane proteins acts as a physical barrier. Consequently, only lipophilic compounds of low molecular weight are able to cross the BBB. However, various transport systems ensure wider exchanges through the BBB, including the ATP-binding cassette (ABC transporters) system (for lipid-soluble molecules) (Benarroch, 2012). The human genome encodes 49 different ABC transporter proteins classified into seven subfamilies (ABCA to ABCG) (Dean et al., 2001). The P-glycoprotein (P-gp) encoded by the multi-drug resistance 1 (MDR1/ABCB1) gene, the breast cancer resistance protein encoded by the ABCG2 gene, and the multidrug resistance-associated proteins 4 and 5 are expressed by the brain endothelial cells and ensure active efflux of lipid-soluble molecules from the brain, reducing penetration of drugs into the brain. Compounds that interact with ABC transporters can be classified as substrates, modulators, or inhibitors. AD drugs interact mainly with P-gp. Thus, ADs that are substrates of P-gp are subject to greater efflux from brain endothelial cells and decreased penetration into the brain. Moreover, drug-drug interactions can also be the consequence of competing/synergic effects on P-gp. Several drugs, including cyclosporine, nifedipine, quinidine, and verapamil, are P-gp inhibitors (O'Brien et al., 2012). In vivo preclinical studies, particularly in P-gp knockout mice, have demonstrated that not all ADs are subject to the same level of limitation to brain penetration by P-gp (Uhr et al., 2000, 2003; Uhr and Grauer, 2003; Karlsson et al., 2013). Moreover, metabolites of some ADs may not be substrates of P-gp, in contrast to their parent molecules (Weiss et al., 2003; Grauer and Uhr, 2004; Wang et al., 2008a). Clinical evidence of the role of P-gp in the response to ADs has been provided by studies of variants of the ABCB1 gene. Several single nucleotide polymorphisms (SNPs) of the ABCB1 gene have been identified and associated with a decreased clinical response to AD (Kato et al., 2008; Uhr et al., 2008; Sarginson et al., 2010; Lin et al., 2011; Singh et al., 2012) as well as a poorer tolerance profile (Roberts et al., 2002; Jensen et al., 2012; de Klerk et al., 2012), although several studies failed to replicate these results (Laika et al., 2006; Mihaljevic Peles et al., 2008; Menu et al., 2010). Furthermore, endogenous and synthetic glucocorticoids also act as P-gp substrates (Ueda et al., 1992; Schinkel et al., 1995; Uhr et al., 2002). Hyperactivity of the hypothalamus-pituitary-adrenal (HPA) axis is one of the most consistent biological hallmarks of MDD, and it has been suggested that increased penetration of glucocorticoids into the brain as a result of P-gp inhibition may contribute to normalization of HPA axis hyperactivity in MDD (O'Brien et al., 2012). These data suggest evaluation of P-gp inhibition as an augmentation strategy for improving response to AD therapy.
Predictors of Poor Response to Antidepressant Therapy: Neurobiological Components
Based on the understanding we have of the neurobiological mechanisms of action of ADs, the response to ADs can be explored at the following levels: brain structures, neurotransmission, and molecular targets. We will now describe each of these mechanisms (Table 1).
Brain Structures and Response to Antidepressants
Various studies have explored brain changes associated with response to ADs by using electroencephalography (EEG) (alpha and theta activities) or neuroimaging (Functional magnetic resonance imaging: fMRI, Positron emission tomography: PET) that allow deducing potential mechanisms and markers of response to ADs.
Brain activity measurements by quantitative EEG in the resting state or during simple tasks have been used to predict response to ADs. Ulrich et al. (1986) observed increased alpha rhythmic activity (8–12 Hz) in the posterior regions of the head on both sides that was higher in amplitude on the dominant side in patients responding to amitriptyline. Subsequently, Knott et al. (1996) observed higher alpha and less theta rhythmic activity (4–7 Hz) among imipramine-responders than non-responders. Bruder et al. (2001) observed a difference in alpha asymmetry between fluoxetine responders and non-responders; non-responders displayed reduced alpha activity over the left hemisphere than the right, whereas responders tended to have the opposite asymmetry. Other studies focused on the brain regions associated with this altered alpha activity. Bruder et al. (2008) demonstrated that the difference between SSRI responders and non-responders involved occipital areas, where differences in alpha asymmetry were also observed. Theta activity was also investigated. EEG theta frequencies are generated in various brain areas, such as the medial prefrontal cortex (PFC), anterior cingulate cortex (ACC), hippocampus, amygdala, and ventral striatum. In the ACC, Pizzagalli et al. (2001) found an association between pre-treatment theta increases in rostral ACC and responses to nortriptyline. Mulert et al. (2007) reported similar findings with citalopram or reboxetine. This pre-treatment change in theta power in relationship to AD outcome has not been consistently observed (Cook et al., 2002). However, they demonstrated that the decrease in prefrontal cordance (i.e., the measure of quantitative EEG power that characterizes PFC function) that occurs after 1 week of treatment only in responders is also predictive of a better final outcome (Cook et al., 2002, 2009). In another study, Bares et al. (2008) found that reduction in the PFC theta quantitative EEG cordance value after the first week of treatment can predict the response to venlafaxine. Quantitative EEG measurements are now considered a promising clinical tool for predicting conventional AD treatment response (Leuchter et al., 2009, 2010). Interestingly, brain electrical activity has also been used to predict outcomes of non-conventional ADs, such as the NMDA receptor antagonist ketamine or non-pharmacological AD therapy, such as deep brain stimulation (DBS). For example, Duncan et al. (2013) recently demonstrated that measuring sleep slow wave activity (0.6–4 Hz) could predict ketamine response in individuals with TRD, whereas frontal theta quantitative EEG cordance has been shown to predict long-term AD response to subcallosal cingulate DBS in TRD patients (Broadway et al., 2012).
The involvement of the rostral ACC in TRD or treatment non-response is also supported by neuroimaging studies. Indeed, PET studies have observed increased baseline rostral ACC activity in MDD patients who subsequently responded to ADs (Mayberg et al., 1997; Saxena et al., 2003) while an fMRI study demonstrated that higher ACC activity during precise tasks, such as processing negative stimuli, was associated with the most robust treatment response (Davidson et al., 2003). Moreover, ACC activation during unsuccessful motor inhibition predicted response to escitalopram (Langenecker et al., 2007). Interestingly, a recent study showed that increased activity in the rostral ACC is a predictor not only of treatment outcome to conventional ADs but also to putative ADs, such as ketamine (Salvadore et al., 2009), or to non-pharmacological therapy, such as sleep deprivation (Wu et al., 1999). Non-response to ADs is correlated with low pre-treatment activity in the rostral ACC (Mayberg et al., 1997; Pizzagalli, 2011), which is one of the most reliable markers for predicting treatment outcome for conventional monoaminergic-acting ADs, intravenous ketamine treatment, and non-pharmacological treatments, such as electroconvulsive therapy (ECT) or repetitive transcranial magnetic stimulation (rTMS) (Pizzagalli, 2011). However, a low level of activity in the rostral ACC predicts a better outcome for cognitive behavioral therapy (CBT) (Fu et al., 2008; Pizzagalli, 2011; Roiser et al., 2012).
Gray matter brain volumes and, more recently, forebrain white matter integrity have also been measured in patients with MDD (first episode, remittent, or TRD). Frontolimbic gray matter areas (medial and orbital PFC) were reduced in the most severely depressed individuals (i.e., treatment-resistant/chronic group) (Serra-Blasco et al., 2013). Moreover, patients that did not respond to escitalopram exhibited microstructural abnormalities in fiber tracts connecting the cortex with limbic structures, such as the ACC (Alexopoulos et al., 2008). In addition, patients with TRD displayed abnormalities of both internal and external capsule integrity connecting cortical to subcortical nuclei and of the corpus callosum (Guo et al., 2012). Interestingly, these corticolimbic pathways are negatively impacted by adverse life events or by genetic polymorphism of the serotonin transporter (Alexopoulos et al., 2009; Choi et al., 2009), and corticolimbic connectivity increased as scores on the HDRS decreased during treatment (Anand et al., 2005). This suggests that assessing corticolimbic connectivity could be used to predict AD outcome. Further, the PFC region is one of the favorite targets for DBS in patients with TRD (Mayberg et al., 2005; Lozano et al., 2008; Malone et al., 2009). In addition, Dunkin et al. (2000) reported pre-treatment difference between fluoxetine responders and non-responders on PFC-related tasks reflecting executive dysfunction. Recently, Gupta et al. (2013) demonstrated that patients with TRD exhibited mildly reduced performance across all neurocognitive domains with a superimposed moderate impairment in verbal working memory. Finally, Bruder et al. (2004) investigated dichotic listening and demonstrated that patients who respond to SSRIs differed from non-responders in favoring left- over right-hemisphere processing of dichotic stimuli. Fluoxetine responders displayed greater left-hemisphere advantage for words and less right-hemisphere advantage for complex tones compared to non-responders. The cognitive, sensorial, or behavioral alterations shown in AD responders vs. non-responders are likely related to differences in the functioning of the brain areas underlying these functions, and thus, this suggests that brain alterations may also be valuable predictors of AD outcome.
The ACC is not the sole brain region whose activity can predict AD response. Indeed, improvement of MDD symptoms after AD has also been associated with lower pre-treatment metabolism (detected by PET) in the amygdala and thalamus and with higher pre-treatment metabolism in the medial PFC (Saxena et al., 2003). The hippocampus has also received some interest, and a recent study showed that MDD patients who met criteria for clinical remission at 8 weeks of AD treatment had larger pre-treatment hippocampal volumes than non-remitters, suggesting involvement of the hippocampus not only in the pathophysiology of MDD but also in treatment outcome (MacQueen et al., 2008; McKinnon et al., 2009). Larger hippocampal volume was also associated with a lower probability of relapse (Kronmüller et al., 2008). Microstructural abnormalities in the hippocampus have been suggested to indicate the vulnerability to treatment resistance (Ruhé et al., 2012). Further, a recent study pointed to a crucial role of the right insula: indeed, insula hypometabolism (detected via PET) was associated with poor response to escitalopram while the opposite was observed concerning cognitive behavior therapy, as insula hypometabolism was associated with good response to this cognitive therapeutic approach (McGrath et al., 2013). This indicates that neuroimaging can also help in selecting the appropriate treatment, and that brain activity does not predict poor response to treatment in a general way, but poor response to a particular therapeutic approach.
The crucial role of corticolimbic areas in the response to AD therapy has also been highlighted by recent impressive preclinical studies. These studies highlight the mechanisms underlying AD action in a given region. For example, Vialou et al. (2010) demonstrated that DeltaFosB induction in the nucleus accumbens was required for the effects of fluoxetine in the social defeat test, whereas Li et al. (2010) demonstrated that the effects of ketamine require synapse formation (particularly mTor-dependent synapse formation) in the PFC. Further, a recent paper showed that neural activity in the visual cortex during emotional processing predicts the response to scopolamine in depression (Furey et al., 2013).
Finally, TRD and/or treatment non-response can also be indirectly approached by studying the mechanism of non-pharmacological treatments, including vagus nerve stimulation therapy (VNS), ECT, and DBS. VNS is approved for the treatment of TRD. In VNS, a battery-powered generator is implanted in the chest wall and connected to a wire wrapped around the left vagus nerve in the neck. This wire sends intermittent electrical pulses through the vagal afferent connections to the brainstem, which may alter information processing in brain regions to which it projects, including the noradrenergic locus cœruleus and serotonergic nuclei as well as the thalamus, hypothalamus, central amygdala nucleus, bed nucleus of the stria terminalis, and nucleus accumbens, which are all disrupted during MDD. Functional imaging suggests that VNS leads to activity changes in the hypothalamus, orbitofrontal cortex, amygdala, hippocampus, insula, medial PFC, and cingulate (Bohning et al., 2001; Zobel et al., 2005), suggesting that VNS might aid in the recovery of MDD patients by reversing the pathophysiological alterations observed in MDD. ECT is based on the administration of brief electrical pulses to the scalp to induce depolarization of cortical neurons and thus brain seizures. It is among the most effective treatments for TRD and AD non-response. The mechanism of action of ECT remains a mystery, but the recent observation that electroconvulsive shock, the animal analogue of ECT, stimulates precursor cell proliferation in the subgranular zone of the dentate gyrus as well as hippocampal neurogenesis in the rat (Madsen et al., 2000) and monkey (Perera et al., 2007) suggests that pharmacological treatments and ECT may target a common endpoint, such as neuronal plasticity. Finally, because a number of clinical studies have demonstrated long-term effects of DBS in terms of improving symptoms of MDD (Bewernick et al., 2012; Lozano et al., 2012), the study of the neurobiological mechanisms underlying the beneficial effects of DBS will contribute to the understanding of TRD and of the mechanisms underlying poor response to AD. For example, a recent study by Schmuckermair et al. (2013) in a mouse model of TRD demonstrated that repeated nucleus accumbens DBS reversed depression-related behavior and coincided with changes in stress-induced neuronal activation of prelimbic, infralimbic, and cingulate areas in the lateral habenula and in the dentate gyrus of the hippocampus, where neurogenesis was also increased. In addition, Hamani et al. (2010) demonstrated that DBS in the rat ventromedial PFC induced a clear AD-like effect that was dependent on the integrity of the serotoninergic system.
Neurotransmission and Response to Antidepressants
Serotoninergic system
Because TCAs and SSRIs increase serotonin (5-HT: 5-hydroxytriptamine) availability in the synaptic cleft, the level of involvement of 5-HT in predicting AD response has been a focus of research. Consistent with predictions, tryptophan depletion (which is a precursor of the 5-HT synthesis) in subjects successfully treated with SSRI prevents AD effects (Delgado et al., 1999), indicating that 5-HT is essential for the action of SSRIs. More recently, the utilization of PET permitted the precise localization of this 5-HT action. SSRI treatment outcome was related to serotonin transporter (SERT) ratios between the raphe nuclei and serotonergic projection areas (habenula, amygdala, hippocampus, and subgenual cingulate cortex) before treatment (Lanzenberger et al., 2012). In animal models, depletion of 5-HT tissue content by para-chlorophenylalanine, an inhibitor of tryptophan hydroxylase (TPH), prevented the acute effects of SSRIs in a bioassay (O'Leary et al., 2007). Rodent studies also permitted the determination of the precise molecular target of AD action, particularly through the use of knockout mice for SERT and several 5-HT receptors. As expected, in a bioassay assessing effects of sub-acute injections of AD, the action of fluoxetine was abolished in SERT knockout mice, whereas the effect of a noradrenaline-preferring AD, desipramine, was conserved (Holmes et al., 2002). Once the blockade of the SERT has been achieved, the increased 5-HT in the synaptic cleft will bind to several 5-HT receptors. One of the most studied is the 5-HT1A postsynaptic receptor. In initial studies, SSRIs failed to alter immobility in 5-HT1A mutant mice, suggesting that 5HT1A receptors are critical for the expression of AD-like responses to SSRIs (Mayorga et al., 2001; Santarelli et al., 2003). However, a more complex picture later emerged as other studies showed that the effects of SSRIs were still present in 5-HT1A mutant mice (Guilloux et al., 2006; Holick et al., 2008). In fact, the use of 5-HT1A mutants did not allow to distinguish the pre- and the post-synaptic 5-HT1A receptors, and it is probable that the involvement of these receptors during chronic SSRIs is dynamic, as in the initial phase of the treatment the action of 5-HT on the 5-HT1A somatodendritic receptors may oppose the action on the post-synaptic receptors, while during the second phase, the presynaptic receptors get desensitized (see below). It has also been shown that desipramine, a NRI, still exerts an AD-like effect in 5HT1A receptor knockout mice despite reduced baseline immobility in the tail suspension test (Mayorga et al., 2001). However, the action on the postsynaptic 5-HT1A receptors is compromised by a concomitant action on 5-HT1A autoreceptors located in the raphe, which reduces the clinical efficacy of SSRIs and partly explains their delayed onset of action. Using conditional knockout mice for pre-synaptic 5-HT1A receptors, it was observed that reduction of 5-HT1A-autoreceptor expression with unchanged post-synaptic 5-HT1A receptor expression induced AD-like behavior and augmented SSRI effects (Richardson-Jones et al., 2010). These observations were recently confirmed using another experimental strategy, intra-raphe infusion of small-interfering RNA (siRNA) sequences directed toward the 5-HT1A autoreceptors. The siRNA decreased the expression of these receptors without affecting post-synaptic 5-HT1A receptors, concomitant with a robust and rapid AD-like effect (Bortolozzi, Castañé, Semakova, Santana, Alvarado and Cortés, 2012).
The involvement of 5-HT1B receptors is complex and depends upon the class of AD used, as well as on the methodology used to study their contribution (knockouts or pharmacological studies). Indeed, in 5HT1B receptor knockout mice, desipramine still has AD-like effects (Mayorga et al., 2001) while pharmacological blockade of the 5-HT1B receptors with GR 127935 or SB216641 (5-HT1B receptor antagonists) potentiated the effects of the drug (Tatarczyñska et al., 2004). Concerning SSRIs, an increased sensitivity to these compounds has been observed in mutants (single mutants for the 5-HT1B receptors) or after 5-HT1B receptors antagonists (Mayorga et al., 2001), whereas other studies reported SSRI resistance in the mutants (Trillat et al., 1998). Finally, in double knockout for 5-HT1A and the 5-HT1B receptors the response to acute SSRI was impacted, but not the one to chronic SSRIs (Guilloux et al., 2011). Other 5-HT receptors are required for AD action, including the 5-HT2B (Diaz et al., 2012), 5-HT2C (Cryan and Lucki, 2000a) and 5-HT4 receptors (Cryan and Lucki, 2000b; Lucas et al., 2007).
Genetic studies confirmed the involvement of 5-HT-related targets in the outcome of 5-HT therapy. For example, different studies indicated a polymorphism in the human gene encoding SERT (SLC6A4) as a predictor of response to AD. Heils et al. (1996) identified a functional polymorphism in the transcriptional region upstream of the SLC6A4-coding sequence (5-HTTLPR) that affects SLC6A4 expression, in which the l allele yields twofold higher SLC6A4 expression in the basal state than the s form. In a meta-analysis of the literature, Kato and Serretti (2010) demonstrated that the l variant is associated with a better response rate to AD than the s allele. Nakamura et al. (2000) further examined the polymorphic region and concluded that the alleles previously reported as s and l should be respectively divided into four (14A, 14B, 14C, and 14D) and six allelic variants (16A–16F). Smeraldi et al. (2006) demonstrated that among carriers of the l variant, 16F l carriers exhibited only a partial response to AD and 16D l carriers exhibited a marginally better response than 16A l allele carriers. Another polymorphism of SLC6A4 has been associated with an increased response to ADs; carriers with the 12 allele displayed a greater response to ADs, particularly when this allele was also associated with the l variant of the SERT-linked polymorphic region (5-HTTLPR) gene (see (Kato and Serretti, 2010) for a review). However, these findings have not been consistently replicated.
Serotonin biosynthesis involves TPH. This enzyme has two isoforms encoded by TPH1 and TPH2 genes, and SNPs of both genes have been reported. A significant association of TPH1 218A/C with response to ADs has been reported (Serretti et al., 2001) but these findings were not replicated (Kato et al., 2007).
A total of 50 SNPs of the 5-HT1A receptor gene have been described. Of particular interest is the 1019C/G (rs6295) SNP, which is related to the altered expression and function of 5-HT1A receptors. Indeed, the G allele is associated with an increase in 5-HT1A autoreceptors and thus a decrease in 5-HT neurotransmission. An association of this polymorphism with AD response was found only in Asian and not in Caucasian populations (Kato and Serretti, 2010). Two important common SNPs of the gene encoding the 5-HT2A receptor, 102T/C and 1438A/G, have been described: the C variant of the 102T/C SNP is associated with lower 5-HT2A receptor expression compared to the T variant, whereas the A variant of the 1438A/G SNP increases promoter activity compared to the G variant. Interestingly, the AD response was higher among the G/G genotype carriers than among the A/G or A/A carriers, although only in the Asian population (Kato and Serretti, 2010).
Polymorphisms combine with environmental factors in the etiology of MDD (El-Hage et al., 2009). For example, stressful life events predict a better response to escitalopram, but polymorphisms in 5-HT related genes, such as 5-HTTLPR, alter these effects (Keers et al., 2011). Assessment of 5-HT-related polymorphisms or pre-treatment PET of 5-HT molecular targets would improve the prediction of treatment response. Specifically, targeting 5-HT1A autoreceptors to eliminate their initial negative contribution during AD therapy would accelerate the onset of the beneficial effects of AD therapy.
Noradrenergic system
Drugs such as the TCA desipramine, and other TCAs that increase noradrenaline and serotonine neurotransmission, as well as the NRI reboxetine act by binding to the noradrenaline transporter which increases noradrenaline levels in the synaptic cleft, activating noradrenergic receptors. The enzyme dopamine beta-hydroxylase (Dbh) is responsible for the synthesis of epinephrine and noradrenaline. Mice unable to synthesize noradrenaline and epinephrine due to targeted disruption of the Dbh gene did not exhibit altered behavior in bioassays for depressive-like behavior, but ADs with a noradrenergic-preferring action such as desipramine or reboxetine failed to exert AD-like effects (Cryan et al., 2001). The same results were obtained with the MAOI pargyline and the atypical AD bupropion (Cryan et al., 2004). Surprisingly, the effects of the SSRIs fluoxetine, sertraline, and paroxetine were also absent or severely attenuated in Dbh knockout mice while the effects of another SSRI, citalopram, were not altered. Restoration of a normal noradrenergic level by L-threo-3,4-dihydroxyphenylserine restored the behavioral effects of both desipramine and paroxetine in the knockout mice, demonstrating that the AD non-response was due to altered noradrenergic function rather than developmental abnormalities resulting from chronic noradrenaline deficiency. Thus, noradrenaline may be involved in the effects of not only noradrenaline-acting AD drugs but also 5-HT-acting compounds. The beneficial action of noradrenergic-acting compounds in the treatment of MDD may be related to the α2-adrenergic receptor because the AD-like effects of desipramine are reversed by α2-adrenergic receptor antagonists, such as yohimbine or idazoxan (Yalcin et al., 2005; Zhang et al., 2009). Other adrenergic receptors are also involved: the cognitive effects of these treatments are mediated by post-synaptic α1-adrenergic receptors in the mPFC (Bondi et al., 2010). However, β2 or β3 adrenergic receptors do not appear to play a pivotal role in AD effects (Zhang et al., 2009; Stemmelin et al., 2010).
Finally, the involvement of organic cation transporter 2 (OCT2) in non-response to AD is also of note. OCT2 is involved in monoamine clearance, and mice deficient in this protein exhibited an altered response to AD (Bacq et al., 2012).
The contribution of the noradrenergic system to AD effects is largely confirmed by genetic data. Several polymorphisms of the gene encoding the noradrenalin transporter (SLC6A4) have been associated with AD response, particularly the rs2242466 (–182T/C) and rs5569 (1287G/A) polymorphisms (Shiroma et al., 2010). Catechol-O-methyltransferase (COMT) plays a pivotal role in the degradation of noradrenalin and dopamine. Interestingly, the Val158Met (rs4680) polymorphism of the COMT gene is associated with AD response (Benedetti et al., 2009; Tsai et al., 2009). Baune et al. (2008) demonstrated a negative influence of the higher activity COMT 158Val/Val genotype on AD response during the first 6 weeks of treatment, possibly due to the consequent decrease in dopamine availability. MAOA is involved in the degradation of monoamines, and polymorphisms of MAOA have been associated with fluoxetine, paroxetine, or mirtazapine response (Yu et al., 2005; Tadić et al., 2007; Domschke et al., 2008b).
Xu et al. (2011) found that early life stress may interact with the SLC6A2 polymorphism to alter AD response. Such effects might occur via epigenetic mechanisms, such as methylation or acetylation.
The assessment of polymorphisms of noradrenergic-related genes would improve the prediction of AD response. Combining ADs with α2-adrenergic receptor agonists could also improve the response rate or accelerate the onset of therapeutic action.
Other neurotransmission systems
One reason for non-response to ADs targeting monoaminergic neurotransmission is that these drugs may be ineffective in patients with alterations of other neurotransmission systems. For example, psychomotor retardation, a symptom exhibited by a subgroup of patients with MDD, has been related to a deficit in dopaminergic neurotransmission, particularly in the dorsolateral PFC. Consequently, these patients may preferentially benefit from treatment directly targeting dopaminergic neurotransmission. This has been explored by Taylor et al. (2006), who demonstrated that MDD patients with reduced pre-treatment performance on neuropsychological tests had a poor outcome after 12 weeks of fluoxetine treatment.
Substance P has also been suggested to have a key role in AD response. For example, decreased substance P in the cerebrospinal fluid has been associated with poor response to ADs (Carpenter et al., 2008). This conclusion is supported by convincing genetic data because the D allele of the angiotensin-converting enzyme (ACE) gene, which is related to higher ACE plasma levels, is associated with higher substance P levels and a more rapid onset of AD response (Baghai et al., 2004; Bondy et al., 2005; Narasimhan and Lohoff, 2012).
There is increasing evidence for the involvement of glutamate neurotransmission in MDD, and glutamate receptors are now being explored as targets for the treatment of MDD. For example, a clinical effect was observed when riluzole, a glutamatergic-acting compound, was added to ongoing AD therapy in TRD patients (Sanacora et al., 2007, 2008). Ketamine has a rapid AD effect and improves symptoms in TRD patients or in patients not responding to ECT (Ibrahim et al., 2011).
This is confirmed by genetic studies. For example, studies have found an association between a SNP of the dystrobrevin-binding-protein 1 gene, which is involved in glutamatergic neurotransmission, and AD response (Pae et al., 2007b; Kim et al., 2008). Furthermore, according to the STAR*D study, a SNP (rs1954787) of the GRIK4 (glutamate receptor ionotropic kainate 4) gene encoding kainate receptor subunit 1 is associated with response to citalopram (Mayer, 2007; Horstmann and Binder, 2009; Stawski et al., 2010; Narasimhan and Lohoff, 2012). This was confirmed in another cohort, the “Munich Antidepressant Response Signature” (MARS) project (Horstmann et al., 2008, 2010; Porcelli et al., 2011). Horstmann et al. (2010) identified another SNP (rs12800734) in the GRIK4 gene that is more strongly associated with response to treatment.
Data to support the involvement of GABA (gamma-aminobutyric acid) in the response to ADs are sparse, and this neurotransmitter does not appear to have a pivotal role in AD effects. However, mice with a deletion of the gene encoding the GABA transporter subtype 1 (GAT1), which transports extracellular GABA into presynaptic neurons, exhibited non-response to fluoxetine and amitriptyline (Liu et al., 2007a).
The endocannabinoid system is a modulatory system with both central and peripheral actions. Two cannabinoid receptors have been characterized: CNR1 located predominantly in the brain, and CNR2 in peripheral immune tissue and in glial cells in the central nervous system. Interestingly, knockout mice for the CNR1 receptor displayed attenuated response to desipramine and paroxetine (Steiner et al., 2008). Mitjans et al. (2012, 2013) demonstrated that genetic variability in endocannabinoid receptors could play a role in clinical response. Specifically, molecular variations in the CNR1 gene appear to differentiate the response to citalopram according to sex. In an analysis of SNP variability in the CNR1 gene, Domschke et al. (2008a) reported that the G allele of rs1049353 leads to increased risk of non-response to in female patients. These results suggest a role of the CNR1 gene in the etiology of MDD and clinical response to citalopram.
Leptin signaling may be involved in the pathophysiology of MDD. Kloiber et al. (2013) recently suggested an association of polymorphisms in the leptin gene with failure of AD to achieve remission. In this study, decreased leptin serum levels and reduced leptin mRNA expression were detected in patients with impaired treatment response, independently of their genotype configuration.
Endogenous opioids are involved in the regulation of mood and behavior. Three receptors (mu, delta, and kappa) interact with a family of endogenous opioid peptides (β-endorphin, enkephalins, and dynorphins). Studies in a mouse model of MDD have demonstrated that the combination of monoaminergic ADs and opioid receptor agonists can produce synergistic AD effects (Berrocoso and Mico, 2009). The mu receptor has been associated with citalopram response (Garriock et al., 2010). Haj-Mirzaian et al. (2013) demonstrated that elevated levels of endogenous opioids and nitric oxide due to bile-duct ligation in mice induced an AD-like effect. The effect was reversed by blockade of the nitrergic and opioid systems, suggesting an involvement of these systems in non-response. However, it is difficult to evaluate the risk-benefit balance of currently available mu opioid receptors agonists as ADs, partly because of their inherent abuse liability.
Neural Plasticity and Response to Antidepressants
Molecular aspects
Once the AD has increased monoamines in the synaptic cleft or bound to post-synaptic serotoninergic or noradrenergic receptors, it activates second messengers, such as the cyclic adenosine monophosphate (cAMP) pathway, leading to the production of cAMP-dependent protein kinase (PKA). This activation may in turn stimulate nuclear transcription factors, such as cAMP response element binding protein (CREB), via phosphorylation. Activated CREB enhances the transcription of many target genes, including brain-derived neurotrophic factor (BDNF), which exerts its effects mainly by binding to its specific receptor: the tyrosine receptor kinase B (TrkB). Consequently, non-response to AD has been investigated in relationship to alterations of these targets, particularly when polymorphisms of the genes encoding these proteins have been reported.
BDNF secretion and intercellular trafficking are related to a SNP in the BDNF gene that causes a valine to methionine substitution (Val66Met). A meta-analysis by Kato and Serretti (2010) indicated a better response to ADs in Met allele carriers. Other neurotrophic/growth factors have also been implicated in TRD and/or in AD response, including vascular endothelial growth factor (VEGF), fibroblast growth factor 2, and insulin-like growth factor 1 (IGF-1).
In CREB mutant mice (CREBaD), the SSRI fluoxetine and NRI desipramine still induce AD-like effect in bioassays for depressive behavior (Conti et al., 2002). This effect is accompanied by a desipramine-induced attenuation of the stress-induced activation of the HPA axis in both CREBaD-deficient and control mice (Conti et al., 2002). Interestingly, the AD-induced increase in BDNF in the cortex and hippocampus is absent in CREBaD-deficient mice (Conti et al., 2002), indicating that CREB may be a critical mediator of the transcriptional effects of AD.
Heterozygous bdnf +/− mice, in which the levels of BDNF in the brain are reduced by approximately half, and mice with an inducible deletion of bdnf in the forebrain exhibited blunted AD response in the forced swim test (Saarelainen et al., 2003; Monteggia et al., 2004). This result is related to the alteration of BDNF in the dentate gyrus of the hippocampus. Indeed, the selective deletion of BDNF in the dentate gyrus but not the CA1 region is sufficient to attenuate the effects of desipramine and citalopram in the forced swim test (Adachi et al., 2008). Further, in the BDNFMet/Met mice, chronic fluoxetine is no more able to reverse stress-related behavior (Chen et al., 2006; Yu et al., 2012), to increase hippocampal BDNF levels and to stimulate dentate gyrus synaptic plasticity (Bath et al., 2012). It is, however, to note that the action of desipramine is still present (Yu et al., 2012), which suggests a specific contribution of the 5-HT system in these effects. These findings are coherent with clinical findings as it was observed that serum levels of BDNF were low in MDD patients and this normalizes after remission (Molendijk et al., 2011). The molecular target of BDNF is TrkB, and mice with a conditional deletion of the TrkB gene restricted to the forebrain do not respond to ADs (Saarelainen et al., 2003). Similar results were observed in Aquaporin-4 (AQP4) knockout animals. AQP4 is a key molecule for maintaining water homeostasis in the CNS that is expressed in adult neural stem cells and astrocytes. AQP4 invalidation disrupts the chronic fluoxetine-induced enhancement of adult mouse hippocampal neurogenesis (which is also a process crucially involved in the AD response; see next section) as well as AD-evoked behavioral improvement under both basal conditions and a chronic, mild stress-evoked depressive state (Kong et al., 2009).
Stress induces changes in the brain that can persist for the lifespan. Variations in genes implicated in 5-HT neurotransmission may interact with environmental factors to influence AD response (El-Hage et al., 2009). One group of signaling pathways involved in the cellular stress response includes the family of mitogen-activated protein kinases (MAPKs). Bruchas et al. (2011), focusing on the dorsal raphe nucleus, a brain region in which corticotropin-releasing factor, kappa-opioid receptors, and 5-HT systems converge, demonstrated that social defeat stress causes an increase in the activity of the intracellular signaling molecule p38α MAPK. They demonstrated that p38α MAPK activation within the dorsal raphe nucleus is responsible for the ability of stress to trigger depressive-like states. They demonstrated that in 5-HT neurons, p38α MAPK acts to directly influence SERT trafficking and ultimately increase the rate of 5-HT reuptake.
Another key molecular player in the response to monoaminergic ADs is p11. Indeed, p11 is downregulated in several brain regions of MDD patients or in animal models of MDD (Svenningsson et al., 2006; Alexander et al., 2010), whereas ADs and ECT increase p11 in the frontal cortex and hippocampus (Svenningsson et al., 2006; Warner-Schmidt et al., 2010; Oh et al., 2013). This response appears to be related to BDNF because p11 is reduced in mice in which BDNF is downregulated and increased in mice in which BDNF is overexpressed (Warner-Schmidt et al., 2010). p11 is also regulated by glucocorticoids (Zhang et al., 2008) and pro-inflammatory cytokines (Warner-Schmidt et al., 2011). Mice that lack p11 throughout their body display a depressive-like phenotype (Svenningsson et al., 2006; Warner-Schmidt et al., 2009, 2010), and more interestingly, the response to AD drugs is reduced in these mice (Svenningsson et al., 2006; Egeland et al., 2011; Eriksson et al., 2013). Interestingly, the accumbens-restricted overexpression of p11 is sufficient to induce the depressive-like phenotype in mice but does not modify the response to ADs (Alexander et al., 2010), whereas ablation of p11 from pyramidal projection neurons in the cortical layer 5A does not alter depressive-like behavior but results in a diminished response to ADs (Schmidt et al., 2012a), indicating that the mechanisms underlying the pathophysiology of MDD might differ from the mechanisms underlying the response to ADs.
Recent studies have provided evidence for a BDNF gene × environment interaction. Stress and ADs have opposing actions on BDNF and neurogenesis. Stress decreases and ADs increase the expression of BDNF in the dentate gyrus granule cell layer. These changes contribute to the regulation of neurogenesis by stress and ADs (Duman and Li, 2012). Mutant mice with a heterozygous deletion of BDNF, which results in the expression of approximately half the normal levels of BDNF, display normal behavior under baseline conditions but exhibit a depressive phenotype upon exposure to stress and an altered response to ADs (Duman et al., 2007; Ibarguen-Vargas et al., 2009).
Other factors have been implicated in the response to AD (e.g., zinc and cytokines). Clinical evidence suggests that zinc deficiency induces depression- and anxiety-like behaviors. Zinc administration improves the efficacy of ADs in MDD patients and may be particularly relevant for TRD patients. Recent investigations on the molecular mechanisms responsible for these observations suggest a role for zinc in the regulation of neurotransmitters, antioxidant mechanisms, neurotrophic factors and neuronal precursor cells. The presynaptic release of zinc from axon terminals of glutamatergic neurons is prominent in the hippocampus, where zinc exerts complex pleiotropic effects on neuronal plasticity, neurogenesis, and neuronal viability, affecting learning, memory, and emotional regulation (Swardfager et al., 2013). The neuroprotective properties of zinc at physiological concentrations may be attributable to the blockade of excitotoxic Ca2+ in?ux and upregulation of cellular antioxidant systems. Chronic zinc administration can increase BDNF expression (Swardfager et al., 2013).
Conboy et al. (2011) explored the expression of macrophage migration inhibitory factor (MIF) in astrocytes and neurogenic cells in the subgranular zone of the rodent dentate gyrus and characterized its presence in stem cells, cells undergoing proliferation, and recently proliferated cells undergoing maturation. They found that MIF deficiency is associated with a phenotype characterized by increased anxiety- and depression-like behaviors. Furthermore, they determined that in the subgranular zone of the hippocampus, macrophage MIF expression is modulated in parallel with cell proliferation by stress, glucocorticoid levels, and fluoxetine. Both the genetic deletion of MIF and chronic treatment with the MIF antagonist Iso-1 resulted in reduced cell proliferation, and MIF deletion also abolished the enhanced proliferation induced by chronic fluoxetine.
Moon et al. (2012) provided evidence that macrophage MIF is regulated by long-term exercise and that it mediates induction of serotonin and neurotrophic factors, resulting in the amelioration of depressive behaviors in a rodent model. MIF was upregulated during both long-term voluntary exercise and repeated electroconvulsive seizure treatments. The authors demonstrated that MIF induced TPH2 and Bdnf expression in the rat brain along with ERK1/2 activation, resulting in an increase in 5-HT levels by MIF. In addition, direct intra-brain administration of MIF induced an AD-like response in the forced swim test. These results demonstrate that MIF induces AD-like behavior and mediates the effect of exercise on mood improvement (Moon et al., 2012). Physical activity has neuroimmune effects that are likely involved in enhanced neuroplasticity, reduced oxidative stress, increases in 5-HT, dopamine, and noradrenaline, and enhanced glucocorticoid sensitivity (Eyre et al., 2013). Thus, physical activity should be recommended in addition to AD therapy to improve drug response.
Cellular targets
In the past 15 years, the process leading to the generation of new neurons in the adult hippocampus (adult neurogenesis) has been shown to be compromised in rodent models of MDD; this effect is prevented by administration of monoaminergic AD drugs (see Hanson et al., 2011; Eisch and Petrik, 2012; Petrik et al., 2012; Bambico and Belzung, 2013; Tanti and Belzung, 2013a,b for recent reviews). These data have been corroborated by clinical studies (Boldrini et al., 2009, 2012, 2013), although some contradictory findings have been initially reported (Reif et al., 2006). Furthermore, these data extend beyond pharmacotherapy, as increase in hippocampal neurogenesis has also been observed after ECT (Malberg et al., 2000) or VNS (Revesz et al., 2008). However, increase in adult hippocampal neurogenesis alone is insufficient to induce AD-like effects (Sahay et al., 2011). Interestingly, studies have established that the ability of AD treatments to stimulate neurogenesis applies to all phases of the generation of new cells (proliferation, maturation) and is observed in the ventral and dorsal hippocampus (Tanti and Belzung, 2013b). Furthermore, adult hippocampal neurogenesis is not only a correlate of the therapeutic action of monoaminergic drugs but appears to be essential to achieve recovery. Indeed, abolition of hippocampal neurogenesis by focal X-ray hippocampal irradiation suppresses some and/or all behavioral effects of fluoxetine and/or imipramine in animals (Santarelli et al., 2003; Airan et al., 2007; Surget et al., 2008, 2011; Wang et al., 2008b; David et al., 2009; Perera et al., 2011). Similar results are observed after genetic ablation of adult neurogenesis (Lehmann et al., 2013). However, the situation is more complicated if we consider the effects of newly developed putative ADs: indeed, whereas the effects of a CNR1 ligand are abolished by suppression of neurogenesis (Jiang et al., 2005) as observed with monoaminergic-acting compounds, most of the AD-like effects of MCHR1 (Melanin-concentrating hormone receptor 1), CRH1 (corticotrophin-releasing hormone), or V1b (Vasopressin V1b) antagonists are not prevented by ablation of neurogenesis (David et al., 2007; Surget et al., 2008). This result indicates that although the ability of the treatments used in the clinic (which all target monoaminergic systems) to elicit remission relies on neurogenesis, the therapeutic effects of a drug can also be achieved via neurogenesis-independent mechanisms. For example, the dual orexine receptor almorexant induces AD-like effects but can decrease neurogenesis in some cases (Nollet et al., 2012), and rTMS suppresses the survival rate of proliferating cells (Czéh et al., 2002). The ability of new cells to contribute to recovery requires the incorporation of the new neurons into a functional network, which occurs when these cells are 4–8 weeks old. Thus, the use of an anti-mitotic agent to suppress neurogenesis revealed that the ablation of 2-week-old cells did not modify the effects of ADs (Bessa et al., 2009), whereas the 4-week-old cells are recruited after successful AD therapy to facilitate the regulation of the HPA axis after disruption by chronic stress (Surget et al., 2011). Therefore, it appears that hippocampal neurogenesis can be considered a process underlying AD non-response, related to the generation of new functional neurons.
The essential role of adult hippocampal neurogenesis in achieving remission is further supported by the observation that environmental factors that contribute to remission also act on adult hippocampal neurogenesis. Indeed, environmental enrichment and running both enable recovery and stimulate neurogenesis. Furthermore, the AD-like effects of environmental enrichment are suppressed by genetic abolition of neurogenesis (Schloesser et al., 2010). Factors associated with higher AD non-response, such as aging, are also related to lower hippocampal neurogenesis (Couillard-Despres et al., 2009).
Several hypotheses have been formulated regarding the function of adult hippocampal neurogenesis. According to some authors, this process might be crucial for pattern separation (Clelland er al., 2009; Sahay et al., 2011; Nakashiba et al., 2012; Tronel et al., 2012), a process that enables the differentiation of two closely overlapping stimuli. Thus, a deficit in pattern separation might lead to overgeneralization and cognitive bias. A restoration of such a deficit could contribute to remission. Others claim that hippocampal neurogenesis is required for contextual memory (Shors et al., 2001; Dupret et al., 2008; Deng et al., 2009; Trouche et al., 2009). Hippocampal neurogenesis is essential for regulation of the HPA axis (Schloesser et al., 2009; Snyder et al., 2011; Surget et al., 2011), and an increase in neurogenesis might facilitate recovery via normalization of HPA axis function. Finally, hippocampal neurogenesis have also been proposed to be crucial for anxiety behavior (Revest et al., 2009; Fuss et al., 2010; Mateus-Pinheiro et al., 2013) or executive functions (Burghardt et al., 2012); if restored, these functions would enable the subject to react more accurately to the environment. Interestingly, all of the above-mentioned processes are disturbed in MDD and could be essential to achieving remission (see Tanti and Belzung, 2013a,b, for reviews).
If hippocampal neurogenesis is crucial in achieving remission, any strategy that leads to an increase in the number of new neurons in this structure could facilitate recovery or accelerate the onset of therapeutic outcome when combined with ADs. Several processes have been shown to increase neurogenesis, including environment enrichment, physical exercise, and learning. Thus, one can speculate that combining any of these activities with AD treatment could increase the therapeutic outcome of pharmacotherapy.
Hormonal Targets and Response to Antidepressants
HPA axis regulation
It has been repeatedly demonstrated that ~50% of patients with MDD exhibit a dysfunction of the tuning of the HPA axis (Young et al., 1991; Holsboer, 2000; Pariante and Miller, 2001; Pariante, 2003), which can be measured using the dexamethasone-suppression test or combined dexamethasone/CRH test. Indeed, these patients exhibit dexamethasone non-suppression, indicating a defect in the negative feedback that enables the suppression of the secretion of glucocorticoids in healthy subjects. This dysregulation is restored after effective AD therapy, and interestingly, the therapeutic action of the treatment only occurs once normal feedback of the HPA axis has been restored (see Belzung and Billette De Villemeur, 2010, for a review), indicating that restoration of the HPA axis regulation is mandatory to enable recovery. Interestingly, normalization of HPA axis overactivity also occurs after successful ECT (Kling et al., 1994), indicating that this property goes beyond classical pharmacotherapy. Thus, the tuning of the HPA axis may be related to the ability to achieve a therapeutic outcome after treatment. If this outcome cannot be achieved, a resistance and/or non-response to treatment should be observed (Ising et al., 2007; Binder et al., 2009; Hennings et al., 2009; Schüle et al., 2009; Horstmann et al., 2010). This relationship has been demonstrated: a defect in HPA axis regulation is not only a signature of MDD or of remission but also a predictor of treatment non-response. Indeed, using the combined dexamethasone/CRH test, Brouwer et al. (2006) demonstrated that the AD response rate in high-ACTH patients was significantly lower than that in intermediate-ACTH patients. Similar results have been reported by Ising et al. (2007), who demonstrated that patients who do not exhibit a reduction of the cortisol response to a dexamethasone/CRH test after 2–3 weeks of treatment are not likely to respond to the current treatment. However, this has not been confirmed in two other studies (Paslakis et al., 2010; Carpenter et al., 2011). For example, Carpenter et al. (2011) rather found an increased pre-treatment cortisol after DEX/CRH to be associated with sertraline response. Possibly, rapid initial improvement of the cortisol response following DEX/CRH would be a more effective predictor of response outcome.
At the molecular level, the negative feedback on the HPA axis mainly occurs via the binding of glucocorticoids, such as cortisol or corticosterone, on specific receptors, such as the glucocorticoid receptors (GRs), which have low affinity for glucocorticoids and are thus only activated when a high level of stress hormones is present. The mode of action of GRs is very complex: in the absence of glucocorticoids, GRs are packaged into a large molecular complex consisting of chaperone heat-shock proteins, such as hsp90 and hsp70, and co-chaperones, such as FKBP51, which bind to the receptor and keep it inactive, thus decreasing the affinity of GRs for glucocorticoids. High FKBP51 induces inhibition of glucocorticoid-related GR activation, and thus, FKBP51 can be considered a negative feedback loop regulating GRs (Schmidt et al., 2012b). Interestingly, in MDD subjects, peripheral FKBP51 reduction is a marker for successful AD therapeutic outcome because FKBP51 levels are diminished in AD responders but not non-responders(Cattaneo et al., 2013).
The contribution of HPA regulation to treatment outcome after AD has been confirmed by studies investigating the contribution of genes involved in the tuning of the stress axis after treatment. Most findings concern the FKBP5 gene. Several reports have investigated the association of FKBP5 polymorphisms with the response to AD drugs. In 2004, a strong association between polymorphisms of the FKBP5 gene and the response to AD was observed in 280 depressed patients of the MARS sample (Binder et al., 2004). These results were subsequently replicated in the STAR*D sample (Lekman et al., 2008) as well as in another German sample (Kirchheiner et al., 2008). Interestingly, this relationship appears to be independent of the class of AD drug because it was observed in patients treated with TCAs, SSRIs, or mirtazapine. However, two smaller studies of Spanish and Korean subjects reported negative associations in small samples (Papiol et al., 2007; Tsai et al., 2007). In fact, in the patients carrying the genotypes associated with faster response to AD, pre-treatment HPA-axis dysregulation was low compared to other patients (Binder et al., 2004), which might have facilitated the normalization of the HPA-axis and thus recovery.
Polymorphisms of the GR gene have also been studied in association with treatment outcome. Studies have demonstrated that the BclI and ER22/23EK polymorphisms are associated with the response to ADs (Brouwer et al., 2006; van Rossum et al., 2006). Other association studies have focused on the influence of polymorphisms in the CRHR1, CRHR2, and CRH-BP genes. Allele G carriers at the rs2270007 site of the CRHR2 gene exhibited a worse response to the SSRI citalopram (Papiol et al., 2007) when compared to other alleles. Similarly, in a Chinese population, polymorphism at the rs242941 site of the CRH1 gene has been associated with fluoxetine response (Liu et al., 2007b). Finally, polymorphisms of genes related to hsp70 protein have also been associated with poor response to AD (Pae et al., 2007a). Taken together, these genetic studies strengthen the view that a pre-treatment defect in HPA axis regulation may predict poor treatment outcome.
Environmental studies further corroborate this assertion. Hyperactivity of the HPA axis is related to chronic stress. Interestingly, two recent publications reported that elevated stress prior to treatment was associated with a good response to SSRIs (Keers et al., 2010; Horacek et al., 2011). However, other studies have shown the opposite (Monroe et al., 1992) or no association (Bock et al., 2009). These discrepancies could be related to gene × environment interactions because the effects of stress on treatment outcome may vary according to genotype. For example, in patients carrying more than one s allele of the SERT gene (which is associated with increased vulnerability to stress), the occurrence of a stressor predicted a poor response to ADs (Mandelli et al., 2009; Keers et al., 2011). Similar findings were obtained using a large sample of patients treated with escitalopram; in patients carrying polymorphisms inducing vulnerability to stress, such as polymorphism at the rs1360780 site of the FKBP5 gene and at the rs110402 site of the CRHR1 gene, stressors were predictive of response to treatment (Keers and Uher, 2012).
Finally, evidence for the involvement of HPA axis feedback dysregulation in AD non-response is also provided by data from patients with diseases that are highly comorbid with MDD, such as Cushing's disease. Cushing's disease is a syndrome related to chronic glucocorticoid excess, due either to an overproduction of ACTH caused by a pituitary adenoma (66% of patients), to an adrenal adenoma or carcinoma (24% of patients), or to ectopic causes (10% of patients). Approximately half of the patients display MDD (Sonino and Fava, 2002; Pereira et al., 2010). Interestingly, MDD patients with Cushing's disease are poor responder to treatment with classical AD therapy (Sonino et al., 1986, 1993), whereas a good therapeutic outcome can be achieved by treatments that normalize the HPA overactivity (Sonino and Fava, 2002; Pereira et al., 2010). These results confirm that excessive function of the HPA axis is associated with AD non-response.
To improve drug response, pre-treatment HPA dysfunction should be assessed with the dexamethasone suppression test or, preferably, the combined dexamethasone/CRH, as this latter test appears to be more specific (Ising et al., 2007). Polymorphisms of HPA-related genes, such as the FKBP5, CRHR1, or GR genes, would also be highly relevant, even if they are not yet currently used in clinical practice. If these markers predict poor HPA function, one could predict that treatments targeting the HPA axis (CRF1 antagonists, V1b receptor antagonists, GR antagonists, FRBP51 inhibitors) could be useful therapeutic strategies to improve drug response as well as the onset of response when combined with more conventional ADs.
Thyrotropin releasing hormone (TRH)
The relationship between thyroid function and MDD is well-known. Indeed, hypothyroidism is associated with MDD, and TRH levels correlate with symptom severity (Bauer et al., 2009). Furthermore, MDD patients exhibit alterations in several markers of thyroid function, particularly of the thyroid hormone triiodothyronine (T3) (see Hage and Azar, 2012 for a review). Interestingly, T3 is widely used to improve the therapeutic outcome of AD drugs in patients who exhibit poor response to treatment (Aronson et al., 1996; Nierenberg et al., 2006), particularly in those treated with TCAs. Successful treatment with T3 alone has also been reported in some older studies (Feldmesser-Reiss, 1958; Flach et al., 1958; Wilson et al., 1974). These findings are further supported by preclinical evidence. Indeed, T3 alone elicited an AD-like effect in a bio-assay for AD response (Lifschytz et al., 2006, 2011), whereas the combination of T3 with chronic fluoxetine increased the effects of the SSRI (Brochet et al., 1987; Eitan et al., 2010). This effect appears to be related to thyroid function because it has also been shown that the effects of TCAs are reduced in rats in which hypothyroidism has been provoked by the addition of propylthiouracil to their drinking water (Martin et al., 1987). The effects of T3 occur via an interaction with nuclear thyroid hormone receptors (TRs). Four such receptors have been described: α-1, α-2, β-1, and β-2. It would be of interest to determine which of these receptors might mediate the effects of T3. A recent study demonstrated that administration of dronedarone, a specific TRα antagonist, prevented the AD-like effects of T3, suggesting that the TRα receptor is responsible for the effects of T3 (Lifschytz et al., 2011). This observation is consistent with the finding that TRα receptors are the most highly expressed TRs in the brain (Williams, 2008) as well as with the observation that mice in which this receptor is mutated display depressive-like behavior (Pilhatsch et al., 2010).
Few studies have investigated the association of polymorphisms of thyroid function-related genes with MDD and AD response. However, the relationship of a polymorphism of the deiodinase type I (D1) gene (D1 converts inactive T4 to active T3) with the ability of T3 to augment the effects of AD has been investigated (Papakostas et al., 2009).
Interestingly, the effects of T3 in patients undergoing AD therapy are particularly remarkable in some subcategories of patients, particularly in women (Altshuler et al., 2001; Agid and Lerer, 2003) and in patients with atypical MDD.
Further progress could certainly be achieved in improving AD response by (a) dosing pre-treatment T3 hormones in patients and (b) studying polymorphisms of thyroid function related genes. Such studies would enable the segregation of patients according to their thyroid hormone status and the initiation of co-therapy with T3 at the beginning of AD therapy in those patients with the highest thyroid dysfunction.
Discussion
To summarize, we know from various evidence from the litterature that response to ADs can be driven but also altered at various levels (Table 1). Derived from these data, several peripheral or central biomarkers can now enable predicting an increased risk of non-response to AD treatment. They include markers genetic testing (polymorphisms of P450, ABCB1, SERT, NERT, COMT, MAOA, leptin, FKBP5, hsp70, GR, BclI, CRHR1, CRHR2, BDBF, D1, TRα genes, etc), plasmatic dosage (BDNF, cortisol, FKBP51, etc.) or brain imaging. Predictors of treatment response can easily be uncovered by deduction from predictors of treatment non-response: for example, if a poor response to ADs is met in Val allele carriers of the BDNF gene, this also indicates that good response might be achieved in the Met carriers. If poor response is shown in patients having low rostral cingulate activity, high response is observed in patients exhibiting elevated pretreatment rostral cingulate activity.
Based on the potential mechanisms of response to AD, several practical implications may be deducted (see Figure 1). Some of these conclusions have entered clinical routine and are now part of practical recommendations, such as augmentation therapy by thyroid hormones, and are sustained by strong clinical evidence (Shelton et al., 2010). However, other innovative strategies may enter further clinical investigation through randomized controlled studies. Among these new strategies, controlling co-medications, smoking status, or weight, assessing pre-treatment hormonal status combined with dexamethasone/CRH tests and T3 levels, investigating SNPs of specific genes known to be implicated in AD non-response, and the use of brain imaging or EEG to identify brain changes known to predict poor response to ADs are among the most promising. A more systematic referral to therapeutic drug monitoring (plasma concentration quantification) would also be useful as it appears to be largely insufficiently used given the considerable inter-individual variability in the pharmacokinetic characteristics of drugs. It enables the adaptation of the dosage of ADs to achieve the plasma drug concentration that ensures the highest probability of response (Hiemke et al., 2011). New augmentation strategies could also be developed based on the evidence reported in the present review, such as combining monoaminergic treatment with physical activity, cognitive training, stress reduction, P-gp inhibitors, mu opioid receptors agonists, zinc, and drugs targeting hormonal dysfunction (T3, FKBP5 inhibitor, or CRH1 or V1b antagonists). Switching ADs from one ineffective AD to a similar or different class of ADs and from SSRI/SNRIs to TCAs, MAOIs, and non-conventional antidepressant drugs, such as NMDA antagonists, may be other valuable strategies, as well as switching to somatic therapies, such as ECT, rTMS, VNS, or DBS.
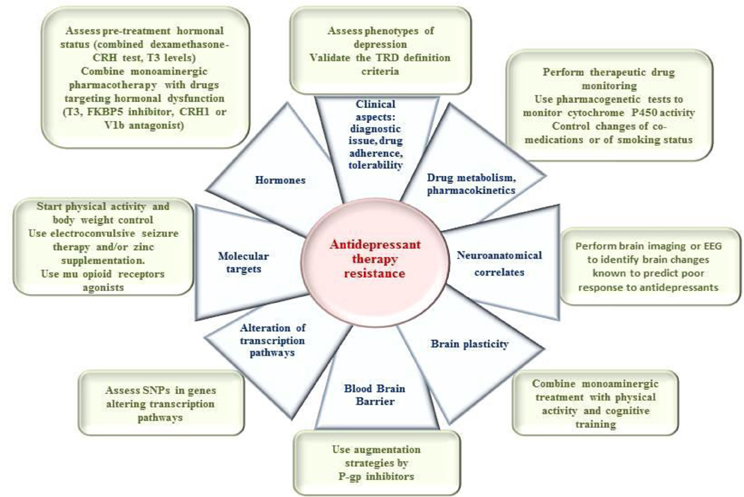
Figure 1. Mechanisms (in blue) associated with antidepressant therapy resistance and recommendations for clinical practice (in green).
One of the main obstacles to improving care strategies is the wide heterogeneity of patients labeled as suffering from TRD and/or showing insufficient response to conventional AD. This heterogeneity is the consequence of the relatively poor specificity of the criteria for diagnosis, which are still based on clinical evaluation; even the rating scales typically used to assess clinical response exhibit relatively good inter-rater and test-retest validity. Moving toward a more systematic use of biomarkers may improve the characterization of clinical phenotypes of MDD and their biological, imaging or genetic, proteomic and metabolomic correlates (Leuchter et al., 2010). As we have extensively reviewed, although TRD and/or treatment non-response is a considerable challenge to improving patient outcome and preventing severe complications of prolonged depressive states, it represents a unique opportunity to better understand mechanism of action of ADs and thus to better understand the pathophysiology of MDD and improve its clinical characterization. This potential makes research on TRD and/or treatment non-response a high priority for new research developments at both the preclinical and clinical levels.
Conflict of Interest Statement
The authors declare that the research was conducted in the absence of any commercial or financial relationships that could be construed as a potential conflict of interest.
References
Adachi, M., Barrot, M., Autry, A. E., Theobald, D., and Monteggia, L. M. (2008). Selective loss of brain-derived neurotrophic factor in the dentate gyrus attenuates antidepressant efficacy. Biol. Psychiatry 63, 642–649. doi: 10.1016/j.biopsych.2007.09.019
Agid, O., and Lerer, B. (2003). Algorithm-based treatment of major depression in an outpatient clinic: clinical correlates of response to a specific serotonin reuptake inhibitor and to triiodothyronine augmentation. Int. J. Neuropsychopharmacol. 6, 41–49. doi: 10.1017/S146114570200322X
Airan, R. D., Meltzer, L. A., Roy, M., Gong, Y., Chen, H., and Deisseroth, K. (2007). High-speed imaging reveals neurophysiological links to behavior in an animal model of depression. Science 317, 819–823. doi: 10.1126/science.1144400
Akerblad, A. C., Bengtsson, F., von Knorring, L., and Ekselius, L. (2006). Response, remission and relapse in relation to adherence in primary care treatment of depression: a 2-year outcome study. Int. Clin. Psychopharmacol. 21, 117–124. doi: 10.1097/01.yic.0000199452.16682.b8
Alexander, B., Warner-Schmidt, J., Eriksson, T., Tamminga, C., Arango-Lievano, M., Ghose, S., et al. (2010). Reversal of depressed behaviors in mice by p11 gene therapy in the nucleus accumbens. Science Transl. Med. 2, 54ra76. doi: 10.1126/scitranslmed.3001079
Alexopoulos, G. S., Murphy, C. F., Gunning-Dixon, F. M., Glatt, C. E., Latoussakis, V., Kelly, et al. (2009). Serotonin transporter polymorphisms, microstructural white matter abnormalities and remission of geriatric depression. J. Affect. Disord. 119, 132–141. doi: 10.1016/j.jad.2009.03.004
Alexopoulos, G. S., Murphy, C. F., Gunning-Dixon, F. M., Latoussakis, V., Kanellopoulos, D., Klimstra, S., et al. (2008). Microstructural white matter abnormalities and remission of geriatric depression. Am. J. Psychiatry 165, 238–244. doi: 10.1176/appi.ajp.2007.07050744
Altshuler, L. L., Bauer, M., Frye, M. A., Gitlin, M. J., Mintz, J., Szuba, M. P., et al. (2001). Does thyroid supplementation accelerate tricyclic antidepressant response. A review and meta-analysis of the literature. Am. J. Psychiatry 158, 1617–1622. doi: 10.1176/appi.ajp.158.10.1617
Anand, A., Li, Y., Wang, Y., Wu, J., Gao, S., Bukhari, L., et al. (2005). Antidepressant effect on connectivity of the mood-regulating circuit: an FMRI study. Neuropsychopharmacology 30, 1334–1344. doi: 10.1038/sj.npp.1300725
Andrade, L., Caraveo-Anduaga, J. J., Berglund, P., Bijl, R. V., de Graaf, R., Vollebergh, W., et al. (2003). The epidemiology of major depressive episodes: results from the International Consortium of Psychiatric Epidemiology (ICPE) Surveys. Int. J. Methods Psychiatr. Res. 12, 3–21. doi: 10.1002/mpr.138
Aronson, R., Offman, H., Joffe, R., and Naylor, C. (1996). Triiodothyronine augmentation in treatment of refractory depression. Arch. Gen. Psychiatry 53, 842–848. doi: 10.1001/archpsyc.1996.01830090090013
Bacq, A., Balasse, L., Biala, G., Guiard, B., Gardier, A. M., Schinkel, A., et al. (2012). Organic cation transporter 2 controls brain norepinephrine and serotonin clearance and antidepressant response. Mol. Psychiatry 17, 926–939. doi: 10.1038/mp.2011.87
Baghai, T., Schule, C., Zill, P., Deiml, T., Eser, D., Zwanzger, P., et al. (2004). The angiotensin I converting enzyme insertion/deletion polymorphism influences therapeutic outcome in major depressed women, but not in men. Neurosci. Lett. 363, 38–42. doi: 10.1016/j.neulet.2004.03.052
Bambico, F. R., and Belzung, C. (2013). “Novel Insights into depression and antidepressants: a synergy between synaptogenesis and neurogenesis,” in Neurogenesis and Neural Plasticity, Vol. 15. eds C. Belzung and P. Wigmore (Berlin; Heidelberg: Springer-Verlag), 243–291. doi: 10.1007/7854_2012_234
Bares, M., Brunovsky, M., Kopecek, M., Novak, T., Stopkova, P., Kozeny, J., et al. (2008). Early reduction in prefrontal theta QEEG cordance value predicts response to venlafaxine treatment in patients with resistant depressive disorder. Eur. Psychiatry 23, 350–355. doi: 10.1016/j.eurpsy.2008.03.001
Bath, K. G., Jing, D. Q., Dincheva, I., Neeb, C. C., Pattwell, S. S., Chao, M. V., et al. (2012). BDNF Val66Met impairs fluoxetine-induced enhancement of adult hippocampus plasticity. Neuropsychopharmacology 37, 1297–1304. doi: 10.1038/npp.2011.318
Bauer, M., Bschor, T., Pfennig, A., Whybrow, P. C., Angst, J., Versiani, M., et al. (2007). World Federation of Societies of Biological Psychiatry (WFSBP) guidelines for biological treatment of unipolar depressive disorders in primary care. World J. Biol. Psychiatry 8, 67–104. doi: 10.1080/15622970701227829
Bauer, M., Silverman, D. H. S., Schlagenhauf, F., London, E. D., Geist, C. L., van Herle, K., et al. (2009). Brain glucose metabolism in hypothyroidism: a positron emission tomography study before and after thyroid hormone replacement therapy. J. Clin. Endocrinol. Metab. 94, 2922–2929. doi: 10.1210/jc.2008-2235
Baune, B. T., Hohoff, C., Berger, K., Neumann, A., Mortensen, S., Roehrs, T., et al. (2008). Association of the COMT val158met variant with antidepressant treatment response in major depression. Neuropsychopharmacology 33, 924–932. doi: 10.1038/sj.npp.1301462
Belzung, C., and Billette De Villemeur, E. (2010). The design of new antidepressants: can formal models help. A first attempt using a model of the hippocampal control over the HPA axis based on a review from the literature. Behav. Pharmacol. 21, 677–689. doi: 10.1097/FBP.0b013e328340d630
Benarroch, E. E. (2012). Blood-brain barrier: recent developments and clinical correlations. Neurology 78, 1268–1276. doi: 10.1212/WNL.0b013e318250d8bc
Benedetti, F., Colombo, C., Pirovano, A., Marino, E., and Smeraldi, E. (2009). The catechol-O-methyltransferase Val(108/158)Met polymorphism affects antidepressant response to paroxetine in a naturalistic setting. Psychopharmacology 203, 155–160. doi: 10.1007/s00213-008-1381-7
Berlim, M. T., and Turecki, G. (2007). What is the meaning of treatment resistant/refractory major depression (TRD). A systematic review of current randomized trials. Eur. Neuropsychopharmacol. 17, 696–707. doi: 10.1016/j.euroneuro.2007.03.009
Berrocoso, E., and Mico, J. A. (2009). Cooperative opioid and serotonergic mechanisms generate superior antidepressant-like effects in a mice model of depression. Int. J. Neuropsychopharmacol. 12, 1033–1044. doi: 10.1017/S1461145709000236
Bessa, J. M., Ferreira, D., Melo, I., Marques, F., Cerqueira, J. J., Palha, J. A., et al. (2009). The mood-improving actions of antidepressants do not depend on neurogenesis but are associated with neuronal remodeling. Mol. Psychiatry 14, 764–773. doi: 10.1038/mp.2008.119
Bewernick, B. H., Kayser, S., Sturm, V., and Schlaepfer, T. E. (2012). Longterm effects of nucleus accumbens deep brain stimulation in treatment-resistant depression: evidence for sustained efficacy. Neuropsychopharmacology 37, 1975–1985. doi: 10.1038/npp.2012.44
Binder, E. B., Künzel, H. E., Nickel, T., Kern, N., Pfennig, A., Majer, M., et al. (2009). HPA-axis regulation at in-patient admission is associated with antidepressant therapy outcome in male but not in female depressed patients. Psychoneuroendocrinology 34, 99–109. doi: 10.1016/j.psyneuen.2008.08.018
Binder, E. B., Salyakina, D., Lichtner, P., Wochnik, G. M., Ising, M., Putz, B., et al. (2004). Polymorphisms in FKBP5 are associated with increased recurrence of depressive episodes and rapid response to antidepressant treatment. Nat. Genet. 36, 1319–1325. doi: 10.1038/ng1479
Bock, C., Bukh, J. D., Vinberg, M., Gether, U., and Kessing, L. V. (2009). Do stressful life events predict medical treatment outcome in first episode of depression. Soc. Psychiatry Psychiatr. Epidemiol. 44, 752–760. doi: 10.1007/s00127-008-0491-1
Bohning, D. E., Lomarev, M. P., Denslow, S., Nahas, Z., Shastri, A., and George, M. S. (2001). Feasibility of vagus nerve stimulation-synchronized blood oxygenation level-dependent functional MRI. Invest. Radiol. 36, 470–479. doi: 10.1097/00004424-200108000-00006
Boldrini, M., Hen, R., Underwood, M. D., Rosoklija, G. B., Dwork, A. J., Mann, J. J., et al. (2012). Hippocampal angiogenesis and progenitor cell proliferation are increased with antidepressant use in major depression. Biol. Psychiatry 72, 562–571. doi: 10.1016/j.biopsych.2012.04.024
Boldrini, M., Santiago, A. N., Hen, R., Dwork, A. J., Rosoklija, G. B., Tamir, H., et al. (2013). Hippocampal granule neuron number and dentate gyrus volume in antidepressant-treated and untreated major depression. Neuropsychopharmacology 38, 1068–1077. doi: 10.1038/npp.2013.5
Boldrini, M., Underwood, M. D., Hen, R., Rosoklija, G. B., Dwork, A. J., John Mann, J., et al. (2009). Antidepressants increase neural progenitor cells in the human hippocampus. Neuropsychopharmacology 34, 2376–2389. doi: 10.1038/npp.2009.75
Bondi, C. O., Jett, J. D., and Morilak, D. A. (2010). Beneficial effects of desipramine on cognitive function of chronically stressed rats are mediated byalpha1-adrenergic receptors in medial prefrontal cortex. Prog. Neuropsychopharmacol. Biol. Psychiatry 34, 913–923. doi: 10.1016/j.pnpbp.2010.04.016
Bondolfi, G., Chautems, C., Rochat, B., Bertschy, G., and Baumann, P. (1996). Non-response to citalopram in depressive patients: pharmacokinetic and clinical consequences of a fluvoxamine augmentation. Psychopharmacology (Berl.) 128, 421–425. doi: 10.1007/s002130050152
Bondy, B., Baghai, T. C., Zill, P., Schule, C., Eser, D., Deiml, T., et al. (2005). Genetic variants in the angiotensin I-converting-enzyme (ACE) and angiotensin II receptor (AT1) gene and clinical outcome in depression. Prog. Neuropsychopharmacol. Biol. Psychiatry 29, 1094–1099. doi: 10.1016/j.pnpbp.2005.03.015
Bortolozzi, A., Castañé, A., Semakova, J., Santana, N., Alvarado, G., Cortés, R., et al. (2012). Selective siRNA-mediated suppression of 5-HT1A autoreceptors evokes strong anti-depressant-like effects. Mol. Psychiatry 17, 612–623. doi: 10.1038/mp.2011.92
Broadway, J. M., Holtzheimer, P. E., Hilimire, M. R., Parks, N. A., Devylder, J. E., Mayberg, H. S., et al. (2012). Frontal theta cordance predicts 6-month antidepressant response to subcallosal cingulate deep brain stimulation for treatment-resistant depression: a pilot study. Neuropsychopharmacology 37, 1764–1772. doi: 10.1038/npp.2012.23
Brochet, D. M., Martin, P., Soubrié, P., and Simon, P. (1987). Triiodothyronine potentiation of antidepressant-induced reversal of learned helplessness in rats. Psychiatry Res. 21, 267–275. doi: 10.1016/0165-1781(87)90031-X
Brosen, K. (2004). Some aspects of genetic polymorphism in the biotransformation of antidepressants. Therapie 59, 5–12. doi: 10.2515/therapie:2004003
Brouwer, J. P., Appelhof, B. C., van Rossum, E. F., Koper, J. W., Fliers, E., Huyser, J., et al. (2006). Prediction of treatment response by HPA-axis and glucocorticoid receptor polymorphisms in major depression. Psychoneuroendocrinology 31, 1154–1163. doi: 10.1016/j.psyneuen.2006.08.001
Bruchas, M. R., Schindler, A. G., Shankar, H., Messinger, D. I., Miyatake, M., Land, B. B., et al. (2011). Selective p38α MAPK deletion in serotonergic neurons produces stress resilience in models of depression and addiction. Neuron 71, 498–511. doi: 10.1016/j.neuron.2011.06.011
Bruder, G. E., Sedoruk, J. P., Stewart, J. W., McGrath, P. J., Quitkin, F. M., and Tenke, C. E. (2008). Electroencephalographic alpha measures predict therapeutic response to a selective serotonin reuptake inhibitor antidepressant: pre- and post-treatment findings. Biol. Psychiatry 63, 1171–1177. doi: 10.1016/j.biopsych.2007.10.009
Bruder, G. E., Stewart, J. W., McGrath, P. J., Deliyannides, D., and Quitkin, F. M. (2004). Dichotic listening tests of functional brain asymmetry predict response to fluoxetine in depressed women and men. Neuropsychopharmacology 29, 1752–1761. doi: 10.1038/sj.npp.1300519
Bruder, G. E., Stewart, J. W., Tenke, C. E., McGrath, P. J., Leite, P., Bhattacharya, N., et al. (2001). Electroencephalographic and perceptual asymmetry differences between responders and nonresponders to an SSRI antidepressant. Biol. Psychiatry 48, 416–425. doi: 10.1016/S0006-3223(00)01016-7
Burghardt, N. S., Park, E. H., Hen, R., and Fenton, A. A. (2012). Adult-born hippocampal neurons promote cognitive flexibility in mice. Hippocampus 22, 1795–1808. doi: 10.1002/hipo.22013
Carpenter, L. L., Bayat, L., Moreno, F., Kling, M. A., Price, L. H., Tyrka, A. R., et al. (2008). Decreased cerebrospinal fluid concentrations of substance P in treatment-resistant depression and lack of alteration after acute adjunct vagus nerve stimulation therapy. Psychiatry Res. 157, 123–129. doi: 10.1016/j.psychres.2007.04.016
Carpenter, L. L., Tyrka, A. R., Lee, J. K., Tracy, A. P., Wilkinson, C. W., and Price, L. H. (2011). A placebo-controlled study of sertraline's effect on cortisol response to the dexamethasone/corticotropin-releasing hormone test in healthy adults. Psychopharmacology (Berl.) 218, 371–379. doi: 10.1007/s00213-011-2336-y
Cattaneo, A., Gennarelli, M., Uher, R., Breen, G., Farmer, A., Aitchison, K. J., et al. (2013). Candidate genes expression profile associated with antidepressants response in the GENDEP study: differentiating between baseline ‘predictors’ and longitudinal ‘targets’. Neuropsychopharmacology 38, 377–385. doi: 10.1038/npp.2012.191
Cheng, A. T., Chen, T. H., Chen, C. C., and Jenkins, R. (2000). Psychosocial and psychiatric risk factors for suicide. Case-control psychological autopsy study. Br. J. Psychiatry 177, 360–365. doi: 10.1192/bjp.177.4.360
Chen, Z. Y., Jing, D., Bath, K. G., Ieraci, A., Khan, T., Siao, C. J., et al. (2006). Genetic variant BDNF (Val66Met) polymorphism alters anxiety-related behavior. Science 314, 140–143. doi: 10.1126/science.1129663
Choi, J., Jeong, B., Rohan, M. L., Polcari, A. M., and Teicher, M. H. (2009). Preliminary evidence for white matter tract abnormalities in young adults exposed to parental verbal abuse. Biol. Psychiatry 65, 227–234. doi: 10.1016/j.biopsych.2008.06.022
Clelland, C. D., Choi, M., Romberg, C., Clemenson, G. D. Jr., Fragniere, A., Tyers, P., et al. (2009). A functional role for adult hippocampal neurogenesis in spatial pattern separation. Science. 325, 210–213. doi: 10.1126/science.1173215
Coelho, P. V., and Brum Cde, A. (2009). Interactions between antidepressants and antihypertensive and glucose lowering drugs among patients in the HIPERDIA Program, Coronel Fabriciano, Minas Gerais State, Brazil. Cad. Saude Publica. 25, 2229–2236. doi: 10.1590/S0102-311X2009001000013
Conboy, L., Varea, E., Castro, J. E., Sakouhi-Ouertatani, H., Calandra, T., Lashuel, H. A., et al. (2011). Macrophage migration inhibitory factor is critically involved in basal and fluoxetine-stimulated adult hippocampal cell proliferation and in anxiety, depression, and memory-related behaviors. Mol. Psychiatry 16, 533–547. doi: 10.1038/mp.2010.15
Conti, A. C., Cryan, J. F., Dalvi, A., Lucki, I., and Blendy, J. A. (2002). cAMP response element-binding protein is essential for the upregulation of brain-derived neurotrophic factor transcription, but not the behavioral or endocrine responses to antidepressant drugs. J. Neurosci. 22, 3262–3268.
Cook, I. A., Hunter, A. M., Abrams, M., Siegman, B., and Leuchter, A. F. (2009). Midline and right frontal brain function as a physiologic biomarker of remission in major depression. Psychiatry Res. 174, 152–157. doi: 10.1016/j.pscychresns.2009.04.011
Cook, I. A., Leuchter, A. F., Morgan, M., Witte, E., Stubbeman, W. F., Abrams, M., et al. (2002). Early changes in prefrontal activity characterize clinical responders to antidepressants. Neuropsychopharmacology 27, 120–131. doi: 10.1016/S0893-133X(02)00294-4
Couillard-Despres, S., Wuertinger, C., Kandasamy, M., Caioni, M., Stadler, K., Aigner, R., et al. (2009). Ageing abolishes the effects of fluoxetine on neurogenesis. Mol. Psychiatry 14, 856–864. doi: 10.1038/mp.2008.147
Cox, G. R., Callahan, P., Churchill, R., Hunot, V., Merry, S. N., Parker, A. G., et al. (2012). Psychological therapies versus antidepressant medication, alone and in combination for depression in children and adolescents. Cochrane Database Syst. Rev. 11:CD008324. doi: 10.1002/14651858.CD008324.pub2
Cryan, J. F., Dalvi, A., Jin, S. H., Hirsch, B. R., Lucki, I., and Thomas, S. A. (2001). Use of dopamine-beta-hydroxylase-deficient mice to determine the role of norepinephrine in the mechanism of action of antidepressant drugs. J. Pharmacol. Exp. Ther. 298, 651–657.
Cryan, J. F., and Lucki, I. (2000a). Antidepressant-like behavioral effects mediated by 5-Hydroxytryptamine2C receptors. J. Pharmacol. Exp. Ther. 295, 1120–1126.
Cryan, J. F., and Lucki, I. (2000b). 5-HT4 receptors do not mediate the antidepressant-like behavioral effects of fluoxetine in a modified forced swim test. Eur. J. Pharmacol. 409, 295–299. doi: 10.1016/S0014-2999(00)00858-X
Cryan, J. F., O'Leary, O. F., Jin, S. H., Friedland, J. C., Ouyang, M., Hirsch, B. R., et al. (2004). Norepinephrine-deficient mice lack responses to antidepressant drugs, including selective serotoninreuptake inhibitors. Proc. Natl. Acad. Sci. U.S.A. 101, 8186–8191. doi: 10.1073/pnas.0401080101
Czéh, B., Welt, T., Fischer, A. K., Erhardt, A., Schmitt, W., Müller, M. B., et al. (2002). Chronic psychosocial stress and concomitant repetitive transcranial magnetic stimulation: effects on stress hormone levels and adult hippocampal neurogenesis. Biol. Psychiatry 52, 1057–1065. doi: 10.1016/S0006-3223(02)01457-9
David, D. J., Klemenhagen, K. C., Holick, K. A., Saxe, M. D., Mendez, I., Santarelli, L., et al. (2007). Efficacy of the MCHR1 antagonist N-[3-(1-{[4-(3, 4-difluorophenoxy)phenyl]methyl}(4-piperidyl))-4-methylphenyl]-2-methylpropanamide (SNAP 94847) in mouse models of anxiety and depression following acute and chronic administration is independent of hippocampal neurogenesis. J. Pharmacol. Exp. Ther. 321, 237–248. doi: 10.1124/jpet.106.109678
David, D. J., Samuels, B. A., Rainer, Q., Wang, J.-W., Marsteller, D., Mendez, I., et al. (2009). Neurogenesis-dependent and -independent effects of fluoxetine in an animal model of anxiety/depression. Neuron 62, 479–493. doi: 10.1016/j.neuron.2009.04.017
Davidson, R. J., Irwin, W., Anderle, M. J., and Kalin, N. H. (2003). The neural substrates of affective processing in depressed patients treated with venlafaxine. Am. J. Psychiatry 160, 64–75. doi: 10.1176/appi.ajp.160.1.64
Dean, M., Rzhetsky, A., and Allikmets, R. (2001). The human ATP-binding cassette (ABC) transporter superfamily. Genome Res. 11, 1156–1166. doi: 10.1101/gr.GR-1649R
de Klerk, O. L., Nolte, I. M., Bet, P. M., Bosker, F. J., Snieder, H., den Boer, J. A., et al. (2012). ABCB1 gene variants influence tolerance to selective serotonin reuptake inhibitors in a large sample of Dutch cases with major depressive disorder. Pharmacogenomics J. 13, 349–353. doi: 10.1038/tpj.2012.16
Delgado, P. L., Miller, H. L., Salomon, R. M., Licinio, J., Krystal, J. H., Moreno, F. A., et al. (1999). Tryptophan-depletion challenge in depressed patients treated with desipramine or fluoxetine: implications for the role of serotonin in the mechanism of antidepressant action. Biol. Psychiatry 46, 212–220. doi: 10.1016/S0006-3223(99)00014-1
Demyttenaere, K., Mesters, P., Boulanger, B., Dewe, W., Delsemme, M. H., Gregoire, J., et al. (2001). Adherence to treatment regimen in depressed patients treated with amitriptyline or fluoxetine. J. Affect. Disord. 65, 243–252. doi: 10.1016/S0165-0327(00)00225-1
Deng, W., Saxe, M. D., Gallina, I. S., and Gage, F. H. (2009). Adult-born hippocampal dentate granule cells undergoing maturation modulate learning and memory in the brain. J. Neurosci. 29, 13532–13542. doi: 10.1523/JNEUROSCI.3362-09.2009
Diaz, S. L., Doly, S., Narboux-Nême, N., Fernández, S., Mazot, P., Banas, S. M., et al. (2012). 5-HT(2B) receptors are required for serotonin-selective antidepressant actions. Mol. Psychiatry 17, 154–163. doi: 10.1038/mp.2011.159
Domschke, K., Dannlowski, U., Ohrmann, P., Lawford, B., Bauer, J., Kugel, H., et al. (2008a). Cannabinoid receptor 1 (CNR1) gene: impact on antidepressant treatment response and emotion processing in major depression. Eur. Neuropsychopharmacol. 18, 751–759. doi: 10.1016/j.euroneuro.2008.05.003
Domschke, K., Hohoff, C., Mortensen, L. S., Roehrs, T., Deckert, J., Arolt, V., et al. (2008b). Monoamine oxidase A variant influences antidepressant treatment response in female patients with Major Depression. Prog. Neuropsychopharmacol. Biol. Psychiatry 32, 224–228. doi: 10.1016/j.pnpbp.2007.08.011
Duman, C. H., Schlesinger, L., Kodama, M., Russell, D. S., and Duman, R. S. (2007). A role for MAP kinase signaling in behavioral models of depression and antidepressant treatment. Biol. Psychiatry 61, 661–670. doi: 10.1016/j.biopsych.2006.05.047
Duman, R. S., and Li, N. (2012). A neurotrophic hypothesis of depression: role of synaptogenesis in the actions of NMDA receptor antagonists. Philos. Transl. R. Soc. Lond. B Biol. Sci. 367, 2475–2484. doi: 10.1098/rstb.2011.0357
Duncan, W. C. Jr., Selter, J., Brutsche, N., Sarasso, S., and Zarate, C. A. Jr. (2013). Baseline delta sleep ratio predicts acute ketamine mood response in major depressive disorder. J. Affect. Disord. 145, 115–119. doi: 10.1016/j.jad.2012.05.042
Dunkin, J. J., Leuchter, A. F., Cook, I. A., Kasl-Godley, J. E., Abrams, M., and Rosenberg-Thompson, S. (2000). Executive dysfunction predicts nonresponse to fluoxetine in major depression. J. Affect. Disord. 60, 13–23. doi: 10.1016/S0165-0327(99)00157-3
Dupret, D., Revest, J.-M., Koehl, M., Ichas, F., De Giorgi, F., Costet, P., et al. (2008). Spatial relational memory requires hippocampal adult neurogenesis. PLoS ONE 3:e1959. doi: 10.1371/journal.pone.0001959
Egeland, M., Warner-Schmidt, J., Greengard, P., and Svenningsson, P. (2011). Co-expression of serotonin 5-HT(1B) and 5-HT(4) receptors in p11 containing cells in cerebral cortex, hippocampus, caudate-putamen and cerebellum. Neuropharmacology 61, 442–450. doi: 10.1016/j.neuropharm.2011.01.046
Eisch, A. J., and Petrik, D. (2012). Depression and hippocampal neurogenesis: a road to remission. Science 338, 72–75. doi: 10.1126/science.1222941
Eitan, R., Landshut, G., Lifschytz, T., Einstein, O., Ben-Hur, T., and Lerer, B. (2010). The thyroid hormone, triiodothyronine, enhances fluoxetine-induced neurogenesis in rats: possible role in antidepressant-augmenting properties. Int. J. Neuropsychopharmacol. 13, 553–561. doi: 10.1017/S1461145709990769
El-Hage, W., Powell, J. F., and Surguladze, S. A. (2009). Vulnerability to depression: what is the role of stress genes in gene x environment interaction. Psychol. Med. 39, 1407–1411. doi: 10.1017/S0033291709005236
Eriksson, T. M., Alvarsson, A., Stan, T. L., Zhang, X., Hascup, K. N., Hascup, E. R., et al. (2013). Bidirectional regulation of emotional memory by 5-HT(1B) receptors involves hippocampal p11. Mol. Psychiatry. 18, 1096–1105. doi: 10.1038/mp.2012.130
Eyre, H. A., Papps, E., and Baune, B. T. (2013). Treating depression and depression-like behavior with physical activity: an immune perspective. Front. Psychiatry 4:3. doi: 10.3389/fpsyt.2013.00003
Fava, M. (2003). Diagnosis and definition of treatment-resistant depression. Biol. Psychiatry 53, 649–659. doi: 10.1016/S0006-3223(03)00231-2
Fekadu, A., Rane, L. J., Wooderson, S. C., Markopoulou, K., Poon, L., and Cleare, A. J. (2012). Prediction of longer-term outcome of treatment-resistant depression in tertiary care. Br. J. Psychiatry 201, 369–375. doi: 10.1192/bjp.bp.111.102665
Fekadu, A., Wooderson, S. C., Markopoulou, K., and Cleare, A. J. (2009). The Maudsley Staging Method for treatment-resistant depression: prediction of longer-term outcome and persistence of symptoms. J. Clin. Psychiatry 70, 952–957. doi: 10.4088/JCP.08m04728
Feldmesser-Reiss, E. E. (1958). The application of triiodothyronine in the treatment of mental disorders. J. Nerv. Ment. Dis. 127, 540–545. doi: 10.1097/00005053-195812000-00007
Flach, F. F., Celian, C. I., and Rawson, R. W. (1958). Treatment of psychiatric disorders with triiodothyronine. Am. J. Psychiatry 114, 841–842.
Fournier, J. C., DeRubeis, R. J., Hollon, S. D., Dimidjian, S., Amsterdam, J. D., Shelton, R. C., et al. (2010). Antidepressant drug effects and depression severity: a patient-level meta-analysis. JAMA 303, 47–53. doi: 10.1001/jama.2009.1943
Fu, C. H., Williams, S. C., Cleare, A. J., Scott, J., Mitterschiffthaler, M. T., Walsh, N. D., et al. (2008). Neural responses to sad facial expressions in major depression following cognitive behavioral therapy. Biol. Psychiatry 64, 505–512. doi: 10.1016/j.biopsych.2008.04.033
Furey, M. L., Drevets, W. C., Hoffman, E. M., Frankel, E., Speer, A. M., and Zarate, C. A. Jr. (2013). Potential of pretreatment neural activity in the visual cortex during emotional processing to predict treatment response to scopolamine in major depressive disorder. JAMA Psychiatry 70, 280–290. doi: 10.1001/2013.jamapsychiatry.60
Fuss, J., Ben Abdallah, N. M. B., Hensley, F. W., Weber, K.-J., Hellweg, R., and Gass, P. (2010). Deletion of running-induced hippocampal neurogenesis by irradiation prevents development of an anxious phenotype in mice. PLoS ONE 5:e12769. doi: 10.1371/journal.pone.0012769
Garriock, H. A., Tanowitz, M., Kraft, J. B., Dang, V. C., Peters, E. J., Jenkins, G. D., et al. (2010). Association of mu-opioid receptor variants and response to citalopram treatment in major depressive disorder. Am. J. Psychiatry 167, 565–573. doi: 10.1176/appi.ajp.2009.08081167
Gijsman, H. J., Geddes, J. R., Rendell, J. M., Nolen, W. A., and Goodwin, G. M. (2004). Antidepressants for bipolar depression: a systematic review of randomized, controlled trials. Am. J. Psychiatry 161, 1537–1547. doi: 10.1176/appi.ajp.161.9.1537
Goel, N., Plyler, K. S., Daniels, D., and Bale, T. L. (2011). Androgenic influence on serotonergic activation of the HPA stress axis. Endocrinology 152, 2001–2010. doi: 10.1210/en.2010-0964
Grasmäder, K., Verwohlt, P. L., Rietschel, M., Dragicevic, A., Müller, M., Hiemke, C., et al. (2004). Impact of polymorphisms of cytochrome-P450 isoenzymes 2C9, 2C19 and 2D6 on plasma concentrations and clinical effects of antidepressants in a naturalistic clinical setting. Eur. J. Clin. Pharmacol. 60, 329–336. doi: 10.1007/s00228-004-0766-8
Grauer, M. T., and Uhr, M. (2004). P-glycoprotein reduces the ability of amitriptyline metabolites to cross the blood brain barrier in mice after a 10-day administration of amitriptyline. J. Psychopharmacol. 18, 66–74. doi: 10.1177/0269881104042831
Guengerich, F. P. (2008). Cytochrome P450 and chemical toxicology. Chem. Res. Toxicol. 21, 70–83. doi: 10.1021/tx700079z
Guilloux, J. P., David, D. J., Guiard, B. P., Chenu, F., Repérant, C., Toth, M., et al. (2006). Blockade of 5-HT1A receptors by (+/−)-pindolol potentiates cortical 5-HT outflow, but not antidepressant-like activity of paroxetine: microdialysis and behavioral approaches in 5-HT1A receptor knockout mice. Neuropsychopharmacology 31, 2162–2172. doi: 10.1038/sj.npp.1301019
Guilloux, J. P., David, D. J., Xia, L., Nguyen, H. T., Rainer, Q., Guiard, B. P., et al. (2011). Characterization of 5-HT(1A/1B)-/- mice: an animal model sensitive to anxiolytic treatments. Neuropharmacology 61, 478–488. doi: 10.1016/j.neuropharm.2011.02.009
Guo, W. B., Liu, F., Chen, J. D., Xu, X. J., Wu, R. R., Ma, C. Q., et al. (2012). Altered white matter integrity of forebrain in treatment-resistant depression: a diffusion tensor imaging study with tract-based spatial statistics. Prog. Neuropsychopharmacol. Biol. Psychiatry 38, 201–206. doi: 10.1016/j.pnpbp.2012.03.012
Gupta, M., Holshausen, K., Best, M. W., Jokic, R., Milev, R., Bernard, T., et al. (2013). Relationships among neurocognition, symptoms, and functioning in treatment-resistant depression. Arch. Clin. Neuropsychol. 28, 272–281. doi: 10.1093/arclin/act002
Hage, M. P., and Azar, S. T. (2012). The link between thyroid function and depression. J. Thyroid Res. 2012:8. doi: 10.1155/2012/590648
Haj-Mirzaian, A., Hamzeh, N., Javadi-Paydar, M., Abdollahzadeh Estakhri, M. R., and Dehpour, A. R. (2013). Resistance to depression through interference of opioid and nitrergic systems in bile-duct ligated mice. Eur. J. Pharmacol. 708, 38–43. doi: 10.1016/j.ejphar.2013.03.013
Hamani, C., Diwan, M., Macedo, C. E., Brandão, M. L., Shumake, J., Gonzalez-Lima, F., et al. (2010). Antidepressant-like effects of medial prefrontal cortex deep brain stimulation in rats. Biol. Psychiatry 67, 117–124. doi: 10.1016/j.biopsych.2009.08.025
Hamilton, M. (1960). A rating scale for depression. J. Neurol. Neurosurg. Psychiatry 23, 56–62. doi: 10.1136/jnnp.23.1.56
Hanson, N. D., Owens, M. J., and Nemeroff, C. B. (2011). Depression, antidepressants, and neurogenesis: a critical reappraisal. Neuropsychopharmacology 36, 2589–2602. doi: 10.1038/npp.2011.220
Heils, A., Teufel, A., Petri, S., Stöber, G., Riederer, P., Bengel, D., et al. (1996). Allelic variation of human serotonin transporter gene expression. J. Neurochem. 66, 2621–2624. doi: 10.1046/j.1471-4159.1996.66062621.x
Hennings, J. M., Owashi, T., Binder, E. B., Horstmann, S., Menke, A., Kloiber, S., et al. (2009). Clinical characteristics and treatment outcome in a representative sample of depressed inpatients—findings from the Munich Antidepressant Response Signature (MARS) project. J. Psychiatr. Res. 43, 215–229. doi: 10.1016/j.jpsychires.2008.05.002
Hiemke, C., Baumann, P., Bergemann, N., Conca, A., Dietmaier, O., Egberts, K., et al. (2011). AGNP consensus guidelines for therapeutic drug monitoring in psychiatry: update 2011. Pharmacopsychiatry 44, 195–235. doi: 10.1055/s-0031-1286287
Holick, K. A., Lee, D. C., Hen, R., and Dulawa, S. C. (2008). Behavioral effects of chronic fluoxetine in BALB/cJ mice do not require adult hippocampal neurogenesis or the serotonin 1A receptor. Neuropsychopharmacology 33, 406–417. doi: 10.1038/sj.npp.1301399
Holmes, A., Yang, R. J., Murphy, D. L., and Crawley, J. N. (2002). Evaluation of antidepressant-related behavioral responses in mice lacking the serotonin transporter. Neuropsychopharmacology 27, 914–923. doi: 10.1016/S0893-133X(02)00374-3
Holsboer, F. (2000). The corticosteroid receptor hypothesis of depression. Neuropsychopharmacology 23, 477–501. doi: 10.1016/S0893-133X(00)00159-7
Horacek, J., Rozehnalova, E., Rosslerova, H., and Dvorak, A. (2011). The influence of stressful life events on development of depression and treatment response in the population of patients in higher age. Ceska Slov. Psychiatr. 106, 311–317.
Hori, H., Yoshimura, R., Ueda, N., Eto, S., Shinkai, K., Sakata, S., et al. (2003). Grapefruit juice-fluvoxamine interaction - is it risky or not. J. Clin. Psychopharmacol. 23, 422–424. doi: 10.1097/01.jcp.0000085423.74359.f2
Horstmann, S., and Binder, E. B. (2009). Pharmacogenomics of antidepressant drugs. Pharmacol. Ther. 124, 57–73. doi: 10.1016/j.pharmthera.2009.06.007
Horstmann, S., Lucae, S., Menke, A., Hennings, J. M., Ising, M., Roeske, D., et al. (2010). Polymorphisms in GRIK4, HTR2A, and FKBP5 show interactive effects in predicting remission to antidepressant treatment. Neuropsychopharmacology 35, 727–740. doi: 10.1038/npp.2009.180
Horstmann, S., Lucae, S., Menke, A., Ising, M., Muller-Myhsok, B., Holsboer, F., et al. (2008). Association of GRIK4 and HTR2A genes with antidepressant treatment in the MARS cohort of depressed inpatients. Eur. Neuropsychopharm. 18, S214–S215. doi: 10.1016/S0924-977X(08)70255-4
Ibarguen-Vargas, Y., Surget, A., Vourc'h, P., Leman, S., Andres, C. R., Gardier, A. M., et al. (2009). Deficit in BDNF does not increase vulnerability to stress but dampens antidepressant-like effects in the unpredictable chronic mild stress. Behav. Brain Res. 202, 245–251. doi: 10.1016/j.bbr.2009.03.040
Ibrahim, L., Diazgranados, N., Luckenbaugh, D. A., Machado-Vieira, R., Baumann, J., Mallinger, A. G., et al. (2011). Rapid decrease in depressive symptoms with an N-methyl-d-aspartate antagonist in ECT-resistant major depression. Prog. Neuropsychopharmacol. Biol. Psychiatry 35, 1155–1159. doi: 10.1016/j.pnpbp.2011.03.019
Iovieno, N., and Papakostas, G. I. (2012). Correlation between different levels of placebo response rate and clinical trial outcome in major depressive disorder: a meta-analysis. J. Clin. Psychiatry 73, 1300–1306. doi: 10.4088/JCP.11r07485
Ising, M., Horstmann, S., Kloiber, S., Lucae, S., Binder, E. B., Kern, N., et al. (2007). Combined dexamethasone/corticotropin releasing hormone test predicts treatment response in major depression—a potential biomarker. Biol. Psychiatry 62, 47–54. doi: 10.1016/j.biopsych.2006.07.039
Jensen, B. P., Roberts, R. L., Vyas, R., Bonke, G., Jardine, D. L., and Begg, E. J. (2012). Influence of ABCB1 (P-glycoprotein) haplotypes on nortriptyline pharmacokinetics and nortriptyline-induced postural hypotension in healthy volunteers. Br. J. Clin. Pharmacol. 73, 619–628. doi: 10.1111/j.1365-2125.2011.04126.x
Jeon-Slaughter, H. (2012). Economic factors in of patients' nonadherence to antidepressant treatment. Soc. Psychiatry Psychiatr. Epidemiol. 47, 1985–1998. doi: 10.1007/s00127-012-0497-6
Jiang, W., Zhang, Y., Xiao, L., Van Cleemput, J., Ji, S.-P., Bai, G., et al. (2005). Cannabinoids promote embryonic and adult hippocampus neurogenesis and produce anxiolytic- and antidepressant-like effects. J. Clin. Investig. 115, 3104–3116. doi: 10.1172/JCI25509
Jin, J., Sklar, G. E., Min Sen Oh, V., and Chuen Li, S. (2008). Factors affecting therapeutic compliance: a review from the patient's perspective. Ther. Clin. Risk Manag. 4, 269–286.
Karlsson, L., Carlsson, B., Hiemke, C., Ahlner, J., Bengtsson, F., Schmitt, U., et al. (2013). Altered brain concentrations of citalopram and escitalopram in P-glycoprotein deficient mice after acute and chronic treatment. Eur. Neuropsychopharmacol. 23, 1636–1644. doi: 10.1016/j.euroneuro.2013.01.003
Kato, M., Fukuda, T., Serretti, A., Wakeno, M., Okugawa, G., Ikenaga, Y., et al. (2008). ABCB1 (MDR1) gene polymorphisms are associated with the clinical response to paroxetine in patients with major depressive disorder. Prog. Neuropsychopharmacol. Biol. Psychiatry 32, 398–404. doi: 10.1016/j.pnpbp.2007.09.003
Kato, M., and Serretti, A. (2010). Review and meta-analysis of antidepressant pharmacogenetic findings in major depressive disorder. Mol. Psychiatry 15, 473–500. doi: 10.1038/mp.2008.116
Kato, M., Wakeno, M., Okugawa, G., Fukuda, T., Azuma, J., Kinoshita, T., et al. (2007). No association of TPH1 218A/C polymorphism with treatment response and intolerance to SSRIs in Japanese patients with major depression. Neuropsychobiology 56, 167–171. doi: 10.1159/000119734
Keers, R., and Uher, R. (2012). Gene-environment interaction in major depression and antidepressant treatment response. Curr. Psychiatry Rep. 14, 129–137. doi: 10.1007/s11920-011-0251-x
Keers, R., Uher, R., Gupta, B., Rietschel, M., Schulze, T. G., Hauser, J., et al. (2010). Stressful life events, cognitive symptoms of depression and response to antidepressants in GENDEP. J. Affect. Disord. 127, 337–342. doi: 10.1016/j.jad.2010.06.011
Keers, R., Uher, R., Huezo-Diaz, P., Smith, R., Jaffee, S., Rietschel, M., et al. (2011). Interaction between serotonin transporter gene variants and life events predicts response to antidepressants in the GENDEP project. Pharmacogenomics J. 11, 138–145. doi: 10.1038/tpj.2010.14
Kessler, R. C., Birnbaum, H. G., Shahly, V., Bromet, E., Hwang, I., McLaughlin, K. A., et al. (2010). Age differences in the prevalence and co-morbidity of DSM-IV major depressive episodes: results from the WHO world mental health survey initiative. Depress. Anxiety. 27, 351–364. doi: 10.1002/da.20634
Kessler, R. C., and Ustun, T. B. (2004). The World Mental Health (WMH) Survey Initiative Version of the World Health Organization (WHO) Composite International Diagnostic Interview (CIDI). Int. J. Methods Psychiatr. Res. 13, 93–121. doi: 10.1002/mpr.168
Khin, N. A., Chen, Y. F., Yang, Y., Yang, P., and Laughren, T. P. (2011). Exploratory analyses of efficacy data from major depressive disorder trials submitted to the US Food and Drug Administration in support of new drug applications. J. Clin. Psychiatry 72, 464–472. doi: 10.4088/JCP.10m06191
Kim, J. J., Mandelli, L., Pae, C. U., De Ronchi, D., Jun, T. Y., Lee, C., et al. (2008). Is there protective haplotype of dysbindin gene (DTNBP1) 3 polymorphisms for major depressive disorder. Prog. Neuropsychopharmacol. Biol. Psychiatry 32, 375–379. doi: 10.1016/j.pnpbp.2007.09.002
Kirchheiner, J., Lorch, R., Lebedeva, E., Seeringer, A., Roots, I., Sasse, J., et al. (2008). Genetic variants in FKBP5 affecting response to antidepressant drug treatment. Pharmacogenomics 9, 841–846. doi: 10.2217/14622416.9.7.841
Kirsch, I., Deacon, B. J., Huedo-Medina, T. B., Scoboria, A., Moore, T. J., and Johnson, B. T. (2008). Initial severity and antidepressant benefits: a meta-analysis of data submitted to the Food and Drug Administration. PLoS Med. 5:e45. doi: 10.1371/journal.pmed.0050045
Kling, M. A., Geracioti, T. T., Licinio, J., Michelson, D., Oldfield, E. H., and Gold, P. W. (1994). Effects of electroconvulsive therapy on the CRH–ACTH– cortisol system in melancholic depression. Psychopharmacol. Bull. 30, 489–494.
Kloiber, S., Ripke, S., Kohli, M. A., Reppermund, S., Salyakina, D., Uher, R., et al. (2013). Resistance to antidepressant treatment is associated with polymorphisms in the leptin gene, decreased leptin mRNA expression, and decreased leptin serum levels. Eur. Neuropsychopharmacol. 23, 653–662. doi: 10.1016/j.euroneuro.2012.08.010
Knott, V. J., Telner, J. I., Lapierre, Y. D., Browne, M., and Horn, E. R. (1996). Quantitative EEG in the prediction of antidepressant response to imipramine. J. Affect. Disord. 39, 175–184. doi: 10.1016/0165-0327(96)00003-1
Kong, H., Sha, L. L., Fan, Y., Xiao, M., Ding, J. H., Wu, J., et al. (2009). Requirement of AQP4 for antidepressive efficiency of fluoxetine: implication in adult hippocampal neurogenesis. Neuropsychopharmacology. 34, 1263–1276. doi: 10.1038/npp.2008.185
Krishnan, V., and Nestler, E. J. (2008). The molecular neurobiology of depression. Nature 455, 894–902. doi: 10.1038/nature07455
Kronmüller, K. T., Pantel, J., Köhler, S., Victor, D., Giesel, F., Magnotta, V. A., et al. (2008). Hippocampal volume and 2-year outcome in depression. Br. J. Psychiatry 192, 472–473. doi: 10.1192/bjp.bp.107.040378
Kupfer, D. J., Frank, E., and Phillips, M. L. (2012). Major depressive disorder: new clinical, neurobiological, and treatment perspectives. Lancet 379, 1045–1055. doi: 10.1016/S0140-6736(11)60602-8
Laika, B., Leucht, S., and Steimer, W. (2006). ABCB1 (P-glycoprotein/MDR1) gene G2677T/a sequence variation (polymorphism): lack of association with side effects and therapeutic response in depressed inpatients treated with amitriptyline. Clin. Chem. 52, 893–895. doi: 10.1373/clinchem.2006.066605
Lam, R. W. (2012). Onset, time course and trajectories of improvement with antidepressants. Eur. Neuropsychopharmacol. 22(Suppl. 3), S492–S498. doi: 10.1016/j.euroneuro.2012.07.005
Langenecker, S. A., Kennedy, S. E., Guidotti, L. M., Briceno, E. M., Own, L. S., Hooven, T., et al. (2007). Frontal and limbic activation during inhibitory control predicts treatment response in major depressive disorder. Biol. Psychiatry 62, 1272–1280. doi: 10.1016/j.biopsych.2007.02.019
Lanzenberger, R., Kranz, G. S., Haeusler, D., Akimova, E., Savli, M., Hahn, A., et al. (2012). Prediction of SSRI treatment response in major depression based on serotonin transporter interplay between median raphe nucleus and projection areas. Neuroimage 63, 874–881. doi: 10.1016/j.neuroimage.2012.07.023
Lehmann, H. E. (1974). Therapy-resistant depressions - a clinical classification. Pharmakopsychiatr. Neuropsychopharmakol. 7, 156–163. doi: 10.1055/s-0028-1094425
Lehmann, M. L., Brachman, R. A., Martinowich, K., Schloesser, R. J., and Herkenham, M. (2013). Glucocorticoids orchestrate divergent effects on mood through adult neurogenesis. J. Neuroscience. 33, 2961–2972. doi: 10.1523/JNEUROSCI.3878-12.2013
Lekman, M., Laje, G., Charney, D., Rush, A. J., Wilson, A. F., Sorant, A. J., et al. (2008). The FKBP5-gene in depression and treatment response—an association study in the Sequenced Treatment Alternatives to Relieve Depression (STAR*D) cohort. Biol. Psychiatry 63, 1103–1110. doi: 10.1016/j.biopsych.2007.10.026
Leuchter, A. F., Cook, I. A., Gilmer, W. S., Marangell, L. B., Burgoyne, K. S., Howland, R. H., et al. (2009). Effectiveness of a quantitative electroencephalographic biomarker for predicting differential response or remission with escitalopram and bupropion in major depressive disorder. Psychiatry Res. 169, 132–138. doi: 10.1016/j.psychres.2009.04.004
Leuchter, A. F., Cook, I. A., Hamilton, S. P., Narr, K. L., Toga, A., Hunter, A. M., et al. (2010). Biomarkers to predict antidepressant response. Curr. Psychiatry Rep. 12, 553–562. doi: 10.1007/s11920-010-0160-4
Li, N., Lee, B., Liu, R. J., Banasr, M., Dwyer, J. M., Iwata, M., et al. (2010). mTOR-dependent synapse formation underlies the rapid antidepressant effects of NMDA antagonists. Science 329, 959–964. doi: 10.1126/science.1190287
Lifschytz, T., Segman, R., Shalom, G., Lerer, B., Gur, E., Golzer, T., et al. (2006). Basic mechanisms of augmentation of antidepressant effects with thyroid hormone. Curr. Drug Targets 7, 203–210. doi: 10.2174/138945006775515482
Lifschytz, T., Zozulinsky, P., Eitan, R., Landshut, G., Ohayon, S., and Lerer, B. (2011). Effect of triiodothyronine on antidepressant screening tests in mice and on presynaptic 5-HT1A receptors: mediation by thyroid hormone α receptors. J. Pharmacol. Exp. Ther. 337, 494–502. doi: 10.1124/jpet.111.179564
Lin, K. M., Chiu, Y. F., Tsai, I. J., Chen, C. H., Shen, W. W., Liu, S. C., et al. (2011). ABCB1 gene polymorphisms are associated with the severity of major depressive disorder and its response to escitalopram treatment. Pharmacogenet. Genomics. 21, 163–170. doi: 10.1097/FPC.0b013e32833db216
Liu, G. X., Cai, G. Q., Cai, Y. Q., Sheng, Z. J., Jiang, J., Mei, Z., et al. (2007a). Reduced anxiety and depression-like behaviors in mice lacking GABA transporter subtype 1. Neuropsychopharmacology 32, 1531–1539. doi: 10.1038/sj.npp.1301281
Liu, Z., Zhu, F., Wang, G., Xiao, Z., Tang, J., Liu, W., et al. (2007b). Association study of corticotropin-releasing hormone receptor1 gene polymorphisms and antidepressant response in major depressive disorders. Neurosci. Lett. 414, 155–158. doi: 10.1016/j.neulet.2006.12.013
Lozano, A. M., Giacobbe, P., Hamani, C., Rizvi, S. J., Kennedy, S. H., Kolivakis, T. T., et al. (2012). A multicenter pilot study of subcallosal cingulate area deep brain stimulation for treatment- resistant depression. J. Neurosurg. 116, 315–322. doi: 10.3171/2011.10.JNS102122
Lozano, A. M., Mayberg, H. S., Giacobbe, P., Hamani, C., Craddock, R. C., and Kennedy, S. H. (2008). Subcallosal cingulate gyrus deep brain stimulation for treatment-resistant depression. Biol. Psychiatry 64, 461–467. doi: 10.1016/j.biopsych.2008.05.034
Lucas, G., Rymar, V. V., Du, J., Mnie-Filali, O., Bisgaard, C., Manta, S., et al. (2007). Serotonin(4) (5-HT(4)) receptor agonists are putative antidepressants with a rapid onset of action. Neuron 55, 712–725. doi: 10.1016/j.neuron.2007.07.041
MacQueen, G. M., Yucel, K., Taylor, V. H., Macdonald, K., and Joffe, R. (2008). Posterior hippocampal volumes are associated with remission rates in patients with major depressive disorder. Biol. Psychiatry 64, 880–883. doi: 10.1016/j.biopsych.2008.06.027
Madsen, T. M., Treschow, A., Bengzon, J., Bolwig, T. G., Lindvall, O., and Tingström, A. (2000). Increased neurogenesis in a model of electroconvulsive therapy. Biol. Psychiatry 47, 1043–1049. doi: 10.1016/S0006-3223(00)00228-6
Malberg, J. E., Eisch, A. J., Nestler, E. J., and Duman, R. S. (2000). Chronic antidepressant treatment increases neurogenesis in adult rat hippocampus. J. Neurosci. 20, 9104–9110.
Malone, D. A. Jr., Dougherty, D. D., Rezai, A. R., Carpenter, L. L., Friehs, G. M., et al. (2009). Deep brain stimulation of the ventral capsule/ventral striatum for treatment-resistant depression. Biol. Psychiatry 65, 267–275. doi: 10.1016/j.biopsych.2008.08.029
Mandelli, L., Marino, E., Pirovano, A., Calati, R., Zanardi, R., Colombo, C., et al. (2009). Interaction between SERTPR and stressful life events on response to antidepressant treatment. Eur. Neuropsychopharmacol. 19, 64–67. doi: 10.1016/j.euroneuro.2008.08.005
March, J., Silva, S., Vitiello, B., and TADS Team. (2006). The Treatment for Adolescents with Depression Study (TADS): methods and message at 12 weeks. J. Am. Acad. Child Adolesc. Psychiatry 45, 1393–1403. doi: 10.1097/01.chi.0000237709.35637.c0
Martin, P., Massol, J., Belon, J. P., Gaudel, G., and Soubrié, P. (1987). Thyroid function and reversal by antidepressant drugs of depressive-like behavior (escape deficits) in rats. Neuropsychobiology 18, 21–26. doi: 10.1159/000118388
Mateus-Pinheiro, A., Pinto, L., Bessa, J. M., Morais, M., Alves, N. D., Monteiro, S., et al. (2013). Sustained remission from depressive-like behavior depends on hippocampal neurogenesis. Transl. Psychiatry 3, e210. doi: 10.1038/tp.2012.141
Mayberg, H. S., Brannan, S. K., Mahurin, R. K., Jerabek, P. A., Brickman, J. S., Tekell, J. L., et al. (1997). Cingulate function in depression: a potential predictor of treatment response. Neuroreport 8, 1057–1061. doi: 10.1097/00001756-199703030-00048
Mayberg, H. S., Lozano, A. M., Voon, V., McNeely, H. E., Seminowicz, D., Hamani, C., et al. (2005). Deep brain stimulation for treatment-resistant depression. Neuron 45, 651–660. doi: 10.1016/j.neuron.2005.02.014
Mayer, M. L. (2007). GRIK4 and the kainate receptor. Am. J. Psychiatry 164, 1148. doi: 10.1176/appi.ajp.2007.07060996
Mayorga, A. J., Dalvi, A., Page, M. E., Zimov-Levinson, S., Hen, R., and Lucki, I. (2001). Antidepressant-like behavioral effects in 5-hydroxytryptamine(1A) and 5-hydroxytryptamine(1B) receptor mutant mice. J. Pharmacol. Exp. Ther. 298, 1101–1107.
McGrath, C. L., Kelley, M. E., Holtzheimer, P. E., Dunlop, B. W., Craighead, W. E., Franco, A. R., et al. (2013). Toward a neuroimaging treatment selection biomarker for major depressive disorder. JAMA Psychiatry 12, 1–9. doi: 10.1001/jamapsychiatry.2013.143
Menu, P., Gressier, F., Verstuyft, C., Hardy, P., Becquemont, L., and Corruble, E. (2010). Antidepressants and ABCB1 gene C3435T functional polymorphism: a naturalistic study. Neuropsychobiology 62, 193–197. doi: 10.1159/000319361
Mihaljevic Peles, A., Bozina, N., Sagud, M., Rojnic Kuzman, M., and Lovric, M. (2008). MDR1 gene polymorphism: therapeutic response to paroxetine among patients with major depression. Prog. Neuropsychopharmacol. Biol. Psychiatry 32, 1439–1444. doi: 10.1016/j.pnpbp.2008.03.018
Miller, M. D., Lenze, E. J., Dew, M. A., Whyte, E., Weber, E., Begley, A. E., et al. (2002). Effect of cerebrovascular risk factors on depression treatment outcome in later life. Am. J. Geriatr. Psychiatry 10, 592–598. doi: 10.1176/appi.ajgp.10.5.592
Mitjans, M., Gastó, C., Catalán, R., Fañanás, L., and Arias, B. (2012). Genetic variability in the endocannabinoid system and 12-week clinical response to citalopram treatment: the role of the CNR1, CNR2 and FAAH genes. J. Psychopharmacol. 26, 1391–1398. doi: 10.1177/0269881112454229
Mitjans, M., Serretti, A., Fabbri, C., Gastó, C., Catalán, R., Fañanás, L., et al. (2013). Screening genetic variability at the CNR1 gene in both major depression etiology and clinical response to citalopram treatment. Psychopharmacology (Berl.) 227, 509–519. doi: 10.1007/s00213-013-2995-y
Molendijk, M. L., Bus, B. A., Spinhoven, P., Penninx, B. W., Kenis, G., Prickaerts, J., et al. (2011). Serum levels of brain-derived neurotrophic factor in major depressive disorder: state-trait issues, clinical features and pharmacological treatment. Mol. Psychiatry 16, 1088–1095. doi: 10.1038/mp.2010.98
Monroe, S. M., Thase, M. E., and Simons, A. D. (1992). Social factors and the psychobiology of depression: relations between life stress and rapid eye movement sleep latency. J. Abnorm. Psychol. 101, 528–537. doi: 10.1037/0021-843X.101.3.528
Monteggia, L. M., Barrot, M., Powell, C. M., Berton, O., Galanis, V., Gemelli, T., et al. (2004). Essential role of brain-derived neurotrophic factor in adult hippocampal function. Proc. Natl. Acad. Sci. U.S.A. 101, 10827–10832. doi: 10.1073/pnas.0402141101
Montgomery, S. A., and Asberg, M. (1979). A new depression scale designed to be sensitive to change. Br. J. Psychiatry 134, 382–389. doi: 10.1192/bjp.134.4.382
Moon, H. Y., Kim, S. H., Yang, Y. R., Song, P., Yu, H. S., Park, H. G., et al. (2012). Macrophage migration inhibitory factor mediates the antidepressant actions of voluntary exercise. Proc. Natl. Acad. Sci. U.S.A. 109, 13094–13099. doi: 10.1073/pnas.1205535109
Mrazek, D. A., Biernacka, J. M., O'Kane, D. J., Black, J. L., Cunningham, J. M., Drews, M. S., et al. (2011). CYP2C19 variation and citalopram response. Pharmacogenet. Genomics. 21, 1–9. doi: 10.1097/FPC.0b013e328340bc5a
Mulert, C., Juckel, G., Brunnmeier, M., Karch, S., Leicht, G., Mergl, R., et al. (2007). Prediction of treatment response in major depression: integration of concepts. J. Affect. Disord. 98, 215–225. doi: 10.1016/j.jad.2006.07.021
McKinnon, M., Yucel, K., Nazarov, A., and MacQueen, G. (2009). A meta-analysis examining clinical predictors of hippocampal volume in patients with major depressive disorder. J. Psychiatry Neurosci. 34, 41–54.
Nakamura, M., Ueno, S., Sano, A., and Tanabe, H. (2000). The human serotonin transporter gene linked polymorphism (5-HTTLPR) shows ten novel allelic variants. Mol. Psychiatry 5, 32–38. doi: 10.1038/sj.mp.4000698
Nakashiba, T., Cushman, J. D., Pelkey, K. A., Renaudineau, S., Buhl, D. L., McHugh, T. J., et al. (2012). Young dentate granule cells mediate pattern separation, whereas old granule cells facilitate pattern completion. Cell 149, 188–201. doi: 10.1016/j.cell.2012.01.046
Narasimhan, S., and Lohoff, F. W. (2012). Pharmacogenetics of antidepressant drugs: current clinical practice and future directions. Pharmacogenomics 13, 441–464. doi: 10.2217/pgs.12.1
Nelson, J. C., Clary, C. M., Leon, A. C., and Schneider, L. S. (2005). Symptoms of late-life depression: frequency and change during treatment. Am. J. Geriatr. Psychiatry 13, 520–526. doi: 10.1176/appi.ajgp.13.6.520
NICE. (2004). Depression: Management of Depression in Primary and Secondary Care. London: Clinical guidelines, CG23, National Institute for Clinical Excellence.
Nierenberg, A. A., and DeCecco, L. M. (2001). Definitions of antidepressant treatment response, remission, nonresponse, partial response, and other relevant outcomes: a focus on treatment-resistant depression. J. Clin. Psychiatry 62 (Suppl. 16), 5–9.
Nierenberg, A. A., Fava, M., Trivedi, M. H., Wisniewski, S. R., Thase, M. R., McGrath, P. J., et al. (2006). A comparison of lithium and T(3) augmentation following two failed medication treatments for depression: a STAR*D report. Am. J. Psychiatry 163, 1519–1530. doi: 10.1176/appi.ajp.163.9.1519
Nollet, M., Gaillard, P., Tanti, A., Girault, V., Belzung, C., and Leman, S. (2012). Neurogenesis-independent antidepressant-like effects on behavior and stress axis response of a dual orexin receptor antagonist in a rodent model of depression. Neuropsychopharmacology 37, 2210–2221. doi: 10.1038/npp.2012.70
O'Brien, F. E., Dinan, T. G., Griffin, B. T., and Cryan, J. F. (2012). Interactions between antidepressants and P-glycoprotein at the blood-brain barrier: clinical significance of in vitro and in vivo findings. Br. J. Pharmacol. 165, 289–312. doi: 10.1111/j.1476-5381.2011.01557.x
Oh, Y. S., Gao, P., Lee, K. W., Ceglia, I., Seo, J. S., Zhang, X., et al. (2013). SMARCA3, a chromatin remodeling factor, is required for p11-dependent antidepressant action. Cell 152, 831–843. doi: 10.1016/j.cell.2013.01.014
O'Leary, O. F., Bechtholt, A. J., Crowley, J. J., Hill, T. E., Page, M. E., and Lucki, I. (2007). Depletion of serotonin and catecholamines block the acute behavioral response to different classes of antidepressant drugs in the mouse tail suspension test. Psychopharmacology (Berl.). 192, 357–371. doi: 10.1007/s00213-007-0728-9
Pae, C. U., Mandelli, L., Serretti, A., Patkar, A. A., Kim, J. J., Lee, C. U., et al. (2007a). Heat-shock protein-70 genes and response to antidepressants in major depression. Prog. Neuropsychopharmacol. Biol. Psychiatry 31, 1006–1011. doi: 10.1016/j.pnpbp.2007.02.011
Pae, C. U., Serretti, A., Mandelli, L., De Ronchi, D., Patkar, A. A., Jun, T. Y., et al. (2007b). Dysbindin associated with selective serotonin reuptake inhibitor antidepressant efficacy. Pharmacogenet. Genomics. 17, 69–75. doi: 10.1097/01.fpc.0000236330.03681.6d
Papakostas, G. I., Cooper-Kazaz, R., Appelhof, B. C., Posternak, M. A., Johnson, D. P., Klibanski, A., et al. (2009). Simultaneous initiation (coinitiation) of pharmacotherapy with triiodothyronine and a selective serotonin reuptake inhibitor for major depressive disorder: a quantitative synthesis of double-blind studies. Int. Clin. Psychopharmacol. 24, 19–25. doi: 10.1097/YIC.0b013e328314dfaf
Papiol, S., Arias, B., Gastó, C., Gutiérrez, B., Catalán, R., and Fañanás, L. (2007). Genetic variability at HPA axis in major depression and clinical response to antidepressant treatment. J. Affect. Disord. 104, 83–90. doi: 10.1016/j.jad.2007.02.017
Pariante, C. M. (2003). Depression, stress and the adrenal axis. J. Neuroendocrinol. 15, 811–812. doi: 10.1046/j.1365-2826.2003.01058.x
Pariante, C. M., and Miller, A. H. (2001). Glucocorticoid receptors in major depression: relevance to pathophysiology and treatment. Biol. Psychiatry 49, 391–404. doi: 10.1016/S0006-3223(00)01088-X
Paslakis, G., Heuser, I., Schweiger, U., and Deuschle, M. (2010). A single DEX/CRH test in male drug-free depressed patients is associated with the clinical response to treatment with fluoxetine. J. Psychiatr. Res. 44, 1154–1157. doi: 10.1016/j.jpsychires.2010.04.010
Pereira, A. M., Tiemensma, J., and Romijn, J. A. (2010). Neuropsychiatric disorders in cushing's syndrome. Neuroendocrinology 92(Suppl. 1), 65–70. doi: 10.1159/000314317
Perera, T. D., Coplan, J. D., Lisanby, S. H., Lipira, C. M., Arif, M., Carpio, C., et al. (2007). Antidepressant-induced neurogenesis in the hippocampus of adult nonhuman primates. J. Neurosci. 27, 4894–4901. doi: 10.1523/JNEUROSCI.0237-07.2007
Perera, T. D., Dwork, A. J., Keegan, K. A., Thirumangalakudi, L., Lipira, C. M., Joyce, N., et al. (2011). Necessity of hippocampal neurogenesis for the therapeutic action of antidepressants in adult nonhuman primates. PLoS ONE 6:e17600. doi: 10.1371/journal.pone.0017600
Peters, E. J., Slager, S. L., Kraft, J. B., Jenkins, G. D., Reinalda, M. S., McGrath, P. J., et al. (2008). Pharmacokinetic genes do not influence response or tolerance to citalopram in the STAR*D sample. PLoS ONE 3:e1872. doi: 10.1371/journal.pone.0001872
Petrik, D., Lagace, D. C., and Eisch, A. J. (2012). The neurogenesis hypothesis of affective and anxiety disorders: are we mistaking the scaffolding for the building. Neuropharmacology 62, 21–34. doi: 10.1016/j.neuropharm.2011.09.003
Pilhatsch, M., Winter, C., Nordström, K., Vennström, B., Bauer, M., and Juckel, G. (2010). Increased depressive behaviour in mice harboring the mutant thyroid hormone receptor alpha 1. Behav. Brain Res. 214, 187–192. doi: 10.1016/j.bbr.2010.05.016
Pizzagalli, D. A. (2011). Frontocingulate dysfunction in depression: toward biomarkers of treatment response. Neuropsychopharmacology 36, 183–206. doi: 10.1038/npp.2010.166
Pizzagalli, D., Pascual-Marqui, R. D., Nitschke, J. B., Oakes, T. R., Larson, C. L., Abercrombie, H. C., et al. (2001). Anterior cingulate activity as a predictor of degree of treatment response in major depression: evidence from brain electrical tomography analysis. Am. J. Psychiatry 158, 405–415. doi: 10.1176/appi.ajp.158.3.405
Porcelli, S., Fabbri, C., Drago, A., Gibiino, S., De Ronchi, D., and Serretti, A. (2011). Genetics and antidepressants: where we are. Clin Neuropsychiatry 8, 99–150.
Price, A., Rayner, L., Okon-Rocha, E., Evans, A., Valsraj, K., Higginson, I. J., et al. (2011). Antidepressants for the treatment of depression in neurological disorders: a systematic review and meta-analysis of randomised controlled trials. J. Neurol. Neurosurg. Psychiatry 82, 914–923. doi: 10.1136/jnnp.2010.230862
Ramasubbu, R., Beaulieu, S., Taylor, V. H., Schaffer, A., and McIntyre, R. S. (2012). The CANMAT task force recommendations for the management of patients with mood disorders and comorbid medical conditions: diagnostic, assessment, and treatment principles. Ann. Clin. Psychiatry 24, 82–90.
Reif, A., Fritzen, S., Finger, M., Strobel, A., Lauer, M., Schmitt, A., et al. (2006). Neural stem cell proliferation is decreased in schizophrenia, but not in depression. Mol. Psychiatry 11, 514–522. doi: 10.1038/sj.mp.4001791
Revest, J. M., Dupret, D., Koehl, M., Funk-Reiter, C., Grosjean, N., Piazza, P. V., et al. (2009). Adult hippocampal neurogenesis is involved in anxiety-related behaviors. Mol. Psychiatry 14, 959–967. doi: 10.1038/mp.2009.15
Revesz, D., Tjernstrom, M., Ben-Menachem, E., and Thorlin, T. (2008). Effects of vagus nerve stimulation on rat hippocampal progenitor proliferation. Exp. Neurol. 214, 259–265. doi: 10.1016/j.expneurol.2008.08.012
Richardson-Jones, J. W., Craige, C. P., Guiard, B. P., Stephen, A., Metzger, K. L., Kung, H. F., et al. (2010). 5-HT1A autoreceptor levels determine vulnerability to stress and response to antidepressants. Neuron 65, 40–52. doi: 10.1016/j.neuron.2009.12.003
Richelson, E. (1998). Pharmacokinetic interactions of antidepressants. J. Clin. Psychiatry 59(Suppl. 10), 22–26. doi: 10.4065/72.9.835
Rivero-Santana, A., Perestelo-Perez, L., Pérez-Ramos, J., Serrano-Aguilar, P., and De Las Cuevas, C. (2013). Sociodemographic and clinical predictors of compliance with antidepressants for depressive disorders: systematic review of observational studies. Patient Prefer. Adherence 7, 151–169. doi: 10.2147/PPA.S39382
Roberts, R. L., Joyce, P. R., Mulder, R. T., Begg, E. J., and Kennedy, M. A. (2002). A common P-glycoprotein polymorphism is associated with nortriptyline-induced postural hypotension in patients treated for major depression. Pharmacogenomics J. 2, 191–196. doi: 10.1038/sj.tpj.6500099
Roiser, J. P., Elliott, R., and Sahakian, B. J. (2012). Cognitive mechanisms of treatment in depression. Neuropsychopharmacology 37, 117–136. doi: 10.1038/npp.2011.183
Ruhé, H. G., van Rooijen, G., Spijker, J., Peeters, F. P., and Schene, A. H. (2012). Staging methods for treatment resistant depression. A systematic review. J. Affect. Disord. 137, 35–45. doi: 10.1016/j.jad.2011.02.020
Rush, A. J., Trivedi, M. H., Wisniewski, S. R., Nierenberg, A. A., Stewart, J. W., Warden, D., et al. (2006). Acute and longer-term outcomes in depressed outpatients requiring one or several treatment steps: a STAR*D report. Am. J. Psychiatry 163, 1905–1917. doi: 10.1176/appi.ajp.163.11.1905
Saarelainen, T., Hendolin, P., Lucas, G., Koponen, E., Sairanen, M., MacDonald, E., et al. (2003). Activation of the TrkB neurotrophin receptor is induced by antidepressant drugs and is required for antidepressant-induced behavioral effects. J. Neurosci. 23, 349–357.
Sackeim, H. A., Prudic, J., Devanand, D. P., Decina, P., Kerr, B., and Malitz, S. (1990). The impact of medication resistance and continuation pharmacotherapy on relapse following response to electroconvulsive therapy in major depression. J. Clin. Psychopharmacol. 10, 96–104. doi: 10.1097/00004714-199004000-00004
Sahay, A., Scobie, K. N., Hill, A. S., O'Carroll, C. M., Kheirbek, M. A., Burghardt, N. S., et al. (2011). Increasing adult hippocampal neurogenesis is sufficient to improve pattern separation. Nature 472, 466–470. doi: 10.1038/nature09817
Salvadore, G., Cornwell, B. R., Colon-Rosario, V., Coppola, R., Grillon, C., Zarate, et al. (2009). Increased anterior cingulate cortical activity in response to fearful faces: a neurophysiological biomarker that predicts rapid antidepressant response to ketamine. Biol. Psychiatry 65, 289–295. doi: 10.1016/j.biopsych.2008.08.014
Sanacora, G., Kendell, S. F., Levin, Y., Simen, A. A., Fenton, L. R., Coric, V., et al. (2007). Preliminary evidence of riluzole efficacy in antidepressant-treated patients with residual depressive symptoms. Biol. Psychiatry 61, 822–825. doi: 10.1016/j.biopsych.2006.08.037
Sanacora, G., Zarate, C. A., Krystal, J. H., and Manji, H. K. (2008). Targeting the glutamatergic system to develop novel, improved therapeutics for mood disorders. Nat. Rev. Drug. Discov. 7, 426–437. doi: 10.1038/nrd2462
Santarelli, L., Saxe, M., Gross, C., Surget, A., Battaglia, F., Dulawa, S., et al. (2003). Requirement of hippocampal neurogenesis for the behavioral effects of antidepressants. Science 301, 805–809. doi: 10.1126/science.1083328
Sarginson, J. E., Lazzeroni, L. C., Ryan, H. S., Ershoff, B. D., Schatzberg, A. F., and Murphy, G. M. Jr. (2010). ABCB1 (MDR1) polymorphisms and antidepressant response in geriatric depression. Pharmacogenet. Genomics. 20, 467–475. doi: 10.1097/FPC.0b013e32833b593a
Sartorius, N. (1974). Description and classification of depressive disorders. Contributions for the definition of the therapy-resistance and of therapy resistant depressions. Pharmakopsychiatr. Neuropsychopharmakol. 7, 76–79. doi: 10.1055/s-0028-1094404
Saxena, S., Brody, A. L., Ho, M. L., Zohrabi, N., Maidment, K. M., and Baxter, L. R. Jr. (2003). Differential brain metabolic predictors of response to paroxetine in obsessive-compulsive disorder versus major depression. Am. J. Psychiatry 160, 522–532. doi: 10.1176/appi.ajp.160.3.522
Scherrer, J. F., Chrusciel, T., Garfield, L. D., Freedland, K. E., Carney, R. M., Hauptman, P. J., et al. (2012). Treatment-resistant and insufficiently treated depression and all-cause mortality following myocardial infarction. Br. J. Psychiatry 200, 137–142. doi: 10.1192/bjp.bp.111.096479
Schinkel, A. H., Wagenaar, E., van Deemter, L., Mol, C. A., and Borst, P. (1995). Absence of the mdr1a P-Glycoprotein in mice affects tissue distribution and pharmacokinetics of dexamethasone, digoxin, and cyclosporin A. J. Clin. Invest. 96, 1698–1705. doi: 10.1172/JCI118214
Schloesser, R. J., Lehmann, M., Martinowich, K., Manji, H. K., and Herkenham, M. (2010). Environmental enrichment requires adult neurogenesis to facilitate the recovery from psychosocial stress. Mol. Psychiatry 15, 1152–1163. doi: 10.1038/mp.2010.34
Schloesser, R. J., Manji, H. K., and Martinowich, K. (2009). Suppression of adult neurogenesis leads to an increased hypothalamo-pituitary-adrenal axis response. Neuroreport 20, 553–557. doi: 10.1097/WNR.0b013e3283293e59
Schmidt, E. F., Warner-Schmidt, J. L., Otopalik, B. G., Pickett, S. B., Greengard, P., and Heintz, N. (2012a). Identification of the cortical neurons that mediate antidepressant responses. Cell 149, 1152–1163. doi: 10.1016/j.cell.2012.03.038
Schmidt, M. V., Paez-Pereda, M., Holsboer, F., and Hausch, F. (2012b). The prospect of FKBP51 as a drug target. Chem. Med. Chem. 7, 1351–1359. doi: 10.1002/cmdc.201200137
Schmuckermair, C., Gaburro, S., Sah, A., Landgraf, R., Sartori, S. B., and Singewald, N. (2013). Behavioral and neurobiological effects of deep brain stimulation in a mouse model of high anxiety- and depression-like behavior. Neuropsychopharmacology 38, 1234–1244. doi: 10.1038/npp.2013.21
Schüle, C., Baghai, T. C., Eser, D., Häfner, S., Born, C., Herrmann, S., et al. (2009). The combined dexamethasone/CRH Test (DEX/CRH test) and prediction of acute treatment response in major depression. PLoS ONE 4:e4324. doi: 10.1371/journal.pone.0004324
Schulz, R., Drayer, R. A., and Rollman, B. L. (2002). Depression as a risk factor for non-suicide mortality in the elderly. Biol. Psychiatry 52, 205–225. doi: 10.1016/S0006-3223(02)01423-3
Serra-Blasco, M., Portella, M. J., Gómez-Ansón, B., de Diego-Adeliño, J., Vives-Gilabert, Y., Puigdemont, D., et al. (2013). Effects of illness duration and treatment resistance on grey matter abnormalities in major depression. Br. J. Psychiatry. 202, 434–440. doi: 10.1192/bjp.bp.112.116228
Serretti, A., Zanardi, R., Rossini, D., Cusin, C., Lilli, R., and Smeraldi, E. (2001). Influence of tryptophan hydroxylase and serotonin transporter genes on fluvoxamine antidepressant activity. Mol. Psychiatry 6, 586–592. doi: 10.1038/sj.mp.4000876
Sheehan, D. V., Keene, M. S., Eaddy, M., Krulewicz, S., Kraus, J. E., and Carpenter, D. J. (2008). Differences in medication adherence and healthcare resource utilization patterns: older versus newer antidepressant agents in patients with depression and/or anxiety disorders. CNS Drugs 22, 963–973. doi: 10.2165/00023210-200822110-00005
Shelton, R. C., Osuntokun, O., Heinloth, A. N., and Corya, S. A. (2010). Therapeutic options for treatment-resistant depression. CNS Drugs 24, 131–161. doi: 10.2165/11530280-000000000-00000
Shiroma, P. R., Geda, Y. E., and Mrazek, D. A. (2010). Pharmacogenomic implications of variants of monoaminergic-related genes in geriatric psychiatry. Pharmacogenomics 11, 1305–1330. doi: 10.2217/pgs.10.118
Shors, T. J., Miesegaes, G., Beylin, A., Zhao, M., Rydel, T., and Gould, E. (2001). Neurogenesis in the adult is involved in the formation of trace memories. Nature 410, 372–376. doi: 10.1038/35066584
Singh, A. B., Bousman, C. A., Ng, C. H., Byron, K., and Berk, M. (2012). ABCB1 polymorphism predicts escitalopram dose needed for remission in major depression. Transl. Psychiatry 2, e198. doi: 10.1038/tp.2012.115
Smeraldi, E., Serretti, A., Artioli, P., Lorenzi, C., and Catalano, M. (2006). Serotonin transporter gene-linked polymorphic region: possible pharmacogenetic implications of rare variants. Psychiatr. Genet. 16, 153–158. doi: 10.1097/01.ypg.0000218611.53064.a0
Snyder, J. S., Soumier, A., Brewer, M., Pickel, J., and Cameron, H. A. (2011). Adult hippocampal neurogenesis buffers stress responses and depressive behaviour. Nature 476, 458–461. doi: 10.1038/nature10287
Sonino, N., Boscaro, M., Ambroso, G., Merola, G., and Mantero, F. (1986). Prolonged treatment of Cushing's disease with metyrapone and aminoglutethimide. IRCS Med. Sci. 14, 485–486.
Sonino, N., and Fava, G. A. (2002). Residual symptoms in depression. An emerging therapeutic concept. Prog. NeuroPsychopharmacol. Biol. Psychiatry 26, 763–770. doi: 10.1016/S0278-5846(02)00200-2
Sonino, N., Fava, G. A., Belluardo, P., Girelli, M. E., and Boscaro, M. (1993). Course of depression in Cushing's syndrome: response to treatment and comparison with Graves' disease. Horm. Res. 39, 202–206. doi: 10.1159/000182736
Souery, D., Amsterdam, J., de Montigny, C., Lecrubier, Y., Montgomery, S., Lipp, O., et al. (1999). Treatment resistant depression: methodological overview and operational criteria. Eur. Neuropsychopharmacol. 9, 83–91. doi: 10.1016/S0924-977X(98)00004-2
Souery, D., Oswald, P., Massat, I., Bailer, U., Bollen, J., Demyttenaere, K., et al. (2007). Group for the study of resistant depression. clinical factors associated with treatment resistance in major depressive disorder: results from a European multicenter study. J. Clin. Psychiatry 68, 1062–1070. doi: 10.4088/JCP.v68n0713
Stawski, P., Janovjak, H., and Trauner, D. (2010). Pharmacology of ionotropic glutamate receptors: a structural perspective. Bioorg. Med. Chem. 18, 7759–7772. doi: 10.1016/j.bmc.2010.09.012
Steiner, M. A., Wanisch, K., Monory, K., Marsicano, G., Borroni, E., Bächli, H., et al. (2008). Impaired cannabinoid receptor type 1 signaling interferes with stress-coping behavior in mice. Pharmacogenomics J. 8, 196–208. doi: 10.1038/sj.tpj.6500466
Stemmelin, J., Cohen, C., Yalcin, I., Keane, P., and Griebel, G. (2010). Implication of beta3-adrenoceptors in the antidepressant-like effects of amibegron using Adrb3 knockout mice in the chronic mild stress. Behav. Brain Res. 206, 310–312. doi: 10.1016/j.bbr.2009.09.003
Surget, A., Saxe, M., Leman, S., Ibarguen-Vargas, Y., Chalon, S., Griebel, G., et al. (2008). Drug-dependent requirement of hippocampal neurogenesis in a model of depression and of antidepressant reversal. Biol. Psychiatry 64, 293–301. doi: 10.1016/j.biopsych.2008.02.022
Surget, A., Tanti, A., Leonardo, E. D., Laugeray, A., Rainer, Q., Touma, C., et al. (2011). Antidepressants recruit new neurons to improve stress response regulation. Mol. Psychiatry 16, 1177–1188. doi: 10.1038/mp.2011.48
Suzuki, Y., Sugai, T., Fukui, N., Watanabe, J., Ono, S., Inoue, Y., et al. (2011). CYP2D6 genotype and smoking influence fluvoxamine steady-state concentration in Japanese psychiatric patients: lessons for genotype-phenotype association study design in translational pharmacogenetics. J. Psychopharmacol. 25, 908–914. doi: 10.1177/0269881110370504
Svenningsson, P., Chergui, K., Rachleff, I., Flajolet, M., Zhang, X., El Yacoubi, M., et al. (2006). Alterations in 5-HT1B receptor function by p11 in depression-like states. Science 311, 77–80. doi: 10.1126/science.1117571
Swardfager, W., Herrmann, N., McIntyre, R. S., Mazereeuw, G., Goldberger, K., Cha, D. S., et al. (2013). Potential roles of zinc in the pathophysiology and treatment of major depressive disorder. Neurosci. Biobehav. Rev. 37, 911–929. doi: 10.1016/j.neubiorev.2013.03.018
Tadić, A., Müller, M. J., Rujescu, D., Kohnen, R., Stassen, H. H., Dahmen, N., et al. (2007). The MAOA T941G polymorphism and short-term treatment response to mirtazapine and paroxetine in major depression. Am. J. Med. Genet. B. Neuropsychiatr. Genet. 144B, 325–331. doi: 10.1002/ajmg.b.30462
Tanti, A., and Belzung, C. (2013a). Hippocampal neurogenesis: a biomarker for depression or antidepressant effects. Methodological considerations and perspectives for future research. Cell Tissue Res. 354, 203–219. doi: 10.1007/s00441-013-1612-z
Tanti, A., and Belzung, C. (2013b). Neurogenesis along the septo-temporal axis of the hippocampus: are depression and the action of antidepressants region-specific. Neuroscience. 252, 234–252. doi: 10.1016/j.neuroscience.2013.08.017
Tao, R., Emslie, G., Mayes, T., Nakonezny, P., Kennard, B., and Hughes, C. (2009). Early prediction of acute antidepressant treatment response and remission in pediatric major depressive disorder. J. Am. Acad. Child Adolesc. Psychiatry 48, 71–78. doi: 10.1097/CHI.0b013e318190043e
Tatarczyñska, E., Kłodziñska, A., Stachowicz, K., and Chojnacka-Wójcik, E. (2004). Effect of combined administration of 5-HT1A or 5-HT1B/1D receptor antagonists and antidepressants in the forced swimming test. Eur. J. Pharmacol. 487, 133–142. doi: 10.1016/j.ejphar.2004.01.008
Taylor, B. P., Bruder, G. E., Stewart, J. W., McGrath, P. J., Halperin, J., Ehrlichman, H., et al. (2006). Psychomotor slowing as a predictor of fluoxetine nonresponse in depressed outpatients. Am. J. Pyschiatry 163, 73–78. doi: 10.1176/appi.ajp.163.1.73
Tedeschini, E., Levkovitz, Y., Iovieno, N., Ameral, V. E., Nelson, J. C., and Papakostas, G. I. (2011). Efficacy of antidepressants for late-life depression: a meta-analysis and meta-regression of placebo-controlled randomized trials. J. Clin. Psychiatry 72, 1660–1668. doi: 10.4088/JCP.10r06531
Thase, M. E., and Rush, A. J. (1997). When at first you don't succeed: sequential strategies for antidepressant nonresponders. J. Clin. Psychiatry 58(Suppl. 13), 23–29.
Tondo, L., Baldessarini, R. J., Vázquez, G., Lepri, B., and Visioli, C. (2013). Clinical responses to antidepressants among 1036 acutely depressed patients with bipolar or unipolar major affective disorders. Acta Psychiatr. Scand. 127, 355–364. doi: 10.1111/acps.12023
Trillat, A. C., Malagié, I., Bourin, M., Jacquot, C., Hen, R., and Gardier, A. M. (1998). Homozygote mice deficient in serotonin 5-HT1B receptor and antidepressant effect of selective serotonin reuptake inhibitors. C. R. Seances Soc. Biol. Fil. 192, 1139–1147.
Trivedi, M. H., Rush, A. J., Wisniewski, S. R., Nierenberg, A. A., Warden, D., Ritz, L., et al. (2006). Evaluation of outcomes with citalopram for depression using measurement-based care in STAR*D: implications for clinical practice. Am. J. Psychiatry 163, 28–40. doi: 10.1176/appi.ajp.163.1.28
Tronel, S., Belnoue, L., Grosjean, N., Revest, J. M., Piazza, P. V., Koehl, M., and Abrous, D. N. (2012). Adult-born neurons are necessary for extended contextual discrimination. Hippocampus 22, 292–298. doi: 10.1002/hipo.20895
Trouche, S., Bontempi, B., Roullet, P., and Rampon, C. (2009). Recruitment of adult-generated neurons into functional hippocampal networks contributes to updating and strengthening of spatial memory. Proc. Natl. Acad. Sci. U.S.A. 106, 5919–5924. doi: 10.1073/pnas.0811054106
Tsai, S. J., Gau, Y. T., Hong, C. J., Liou, Y. J., Yu, Y. W., and Chen, T. J. (2009). Sexually dimorphic effect of catechol-O-methyltransferase val158met polymorphism on clinical response to fluoxetine in major depressive patients. J. Affect. Disord. 113, 183–187. doi: 10.1016/j.jad.2008.04.017
Tsai, S. J., Hong, C. J., Chen, T. J., and Yu, Y. W. (2007). Lack of supporting evidence for a genetic association of the FKBP5 polymorphism and response to antidepressant treatment. Am. J. Med. Genet. B. Neuropsychiatr. Genet. 144B, 1097–1098. doi: 10.1002/ajmg.b.30246
Ueda, K., Okamura, N., Hirai, M., Tanigawara, Y., Saeki, T., Kioka, N., et al. (1992). Human P-glycoprotein transports cortisol, aldosterone, and dexamethasone, but not progesterone. J. Biol. Chem. 267, 24248–24252.
Ueda, N., Yoshimura, R., Umene-Nakano, W., Ikenouchi-Sugita, A., Hori, H., Hayashi, K., et al. (2009). Grapefruit juice alters plasma sertraline levels after single ingestion of sertraline in healthy volunteers. World J. Biol. Psychiatry 10(4 Pt 3), 832–835. doi: 10.1080/15622970802688069
Uhr, M., and Grauer, M. T. (2003). abcb1ab P-glycoprotein is involved in the uptake of citalopram and trimipramine into the brain of mice. J. Psychiatr. Res. 37, 179–185. doi: 10.1016/S0022-3956(03)00022-0
Uhr, M., Grauer, M. T., and Holsboer, F. (2003). Differential enhancement of antidepressant penetration into the brain in mice with abcb1ab (mdr1ab) P-glycoprotein gene disruption. Biol. Psychiatry 54, 840–846. doi: 10.1016/S0006-3223(03)00074-X
Uhr, M., Holsboer, F., and Müller, M. B. (2002). Penetration of endogenous steroid hormones corticosterone, cortisol, aldosterone and progesterone into the brain is enhanced in mice deficient for both mdr1a and mdr1b P-glycoproteins. J. Neuroendocrinol. 14, 753–759. doi: 10.1046/j.1365-2826.2002.00836.x
Uhr, M., Steckler, T., Yassouridis, A., and Holsboer, F. (2000). Penetration of amitriptyline, but not of fluoxetine, into brain is enhanced in mice with blood-brain barrier deficiency due to mdr1a P-glycoprotein gene disruption. Neuropsychopharmacology 22, 380–387. doi: 10.1016/S0893-133X(99)00095-0
Uhr, M., Tontsch, A., Namendorf, C., Ripke, S., Lucae, S., Ising, M., et al. (2008). Polymorphisms in the drug transporter gene ABCB1 predict antidepressant treatment response in depression. Neuron 57, 203–209. doi: 10.1016/j.neuron.2007.11.017
Ulrich, G., Renfordt, E., and Frick, K. (1986). The topographical distribution of alpha-activity in the resting EEG of endogenous-depressive inpatients with and without clinical-response to pharmacotherapy. Pharmacopsychiatry 19, 272–273. doi: 10.1055/s-2007-1017230
van Rossum, E. F., Binder, E. B., Majer, M., Koper, J. W., Ising, M., Modell, S., et al. (2006). Polymorphisms of the glucocorticoid receptor gene and major depression. Biol. Psychiatry 59, 681–688. doi: 10.1016/j.biopsych.2006.02.007
Vázquez, G. H., Tondo, L., Undurraga, J., and Baldessarini, R. J. (2013). Overview of antidepressant treatment of bipolar depression. Int. J. Neuropsychopharmacol. 22, 1–13. doi: 10.1017/S1461145713000023
Vergouwen, A. C., Bakker, A., Katon, W. J., Verheij, T. J., and Koerselman, F. (2003). Improving adherence to antidepressants: a systematic review of interventions. J. Clin. Psychiatry 64, 1415–1420. doi: 10.4088/JCP.v64n1203
Vermeiden, M., van den Broek, W. W., Mulder, P. G., and Birkenhäger, T. K. (2010). Influence of gender and menopausal status on antidepressant treatment response in depressed inpatients. J. Psychopharmacol. 24, 497–502. doi: 10.1177/0269881109105137
Vialou, V., Robison, A. J., Laplant, Q. C., Covington, H. E. 3rd, Dietz, D. M., et al. (2010). DeltaFosB in brain reward circuits mediates resilience to stress and antidepressant responses. Nat. Neurosci. 13, 745–752. doi: 10.1038/nn.2551
Wang, J. S., Zhu, H. J., Gibson, B. B., Markowitz, J. S., Donovan, J. L., and DeVane, C. L. (2008a). Sertraline and its metabolite desmethylsertraline, but not bupropion or its three major metabolites, have high affinity for P-glycoprotein. Biol. Pharm. Bull. 31, 231–234. doi: 10.1248/bpb.31.231
Wang, J. W., David, D. J., Monckton, J. E., Battaglia, F., and Hen, R. (2008b). Chronic fluoxetine stimulates maturation and synaptic plasticity of adult-born hippocampal granule cells. J. Neuroscience 28, 1374–1384. doi: 10.1523/JNEUROSCI.3632-07.2008
Warner-Schmidt, J. L., Chen, E. Y., Zhang, X., Marshall, J. J., Morozov, A., Svenningsson, P., et al. (2010). A role for p11 in the antidepressant action of brainderived neurotrophic factor. Biol. Psychiatry 68, 528–535. doi: 10.1016/j.biopsych.2010.04.029
Warner-Schmidt, J. L., Flajolet, M., Maller, A., Chen, E. Y., Qi, H., Svenningsson, P., et al. (2009). Role of p11 in cellular and behavioral effects of 5-HT4 receptor stimulation. J. Neurosci. 29, 1937–1946. doi: 10.1523/JNEUROSCI.5343-08.2009
Warner-Schmidt, J. L., Vanover, K. E., Chen, E. Y., Marshall, J. J., and Greengard, P. (2011). Antidepressant effects of selective serotonin reuptake inhibitors (SSRIs) are attenuated by antiinflammatory drugs in mice and humans. Proc. Natl. Acad. Sci. U.S.A. 108, 9262–9267. doi: 10.1073/pnas.1104836108
Weiss, J., Dormann, S. M., Martin-Facklam, M., Kerpen, C. J., Ketabi-Kiyanvash, N., and Haefeli, W. E. (2003). Inhibition of P-glycoprotein by newer antidepressants. J. Pharmacol. Exp. Ther. 305, 197–204. doi: 10.1124/jpet.102.046532
Williams, G. R. (2008). Neurodevelopmental and neurophysiological actions of thyroid hormone. J. Neuroendocrinol. 20, 784–794. doi: 10.1111/j.1365-2826.2008.01733.x
Wilson, I. C., Prange, A. J. Jr., and Lara, P. P. (1974). “Triiodothyronine alone and with imipramine in the treatment of depressed women,” in The Thyroid Axis, Drugs and Behavior, ed A. J. Prange (New York, NY: Raven Press), 49–61.
Winner, J., Allen, J. D., Altar, C. A., and Spahic-Mihajlovic, A. (2013). Psychiatric pharmacogenomics predicts health resource utilization of outpatients with anxiety and depression. Transl. Psychiatry 3, e242. doi: 10.1038/tp.2013.2
Wu, J., Buchsbaum, M. S., Gillin, J. C., Tang, C., Cadwell, S., Wiegand, M., et al. (1999). Prediction of antidepressant effects of sleep deprivation by metabolic rates in the ventral anterior cingulate and medial prefrontal cortex. Am. J. Psychiatry 156, 1149–1158.
Xu, Z., Zhang, Z., Shi, Y., Pu, M., Yuan, Y., Zhang, X., et al. (2011). Influence and interaction of genetic polymorphisms in catecholamine neurotransmitter systems and early life stress on antidepressant drug response. J. Affect. Disord. 133, 165–173. doi: 10.1016/j.jad.2011.04.011
Yalcin, I., Aksu, F., and Belzung, C. (2005). Effects of desipramine and tramadol in a chronic mild stress model in mice are altered by yohimbine but not by pindolol. Eur. J. Pharmacol. 514, 165–174. doi: 10.1016/j.ejphar.2005.03.029
Yin, O. Q., Wing, Y. K., Cheung, Y., Wang, Z. J., Lam, S. L., Chiu, H. F., et al. (2006). Phenotype-genotype relationship and clinical effects of citalopram in Chinese patients. J. Clin. Psychopharmacol. 26, 367–372. doi: 10.1097/01.jcp.0000227355.54074.14
Young, E. A., Haskett, R. F., Murphy-Weinberg, V., Watson, S. J., and Akil, H. (1991). Loss of glucocorticoid fast feedback in depression. Arch. Gen. Psychiatry 48, 693–699. doi: 10.1001/archpsyc.1991.01810320017003
Yu, H., Wang, D. D., Wang, Y., Liu, T., Lee, F. S., and Chen, Z. Y. (2012). Variant brain-derived neurotrophic factor Val66Met polymorphism alters vulnerability to stress and response to antidepressants. J. Neurosci. 32, 4092–4101. doi: 10.1523/JNEUROSCI.5048-11.2012
Yu, Y. W., Tsai, S. J., Hong, C. J., Chen, T. J., Chen, M. C., and Yang, C. W. (2005). Association study of a monoamine oxidase a gene promoter polymorphism with major depressive disorder and antidepressant response. Neuropsychopharmacology 30, 1719–1723. doi: 10.1038/sj.npp.1300785
Zhang, H. T., Whisler, L. R., Huang, Y., Xiang, Y., and O'Donnell, J. M. (2009). Postsynaptic alpha-2 adrenergic receptors are critical for the antidepressant-like effects of desipramine on behavior. Neuropsychopharmacology 34, 1067–1077. doi: 10.1038/npp.2008.184
Zhang, L., Li, H., Su, T. P., Barker, J. L., Maric, D., Fullerton, C. S., et al. (2008). p11 is up-regulated in the forebrain of stressed rats by glucocorticoid acting via two specific glucocorticoid response elements in the p11 promoter. Neuroscience 153, 1126–1134. doi: 10.1016/j.neuroscience.2008.03.022
Keywords: major depression, resistance, antidepressants, treatment-resistant depression, monoamine
Citation: El-Hage W, Leman S, Camus V and Belzung C (2013) Mechanisms of antidepressant resistance. Front. Pharmacol. 4:146. doi: 10.3389/fphar.2013.00146
Received: 11 June 2013; Accepted: 05 November 2013;
Published online: 22 November 2013.
Edited by:
Thibault Renoir, Florey Institute of Neuroscience and Mental Health, AustraliaReviewed by:
Jean-Philippe Guilloux, Université Paris Sud, FranceAndre Carvalho, Federal University of Ceara, Brazil
Copyright © 2013 El-Hage, Leman, Camus and Belzung. This is an open-access article distributed under the terms of the Creative Commons Attribution License (CC BY). The use, distribution or reproduction in other forums is permitted, provided the original author(s) or licensor are credited and that the original publication in this journal is cited, in accordance with accepted academic practice. No use, distribution or reproduction is permitted which does not comply with these terms.
*Correspondence: Catherine Belzung, INSERM 930, Faculté de Sciences et Techniques, Université François Rabelais, Parc Grandmont, F-37200 Tours, France e-mail:Y2F0aGVyaW5lLmJlbHp1bmdAdW5pdi10b3Vycy5mcg==