- Global Regulatory Affairs HMOs, Early Life & Medical Nutrition, DSM-Firmenich, Hørsholm, Denmark
Human milk oligosaccharides (HMOs) are a diverse group of structures and an abundant bioactive component of breastmilk that contribute to infant health and development. Preclinical studies indicate roles for HMOs in shaping the infant gut microbiota, inhibiting pathogens, modulating the immune system, and influencing cognitive development. In the past decade, several industrially produced HMOs have become available to fortify infant formula. Clinical intervention trials with manufactured HMOs have begun to corroborate some of the physiological effects reported in preclinical studies, especially modulation of the gut microbiota in the direction of breastfed infants. As more HMOs become commercially available and as HMOs have some shared mechanisms of action, there is a need to better understand the unique and differential effects of individual HMOs and the benefits of combining multiple HMOs. This review focuses on the differential effects of different HMO structural classes and individual structures and presents a scientific rationale for why combining multiple structurally diverse HMOs is expected to exert greater biological effects.
1 Introduction
The associations between breastfeeding, intestinal bacteria composition, and improved infant health and survival were recognized already in the late 19th century. Pioneering work by many scientists and physicians in the early 20th century led to the discovery and characterization of the “bifidus factor” in breastmilk as the carbohydrate fraction composed of human milk oligosaccharides (HMOs) (1). Today, more than 200 molecular species of HMOs have been detected (2), and the structures of more than 160 HMOs have been elucidated (3). Although many different HMO structures are present in breastmilk, the 10 most abundant HMOs have been reported to account for more than 70% of the total HMO concentration (4). The role of HMOs in shaping the infant gut microbiota has been well-established, and evidence also indicates roles for HMOs in pathogen inhibition, immune modulation and cognitive development (5–7).
The benefits of breastfeeding on growth, immunity, cognition, and other functions important for optimal development are substantiated by an extensive body of scientific research (8–11). The World Health Organization recommends exclusive breastfeeding during the first six months of life and continued breastfeeding for up to two years or beyond (12). However, due to a variety of social, economic and medical factors, fewer than half of infants under 6 months old are exclusively breastfed (12). The only suitable alternative to breastmilk that meets the nutritional requirements of infants is infant formula, which aims to resemble the composition and functionality of breastmilk as closely as possible (13). As HMOs are the third most abundant solid component of breastmilk (14), the HMO fraction represents the largest compositional gap between breastmilk and infant formula.
Despite awareness of the compositional and physiological importance of HMOs in breastmilk, HMOs could not be produced efficiently at large scale during the 20th century. To mimic the bifidogenic effect of HMOs, other oligosaccharides such as galacto-oligosaccharides (GOS), fructo-oligosaccharides (FOS) and polydextrose (PDX) were introduced to infant formula (15) and are still commonly found in infant formula products today. However, these oligosaccharides are not present at appreciable levels (GOS) or at all (FOS, PDX) in human milk, and they are structurally distinct from HMOs, lacking the monosaccharide units present in HMOs such as fucose, N-acetylglucosamine (GlcNAc) and N-acetyl-D-neuraminic acid (Neu5Ac; also known as sialic acid).
Only in the past decade have technological developments made it possible to produce HMOs at large scale and to fortify infant formula with HMOs. The first HMOs to receive regulatory approvals were 2′-fucosyllactose (2′-FL) and lacto-N-neotetraose (LNnT), which received approvals in the U.S. in September and October 2015 and in the EU in March 2016 (16). Infant formula products fortified with 2′-FL or the combination of 2′-FL and LNnT were introduced to the market soon thereafter. Currently, there are regulatory approvals for eight HMOs in the U.S., EU and UK and five to six HMOs in many jurisdictions worldwide such as Australia/New Zealand, Brazil, Thailand and Singapore. In 2023, 2′-FL and LNnT received the first regulatory approvals for HMOs in China. Oral supplementation with manufactured HMOs has been evaluated in at least 26 clinical trials (17). The majority of HMO intervention trials have been conducted in infants, but HMO supplementation has been evaluated in children and adults as well. All these clinical trials reported that consumption of manufactured HMOs was safe and well-tolerated, and many of the trials in infants also reported physiological effects of HMOs, including gut microbiota composition and stooling patterns more similar to breastfed infants and reduced incidence of infections (17).
Despite the progress in HMO production capabilities, clinical evidence and regulatory approvals, infant formula products fortified with HMOs represent a small fraction of the infant formula market today. Although a few infant formula manufacturers have launched products with five or six HMOs, currently most infant formula brands that do contain HMOs are fortified with only one or two. As the number of HMOs that can be produced large-scale increases, there is also increased scrutiny regarding what benefits each additional HMO can provide. This review aims to present evidence regarding the biological effects and potential health benefits of combining multiple HMOs with diverse structures.
2 Expected benefits of combining multiple HMOs
Due to the complex logistics and sample sizes necessary for conducting clinical trials in young infants, it is not feasible to compare the physiological effects of many individual HMOs and combinatorial mixtures of HMOs simultaneously in a clinical trial. Hence, preclinical studies provide much of the knowledge on the mechanisms by which different HMOs may exert effects on gut microbiota composition, pathogen inhibition and cognitive development. Although HMOs have many overlapping and redundant effects, there is some evidence for unique and differential benefits of different structural classes of HMOs and different individual HMO structures. Here, the rationale and scientific evidence are presented for why supplementation with multiple HMOs is expected to bring greater health benefits to infants and young children compared to addition of only one or two HMOs, namely:
1. Microbial degradation of HMOs from the three major structural classes (fucosylated, neutral core, sialylated) releases three different molecules: fucose, N-acetylglucosamine (GlcNAc) and N-acetyl-D-neuraminic acid (Neu5Ac; also known as sialic acid), which have unique structures and biological functions. These HMO-derived monosaccharides can cross-feed to support growth of specific commensal gut bacteria.
2. Infant gut commensal bacteria co-evolved with HMO structures, and the capacity to utilize different HMOs varies among commensal bacteria (18, 19). Therefore, increasing the number and structural diversity of HMOs is expected to support a wider array of beneficial bacteria, which consequently beneficially impacts the gut metabolic profile. This concept is supported by data from an infant fecal fermentation study that systematically compared the effects of one, two and six HMOs on the infant microbiota (20).
3. HMOs have a variety of anti-pathogenic effects, including inhibiting growth and colonization of pathogens that cannot utilize HMOs as growth substrates (competitive exclusion), functioning as decoy receptors for pathogenic viruses and bacteria, and modulating the neonatal immune system through direct interactions with epithelial and immune cells (21, 22). Due to the structural specificity of HMO binding interactions with receptors on human cells and with pathogens, supplementation with a greater number of HMOs is expected to support defense against a broader array of viruses and bacteria.
4. Data from observational human studies and experimental animal models indicate that fucosylated and sialylated HMOs could play important roles in brain development and cognitive function and that these different classes of HMOs function through distinct mechanisms (23). Preclinical studies show that sialic acid derived from sialylated HMOs can cross the blood-brain barrier, whereas fucose cannot, and fucosylated HMOs appear to function through the microbiome-gut-brain axis.
2.1 Degradation of HMOs from different structural classes releases different monosaccharides
There are five monosaccharide building blocks that comprise HMOs: glucose, galactose, fucose, GlcNAc and sialic acid. HMOs contain lactose at the reducing end and are covalently bound to one or more monosaccharides or disaccharides. Lactose can be fucosylated or sialylated through different linkages to generate the smallest trisaccharide HMOs: 2′-FL, 3-FL, 3′-SL and 6′-SL. Lactose can also be extended by addition of the disaccharides lacto-N-biose [galactose bound to GlcNAc through a β1-3 linkage, as found in lacto-N-tetraose (LNT)] and/or N-acetyllactosamine (galactose bound to GlcNAc through a β1-4 linkage, as found in LNnT). Lactose can be elongated by one or more of these disaccharides in either a linear or branching pattern and can be further modified by addition of one or more fucose and/or sialic acid residues through various glycosidic linkages. These possibilities for HMO synthesis generate a pool of diverse structures that vary in size, composition and conformation (see examples in Table 1). Depending on their monosaccharide composition, HMOs are generally classified as belonging to one of three major structural classes: fucosylated, neutral core or sialylated, although fucosylated acidic HMOs (i.e., HMOs that contain both fucose and sialic acid molecules) also exist and may be considered a hybrid of the fucosylated and sialylated structural classes. Depending on structural class, different molecules can be released following degradation of HMOs by the gut microbiota, including fucose, GlcNAc and sialic acid. These three molecules have unique structures and biological functions and can be further utilized by intestinal bacteria to produce specific secondary metabolites, affect intestinal function, or be absorbed and incorporated in distal organs such as the brain.
Fucosylated HMOs account for the greatest proportion of the total HMO pool, typically at 60%-70%, with some variation according to geographic location and genetic factors (25). The majority of the global population, ranging from 65%–98% in different geographic locations (26), have a functional 2-α-L-fucosyltransferase 2 (FUT-2) gene, produce high levels of α1,2-fucosylated HMOs such as 2′-FL and LNFP-I, and are referred to as secretors. In contrast, non-secretors have polymorphisms that render FUT-2 non-functional and produce little to no α1,2-fucosylated HMOs, although they can produce α1,3-fucosylated HMOs such as 3-FL and LNFP-III. Given the differences in the types and total concentrations of fucosylated HMOs in the breastmilk of secretors and non-secretors, several studies have investigated whether mothers’ secretor status affects infants’ gut microbiota composition. Two studies that sampled during the early lactation period (6 to 120 or 180 days post-partum) in North American and Chinese breastfed infants found that bifidobacteria were established earlier and at higher levels in infants of secretor mothers (27, 28). However, studies of Indonesian, Finnish and Danish breastfed infants sampled at 60–65 days, 3 months and 5 months, respectively, reported that maternal secretor status was not associated with infants’ microbiota composition and that there were no significant differences in the relative abundance of bifidobacteria (29–31). One exception was the caesarean-born subgroup of the Finnish cohort, where the infants of non-secretors had fewer bifidobacteria and more enterococci (30). Thus, a consistent effect of secretor status on infant microbiota composition cannot be concluded and is likely challenged by heterogeneity in the study cohorts with regards to sample population, birth mode, lactation period and the extent to which infants are exclusively breastfed.
Fucose that is liberated by degradation of fucosylated HMOs can be further metabolized by infant gut-associated bacteria such as Bifidobacterium longum subsp. infantis and Bifidobacterium longum subsp. suis to produce 1,2-propanediol (32), which can cross-feed to other gut commensal bacteria such as Anaerobutyricum hallii (formerly Eubacterium hallii) to produce propionic acid (33). Short chain fatty acids such as propionic acid are important cellular energy sources and cell signaling molecules. Fucose may also support growth of diverse gut bacteria, as several species of Bacteroides and specific strains of Lacticaseibacillus rhamnosus have been shown to grow on fucose as a sole carbon source (34). In addition to supporting trophic interactions of the microbiota, fucose has also been shown to suppress virulence genes in pathogens such as E. coli, and fucosylated glycans have been shown to protect against intestinal and systemic inflammation in animal models (35). By providing a source of free fucose in the intestine, fucosylated HMOs appear to play multiple roles in shaping the gut microbiota and maintaining intestinal homeostasis.
Like fucose, GlcNAc supports trophic interactions in the complex ecosystem of the gut microbiota. Liberating GlcNAc from neutral core HMOs such as LNnT and LNT requires degrading both the linkage of the terminal galactose to GlcNAc and the linkage of GlcNAc to the lactose core. Several species of infant gut-associated bifidobacteria including B. infantis and B. bifidum possess the necessary enzymes to degrade these linkages (36). The enzymatic capability to degrade lacto-N-triose II (LNT2, a degradation intermediate of LNnT and LNT) to liberate GlcNAc and lactose has been demonstrated for B. breve UCC2003 (37). Subsequently, free GlcNAc can potentially be utilized by other gut bacteria, as it has been demonstrated that specific strains of Lactobacillus species such as L. plantarum and L. paracasei can grow on GlcNAc as a sole carbon source (38). In addition, an animal study showed that oral supplementation with GlcNAc enhanced intestinal barrier function and protected against chemically-induced colitis (39). A pilot clinical study that tested oral administration of GlcNAc in children with treatment-resistant inflammatory bowel disease showed promising results in improvement of symptoms (40). These results suggest that GlcNAc itself may influence barrier function and inflammation in the intestine.
Sialylated HMOs are typically present at 10%–20% of the total HMO pool, with geographic variation (25). Degradation of sialylated HMOs, such as 3′-SL and 6′-SL, by intestinal bacteria releases sialic acid. In contrast to fucose and GlcNAc, which can be utilized for growth by several Bacteroides and Lactobacillus species as described above, the ability to utilize sialic acid appears to be uncommon among typical infant gut-associated bacteria. In a screen of 12 Bifidobacterium strains and 12 Lactobacillus strains, only one strain, L. plantarum LP-66, was able to grow on sialic acid as a sole carbon source, whereas half of the strains were able to grow on GlcNAc (38). This apparent limited capacity to utilize sialic acid may mean that it is more available to be absorbed into circulation and incorporated into distal organs such as the brain.
2.2 Combining structurally diverse HMOs should support a wider array of beneficial bacteria
Typically, the most abundant bacterial species found in the breastfed infant gut microbiota are Bifidobacterium species, including B. longum subsp. infantis, B. bifidum, B. longum subsp. longum and B. breve (41, 42). While B. longum subsp. infantis and B. bifidum possess many glycoside hydrolase enzymes and can degrade a wide range of structurally distinct HMOs, other species commonly associated with the breastfed infant gut microbiota, such as B. longum subsp. longum and B. breve, generally can degrade only neutral core HMOs, especially LNT [reviewed in (43); summarized in Table 2].
In a screen of 21 B. longum subsp. infantis strains and 13 B. bifidum strains tested for their ability to grow on each of five individual HMOs provided as the sole carbon source, all the B. longum subsp. infantis strains and the majority of B. bifidum strains grew on 2′-FL, 3-FL, LNT, LNnT and 6′-SL (44). In contrast, of 17 B. longum subsp. longum strains tested for their ability to grow on each of six individual HMOs, only one strain grew on 2′-FL and 3-FL, none of the strains grew on 3′-SL or 6′-SL, and the capacity to grow on LNnT was highly variable (45). However, all but one of the 17 B. longum subsp. longum strains exhibited high growth on LNT (45). Similar to B. longum subsp. longum, a screen of 24 B. breve strains showed that the ability to grow on 2′-FL or 3-FL was rare (high growth on 2′-FL for only two of 24 strains tested), and none of the strains grew on 3′-SL or 6′-SL (47). However, all 24 B. breve strains exhibited high growth on LNT and LNnT. Despite this relatively limited capacity to degrade HMOs, B. breve is frequently identified in infant stool samples, which is attributed to its ability to function as a scavenger species that can cross-feed on HMO-derived monosaccharides (43). The in vitro data suggest that inclusion of neutral core HMOs is likely to support growth of infant gut-associated B. longum subsp. longum and B. breve species to a greater extent than what would be expected for fucosylated or sialylated HMOs.
In addition to Bifidobacterium species, other infant gut commensal bacteria are capable of utilising HMOs, including several Bacteroides species and Akkermansia muciniphila (46, 48–50). Although these species are typically less abundant in the breastfed-infant gut microbiota compared to B. infantis and B. bifidum, they may nevertheless play important and specialized roles, for example in metabolite production, immune modulation, and mucin degradation (51). Since Bacteroides are generally equipped with diverse enzymes for degrading complex plant polysaccharides (52), they may play a key role during the transition to solid foods. Although the capability to utilize HMOs is quite limited among Lactobacillus species, some lactobacilli can cross-feed on HMO-derived monosaccharides, as described in the previous examples. Thus, as the number and structural diversity of HMOs increases, it is expected that HMOs and their degradation products will support growth of a wider array of beneficial gut bacteria.
Results of a recent infant fecal fermentation study support this hypothesis. The effects of 2′-FL alone, 2′-FL+LNnT, and a 6 HMO mix (containing 2′-FL, DFL, LNT, LNnT, 3′-SL and 6′-SL) on the infant microbiota were compared in a long-term in vitro fermentation study that featured a 2-week stabilization period, 2-week baseline period and 3-week treatment period (20). Although all HMO treatments promoted increases in Bifidobacteriacaea, the 6 HMO mix promoted the highest abundance and greatest diversity of Bifidobacteriacaea. In addition, the 6 HMO mix was the only treatment that promoted increases in Bacteroidaceae at the end of the treatment period, as well as increases in beneficial butyrate-producing bacteria such as Faecalibacterium prausnitzii. Furthermore, filtered supernatant from only the 6 HMO mix significantly enhanced intestinal barrier function in vitro, indicating that metabolites produced by a microbiota supplemented with a complex mixture of HMOs have more substantial positive effects on intestinal physiology.
These preclinical data on the effects of HMOs on the infant gut microbiota are substantiated by results from several clinical intervention studies, which reported that supplementing infant formulae with mixtures of 2 or 5 HMOs brings the gut microbiota composition closer to that of breastfed infants (summarized in Table 3). In one study, infants receiving infant formula supplemented with 2′-FL, DFL, LNT, 3′-SL and 6′-SL at total doses of either 1.5 or 2.5 g/L had higher relative abundances of total Bifidobacterium and B. longum subsp. infantis, which were significantly higher than the control group and similar to or approaching the breastfed reference group (59). Significant differences between the HMO test groups and the control formula group were also observed for several other bacterial taxa including Clostridia, Lactobacillus, Peptostreptococcaceae, and Streptococcus. For example, the test group receiving the higher HMO dose had a significantly higher relative abundance of Lactobacillus, approaching that of the breastfed group (59). In another clinical study, infants receiving infant formula supplemented with 2′-FL, 3-FL, LNT, 3′-SL and 6′-SL at a total dose of 5.75 g/L had higher relative abundances of Bifidobacterium, particularly Bifidobacterium species capable of producing aromatic lactic acids, and lower relative abundances of Escherichia and Enterococcus, compared to the control group (57). Importantly, there were notable differences in the abundances of Bifidobacterium (sub)-species observed in a clinical trial that evaluated the effects of infant formula supplemented with 7.2 g/L bovine milk-derived oligosaccharides (BMOS), consisting of majority GOS and 0.06 g/L sialylated oligosaccharides (60). The group receiving BMOS had higher relative abundances of B. dentium, B. longum subsp. longum, and B. breve, whereas the breastfed reference group had higher relative abundances of the efficient HMO-utilizing species B. longum subsp. infantis and B. bifidum, indicating that GOS does not modulate the infant gut microbiota with the same specificity as HMOs.
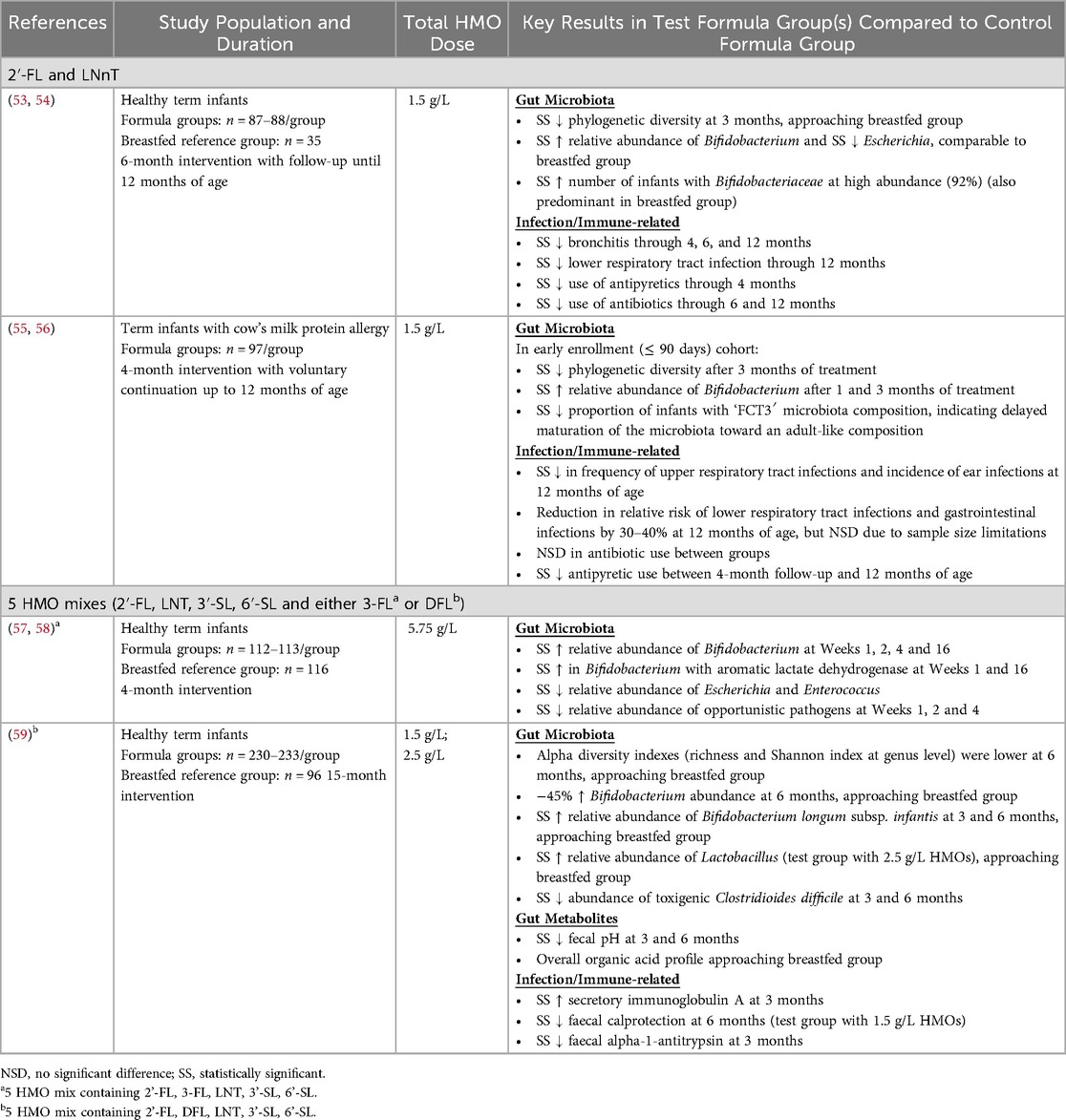
Table 3. Randomized controlled clinical trials that evaluated combinations of manufactured HMOs in infants and reported physiological outcomes related to gut microbiota and immunity.
2.3 Increasing the number of HMOs may support defense against a broader range of pathogens
Systematic reviews have concluded that exclusive breastfeeding reduces the risk of gastrointestinal and respiratory infections (8, 9). Among the many bioactive components in breastmilk that may protect against infections, HMOs are important contributors. One mechanism by which HMOs support defense against infections is modulation of the neonatal immune system. HMOs share structural similarities with blood group glycans and can bind to several classes of lectins, including C-type lectins, galectins and siglecs, which are found on the cell surface of different human cell types, especially immune cells (22). Due to the structural specificity of HMO-lectin interactions, different HMOs would be expected to have different immunomodulatory effects. For example, C-type lectins like DC-SIGN, which is expressed on the surface of dendritic cells, have high specificity for α-fucosylated glycans and have been shown to bind 2′-FL and 3-FL, but not LNT (61). Galectins, which are expressed by intestinal epithelial cells, lymphocytes and antigen-presenting cells, exhibit specificity for glycans containing N-acetyllactosamine and basic disaccharide units such as Galβ1-3/4GlcNAc (22, 62), which are found in neutral core HMOs such as LNT and LNnT. Siglecs are expressed on multiple immune cell types and exhibit specific binding affinity to sialyllactoses (22, 61).
Although relatively few studies to-date have compared the immunomodulatory potential of several different HMOs, a recent study that evaluated six different HMOs on several in vitro models of human intestinal epithelial and immune cells reported some differential effects of HMOs. Whereas sialylated HMOs (3′-SL, 6′-SL) and 3-FL stimulated cytokine release from LPS-activated dendritic cells and M1 macrophages, neutral core HMOs (LNT, LNT2) and fucosylated HMOs (2′-FL, 3-FL) had the most pronounced effects on enhancing intestinal barrier integrity of epithelial cells (63). Animal model data support that combinations of HMOs affect immune cell populations. Compared to newborn piglets fed a control sow milk replacer formula, piglets who received formula supplemented with 4 g/L HMOs (including 2′-FL, LNnT, 6′-SL, 3′-SL and free sialic acid) had significantly more circulating natural killer cells and effector memory T cells in mesenteric lymph nodes, both under noninfected and rotavirus-infected conditions (64). HMO-fed piglets also exhibited reduced duration of rotavirus-induced diarrhea (65). Piglets that received 4 g/L of a combination of GOS and FOS displayed intermediate levels of these immune cell populations, suggesting that HMOs may have more specific and pronounced immunomodulatory effects (64). In another study, newborn piglets received control formula or formula supplemented with either 6.5 g/L BMOS (majority GOS plus 3′-SL and 6′-SL, as described previously), 1.5 g/L of HMOs (2′-FL and LNnT), or the combination of BMOS and HMOs. While piglets that received only BMOS or only HMOs displayed the same percentage of PBMC T-helper cells as the control formula group, piglets that received the combination of BMOS and HMOs had a significantly lower PBMC T-helper cell population (66). These findings are consistent with results from studies of both human infants and piglets, where groups that received mother's milk had significantly lower PBMC T-helper cell populations compared to formula-fed groups (67, 68). Further research is warranted to gain a better understanding of the immunomodulatory potential of HMOs, both as individual structures and in combinations.
In addition to their ability to modulate the immune system, HMOs can function as decoy receptors, binding infectious pathogens and their toxins and thereby blocking pathogen adhesion and infection of host cells (21, 69). Due to structural constraints of receptor binding domains, these interactions are very specific between a particular pathogen and one or a limited number of (often structurally related) HMOs. In mapping the literature of known HMO-virus interactions, a broad trend emerges for HMOs of different structural classes binding to different virus families/genera (see Table 4), although it should be noted that exceptions to this general trend do exist. For example, fucosylated HMOs such as 2′-FL, but not neutral core HMOs (LNT and LNnT), have been shown to bind to several different norovirus strains (70, 74), whereas LNT and LNnT have been shown to bind to several different rotavirus strains (77, 79, 80). For the sialylated HMOs, the weight of evidence for virus binding is for respiratory viruses, including influenza viruses, RSV and mumps virus (81, 82, 84). Since the nasopharyngeal tract is bathed in milk during breastfeeding, it has been postulated that low levels of HMOs may be present in the nasopharynx (88), and this hypothesis is supported by the observation of a low abundance of Bifidobacterium in the nasal microbiome of neonates (89). Thus, HMOs that are retained in the nasopharynx or that travel to the respiratory tract via the circulation upon absorption from the intestine may be able to support direct inhibition of respiratory pathogens in the respiratory tract.
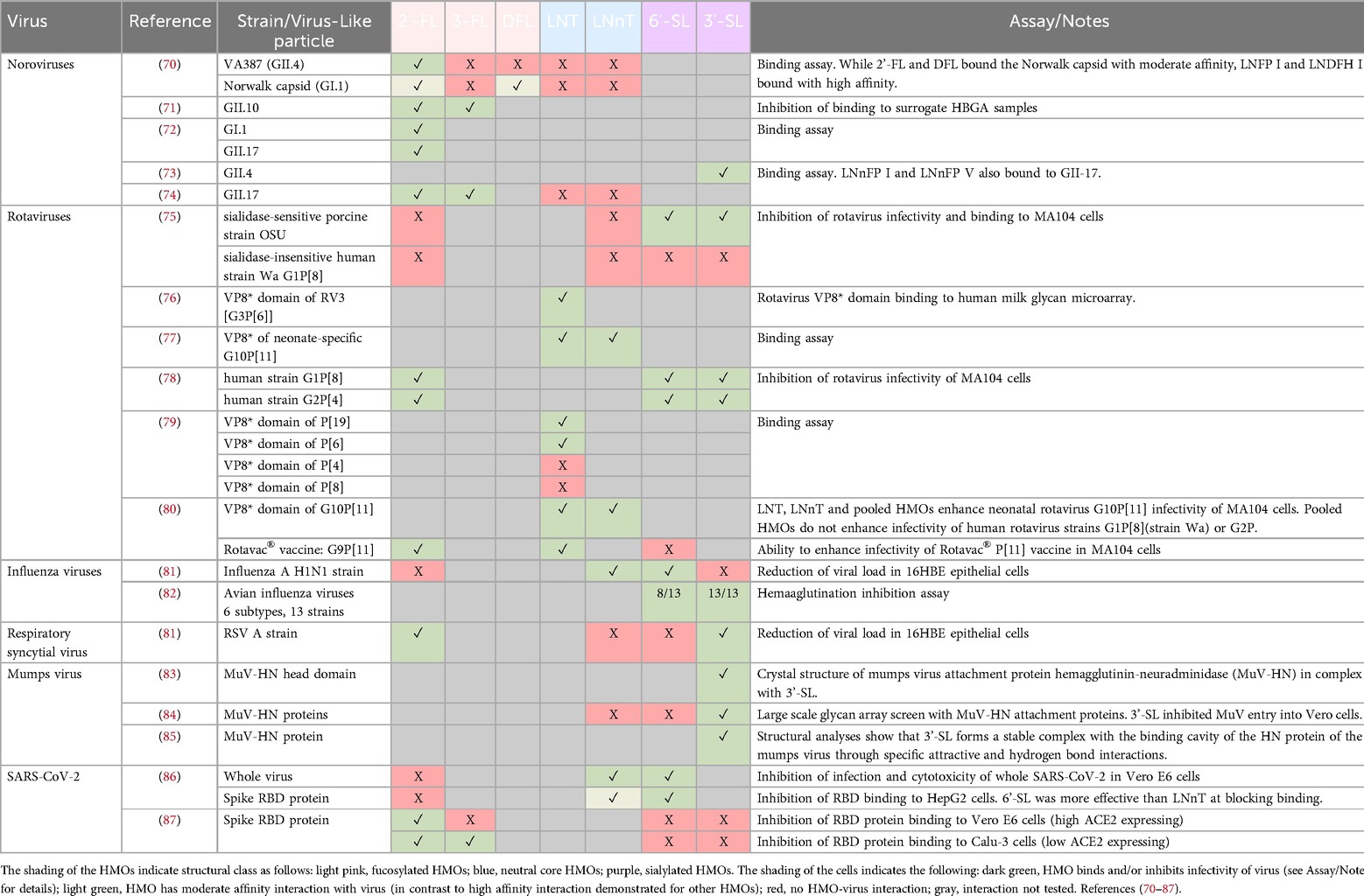
Table 4. Interactions between viruses and HMOs demonstrated in binding assays and structural analyses.
Beyond the broad pattern of different structural classes of HMOs binding to different virus families/genera, there are important details regarding the specificity of HMO-virus interactions. For example, 6′-SL, but not 3′-SL, could reduce viral load of an Influenza A H1N1 strain in 16HBE epithelial cells, whereas 3′-SL, but not 6′-SL, could reduce viral load of an RSV A strain in the same cell type (81). These data suggest that the interactions are not generic to all sialylated HMOs, but rather very specific to the particular HMO structures and the virus strains, constituting a primary example for the common concept of glycan evolution in light of a host-pathogen arms race (90, 91). Similarly, when two different norovirus strains were tested against a panel of fucosylated and neutral core HMOs, the VA387 (GII.4) strain bound with high affinity only to 2′-FL and LNFP-III, whereas the Norwalk capsid (GI.1) bound with high affinity to LNFP-I and LNDFH-I (70). Therefore, to support defense against a broader range of viral pathogens, supplementation with multiple HMOs with diverse structures is likely to be beneficial.
In addition to these specific HMO-virus interactions, HMOs also have specific and differential interactions with pathogenic bacteria, bacterial toxins and parasites [reviewed in (5)]. For example, in a screen of 16 individual HMOs, only DFL and one other HMO could reduce growth of Streptococcus agalactiae (Group B Strep, a significant pathogen for pregnant women and newborns) by more than 50% (92). Despite their structural similarity to DFL, 2′-FL and 3-FL reduced growth by only 8% and 15%, respectively. This study also showed that a heterogeneous extract of HMOs could reduce growth of S. agalactiae by 82%, underscoring the potential for greater biological effects with blends of many HMOs compared to individual HMOs. In another notable example, only five of 20 individual HMOs, including 2′-FL, LNT and LNFP I-III, could bind to both toxin A (TcdA) and toxin B (TcdB) of Clostridioides difficile (93), and both 2′-FL and LNnT could inhibit growth of C. difficile in in vitro fecal fermentation models (94, 95). Clinical data from HMO intervention studies corroborate these preclinical findings, as infants who received infant formula supplemented with either 2′-FL or a mix of 5 HMOs (including 2′-FL, DFL, LNT, 3′-SL and 6′-SL) had relative abundances of C. difficile that were significantly lower than the control formula groups, and comparable with the breastfed reference groups (59, 96). Current knowledge on HMO-pathogen interactions may be biased by which HMOs have been most readily available and therefore most studied. Although some studies of HMO-pathogen interactions focused on a limited number of HMOs due to known structural interactions (e.g., influenza viruses use sialic acid receptors to invade host cells), many studies likely tested a small number of HMO structures due to limited availability. Now that HMO manufacturers can produce many HMOs at high purity and in reasonable quantities, systematic testing of larger panels of HMOs could reveal more insights into the differential roles of HMOs in pathogen inhibition.
The preclinical data indicating that HMOs have immunomodulatory and anti-pathogenic effects are supported by some clinical data showing that combinations of HMOs may enhance defense against infections (summarized in Table 3). Two clinical trials that evaluated the combination of 2′-FL and LNnT reported statistically significant reductions in respiratory tract infections and antipyretic use in the groups that received HMOs as compared with controls (53, 55). HMO supplementation has also been reported to modulate biomarkers of immune function. Fecal samples from infants who received infant formula supplemented with a 5-HMO mix exhibited significantly higher levels of secretory immunoglobulin A and significantly lower levels of calprotectin compared with the control group (59). Further clinical research is needed to substantiate these results and to better understand which combinations of HMOs can exert the most beneficial effects on immune system development and defense against infections. However, attributing reduced infection incidence to an infant formula ingredient can be challenging, as it requires sufficient infection incidence in the study population and an adequately powered sample size in order to show a statistically significant difference between control and test groups.
2.4 Fucosylated and sialylated HMOs may contribute to brain development and cognitive function through distinct mechanisms
The effect of breastfeeding on cognitive development has been extensively studied, and several systematic reviews have concluded that breastfeeding is positively associated with improved performance on intelligence tests, even when correcting for confounding factors such as maternal IQ and home environment (10, 11). Breastmilk contains multiple bioactives that may contribute to neurodevelopment, including fucosylated and sialylated HMOs (23). Sialic acid is an essential building block of brain gangliosides, and in humans, the highest concentration of sialic acid is found in the brain (97). Free sialic acid and sialic acid derived from dietary sialyllactose can cross the blood-brain barrier and be incorporated into brain tissue, as demonstrated by isotope-labeled studies in rodent pups (98, 99). Animal studies suggest that sialic acid derived from sialyllactoses is more slowly released and more readily incorporated in tissues compared to free sialic acid, which is quickly excreted into urine (100, 101). In contrast to sialylated HMOs, animal studies with isotope-labelled 2′-FL indicate that fucosylated HMOs may influence cognitive development through indirect mechanisms involving the gut microbiota and the gut-brain axis. Oral administration of 13C-labelled 2′-FL resulted in 13C-enrichment in plasma, brain and other organs in wild-type mice, but not in germ-free mice where 13C remained in intestinal and fecal samples, suggesting that microbial metabolism of 2′-FL is necessary for absorption and incorporation into organs (102). A follow-up study with 13C-labelled fucose indicated that, although fucose was rapidly absorbed into plasma after oral dosing, 13C-enrichment in the brain did not occur until after more than 3 h, coinciding with arrival of the 13C-fucose bolus in the colon (103). Furthermore, neither 13C-2′-FL nor 13C-fucose administered intravenously resulted in 13C-enrichment in the brain, indicating than circulating 2′-FL and fucose do not cross the blood-brain barrier (102, 103).
Observational studies in breastfed infants have indicated positive associations between specific HMOs and various aspects of cognitive development. Several positive associations have been reported for sialylated HMOs and cognitive outcomes. In particular, concentrations of 3′-SL have been positively associated with early language learning scores (104), and concentrations of 6′-SL at 1 month of age have been positively associated with cognitive and motor scale scores at 18 months (105). Observational studies have also found positive associations between 2′-FL concentrations and improved cognitive outcomes, including positive motor scores at 6 months of age (105), higher cognitive development scores at 24 months (106), and better executive functioning at three years of age (107). To date, most observational studies that have evaluated cognitive outcomes have focused on associations with individual HMOs. However, a few studies have reported positive associations of total fucosylated HMOs or total sialylated HMOs, for example with language development at 18 months and executive functioning at three years old (107, 108). Although these data are generally supportive, results of observational studies should be interpreted with caution, since cognitive development is a complex, multifaceted process that is influenced by many factors, including genetics and environment. Limitations of these studies include imprecise data on breastmilk/HMO intake and assessment of cognitive outcomes based on parent-reported questionnaires. Thus, experimental animal studies, where genetics, environment, HMO dosing, and assessment of cognitive function can be highly controlled, are an important tool for investigating the effects of individual and multiple HMOs on cognitive development. These preclinical studies were recently reviewed in depth (23), but key results will be highlighted here.
Piglets are an established model for studying the influence of pediatric nutrition on neurodevelopment, since piglets and human infants have similar nutritional requirements and brain development patterns (109). Consistent with results of HMO intervention trials in human infants, supplementation of milk replacer formula with 2′-FL, 3′-SL or 6′-SL individually or with bovine-derived whey enriched with sialyllactose was well-tolerated and supported normal growth of piglets (17, 110–112). Studies of piglets supplemented with sialylated HMOs have yielded mixed results with regards to cognitive outcomes. A few studies have found positive effects of sialylated HMOs on memory and learning. For example, among piglets delivered preterm, a higher proportion of those who were supplemented with sialyllactoses (3′-SL + 6′-SL) reached T-maze learning criteria compared to controls (113). In addition, genes related to sialic acid metabolism, myelination, and ganglioside biosynthesis were upregulated in the hippocampus of piglets who received sialyllactoses. In another study, minipigs supplemented with sialyllactoses in milk formula demonstrated improved reference memory during reversal learning in the period corresponding to adolescence, suggesting that sialyllactoses may promote cognitive flexibility (114). However, sialyllactose supplementation did not affect the performance of minipigs on several other cognitive tests, including open field, novel object recognition and runway tasks. Two additional studies also reported that there were no differences in performance on novel object recognition tests between control and test groups of piglets supplemented with either 3′-SL, 6′-SL or sialyllactose (115, 116). These discrepancies might be explained by the fact that the different cognitive tests used to assess cognitive development require different neural pathways (e.g., spatial awareness versus exploration of novelty). It is possible that sialyllactose supplementation supports development of specific neural pathways and therefore enhances performance only on some types of cognitive tasks.
In addition to the studies in piglets, the effects of 3′-SL and 6′-SL on cognition have also been evaluated in rodents. Rat pups who received 6′-SL during the lactation period demonstrated significantly improved long-term potentiation (LTP; the persistent strengthening of synapses between neurons in the brain that is involved in learning and memory) at one year of age, indicating that early life exposure to 6′-SL had long-term benefits on learning and memory (117). As a complement to the studies on sialyllactose supplementation, several mouse knockout model studies have shown that deficiencies in sialylated oligosaccharide synthesis in dams result in reduced cognitive abilities in fostered offspring. Mouse pups deprived of 3′-SL, 6′-SL or both during lactation exhibited reduced cognitive functions in adulthood, such as decreased spatial and working memory (118–120).
Similar to the findings of 3′-SL and 6′-SL supplementation in piglets, dietary administration of 1 g/L 2′-FL, either alone or in a synbiotic combination with B. longum subsp. infantis Bi-26, did not affect novel object recognition memory after 48 h. However, the 2′-FL group had a higher number of object visits, and both the 2′-FL and 2′-FL + Bi-26 groups exhibited a significantly larger relative volume of the pons region of the brain, indicating effects of 2′-FL on both brain structure and behavior (121). Interestingly, in a similar study that evaluated dietary administration of 5 g/L oligofructose (OF), either alone or in combination with 1 g/L 2′-FL, the OF group displayed increased recognition memory after 1 h, but not 48 h, whereas the OF + 2′-FL group displayed increased recognition memory after 48 h only (122). These results suggest that the combination of oligosaccharides specifically affected long-term recognition memory.
Results from rodent studies support effects of 2′-FL on cognitive development and a mechanism of action through the gut-brain axis. Oral administration of 2′-FL to rat pups during the suckling period enhanced LTP in both 6-week-old and 1-year-old rats. Although 2′-FL-supplemented and control animals performed similarly on behavioural tests after weaning (4–6 weeks), rats that had received 2′-FL performed significantly better in the novel object recognition and Y maze paradigms at 1 year of age (123). A study in adult rats also showed that dietary 2′-FL, but not fucose, enhanced LTP in the hippocampus. Vagotomy inhibited the effects of 2′-FL on LTP, indicating that the cognitive benefits of 2′-FL are mediated via the vagus nerve and the gut-brain axis (124).
To date, only a few studies have evaluated the effects of combinations of HMOs on cognitive outcomes in experimental animal models. In a comprehensive study, the diets of Göttingen minipigs were supplemented with different combinations of HMOs from 2 to 11 weeks of age: either 4 g/L of fucosylated and neutral HMOs (FN; comprised of 2′-FL, DFL, LNT, LNnT); 0.68 g/L sialylated HMOs (SL; 3′-SL, 6′-SL); or 4 g/L of the combination (FN + SL). The minipigs were evaluated on multiple cognitive tests during and after the HMO supplementation period at three time points corresponding to infancy, adolescence and adulthood. Out of all the supplementation groups, cognitive tests and time periods, the only significant improvement associated with HMO supplementation was for the SL group on reference memory during the adolescence period, as mentioned above (114). This study underscores that cognitive effects of HMOs may be specific to the type of HMO, the particular cognitive task, and the developmental timeframe. Another study that evaluated combinations of HMOs also reported distinct effects dependent on HMOs and timing. In this study, newborn piglets received control milk replacer formula or formula supplemented with either 6.5 g/L BMOS (GOS, 3′-SL, 6′-SL), 1.5 g/L of HMOs (2′-FL, LNnT), or the combination of BMOS and HMOs. The group receiving HMOs displayed recognition memory after 1 h (125). Interestingly, in contrast to other studies where supplementation with 2′-FL, 3′-SL or 6′-SL individually did not enhance recognition memory at 48 h (116, 121), in this study, the group receiving the combination of BMOs and HMOs displayed recognition memory after 48 h (125). However, since the doses of the different HMOs varied considerably between and within studies (because they were intended to reflect clinically tested levels in infant formula), it is difficult to discern whether the effect might be a result of increased number and/or increased total dose of HMOs.
Collectively, the results from experimental animal studies indicate that 2′-FL, 3′-SL and 6′-SL may influence specific aspects of cognitive development through distinct mechanisms. However, these experimental studies also provide several examples where HMOs do not affect cognitive outcomes. In addition to the fact that the type and timing of a cognitive test may influence whether a positive effect is observed, there are several factors that limit the translation of animal models to human infants. First, milk oligosaccharide composition and gut microbiota composition are different in pigs and mice as compared to humans, with pigs and mice being deficient in fucosylated milk oligosaccharides and bifidobacteria (126–129). Supplementation of piglets with BMOS and/or HMOs did modulate gut microbiota composition, but with increased relative abundances of Bacteroides and/or Blautia (130), rather than of Bifidobacterium, as is typical in human infants. Since microbial metabolism of HMOs appears to be an important mechanism for their effects on cognitive development, particularly for the fucosylated HMOs, and the gut microbiota of pigs and mice did not evolve to utilize HMOs, negative results of cognitive studies should be interpreted with caution. Second, the cognitive demands of human infants are quite different from pigs and mice, for example with regards to language acquisition. Although animal models are an important tool for testing individual and combined HMOs in a controlled manner and for deciphering mechanisms, the roles of fucosylated and sialylated HMOs in cognitive development ultimately need to be substantiated by clinical interventional trials with manufactured HMOs.
3 Discussion
HMOs naturally occur as a complex mixture of molecules in breastmilk. By increasing the number of HMOs from one (2′-FL) or two (2′-FL and LNnT) to the seven HMOs that are available from large-scale production today and have been clinically investigated (2′-FL, 3-FL, DFL, LNnT, LNT, 3′-SL, 6′-SL), representation of the total HMO fraction in mature breastmilk increases from approximately 20%–24% to 45% (4). Although HMOs share some common biological functions, such as the promotion of bifidobacteria, HMOs also have distinct structural differences and unique biological functions, as presented in the examples above. By combining structurally diverse HMOs composed of different monosaccharide building blocks (fucose, GlcNAc, sialic acid), a closer recapitulation of the composition of breastmilk is achieved and greater health benefits are expected, including increased beneficial gut bacteria, defense against a broader range of pathogens, and potentially enhanced cognitive development.
Current knowledge on the mechanisms and health benefits of HMOs is based mostly on research that has been conducted on the smaller HMO structures, particularly the tri- and tetrasaccharides, due to their availability. However, several of the most abundant HMOs in breastmilk are pentasaccharides such as the lacto-N-fucopentaoses (LNFP I-VI) and the sialyllacto-N-tetraoses (LSTa-c) and hexasaccharides such as the lacto-difucohexaoses (LNDFH-I-III) and disialyllacto-N-tetraose (DSLNT) (4). In the context of precision fermentation technology, these larger HMO structures pose certain technical challenges. The enzymes required to catalyze certain glycosidic linkages in larger HMO structures are rare or lacking in the bacterial domain. Production of larger HMOs involves more enzymatic steps, which may lead to a more complex set of side products, and larger HMOs may not be efficiently transported out of the production organism, which is necessary to achieve high yields. Hence, alternative approaches need to be developed and optimized to produce larger HMO structures.
Efforts to manufacture larger HMOs may be warranted, since some of the larger, more structurally complex HMOs may provide unique and important health benefits. For example, lower concentrations of DSLNT have been associated with development of necrotizing enterocolitis in preterm infants (131, 132), motivating additional research to examine whether supplementation with DSLNT could prevent this life-threatening disease. In addition, a large-scale screen of a human milk glycan library that evaluated binding of a human neonatal rotavirus strain revealed that the seven high-affinity binding targets were all hexasaccharides or larger structures (76). This example highlights that there are likely important and not yet discovered roles for larger and more complex HMOs, particularly in the ability of HMOs to bind pathogens and reduce the risk of infection, since binding interactions are very specific due to structural constraints.
Scientific knowledge and production capabilities for HMOs have made enormous progress since the first observations on the unique bacterial composition of breastfed infant stool and the carbohydrate fraction of human milk. Although fortification with HMOs brings infant formula compositionally closer to breastmilk, additional clinical data in infants is needed to confirm some of the health benefits supported only by preclinical data, such as beneficial immunomodulation or enhanced cognitive development. Since HMO exposure occurs during a critical window of development, the potential longer-term benefits of HMO supplementation during infancy on childhood health outcomes such as immune function (e.g., allergy) and metabolism (e.g., obesity) need to be evaluated. Much work remains to increase understanding on the redundant and differential benefits of specific HMOs, to evaluate the full range of HMO physiological effects in clinical trials, and to increase the number and availability of manufactured HMOs worldwide.
Author contributions
AW: Conceptualization, Writing – original draft, Writing – review & editing.
Funding
The author declares that no financial support was received for the research, authorship, and/or publication of this article.
Acknowledgments
The author would like to thank Danica Bajic, Colin Cercamondi, Jim Richards, Christoph Röhrig and Anneleen Spooren for helpful comments and suggestions on this manuscript.
Conflict of interest
The author is an employee of DSM-Firmenich, a company that produces and sells HMOs.
Publisher's note
All claims expressed in this article are solely those of the authors and do not necessarily represent those of their affiliated organizations, or those of the publisher, the editors and the reviewers. Any product that may be evaluated in this article, or claim that may be made by its manufacturer, is not guaranteed or endorsed by the publisher.
References
1. Kunz C. Historical aspects of human milk oligosaccharides. Adv Nutr. (2012) 3:430S–9. doi: 10.3945/an.111.001776
2. Ninonuevo MR, Park Y, Yin H, Zhang J, Ward RE, Clowers BH, et al. A strategy for annotating the human milk glycome. J Agric Food Chem. (2006) 54:7471–80. doi: 10.1021/jf0615810
3. Urashima T, Katayama T, Fukuda K, Hirabayashi J. “Human milk oligosaccharides and innate immunity”. In: Barchi JJ, editor. Comprehensive Glycoscience. 2nd ed. Oxford, UK: Elsevier (2021). p. 389–439.
4. Soyyılmaz B, Mikš MH, Röhrig CH, Matwiejuk M, Meszaros-Matwiejuk A, Vigsnæs LK. The mean of milk: a review of human milk oligosaccharide concentrations throughout lactation. Nutrients. (2021) 13:2737. doi: 10.3390/nu13082737
5. Hill DR, Chow JM, Buck RH. Multifunctional benefits of prevalent HMOS: implications for infant health. Nutrients. (2021) 13:3364. doi: 10.3390/nu13103364
6. Sprenger N, Tytgat HLP, Binia A, Austin S, Singhal A. Biology of human milk oligosaccharides: from basic science to clinical evidence. J Hum Nutr Diet. (2022) 35:280–99. doi: 10.1111/jhn.12990
7. Dinleyici M, Barbieur J, Dinleyici EC, Vandenplas Y. Functional effects of human milk oligosaccharides (HMOs). Gut Microbes. (2023) 15:2186115. doi: 10.1080/19490976.2023.2186115
8. Kramer MS, Kakuma R. Optimal duration of exclusive breastfeeding. Cochrane Database Syst Rev. (2012) 2012:CD003517. doi: 10.1002/14651858.CD003517.pub2
9. Hossain S, Mihrshahi S. Exclusive breastfeeding and childhood morbidity: a narrative review. Int J Environ Res Public Health. (2022) 19:14804. doi: 10.3390/ijerph192214804
10. Horta BL, Victora CG. Long-Term Effects of Breastfeeding: A Systematic Review. Geneva, Switzerland: World Health Organization (WHO) (2013). https://www.who.int/publications/i/item/9789241505307 (Accessed: May 3, 2024).
11. McGowan C, Bland R. The benefits of breastfeeding on child intelligence, behavior, and executive function: a review of recent evidence. Breastfeed Med. (2023) 18:172–87. doi: 10.1089/bfm.2022.0192
12. World Health Organization. Exclusive Breastfeeding (2024). Available online at: https://www.who.int/health-topics/breastfeeding#tab=tab_2 (Accessed: May 3, 2024).
13. Codex Alimentarius. Standard for Infant Formula and Formulas for Special Medical Purposes Intended for Infants. (CODEX STAN 72, adopted in 1981. Amendment: 1983, 1985, 1987, 2011, 2015, 2016, Revision: 2007) (2007). Available online at: https://www.isdi.org/wp-content/uploads/2020/04/CODEX-STAN-72-1981.pdf (accessed: May 3, 2024).
14. Urashima T, Asakuma S, Leo F, Fukuda K, Messer M, Oftedal OT. The predominance of type I oligosaccharides is a feature specific to human breast milk. Adv Nutr. (2012) 3:473S–82. doi: 10.3945/an.111.001412
15. Verkhnyatskaya S, Ferrari M, de Vos P, Walvoort MTC. Shaping the infant microbiome with non-digestible carbohydrates. Front Microbiol. (2019) 10:343. doi: 10.3389/fmicb.2019.00343
16. Bych K, Mikš MH, Johanson T, Hederos MJ, Vigsnæs LK, Becker P. Production of HMOs using microbial hosts—from cell engineering to large scale production. Curr Opin Biotechnol. (2019) 56:130–7. doi: 10.1016/j.copbio.2018.11.003
17. Schönknecht YB, Moreno Tovar MV, Jensen SR, Parschat K. Clinical studies on the supplementation of manufactured human milk oligosaccharides: a systematic review. Nutrients. (2023) 15:3622. doi: 10.3390/nu15163622
18. Duranti S, Lugli GA, Milani C, James K, Mancabelli L, Turroni F, et al. Bifidobacterium bifidum and the infant gut microbiota: an intriguing case of microbe-host co-evolution. Environ Microbiol. (2019) 21:3683–95. doi: 10.1111/1462-2920.14705
19. Kumar H, Collado MC, Wopereis H, Salminen S, Knol J, Roeselers G. The bifidogenic effect revisited—ecology and health perspectives of bifidobacterial colonization in early life. Microorganisms. (2020) 8:1855. doi: 10.3390/microorganisms8121855
20. Natividad JM, Marsaux B, Rodenas CLG, Rytz A, Vandevijver G, Marzorati M, et al. Human milk oligosaccharides and lactose differentially affect infant gut microbiota and intestinal barrier in vitro. Nutrients. (2022) 14:2546. doi: 10.3390/nu14122546
21. Moore RE, Xu LL, Townsend SD. Prospecting human milk oligosaccharides as a defense against viral infections. ACS Infect Dis. (2021) 7:254–63. doi: 10.1021/acsinfecdis.0c00807
22. Triantis V, Bode L, van Neerven RJJ. Immunological effects of human milk oligosaccharides. Front Pediatr. (2018) 6:190. doi: 10.3389/fped.2018.00190
23. Fan Y, McMath AL, Donovan SM. Review on the impact of milk oligosaccharides on the brain and neurocognitive development in early life. Nutrients. (2023) 15:3743. doi: 10.3390/nu15173743
24. Chen X. Human milk oligosaccharides (HMOS): structure, function, and enzyme-catalyzed synthesis. Adv Carbohydr Chem Biochem. (2015) 72:113–90. doi: 10.1016/bs.accb.2015.08.002
25. Vinjamuri A, Davis JCC, Totten SM, Wu LD, Klein LD, Martin M, et al. Human milk oligosaccharide compositions illustrate global variations in early nutrition. J Nutr. (2022) 152:1239–53. doi: 10.1093/jn/nxac027
26. McGuire MK, Meehan CL, McGuire MA, Williams JE, Foster J, Sellen DW, et al. What’s normal? Oligosaccharide concentrations and profiles in milk produced by healthy women vary geographically. Am J Clin Nutr. (2017) 105:1086–100. doi: 10.3945/ajcn.116.139980
27. Lewis ZT, Totten SM, Smilowitz JT, Popovic M, Parker E, Lemay DG, et al. Maternal fucosyltransferase 2 status affects the gut bifidobacterial communities of breastfed infants. Microbiome. (2015) 3:13. doi: 10.1186/s40168-015-0071-z
28. Bai Y, Tao J, Zhou J, Fan Q, Liu M, Hu Y, et al. Fucosylated human milk oligosaccharides and N-glycans in the milk of Chinese mothers regulate the gut microbiome of their breast-fed infants during different lactation stages. mSystems. (2018) 3:e00206–18. doi: 10.1128/mSystems.00206-18
29. Wang A, Diana A, Rahmannia S, Gibson RS, Houghton LA, Slupsky CM. Impact of milk secretor status on the fecal metabolome and microbiota of breastfed infants. Gut Microbes. (2023) 15:2257273. doi: 10.1080/19490976.2023.2257273
30. Korpela K, Salonen A, Hickman B, Kunz C, Sprenger N, Kukkonen K, et al. Fucosylated oligosaccharides in mother’s milk alleviate the effects of caesarean birth on infant gut microbiota. Sci Rep. (2018) 8:13757. doi: 10.1038/s41598-018-32037-6
31. Laursen MF, Pekmez CT, Larsson MW, Lind MV, Yonemitsu C, Larnkjær A, et al. Maternal milk microbiota and oligosaccharides contribute to the infant gut microbiota assembly. ISME Commun. (2021) 1:21. doi: 10.1038/s43705-021-00021-3
32. Bunesova V, Lacroix C, Schwab C. Fucosyllactose and L-fucose utilization of infant Bifidobacterium longum and Bifidobacterium kashiwanohense. BMC Microbiol. (2016) 16:248. doi: 10.1186/s12866-016-0867-4
33. Engels C, Ruscheweyh HJ, Beerenwinkel N, Lacroix C, Schwab C. The common gut microbe Eubacterium hallii also contributes to intestinal propionate formation. Front Microbiol. (2016) 7:713. doi: 10.3389/fmicb.2016.00713
34. Salli K, Hirvonen J, Siitonen J, Ahonen I, Anglenius H, Maukonen J. Selective utilization of the human milk oligosaccharides 2′-fucosyllactose, 3-fucosyllactose, and difucosyllactose by various probiotic and pathogenic bacteria. J Agric Food Chem. (2021) 69:170–82. doi: 10.1021/acs.jafc.0c06041
35. Pickard JM, Chervonsky AV. Intestinal fucose as a mediator of host-microbe symbiosis. J Immunol. (2015) 194:5588–93. doi: 10.4049/jimmunol.1500395
36. Ioannou A, Knol J, Belzer C. Microbial glycoside hydrolases in the first year of life: an analysis review on their presence and importance in infant gut. Front Microbiol. (2021) 12:631282. doi: 10.3389/fmicb.2021.631282
37. James K, Motherway MO, Bottacini F, van Sinderen D. Bifidobacterium breve UCC2003 metabolises the human milk oligosaccharides lacto-N-tetraose and lacto-N-neo-tetraose through overlapping, yet distinct pathways. Sci Rep. (2016) 6:38560. doi: 10.1038/srep38560
38. Thongaram T, Hoeflinger JL, Chow J, Miller MJ. Human milk oligosaccharide consumption by probiotic and human-associated bifidobacteria and lactobacilli. J Dairy Sci. (2017) 100:7825–33. doi: 10.3168/jds.2017-12753
39. Choi S-I, Shin YC, Lee JS, Yoon YC, Kim JM, Sung M-K. N-Acetylglucosamine and its dimer ameliorate inflammation in murine colitis by strengthening the gut barrier function. Food Funct. (2023) 14:8533–44. doi: 10.1039/D3FO00282A
40. Salvatore S, Heuschkel R, Tomlin S, Davies SE, Edwards S, Walker-Smith JA, et al. A pilot study of N-acetyl glucosamine, a nutritional substrate for glycosaminoglycan synthesis, in paediatric chronic inflammatory bowel disease. Aliment Pharmacol Ther. (2000) 14:1567–79. doi: 10.1046/j.1365-2036.2000.00883.x
41. Turroni F, Peano C, Pass DA, Foroni E, Severgnini M, Claesson MJ, et al. Diversity of bifidobacteria within the infant gut microbiota. PLoS One. (2012) 7:e36957. doi: 10.1371/journal.pone.0036957
42. Lordan C, Roche AK, Delsing D, Nauta A, Groeneveld A, MacSharry J, et al. Linking human milk oligosaccharide metabolism and early life gut microbiota: bifidobacteria and beyond. Microbiol Mol Biol Rev. (2024) 88:e0009423. doi: 10.1128/mmbr.00094-23
43. Kiely LJ, Busca K, Lane JA, van Sinderen D, Hickey RM. Molecular strategies for the utilisation of human milk oligosaccharides by infant gut-associated bacteria. FEMS Microbiol Rev. (2023) 47:fuad056. doi: 10.1093/femsre/fuad056
44. Garrido D, Ruiz-Moyano S, Lemay DG, Sela DA, German JB, Mills DA. Comparative transcriptomics reveals key differences in the response to milk oligosaccharides of infant gut-associated bifidobacteria. Sci Rep. (2015) 5:13517 [erratum, 5:15311]. doi: 10.1038/srep13517
45. Garrido D, Ruiz-Moyano S, Kirmiz N, Davis JC, Totten SM, Lemay DG, et al. A novel gene cluster allows preferential utilization of fucosylated milk oligosaccharides in Bifidobacterium longum subsp. longum SC596. Sci Rep. (2016) 6:35045. doi: 10.1038/srep35045
46. Yu Z-T, Chen C, Newburg DS. Utilization of major fucosylated and sialylated human milk oligosaccharides by isolated human gut microbes. Glycobiology. (2013) 23:1281–92. doi: 10.1093/glycob/cwt065
47. Ruiz-Moyano S, Totten SM, Garrido DA, Smilowitz JT, German JB, Lebrilla CB, et al. Variation in consumption of human milk oligosaccharides by infant gut-associated strains of Bifidobacterium breve. Appl Environ Microbiol. (2013) 79:6040–9. doi: 10.1128/AEM.01843-13
48. Chia LW, Mank M, Blijenberg B, Aalvink S, Bongers RS, Stahl B, et al. Bacteroides thetaiotaomicron fosters the growth of butyrate-producing anaerostipes caccae in the presence of lactose and total human milk carbohydrates. Microorganisms. (2020) 8:1513. doi: 10.3390/microorganisms8101513
49. Kijner S, Cher A, Yassour M. The infant gut commensal Bacteroides dorei presents a generalized transcriptional response to various human milk oligosaccharides. Front Cell Infect Microbiol. (2022) 12:854122. doi: 10.3389/fcimb.2022.854122
50. Luna E, Parkar SG, Kirmiz N, Hartel S, Hearn E, Hossine M, et al. Utilization efficiency of human milk oligosaccharides by human-associated Akkermansia is strain dependent. Appl Environ Microbiol. (2022) 88:e0148721. doi: 10.1128/AEM.01487-21
51. Milani C, Duranti S, Bottacini F, Casey E, Turroni F, Mahony J, et al. The first microbial colonizers of the human gut: composition, activities, and health implications of the infant gut microbiota. Microbiol Mol Biol Rev. (2017) 81:e00036–17. doi: 10.1128/MMBR.00036-17
52. Masi AC, Stewart CJ. Untangling human milk oligosaccharides and infant gut microbiome. iScience. (2022) 25:103542. doi: 10.1016/j.isci.2021.103542
53. Puccio G, Alliet P, Cajozzo C, Janssens E, Corsello G, Sprenger N, et al. Effects of infant formula with human milk oligosaccharides on growth and morbidity: a randomized multicenter trial. J Pediatr Gastroenterol Nutr. (2017) 64:624–31. doi: 10.1097/MPG.0000000000001520
54. Berger B, Porta N, Foata F, Grathwohl D, Delley M, Moine D, et al. Linking human milk oligosaccharides, infant fecal community types, and later risk to require antibiotics. mBio. (2020) 11:e03196–9. doi: 10.1128/mBio.03196-19
55. Vandenplas Y, Żołnowska M, Berni Canani R, Ludman S, Tengelyi Z, Moreno-Álvarez A, et al. Effects of an extensively hydrolyzed formula supplemented with two human milk oligosaccharides on growth, tolerability, safety and infection risk in infants with cow’s milk protein allergy: a randomized, multi-center trial. Nutrients. (2022) 14:530. doi: 10.3390/nu14030530
56. Boulangé CL, Pedersen HK, Martin FP, Siegwald L, Pallejà Caro A, Eklund AC, et al. An extensively hydrolyzed formula supplemented with two human milk oligosaccharides modifies the fecal microbiome and metabolome in infants with cow’s milk protein allergy. Int J Mol Sci. (2023) 24:11422. doi: 10.3390/ijms241411422
57. Holst AQ, Myers P, Rodríguez-García P, Hermes GDA, Melsaether C, Baker A, et al. Infant formula supplemented with five human milk oligosaccharides shifts the fecal microbiome of formula-fed infants closer to that of breastfed infants. Nutrients. (2023) 15:3087. doi: 10.3390/nu15143087
58. Parschat K, Melsaether C, Jäpelt KR, Jennewein S. Clinical evaluation of 16-week supplementation with 5HMO-mix in healthy-term human infants to determine tolerability, safety, and effect on growth. Nutrients. (2021) 13:2871. doi: 10.3390/nu13082871
59. Bosheva M, Tokodi I, Krasnow A, Pedersen HK, Lukjancenko O, Eklund AC, et al. Infant formula with a specific blend of five human milk oligosaccharides drives the gut microbiota development and improves gut maturation markers: a randomized controlled trial. Front Nutr. (2022) 9:920362. doi: 10.3389/fnut.2022.920362
60. Estorninos E, Lawenko RB, Palestroque E, Sprenger N, Benyacoub J, Kortman GAM, et al. Term infant formula supplemented with milk-derived oligosaccharides shifts the gut microbiota closer to that of human milk-fed infants and improves intestinal immune defense: a randomized controlled trial. Am J Clin Nutr. (2022) 115:142–53. doi: 10.1093/ajcn/nqab336
61. Noll AJ, Yu Y, Lasanajak Y, Duska-McEwen G, Buck RH, Smith DF, et al. Human DC-SIGN binds specific human milk glycans. Biochem J. (2016) 473:1343–53. doi: 10.1042/BCJ20160046
62. Hirabayashi J, Hashidate T, Arata Y, Nishi N, Nakamura T, Hirashima M, et al. Oligosaccharide specificity of galectins: a search by frontal affinity chromatography. Biochim Biophys Acta. (2002) 1572:232–54. doi: 10.1016/S0304-4165(02)00311-2
63. Boll EJ, Lopez DV, Terne M, Hessing S, Parschat K, Jensen SR. Human milk oligosaccharides differentially support gut barrier integrity and enhance Th1 and Th17 cell effector responses in vitro. Front Immunol. (2024) 15:1359499. doi: 10.3389/fimmu.2024.1359499
64. Comstock SS, Li M, Wang M, Monaco MH, Kuhlenschmidt TB, Kuhlenschmidt MS, et al. Dietary human milk oligosaccharides but not prebiotic oligosaccharides increase circulating natural killer cell and mesenteric lymph node memory T cell populations in noninfected and rotavirus-infected neonatal piglets. J Nutr. (2017) 147:1041–7. doi: 10.3945/jn.116.243774
65. Li M, Monaco MH, Wang M, Comstock SS, Kuhlenschmidt TB, Fahey GC Jr, et al. Human milk oligosaccharides shorten rotavirus-induced diarrhea and modulate piglet mucosal immunity and colonic microbiota. ISME J. (2014) 8:1609–20. doi: 10.1038/ismej.2014.10
66. Monaco MH, Wang M, Hauser J, Yan J, Dilger RN, Donovan SM. Formula supplementation with human and bovine milk oligosaccharides modulates blood IgG and T-helper cell populations, and ex vivo LPS-stimulated cytokine production in a neonatal preclinical model. Front Immunol. (2023) 14:1327853. doi: 10.3389/fimmu.2023.1327853
67. Andersson Y, Hammarström ML, Lönnerdal B, Graverholt G, Fält H, Hernell O. Formula feeding skews immune cell composition toward adaptive immunity compared to breastfeeding. J Immunol. (2009) 183:4322–28. doi: 10.4049/jimmunol.0900829
68. Comstock SS, Wang M, Hester SN, Li M, Donovan SM. Select human milk oligosaccharides directly modulate peripheral blood mononuclear cells isolated from 10-d-old pigs. Br J Nutr. (2014) 111:819–28. doi: 10.1017/S0007114513003267
69. Bode L. Human milk oligosaccharides: every baby needs a sugar mama. Glycobiology. (2012) 22:1147–62. doi: 10.1093/glycob/cws074
70. Shang J, Piskarev VE, Xia M, Huang P, Jiang X, Likhosherstov LM, et al. Identifying human milk glycans that inhibit norovirus binding using surface plasmon resonance. Glycobiology. (2013) 23:1491–8. doi: 10.1093/glycob/cwt077
71. Weichert S, Koromyslova A, Singh BK, Hansman S, Jennewein S, Schroten H, et al. Structural basis for norovirus inhibition by human milk oligosaccharides. J Virol. (2016) 90:4843–8. doi: 10.1128/JVI.03223-15
72. Koromyslova A, Tripathi S, Morozov V, Schroten H, Hansman GS. Human norovirus inhibition by a human milk oligosaccharide. Virology. (2017) 508:81–9. doi: 10.1016/j.virol.2017.04.032
73. Wegener H, Mallagaray Á, Schöne T, Peters T, Lockhauserbäumer J, Yan H, et al. Human norovirus GII.4(MI001) P dimer binds fucosylated and sialylated carbohydrates. Glycobiology. (2017) 27:1027–37. doi: 10.1093/glycob/cwx078
74. Derya SM, Spiegel H, Hanisch FG, Morozov V, Schroten H, Jennewein S, et al. Biotechnologically produced fucosylated oligosaccharides inhibit the binding of human noroviruses to their natural receptors. J Biotechnol. (2020) 318:31–8. doi: 10.1016/j.jbiotec.2020.05.001
75. Hester SN, Chen X, Li M, Monaco MH, Comstock SS, Kuhlenschmidt TB, et al. Human milk oligosaccharides inhibit rotavirus infectivity in vitro and in acutely infected piglets. Br J Nutr. (2013) 110:1233–42. doi: 10.1017/S0007114513000391
76. Yu Y, Lasanajak Y, Song X, Hu L, Ramani S, Mickum ML, et al. Human milk contains novel glycans that are potential decoy receptors for neonatal rotaviruses. Mol Cell Proteomics. (2014) 13:2944–60. doi: 10.1074/mcp.M114.039875
77. Hu L, Ramani S, Czako R, Sankaran B, Yu Y, Smith DF, et al. Structural basis of glycan specificity in neonate-specific bovine-human reassortant rotavirus. Nat Commun. (2015) 6:8346. doi: 10.1038/ncomms9346
78. Laucirica DR, Triantis V, Schoemaker R, Estes MK, Ramani S. Milk oligosaccharides inhibit human rotavirus infectivity in MA104 cells. J Nutr. (2017) 147:1709–14. doi: 10.3945/jn.116.246090
79. Sun X, Li D, Qi J, Chai W, Wang L, Wang L, et al. Glycan binding specificity and mechanism of human and porcine P[6]/P[19] rotavirus VP8*s. J Virol. (2018) 92:e00538–18. doi: 10.1128/jvi.00538-18
80. Ramani S, Stewart CJ, Laucirica DR, Ajami NJ, Robertson B, Autran CA, et al. Human milk oligosaccharides, milk microbiome and infant gut microbiome modulate neonatal rotavirus infection. Nat Commun. (2018) 9:5010. doi: 10.1038/s41467-018-07476-4
81. Duska-McEwen G, Senft A, Ruetschilling T, Barrett E, Buck R. Human milk oligosaccharides enhance innate immunity to respiratory syncytial virus and influenza in vitro. Food Nutr Sci. (2014) 5:1387–98. doi: 10.4236/fns.2014.514151
82. Pandey RP, Kim DH, Woo J, Song J, Jang SH, Kim JB, et al. Broad-spectrum neutralization of avian influenza viruses by sialylated human milk oligosaccharides: in vivo assessment of 3'-sialyllactose against H9N2 in chickens. Sci Rep. (2018) 8:2563. doi: 10.1038/s41598-018-20955-4
83. Kubota M, Takeuchi K, Watanabe S, Ohno S, Matsuoka R, Kohda D, et al. Trisaccharide containing α2,3-linked sialic acid is a receptor for mumps virus. Proc Natl Acad Sci USA. (2016) 113:11579–84. doi: 10.1073/pnas.1608383113
84. Kubota M, Matsuoka R, Suzuki T, Yonekura K, Yanagi Y, Hashiguchi T. Molecular mechanism of the flexible glycan receptor recognition by mumps virus. J Virol. (2019) 93:e00344–19. doi: 10.1128/JVI.00344-19
85. Khavani M, Mehranfar A, Mofrad MRK. On the potentials of sialic acid derivatives as inhibitors for the mumps virus: a molecular dynamics and quantum chemistry investigation. Virus Res. (2023) 326:199050. doi: 10.1016/j.virusres.2023.199050
86. Sheng Y, Vinjamuri A, Alvarez MRS, Xie Y, McGrath M, Chen S, et al. Host cell glycocalyx remodeling reveals SARS-CoV-2 spike protein glycomic binding sites. Front Mol Biosci. (2022) 9:799703. doi: 10.3389/fmolb.2022.799703
87. Yu W, Li Y, Liu D, Wang Y, Li J, Du Y, et al. Evaluation and mechanistic investigation of human milk oligosaccharide against SARS-CoV-2. J Agric Food Chem. (2023) 71:16102–13. doi: 10.1021/acs.jafc.3c04275
88. Binia A, Siegwald L, Sultana S, Shevlyakova M, Lefebvre G, Foata F, et al. Influence of FUT2 and FUT3 polymorphisms and nasopharyngeal microbiome on respiratory infections in breastfed Bangladeshi infants from the microbiota and health study. mSphere. (2021) 6:e0068621. doi: 10.1128/mSphere.00686-21
89. Sakwinska O, Foata F, Berger B, Brüssow H, Combremont S, Mercenier A, et al. Does the maternal vaginal microbiota play a role in seeding the microbiota of neonatal gut and nose? Benef Microbes. (2017) 8:763–78. doi: 10.3920/BM2017.0064
90. Gagneux P, Cheriyan M, Hurtado-Ziola N, van der Linden EC, Anderson D, McClure H, et al. Human-specific regulation of α2-6-linked sialic acids. J Biol Chem. (2003) 278:48245–50. doi: 10.1074/jbc.M309813200
91. Lewis AL, Varki A. “Evolutionary considerations in studying the sialome: sialic acids and the host-pathogen interface”. In: von der Lieth CW, Luetteke T, Frank M, editors. Bioinformatics for Glycobiology and Glycomics: An Introduction. New York: John Wiley & Sons, Inc. (2009). p. 69–88.
92. Craft KM, Townsend SD. Mother knows best: deciphering the antibacterial properties of human milk oligosaccharides. Acc Chem Res. (2019) 52:760–8. doi: 10.1021/acs.accounts.8b00630
93. El-Hawiet A, Kitova EN, Kitov PI, Eugenio L, Ng KK, Mulvey GL, et al. Binding of Clostridium difficile toxins to human milk oligosaccharides. Glycobiology. (2011) 21:1217–27. doi: 10.1093/glycob/cwr055
94. Vigsnaes LK, Ghyselinck J, Van den Abbeele P, McConnell B, Moens F, Marzorati M, et al. 2‘FL And LNnT exert antipathogenic effects against C. difficile ATCC 9689 in vitro, coinciding with increased levels of Bifidobacteriaceae and/or secondary bile acids. Pathogens. (2021) 10:927. doi: 10.3390/pathogens10080927
95. Wiese M, Schuren FHJ, Smits WK, Kuijper EJ, Ouwens A, Heerikhuisen M, et al. 2'-Fucosyllactose Inhibits proliferation of clostridioides difficile ATCC 43599 in the CDi-screen, an in vitro model simulating clostridioides difficile infection. Front Cell Infect Microbiol. (2022) 12:991150. doi: 10.3389/fcimb.2022.991150
96. Alliet P, Vandenplas Y, Roggero P, Jespers SNJ, Peeters S, Stalens JP, et al. Safety and efficacy of a probiotic-containing infant formula supplemented with 2'-fucosyllactose: a double-blind randomized controlled trial. Nutr J. (2022) 21:11. doi: 10.1186/s12937-022-00764-2
97. Wang B. Sialic acid is an essential nutrient for brain development and cognition. Annu Rev Nutr. (2009) 29:177–222. doi: 10.1146/annurev.nutr.28.061807.155515
98. Witt W, von Nicolai H, Zilliken F. Uptake and distribution of orally applied N-acetyl-(14C)neuraminosyl-lactose and N-acetyl-(14C)neuraminic acid in the organs of newborn rats. Nutr Metab. (1979) 23:51–61. doi: 10.1159/000176241
99. Nöhle U, Schauer R. Metabolism of sialic acids from exogenously administered sialyllactose and mucin in mouse and rat. Hoppe Seylers Z Physiol Chem. (1984) 365:1457–67. doi: 10.1515/bchm2.1984.365.2.1457
100. Banda K, Gregg CJ, Chow R, Varki NM, Varki A. Metabolism of vertebrate amino sugars with N-glycolyl groups: mechanisms underlying gastrointestinal incorporation of the non-human sialic acid xeno-autoantigen N-glycolylneuraminic acid. J Biol Chem. (2012) 287:28852–64. doi: 10.1074/jbc.M112.364182
101. Yonekawa T, Malicdan MC, Cho A, Hayashi YK, Nonaka I, Mine T, et al. Sialyllactose ameliorates myopathic phenotypes in symptomatic GNE myopathy model mice. Brain. (2014) 137:2670–9. doi: 10.1093/brain/awu210
102. Kuntz S, Kunz C, Borsch C, Vazquez E, Buck R, Reutzel M, et al. Metabolic fate and distribution of 2´-fucosyllactose: direct influence on gut microbial activity but not on brain. Mol Nutr Food Res. (2019) 63:e1900035. doi: 10.1002/mnfr.201900035
103. Rudloff S, Kuntz S, Borsch C, Vazquez E, Buck R, Reutzel M, et al. Fucose as a cleavage product of 2’fucosyllactose does not cross the blood-brain barrier in mice. Mol Nutr Food Res. (2021) 65:e2100045. doi: 10.1002/mnfr.202100045
104. Cho S, Zhu Z, Li T, Baluyot K, Howell BR, Hazlett HC, et al. Human milk 3'-sialyllactose is positively associated with language development during infancy. Am J Clin Nutr. (2021) 114:588–97. doi: 10.1093/ajcn/nqab103
105. Oliveros E, Martin M, Torres-Espinola FJ, Segura-Moreno T, Ramirez M, Santos-Fandila A, et al. Human milk levels of 2’-fucosyllactose and 6’-sialyllactose are positively associated with infant neurodevelopment and are not impacted by maternal BMI or diabetic status. Henry J Nutr Food Sci. (2021) 4:100024.
106. Berger PK, Plows JF, Jones RB, Alderete TL, Yonemitsu C, Poulsen M, et al. Human milk oligosaccharide 2'-fucosyllactose links feedings at 1 month to cognitive development at 24 months in infants of normal and overweight mothers. PLoS One. (2020) 15:e0228323. doi: 10.1371/journal.pone.0228323
107. Willemsen Y, Beijers R, Gu F, Vasquez AA, Schols HA, de Weerth C. Fucosylated human milk oligosaccharides during the first 12 postnatal weeks are associated with better executive functions in toddlers. Nutrients. (2023) 15:1463. doi: 10.3390/nu15061463
108. Jorgensen JM, Young R, Ashorn P, Ashorn U, Chaima D, Davis JCC, et al. Associations of human milk oligosaccharides and bioactive proteins with infant growth and development among Malawian mother-infant dyads. Am J Clin Nutr. (2021) 113:209–20. doi: 10.1093/ajcn/nqaa272
109. Mudd AT, Dilger RN. Early-life nutrition and neurodevelopment: use of the piglet as a translational model. Adv Nutr. (2017) 8:92–104. doi: 10.3945/an.116.013243
110. Daniels VC, Monaco MH, Wang M, Hirvonen J, Jensen HM, Ouwehand AC, et al. Evaluation of 2’-fucosyllactose and Bifidobacterium longum subspecies infantis on growth, organ weights, and intestinal development of piglets. Nutrients. (2021) 14:199. doi: 10.3390/nu14010199
111. Golden RK, Sutkus LT, Bauer LL, Donovan SM, Dilger RN. Determining the safety and efficacy of dietary supplementation with 3'-sialyllactose or 6'-sialyllactose on growth, tolerance, and brain sialic acid concentrations. Front Nutr. (2023) 10:1278804. doi: 10.3389/fnut.2023.1278804
112. Monaco MH, Wang M, Pan X, Li Q, Richards JD, Chichlowski M, et al. Evaluation of sialyllactose supplementation of a prebiotic-containing formula on growth, intestinal development, and bacterial colonization in the neonatal piglet. Curr Dev Nutr. (2018) 2:nzy067. doi: 10.1093/cdn/nzy067
113. Obelitz-Ryom K, Bering SB, Overgaard SH, Eskildsen SF, Ringgaard S, Olesen JL, et al. Bovine milk oligosaccharides with sialyllactose improves cognition in preterm pigs. Nutrients. (2019) 11:1335. doi: 10.3390/nu11061335
114. Clouard C, Reimert I, Fleming SA, Koopmans SJ, Schuurman T, Hauser J. Dietary sialylated oligosaccharides in early-life may promote cognitive flexibility during development in context of obesogenic dietary intake. Nutr Neurosci. (2022) 25:2461–78. doi: 10.1080/1028415X.2021.1975877
115. Fleming SA, Chichlowski M, Berg BM, Donovan SM, Dilger RN. Dietary sialyllactose does not influence measures of recognition memory or diurnal activity in the young pig. Nutrients. (2018) 10:395. doi: 10.3390/nu10040395
116. Golden RK, Sutkus LT, Donovan SM, Dilger RN. Dietary supplementation of 3'-sialyllactose or 6'-sialyllactose elicits minimal influence on cognitive and brain development in growing pigs. Front Behav Neurosci. (2023) 17:1337897. doi: 10.3389/fnbeh.2023.1337897
117. Oliveros E, Vázquez E, Barranco A, Ramírez M, Gruart A, Delgado-García JM, et al. Sialic acid and sialylated oligosaccharide supplementation during lactation improves learning and memory in rats. Nutrients. (2018) 10:1519. doi: 10.3390/nu10101519
118. Pisa E, Martire A, Chiodi V, Traversa A, Caputo V, Hauser J, et al. Exposure to 3′sialyllactose-poor milk during lactation impairs cognitive capabilities in adulthood. Nutrients. (2021) 13:4191. doi: 10.3390/nu13124191
119. Hauser J, Pisa E, Arias Vásquez A, Tomasi F, Traversa A, Chiodi V, et al. Sialylated human milk oligosaccharides program cognitive development through a non-genomic transmission mode. Mol Psychiatry. (2021) 26:2854–71. doi: 10.1038/s41380-021-01054-9
120. Pisa E, Traversa A, Caputo V, Ottomana AM, Hauser J, Macrì S. Long-term consequences of reduced availability and compensatory supplementation of sialylated HMOs on cognitive capabilities. Front Cell Neurosci. (2023) 17:1091890. doi: 10.3389/fncel.2023.1091890
121. Sutkus LT, Joung S, Hirvonen J, Jensen HM, Ouwehand AC, Mukherjea R, et al. Influence of 2′-fucosyllactose and Bifidobacterium longum subspecies infantis supplementation on cognitive and structural brain development in young pigs. Front Neurosci. (2022) 16:860368. doi: 10.3389/fnins.2022.860368
122. Fleming SA, Mudd AT, Hauser J, Yan J, Metairon S, Steiner P, et al. Dietary oligofructose alone or in combination with 2′-fucosyllactose differentially improves recognition memory and hippocampal MRNA expression. Nutrients. (2020) 12:2131. doi: 10.3390/nu12072131
123. Oliveros E, Ramirez M, Vazquez E, Barranco A, Gruart A, Delgado-Garcia JM, et al. Oral supplementation of 2'-fucosyllactose during lactation improves memory and learning in rats. J Nutr Biochem. (2016) 31:20–7. doi: 10.1016/j.jnutbio.2015.12.014
124. Vazquez E, Barranco A, Ramirez M, Gruart A, Delgado-Garcia JM, Jimenez ML, et al. Dietary 2′-fucosyllactose enhances operant conditioning and long-term potentiation via gut-brain communication through the vagus nerve in rodents. PLoS One. (2016) 11:e0166070. doi: 10.1371/journal.pone.0166070
125. Fleming SA, Mudd AT, Hauser J, Yan J, Metairon S, Steiner P, et al. Human and bovine milk oligosaccharides elicit improved recognition memory concurrent with alterations in regional brain volumes and hippocampal mRNA expression. Front Neurosci. (2020) 14:770. doi: 10.3389/fnins.2020.00770
126. Zhang S, Chen F, Zhang Y, Lv Y, Heng J, Min T, et al. Recent progress of porcine milk components and mammary gland function. J Anim Sci Biotechnol. (2018) 9:77. doi: 10.1186/s40104-018-0291-8
127. Liu F, Tol AJC, Kuipers F, Oosterveer MH, van der Beek EM, van Leeuwen SS. Characterization of milk oligosaccharide and sialic acid content and their influence on brain sialic acid in a lean mouse model for gestational diabetes. Heliyon. (2024) 10:e24539. doi: 10.1016/j.heliyon.2024.e24539
128. Wang M, Radlowski EC, Li M, Monaco MH, Donovan SM. Feeding mode, but not prebiotics, affects colonic microbiota composition and volatile fatty acid concentrations in sow-reared, formula-fed, and combination-fed piglets. J Nutr. (2019) 149:2156–63. doi: 10.1093/jn/nxz183
129. Yuu EY, Bührer C, Eckmanns T, Fulde M, Herz M, Kurzai O, et al. The gut microbiome, resistome, and mycobiome in preterm newborn infants and mouse pups: lack of lasting effects by antimicrobial therapy or probiotic prophylaxis. Gut Pathog. (2024) 16:27. doi: 10.1186/s13099-024-00616-w
130. Wang M, Monaco MH, Hauser J, Yan J, Dilger RN, Donovan SM. Bovine milk oligosaccharides and human milk oligosaccharides modulate the gut microbiota composition and volatile fatty acid concentrations in a preclinical neonatal model. Microorganisms. (2021) 9:884. doi: 10.3390/microorganisms9050884
131. Autran CA, Kellman BP, Kim JH, Asztalos E, Blood AB, Spence ECH, et al. Human milk oligosaccharide composition predicts risk of necrotising enterocolitis in preterm infants. Gut. (2018) 67:1064–70. doi: 10.1136/gutjnl-2016-312819
Keywords: human milk oligosaccharides, pediatric nutrition, gut microbiota, pathogen inhibition, cognitive development
Citation: Wichmann A (2024) Biological effects of combinations of structurally diverse human milk oligosaccharides. Front. Pediatr. 12:1439612. doi: 10.3389/fped.2024.1439612
Received: 28 May 2024; Accepted: 14 October 2024;
Published: 5 November 2024.
Edited by:
Pedro Gutierrez-Castrellon, International Scientific Council for Probiotics A.C., MexicoReviewed by:
Sharon Marie Donovan, University of Illinois, Urbana-Champaign, United StatesAndrea Monteagudo-Mera, University of Reading, United Kingdom
Copyright: © 2024 Wichmann. This is an open-access article distributed under the terms of the Creative Commons Attribution License (CC BY). The use, distribution or reproduction in other forums is permitted, provided the original author(s) and the copyright owner(s) are credited and that the original publication in this journal is cited, in accordance with accepted academic practice. No use, distribution or reproduction is permitted which does not comply with these terms.
*Correspondence: Anita Wichmann, YW5pdGEud2ljaG1hbm4tZ3VzdGFmc3NvbkBkc20tZmlybWVuaWNoLmNvbQ==