- 1Collège de France, Center for Interdisciplinary Research in Biology/Centre National de la Recherche Scientifique, Unité Mixte de Recherche 7241/Institut National de la Santé et de la Recherche Médicale U1050, Paris, France
- 2University Pierre et Marie Curie, Paris, France
- 3MEMOLIFE Laboratory of Excellence and Paris Science Lettre Research University, Paris, France
- 4Physiology Group, Department of Basic Medical Sciences, Faculty of Medicine and Health Sciences, Ghent University, Ghent, Belgium
- 5Department of Cellular and Physiological Sciences, Life Sciences Institute, Faculty of Medicine, University of British Columbia, Vancouver, BC, Canada
- 6Departamento de Fisiología, Pontificia Universidad Católica de Chile, Santiago, Chile
- 7Instituto Milenio, Centro Interdisciplinario de Neurociencias de Valparaíso, Valparaíso, Chile
Functional interaction between neurons and glia is an exciting field that has expanded tremendously during the past decade. Such partnership has multiple impacts on neuronal activity and survival. Indeed, numerous findings indicate that glial cells interact tightly with neurons in physiological as well as pathological situations. One typical feature of glial cells is their high expression level of gap junction protein subunits, named connexins (Cxs), thus the membrane channels they form may contribute to neuroglial interaction that impacts neuronal activity and survival. While the participation of gap junction channels in neuroglial interactions has been regularly reviewed in the past, the other channel function of Cxs, i.e., hemichannels located at the cell surface, has only recently received attention. Gap junction channels provide the basis for a unique direct cell-to-cell communication, whereas Cx hemichannels allow the exchange of ions and signaling molecules between the cytoplasm and the extracellular medium, thus supporting autocrine and paracrine communication through a process referred to as “gliotransmission,” as well as uptake and release of metabolites. More recently, another family of proteins, termed pannexins (Panxs), has been identified. These proteins share similar membrane topology but no sequence homology with Cxs. They form multimeric membrane channels with pharmacology somewhat overlapping with that of Cx hemichannels. Such duality has led to several controversies in the literature concerning the identification of the molecular channel constituents (Cxs versus Panxs) in glia. In the present review, we update and discuss the knowledge of Cx hemichannels and Panx channels in glia, their properties and pharmacology, as well as the understanding of their contribution to neuroglial interactions in brain health and disease.
Introduction
For a long time, it has been taken as dogma that connexin (Cx) proteins can only function as gap junction channels. Indeed, before the aggregation of Cxs at the junctional plaque and subsequent formation of gap junctions, hexameric rings of Cxs, termed connexons, were initially assumed to remain closed. An obvious reason for this occlusion was that, as gap junction channels are “poorly” selective for ions and permeable to low molecular weight molecules (<1 to 1.2 KDa), if once at the membrane connexons could open, the cell would lose its integrity or at least would have to spend substantial energy to maintain this energetically unfavorable condition. Such statements began to be challenged when evidence for “functional hemichannels,” a term proposed to substitute for plasma membrane connexons, were reported in the early 1990s. In these pioneering studies, Cx hemichannels were opened either by large depolarization in Xenopus oocytes (Paul et al., 1991) or by lowering the extracellular calcium ion (Ca2+) concentration in horizontal cells (DeVries and Schwartz, 1992). Later on, the occurrence of functional hemichannels (i.e., hemichannels that can be turned into the open state) composed of Cx43 was demonstrated in primary cultures of astrocytes in the absence of external Ca2+ (Hofer and Dermietzel, 1998). This observation was followed by the demonstration that metabolic inhibition performed in the presence of normal external Ca2+ concentrations (1–2 mM) induced cell permeabilization, due to Cx43 hemichannel opening, before loss of membrane integrity (Contreras et al., 2002). More recently, hemichannel opening in astrocytes was triggered either by treatment with pro-inflammatory cytokines or selective lipopolysaccharide (LPS) stimulation of microglia co-cultured with astrocytes, again in the presence of external Ca2+ (Retamal et al., 2007a). The opening of Cx43 hemichannels in astrocytes was also demonstrated to occur in experiments designed to decipher the mechanism of intercellular Ca2+ wave propagation in astrocytes to which both gap junction channels and hemichannels contribute (Scemes and Giaume, 2006; Leybaert and Sanderson, 2012). In this case, the release of ATP through Cx43 hemichannels turned out to play a key role in Ca2+ wave propagation mediated by an extracellular paracrine signaling component (Cotrina et al., 1998; Arcuino et al., 2002). All these initial experiments on Cx hemichannels utilized astrocytes as a cell model giving to these cells new roles in neuroglial interaction (Bennett et al., 2003).
At the turn of the century another membrane channel family, the pannexins (Panxs), was identified (Panchin et al., 2000) and demonstrated to be orthologs of innexins, the gap junction proteins expressed in invertebrates (Baranova et al., 2004). So far, there are no reports of gap junctional communication supported by native Panxs, although over expression of Panx1 enhances gap junctional coupling in glioma cells (Lai et al., 2007). They form membrane channels contributing to membrane permeabilization, similar to Cx hemichannels, and they are expressed in glial cells (Orellana et al., 2009; Scemes, 2012). The aim of the present review is to summarize what is actually known about Cx hemichannels and Panx channels in glia (mainly astrocytes and microglia) and to discuss their contribution to neuroglial interactions taken in the normal and pathological context. An overview of methods and approaches to investigate Cx hemichannels and Panx channels has recently been published (Giaume et al., 2012); we refer the reader to this paper for a more detailed discussion of methodological aspects.
Connexin and Pannexin Expression in Glial Cells
Gap junctions provide a unique direct conduit between cells, influencing crucial cellular processes through activities including electrical conductance and metabolic cooperation, mediated by the passage of ions, amino acids, glucose, glutathione, ATP, and small signaling molecules (reviewed in Sohl and Willecke, 2004), as well as microRNAs (Katakowski et al., 2010). When one considers Cx expression in the brain (Theis et al., 2005), it is evident that they are most abundant in glia, namely the astrocytes, oligodendrocytes, and microglia (Rouach et al., 2002). With the genomic characterization of Cx (Willecke et al., 2002) and Panx (Baranova et al., 2004) gene families, it has been determined that there are at least 10 Cxs and 2 Panxs expressed in the brain (see below). There is also a temporal (i.e., developmental) and spatial (i.e., cell type and brain region) pattern regarding this expression and localization. Furthermore, these channel proteins can have different roles in different cell types, either as the canonical gap junction intercellular channel or as a hemichannel (Goodenough and Paul, 2003; Figure 1). Cx hemichannels and Panx1 channels mediate the release of ATP that activates P2 receptors, promoting rises in intracellular Ca2+ concentrations; gap junction channels mediate the intercellular transfer of second messenger propagated calcium signal (Suadicani et al., 2004; Anselmi et al., 2008; Leybaert and Sanderson, 2012). Under pathological conditions, Panx1 channels have been proposed to mediate activation of the inflammasome both in neurons and astrocytes (Iglesias et al., 2009; Silverman et al., 2009), whereas Cx43 hemichannels are involved in acceleration of astroglia and neuronal cell death triggered by hypoxia-reoxygenation in high glucose (Orellana et al., 2010) and β-amyloid peptide, respectively (Orellana et al., 2011b,c). A further level of complexity can be envisioned in the context of Cxs, where the heteromeric assembly of different Cx proteins into a single channel can result in distinct differences in biophysical properties (Bevans et al., 1998; Harris, 2007). This feature is also true for Panxs since Panx1 can form both homomeric and heteromeric channels with Panx2 (Bruzzone et al., 2005). Thus for specific cellular functions, the complement of Cxs and Panxs expressed is important in the physiological or pathological context being considered. While other “non-channel” functions are emerging for Cxs (Vinken et al., 2012) and Panxs (MacVicar and Thompson, 2010), this review will be limited to channel-related functions and properties.
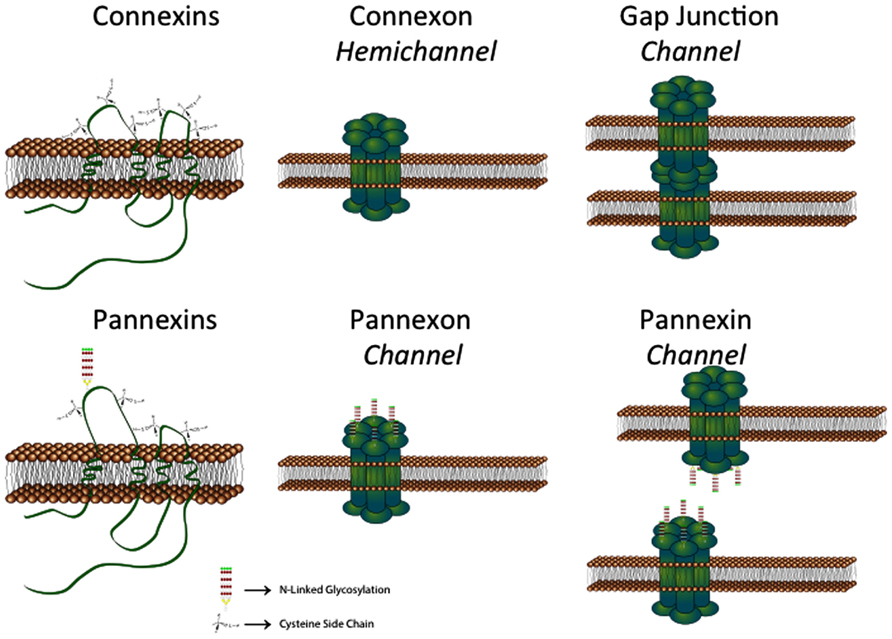
FIGURE 1. Schematic showing that connexins can from both hemichannels and gap junction channels. In contrast, pannexins normally form channels equivalent to hemichannels. See text for details (provided by Stephen Bond, Ph.D., UBC).
While this review will focus on gap junction channels and hemichannels in brain glial cells, it is important to note that gap junction proteins have also been identified in brain neurons (Andrew et al., 1981; Söhl et al., 2005). These include Cx26, Cx30.2, Cx36, Cx45, and Cx57 (Rouach et al., 2002). Neurons also express Panx1 in vivo (Bruzzone et al., 2003), and recently it has been reported that neural progenitors in the adult rodent hippocampus express Panx2 (Swayne et al., 2010). It is thus possible, and has been reported in some situations, that neurons form gap junctions with astrocytes since some of the same Cxs are expressed in both cell types (Fróes et al., 1999; Alvarez-Maubecin et al., 2000). Astrocytes express multiple Cxs, including Cx43, Cx26, Cx30, Cx40, Cx45, and Cx46 (Giaume et al., 2012), as well as Panx1 (Iglesias et al., 2009) and Panx2 (Zappala et al., 2007). Non-treated microglia express Cx43 (Eugenín et al., 2001; Garg et al., 2005), Cx36 (Dobrenis et al., 2005), and Cx32 (Maezawa and Jin, 2010), as well as Panx1 (Orellana et al., 2011b). Finally, oligodendrocytes express Cx32, Cx47, and Cx29 in vivo (Altevogt et al., 2002; Wasseff and Scherer, 2011). The complexity of Cxs and Panxs functioning as gap junction channels/hemichannels and channels, respectively, in these various cell types can be viewed as providing a background level of activity to support and modulate the complex neural activity mediated via gliotransmission.
The expression profiles noted have been associated with mature neurons and glial cell types. However, these various cell types arise developmentally from neuronal and glial precursors. Several studies have demonstrated changes in expression of Cxs and Panxs during brain development (reviewed in Vogt et al., 2005; Belousov and Fontes, 2013). Given that several reports have described disturbed neural development when expression of some Cxs is modified through the use of gene knockdown or knockout strategies, gap junction channels and hemichannels are likely to play significant roles in processes including cell proliferation, migration, and differentiation (Fushiki et al., 2003; Elias et al., 2007; Wiencken-Barger et al., 2007; Cina et al., 2009; Liu et al., 2012).
Initial studies on Cx functions in neural cells in culture and in brain slices focused on their expected role in forming gap junction channels, confirming their function via electrophysiological analysis or methods using low molecular weight dye tracers, which can pass from cell to cell through these intercellular channels (Giaume et al., 1991). Therefore, many of the phenotypic changes observed when enhancing or decreasing Cx expression were attributed to changes in gap junctional communication. With the recognition that Cxs can also form hemichannels, the consequences of Cx expression began to be considered in a new way (Contreras et al., 2004), and appropriate methods developed to assess their presence and activity (Giaume and Theis, 2010). Cx hemichannels and Panx channels are more typical of most membrane channels, like ion channels, in their direct communication between intracellular and extracellular space. However, they are unique in their pore size and conductance properties (Thompson and Macvicar, 2008).
The formation of gap junction channels and hemichannels by Cxs can help explain some contradictory effects of altering Cx expression. For example, enhanced Cx43 expression in brain glial cells, in culture and in vivo, has been shown to be both neuroprotective and neurodestructive in ischemic and excitotoxic situations (reviewed in Orellana et al., 2009; Kozoric and Naus, 2013). Recent studies have indicated that gap junction channels formed by Cx43 can be neuroprotective, while Cx43 hemichannels may be more detrimental to neuronal survival. The following sections of this review provide further insight into the distinct properties of hemichannels and how these impact the progression of neurological disorders.
Cx Hemichannel and Panx Channel Properties in GLIA
Biophysics
Today, most Cxs expressed in rodent glial cells, including Cx26, Cx30, Cx32, Cx43, and Cx45, have been shown to form functional hemichannels in endogenous and/or exogenous expression systems (Bennett et al., 2003). Nevertheless, it remains unknown if this is also the case for Cx29 and Cx47 found in oligodendrocytes. In exogenous expression systems, Cx30, Cx32, Cx43, and Cx45 hemichannels show a very low open probability at resting membrane potential and normal concentration of the extracellular divalent cations, Ca2+ and Mg2+. For Cx26 hemichannels, these properties are species-specific; human and sheep but not rat Cx26 forms hemichannels that at resting membrane potential and normal concentration of extracellular divalent cations show opening activity but the rat protein is characterized by a single evolutionary amino acid change (D159N) that limits this feature (González et al., 2006).
Opening of Cx hemichannels studied so far can be promoted by positive transmembrane voltages and reduced concentrations of extracellular divalent cations (Bennett et al., 2003). These conditions have been most frequently used to gain knowledge on biophysical features of hemichannels, including permeability properties (see below), identification of voltage sensitivity, unitary conductance, kinetic properties, and polarity of closure. However, other gating mechanisms can also promote opening of hemichannels, as described below (see Regulation). In general, hemichannels show a time- and voltage-dependent macroscopic current, activating with depolarization and inactivating with hyperpolarization. However, this feature is not equal in hemichannels formed by different Cxs. For example, the voltage dependency is strong for mouse Cx30 hemichannels (Valiunas and Weingart, 2000) but detectable only at very high positive potentials for rat Cx43 hemichannels (Contreras et al., 2003). For Cx32 hemichannels, the conductance is determined by charges dispersed over the pore pathway (Oh et al., 2008). Hemichannels formed by human Cx26 or rat Cx43 show bipolar voltage behavior (Contreras et al., 2003; González et al., 2006); however, this appears to be less well established for Cx43, for which unitary current events were found to monotonically rise in the voltage range of +30 to +90 mV (Wang et al., 2012). Hemichannels present two distinct voltage-gating mechanisms, which are called loop- (slow) and V(j)- (fast) gating, respectively (Contreras et al., 2003; Oh et al., 2008). For Cx32 hemichannels the loop-gate is mediated by a conformational change that reduces the pore diameter in a region located close to the first transmembrane domain and first extracellular loop (Bargiello et al., 2012). It was recently also proposed that the intracellular pore entrance narrows from 15 to 10 Å with loop-gated but not with V(j)-gated channel closure. Moreover, it was shown that the extracellular entrance does not undergo evident large conformational changes with either voltage-gating mechanisms (Kwon et al., 2013). The unitary conductance is characteristic for hemichannels with specific Cx composition; the fully open state for Cx26 hemichannels is characterized by ~320 pS unitary conductance (González et al., 2006), for Cx32 hemichannels this is ~90 pS, for Cx43 hemichannels ~220 pS (Contreras et al., 2003; Retamal et al., 2007a; Wang et al., 2012) and for Cx45 hemichannels ~57 pS (Valiunas, 2002). They also present conductance substates as a result of an inactivation mechanism that hinders complete activation of hemichannels under prolonged depolarization (Valiunas, 2002; Contreras et al., 2003; Gómez-Hernández et al., 2003). The most general features mentioned above have been useful to demonstrate and understand the involvement of hemichannels in different physiologic functions and also to identify hemichannels with specific Cx composition in glial cells (Orellana et al., 2011c). However, much more remains to be investigated since Cxs are post-translationally modified and thus, their biophysical features might be affected according to the metabolic state of the cell.
The biophysical properties of Panx channels are much less documented than those of Cx hemichannels, possibly as a result of their more recent discovery. Panx1 channels have been so far demonstrated to be present in microglia, astrocytes, and oligodendrocytes (Iglesias et al., 2009; Domercq et al., 2010; Orellana et al., 2011a), whereas Panx2 is expressed in post-ischemic astrocytes (Zappala et al., 2007). The open state of Panx1 channels is promoted by positive membrane voltages and elevation of intracellular free Ca2+ concentration. Voltage clamp experiments have indicated widely divergent unitary conductances for Panx1 channels, varying from 68 to 550 pS (Locovei et al., 2006; Kienitz et al., 2011; Ma et al., 2012). This controversy in the single channel conductance of Panx1 channels has not been resolved yet and might be explained in part by differences in recording conditions or solutions, cell type, phenotypic differences, and post-translational modifications. It could be partially explained by simultaneous opening of channels and thus, high conductance values might represent multiples of a single channel with a lower conductance value. An issue to keep in mind is that biophysical properties of exogenously expressed Panx channels might be different from those expressed simultaneously with all membrane and intracellular proteins (e.g., P2X receptors) that might interact with them in endogenous expression systems.
Permeability
In addition to selective ion channels or transporters, other channels can alter the permeability of the cell membrane under physiological and pathological conditions (Schalper et al., 2009). The origin of these permeability changes could be Cx- and Panx-based channels located at the cell surface. However, other membrane channels might also be involved in drastic changes in membrane permeability [e.g., P2X7 receptors, transient receptor potential (TRP) channels and calcium homeostasis modulator 1 (CALHM1) ion channel] and their possible involvement should be proved or disproved when testing the permeability of Cx hemichannels or Panx channels in a particular cell type.
Net changes in membrane permeability due to hemichannels have been most frequently studied through the use of inhibitors or molecular biology approaches that induce or abolish the expression of Cxs or Panxs. With these experimental approaches it has been proposed that hemichannels (Panx1 and/or Cx26, Cx32, and Cx43) allow the release of precursor or signaling molecules [e.g., including NAD+, ATP, adenosine, inositol trisphosphate (IP3), and glutamate; Bevans et al., 1998; Stout et al., 2002; Ye et al., 2003; Anderson et al., 2004; Bao et al., 2004a; Locovei et al., 2006; Gossman and Zhao, 2008; Kang et al., 2008; Song et al., 2011], uptake of second messengers [e.g., cyclic ADP-ribose (cADPR), nicotinic acid adenine dinucleotide phosphate (NAADP), nitric oxide (NO), and Ca2+; Heidemann et al., 2005; Sánchez et al., 2009, 2010; Schalper et al., 2010; Song et al., 2011; De Bock et al., 2012; Figueroa et al., 2013) and uptake of various metabolites (e.g., glucose, glutathione, and ascorbate; Bruzzone et al., 2001; Ahmad and Evans, 2002; Rana and Dringen, 2007; Retamal et al., 2007a; see Table 1 for Cx43 hemichannels and Panx1 channels). In addition, it has been demonstrated that Cx hemichannels are permeable to synthetic molecules [e.g., Lucifer yellow, ethidium, calcein, propidium, 5(6)-carboxyfluorescein, and 2-(4-amidinophenyl)-1H-indole-6-carboxamide (DAPI); for review, see Sáez et al., 2010]. The fact that Cx26 and Cx32 hemichannels reconstituted in lipid membranes are permeable to Ca2+ and cyclic nucleotides, respectively (Harris, 2007; Schalper et al., 2010; Fiori et al., 2012), indicates that no additional binding partners or associated molecules are required for hemichannels to function as conduits for small molecules or ions across the cell membrane. Since the membrane permeability change due to transient hemichannel opening may allow release of enough molecules to reach effective concentrations in a confined extracellular space, astroglial hemichannels have been proposed as membrane pathways for paracrine/autocrine cell signaling with physiological and pathological implications for glial and neuronal cells (Orellana et al., 2011c; Stehberg et al., 2012).
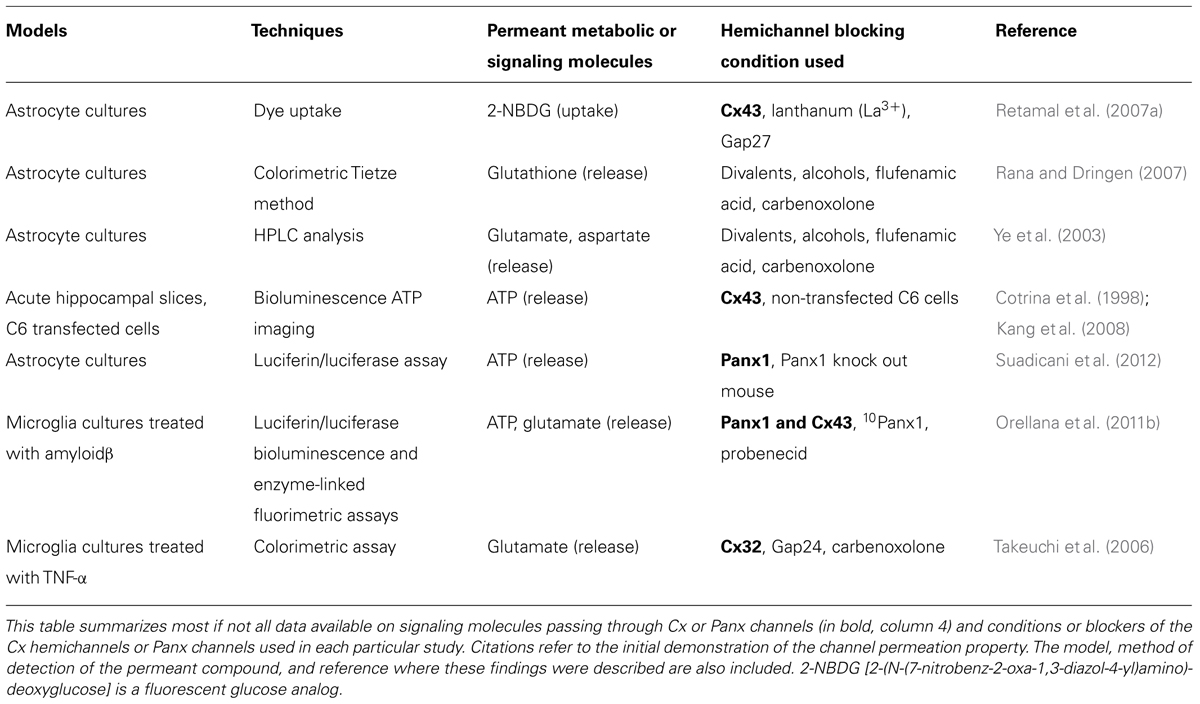
TABLE 1. Overview of substances passing through glial Cx hemichannels and Panx channels and compounds that block these membrane pathways.
Membrane permeability changes via Cx hemichannels are in part explained by variations in the number of hemichannels available at the cell surface. With regard to this, an increase in the number of surface Cx43 or Cx45 hemichannels explains the increase in membrane permeability induced by fibroblast growth factor-1 (FGF-1) in cells that express these proteins (Schalper et al., 2008b). However, in other conditions such as exposure to extracellular solution without divalent cations (Schalper et al., 2008a) or tanycytes treated with extracellular glucose (Orellana et al., 2012a), the increase in membrane permeability is explained by opening of hemichannels via a gating mechanism. In both cases there is a net increase in membrane permeability mediated by hemichannels, possibly without changes in intrinsic permeability properties of hemichannels themselves. Nonetheless, changes in membrane permeability could also result from alterations in permeability features of hemichannels. For instance, protein kinase C (PKC)-mediated phosphorylation of the six subunits of Cx43 hemichannels reconstituted in lipid membranes reduces the pore permeability allowing permeation of the small hydrophilic solute ethylene glycol (Mr 62) but not sucrose (Mr 342; Bao et al., 2007). Changes in other hemichannel permeability properties, such as affinity or charge selectivity induced by covalent modification including phosphorylation by other protein kinases or nitrosylation, cannot be ruled out yet.
The permeability properties depend on the size, shape, and net charge of each permeant ion or molecule while the concentration gradient across the cell membrane acts as the driving force. Since the study of permeability characteristics of a membrane transporter should be done with a rapid time scale (within a few seconds to a minute) it is important to have a library of permeant molecules that allow real time determination of their disappearance or appearance. Transport via Cx43 hemichannels has been shown to be cooperative and saturable with parameters (e.g., Vmax, Km, and Hill coefficient) depending on the permeant cationic species (Orellana et al., 2011a). These findings imply that opening of hemichannels does not necessarily lead to loss of important intracellular molecules because of competition effects with other, less important molecules or ions and this interpretation might be valid to transport in both directions across the cell membrane. Of note, the permeability properties of hemichannels to anionic molecules have not been reported yet. To the best of our knowledge, the permeability properties of Panx1 channels remain equally unknown. It is also not known whether channels formed by different Cxs or Panxs show different permeability properties such as size exclusion and charge selectivity. In general, quantitative kinetic properties of permeation of Cx hemichannels and Panx channels remain largely unexplored. Table 1 summarizes current evidence on the permeability of Cx43, Cx32 hemichannels, and Panx1 channels in brain glial cells.
Regulation
Hemichannels are regulated by diverse conditions of the extracellular and intracellular microenvironments. They include Ca2+ concentration outside and inside the cell, monovalent cation concentration, pH, mechanical stress, extracellular ligands, protein kinases, protein phosphatases, as well as oxidant and reducing agents. Here, the most recent studies not included in previous reviews (Sáez et al., 2010; Wang et al., 2013), will be presented.
Hemichannel Opening and Modulation by Ca2+
Unapposed Cx hemichannels of cells in culture are preferentially closed but can be opened by several trigger conditions (reviewed in Sáez et al., 2005, 2010). These include, transmembrane voltage with activation threshold of -30 mV for Cx30 and +50 mV for Cx43 in the presence of normal extracellular Ca2+ (Valiunas and Weingart, 2000; Wang et al., 2012), mechanical forces/strain (Bao et al., 2004b; Luckprom et al., 2011), a decrease of extracellular Ca2+ (Li et al., 1996; Stout et al., 2002; Ye et al., 2003; Ramachandran et al., 2007; Torres et al., 2012), an increase of intracellular free Ca2+ (De Vuyst et al., 2006, 2009; Wang et al., 2012), alterations in phosphorylation status (Bao et al., 2004c) and redox status (Retamal et al., 2007b), and ischemia-mimicking conditions (John et al., 1999; Contreras et al., 2002; Wang et al., 2013).
Role of extracellular Ca2+. A decrease of extracellular divalent cation concentration was one of the first conditions reported to stimulate Cx43 hemichannel opening, as introduced above. Work in a hepatoma cell line expressing Cx43 demonstrated that a decrease of the extracellular Ca2+ concentration triggered the uptake of Lucifer yellow, a hemichannel-permeable fluorescent dye (Li et al., 1996). Similar observations were obtained in astrocytes, where hemichannel opening was demonstrated to trigger the release of glutamate and aspartate (Ye et al., 2003). Lowering extracellular Mg2+ appeared to promote low Ca2+-triggered hemichannel opening but Ca2+ appeared to be the dominant factor. It is thought that normal physiological extracellular Ca2+ concentrations (~1.8 mM) keep non-junctional Cx hemichannels closed in the plasma membrane, until they become incorporated into gap junction channels where they open by a process called “loop gating” as a consequence of extracellular loop interactions. In general, the half-maximal effective concentration for Ca2+-related opening of Cx43 hemichannels is in the order of 100 μM extracellular Ca2+. The proposed mechanism of millimolar Ca2+ closure of Cx hemichannels involves an interaction of extracellular Ca2+ with a Ca2+ binding site that consists of a ring of aspartate residues at the extracellular vestibule of the hemichannel pore thereby closing the channel (Gómez-Hernández et al., 2003). Such a mechanism has been best documented for Cx32 but appears to be also plausible for the astroglial Cxs, Cx30 and Cx43. Recently, Torres et al. (2012) have elegantly exploited the extracellular Ca2+-sensitivity of astroglial hemichannels to trigger their opening by photo-activating a Ca2+ buffer (thereby increasing its Ca2+ affinity) added to the interstitial fluid of a hippocampal acute slice preparation (discussed further below under Section “Impact of Hemichannel-Mediated Gliotransmission on Synaptic Activity and Behavior”).
Role of intracellular Ca2+. Besides extracellular Ca2+, intracellular cytoplasmic Ca2+ also influences hemichannel function. In fact, it was found that Cx hemichannel opening triggered by a decrease of extracellular Ca2+ could be inhibited by ester-loading the cells with the Ca2+ buffer 1,2-bis(o-aminophenoxy)ethane-N,N,N′,N′-tetra-acetic acid (BAPTA), added to dampen intracellular Ca2+ changes (De Vuyst et al., 2006). Further investigations demonstrated that Cx32 hemichannels are activated by moderate (below 500 nM) changes in intracellular Ca2+ and inhibited by Ca2+ changes above 500 nM. Subsequent work showed that Cx43 hemichannels are also characterized by such a biphasic, bell-shaped, response to changes in intracellular Ca2+ concentration, in ATP release hemichannel studies as well as in dye uptake studies in glioma cells and other cell types (De Vuyst et al., 2009; De Bock et al., 2012). Recently, single channel studies of Cx43 hemichannels robustly confirmed these observations (Wang et al., 2012). Cx hemichannels are Ca2+ permeable (Sánchez et al., 2009, 2010; Schalper et al., 2010; Fiori et al., 2012) and the biphasic dependency on intracellular Ca2+ thus confers a positive feedback below 500 nM intracellular Ca2+ concentration (Ca2+-induced Ca2+ entry via Cx43 hemichannels) and negative feedback at higher concentrations. Interestingly, IP3 receptor channels present in the endoplasmic reticulum (ER) are Ca2+ release channels that have a similar bell-shaped Ca2+ dependency (Bezprozvanny et al., 1991). Positive and negative Ca2+ feedback at IP3 receptor channels forms the basis of Ca2+ oscillations, thus, it was hypothesized that Ca2+ feedback at Cx hemichannels could also play a role in Ca2+ oscillations (De Bock et al., 2012). Experimental work with various peptides targeting Cx hemichannels indeed demonstrated that inhibiting hemichannel opening, or preventing their closure in response to high intracellular Ca2+, blocked Ca2+ oscillations triggered by bradykinin. Thus, Cx hemichannels constitute a putative gliotransmitter release pathway. Also, they may contribute to Ca2+ oscillations in astrocytes and thereby potentially lead to gliotransmitter release via other Ca2+-dependent pathways (Oliet and Mothet, 2006; Zorec et al., 2012). In brain endothelial cells, it was found that Cx hemichannel blocking peptides prevented the opening of the blood–brain barrier, triggered by bradykinin, by inhibiting Ca2+ oscillations in the endothelial cells (De Bock et al., 2011). Below, we discuss in more detail some peptide-based hemichannel inhibitors.
Influence of Monovalent Cations
Elevations in extracellular K+ shift the activation potential of Panx1 hemichannels to a more physiological range. Panx1 hemichannels expressed in astrocytes might serve as K+ sensors for changes in the extracellular milieu such as those occurring under pathological conditions (Suadicani et al., 2012). This type of regulation might also occur under physiological conditions with high neuronal activity. Thus, elevations in extracellular K+ of the magnitude occurring during periods of high neuronal activity have been proposed to affect the intercellular signaling among astrocytes (Scemes and Spray, 2012). Accordingly, the gliotransmitter release in the hippocampus in response to high neuronal activity is sensitive to P2 receptor and Panx1 channel blockers (Heinrich et al., 2012). Cx30 gap junction channels have recently been demonstrated to be regulated by the concentration of external K+ (Roux et al., 2011); whether astroglial Cx hemichannels are influenced by the extracellular K+ concentration is currently not known.
Influence of pH
Under pathological conditions, the intracellular and extracellular pH may display abrupt changes. Related to this issue and in the presence of extracellular divalent cations, Cx43 hemichannels are opened by alkaline pH applied at the extracellular side and preferentially closed at physiologic pH (Schalper et al., 2010). The activity of Cx45 hemichannels recorded at positive membrane potentials and in the absence of extracellular Ca2+ is drastically reduced by acidification (Valiunas, 2002). In addition, the pH sensitivity might be potentiated by protonated aminosulfonates (Bevans and Harris, 1999), such as taurine that could be in millimolar concentration in the cytoplasm of several cell types including astrocytes (Olson, 1999). This effect is Cx specific; Cx26 but not Cx32 hemichannels are closed by aminosulfonates at constant pH (Bevans and Harris, 1999). At the ultrastructural level (high-resolution atomic force microscopy), Cx26 hemichannels are closed at pH < 6.5 [4-(2-hydroxyethyl)-1-piperazineethanesulfonic acid (HEPES) buffer] and opened reversibly by increasing the pH to 7.6 (Yu et al., 2007). Moreover, molecular studies have revealed that Cx26 hemichannel closure induced by taurine requires the interaction between the cytoplasmic loop and the C-terminal (CT; Locke et al., 2011).
Influence of Mechanical Forces
Mechanical stress is another condition that might be relevant under pathological situations. For example, after brain trauma the extracellular medium becomes hypertonic due to the release of intracellular solutes to the extracellular microenvironment (Somjen, 2002). In astrocytes, hypertonicity induces glutamate release through Cx43 hemichannels (Jiang et al., 2011). This response could be mediated by integrin α5β1 (Batra et al., 2012), a RhoA GTPase and the contractile system (Ponsaerts et al., 2010). This sensitivity is likely to result from the interaction between negatively charged amino acid residues of the CT end (Asp278 and Asp279) and a domain of the intracellular loop (D’Hondt et al., 2013). Activation of Panx1 channels by mechanical stress is not present in all cell types (Bao et al., 2004a; Reyes et al., 2009), suggesting that it should be tested in glial cells in order to validate its possible relevance in these cells.
Influence of Extracellular Factors and Signals
The functional state of glial Cx hemichannels and Panx1 channels is actively regulated by extracellular signals. In primary cultures of mouse astrocytes, stomatin inhibits Panx1-mediated whole cell currents by interacting with its CT (Zhan et al., 2012). In contrast, pro-inflammatory agents such as the β-amyloid peptide increase the surface expression and activity of Cx43 hemichannels and Panx1 channels in microglia and Cx43 hemichannels in astrocytes (Orellana et al., 2011c). Moreover, in brain astrocytes, epidermal growth factor (EGF) and FGF-2 inhibit Cx hemichannel activity via the mitogen-activated protein kinase cascade, and the effect of the growth factors is reversed by interleukin-1β (IL-1β; Morita et al., 2007). In contrast, pro-inflammatory cytokines [tumor necrosis factor alpha (TNF-α) and IL-1β] released by LPS-activated microglia increase the activity of astroglial Cx43 hemichannels via p38 kinase (Retamal et al., 2007b; Orellana et al., 2011c) and this response is inhibited by activation of CB1 receptors by synthetic cannabinoid agonists (Froger et al., 2009). The orchestrated involvement of Cx hemichannels and Panx1 channels has also been found in other experimental preparations. In spinal cord astrocytes, FGF-1 leads to vesicular ATP release followed by sequential activation of Panx1 and Cx43 hemichannels (Garré et al., 2010). Moreover, up-regulation of astroglial Panx1 channels and Cx43 hemichannels has been found in brain abscess (Karpuk et al., 2011).
Cx43 hemichannels expressed by tanycytes, glial cells present in the hypothalamus, open in seconds after exposure to physiological concentrations of extracellular glucose. This response is mediated by the sequential activation of glucosensing proteins (Orellana et al., 2012a), a response that is absent in cortical astrocytes (Orellana et al., 2010). However, high glucose levels enhance the increase in astroglial Cx43 hemichannel activity induced by hypoxia-reoxygenation (Orellana et al., 2010).
As a result of cell interaction with extracellular signals or conditions that affect the energy supply, intracellular metabolic pathways involving levels of free Ca2+ concentration, activity of protein kinases and phosphatases, as well as levels or activity of intracellular redox molecules, are affected. Therefore, drastic reduction in ATP levels are expected to favor the dephosphorylated state of Cx43 hemichannels and thus, they could present an increased activity (Bao et al., 2007). Moreover, the lack of energy leads to a rise in intracellular free Ca2+ that favors the generation of reactive oxygen and nitrogen species including NO. The latter induce rapid nitrosylation of Cx43 and opening of hemichannels (Retamal et al., 2007b; Figueroa et al., 2013). This mechanism might partially explain the increase in Cx43 hemichannel activity observed in astrocytes under ischemia-like conditions since free radical scavengers, dithiothreitol, a sulfhydryl (SH) reducing reagent, and high intracellular levels of reduced glutathione revert the hemichannel activity to levels found in normal cells (Contreras et al., 2002; Retamal et al., 2007b; Orellana et al., 2010). Finally, extracellular ATP mediates the ischemic damage to oligodendrocytes and is partially explained by the activation of Panx1 channels (Domercq et al., 2010). In contrast to Cx43 hemichannels, S-nitrosylation of Panx1 channels reduces their activity (Lohman et al., 2012), suggesting that their opening might be relevant only under conditions where NO is overcome by -SH reducing agents such as reduced glutathione.
Hemichannel Blockers – Mimetic Peptides and Others
Specifically targeting unapposed hemichannels is difficult because they are composed of the same Cx building blocks as gap junction channels. As a consequence, most gap junction blockers also inhibit hemichannels (see Giaume and Theis, 2010). Moreover, many Cx hemichannel/gap junction channel blockers also block Panx channels. Gap junction blockers include glycyrrhetinic acid and its derivative carbenoxolone, long-chain alcohols like heptanol or octanol, halogenated general volatile anesthetics like halothane, fatty acids like arachidonic acid and oleic acid, fatty acid amides like oleamide and anandamide, and fenamates like flufenamic acid, niflumic acid, or meclofenamic acid (reviewed in Bodendiek and Raman, 2010). Most of these substances have been used in the past to block hemichannels but the results obtained can only be unequivocally interpreted in terms of hemichannels when the contribution of gap junction channels is negligible or absent. Substances like the trivalent ions gadolinium (Gd3+) and lanthanum (La3+) block hemichannels without inhibiting gap junction channels or Panx1 channels, but these ions also inhibit other channels including maxi-anion channels (Liu et al., 2006) and Ca2+ channels (Mlinar and Enyeart, 1993). A possible way to differentiate between Cx hemichannels and Panx channels is the use of long-chain alcohols that block Cx hemichannels but have only a very small effect of Panx channels. In contrast, low concentration (5–10 μM) of carbenoxolone blocks Panx1 channels and only has a minor inhibitory effect on Cx hemichannels (Schalper et al., 2008a). However, all these compounds are not specific and in addition to their inhibitory effects on Cx and Panx channels (including gap junctions channels and hemichannels) they also affect other neuronal and glial membrane properties which limits their use (see Giaume and Theis, 2010).
A more specific targeting of Cx channels is to be expected, at least in principle, from mimetic peptides of Cx proteins. Peptides identical to certain sequences on the Cx protein have been extensively used to interfere with the Cx channel function (Figure 2). The first Cx mimetic peptides that were introduced in the 1990s were identical to specific domains on the extracellular loops (first or second extracellular loop) of the Cx protein. The sequences mimicked were located in domains thought to be involved in the docking of two hemichannels during formation of a full gap junction channel (Warner et al., 1995). Exogenous addition of peptides mimicking parts of these domains were hypothesized to interact with yet unknown extracellular loop domains, thereby preventing extracellular loop interactions of apposed hemichannels during docking and thus hindering gap junction channel formation. These domains, known as Gap26 and Gap27, are present on the first and second extracellular loops, respectively. Accordingly, Gap26 and Gap27 peptides were indeed found to inhibit gap junctional coupling (Evans and Boitano, 2001). Interestingly, subsequent work demonstrated that Gap26 and Gap27 peptides also inhibited unapposed hemichannels with which these peptides are supposed to interact (Braet et al., 2003; Evans et al., 2006, 2012; Desplantez et al., 2012; Wang et al., 2012). Currently, clear evidence that these peptides indeed interact with the extracellular loops is only available for Gap26 (Liu et al., 2006). Nevertheless, both Gap26 and Gap27 rapidly inhibit hemichannels within minutes, followed by a somewhat delayed inhibition of gap junction channels, often in the range of hours, with some exceptions (Matchkov et al., 2006). The exact mechanism of hemichannel block is currently not known. It is known that Gap26/27 influence voltage-dependent gating (Wang et al., 2012) but this does not explain their inhibitory action on hemichannels opened by triggers other than voltage. It has been suggested that these peptides inhibit hemichannels by just blocking the pore because of steric hindrance effects (Wang et al., 2007). However, this has been carefully checked and it was found that this only occurs at very high (1 mM and above) concentrations (Wang et al., 2012). Although Gap26/27 peptides proved to be interesting tools to inhibit Cx hemichannels, their delayed inhibition of gap junction channels remains a serious drawback. Moreover, Gap26/27 peptides also show rather limited specificity toward different Cx types. Indeed, the extracellular loop domains mimic sequences that show pronounced homology between different Cx proteins. Thus, Gap27 directed against Cx43 in astrocytes is also known to inhibit channels or hemichannels composed of Cx37, a vascular Cx present in brain blood vessels (Martin et al., 2005).
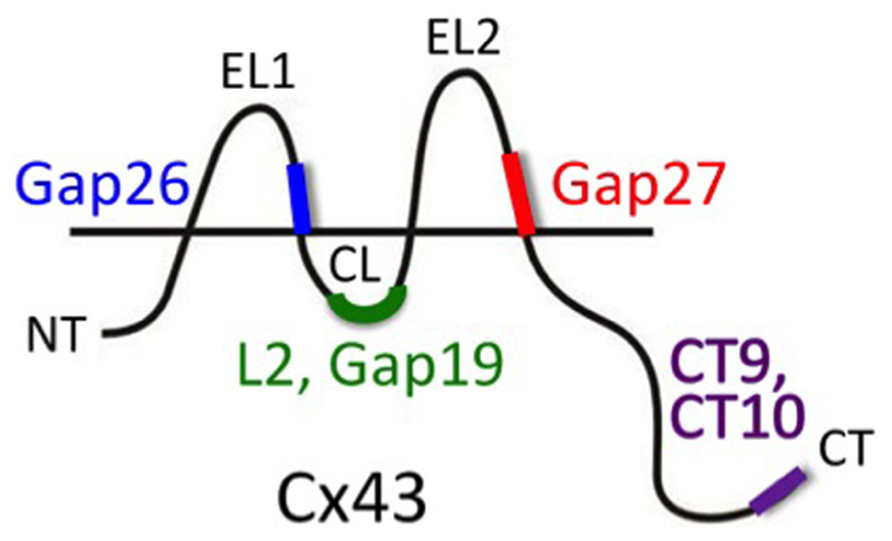
FIGURE 2. Peptide tools to interfere with Cx hemichannel function. Gap26 and Gap27 are composed of sequences located on the extracellular loops 1 (EL1) and 2 (EL2), respectively (amino acids 64–76 and 201–210, respectively) of Cx43 (mouse). These EL-mimetic peptides first inhibit hemichannels and with some delay also gap junction channels. The sequences they mimic are well conserved between different Cxs and peptides based on Cx43 sequences could also inhibit channels composed of other Cxs (e.g., Cx37). L2 peptide is identical to the L2 domain on the cytoplasmic loop (CL; amino acids 119–144). Gap19 is a nonapeptide located within the L2 domain (amino acids 128–136). L2 and Gap19 peptides block Cx43 hemichannels without blocking gap junctions and without blocking Cx40 hemichannels or Panx1 channels (other Cxs still need to be tested). CT10 and CT9 are the last 10 and last 9 amino acids of the C-terminal end (CT; amino acids 373–382 and 374–382, respectively). CT9/CT10 peptides remove the closure of Cx43 hemichannels with high micromolar cytoplasmic Ca2+ concentration and thus stimulate the opening of hemichannels. This particular effect of the CT-peptides is independent of the last amino acid (isoleucine 382) that is involved in linking Cx43 to scaffolding proteins involving ZO-1 interactions (the amino acid sequence 374–381 has the same effect as CT9/CT10; De Bock et al., 2012).
Besides peptides, antibodies have also been used to target the free extracellular loops of unapposed Cx hemichannels resulting in their inhibition. These tools offer additional advantage because they allow both functional blocking of the hemichannels and visualizing their distribution (Hofer and Dermietzel, 1998; Clair et al., 2008; Riquelme et al., 2013). However, currently single channel electrophysiological data showing hemichannel block by an antibody directed against an extracellular epitope is only demonstrated for Cx43 hemichannel activity induced in β-amyloid-treated astrocytes (Orellana et al., 2011c). Additionally, the specificity toward effects on other Cxs or Panxs still needs to be determined.
To improve specificity toward different Cxs, peptides have been devised that should target sequences on the intracellular domains of the Cx protein. The intracellular sequences are poorly conserved and very different between different Cxs. The L2 peptide is identical to a sequence on the cytoplasmic loop of Cx43. This peptide is known to prevent the closure of gap junction channels by binding to the CT tail, thereby preventing interaction of the CT tail with the cytoplasmic loop of Cx43 where the L2 domain is located (Seki et al., 2004; Hirst-Jensen et al., 2007 and references therein). Conceptually, interaction between the CT tail and the L2 domain is thought to block the gap junction channel pore upon exposure to acidification according to a particle-receptor model, and exogenous addition of L2 peptide prevents this interaction and its associated channel closure. Unexpectedly, it was found that the L2 peptide inhibited Cx43 hemichannels, based on ATP release studies, indicating that this peptide has distinct effects on unapposed hemichannels and those incorporated into gap junctions (Ponsaerts et al., 2010). Further work with Gap19, a mimetic nonapeptide located within the Cx43 L2 domain, demonstrated a similar action; it inhibited single channel hemichannel currents and ATP release via Cx43 hemichannels while it did not influence gap junctional coupling after 30 min and stimulated coupling after 24 h (Wang et al., 2013). Moreover, the effect appeared to be specific for Cx43 as Gap19 had no effect on Cx40 hemichannels or Panx1 channels. While the effects of L2 and Gap19 are understood at a molecular operational scale, the full details of why preventing interactions between the CT tail and the cytoplasmic loop have such different effects on hemichannels as compared to gap junction channels still needs further clarification. However, distinct effects on hemichannels and gap junction channels have been reported for other Cx channel influencing molecules or conditions; for example, basic FGF (bFGF) and cytokines like TNF-α or IL-1β have all been demonstrated to promote Cx43 hemichannel function while inhibiting gap junctions (De Vuyst et al., 2007; Retamal et al., 2007a). Given the specific actions of L2 and Gap19 peptides at the level of both Cx types and channel types (hemichannels versus gap junction channels), these peptides offer promising possibilities to explore the role of astroglial Cx43 hemichannels in the complex environment of intact neural tissues, ex vivo or in vivo. Recent work has, for example, demonstrated that injection of L2 peptide in the rat amygdala strongly suppresses fear memory (Stehberg et al., 2012; discussed further below under Section “Impact of Hemichannel-Mediated Gliotransmission on Synaptic Activity and Behavior”). Equally interesting with respect to identifying the role and functions of Cx43 hemichannels is the G138R mutation of Cx43 in which a Gly at position 138 is replaced by an Arg. This is one of the several mutants found in the inherited human disease oculodentodigital dysplasia (ODDD). Importantly, G138R mutant Cx43 is characterized by a loss-of-function of gap junctional coupling and a gain-of-function of hemichannels (Dobrowolski et al., 2007, 2008). Thus, this particular mutant Cx43 can be used to stimulate Cx43 hemichannel function as a complementary experimental approach to hemichannel inhibition. Recently, Torres et al. (2012) have exploited such an approach to investigate the role of hemichannels in glial–neuronal communication.
Roles of Glial Hemichannels in Health and Disease
Astroglial Ca2+ Waves
Initially, gap junction channels were demonstrated to constitute the pathway allowing the propagation of intercellular Ca2+ signaling between astrocytes, termed as Ca2+ waves (Finkbeiner, 1992; Venance et al., 1995). Then, after the discovery of an external component that also contributes to this interglial signaling process (Hassinger et al., 1996), the involvement of Cx43 hemichannels and ATP release was identified (Cotrina et al., 1998; Stout et al., 2002). There is a consensus on the interpretation of these two sets of in vitro studies and it is admitted that both Cx channel functions are contributing. Indeed, they likely participate in various proportions to Ca2+ waves depending on the brain structure, the mode of stimulation to trigger the waves, the age and the in vitro conditions (Scemes and Giaume, 2006; Leybaert and Sanderson, 2012). In addition, several of these points could also account for the alternative proposal claiming that in astrocytes the external component is not supported by Cx43 hemichannels but by Panx1 channels (Suadicani et al., 2006; Scemes et al., 2007). This point of controversy remains to be clarified in the future although it is likely that both might participate in various proportions under different conditions.
More recently, several reports have confirmed that Ca2+ waves in astrocytes are present in vivo in the normal brain (Hoogland et al., 2009; Kuga et al., 2011; Mathiesen et al., 2013) as well as in pathological situations (Kuchibhotla et al., 2009; Mathiesen et al., 2013). As expected, the occurrence, amplitude and extent of in vivo Ca2+ waves are much more limited compared to the initial in vitro observations. However, as Ca2+ signaling is considered as the mode of cellular excitability in astrocytes, such waves certainly play a role in the spatial and temporal features of neuroglial interactions. As a consequence, this means that in many cases dynamic neuroglial interactions are not limited to the sole tri-partite synapse but also occur at a more integrated level involving larger cerebral areas (Giaume et al., 2010). This situation is also likely true at the gliovascular interface where there is a high level of Cx expression between astroglial perivascular endfeet (Yamamoto et al., 1990; Simard et al., 2003; Rouach et al., 2008) and where Ca2+ waves propagate from one initial endfoot to its neighbors (Mulligan and MacVicar, 2004). Such features might optimize the contribution of astrocytes to the control of the cerebral blood flow. However, while a role of Cx43 gap junction channels/hemichannels in pial arteriole dilation has been demonstrated in vivo for the glia limitans in response to sciatic stimulation (Xu and Pelligrino, 2007), a similar mechanism remains to be investigated for perivascular astrocytes within the brain parenchyma.
Hemichannels as Ca2+ Channels
As indicated above, glial cells express several Cxs and out of these at least four of them (Cx26, Cx30, Cx32, and Cx43) have been demonstrated to form functional hemichannels (Li et al., 1996; Rhee et al., 1996; Valiunas and Weingart, 2000; Ripps et al., 2004), and at least Cx26, Cx32, and Cx43 serve as a membrane pathway for cell (Sánchez et al., 2009, 2010; Schalper et al., 2010) or liposome (Schalper et al., 2010; Fiori et al., 2012) Ca2+ inflow. Of course, brief opening of Cx hemichannels could serve to transiently increase the intracellular Ca2+ concentration that could affect a broad variety of physiological cell functions. For example, Cx43 hemichannels contribute to maintain higher intracellular levels of free Ca2+ in cells treated with FGF-1 that proliferate and remain healthy (Schalper et al., 2008b). However, sustained hemichannel opening could promote deleterious results, including cell death (Decrock et al., 2009, 2011), but the bell-shaped action of intracellular Ca2+ might prevent this ending process. Panx1 has also been detected in glial cells, as mentioned above (Iglesias et al., 2009; Domercq et al., 2010; Orellana et al., 2012b). This glycoprotein has been shown to form channels in the ER permeable to Ca2+ (Vanden Abeele et al., 2006; D’Hondt et al., 2011) but the permeability to Ca2+ of Panx1 channels present in the cell surface remains elusive. Moreover, direct demonstration of either Cx- or Panx-based channels as a Ca2+ membrane pathway in any glial cell type remains to be demonstrated.
Alternative Pathway for Glucose Entry in Inflammatory Astrocytes
Through the so-called astrocyte–neuron lactate shuttle, astrocytes participate in an activity-dependent manner to the uptake of glucose from the blood supply and the delivery of lactate to neurons (Pellerin and Magistretti, 2012). In normal conditions, glucose and lactate transporters are the membrane elements that support this astroglial contribution to brain metabolism. While glucose is well known to be essential for correct brain function, its homeostasis is altered by inflammatory conditions (Allaman et al., 2011). In vitro, an inflammatory situation for astrocytes can be mimicked either by co-culturing them with microglia selectively activated by the endotoxin LPS or by directly treating them with pro-inflammatory cytokines, such as TNF-α and IL-1β. Interestingly, in both cases these treatments induce an opposite regulation of Cx43 channels at least in cultured astrocytes: a decrease in gap junctional communication and an activation of hemichannels (Retamal et al., 2007a). In these conditions Cx43 hemichannels were shown to allow the influx of 2-(N-(7-nitrobenz-2-oxa-1,3-diazol-4-yl)amino)-2-deoxyglucose (2-NBDG), a glucose fluorescent analog, demonstrating that reactive or inflamed astrocytes can take up more glucose via Cx43 hemichannels when their metabolic coupling through gap junction channels is reduced (Rouach et al., 2008). Such opening of Cx43 hemichannels may represent an alternative metabolic pathway for glucose entry in astrocytes during pathological conditions associated with an inflammation status (Sofroniew and Vinters, 2010). Interestingly, this observation identifies a pathway that could contribute to the increase of glucose uptake found in several uncoupling situations (Tabernero et al., 2006); this was correlated with the up-regulation of GLUT-1 (glucose transporter 1) expression and to the induction of the expression of GLUT-3, an isoform that is not normally expressed in astrocytes, as well for type I and type II hexokinases, respectively (Sánchez-Alvarez et al., 2004). However, the respective contribution of hemichannels and glucose transporters in inflammatory conditions remains to be established. Because the permeability of the blood–brain barrier is increased during the inflammatory response (Schnell et al., 1999), more glucose coming from the circulation could become available to the brain. Thus, in addition to transporters, the entry of glucose through Cx43 hemichannels could contribute to an increase of lactate formation by astrocytes that are adapted for anaerobic metabolism. Finally, the opposite regulation of Cx43 channels (Retamal et al., 2007a) may lead to a failure in intercellular glucose trafficking (Rouach et al., 2008) that could be compensated for by the increase in glucose influx through Cx43 hemichannels in individual astrocytes. Moreover, although not demonstrated yet, Cx43 hemichannels could also support lactate efflux to feed neurons since Cx43 gap junction channels in astrocytes are permeable to lactate (Tabernero et al., 1996; Rouach et al., 2008). As a whole, Cx43 hemichannel activation should certainly contribute to modify the metabolic status of reactive astrocytes, a situation that should be taken into account when considering the role of astroglia in brain inflammation.
Impact of Hemichannel-Mediated Gliotransmission on Synaptic Activity and Behavior
By analogy with the concept of a neurotransmitter, the term “gliotransmitter” has been proposed to name active molecules that are released by astrocytes and regulate neuronal activity as well as synaptic strength and plasticity (Haydon and Carmignoto, 2006). There are mainly three candidates that are proposed to play this role: glutamate, ATP, and D-serine. All of them can be released by a Ca2+-dependent vesicular release mechanism in astrocytes (Zorec et al., 2012). However, at least glutamate and ATP have been demonstrated to permeate Cx43 hemichannels in astrocytes (Ye et al., 2003; Kang et al., 2008; Orellana et al., 2011b; Iglesias and Spray, 2012) as well as Panx1 channels in microglia (Orellana et al., 2011c), thus Cx43 and Panx1 channels could also play a role in gliotransmission. Recently, the activation of Cx43 hemichannels in astrocytes was shown to impact synaptic activity and plasticity (Stehberg et al., 2012; Torres et al., 2012). The first evidence comes from hippocampal acute slices in which a decrease in extracellular Ca2+ concentration is generated by the local photolysis of a photolabile Ca2+ buffer. This opens Cx43 hemichannels in astrocytes through which ATP is released and activates P2Y1 purinergic receptors on a subset of inhibitory interneurons, initiating the generation of spikes by interneurons that in turn enhances synaptic inhibition at glutamatergic synapses (Torres et al., 2012). A similar neuroglial loop of interaction acting as a negative feedback mechanism is expected to occur during excessive glutamatergic activity that is associated with a decrease in extracellular Ca2+ concentration (Rusakov and Fine, 2003). The second set of evidence comes from in vivo experiments in which Cx43 hemichannels were inhibited (Stehberg et al., 2012). In this study, the rat basolateral amygdala was microinfused with the TAT-L2 peptide that selectively inhibits Cx43 hemichannels assumed to be only present in astrocytes. Such in vivo blockade of Cx43 hemichannels during memory consolidation induces amnesia for auditory fear conditioning, as assessed 24 h after training, without affecting short-term memory, locomotion, or shock reactivity. Moreover, the amnesic effect is transitory, specific for memory consolidation. Learning capacity recovers after co-infusion of the Cx43 hemichannel blocker and a mixture of gliotransmitters including glutamate and ATP. These observations suggest that gliotransmission mediated by Cx43 hemichannels is necessary for fear memory consolidation at the basolateral amygdala. Thus, the study of Stehberg et al. (2012) represents the first in vivo demonstration of a physiological role for astroglial Cx43 hemichannels in brain cognitive function. Finally, an in vivo contribution of Panx1 channels to cognition has recently been addressed by using a Panx1 knock out mouse (Prochnow et al., 2012). This constitutive knock out mouse was found to have increased excitability and potently enhanced early and persistent long-term potentiation (LTP) responses in the CA1 hippocampal region with additionally impaired spatial memory and object recognition memory. However, while the expression of Panx1 is well documented in hippocampal neurons (MacVicar and Thompson, 2010), its detection at the membrane of astrocytes is still debated (Iglesias et al., 2009; but see Orellana et al., 2010, 2011c). Consequently, it is premature to state that these Panx1-dependent changes in hippocampal models of learning and memory have an astroglial origin.
Neuronal Death and Brain Pathologies
While there are now a number of reports indicating that the expression of glial Cxs changes in many brain pathologies and injuries (Giaume et al., 2010), this remains to be investigated in detail for Panxs. On this basis, the question of Cx channels contributing to neuronal death and brain diseases has been debated for many years. Indeed, opposite perspectives regarding the roles of glial Cxs in neuronal death have been discussed and reviewed (see Kirchhoff et al., 2001; Rouach et al., 2002; Perez Velazquez et al., 2003; Nakase and Naus, 2004; Farahani et al., 2005; Giaume et al., 2007; Orellana et al., 2009). In general, this duality was attributed to differences in (i) the experimental models (in vitro, ex vivo, and in vivo) or the protocols used to induce neuronal death, (ii) the mode (pharmacology versus transgenic animals) of Cx channel inhibition selected, (iii) the type of neuronal death or pathology investigated, and (iv) the time points considered. However, retrospectively the opposite roles attributed to Cx channels could be simply due to the fact that for a long time this question was solely taking into account the gap junctional function of Cxs. Indeed, in most cases, interpretation of the data was not considering the hemichannel and channel functions of Cxs and Panxs, respectively. Nevertheless, the development of compounds and transgenic animals that discriminate between Cx gap junction channels, Cx hemichannels and Panx channels (see above) has allowed identification of their respective contribution in various in vitro and ex vivo models of brain pathologies (see Kielian, 2008; Orellana et al., 2009; Bennett et al., 2012). Although hemichannels have been reported to be involved in several brain pathologies or injuries (reviewed in Bennett et al., 2012) as well as infection (Karpuk et al., 2011; Xiong et al., 2012), we will illustrate this situation by two recent examples that demonstrate the impact of glial Cx hemichannel and Panx channel activation in cells on neuronal death.
Modifications in the pattern of Cx and Panx expression in glia are associated with phenotypic changes observed during neuroinflammation and the “reactive gliosis” that is a common feature of most brain diseases (see Sofroniew and Vinters, 2010). Indeed, activated microglia and reactive astrocytes exhibit changes in their respective pattern of expression for Panx1 and Cx43 as well as in their hemichannel function leading to neuronal damage and death (Bennett et al., 2012; Orellana et al., 2012b). When an inflammatory context is mimicked in vitro by using LPS treatment, Cx43 hemichannel activity is triggered in astrocytes through the production of TNF-α and IL-1β by activated microglia. These events are not observed when astrocytes are cultured from Cx43 knock out mice (Retamal et al., 2007a). Furthermore, in neuron–astrocyte co-cultures the N-methyl-D-aspartate (NMDA)-induced neuronal excitotoxicity is reinforced by TNF-α + IL-1β treatment, while such a potentiating effect is not observed in cultures of neurons alone treated with the two cytokines (Froger et al., 2010). This observation suggests that the well-known protective role of astrocytes against excitotoxicity (Chen and Swanson, 2003) is impaired under pro-inflammatory conditions when astroglial Cx43 hemichannels are activated. Neuron–astrocyte co-cultures, made with astrocytes from Cx43 knock out and neurons from wild-type mice, revealed that the NMDA excitotoxicity is not reinforced by the pro-inflammatory treatment applied on these co-cultures made with Cx43-deficient astrocytes (Froger et al., 2010). This result suggests that astroglial Cx43 is involved in the potentiated neurotoxicity induced by pro-inflammatory treatments. Interestingly, application (<30 min) of mimetic peptides (Gap26, Gap27) that inhibit Cx43 hemichannels and have no effect on Cx43 gap junctional communication (Retamal et al., 2007a), prevents the potentiated neurotoxicity response induced by pro-inflammatory cytokines (Froger et al., 2010; Orellana et al., 2012b).
Connexin hemichannels might also play a role in the pathological context of neurodegenerative diseases. Indeed, it is becoming increasingly clear that glial cells are critical players in the progressive neurodegeneration of Alzheimer’s disease (Kraft et al., 2013). Among others, the amyloid cascade represents one of the key pathways involved in the pathogenesis of the disease (see Pimplikar, 2009). Using separate primary cultures and co-cultures of microglia, astrocytes, and neurons it was shown that treatment with the β-amyloid peptide (Aβ) first activates microglia, resulting in the opening of Cx43 hemichannels and Panx1 channels in these cells. Such channel activations allow the release of glutamate and ATP that impair neuronal survival. Aβ-activated microglia also produces TNF-α and IL-1β that, as indicated above, induce Cx43 astroglial hemichannel activation that again results in the release of glutamate and ATP (Orellana et al., 2011c). In fine these gliotransmitters originating from glial cells trigger neuronal damage and induce their death. Thus, such a cascade of Cx glial hemichannel and Panx channel activation could partially account for the progression of the neurodegenerative disease. These in vitro and ex vivo observations could be related to the changes in Cx43 expression observed in reactive astrocytes that contact amyloid deposit in a murine model of Alzheimer’s disease (Mei et al., 2010) and in brain from Alzheimer’s patients (Nagy et al., 1996; Koulakoff et al., 2012).
Concluding Remarks
During the last decade our view and understanding of the structure (Maeda and Tsukihara, 2011), mutations (Pfenniger et al., 2011), properties (Rackauskas et al., 2010), and biological roles (Kar et al., 2012) of Cx channels has been incredibly enlarged, and to some extent this is also true for Panx channels (MacVicar and Thompson, 2010; Penuela et al., 2013). Interestingly, in glial cells this feature is exemplary because in addition to their well-documented molecular support for their two channel functions, non-channel functions have been also identified for Cxs, including cell adhesion mechanisms involved in radial migration of cortical neurons (Elias et al., 2007; Cina et al., 2009), modulation of P2Y purinergic receptors signaling (Scemes, 2008), regulation of cytoskeletal dynamics (Olk et al., 2010) and control of gene expression (Iacobas et al., 2004). Such a view could even be further enlarged by the recent report of another new class of membrane protein called calcium homeostasis modulator CALHM1 that shares structural similarities with Cx, Panx, and innexin channels and functional properties similar to hemichannels, such as ATP release in taste transduction (Siebert et al., 2013; Taruno et al., 2013).
In the present review, we have focused on a Cx/Panx-based channel function which 10 years ago was only sparsely considered in the central nervous system and in neuroglial interactions. Since then, the identification of external and intracellular signals that trigger the activation of hemichannels, the understanding of their biophysics and their regulation have provided a better understanding of the roles that glial Cxs and Panxs play in healthy and diseased brains. Indeed, as reported above, there is now strong evidence to state that Cx and Panx channels in glia have an impact on neuronal activity and survival. However, there are at least two aims that remain to be achieved in order to determine whether and how the contribution of glial Cxs and/or Panxs is causal or secondary in normal and pathological situations. One is to distinguish what is due to gap junction channels versus hemichannels, the other is to discriminate between Cx hemichannels and Panx channels. To achieve these goals it is essential to have available pharmacological and genetic tools with glial cell type, Cx type, and Panx type specificities (see Giaume and Theis, 2010). Through a simple pharmacological approach this is unlikely to be achieved since Cx and Panx channels are sensitive to the same agents so far described. However, strategies using short-term treatment with mimetic peptides (Evans et al., 2012) or antibodies targeting extracellular domains (Riquelme et al., 2013) could be developed for all the glial Cxs/Panxs and their specificity demonstrated. In contrast, due to the cytoplasmic location of their two channel ends, gap junction channels are likely less easy to target by a pharmacological strategy. Another more accurate approach consists in the development of transgenic mice with appropriate mutations that prevent channel pairing without affecting hemichannel activity or that block one but not the other channel function. Alternatively, small interfering RNA (siRNA) and transgenic/viral techniques have been used with some success (see Giaume and Theis, 2010). But here again, the expression of multiple Cxs in glial cells makes the task difficult with genetic and molecular approaches. A recent report by Nedergaard’s group of an enhanced synaptic plasticity (LTP) due to the engraftment of human glial progenitors into immunodeficient mice (Han et al., 2013) gives weight to achieve such difficult challenges. Indeed, upon maturation in these grafted mice, human astrocytes were shown to be coupled to host astrocytes, suggesting a contribution of Cx channels in neuroglial interactions during synaptic plasticity. In line with such contribution, double Cx43/Cx30 knock out is associated with reduced LTP (Pannasch et al., 2011). Also, the observation of an up-regulation of astroglial Cxs in reactive astrocytes contacting amyloid plaques in a transgenic murine model of Alzheimer’s disease has been validated in human patient brain samples (Nagy et al., 1996; Koulakoff et al., 2012), giving another example of the value in searching for strategies that block Cx channels.
Conflict of Interest Statement
The authors declare that the research was conducted in the absence of any commercial or financial relationships that could be construed as a potential conflict of interest.
Acknowledgments
This review arose from discussions made possible by a Colloquium Abroad grant from the Peter Wall Institute for Advanced Studies, University of British Columbia, in partnership with the Collège de France and the Fondation Hugo du Collège de France, Paris, France, as well as funds from the Vice President Research & International, University of British Columbia. This study includes reference to research that was supported by Grants from ECOS-CONICYT (to Christian Giaume and Juan C. Sáez), The Caisse de Retraite et de Prévoyance des Clercs et Employés de Notaires and the Ligue Européenne Contre la Maladie d’Alzheimer (to Christian Giaume), the Fund for Scientific Research Flanders (to Luc Leybaert) and Belgian Science Policy (Interuniversity Attraction Poles to Luc Leybaert), Fondecyt project 1120214 (to Juan C. Sáez), 1111033 (to Juan C. Sáez), Chilean Science Millennium Institute (P09-022-F; to Juan C. Sáez), the Canadian Institutes for Health Research (to Christian C. Naus), and the Heart and Stroke Foundation of Canada (Christian C. Naus).
References
Ahmad, S., and Evans, W. H. (2002). Post-translational integration and oligomerization of connexin 26 in plasma membranes and evidence of formation of membrane pores: implications for the assembly of gap junctions. Biochem. J. 365, 693–699. doi: 10.1042/BJ20011572
Allaman, I., Belanger, M., and Magistretti, P. J. (2011). Astrocyte–neuron metabolic relationships: for better and for worse. Trends Neurosci. 34, 76–87. doi: 10.1016/j.tins.2010.12.001
Altevogt, B. M., Kleopa, K. A., Postma, F. R., Scherer, S. S., and Paul, D. L. (2002). Connexin29 is uniquely distributed within myelinating glial cells of the central and peripheral nervous systems. J. Neurosci. 22, 6458–6470.
Alvarez-Maubecin, V., García-Hernández, F., Williams, J. T., and Van Bockstaele, E. J. (2000). Functional coupling between neurons and glia. J. Neurosci. 20, 4091–4098.
Anderson, C. M., Bergher, J. P., and Swanson, R. A. (2004). ATP-induced ATP release from astrocytes. J. Neurochem. 88, 246–256. doi: 10.1111/j.1471-4159.2004.02204.x
Andrew, R. D., MacVicar, B. A., Dudek, F. E., and Hatton, G. I. (1981). Dye transfer through gap junctions between neuroendocrine cells of rat hypothalamus. Science 211, 1187–1189. doi: 10.1126/science.7466393
Anselmi, F., Hernandez, V. H., Crispino, G., Seydel, A., Ortolano, S., Roper, S. D., et al. (2008). ATP release through connexin hemichannels and gap junction transfer of second messengers propagate Ca2+ signals across the inner ear. Proc. Natl. Acad. Sci. U.S.A. 105, 18770–18775. doi: 10.1073/pnas.0800793105
Arcuino, G., Lin, J. H., Takano, T., Liu, C., Jiang, L., Gao, Q., et al. (2002). Intercellular calcium signaling mediated by point-source burst release of ATP. Proc. Natl. Acad. Sci. U.S.A. 99, 9840–9845. doi: 10.1073/pnas.152588599
Bao, L., Locovei, S., and Dahl, G. (2004a). Pannexin membrane channels are mechanosensitive conduits for ATP. FEBS Lett. 572, 65–68. doi: 10.1016/j.febslet.2004.07.009
Bao, L., Sachs, F., and Dahl, G. (2004b). Connexins are mechanosensitive. Am. J. Physiol. Cell Physiol. 287, C1389–C1395. doi: 10.1152/ajpcell.00220.2004
Bao, X., Reuss, L., and Altenberg, G. A. (2004c). Regulation of purified and reconstituted connexin 43 hemichannels by protein kinase C-mediated phosphorylation of Serine 368. J. Biol. Chem. 279, 20058–20066. doi: 10.1074/jbc.M311137200
Bao, X., Lee, S. C., Reuss, L., and Altenberg, G. A. (2007). Change in permeant size selectivity by phosphorylation of connexin 43 gap-junctional hemichannels by PKC. Proc. Natl. Acad. Sci. U.S.A. 104, 4919–4924. doi: 10.1073/pnas.0603154104
Baranova, A., Ivanov, D., Petrash, N., Pestova, A., Skoblov, M., Kelmanson, I., et al. (2004). The mammalian pannexin family is homologous to the invertebrate innexin gap junction proteins. Genomics 83, 706–716. doi: 10.1016/j.ygeno.2003.09.025
Bargiello, T. A., Tang, Q., Oh, S., and Kwon, T. (2012). Voltage-dependent conformational changes in connexin channels. Biochim. Biophys. Acta 1818, 1807–1822. doi: 10.1016/j.bbamem.2011.09.019
Batra, N., Burra, S., Siller-Jackson, A. J., Gu, S., Xia, X., Weber, G. F., et al. (2012). Mechanical stress-activated integrin alpha5beta1 induces opening of connexin 43 hemichannels. Proc. Natl. Acad. Sci. U.S.A. 109, 3359–3364. doi: 10.1073/pnas.1115967109
Belousov, A. B., and Fontes, J. D. (2013). Neuronal gap junctions: making and breaking connections during development and injury. Trends Neurosci. 36, 227–236. doi: 10.1016/j.tins.2012.11.001
Bennett, M. V., Contreras, J. E., Bukauskas, F. F., and Sáez, J. C. (2003). New roles for astrocytes: gap junction hemichannels have something to communicate. Trends Neurosci. 26, 610–617. doi: 10.1016/j.tins.2003.09.008
Bennett, M. V., Garré, J. M., Orellana, J. A., Bukauskas, F. F., Nedergaard, M., and Sáez, J. C. (2012). Connexin and pannexin hemichannels in inflammatory responses of glia and neurons. Brain Res. 1487, 3–15. doi: 10.1016/j.brainres.2012.08.042
Bevans, C. G., and Harris, A. L. (1999). Regulation of connexin channels by pH. Direct action of the protonated form of taurine and other aminosulfonates. J. Biol. Chem. 274, 3711–3719. doi: 10.1074/jbc.274.6.3711
Bevans, C. G., Kordel, M., Rhee, S. K., and Harris, A. L. (1998). Isoform composition of connexin channels determines selectivity among second messengers and uncharged molecules. J. Biol. Chem. 273, 2808–2816. doi: 10.1074/jbc.273.5.2808
Bezprozvanny, I., Watras, J., and Ehrlich, B. E. (1991). Bell-shaped calcium-response curves of Ins(1,4,5)P3- and calcium-gated channels from endoplasmic reticulum of cerebellum. Nature 351, 751–754. doi: 10.1038/351751a0
Bodendiek, S. B., and Raman, G. (2010). Connexin modulators and their potential targets under the magnifying glass. Curr. Med. Chem. 17, 4191–4230. doi: 10.2174/092986710793348563
Braet, K., Vandamme, W., Martin, P. E., Evans, W. H., and Leybaert, L. (2003). Photoliberating inositol-1,4,5-trisphosphate triggers ATP release that is blocked by the connexin mimetic peptide gap 26. Cell Calcium 33, 37–48. doi: 10.1016/S0143-4160(02)00180-X
Bruzzone, R., Barbe, M. T., Jakob, N. J., and Monyer, H. (2005). Pharmacological properties of homomeric and heteromeric pannexin hemichannels expressed in Xenopus oocytes. J. Neurochem. 92, 1033–1043. doi: 10.1111/j.1471-4159.2004.02947.x
Bruzzone, R., Hormuzdi, S. G., Barbe, M. T., Herb, A., and Monyer, H. (2003). Pannexins, a family of gap junction proteins expressed in brain. Proc. Natl. Acad. Sci. U.S.A. 100, 13644–13649. doi: 10.1073/pnas.2233464100
Bruzzone, S., Guida, L., Zocchi, E., Franco, L., and De Flora, A. (2001). Connexin 43 hemi channels mediate Ca2+-regulated transmembrane NAD+ fluxes in intact cells. FASEB J. 15, 10–12.
Chen, Y., and Swanson, R. A. (2003). Astrocytes and brain injury. J. Cereb. Blood Flow Metab. 23, 137–149. doi: 10.1097/00004647-200302000-00001
Cina, C., Maass, K., Theis, M., Willecke, K., Bechberger, J. F., and Naus, C. C. (2009). Involvement of the cytoplasmic C-terminal domain of connexin43 in neuronal migration. J. Neurosci. 29, 2009–2021. doi: 10.1523/JNEUROSCI.5025-08.2009
Clair, C., Combettes, L., Pierre, F., Sansonetti, P., and Tran Van Nhieu, G. (2008). Extracellular-loop peptide antibodies reveal a predominant hemichannel organization of connexins in polarized intestinal cells. Exp. Cell Res. 314, 1250–1265. doi: 10.1016/j.yexcr.2007.12.021
Contreras, J. E., Sáez, J. C., Bukauskas, F. F., and Bennett, M. V. (2003). Gating and regulation of connexin 43 (Cx43) hemichannels. Proc. Natl. Acad. Sci. U.S.A. 100, 11388–11393. doi: 10.1073/pnas.1434298100
Contreras, J. E., Sánchez, H. A., Eugenín, E. A., Speidel, D., Theis, M., Willecke, K., et al. (2002). Metabolic inhibition induces opening of unapposed connexin 43 gap junction hemichannels and reduces gap junctional communication in cortical astrocytes in culture. Proc. Natl. Acad. Sci. U.S.A. 99, 495–500. doi: 10.1073/pnas.012589799
Contreras, J. E., Sánchez, H. A., Véliz, L. P., Bukauskas, F. F., Bennett, M. V., and Sáez, J. C. (2004). Role of connexin-based gap junction channels and hemichannels in ischemia-induced cell death in nervous tissue. Brain Res. Brain Res. Rev. 47, 290–303. doi: 10.1016/j.brainresrev.2004.08.002
Cotrina, M. L., Lin, J. H., Alves-Rodrigues, A., Liu, S., Li, J., Azmi-Ghadimi, H., et al. (1998). Connexins regulate calcium signaling by controlling ATP release. Proc. Natl. Acad. Sci. U.S.A. 95, 15735–15740. doi: 10.1073/pnas.95.26.15735
De Bock, M., Culot, M., Wang, N., Bol, M., Decrock, E., De Vuyst, E. et al. (2011). Connexin channels provide a target to manipulate brain endothelial calcium dynamics and blood-brain barrier permeability. J. Cereb. Blood Flow Metab. 31, 1942–1957. doi: 10.1038/jcbfm.2011.86
De Bock, M., Wang, N., Bol, M., Decrock, E., Ponsaerts, R., Bultynck, G., et al. (2012). Connexin 43 hemichannels contribute to cytoplasmic Ca2+ oscillations by providing a bimodal Ca2+-dependent Ca2+ entry pathway. J. Biol. Chem. 287, 12250–12266. doi: 10.1074/jbc.M111.299610
Decrock, E., De Vuyst, E., Vinken, M., Van Moorhem, M., Vranckx, K., Wang, N., et al. (2009). Connexin 43 hemichannels contribute to the propagation of apoptotic cell death in a rat C6 glioma cell model. Cell Death Differ. 16, 151–163. doi: 10.1038/cdd.2008.138
Decrock, E., Vinken, M., Bol, M., D’Herde, K., Rogiers, V., Vandenabeele, P., et al. (2011). Calcium and connexin-based intercellular communication, a deadly catch? Cell Calcium 50, 310–321. doi: 10.1016/j.ceca.2011.05.007
D’Hondt, C., Iyyathurai, J., Wang, N., Gourdie, R. G., Himpens, B., Leybaert, L., et al. (2013). Negatively charged residues (Asp378 and Asp379) in the last ten amino acids of the C-terminal tail of Cx43 hemichannels are essential for loop/tail interactions. Biochem. Biophys. Res. Commun. 432, 707–712. doi: 10.1016/j.bbrc.2013.01.066
D’Hondt, C., Ponsaerts, R., De Smedt, H., Vinken, M., De Vuyst, E., De Bock, M., et al. (2011). Pannexin channels in ATP release and beyond: an unexpected rendezvous at the endoplasmic reticulum. Cell. Signal. 23, 305–316. doi: 10.1016/j.cellsig.2010.07.018
Desplantez, T., Verma, V., Leybaert, L., Evans, W. H., and Weingart, R. (2012). Gap26, a connexin mimetic peptide, inhibits currents carried by connexin43 hemichannels and gap junction channels. Pharmacol. Res. 65, 546–552. doi: 10.1016/j.phrs.2012.02.002
DeVries, S. H., and Schwartz, E. A. (1992). Hemi-gap-junction channels in solitary horizontal cells of the catfish retina. J. Physiol. 445, 201–230.
De Vuyst, E., Decrock, E., Cabooter, L., Dubyak, G. R., Naus, C. C., Evans, W. H., et al. (2006). Intracellular calcium changes trigger connexin 32 hemichannel opening. EMBO J. 25, 34–44. doi: 10.1038/sj.emboj.7600908
De Vuyst, E., Decrock, E., De Bock, M., Yamasaki, H., Naus, C. C., Evans, W. H., et al. (2007). Connexin hemichannels and gap junction channels are differentially influenced by lipopolysaccharide and basic fibroblast growth factor. Mol. Biol. Cell 18, 34–46. doi: 10.1091/mbc.E06-03-0182
De Vuyst, E., Wang, N., Decrock, E., De Bock, M., Vinken, M., Van Moorhem, M., et al. (2009). Ca(2+) regulation of connexin 43 hemichannels in C6 glioma and glial cells. Cell Calcium 46, 176–187. doi: 10.1016/j.ceca.2009.07.002
Dobrenis, K., Chang, H. Y., Pina-Benabou, M. H., Woodroffe, A., Lee, S. C., Rozental, R., et al. (2005). Human and mouse microglia express connexin36, and functional gap junctions are formed between rodent microglia and neurons. J. Neurosci. Res. 82, 306–315. doi: 10.1002/jnr.20650
Dobrowolski, R., Sasse, P., Schrickel, J. W., Watkins, M., Kim, J. S., Rackauskas, M., et al. (2008). The conditional connexin43G138R mouse mutant represents a new model of hereditary oculodentodigital dysplasia in humans. Hum. Mol. Genet. 17, 539–554. doi: 10.1093/hmg/ddm329
Dobrowolski, R., Sommershof, A., and Willecke, K. (2007). Some oculodentodigital dysplasia-associated Cx43 mutations cause increased hemichannel activity in addition to deficient gap junction channels. J. Membr. Biol. 219, 9–17. doi: 10.1007/s00232-007-9055-7
Domercq, M., Perez-Samartin, A., Aparicio, D., Alberdi, E., Pampliega, O., and Matute, C. (2010). P2X7 receptors mediate ischemic damage to oligodendrocytes. Glia 58, 730–740. doi: 10.1002/glia.20958
Elias, L. A., Wang, D. D., and Kriegstein, A. R. (2007). Gap junction adhesion is necessary for radial migration in the neocortex. Nature 448, 901–907. doi: 10.1038/nature06063
Eugenín, E. A., Eckardt, D., Theis, M., Willecke, K., Bennett, M. V., and Sáez, J. C. (2001). Microglia at brain stab wounds express connexin 43 and in vitro form functional gap junctions after treatment with interferon-gamma and tumor necrosis factor-alpha. Proc. Natl. Acad. Sci. U.S.A. 98, 4190–4195. doi: 10.1073/pnas.051634298
Evans, W. H., and Boitano, S. (2001). Connexin mimetic peptides: specific inhibitors of gap-junctional intercellular communication. Biochem. Soc. Trans. 29, 606–612. doi: 10.1042/BST0290606
Evans, W. H., Bultynck, G., and Leybaert, L. (2012). Manipulating connexin communication channels: use of peptidomimetics and the translational outputs. J. Membr. Biol. 245, 437–449. doi: 10.1007/s00232-012-9488-5
Evans, W. H., De Vuyst, E., and Leybaert, L. (2006). The gap junction cellular internet: connexin hemichannels enter the signalling limelight. Biochem. J. 397, 1–14. doi: 10.1042/BJ20060175
Farahani, R., Pina-Benabou, M. H., Kyrozis, A., Siddiq, A., Barradas, P. C., Chiu, F. C., et al. (2005). Alterations in metabolism and gap junction expression may determine the role of astrocytes as “good samaritans” or executioners. Glia 50, 351–361. doi: 10.1002/glia.20213
Figueroa, X. F., Lillo, M. A., Gaete, P. S., Riquelme, M., and Sáez, J. C. (2013). Diffusion of nitric oxide across cell membranes of the vascular wall requires specific connexin-based channels. Neuropharmacology. doi: 10.1016/j.neuropharm.2013.02.022 [Epub ahead of print].
Finkbeiner, S. (1992). Calcium waves in astrocytes-filling in the gaps. Neuron 8, 1101–1108. doi: 10.1016/0896-6273(92)90131-V
Fiori, M. C., Figueroa, V., Zoghbi, M. E., Sáez, J. C., Reuss, L., and Altenberg, G. A. (2012). Permeation of calcium through purified connexin 26 hemichannels. J. Biol. Chem. 287, 40826–40834. doi: 10.1074/jbc.M112.383281
Fróes, M. M., Correia, A. H., Garcia-Abreu, J., Spray, D. C., Campos de Carvalho, A. C., and Neto, M. V. (1999). Gap-junctional coupling between neurons and astrocytes in primary central nervous system cultures. Proc. Natl. Acad. Sci. U.S.A. 96, 7541–7546. doi: 10.1073/pnas.96.13.7541
Froger, N., Orellana, J. A., Calvo, C. F., Amigou, E., Kozoriz, M. G., Naus, C. C., et al. (2010). Inhibition of cytokine-induced connexin43 hemichannel activity in astrocytes is neuroprotective. Mol. Cell. Neurosci. 45, 37–46. doi: 10.1016/j.mcn.2010.05.007
Froger, N., Orellana, J. A., Cohen-Salmon, M., Ezan, P., Amigou, E., Sáez, J. C., et al. (2009). Cannabinoids prevent the opposite regulation of astroglial connexin43 hemichannels and gap junction channels induced by pro-inflammatory treatments. J. Neurochem. 111, 1383–1397.
Fushiki, S., Perez Velazquez, J. L., Zhang, L., Bechberger, J. F., Carlen, P. L., and Naus, C. C. (2003). Changes in neuronal migration in neocortex of connexin43 null mutant mice. J. Neuropathol. Exp. Neurol. 62, 304–314.
Garg, S., Md Syed, M., and Kielian, T. (2005). Staphylococcus aureus-derived peptidoglycan induces Cx43 expression and functional gap junction intercellular communication in microglia. J. Neurochem. 95, 475–483. doi: 10.1111/j.1471-4159.2005.03384.x
Garré, J. M., Retamal, M. A., Cassina, P., Barbeito, L., Bukauskas, F. F., Sáez, J. C., et al. (2010). FGF-1 induces ATP release from spinal astrocytes in culture and opens pannexin and connexin hemichannels. Proc. Natl. Acad. Sci. U.S.A. 107, 22659–22664. doi: 10.1073/pnas.1013793107
Giaume, C., Fromaget, C., el Aoumari, A., Cordier, J., Glowinski, J., and Gros, D. (1991). Gap junctions in cultured astrocytes: single-channel currents and characterization of channel-forming protein. Neuron 6, 133–143. doi: 10.1016/0896-6273(91)90128-M
Giaume, C., Kirchhoff, F., Matute, C., Reichenbach, A., and Verkhratsky, A. (2007). Glia: the fulcrum of brain diseases. Cell Death Differ. 14, 1324–1335. doi: 10.1038/sj.cdd.4402144
Giaume, C., Koulakoff, A., Roux, L., Holcman, D., and Rouach, N. (2010). Astroglial networks: a step further in neuroglial and gliovascular interactions. Nat. Rev. Neurosci. 11, 87–99. doi: 10.1038/nrn2757
Giaume, C., Orellana, J. A., Abudara, V., and Sáez, J. C. (2012). Connexin-based channels in astrocytes: how to study their properties. Methods Mol. Biol. 814, 283–303. doi: 10.1007/978-1-61779-452-0_19
Giaume, C., and Theis, M. (2010). Pharmacological and genetic approaches to study connexin-mediated channels in glial cells of the central nervous system. Brain Res. Rev. 63, 160–176. doi: 10.1016/j.brainresrev.2009.11.005
Gómez-Hernández, J. M., de Miguel, M., Larrosa, B., Gonzalez, D., and Barrio, L. C. (2003). Molecular basis of calcium regulation in connexin-32 hemichannels. Proc. Natl. Acad. Sci. U.S.A. 100, 16030–16035. doi: 10.1073/pnas.2530348100
González, D., Gomez-Hernandez, J. M., and Barrio, L. C. (2006). Species specificity of mammalian connexin-26 to form open voltage-gated hemichannels. FASEB J. 20, 2329–2338. doi: 10.1096/fj.06-5828com
Goodenough, D. A., and Paul, D. L. (2003). Beyond the gap: functions of unpaired connexon channels. Nat. Rev. Mol. Cell Biol. 4, 285–294. doi: 10.1038/nrm1072
Gossman, D. G., and Zhao, H. B. (2008). Hemichannel-mediated inositol 1,4,5-trisphosphate (IP3) release in the cochlea: a novel mechanism of IP3 intercellular signaling. Cell Commun. Adhes. 15, 305–315. doi: 10.1080/15419060802357217
Han, X., Chen, M., Wang, F., Windrem, M., Wang, S., Shanz, S., et al. (2013). Forebrain engraftment by human glial progenitor cells enhances synaptic plasticity and learning in adult mice. Cell Stem Cell 12, 342–353. doi: 10.1016/j.stem.2012.12.015
Harris, A. L. (2007). Connexin channel permeability to cytoplasmic molecules. Prog. Biophys. Mol. Biol. 94, 120–143. doi: 10.1016/j.pbiomolbio.2007.03.011
Hassinger, T. D., Guthrie, P. B., Atkinson, P. B., Bennett, M. V., and Kater, S. B. (1996). An extracellular signaling component in propagation of astrocytic calcium waves. Proc. Natl. Acad. Sci. U.S.A. 93, 13268–13273. doi: 10.1073/pnas.93.23.13268
Haydon, P. G., and Carmignoto, G. (2006). Astrocyte control of synaptic transmission and neurovascular coupling. Physiol. Rev. 86, 1009–1031. doi: 10.1152/physrev.00049.2005
Heidemann, A. C., Schipke, C. G., and Kettenmann, H. (2005). Extracellular application of nicotinic acid adenine dinucleotide phosphate induces Ca2+ signaling in astrocytes in situ. J. Biol. Chem. 280, 35630–35640. doi: 10.1074/jbc.M507338200
Heinrich, A., Ando, R. D., Turi, G., Rozsa, B., and Sperlagh, B. (2012). K+ depolarization evokes ATP, adenosine and glutamate release from glia in rat hippocampus: a microelectrode biosensor study. Br. J. Pharmacol. 167, 1003–1020. doi: 10.1111/j.1476-5381.2012.01932.x
Hirst-Jensen, B. J., Sahoo, P., Kieken, F., Delmar, M., and Sorgen, P. L. (2007). Characterization of the pH-dependent interaction between the gap junction protein connexin43 carboxyl terminus and cytoplasmic loop domains. J. Biol. Chem. 282, 5801–5813. doi: 10.1074/jbc.M605233200
Hofer, A., and Dermietzel, R. (1998). Visualization and functional blocking of gap junction hemichannels (connexons) with antibodies against external loop domains in astrocytes. Glia 24, 141–154. doi: 10.1002/(SICI)1098-1136(199809)24:1
Hoogland, T. M., Kuhn, B., Gobel, W., Huang, W., Nakai, J., Helmchen, F., et al. (2009). Radially expanding transglial calcium waves in the intact cerebellum. Proc. Natl. Acad. Sci. U.S.A. 106, 3496–3501. doi: 10.1073/pnas.0809269106
Iacobas, D. A., Scemes, E., and Spray, D. C. (2004). Gene expression alterations in connexin null mice extend beyond the gap junction. Neurochem. Int. 45, 243–250. doi: 10.1016/j.neuint.2003.12.008
Iglesias, R., Dahl, G., Qiu, F., Spray, D. C., and Scemes, E. (2009). Pannexin 1: the molecular substrate of astrocyte “hemichannels”. J. Neurosci. 29, 7092–7097. doi: 10.1523/JNEUROSCI.6062-08.2009
Iglesias, R. M., and Spray, D. C. (2012). Pannexin1-mediated ATP release provides signal transmission between Neuro2A cells. Neurochem. Res. 37, 1355–1363. doi: 10.1007/s11064-012-0720-6
Jiang, S., Yuan, H., Duan, L., Cao, R., Gao, B., Xiong, Y. F., et al. (2011). Glutamate release through connexin 43 by cultured astrocytes in a stimulated hypertonicity model. Brain Res. 1392, 8–15. doi: 10.1016/j.brainres.2011.03.056
John, S. A., Kondo, R., Wang, S. Y., Goldhaber, J. I., and Weiss, J. N. (1999). Connexin-43 hemichannels opened by metabolic inhibition. J. Biol. Chem. 274, 236–240. doi: 10.1074/jbc.274.1.236
Kang, J., Kang, N., Lovatt, D., Torres, A., Zhao, Z., Lin, J., et al. (2008). Connexin 43 hemichannels are permeable to ATP. J. Neurosci. 28, 4702–4711. doi: 10.1523/JNEUROSCI.5048-07.2008
Kar, R., Batra, N., Riquelme, M. A., and Jiang, J. X. (2012). Biological role of connexin intercellular channels and hemichannels. Arch. Biochem. Biophys. 524, 2–15. doi: 10.1016/j.abb.2012.03.008
Karpuk, N., Burkovetskaya, M., Fritz, T., Angle, A., and Kielian, T. (2011). Neuroinflammation leads to region-dependent alterations in astrocyte gap junction communication and hemichannel activity. J. Neurosci. 31, 414–425. doi: 10.1523/JNEUROSCI.5247-10.2011
Katakowski, M., Buller, B., Wang, X., Rogers, T., and Chopp, M. (2010). Functional microRNA is transferred between glioma cells. Cancer Res. 70, 8259–8263. doi: 10.1158/0008-5472.CAN-10-0604
Kielian, T. (2008). Glial connexins and gap junctions in CNS inflammation and disease. J. Neurochem. 106, 1000–1016. doi: 10.1111/j.1471-4159.2008.05405.x
Kienitz, M. C., Bender, K., Dermietzel, R., Pott, L., and Zoidl, G. (2011). Pannexin 1 constitutes the large conductance cation channel of cardiac myocytes. J. Biol. Chem. 286, 290–298. doi: 10.1074/jbc.M110.163477
Kirchhoff, F., Dringen, R., and Giaume, C. (2001). Pathways of neuron–astrocyte interactions and their possible role in neuroprotection. Eur. Arch. Psychiatry Clin. Neurosci. 251, 159–169. doi: 10.1007/s004060170036
Koulakoff, A., Mei, X., Orellana, J. A., Sáez, J. C., and Giaume, C. (2012). Glial connexin expression and function in the context of Alzheimer’s disease. Biochim. Biophys. Acta 1818, 2048–2057. doi: 10.1016/j.bbamem.2011.10.001
Kozoric, M., and Naus, C. C. (2013). “Gap junction-mediated neuroprotection,” in Gap Junctions in the Brain: Physiological and Pathological Roles, ed. E. Dere (London: Academic Press), 231–246. doi: 10.1016/B978-0-12-415901-3.00014-1
Kraft, A. W., Hu, X., Yoon, H., Yan, P., Xiao, Q., Wang, Y., et al. (2013). Attenuating astrocyte activation accelerates plaque pathogenesis in APP/PS1 mice. FASEB J. 27, 187–198. doi: 10.1096/fj.12-208660
Kuchibhotla, K. V., Lattarulo, C. R., Hyman, B. T., and Bacskai, B. J. (2009). Synchronous hyperactivity and intercellular calcium waves in astrocytes in Alzheimer mice. Science 323, 1211–1215. doi: 10.1126/science.1169096
Kuga, N., Sasaki, T., Takahara, Y., Matsuki, N., and Ikegaya, Y. (2011). Large-scale calcium waves traveling through astrocytic networks in vivo. J. Neurosci. 31, 2607–2614. doi: 10.1523/JNEUROSCI.5319-10.2011
Kwon, T., Tang, Q., and Bargiello, T. A. (2013). Voltage-dependent gating of the Cx32*43E1 hemichannel: conformational changes at the channel entrances. J. Gen. Physiol. 141, 243–259. doi: 10.1085/jgp.201210839
Lai, C. P., Bechberger, J. F., Thompson, R. J., MacVicar, B. A., Bruzzone, R., and Naus, C. C. (2007). Tumor-suppressive effects of pannexin 1 in C6 glioma cells. Cancer Res. 67, 1545–1554. doi: 10.1158/0008-5472.CAN-06-1396
Leybaert, L., and Sanderson, M. J. (2012). Intercellular Ca(2+) waves: mechanisms and function. Physiol. Rev. 92, 1359–1392.doi: 10.1152/physrev.00029.2011
Li, H., Liu, T. F., Lazrak, A., Peracchia, C., Goldberg, G. S., Lampe, P. D., et al. (1996). Properties and regulation of gap junctional hemichannels in the plasma membranes of cultured cells. J. Cell Biol. 134, 1019–1030. doi: 10.1083/jcb.134.4.1019
Liu, F., Arce, F. T., Ramachandran, S., and Lal, R. (2006). Nanomechanics of hemichannel conformations: connexin flexibility underlying channel opening and closing. J. Biol. Chem. 281, 23207–23217. doi: 10.1074/jbc.M605048200
Liu, X., Sun, L., Torii, M., and Rakic, P. (2012). Connexin 43 controls the multipolar phase of neuronal migration to the cerebral cortex. Proc. Natl. Acad. Sci. U.S.A. 109, 8280–8285. doi: 10.1073/pnas.1205880109
Locke, D., Kieken, F., Tao, L., Sorgen, P. L., and Harris, A. L. (2011). Mechanism for modulation of gating of connexin26-containing channels by taurine. J. Gen. Physiol. 138, 321–339. doi: 10.1085/jgp.201110634
Locovei, S., Wang, J., and Dahl, G. (2006). Activation of pannexin 1 channels by ATP through P2Y receptors and by cytoplasmic calcium. FEBS Lett. 580, 239–244. doi: 10.1016/j.febslet.2005.12.004
Lohman, A. W., Weaver, J. L., Billaud, M., Sandilos, J. K., Griffiths, R., Straub, A. C., et al. (2012). S-nitrosylation inhibits pannexin 1 channel function. J. Biol. Chem. 287, 39602–39612. doi: 10.1074/jbc.M112.397976
Luckprom, P., Kanjanamekanant, K., and Pavasant, P. (2011). Role of connexin43 hemichannels in mechanical stress-induced ATP release in human periodontal ligament cells. J. Periodontal Res. 46, 607–615. doi: 10.1111/j.1600-0765.2011.01379.x
Ma, W., Compan, V., Zheng, W., Martin, E., North, R. A., Verkhratsky, A., et al. (2012). Pannexin 1 forms an anion-selective channel. Pflügers Arch. 463, 585–592. doi: 10.1007/s00424-012-1077-z
MacVicar, B. A., and Thompson, R. J. (2010). Non-junction functions of pannexin-1 channels. Trends Neurosci. 33, 93–102. doi: 10.1016/j.tins.2009.11.007
Maeda, S., and Tsukihara, T. (2011). Structure of the gap junction channel and its implications for its biological functions. Cell. Mol. Life Sci. 68, 1115–1129. doi: 10.1007/s00018-010-0551-z
Maezawa, I., and Jin, L. W. (2010). Rett syndrome microglia damage dendrites and synapses by the elevated release of glutamate. J. Neurosci. 30, 5346–5356. doi: 10.1523/JNEUROSCI.5966-09.2010
Martin, P. E., Wall, C., and Griffith, T. M. (2005). Effects of connexin-mimetic peptides on gap junction functionality and connexin expression in cultured vascular cells. Br. J. Pharmacol. 144, 617–627. doi: 10.1038/sj.bjp.0706102
Matchkov, V. V., Rahman, A., Bakker, L. M., Griffith, T. M., Nilsson, H., and Aalkjaer, C. (2006). Analysis of effects of connexin-mimetic peptides in rat mesenteric small arteries. Am. J. Physiol. Heart Circ. Physiol. 291, H357–H367. doi: 10.1152/ajpheart.00681.2005
Mathiesen, C., Brazhe, A., Thomsen, K., and Lauritzen, M. (2013). Spontaneous calcium waves in Bergman glia increase with age and hypoxia and may reduce tissue oxygen. J. Cereb. Blood Flow Metab. 33, 161–169. doi: 10.1038/jcbfm.2012.175
Mei, X., Ezan, P., Giaume, C., and Koulakoff, A. (2010). Astroglial connexin immunoreactivity is specifically altered at beta-amyloid plaques in beta-amyloid precursor protein/presenilin1 mice. Neuroscience 171, 92–105. doi: 10.1016/j.neuroscience.2010.08.001
Mlinar, B., and Enyeart, J. J. (1993). Block of current through T-type calcium channels by trivalent metal cations and nickel in neural rat and human cells. J. Physiol. 469, 639–652.
Morita, M., Saruta, C., Kozuka, N., Okubo, Y., Itakura, M., Takahashi, M., et al. (2007). Dual regulation of astrocyte gap junction hemichannels by growth factors and a pro-inflammatory cytokine via the mitogen-activated protein kinase cascade. Glia 55, 508–515. doi: 10.1002/glia.20471
Mulligan, S. J., and MacVicar, B. A. (2004). Calcium transients in astrocyte endfeet cause cerebrovascular constrictions. Nature 431, 195–199. doi: 10.1038/nature02827
Nagy, J. I., Li, W., Hertzberg, E. L., and Marotta, C. A. (1996). Elevated connexin43 immunoreactivity at sites of amyloid plaques in Alzheimer’s disease. Brain Res. 717, 173–178. doi: 10.1016/0006-8993(95)01526-4
Nakase, T., and Naus, C. C. (2004). Gap junctions and neurological disorders of the central nervous system. Biochim. Biophys. Acta 1662, 149–158. doi: 10.1016/j.bbamem.2004.01.009
Oh, S., Verselis, V. K., and Bargiello, T. A. (2008). Charges dispersed over the permeation pathway determine the charge selectivity and conductance of a Cx32 chimeric hemichannel. J. Physiol. 586, 2445–2461. doi: 10.1113/jphysiol.2008.150805
Oliet, S. H., and Mothet, J. P. (2006). Molecular determinants of D-serine-mediated gliotransmission: from release to function. Glia 54, 726–737. doi: 10.1002/glia.20356
Olk, S., Turchinovich, A., Grzendowski, M., Stühler, K., Meyer, H. E., Zoidl, G., et al. (2010). Proteomic analysis of astroglial connexin43 silencing uncovers a cytoskeletal platform involved in process formation and migration. Glia 58, 494–505. doi: 10.1002/glia.20942
Olson, J. E. (1999). Osmolyte contents of cultured astrocytes grown in hypoosmotic medium. Biochim. Biophys. Acta 1453, 175–179. doi: 10.1016/S0925-4439(98)00090-8
Orellana, J. A., Díaz, E., Schalper, K. A., Vargas, A. A., Bennett, M. V., and Sáez, J. C. (2011a). Cation permeation through connexin 43 hemichannels is cooperative, competitive and saturable with parameters depending on the permeant species. Biochem. Biophys. Res. Commun. 409, 603–609. doi: 10.1016/j.bbrc.2011.05.031
Orellana, J. A., Froger, N., Ezan, P., Jiang, J. X., Bennett, M. V., Naus, C. C., et al. (2011b). ATP and glutamate released via astroglial connexin 43 hemichannels mediate neuronal death through activation of pannexin 1 hemichannels. J. Neurochem. 118, 826–840. doi: 10.1111/j.1471-4159.2011.07210.x
Orellana, J. A., Shoji, K. F., Abudara, V., Ezan, P., Amigou, E., Sáez, P. J., et al. (2011c). Amyloid beta-induced death in neurons involves glial and neuronal hemichannels. J. Neurosci. 31, 4962–4977. doi: 10.1523/JNEUROSCI.6417-10.2011
Orellana, J. A., Hernández, D. E., Ezan, P., Velarde, V., Bennett, M. V., Giaume, C., et al. (2010). Hypoxia in high glucose followed by reoxygenation in normal glucose reduces the viability of cortical astrocytes through increased permeability of connexin 43 hemichannels. Glia 58, 329–343. doi: 10.1002/glia.20926
Orellana, J. A., Sáez, P. J., Cortes-Campos, C., Elizondo, R. J., Shoji, K. F., Contreras-Duarte, S., et al. (2012a). Glucose increases intracellular free Ca2+ in tanycytes via ATP released through connexin 43 hemichannels. Glia 60, 53–68. doi: 10.1002/glia.21246
Orellana, J. A., von Bernhardi, R., Giaume, C., and Sáez, J. C. (2012b). Glial hemichannels and their involvement in aging and neurodegenerative diseases. Rev. Neurosci. 23, 163–177. doi: 10.1515/revneuro-2011-0065
Orellana, J. A., Sáez, P. J., Shoji, K. F., Schalper, K. A., Palacios-Prado, N., Velarde, V., et al. (2009). Modulation of brain hemichannels and gap junction channels by pro-inflammatory agents and their possible role in neurodegeneration. Antioxid. Redox Signal. 11, 369–399. doi: 10.1089/ars.2008.2130
Panchin, Y., Kelmanson, I., Matz, M., Lukyanov, K., Usman, N., and Lukyanov, S. (2000). A ubiquitous family of putative gap junction molecules. Curr. Biol. 10, R473–R474. doi: 10.1016/S0960-9822(00)00576-5
Pannasch, U., Vargová, L., Reingruber, J., Ezan, P., Holcman, D., Giaume, C., et al. (2011). Astroglial networks scale synaptic activity and plasticity. Proc. Natl. Acad. Sci. U.S.A. 108, 8467–8472. doi: 10.1073/pnas.1016650108
Paul, D. L., Ebihara, L., Takemoto, L. J., Swenson, K. I., and Goodenough, D. A. (1991). Connexin46, a novel lens gap junction protein, induces voltage-gated currents in nonjunctional plasma membrane of Xenopus oocytes. J. Cell Biol. 115, 1077–1089. doi: 10.1083/jcb.115.4.1077
Pellerin, L., and Magistretti, P. J. (2012). Sweet sixteen for ANLS. J. Cereb. Blood Flow Metab. 32, 1152–1166. doi: 10.1038/jcbfm.2011.149
Penuela, S., Gehi, R., and Laird, D. W. (2013). The biochemistry and function of pannexin channels. Biochim. Biophys. Acta 1828, 15–22. doi: 10.1016/j.bbamem.2012.01.017
Perez Velazquez, J. L., Frantseva, M. V., and Naus, C. C. (2003). Gap junctions and neuronal injury: protectants or executioners? Neuroscientist 9, 5–9. doi: 10.1177/1073858402239586
Pfenniger, A., Wohlwend, A., and Kwak, B. R. (2011). Mutations in connexin genes and disease. Eur. J. Clin. Invest. 41, 103–116. doi: 10.1111/j.1365-2362.2010.02378.x
Pimplikar, S. W. (2009). Reassessing the amyloid cascade hypothesis of Alzheimer’s disease. Int. J. Biochem. Cell Biol. 41, 1261–1268. doi: 10.1016/j.biocel.2008.12.015
Ponsaerts, R., De Vuyst, E., Retamal, M., D’Hondt, C., Vermeire, D., Wang, N., et al. (2010). Intramolecular loop/tail interactions are essential for connexin 43-hemichannel activity. FASEB J. 24, 4378–4395. doi: 10.1096/fj.09-153007
Prochnow, N., Abdulazim, A., Kurtenbach, S., Wildforster, V., Dvoriantchikova, G., Hanske, J., et al. (2012). Pannexin1 stabilizes synaptic plasticity and is needed for learning. PLoS ONE 7:e51767. doi: 10.1371/journal.pone.0051767
Rackauskas, M., Neverauskas, V., and Skeberdis, V. A. (2010). Diversity and properties of connexin gap junction channels. Medicina (Kaunas) 46, 1–12.
Ramachandran, S., Xie, L. H., John, S. A., Subramaniam, S., and Lal, R. (2007). A novel role for connexin hemichannel in oxidative stress and smoking-induced cell injury. PLoS ONE 2:e712. doi: 10.1371/journal.pone.0000712
Rana, S., and Dringen, R. (2007). Gap junction hemichannel-mediated release of glutathione from cultured rat astrocytes. Neurosci. Lett. 415, 45–48. doi: 10.1016/j.neulet.2006.12.043
Retamal, M. A., Froger, N., Palacios-Prado, N., Ezan, P., Sáez, P. J., Sáez, J. C., et al. (2007a). Cx43 hemichannels and gap junction channels in astrocytes are regulated oppositely by proinflammatory cytokines released from activated microglia. J. Neurosci. 27, 13781–13792. doi: 10.1523/JNEUROSCI.2042-07.2007
Retamal, M. A., Schalper, K. A., Shoji, K. F., Bennett, M. V., and Sáez, J. C. (2007b). Opening of connexin 43 hemichannels is increased by lowering intracellular redox potential. Proc. Natl. Acad. Sci. U.S.A. 104, 8322–8327. doi: 10.1073/pnas.0702456104
Reyes, J. P., Hernandez-Carballo, C. Y., Pérez-Flores, G., Pérez-Cornejo, P., and Arreola, J. (2009). Lack of coupling between membrane stretching and pannexin-1 hemichannels. Biochem. Biophys. Res. Commun. 380, 50–53. doi: 10.1016/j.bbrc.2009.01.021
Rhee, S. K., Bevans, C. G., and Harris, A. L. (1996). Channel-forming activity of immunoaffinity-purified connexin32 in single phospholipid membranes. Biochemistry 35, 9212–9223. doi: 10.1021/bi960295m
Ripps, H., Qian, H., and Zakevicius, J. (2004). Properties of connexin26 hemichannels expressed in Xenopus oocytes. Cell. Mol. Neurobiol. 24, 647–665. doi: 10.1023/B:CEMN.0000036403.43484.3d
Riquelme, M. A., Kar, R., Gu, S., and Jiang, J. X. (2013). Antibodies targeting extracellular domain of connexins for studies of hemichannels. Neuropharmacology. doi: 10.1016/j.neuropharm.2013.02.021 [Epub ahead of print]
Rouach, N., Avignone, E., Meme, W., Koulakoff, A., Venance, L., Blomstrand, F., et al. (2002). Gap junctions and connexin expression in the normal and pathological central nervous system. Biol. Cell 94, 457–475. doi: 10.1016/S0248-4900(02)00016-3
Rouach, N., Koulakoff, A., Abudara, V., Willecke, K., and Giaume, C. (2008). Astroglial metabolic networks sustain hippocampal synaptic transmission. Science 322, 1551–1555. doi: 10.1126/science.1164022
Roux, L., Benchenane, K., Rothstein, J. D., Bonvento, G., and Giaume, C. (2011). Plasticity of astroglial networks in olfactory glomeruli. Proc. Natl. Acad. Sci. U.S.A. 108, 18442–18446. doi: 10.1073/pnas.1107386108
Rusakov, D. A., and Fine, A. (2003). Extracellular Ca2+ depletion contributes to fast activity-dependent modulation of synaptic transmission in the brain. Neuron 37, 287–297. doi: 10.1016/S0896-6273(03)00025-4
Sáez, J. C., Retamal, M. A., Basilio, D., Bukauskas, F. F., and Bennett, M. V. (2005). Connexin-based gap junction hemichannels: gating mechanisms. Biochim. Biophys. Acta 1711, 215–224. doi: 10.1016/j.bbamem.2005.01.014
Sáez, J. C., Schalper, K. A., Retamal, M. A., Orellana, J. A., Shoji, K. F., and Bennett, M. V. (2010). Cell membrane permeabilization via connexin hemichannels in living and dying cells. Exp. Cell Res. 316, 2377–2389. doi: 10.1016/j.yexcr.2010.05.026
Sánchez, H. A., Mese, G., Srinivas, M., White, T. W., and Verselis, V. K. (2010). Differentially altered Ca2+ regulation and Ca2+ permeability in Cx26 hemichannels formed by the A40V and G45E mutations that cause keratitis ichthyosis deafness syndrome. J. Gen. Physiol. 136, 47–62. doi: 10.1085/jgp.201010433
Sánchez, H. A., Orellana, J. A., Verselis, V. K., and Sáez, J. C. (2009). Metabolic inhibition increases activity of connexin-32 hemichannels permeable to Ca2+ in transfected HeLa cells. Am. J. Physiol. Cell Physiol. 297, C665–C678. doi: 10.1152/ajpcell.00200.2009
Sánchez-Alvarez, R., Tabernero, A., and Medina, J. M. (2004). Endothelin-1 stimulates the translocation and upregulation of both glucose transporter and hexokinase in astrocytes: relationship with gap junctional communication. J. Neurochem. 89, 703–714. doi: 10.1046/j.1471-4159.2004.02398.x
Scemes, E. (2008). Modulation of astrocyte P2Y1 receptors by the carboxyl terminal domain of the gap junction protein Cx43. Glia 56, 145–153. doi: 10.1002/glia.20598
Scemes, E. (2012). Nature of plasmalemmal functional “hemichannels.” Biochim. Biophys. Acta 1818, 1880–1883. doi: 10.1016/j.bbamem.2011.06.005
Scemes, E., and Giaume, C. (2006). Astrocyte calcium waves: what they are and what they do. Glia 54, 716–725. doi: 10.1002/glia.20374
Scemes, E., and Spray, D. C. (2012). Extracellular K and astrocyte signaling via connexin and pannexin channels. Neurochem. Res. 37, 2310–2316. doi: 10.1007/s11064-012-0759-4
Scemes, E., Suadicani, S. O., Dahl, G., and Spray, D. C. (2007). Connexin and pannexin mediated cell–cell communication. Neuron Glia Biol. 3, 199–208. doi: 10.1017/S1740925X08000069
Schalper, K. A., Orellana, J. A., Berthoud, V. M., and Sáez, J. C. (2009). Dysfunctions of the diffusional membrane pathways mediated by hemichannels in inherited and acquired human diseases. Curr. Vasc. Pharmacol. 7, 486–505. doi: 10.2174/157016109789043937
Schalper, K. A., Palacios-Prado, N., Orellana, J. A., and Sáez, J. C. (2008a). Currently used methods for identification and characterization of hemichannels. Cell Commun. Adhes. 15, 207–218. doi: 10.1080/15419060802014198
Schalper, K. A., Palacios-Prado, N., Retamal, M. A., Shoji, K. F., Martínez, A. D., and Sáez, J. C. (2008b). Connexin hemichannel composition determines the FGF-1-induced membrane permeability and free [Ca2+]i responses. Mol. Biol. Cell 19, 3501–3513. doi: 10.1091/mbc.E07-12-1240
Schalper, K. A., Sanchez, H. A., Lee, S. C., Altenberg, G. A., Nathanson, M. H., and Sáez, J. C. (2010). Connexin 43 hemichannels mediate the Ca2+ influx induced by extracellular alkalinization. Am. J. Physiol. Cell Physiol. 299, C1504–C1515. doi: 10.1152/ajpcell.00015.2010
Schnell, L., Fearn, S., Klassen, H., Schwab, M. E., and Perry, V. H. (1999). Acute inflammatory responses to mechanical lesions in the CNS: differences between brain and spinal cord. Eur. J. Neurosci. 11, 3648–3658. doi: 10.1046/j.1460-9568.1999.00792.x
Seki, A., Duffy, H. S., Coombs, W., Spray, D. C., Taffet, S. M., and Delmar, M. (2004). Modifications in the biophysical properties of connexin43 channels by a peptide of the cytoplasmic loop region. Circ. Res. 95, e22–e28. doi: 10.1161/01.RES.0000140737.62245.c5
Siebert, A. P., Ma, Z., Grevet, J. D., Demuro, A., Parker, I., and Foskett, J. K. (2013). Structural and functional similarities of calcium homeostasis modulator 1 (CALHM1) ion channel with connexins, pannexins, and innexins. J. Biol. Chem. 288, 6140–6153. doi: 10.1074/jbc.M112.409789
Silverman, W. R., de Rivero Vaccari, J. P., Locovei, S., Qiu, F., Carlsson, S. K., Scemes, E., et al. (2009). The pannexin 1 channel activates the inflammasome in neurons and astrocytes. J. Biol. Chem. 284,18143–18151. doi: 10.1074/jbc.M109.004804
Simard, M., Arcuino, G., Takano, T., Liu, Q. S., and Nedergaard, M. (2003). Signaling at the gliovascular interface. J. Neurosci. 23, 9254–9262.
Sofroniew, M. V., and Vinters, H. V. (2010). Astrocytes: biology and pathology. Acta Neuropathol. 119, 7–35. doi: 10.1007/s00401-009-0619-8
Söhl, G., Maxeiner, S., and Willecke, K. (2005). Expression and functions of neuronal gap junctions. Nat. Rev. Neurosci. 6, 191–200. doi: 10.1038/nrn1627
Sohl, G., and Willecke, K. (2004). Gap junctions and the connexin protein family. Cardiovasc. Res. 62, 228–232. doi: 10.1016/j.cardiores.2003.11.013
Somjen, G. G. (2002). Ion regulation in the brain: implications for pathophysiology. Neuroscientist 8, 254–267. doi: 10.1177/1073858402008003011
Song, E. K., Rah, S. Y., Lee, Y. R., Yoo, C. H., Kim, Y. R., Yeom, J. H., et al. (2011). Connexin-43 hemichannels mediate cyclic ADP-ribose generation and its Ca2+-mobilizing activity by NAD+/cyclic ADP-ribose transport. J. Biol. Chem. 286, 44480–44490. doi: 10.1074/ jbc.M111.307645
Stehberg, J., Moraga-Amaro, R., Salazar, C., Becerra, A., Echeverría, C., Orellana, J. A., et al. (2012). Release of gliotransmitters through astroglial connexin 43 hemichannels is necessary for fear memory consolidation in the basolateral amygdala. FASEB J. 26, 3649–3657. doi: 10.1096/fj.11-198416
Stout, C. E., Costantin, J. L., Naus, C. C., and Charles, A. C. (2002). Intercellular calcium signaling in astrocytes via ATP release through connexin hemichannels. J. Biol. Chem. 277, 10482–10488. doi: 10.1074/jbc.M109902200
Suadicani, S. O., Brosnan, C. F., and Scemes, E. (2006). P2X7 receptors mediate ATP release and amplification of astrocytic intercellular Ca2+ signaling. J. Neurosci. 26, 1378–1385. doi: 10.1523/JNEUROSCI.3902-05.2006
Suadicani, S. O., Flores, C. E., Urban-Maldonado, M., Beelitz, M., and Scemes, E. (2004). Gap junction channels coordinate the propagation of intercellular Ca2+ signals generated by P2Y receptor activation. Glia 48, 217–229. doi: 10.1002/glia.20071
Suadicani, S. O., Iglesias, R., Wang, J., Dahl, G., Spray, D. C., and Scemes, E. (2012). ATP signaling is deficient in cultured Pannexin1-null mouse astrocytes. Glia 60, 1106–1116. doi: 10.1002/glia.22338
Swayne, L. A., Sorbara, C. D., and Bennett, S. A. (2010). Pannexin 2 is expressed by postnatal hippocampal neural progenitors and modulates neuronal commitment. J. Biol. Chem. 285, 24977–24986. doi: 10.1074/jbc.M110.130054
Tabernero, A., Giaume, C., and Medina, J. M. (1996). Endothelin-1 regulates glucose utilization in cultured astrocytes by controlling intercellular communication through gap junctions. Glia 16, 187–195. doi: 10.1002/(SICI)1098-1136(199603)16:3
Tabernero, A., Medina, J. M., and Giaume, C. (2006). Glucose metabolism and proliferation in glia: role of astrocytic gap junctions. J. Neurochem. 99, 1049–1061. doi: 10.1111/j.1471-4159.2006.04088.x
Takeuchi, H., Jin, S., Wang, J., Zhang, G., Kawanokuchi, J., Kuno, R., et al. (2006). Tumor necrosis factor-alpha induces neurotoxicity via glutamate release from hemichannels of activated microglia in an autocrine manner. J. Biol. Chem. 281, 21362–21368. doi: 10.1074/jbc.M600504200
Taruno, A., Vingtdeux, V., Ohmoto, M., Ma, Z., Dvoryanchikov, G., Li, A., et al. (2013). CALHM1 ion channel mediates purinergic neurotransmission of sweet, bitter and umami tastes. Nature 495, 223–226. doi: 10.1038/nature11906
Theis, M., Söhl, G., Eiberger, J., and Willecke, K. (2005). Emerging complexities in identity and function of glial connexins. Trends Neurosci. 28, 188–195. doi: 10.1016/j.tins.2005.02.006
Thompson, R. J., and Macvicar, B. A. (2008). Connexin and pannexin hemichannels of neurons and astrocytes. Channels (Austin) 2, 81–86. doi: 10.4161/chan.2.2.6003
Torres, A., Wang, F., Xu, Q., Fujita, T., Dobrowolski, R., Willecke, K., et al. (2012). Extracellular Ca2+ acts as a mediator of communication from neurons to glia. Sci. Signal. 5, ra8. doi: 10.1126/scisignal.2002160
Valiunas, V. (2002). Biophysical properties of connexin-45 gap junction hemichannels studied in vertebrate cells. J. Gen. Physiol. 119, 147–164. doi: 10.1085/jgp.119.2.147
Valiunas, V., and Weingart, R. (2000). Electrical properties of gap junction hemichannels identified in transfected HeLa cells. Pflügers Arch. 440, 366–379. doi: 10.1007/s004240000294
Vanden Abeele, F., Bidaux, G., Gordienko, D., Beck, B., Panchin, Y. V., Baranova, A. V., et al. (2006). Functional implications of calcium permeability of the channel formed by pannexin 1. J. Cell Biol. 174, 535–546. doi: 10.1083/jcb.200601115
Venance, L., Piomelli, D., Glowinski, J., and Giaume, C. (1995). Inhibition by anandamide of gap junctions and intercellular calcium signalling in striatal astrocytes. Nature 376, 590–594. doi: 10.1038/376590a0
Vinken, M., Decrock, E., Leybaert, L., Bultynck, G., Himpens, B., Vanhaecke, T., et al. (2012). Non-channel functions of connexins in cell growth and cell death. Biochim. Biophys. Acta 1818, 2002–2008. doi: 10.1016/j.bbamem.2011.06.011
Vogt, A., Hormuzdi, S. G., and Monyer, H. (2005). Pannexin1 and Pannexin2 expression in the developing and mature rat brain. Brain Res. Mol. Brain Res. 141, 113–120. doi: 10.1016/j.molbrainres.2005.08.002
Wang, J., Ma, M., Locovei, S., Keane, R. W., and Dahl, G. (2007). Modulation of membrane channel currents by gap junction protein mimetic peptides: size matters. Am. J. Physiol. Cell Physiol. 293, C1112–C1119. doi: 10.1152/ajpcell.00097.2007
Wang, N., De Bock, M., Antoons, G., Gadicherla, A. K., Bol, M., Decrock, E., et al. (2012). Connexin mimetic peptides inhibit Cx43 hemichannel opening triggered by voltage and intracellular Ca2+ elevation. Basic Res. Cardiol. 107, 304. doi: 10.1007/s00395-012-0304-2
Wang, N., De Vuyst, E., Ponsaerts, R., Boengler, K., Palacios-Prado, N., Wauman, J., et al. (2013). Selective inhibition of Cx43 hemichannels by Gap19 and its impact on myocardial ischemia/reperfusion injury. Basic Res. Cardiol. 108, 309. doi: 10.1007/s00395-012-0309-x
Warner, A., Clements, D. K., Parikh, S., Evans, W. H., and DeHaan, R. L. (1995). Specific motifs in the external loops of connexin proteins can determine gap junction formation between chick heart myocytes. J. Physiol. 488 (Pt 3), 721–728.
Wasseff, S. K., and Scherer, S. S. (2011). Cx32 and Cx47 mediate oligodendrocyte:astrocyte and oligodendrocyte:oligodendrocyte gap junction coupling. Neurobiol. Dis. 42, 506–513. doi: 10.1016/j.nbd.2011.03.003
Wiencken-Barger, A. E., Djukic, B., Casper, K. B., and McCarthy, K. D. (2007). A role for Connexin43 during neurodevelopment. Glia 55, 675–686. doi: 10.1002/glia.20484
Willecke, K., Eiberger, J., Degen, J., Eckardt, D., Romualdi, A., Guldenagel, M., et al. (2002). Structural and functional diversity of connexin genes in the mouse and human genome. Biol. Chem. 383, 725–737. doi: 10.1515/BC.2002.076
Xiong, J., Burkovetskaya, M., Karpuk, N., and Kielian, T. (2012). IL-1RI (interleukin-1 receptor type I) signalling is essential for host defence and hemichannel activity during acute central nervous system bacterial infection. ASN Neuro 4, pii: e00085. doi: 10.1042/AN20120008.
Xu, H. L., and Pelligrino, D. A. (2007). ATP release and hydrolysis contribute to rat pial arteriolar dilatation elicited by neuronal activation. Exp. Physiol. 92, 647–651. doi: 10.1113/expphysiol.2006.036863
Yamamoto, T., Ochalski, A., Hertzberg, E. L., and Nagy, J. I. (1990). On the organization of astrocytic gap junctions in rat brain as suggested by LM and EM immunohistochemistry of connexin43 expression. J. Comp. Neurol. 302, 853–883. doi: 10.1002/cne.903020414
Ye, Z. C., Wyeth, M. S., Baltan-Tekkok, S., and Ransom, B. R. (2003). Functional hemichannels in astrocytes: a novel mechanism of glutamate release. J. Neurosci. 23, 3588–3596.
Yu, J., Bippes, C. A., Hand, G. M., Muller, D. J., and Sosinsky, G. E. (2007). Aminosulfonate modulated pH-induced conformational changes in connexin26 hemichannels. J. Biol. Chem. 282, 8895–8904. doi: 10.1074/jbc.M609317200
Zappala, A., Li Volti, G., Serapide, M. F., Pellitteri, R., Falchi, M., et al. (2007). Expression of pannexin2 protein in healthy and ischemized brain of adult rats. Neuroscience 148, 653–667. doi: 10.1016/j.neuroscience.2007.06.028
Zhan, H., Moore, C. S., Chen, B., Zhou, X., Ma, X. M., Ijichi, K., et al. (2012). Stomatin inhibits pannexin-1-mediated whole-cell currents by interacting with its carboxyl terminal. PLoS ONE 7:e39489. doi: 10.1371/journal.pone.0039489
Keywords: astrocytes, oligodendrocytes, microglia, gap junctions, neuroglial interactions
Citation: Giaume C, Leybaert L, Naus CC and Sáez JC (2013) Connexin and pannexin hemichannels in brain glial cells: properties, pharmacology, and roles. Front. Pharmacol. 4:88. doi: 10.3389/fphar.2013.00088
Received: 23 April 2013; Paper pending published: 21 May 2013;
Accepted: 21 June 2013; Published online: 17 July 2013.
Edited by:
Aida Salameh, Heart Centre University of Leipzig, GermanyReviewed by:
Gregory E. Morley, New York University School of Medicine, USARoger J. Thompson, University of Calgary, Canada
Panagiotis Bargiotas, University of Heidelberg, Germany
Copyright: © 2013 Giaume, Leybaert, Naus and Sáez. This is an open-access article distributed under the terms of the Creative Commons Attribution License, which permits use, distribution and reproduction in other forums, provided the original authors and source are credited and subject to any copyright notices concerning any third-party graphics etc.
*Correspondence: Christian Giaume, Collège de France, Center for Interdisciplinary Research in Biology/Centre National de la Recherche Scientifique, Unité Mixte de Recherche 7241/Institut National de la Santé et de la Recherche Médicale U1050, 11 Place Marcelin Berthelot, 75231 Paris Cedex 05, France e-mail: christian.giaume@college-de-france.fr