- 1 InViTe Research Institute, Albstadt-Sigmaringen University, Sigmaringen, Germany
- 2 Brighton and Sussex Medical School, Brighton, UK
The field of automated patch-clamp electrophysiology has emerged from the tension between the pharmaceutical industry’s need for high-throughput compound screening versus its need to be conservative due to regulatory requirements. On the one hand, hERG channel screening was increasingly requested for new chemical entities, as the correlation between blockade of the ion channel coded by hERG and torsades de pointes cardiac arrhythmia gained increasing attention. On the other hand, manual patch-clamping, typically quoted as the “gold-standard” for understanding ion channel function and modulation, was far too slow (and, consequently, too expensive) for keeping pace with the numbers of compounds submitted for hERG channel investigations from pharmaceutical R&D departments. In consequence it became more common for some pharmaceutical companies to outsource safety pharmacological investigations, with a focus on hERG channel interactions. This outsourcing has allowed those pharmaceutical companies to build up operational flexibility and greater independence from internal resources, and allowed them to obtain access to the latest technological developments that emerged in automated patch-clamp electrophysiology – much of which arose in specialized biotech companies. Assays for nearly all major cardiac ion channels are now available by automated patch-clamping using heterologous expression systems, and recently, automated action potential recordings from stem-cell derived cardiomyocytes have been demonstrated. Today, most of the large pharmaceutical companies have acquired automated electrophysiology robots and have established various automated cardiac ion channel safety screening assays on these, in addition to outsourcing parts of their needs for safety screening.
Ion Channel Activity Determines ECG-Detectable Cardiovascular Risk
The coordinated electrical activity of the heart determines its ability to beat and pump oxygenated blood successfully, and the overall electrical activity is determined by the cellular action potentials (APs) at the level of the cardiomyocyte. Ion channels mediate transmembrane currents controlling the duration of cardiomyocyte APs, which en masse can be detected at the skin via the electrocardiogram (ECG; Sanguinetti and Tristani-Firouzi, 2006; Witchel, 2011). The QT interval, which is measured as an in vivo ECG parameter, has been shown to be an imperfect surrogate marker for arrhythmogenic risk (Roden et al., 1996; Witchel et al., 2003; Hondeghem, 2006). If prolonged in the presence of a drug, it may indicate that a compound could cause acquired long QT syndrome (aLQTS), and thus have a cardiac safety liability (Roden, 2004). aLQTS is a potentially fatal drug-induced disorder associated with the risk of sudden cardiac death mediated by the polymorphic ventricular tachyarrhythmia torsades de pointes (TdP; Viskin, 1999). In many cases the pathophysiological basis of aLQTS is based on a drug (or combination of drugs) interfering with the activity or production of either one or a range of ion channels; thus, the testing of drugs for effects on ion channels has become a major concern (and industry) within drug development (Brown, 2008). For drug development programs that must establish cardiac safety profiles to achieve regulatory approval, the QT interval, therefore, remains one of the most important ECG parameters.
Control of the duration of the cardiac ventricular AP is mediated by the equilibrium between inward and outward currents across the cell membrane (Carmeliet, 1993). Under physiological conditions the AP progresses through the phases of depolarization, plateau phase, and repolarization over the course of 200–300 ms, but in LQTS repolarization is delayed and the AP duration at times will be substantially prolonged. Electrophysiologically, this delay in repolarization implies a deviation from the usual balance of currents across the myocyte membrane responsible for normal repolarization. Thus, prolongation of the AP could theoretically arise from an increase in inward (depolarizing) current, or alternatively, from a decrease in outward (repolarizing) current carried by potassium ions. Both of these conditions have been found to exist as the genetic basis of pathology in various familial LQTS patients (Splawski et al., 2000).
Potassium channels play prominent roles in AP repolarization, as well as during the plateau phase. The rate of net K+ efflux, and by extension the rate of repolarization, is determined by the density and gating properties of different K+ channels. K+ channels mediating an inwardly rectifying current (IK1) are important in maintaining the normal resting potential of ventricular cardiomyocytes, and during the final stage of AP repolarization (Carmeliet, 1993). A rapidly activating and inactivating transient outward current (Ito) contributes to early AP repolarization and underlies the initial “notch” before the plateau phase. Of particular relevance to the AP plateau is the delayed rectifier current (IK) comprised of rapid (IKr) and slow (IKs) components mediated by distinct channel subtypes with distinct kinetic properties (Sanguinetti and Jurkiewicz, 1990; Sanguinetti and Keating, 1997). IK develops gradually during the plateau phase, opposing the inward currents underlying sustained depolarization. As the net balance of current alters, and net outward current exceeds inward current, repolarization occurs. IK and IK1 can be considered to regulate ventricular AP repolarization over the plateau range, and final rapid repolarization phase, respectively (Sanguinetti and Keating, 1997). A schematic showing the ventricular AP and the currents that comprise it are shown in Figure 1.
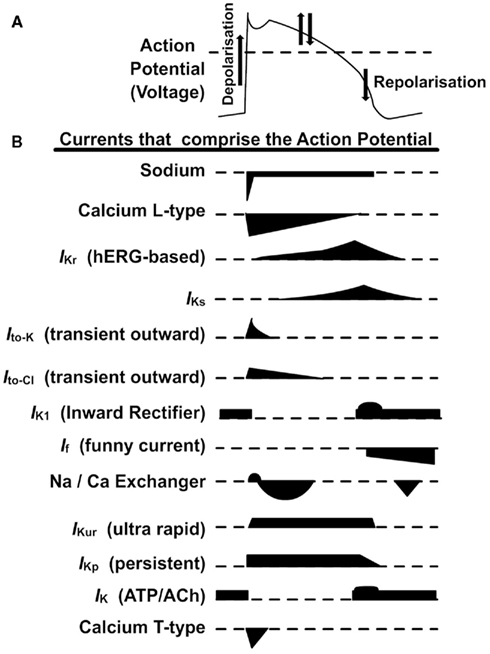
Figure 1. Ionic activity during the cardiac ventricular action potential. (A) An idealized ventricular action potential (AP) is shown in the top panel; the upward slope represents depolarization (positive voltages), and the downward slope repolarization (negative voltages). Arrows indicate the effects of the dominant currents on the potential: during the plateau phase, depolarizing calcium currents, and repolarizing potassium currents balance each other. (B) The main transmembrane ionic currents contributing to the AP over time; inward (depolarizing) currents are shown below each axis, and outward (repolarizing) currents are shown above each axis. Relative polarities and time courses are indicated; relative amplitudes are not to scale. IKr Rapid delayed rectifier current. Adapted with permission from Witchel (2011).
The cellular electrophysiological testing of drugs for cardiac safety tends to involve cardiac potassium channels, the cardiac sodium channel and the L-type calcium channel, because the association of these channels with LQTS is well-established. The protein comprising the pore of IKr is made from four hERG1a alpha subunits, and when these are expressed heterologously, the channel mediating the resulting transmembrane current is commonly referred to as the hERG channel. The hERG channel is the most commonly tested ion channel for cardiac liability of new drugs. Other ion channels that are relevant to the cardiac AP (Figure 1) are also tested commercially, including IKs (the slow component of the delayed rectifier current in cardiac myocytes), IK1 (the inward component), IKur (the ultra rapid component), IKto (the transient outward component), and If (the “funny current”). Sympathetic influences also affect arrhythmogenic risk, and β-adrenergic effects (using GPCR-screening methods) can thus also be tested for.
The most notorious ion channel associated with an aLQTS liability is the hERG (originally named human Ether-à-go-go related gene, also now known as Kv11.1) potassium (K+) channel; the reason so many drugs were linked to aLQTS mediated by hERG is presumed to be due to the structure of the channel’s inner cavity, which allows the channel to make promiscuous interactions with many different small molecules (Mitcheson, 2008). Mutations in this alpha subunit were initially shown to be the cause of genetic long QT syndrome, and it was proposed that iatrogenic inhibition of the channel could lead to pathology by failing to protect against calcium-induced early after depolarizations (EADs) that could initiate arrhythmogenic electrical activity (January and Moscucci, 1992; Curran et al., 1995; Sanguinetti et al., 1995; Sanguinetti and Keating, 1997).
For many years the only test of drug-induced channel blockade that was viewed as critical for cardiac safety by drug regulators was for hERG (Committee for Proprietary Medicinal Products, 1997), despite the fact that an ensemble of currents contributes to the AP (and the ECG). It is generally understood that changing the activity of ion channels on the cell surface of cardiac myocytes can both contribute to arrhythmogenesis and to its amelioration (Bril et al., 1996; ICH, 2005; Hansen et al., 2007), and that although blockade of hERG may increase the substrate for arrhythmogenesis, that concurrent blocking of L-type calcium channels may serve to reduce EADs and the arrhythmogenic substrate (January and Riddle, 1989; Bril et al., 1998; Chouabe et al., 1998). A number of investigators pointed out that extrapolating the net drug-induced effects on a range of channels based on measuring only one type of current can give false picture (Witchel et al., 2003). The pharmaceutical industry is therefore concerned with developing in vitro cardiac safety assays that provide an early measure of cardiac safety, integrating more than only the hERG ion channel current (Moeller, 2011).
Automated Patch-Clamp Electrophysiology Alleviates a Bottleneck in Cardiac Ion Channel Safety Screening
Automated Patch-Clamp Electrophysiology Provides a Direct Biophysical Readout of Ion Channel Function
The flux of ions through transmembrane channels is accompanied by a charge transfer across the biological membrane. This charge transfer can be measured by biophysical readouts, and the manual patch-clamp technique (Neher and Sakmann, 1976; Hamill et al., 1981) is typically referred to as the “gold-standard” method for achieving this. Attempts to automate and simplify the classical, relatively slow, and technically challenging patch-pipette-based approach unfolded at NeuroSearch in the late 1990s with the development of NeuroPatch, later introduced as Apatchi-1 (Asmild et al., 2003). Subsequently, a few other systems have been developed that automate the classical method of pipette-based patch-clamping; however, none of these appear to have yielded a major revolution in patch-clamp throughput.
The technical realization that cells could form high-resistance (gigaseal) connections to planar glass substrates allowed for the introduction of chip-based systems for automated patch-clamp electrophysiology. This finding, approximately 10 years ago (Fertig et al., 2001, 2002; Sigworth and Klemic, 2002), has allowed scientists to increase the throughput of automated patch-clamping to the next level, and has started a technological revolution that alleviated the major bottleneck of throughput in ion channel patch-clamp research. A couple of biotech startup companies were launched and then contributed to bringing this (or a closely related) new technology to the market [including Nanion Technologies GmbH, Munich; Cytocentrics AG, Rostock; Flyion GmbH, Tübingen; Essen Instruments (now Essen BioScience, Inc.), Cytion SA (Lausanne, acquired by Molecular Devices, LLC); Cellectricon AB (Mölndal); Sophion A/S (Copenhagen); Fluxion LLC (San Francisco)], and the technology was also a part of development activities in established companies [especially Axon Instruments, now part of Molecular Devices, LLC (MDS)].
The Requirement for hERG Channel Data Fostered the Development of Automated Patch-Clamp Robots
Around the beginning of this century, awareness for the relevance of a blockade of the cardiac hERG ion channel for the development of drug-induced cardiac arrhythmias arose and was reflected in regulatory guidelines (ICH S7A, ICH S7B). Consequently the pharmaceutical industry was in huge need of hERG channel safety screening data of new drug candidates. A couple of small and medium-sized university spin-offs and biotech companies started to offer this kind of investigations for pharmaceutical companies, by employing different technologies: high-throughput assays (e.g., Fluorescence, binding, or flux assays: these represent an indirect readout, but are relatively cheap and offer a relatively high throughput), or manual patch-clamp assays (with only low compound throughput, and consequently at relatively high costs per data point). Some of these companies presented a showcase for a time-matched introduction of a scientifically sound and highly relevant expert service, which the pharmaceutical industry was in dire need of, and which in some cases developed into a great commercial success. With increasing demand for hERG channel data, it is not surprising that the hERG ion channel was one of the first channels for which an assay was established on the newly developed automated patch-clamp instruments (Kiss et al., 2003), and in turn the need for high-throughput hERG channel patch-clamp data was a major accelerator in the further advancement of automated patch-clamp robots.
Protocols for Automated Patch-Clamp Assays Need to Be Carefully Set Up and Validated
Patch-clamp robots available today typically employ planar substrates on which cells delivered from suspension can form gigaseals (for an excellent review on automated patch-clamp instruments, see Dunlop et al., 2008). Protocols and details for the investigation of a number of different cell types by automated patch-clamp are given in the excellent paper by Milligan et al. (2009). Since its first application to measuring the hERG channel for safety screening (Kiss et al., 2003), this technique has been well validated for a number of robots. It is now widely applied to reduce the liability of compound series’ towards the hERG channel as part of safety pharmacology assessment early during medicinal chemistry compound development (Kutchinsky et al., 2003; Davenport et al., 2010; Riether et al., 2011; Tye et al., 2011). Reported correlations of biophysical channel properties (Figure 2) as well as pharmacological compound properties between automated (planar, chip-based) and manual (pipette-based) patch-clamp investigations are generally excellent (Kutchinsky et al., 2003; Xu et al., 2003; Tao et al., 2004; Bridgland-Taylor et al., 2006; Jones et al., 2009; Davenport et al., 2010; Golden et al., 2011). The reported discrepancies between manual and automated patch-clamping have been well investigated, and a number of reasons for reported discrepancies have been identified. It is important to note that all of these reported discrepancies are not attributed to the technological principle of chip-based patch-clamping. Rather, one or several of the following points have been identified as the major source of potential discrepancies (Mathes, 2006; Ly et al., 2007; Farre et al., 2009; Mo et al., 2009; Davenport et al., 2010; Moller and Slack, 2010) if automated patch-clamp assays are not well validated: (1) Plate material in automated robots: in manual patch-clamping, test solutions are typically prepared in relatively large quantities and are continuously perfused, while in automated robots, microtiter plates with a smaller volume are used, and relatively small volumes of test solutions are added (one or several times) to the patched cells. The unfavorable surface-to-volume ratio in microtiter plates can lead to a potential reduction of compound concentrations in automated patch-clamping, especially if a hydrophobic plate material is employed. This would lead to a shift of IC50 curves to larger nominal compound concentrations. It has therefore become good practice to use glass plates in automated electrophysiology robots, in particular for all aqueous solutions. In this context it is important to also consider the material of the patch-clamp chip. (2) Timing of compound preparation: in manual patch-clamp experiments, it is relatively easy to prepare test solutions directly before the patch-clamp experiment. This time-matched preparation of test solutions can become a challenge in automated experiments, due to the higher throughput, and the corresponding requirement of a larger number of different test solutions. A time lag between the preparation of test solutions and the experiment can however result in a precipitation of compounds in test solution over time, resulting in IC50 curves shifted to larger nominal compound concentrations. (3) Buffer compositions: the ionic strength of buffers can shift pharmacological profiles of compounds (e.g., different ions can have an effect on channel gating, and certain compounds interact preferably with certain states of the channel). Consequently, it appears advisable to use buffers that mimic physiological conditions as closely as possible. (4) It is important to note that also manually measured compound IC50 values often span a relatively large range between different labs, often owing to different voltage protocols that are applied, or to different experimental temperatures.
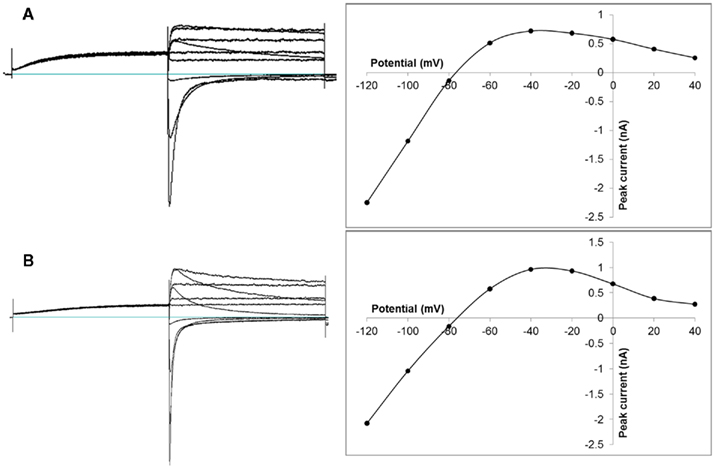
Figure 2. Representative hERG channel whole-cell current–voltage relationships by manual and automated patch-clamp electrophysiology from CHO cells stably expressing the channel. The characteristic bell-shaped current–voltage relation can be clearly seen during both partial repolarization phases [different current–voltage protocols for recording hERG channel currents have been described in the literature (e.g., Hancox et al., 1998; Su et al., 2004, 2009; Deisemann et al., 2008)]. (A) Recorded by manual patch-clamp using the following stimulation protocol: cells were held at −80 mV, depolarized to +20 mV for 1 s, and repolarized in steps from −120 to +40 mV in 10 mV intervals for 1 s each. Stimulation frequency was 0.1 Hz. (B) Recorded by automated planar patch-clamp on the Patchliner (Nanion Technologies) using the following stimulation protocol: cells were held at −80 mV, depolarized to +40 mV for 500 ms, and repolarized in steps from −140 to +40 mV in 20 mV intervals for 500 ms each. Stimulation frequency was 0.1 Hz. Adapted with permission from Moeller (2010). For more experimental details see Davenport et al. (2010).
Technological developments have further increased the throughput of automated patch-clamp robots during the past years. This has happened on the one hand by increasing parallelization of measurement electrodes, thereby allowing the execution of more patch-clamp recordings in parallel in one experimental run. In a different approach, it has been shown that an increased experiment success rate could be reached if seals with reduced resistance are included into the data evaluation. This notion has allowed reducing the technological demands for controlled seal formation, and has thereby facilitated an even higher rate of parallelization, albeit at potentially reduced data quality owing to the lack of high-resistance gigaseals. Interestingly, the development of ensemble patch-recordings (Finkel et al., 2006), as used in various of today’s instruments, averages out biological variability of individual cells, and thereby increases data consistency and reproducibility even in low-resistance experiments for most cell systems and ion channels (Finkel et al., 2006; Cao et al., 2010).
Automated Patch-Clamp Assays Go Beyond hERG
Most of the relevant cardiac ion channel safety targets that are discussed above have been shown to be amenable to measurements on different electrophysiological platforms in heterologous expression systems (Wible et al., 2008; Balasubramanian et al., 2009; Farre et al., 2009; Cao et al., 2010; Penniman et al., 2010), and form a standard resource within safety departments of many pharmaceutical companies. In addition, a number of competitive specialized Biotech Contract Research Organizations (CRO) are offering investigations of cardiac (and other) ion channels using well validated automated patch-clamp electrophysiology assays under fee-for-service or alliance contract agreements (e.g., Chantest, OH, USA; Millipore, MA, USA (now part of Merck KGaA); AvivaBioSciences, California; Evotec AG, Hamburg). Thereby these companies are offering an established flexible resource to meet outsourcing requirements of the pharmaceutical and the biotech industry.
It has been described that some compounds are more potent hERG-inhibitors at physiological temperature as compared to room temperature: measurements at the (higher) physiological temperature might affect the IC50s for the many open-state hERG channel blockers (e.g., methanesulfonanilides), as the faster kinetics at the higher temperature would make more of the channel available for open-state blockade (Zhou et al., 1998). This is especially relevant for safety investigations, where compounds could be incorrectly classified as non-hERG active if they are investigated at room temperature. It has therefore become good practice to perform hERG investigations (especially the relatively late-stage GLP) at (or near) physiological temperature. While the first automated patch-clamp instruments were only capable of operating at room temperature, some manufacturers of automated patch-clamp equipment have responded to this need for automated patch-clamp investigations at (or near) physiological temperature (Farre et al., 2007; Golden et al., 2011).
Automated Patch-Clamping of Cardiomyocytes Provides Physiologically Relevant Action Potential Data
As discussed above, the cardiac AP is comprised of a well-orchestrated ensemble of individual ion channel currents. Considering only selected ion channels for cardiac risk assessment can therefore give a false picture, as effects of compounds on several channels can potentiate or alleviate a potential arrhythmic effect (Witchel et al., 2003; Fenichel et al., 2004; Moeller, 2011). Patch-clamp recordings in cardiomyocytes have been shown to provide a picture of the effect of compounds on the whole ensemble of cardiac ion channel currents, and to reproduce the effects of selected reference compounds on the AP. With improved protocols to differentiate stem-cells of various sources into a homogenous cardiomyocyte population, the availability of cardiomyocytes increases, and the suitability of stem-cell derived cardiomyocytes for pharmacological investigations by patch-clamping has been convincingly demonstrated (Harding et al., 2007; Zhang et al., 2009; Kuryshev et al., 2010; Foldes et al., 2011). Recently, stem-cell derived cardiomyocytes from mouse have been applied to planar patch-clamp chips, and the pharmacology of a set of reference compounds was reproduced in AP recordings (Stoelzle et al., 2011). Also, data from human iPS-derived cardiomyocytes were shown (Anson, 2011). This proves the potential of such recordings and certainly represents an extremely interesting and physiologically potentially highly relevant approach. It should however be considered that (automated) AP recordings from myocytes still require a more thorough validation before their sensitivity and the correlation of the data to adult cardiac physiology and pharmacology is better understood. In particular two potential problems with data from stem-cell derived cardiomyocytes need to be considered: (1) Studies have given evidence that iPS and ES derived cardiomyocytes, depending on their age, most likely represent a neonatal state, and thus contain an ion channel population that most likely does not represent the adult one (Binah et al., 2007; Xi et al., 2010). This would certainly also influence the AP response and pharmacology. (2) While stem-cell derived cardiac myocytes certainly represent a more physiological test system than heterologous expression systems, it must be considered that in automated patch-clamp setups the cells reside out of their physiological surrounding, in (more or less) artificial buffer solutions. Also, the cells have lost their cell–cell network contacts, and therefore require an artificial stimulation to activate their AP.
Automated Cardiac Safety Ion Channel Patch-Clamp Electrophysiology: Quo Vadis?
The automated patch-clamp electrophysiology technology has been referred to as an “enabling technology” for ion channel drug discovery and research. Never before has it been practically possible for pharmaceutical companies to screen compounds on the order of tens of thousands against an ion channel target by use of a direct functional readout, and thus avoiding the potential false positives and/or negatives associated to other, more indirect (such as fluorescence assay or binding techniques), high-throughput screening methods. This has allowed drug developers to move cardiac ion channel safety screening earlier into the drug development pipeline, and to mitigate potential cardiac safety liabilities of potent compound series (Davenport et al., 2010; Riether et al., 2011; Tye et al., 2011).
The two currently known instruments with the highest rate of parallelization and the largest throughput are – at the time of writing this manuscript – the SyncroPatch 96 (Nanion Technologies) and the IonWorks Barracuda (MDS). While the SyncroPatch is capable of handling up to 96 parallel high-quality Gigaseal recordings under continuous voltage control (using 96 well plates), the Barracuda can perform 384 parallel recordings – albeit potentially at the price of reduced data quality or limited voltage control, as the Barracuda relies on low-resistance seals and employs the population patch-clamp (PPC) technology (Finkel et al., 2006).
Furthermore, customer suggestions have led to other new developments to the existing automated patch-clamp robots. Recently there have been further improvements of the seal rates and seal stabilities (e.g., by optimizing chip substrates) as well as the addition of more sophisticated automated cell culture preparations on board of some instruments (Mathes et al., 2009). In the future there is likely to be even further increases in throughput, which could be achieved by an additional increase in parallelization.
Other expected development steps concern the biological side, as well as the pharmacological relevance of the data obtained by automated patch-clamping: at the time of writing this manuscript, only a few of the amplifiers from automated patch-clamp instruments are capable of operating in current clamp mode, and thus are able to perform AP recordings from cardiomyocytes, which are of great interest to the safety pharmacology community. Although neither drug-induced prolongation nor triangulation of AP in cardiac myocytes (e.g., Purkinje fibers) are perfect surrogates of risk (Gintant et al., 2001; Martin et al., 2006), if affordable and capable of high-throughput, these measures might become valuable complementary surrogates for QT prolongation (or for the need to provide a warning label) compared to hERG safety margins alone (Gintant, 2011). With improved robust protocols to differentiate stem-cells into cardiomyocytes, and increasing availability of cardiomyocytes from commercial sources, we expect that more comparative and validation data from these cells will be published. This will certainly help the safety pharmacology community to better assess the physiological relevance of automated AP recordings by patch-clamping, and evaluate the implications of these data for safety pharmacology investigations.
Conflict of Interest Statement
The authors declare that the research was conducted in the absence of any commercial or financial relationships that could be construed as a potential conflict of interest.
References
Anson, B. (2011). Pure human iPS-derived cardiomyocytes; a potential source of cardiomyocytes for automated patch-clamp screening. Presentation at the Nanion Technologies User Meeting, Munich.
Asmild, M., Oswald, N., Krzywkowski, K. M., Friis, S., Jacobsen, R. B., Reuter, D., Taboryski, R., Kutchinsky, J., Vestergaard, R. K., Schroder, R. L., Sorensen, C. B., Bech, M., Korsgaard, M. P., and Willumsen, N. J. (2003). Upscaling and automation of electrophysiology: toward high throughput screening in ion channel drug discovery. Receptors Channels 9, 49–58.
Balasubramanian, B., Imredy, J. P., Kim, D., Penniman, J., Lagrutta, A., and Salata, J. J. (2009). Optimization of Ca(v)1.2 screening with an automated planar patch clamp platform. J. Pharmacol. Toxicol. Methods 59, 62–72.
Binah, O., Dolnikov, K., Sadan, O., Shilkrut, M., Zeevi-Levin, N., Amit, M., Danon, A., and Itskovitz-Eldor, J. (2007). Functional and developmental properties of human embryonic stem cells-derived cardiomyocytes. J. Electrocardiol. 40, S192–S196.
Bridgland-Taylor, M. H., Hargreaves, A. C., Easter, A., Orme, A., Henthorn, D. C., Ding, M., Davis, A. M., Small, B. G., Heapy, C. G., Abi-Gerges, N., Persson, F., Jacobson, I., Sullivan, M., Albertson, N., Hammond, T. G., Sullivan, E., Valentin, J. P., and Pollard, C. E. (2006). Optimisation and validation of a medium-throughput electrophysiology-based hERG assay using IonWorks HT. J. Pharmacol. Toxicol. Methods 54, 189–199.
Bril, A., Forest, M. C., Cheval, B., and Faivre, J. F. (1998). Combined potassium and calcium channel antagonistic activities as a basis for neutral frequency dependent increase in action potential duration: comparison between BRL-32872 and azimilide. Cardiovasc. Res. 37, 130–140.
Bril, A., Gout, B., Bonhomme, M., Landais, L., Faivre, J. F., Linee, P., Poyser, R. H., and Ruffolo, R. R. J. (1996). Combined potassium and calcium channel blocking activities as a basis for antiarrhythmic efficacy with low proarrhythmic risk: experimental profile of BRL-32872. J. Pharmacol. Exp. Ther. 276, 637–646.
Brown, A. M. (2008). High throughput functional screening of an ion channel library for drug safety and efficacy. Eur. Biophys. J. 38, 273–278.
Cao, X., Lee, Y. T., Holmqvist, M., Lin, Y., Ni, Y., Mikhailov, D., Zhang, H., Hogan, C., Zhou, L., Lu, Q., Digan, M. E., Urban, L., and Erdemli, G. (2010). Cardiac ion channel safety profiling on the IonWorks Quattro automated patch clamp system. Assay Drug Dev. Technol. 8, 766–780.
Chouabe, C., Drici, M. D., Romey, G., Barhanin, J., and Lazdunski, M. (1998). HERG and KvLQT1/IsK, the cardiac K+ channels involved in long QT syndromes, are targets for calcium channel blockers. Mol. Pharmacol. 54, 695–703.
Committee for Proprietary Medicinal Products. (1997) “Points to consider: the assessment for the potential for QT prolongation by non-cardiovascular medicinal products,” in European Agency for the Evaluation of Medicinal Products, London.
Curran, M. E., Splawski, I., Timothy, K. W., Vincent, G. M., Green, E. D., and Keating, M. T. (1995). A molecular basis for cardiac arrhythmia: HERG mutations cause long QT syndrome. Cell 80, 795–803.
Davenport, A. J., Moller, C., Heifetz, A., Mazanetz, M. P., Law, R. J., Ebneth, A., and Gemkow, M. J. (2010). Using electrophysiology and in silico three-dimensional modeling to reduce human Ether-a-go-go related gene K(+) channel inhibition in a histamine H3 receptor antagonist program. Assay Drug Dev. Technol. 8, 781–789.
Deisemann, H., Ahrens, N., Schlobohm, I., Kirchhoff, C., Netzer, R., and Moller, C. (2008). Effects of common antitussive drugs on the hERG potassium channel current. J. Cardiovasc. Pharmacol. 52, 494–499.
Dunlop, J., Bowlby, M., Peri, R., Vasilyev, D., and Arias, R. (2008). High-throughput electrophysiology: an emerging paradigm for ion-channel screening and physiology. Nat. Rev. Drug Discov. 7, 358–368.
Farre, C., Haythornthwaite, A., Haarmann, C., Stoelzle, S., Kreir, M., George, M., Bruggemann, A., and Fertig, N. (2009). Port-a-patch and patchliner: high fidelity electrophysiology for secondary screening and safety pharmacology. Comb. Chem. High Throughput Screen. 12, 24–37.
Farre, C., Stoelzle, S., Haarmann, C., George, M., Bruggemann, A., and Fertig, N. (2007). Automated ion channel screening: patch clamping made easy. Expert Opin. Ther. Targets 11, 557–565.
Fenichel, R. R., Malik, M., Antzelevitch, C., Sanguinetti, M., Roden, D. M., Priori, S. G., Ruskin, J. N., Lipicky, R. J., and Cantilena, L. R. (2004). Drug-induced torsades de pointes and implications for drug development. J. Cardiovasc. Electrophysiol. 15, 475–495.
Fertig, N., Blick, R. H., and Behrends, J. C. (2002). Whole cell patch clamp recording performed on a planar glass chip. Biophys. J. 82, 3056–3062.
Fertig, N., Meyer, C., Blick, R. H., Trautmann, C., and Behrends, J. C. (2001). Microstructured glass chip for ion-channel electrophysiology. Phys. Rev. E Stat. Nonlin. Soft Matter Phys. 64, 040901.
Finkel, A., Wittel, A., Yang, N., Handran, S., Hughes, J., and Costantin, J. (2006). Population patch clamp improves data consistency and success rates in the measurement of ionic currents. J. Biomol. Screen. 11, 488–496.
Foldes, G., Mioulane, M., Wright, J. S., Liu, A. Q., Novak, P., Merkely, B., Gorelik, J., Schneider, M. D., Ali, N. N., and Harding, S. E. (2011). Modulation of human embryonic stem cell-derived cardiomyocyte growth: a testbed for studying human cardiac hypertrophy? J. Mol. Cell. Cardiol. 50, 367–376.
Gintant, G. (2011). An evaluation of hERG current assay performance: translating preclinical safety studies to clinical QT prolongation. Pharmacol. Ther. 129, 109–119.
Gintant, G. A., Limberis, J. T., McDermott, J. S., Wegner, C. D., and Cox, B. F. (2001). The canine Purkinje fiber: an in vitro model system for acquired long QT syndrome and drug-induced arrhythmogenesis. J. Cardiovasc. Pharmacol. 37, 607–618.
Golden, A. P., Li, N., Chen, Q., Lee, T., Nevill, T., Cao, X., Johnson, J., Erdemli, G., Ionescu-Zanetti, C., Urban, L., and Holmqvist, M. (2011). IonFlux: a microfluidic patch clamp system evaluated with human ether-a-go-go related gene channel physiology and pharmacology. Assay Drug Dev. Technol. doi: 10.1089/adt.2010.0362. [Epub ahead of print].
Hamill, O. P., Marty, A., Neher, E., Sakmann, B., and Sigworth, F. J. (1981). Improved patch-clamp techniques for high-resolution current recording from cells and cell-free membrane patches. Pflugers Arch. 391, 85–100.
Hancox, J. C., Levi, A. J., and Witchel, H. J. (1998). Time course and voltage dependence of expressed HERG current compared with native “rapid” delayed rectifier K current during the cardiac ventricular action potential. Pflugers Arch. 436, 843–853.
Hansen, R. S., Olesen, S. P., and Grunnet, M. (2007). Pharmacological activation of rapid delayed rectifier potassium current suppresses bradycardia-induced triggered activity in the isolated guinea pig heart. J. Pharmacol. Exp. Ther. 321, 996–1002.
Harding, S. E., Ali, N. N., Brito-Martins, M., and Gorelik, J. (2007). The human embryonic stem cell-derived cardiomyocyte as a pharmacological model. Pharmacol. Ther. 113, 341–353.
Hondeghem, L. M. (2006). Thorough QT/QTc not so thorough: removes torsadogenic predictors from the T-wave, incriminates safe drugs, and misses profibrillatory drugs. J. Cardiovasc. Electrophysiol. 17, 337–340.
ICH. (2005). “The non-clinical evaluation of the potential for delayed ventricular repolarization (QT interval prolongation) by human pharmaceuticals: S7B,” in Proceedings of the Expert Working Group (Safety) of the International Conference on Harmonisation of Technical Requirements for Registration of Pharmaceuticals for Human Use and the FDA (Food and Drug Administration, USA), Rockville, MD.
January, C. T., and Moscucci, A. (1992). Cellular mechanisms of early afterdepolarizations. Ann. N. Y. Acad. Sci. 644, 23–32.
January, C. T., and Riddle, J. M. (1989). Early afterdepolarizations: mechanism of induction and block. A role for L-type Ca2+ current. Circ. Res. 64, 977–990.
Jones, K. A., Garbati, N., Zhang, H., and Large, C. H. (2009). Automated patch clamping using the QPatch. Methods Mol. Biol. 565, 209–223.
Kiss, L., Bennett, P. B., Uebele, V. N., Koblan, K. S., Kane, S. A., Neagle, B., and Schroeder, K. (2003). High throughput ion-channel pharmacology: planar-array-based voltage clamp. Assay Drug Dev. Technol. 1, 127–135.
Kuryshev, Y. A., Bruening-Wright, A., Brown, A. M., and Kirsch, G. E. (2010). Increased cardiac risk in concomitant methadone and diazepam treatment: pharmacodynamic interactions in cardiac ion channels. J. Cardiovasc. Pharmacol. 56, 420–430.
Kutchinsky, J., Friis, S., Asmild, M., Taboryski, R., Pedersen, S., Vestergaard, R. K., Jacobsen, R. B., Krzywkowski, K., Schroder, R. L., Ljungstrom, T., Helix, N., Sorensen, C. B., Bech, M., and Willumsen, N. J. (2003). Characterization of potassium channel modulators with QPatch automated patch-clamp technology: system characteristics and performance. Assay Drug Dev. Technol. 1, 685–693.
Ly, J. Q., Shyy, G., and Misner, D. L. (2007). Assessing hERG channel inhibition using PatchXpress. Clin. Lab. Med. 27, 201–208.
Martin, R. L., Su, Z., Limberis, J. T., Palmatier, J. D., Cowart, M. D., Cox, B. F., and Gintant, G. A. (2006). In vitro preclinical cardiac assessment of tolterodine and terodiline: multiple factors predict the clinical experience. J. Cardiovasc. Pharmacol. 48, 199–206.
Mathes, C. (2006). QPatch: the past, present and future of automated patch clamp. Expert Opin. Ther. Targets 10, 319–327.
Mathes, C., Friis, S., Finley, M., and Liu, Y. (2009). QPatch: the missing link between HTS and ion channel drug discovery. Comb. Chem. High Throughput Screen. 12, 78–95.
Milligan, C. J., Li, J., Sukumar, P., Majeed, Y., Dallas, M. L., English, A., Emery, P., Porter, K. E., Smith, A. M., McFadzean, I., Beccano-Kelly, D., Bahnasi, Y., Cheong, A., Naylor, J., Zeng, F., Liu, X., Gamper, N., Jiang, L. H., Pearson, H. A., Peers, C., Robertson, B., and Beech, D. J. (2009). Robotic multiwell planar patch-clamp for native and primary mammalian cells. Nat. Protoc. 4, 244–255.
Mitcheson, J. S. (2008). hERG potassium channels and the structural basis of drug-induced arrhythmias. Chem. Res. Toxicol. 21, 1005–1010.
Mo, Z. L., Faxel, T., Yang, Y. S., Gallavan, R., Messing, D., and Bahinski, A. (2009). Effect of compound plate composition on measurement of hERG current IC(50) using PatchXpress. J. Pharmacol. Toxicol. Methods 60, 39–44.
Moeller, C. (2010). Challenging automated patch-clamping: quo vadis? Presentation at the Nanion Technologies User Meeting, Munich.
Moeller, C. (2011). Keeping the Rhythm: hERG and beyond in Cardiovascular Safety Pharmacology. Expert Rev. Clin. Pharmacol. 3, 321–329.
Moller, C., and Slack, M. (2010). Impact of new technologies for cellular screening along the drug value chain. Drug Discov. Today 15, 384–390.
Neher, E., and Sakmann, B. (1976). Single-channel currents recorded from membrane of denervated frog muscle fibres. Nature 260, 799–802.
Penniman, J. R., Kim, D. C., Salata, J. J., and Imredy, J. P. (2010). Assessing use-dependent inhibition of the cardiac Na(+/−) current (I(Na)) in the PatchXpress automated patch clamp. J. Pharmacol. Toxicol. Methods 62, 107–118.
Riether, D., Wu, L., Cirillo, P. F., Berry, A., Walker, E. R., Ermann, M., Noya-Marino, B., Jenkins, J. E., Albaugh, D., Albrecht, C., Fisher, M., Gemkow, M. J., Grbic, H., Lobbe, S., Moller, C., O’Shea, K., Sauer, A., Shih, D. T., and Thomson, D. S. (2011). 1,4-Diazepane compounds as potent and selective CB2 agonists: optimization of metabolic stability. Bioorg. Med. Chem. Lett. 21, 2011–2016.
Roden, D. M., Lazzara, R., Rosen, M., Schwartz, P. J., Towbin, J., and Vincent, G. M. (1996). Multiple mechanisms in the long-QT syndrome. Current knowledge, gaps, and future directions. The SADS Foundation Task Force on LQTS. Circulation 94, 1996–2012.
Sanguinetti, M. C., Jiang, C., Curran, M. E., and Keating, M. T. (1995). A mechanistic link between an inherited and an acquired cardiac arrhythmia: HERG encodes the IKr potassium channel. Cell 81, 299–307.
Sanguinetti, M. C., and Jurkiewicz, N. K. (1990). Two components of cardiac delayed rectifier K+ current. Differential sensitivity to block by class III antiarrhythmic agents. J. Gen. Physiol. 96, 195–215.
Sanguinetti, M. C., and Keating, M. T. (1997). Role of delayed rectifier potassium channels in cardiac repolarization and arrhythmias. News Physiol. Sci. 12, 152–157.
Sanguinetti, M. C., and Tristani-Firouzi, M. (2006). hERG potassium channels and cardiac arrhythmia. Nature 440, 463–469.
Splawski, I., Shen, J., Timothy, K. W., Lehmann, M. H., Priori, S., Robinson, J. L., Moss, A. J., Schwartz, P. J., Towbin, J. A., Vincent, G. M., and Keating, M. T. (2000). Spectrum of mutations in long-QT syndrome genes. KVLQT1, HERG, SCN5A, KCNE1, and KCNE2. Circulation 102, 1178–1185.
Stoelzle, S., Haythornthwaite, A., Kettenhofen, R., Kolossov, E., Bohlen, H., George, M., Bruggemann, A., and Fertig, N. (2011). Automated patch clamp on mESC-derived cardiomyocytes for cardiotoxicity prediction. J. Biomol. Screen. 16, 910–916.
Su, Z., Limberis, J., Souers, A., Kym, P., Mikhail, A., Houseman, K., Diaz, G., Liu, X., Martin, R. L., Cox, B. F., and Gintant, G. A. (2009). Electrophysiologic characterization of a novel hERG channel activator. Biochem. Pharmacol. 77, 1383–1390.
Su, Z., Martin, R., Cox, B. F., and Gintant, G. (2004). Mesoridazine: an open-channel blocker of human ether-a-go-go-related gene K+ channel. J. Mol. Cell. Cardiol. 36, 151–160.
Tao, H., Santa, A. D., Guia, A., Huang, M., Ligutti, J., Walker, G., Sithiphong, K., Chan, F., Guoliang, T., Zozulya, Z., Saya, S., Phimmachack, R., Sie, C., Yuan, J., Wu, L., Xu, J., and Ghetti, A. (2004). Automated tight seal electrophysiology for assessing the potential hERG liability of pharmaceutical compounds. Assay Drug Dev. Technol. 2, 497–506.
Tye, H., Mueller, S. G., Prestle, J., Scheuerer, S., Schindler, M., Nosse, B., Prevost, N., Brown, C. J., Heifetz, A., Moeller, C., Pedret-Dunn, A., and Whittaker, M. (2011). Novel 6,7,8,9-tetrahydro-5H-1,4,7,10a-tetraaza-cyclohepta[f]indene analogues as potent and selective 5-HT(2C) agonists for the treatment of metabolic disorders. Bioorg. Med. Chem. Lett. 21, 34–37.
Wible, B. A., Kuryshev, Y. A., Smith, S. S., Liu, Z., and Brown, A. M. (2008). An ion channel library for drug discovery and safety screening on automated platforms. Assay Drug Dev. Technol. 6, 765–780.
Witchel, H. J., Hancox, J. C., and Nutt, D. J. (2003). Psychotropic drugs, cardiac arrhythmia, and sudden death. J. Clin. Psychopharmacol. 23, 58–77.
Xi, J., Khalil, M., Shishechian, N., Hannes, T., Pfannkuche, K., Liang, H., Fatima, A., Haustein, M., Suhr, F., Bloch, W., Reppel, M., Saric, T., Wernig, M., Janisch, R., Brockmeier, K., Hescheler, J., and Pillekamp, F. (2010). Comparison of contractile behavior of native murine ventricular tissue and cardiomyocytes derived from embryonic or induced pluripotent stem cells. FASEB J. 24, 2739–2751.
Xu, J., Guia, A., Rothwarf, D., Huang, M., Sithiphong, K., Ouang, J., Tao, G., Wang, X., and Wu, L. (2003). A benchmark study with sealchip planar patch-clamp technology. Assay Drug Dev. Technol. 1, 675–684.
Zhang, J., Wilson, G. F., Soerens, A. G., Koonce, C. H., Yu, J., Palecek, S. P., Thomson, J. A., and Kamp, T. J. (2009). Functional cardiomyocytes derived from human induced pluripotent stem cells. Circ. Res. 104, e30–e41.
Keywords: hERG, cardiac ion channel, LQT, torsades de pointes, automated patch-clamp, planar patch-clamp, action potential, stem cell
Citation: Möller C and Witchel H (2011) Automated electrophysiology makes the pace for cardiac ion channel safety screening. Front. Pharmacol. 2:73. doi: 10.3389/fphar.2011.00073
Received: 15 August 2011;
Paper pending published: 17 September 2011;
Accepted: 06 November 2011;
Published online: 23 November 2011.
Edited by:
Ralf Franz Kettenhofen, Axiogenesis AG, GermanyReviewed by:
Anthony Bahinski, Wyss Institute for Biologically Inspired Engineering at Harvard University, USAHerbert Himmel, Bayer Pharma AG, Germany
Timm Danker, NMI TT GmbH, Germany
Copyright: © 2011 Möller and Witchel. This is an open-access article subject to a non-exclusive license between the authors and Frontiers Media SA, which permits use, distribution and reproduction in other forums, provided the original authors and source are credited and other Frontiers conditions are complied with.
*Correspondence: Clemens Möller, InViTe Research Institute, Albstadt-Sigmaringen University, 72488 Sigmaringen, Germany. e-mail: office@biophysicalconsulting.de