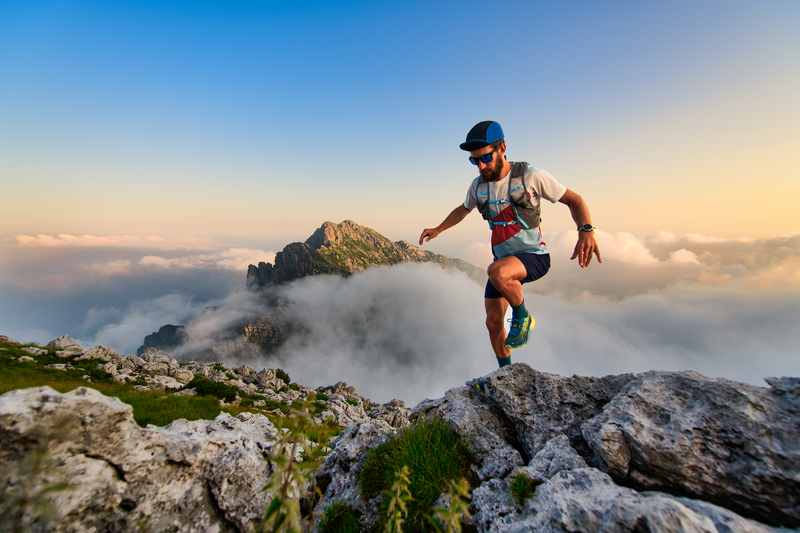
95% of researchers rate our articles as excellent or good
Learn more about the work of our research integrity team to safeguard the quality of each article we publish.
Find out more
REVIEW article
Front. Pediatr. , 09 February 2024
Sec. Pediatric Urology
Volume 12 - 2024 | https://doi.org/10.3389/fped.2024.1342906
The management of severe urethral stricture has always posed a formidable challenge. Traditional approaches such as skin flaps, mucosal grafts, and urethroplasty may not be suitable for lengthy and intricate strictures. In the past two decades, tissue engineering solutions utilizing acellular dermal matrix have emerged as potential alternatives. Acellular dermal matrix (ADM) is a non-immunogenic biological collagen scaffold that has demonstrated its ability to induce layer-by-layer tissue regeneration. The application of ADM in urethral reconstruction through tissue engineering has become a practical endeavor. This article provides an overview of the preparation, characteristics, advantages, and disadvantages of ADM along with its utilization in urethral reconstruction via tissue engineering.
The urethra is a tubular structure that connects the urinary bladder to the external environment. It primarily consists of two distinct cell types: epithelial cells and smooth muscle cells. In comparison to the female urethra, the male urethra is slender and can be anatomically divided into penile (cavernous), bulbous, membranous, and prostatic segments (1). There are multiple etiologies for urethral injury, including iatrogenic causes, infections, trauma, tumors, and congenital hypospadias (2, 3). Hypospadias is a common congenital malformation that occurs in approximately ∼1/150–1/300 live births (3). Following urethral injury, the organization and distribution of fibroblasts in normal tissues become disrupted, leading to the development of urethral strictures (4). Patients may experience abnormal urination, pain, urinary tract infections, and potential impairment of kidney function or overall quality of life. Anastomotic urethroplasty (AR), urethral dilation (UD), or direct vision internal urethrotomy (DVIU) are commonly employed treatment options for managing urethral strictures. Steenkamp et al. (5) described changes in sexual function and reproductive sensitivity following AR repair for bulbous urethral stricture; temporary decline in erectile and ejaculatory function can occur as a result of AR intervention. Moreover, both UD and DVIU exhibit reduced efficacy with increasing length of the stricture. Historically oral mucosal grafts considered as “gold standard” materials for repairing urethral strictures or defects due to their favorable therapeutic outcomes; autologous replacement tissues such as penile or scrotal skin grafts bladder mucosa grafts, and oral mucosal grafts often give rise to various complications at the donor site (6).
In the past two decades, with the advancement of tissue engineering, various strategies have been proposed to address the challenge of limited sources of autogenous tissue for urethral reconstruction (7, 8). Tissue engineering is a scientific discipline that applies principles from cell biology and engineering to develop active biological substitutes capable of repairing damaged tissues and enhancing their functionality. Its fundamental principle involves creating a three-dimensional complex composed of cells and biological materials (9). Numerous biodegradable materials are currently under investigation, including acellular matrix, polylactic acid (PLA), polyglycolic acid (PGA), as well as PLA-PGA copolymers (10–12). Hu et al. (13) described the biocompatibility and application of polylactic acid-glycolic acid copolymer (PLGA) and PLGA-collagen scaffolds in canine urethral reconstruction. Although urothelial cells implanted on both scaffolds exhibited satisfactory growth, resulting in multiple layers formation within proximal and distal segments of the reconstructed urethra, post-surgical complications such as strictures and urethral discontinuities were observed. It is evident that the performance of PLGA and PLGA-collagen scaffolds was suboptimal. The acidic degradation products commonly associated with synthetic polymeric materials, such as PLGA, may impede proper cellular growth in the surrounding environment (14). However, acellular matrices closely resemble the native extracellular matrix, making acellular dermal matrix (ADM) an innovative treatment approach to overcome these challenges in urethral reconstruction. This article presents a comprehensive review on the preparation and characteristics of ADM materials, along with their research advancements in tissue engineering for urethral reconstruction.
ADM is produced using acellular techniques that involve the enzymatic removal of the epidermis and complete elimination of residual cells from the dermis, resulting in an acellular collagen-elastin biomaterial matrix with minimal antigenicity and exceptional biological and mechanical properties (15). Various physical, chemical, and biological methods are currently employed for preparing ADM (Refer to Table 1).
The physical method disrupts the cell membrane, induces cell lysis, and facilitates the transport of cellular debris (16–19). Commonly employed physical methods include repeated cycles of freezing and thawing, ultrasonic vibration, supercritical carbon dioxide, high hydrostatic pressure treatment, mechanical compression, and electroporation. In recent years, numerous studies have attempted to utilize physical techniques in facilitating decellularization and demonstrated its feasibility.
The freeze-thaw method can be optimized by increasing the temperature differential or adjusting the number of cycles, thereby enhancing its efficiency. Multiple freeze-thaw cycles may be employed throughout the decellularization process without compromising matrix protein preservation (20). It is important to note that complete removal of nuclear material should not be reported, as the formation of ice crystals during freezing and thawing disrupts cell membranes in tissues or organs (21). While this process effectively preserves biochemical composition and mechanical properties, insufficient removal of genetic material may lead to potential immunological rejection. To disrupt cell membranes, high hydrostatic pressure exceeding 600 MPa is applied; however, it should be acknowledged that such high pressure can induce protein deformation, as evidenced by observed collagen and elastic fiber deformations in decellularized blood vessels. Consequently, this results in a reduction of approximately 50% in the tensile strength of fibers compared to their original tissue state (22).
Supercritical carbon dioxide exhibits low viscosity and high transport properties, allowing for minimal damage to tissue mechanical properties when passed through at a controlled speed similar to the critical point. Various studies have demonstrated its ability to induce non-deformability in tissue fibers (23). However, its poor solubility for polymers and polar substances is a drawback that can be partially addressed with entraining agents such as ethanol, though this may introduce new impurities (24).
Nonthermal irreversible electroporation, typically achieved through the application of microsecond electrical pulses, disrupts the transmembrane electrical potential and induces micropore formation in the plasma membrane. Ultimately, this leads to cell death by perturbing its steady-state electrical balance while largely preserving the three-dimensional structure of tissues and organs. A novel porcine acellular dermal matrix (PADM) prepared by Xia et al. (25), utilizing laser micropore technology, was demonstrated to be both safe and effective in animal transplantation.
Chemical decellularization methods commonly employ a variety of detergents, including surfactants, acids, bases, hypertonic and hypotonic solutions, and chelating agents. Surfactants can be classified based on their charge as ionic, nonionic or zwitterionic; all of which have the ability to disrupt cell membranes and degrade DNA. Hogg et al. (26) utilized chemical treatment to remove the epidermis, followed by immersion in a hypotonic buffer solution for cell dissolution and subsequent addition of nuclease buffer to eliminate residual nucleic acid substances. This approach achieved rapid and efficient preparation of ADM. Draguňova et al. (27) also developed an effective method for preparing decellularized matrices using only a few chemicals with minimal procedures. Bera et al. (28) employed a method based on hypotonic/hypertonic saline solution to decellularize goat skin before formulating an ADM bio-ink and 3D bioprinting it. Ultimately, this resulted in an ADM with exceptional mechanical properties and cell adhesion.
Organisms (enzymes) selectively cleave the arginine carboxyl side of cell adhesion proteins, resulting in detachment and lysis of cells from the adjacent matrix. Commonly employed enzymes include trypsin, collagenase, nuclease, thermophilic protease, and dispase (29, 30). Excessive utilization of enzymes may lead to the degradation of natural matrix components, including collagen, elastin, and glycosaminoglycans (31). Following enzymatic decellularization, thorough rinsing of the tissue is essential to eliminate or neutralize any residual enzyme components and cellular debris (32). Therefore, it is recommended to use trypsin for short-term periods to prevent damage to the matrix components. It should be noted that trypsin does not exhibit cytotoxic effects on bioengineered materials which are crucial for in vitro cell culture.
As each method possesses its own set of advantages and disadvantages, a combination of methodologies can be employed to explore a more efficacious acellular process and prepare an ideal ADM with enhanced decellularization efficiency. While ionic SDS has demonstrated commendable efficacy in eluting cells and removing cellular components, it also leads to the degradation of the extracellular matrix due to the elution of other substances. Triton X-100, a nonionic eluent, exhibits inefficacy against the eluted cells while causing minimal damage to the extracellular matrix. Although enzymatic hydrolysis can selectively eliminate DNA and other cellular components, its sole effect on elution is suboptimal and may result in certain damages to blood vessels and ultrastructure. Therefore, combining physical, chemical, and enzymatic methods can yield superior outcomes in terms of decellularization.
The sources of ADM can vary, including human, porcine, bovine, ovine or piscine origins (33–37). Human-derived ADMs require tissue screening for infectious pathogens such as HIV, hepatitis, and syphilis. However, the limited availability and high costs significantly restrict their utilization (38). Additionally, the presence of residual heterologous antigens like α-1,3-galactose (α-Gal) may trigger an immune response. Fish skin-derived ADM lacks the α-Gal antigen and poses a low risk of viral infection, making it an economical and sustainable source (39). Nevertheless, the primary constituents of fish skin-derived ADM are generally less thermally stable and more susceptible to degradation compared to human-derived ADMs (40). Bovine-derived ADM offers broader sourcing options and lower costs in comparison with human-derived alternatives. It is considered a favorable choice for repairing eyelid contractures due to its good short-term results; however, there is insufficient literature on the long-term safety and effectiveness of bovine ADM grafts available currently (41). Given the hist skin and human skin as well as its economic feasibility factor into consideration in clinical practice settings; pigs have become the primary source for xenogeneic ADM.
ADM products have been developed to mimic the extracellular matrix (ECM) of the host. Table 2 provides an overview of the currently available and widely used ADM products in the market (33, 42–44). Among them, Alloderm and Cortiva are two commonly utilized commercial ADM products (45). The natural animal dermal matrix offers abundant sources and low cost, which presents significant advantages for clinical development and holds immense application prospects.
ADM removes cellular elements that have potential immunogenicity while retaining the original extracellular matrix as a supportive framework (46). This three-dimensional structure comprises biomaterials such as collagen, elastin, and proteoglycan that serve as an ideal substrate for epithelial cell growth, fibroblast proliferation, and neovascularization post-transplantation (47). ADM can be regarded as a collagen-based scaffold with preserved three-dimensional structure obtained through physical-chemical-biological methods involving elimination of epidermal cells and other constituents from natural skin tissue. Consequently, ADM possesses slight variations in its physicochemical characteristics compared to native tissues; particularly exhibiting inferior mechanical properties along with reduced resistance against enzymatic degradation (48).
The xenogeneic ADM undergoes a process where immunogenic cells and skin appendages are removed, resulting in the presence of mainly collagen, laminin, hyaluronic acid, elastin, keratan sulfate, fibronectin, and a small amount of cell-associated proteins along with abundant type I collagen (49, 50). Being a xenogeneic protein, the telopeptide of this however, numerous experiments have demonstrated that the immunogenicity of collagen is relatively weak. The primary constituent found in ADM is collagen I which exhibits excellent histocompatibility (51). It should be noted that xenogeneic ADMs elicit a more severe inflammatory response compared to allografts possibly due to the presence of basement membrane constituents such as collagen IV and laminin in the superficial dermis.
In practical applications, ADM often requires properties similar to native tissue in order to meet the specific requirements. Therefore, for better application performance, a certain degree of cross-linking modification is advantageous in further reducing the immune response triggered by the material. Commonly used modification methods include physical and chemical approaches, with thermal modification, radiation irradiation, and drilling being the primary physical methods. Chemical methods involve crosslinking modifications using glutaraldehyde, epoxides, carbodiimide, nanomaterials, dialdehyde polysaccharides and others. Previous studies have demonstrated that cross-linking can effectively decrease immunogenicity by inducing covalent bond formation between amino acid residues in ADM and collagen molecules which can mask tissue antigenicity and reduce immune responses (52).
Chen et al. (53) employed chemical cross-linking and the synergistic binding of ADM and chitosan under freezing conditions to fabricate a composite scaffold with dual physical and chemical, thereby significantly enhancing the survival rate of autologous rat skin grafts. Feng et al. (54) synthesized glutaraldehyde-modified heparin with cross-linked active aldehyde groups, which was subsequently cross-linked with porcine ADM and chemically modified to enhance its anticoagulant performance. The results demonstrated that the modified ADM exhibited superior thermal stability and biocompatibility, particularly in terms of its enhanced anticoagulant and anti-platelet adhesion properties, leading to a reduced incidence of coagulation, thrombocytopenia, and bleeding. Improved properties can be achieved through the utilization of newly developed nanoengineered ECM scaffold technologies (55, 56). The complete elimination of immunogenicity in ADM cannot be guaranteed solely by removing cellular components alone. The rate at which residual cellular components are removed from dermis can be enhanced by increasing temperature differentials and altering freeze-thaw cycles (57).
The development of ADM aims to harness the properties of native ECM and facilitate tissue regeneration in practical clinical settings. ADM exhibits properties such as toughness, elasticity, water retention, and robust mechanical force buffering (58). It can serve as a dermal scaffold, facilitating the rapid migration and infiltration of various host cells including fibroblasts, myofibroblasts, lymphocytes, macrophages, granulocytes, mast cells and more (47, 58,59). Following inflammatory cell infiltration, there increased levels of collagen and elastin production leading to the formation of new connective tissue and blood vessels (61). ADM demonstrates excellent biological strength and effectively supports fixation while reducing tension during cell proliferation (58).
Secondly, ADM harbors a multitude of signaling factors, such as vascular endothelial growth factor (VEGF), transforming growth factor β (TGF-β), basic fibroblast growth factor (BFGF), and others (62). These growth factors have demonstrated the ability to retain their biological activity post-sterilization and during extended storage, while also promoting cell adhesion, proliferation, differentiation, and tissue formation (59). Additionally, ADM exhibits low antigenicity and excellent histocompatibility, creating an advantageous environment for the growth and proliferation of seed cells (63, 64). Following implantation in the body, ADM gradually undergoes degradation and is subsequently replaced by new tissue. Compared to other biological materials, ADM provides ample space for normal cell growth, making it a suitable candidate for use as a biological injection material (65–67). ADM can be considered as an “off-the-shelf” natural biomaterial that effectively addresses the issue of donor site insufficiency within the host itself (68). In summary, ADM scaffolds possess a complex composition consisting of diverse molecules that play pivotal roles in the process of tissue regeneration.
Traditional techniques for urethral reconstruction involve the utilization of external genital skin, oral mucosa, and bladder mucosa for repair. However, this approach a risk of necrosis in the transplanted skin and mucosal tissue. Moreover, it fails to achieve functional restoration of the urethral epithelium (69, 70). Tissue engineering techniques offer the advantage of not necessitating large quantities of autologous tissue, particularly in cases where an increase in urethral length is required instead of autologous tissue harvesting. ADM is a three-dimensional extracellular matrix framework derived from the skin that serves as a reparative material. Although initially used in patients with severe burns, ADM has recently gained widespread application across various clinical fields including plastic surgery, breast surgery, head and neck surgery, otolaryngology, urology, oral surgery abdominal wall reconstruction gynecology and ophthalmology (71–77). ADM has gradually become a popular choice for urethral repair and reconstruction in tissue engineering. Currently scaffold-based urethral reconstruction emphasizes the use of either cell-free or cell-seeded ADMs.
In the initial stage, Lin et al. (78) successfully achieved the reconstruction of 16 male urethral diseases by suturing ADM into a tubular structure, which included 13 complicated urethral strictures and one urethral hypospadias. The average length of urethral replacement was 4.7 cm, ranging from 2 to 10 cm. Postoperative urethrography demonstrated excellent integration of ADM with the surrounding tissue, while urethroscope examination revealed complete epithelialization of the graft urethra. No postoperative infections or rejections were observed; however, three cases experienced postoperative urethral strictures that were effectively managed through dilatation or incision. ADM is tentatively considered an ideal substitute for the urethra. On the other hand, Liu et al. (79) implanted sutured allogeneic ac dermal matrix into a tubular structure to repair 5.0 cm long urethral defects in 15 dogs. After a follow-up period of 24 weeks, no infections or anastomotic stenosis occurred, and there was no histological evidence of rejection with significant infiltration of inflammatory cells at any time point during evaluation. However, it should be noted that achieving normal structural approximation in the central area of ADM after transplantation requires an extended duration. Yang et al. (80) surgically treated five patients with anterior urethral strictures ranging from 5 to 9 cm in length by excising the affected segment and replacing it with tubular ADM. Three patients achieved successful voiding after catheter removal, while the remaining two underwent intermittent urethral dilation for a period of 2 months. All five patients demonstrated satisfactory voiding function 3 months post-operation, as confirmed by urethrography which revealed excellent continuity of the reconstructed urethra. ADM as a substitute for autologous tissue is a safe, effective, invasive, and cost-efficient method to avoid the trauma associated with harvesting autologous tissue in previous procedures. However, long-term outcomes regarding urethral stenosis remain uncertain.
Tang et al. (81) conducted a review of 49 patients with urethral strictures ranging from 1.5 to 3.8 cm in length who underwent urethroplasty with ADM and were followed up for 12 months. Cystoscopy revealed satisfactory coverage of the urethral epithelial mucosa in 11 cases. Infection occurred in two cases within the postoperative period of 2–4 weeks, while one case developed a urethral fistula at 5 months and seven cases experienced non-infective urethral strictures between 6 and 10 months after surgery. In this study, one out of two patients who developed postoperative infection had a stricture length of 3.0 cm, while the patient with a urethral fistula also presented with a stricture length close to 3.0 cm. Therefore, the use of ADM without seeded cells in an Onlay method for urethral reconstruction may have limited therapeutic efficacy for strictures longer than 3.0 cm. Additionally, the health status of the urethral bed should also be taken into consideration (82). El-Kassaby et al. (83) reported that acellular matrix would be suitable for use in surgical treatment of early strictures with an apparently healthy urethral bed and minimal spongiofibrosis. Primary ADM should not be performed in patients with preoperative infection complicated by urethral stones due to long-term stone irritation and poor local mucosal conditions, which increase the risk of infection or poor healing outcomes (81). In general, ADM represents a novel approach for material selection in urethral repair and reconstruction that offers benefits such as straightforward implementation, convenience, minimal invasiveness, and no additional sampling.
The most direct tissue engineering strategy for urethral reconstruction involves the utilization of natural or synthetic cell-free scaffolds, which are subsequently infiltrated with host cells and eventually degraded to form new tissue. The successful implantation of cell-free grafts relies on a healthy urethral bed, sufficient vascular supply, and the absence of spongiform fibrosis; otherwise, there is a risk of graft atrophy, inadequate tissue regeneration, and fibrosis. Considering that urethral stricture is characterized by ischemic cavernous fibrosis as a pathological process, it may impact the quality of the newly constructed urethra. Therefore, this simplified procedure can only be considered as an option for patients with short to moderate urethral defects. Clinical data demonstrates a high failure rate in treating urethral strictures longer than 4.0 cm.
The utilization of acellular fetal skin offers potential advantages compared to other acellular matrices. Sobhani et al. (84) employed an early gestational age fetal ADM scaffold for repairing hypospadias in a rabbit model. The decellularized fetal skin demonstrated favorable angiogenesis and re-epithelialization, resulting in reduced postoperative complications and shortened operation time, thereby establishing a solid foundation for treating complex hypospadias. The composition of the fetal dermis plays a critical role in scar-free wound healing and reducing complications associated with fetal dermal grafting (85). There is a significant disparity between the extracellular matrix of adult skin and fetal skin, primarily due to type Ⅰ collagen being the primary component. However, compared to adult skin, fetal skin exhibits higher levels of type Ⅲ collagen, type Ⅰ collagen, and cutin (86), which may explain its suitability as a “ready-made” material for tissue engineering urethra. Furthermore, recent studies have shown that utilizing ADM grafts in the second stage repair of hypospadias for ventral side elongation effectively corrects ventral curvature without increasing the risk of urethroplasty complications while also providing aesthetic benefits (87).
In order to enhance the efficacy of ADM materials, scholars have compared various preparation methods. Morgante et al. (88) conducted a study on porcine urethral repair using ADM and compared two types of acellular matrices: full-thickness porcine bladder matrix (PABM) and commercially derived cross-linked porcine dermal matrix (Permacol™). Anatomical and immunohistochemical evaluations were performed 3 months after the operation. The PABM graft showed complete fusion, while the Permacol™ graft remained palpable. Immunohistochemical analysis demonstrated a non-inflammatory remodeling response to both biomaterials. PABM implants displayed extensive infiltration of interstitial cells and neovascularization with significantly higher cell density than Permacol™. We believe that the poor growth of cells in Permacol™ may be attributed to variations in decellularization methods employed by different research groups, resulting in differential effects on cell removal, extracellular matrix composition, and subsequent tissue remodeling. It is evident that natural acellular matrices hold great promise as biomaterials for reconstructive and regenerative surgery; however, their physical and biological properties can potentially be influenced by chemical or radiation exposure. To fully realize the clinical potential of ADMs, it is crucial to conduct comprehensive evaluations of their safety and efficacy both in vitro and in vivo while also establishing standardized protocols for their utilization in clinical practice (Table 3).
Cell seeding with acellular dermal matrix is more suitable for tubular implants (92). The biological scaffolds are seeded with different types of cells, which can effectively promote cell expansion by releasing growth factors, cytokines, etc., thereby improving the mechanical properties of the grafts (93). Studies have demonstrated that in animal models, cell-inoculated matrix have been compared with uninoculated matrix, and the results are better with the former, which provides a robust empirical foundation for clinical research (94). The utilization of cell-based grafts has exhibited a remarkable 5.7-fold increase in long-term success rates compared to unseeded grafts (95). Importantly, incorporating cells within grafts has been shown to significantly reduce the incidence of stenosis, fistula formation, and infection. This can be attributed to the potential promotion of vascularization and urothelial barrier formation by cellular components, effectively mitigating local inflammation and fibrosis resulting from urine leakage. Autologous stem cells hold immense promise in urethral tissue engineering, with several scholars exploring the feasibility of combining ADM with stem cells. Fu et al. (96) employed bone marrow mesenchymal stem cells seeded on ADM, subsequently repairing rabbit urethral injuries. Histological examination using HE staining revealed that over time, the reconstructed area in the experimental group exhibited a transition from a single layer of urethral epithelial cells to multiple layers, closely resembling the structure of normal urethral tissue at 12 weeks post-operation. In contrast, the control group without the use of bone marrow mesenchymal stem cells displayed inferior urethral regeneration. Urethrography demonstrated an absence of urinary fistulas and urethral strictures or other complications in the experimental group, while such complications were observed in the control group. These findings indicate that combining bone marrow mesenchymal stem cells with ADM yields superior outcomes for repairing urethral injuries compared to using ADM alone. Therefore, ADM provides support for cell adhesion, growth, and proliferation, facilitating material exchange and signal transduction pathway formation. Both cellular-matrix interaction and tissue engineered graft-host tissue environment interaction are crucial factors for successful outcomes in urethral reconstruction. However, the mechanism by which seed cells participate in the regeneration process after binding to ADM remains unclear. Although preclinical animal studies tend to suggest that cell-bound ADM is more effective with fewer complications, these findings have not been substantiated by a limited number of clinical studies.
Currently, there is a limited number of clinical reports available on the construction of urethra using cell-loaded ADM grafts. Liu et al. (79) sutured allogeneic acellular dermal matrix into a tube to repair two patients with urethral defects measuring 12.0 cm and 12.7 cm in length, respectively. Urethral mucosa homogenate and bladder mucosa homogenate were applied on the inner surface of the ADM cavity, respectively. No anastomotic stenosis was observed. In the clinical application of long segment urethral reconstruction using ADM, the repair process relies on host cell regeneration at both ends, resulting in time-consuming procedures. However, by utilizing homogenization seeding of host urothelium and multicentric growth of epithelium based on nutrients infiltrated into the ADM, it is possible to effectively shorten the repair time. Fossum et al. (97) conducted a study in which autologous urethral epithelial cells were cultured in vitro and subsequently transplanted onto ADM for surgical treatment of 6 patients with severe hypospadias, aged between 14 and 44 months. All patients underwent a two-stage surgical approach. Initially, urothelial cells were obtained through bladder lavage and then seeded onto ADM. In the second operation, an ADM scaffold containing urothelial cells was implanted to construct a new urethra. During the follow-up period of 3.5–5 years, one patient experienced partial stenosis without receiving any specific treatment, one patient developed proximal anastomotic obstruction, and two patients developed urinary fistula requiring surgical correction. Urethroscopy performed on all patients revealed widening of the newly constructed urethra, while biopsy results from three patients indicated that the inner mucosa consisted of urothelial cells. The authors Bhargava et al. (82) performed surgical treatment on five patients with urethral strictures ranging from 4 to 11 cm buccal mucosa keratinocytes and fibroblasts on ADM. Buccal mucosa biopsies were obtained from each patient for cell collection. These grafts were utilized for two-stage procedures (n = 3) and urethroplasty (n = 2). Results revealed that two patients experienced complications of urethral fibrosis and constriction, resulting in complete or partial removal of the graft. Three other patients required internal fixation after a follow-up period of 33 months. The application of tissue engineering techniques for implanting expanded cells on ADM can effectively enhance urethral tissue regeneration and accelerate lesion healing, making it particularly suitable for patients with long and complex urethral strictures or defects. However, the optimal conditions for cell differentiation and maturation in cell-loaded ADM remain unclear, and there are stringent requirements for in vitro cell culture, especially when considering human treatment. Once a tissue-engineered urethra is successfully constructed in vitro, timely scheduling of the implantation procedure is crucial to prevent graft failure. Additionally, it should be noted that using cell-inoculated matrices would result in higher overall costs compared to using cell-free matrices.
Another aspect that has received limited attention in the design of tissue engineered urethral scaffolds is the prevention of urinary fistulas. Recent research indicates that the utilization of ADM derived from bovine skin as a coverage material for proximal hypospadias repair can effectively decrease the occurrence of urinary fistula formation (89). Wu et al. (91) investigated the use of ADM in hypospadias repair and demonstrated a significant reduction in urinary fistula complications without an increased risk of infection and urethral stricture. Given the detrimental effects of urine on the cellular components of tissue-engineered urethra, it is crucial for the scaffold to possess sufficient impermeability as an isolation barrier. Furthermore, appropriately modified ADM materials can effectively decrease the incidence of associated complications and other adverse reactions.
Wang et al. (90) utilized bovine ADM loaded with collagen binding vascular endothelial growth factor (CBD-VEGF) for the repair of canine urethral injury. A few months later, the group with urethral injury was compared to the groups receiving ADM implantation and CBD-VEGF modified ADM implantation, respectively. The findings revealed that while the control group experienced urethral stricture and diverticulum, one case in the ADM group developed urinary fistula. However, no associated complications or adverse reactions were observed in the CBD-VEGF modified ADM implantation group. Importantly, ADM effectively prevented both urethral stricture and diverticulum when compared to the control group, demonstrating its efficacy in averting these conditions. Notably, combining ADM with growth factors promotes functional vascular system formation, thereby maintaining extracellular matrix metabolism balance and facilitating urethra remodeling without inducing severe inflammation or scar hyperplasia (Table 4).
Given the limited availability of tissue, coupled with a high incidence rate and challenges in achieving complete structural and functional restoration of the urethra, treatment outcomes for long urethral strictures remain unsatisfactory. ADM-based tissue engineering solutions have emerged as promising alternatives. ADM is derived from natural extracellular matrix (ECM), exhibiting exceptional biocompatibility along with appropriate mechanical properties and controlled non-toxic degradability. ADM creates a favorable microenvironment conducive to nurturing urethral parietal cell components while significantly enhancing the development of tissue-engineered substitutes for the urethra. However, further investigation is warranted in refining preparation technologies aiming to more accurately replicate ADM's inherent extracellular environment. Additionally, it is imperative to implement suitable modifications including integration of bioactive molecules.
The acellular allogeneic dermal matrix is highly versatile and readily available as an off-the-shelf material. It is the most commonly utilized scaffold type in clinical practice, devoid of cells. In terms of surgical approach, inlay grafting should be preferred over tubular reconstruction due to the latter's high failure rate. This is primarily attributed to the limited extent for adequate tissue regeneration on the stroma from the urethral wall boundary, which has been reported to be approximately 1.5 cm. We consider acellular allogeneic dermal matrix without cells as a valuable alternative when there is a scarcity of tissue sources, increased risk of donor site complications, and unsatisfactory outcomes in treating long urethral strictures. On the contrary, recent reports have highlighted the utilization of cell-seeded matrices in urethral reconstruction, indicating advancements in cell biology and biomaterials as scaffolds. Despite not fully replicating the intricate ECM microenvironment, animal studies on tissue-engineered urethra have successfully incorporated growth factors and cell co-culture. However, there are only three documented cases of cell-seeded scaffolds being used for human urethroplasty with a limited number of patients. Only 2 study that used a tubularized ADM as a construct has been published in the literature. In two cases where patients had urethral defects longer than 12.0 cm, repair was performed using acellular allogeneic dermal matrix seeded with homogenized urethral mucosa and bladder mucosa. Notably, no instances of postoperative urethral stricture were observed among these patients—an occurrence rarely reported in clinical practice (79). Additionally, acellular dermis seeded with keratinocytes and fibroblasts was employed to treat five patients with urethral stricture; however, all experienced recurrent strictures (92). The failure can be attributed to the progressive and incurable nature of lichen sclerosis itself. Additionally, other contributing factors to this outcome may include the preparation of acellular dermal matrix, selection of cell type for inoculation, and surgical technique employed. A study involving six children underwent hypospadias repair using urothelial cells inoculated into acellular dermis (94). In this study, one child required surgical intervention due to urethral restenosis, while two other patients underwent correction for urethral fistula. Human studies utilizing acellular allogeneic dermal matrices seeded with cells failed to replicate the promising results observed in animal models. However, we discovered that applying ADM coverage during urethroplasty significantly reduced the incidence of urinary fistula after hypospadias surgery—an aspect that has been relatively overlooked. In conclusion, cell scaffolds seem to hold promising prospects in the future. In particular, tubular scaffolds constructed from co-cultured cells may be a more appropriate orientation for TE urethral reconstruction.
The treatment of urethral stricture depends on a variety of factors, including patient age, etiology, length and location of stricture, preoperative intervention, and surgical experience. The results of clinical studies of ADM Tissue Engineering grafts remain uncertain due to the inadequacy of available trials. However, through the combination of ADM with an optimized decellularization protocol, novel nanoengineering technology, and 3D bioprinting technology, it holds great promise as a material for tissue-engineered urethral repair. The utilization of 3D bioprinting can simplify the creation of seeded tubular urethral structures, enhance patient-specific design options, and improve the efficiency of generating tissue-engineered urethra.
JA: Writing – original draft. LY: Conceptualization, Writing – review & editing. SC: Formal Analysis, Writing – review & editing. ZH: Funding acquisition, Writing – review & editing.
The author(s) declare that no financial support was received for the research, authorship, and/or publication of this article.
The authors declare that the research was conducted in the absence of any commercial or financial relationships that could be construed as a potential conflict of interest.
All claims expressed in this article are solely those of the authors and do not necessarily represent those of their affiliated organizations, or those of the publisher, the editors and the reviewers. Any product that may be evaluated in this article, or claim that may be made by its manufacturer, is not guaranteed or endorsed by the publisher.
1. Sam P, Jiang J, Leslie SW, LaGrange CA. Anatomy, abdomen and pelvis, sphincter urethrae. StatPearls [Internet]. Treasure Island, FL: StatPearls Publishing (2024). PMID: 29494045
2. Krughoff K, Shapiro J, Peterson AC. Pelvic fracture urethral distraction defect. Urol Clin North Am. (2022) 49(3):383–91. doi: 10.1016/j.ucl.2022.04.003
3. Leslie SW, Nelson Q, Baker J. Urethral injury. StatPearls [Internet]. Treasure Island, FL: StatPearls Publishing (2024). PMID: 32119462
4. Flynn H, Ong M, De Win G, Desai D. Narrowing in on urethral strictures. Aust J Gen Pract. (2021) 50(4):214–8. doi: 10.31128/AJGP-03-20-5280
5. Steenkamp JW, Heyns CF, de Kock ML. Internal urethrotomy versus dilation as treatment for male urethral strictures: a prospective, randomized comparison. J Urol. (1997) 157(1):98–101. doi: 10.1016/S0022-5347(01)65296-0
6. Mangera A, Patterson JM, Chapple CR. A systematic review of graft augmentation urethroplasty techniques for the treatment of anterior urethral strictures. Eur Urol. (2011) 59(5):797–814. doi: 10.1016/j.eururo.2011.02.010
7. Abbas TO, Mahdi E, Hasan A, AlAnsari A, Pennisi CP. Current status of tissue engineering in the management of severe hypospadias. Front Pediatr. (2018) 5:283. doi: 10.3389/fped.2017.00283
8. Ashammakhi N, GhavamiNejad A, Tutar R, Fricker A, Roy I, Chatzistavrou X, et al. Highlights on advancing frontiers in tissue engineering. Tissue Eng Part B Rev. (2022) 28(3):633–64. doi: 10.1089/ten.TEB.2021.0012
9. Guan Y, Yang B, Xu W, Li D, Wang S, Ren Z, et al. Cell-derived extracellular matrix materials for tissue engineering. Tissue Eng Part B Rev. (2022) 28(5):1007–21. doi: 10.1089/ten.TEB.2021.0147
10. Braccini S, Pecorini G, Chiellini F, Bakos D, Miertus S, Frecer V. Adhesion of fibroblast cells on thin films representing surfaces of polymeric scaffolds of human urethra rationalized by molecular models of integrin binding: cell adhesion on polymeric scaffolds for regenerative medicine. J Biotechnol. (2020) 324:233–8. doi: 10.1016/j.jbiotec.2020.11.001
11. Shahverdi M, Seifi S, Akbari A, Mohammadi K, Shamloo A, Movahhedy MR. Melt electrowriting of PLA, PCL, and composite PLA/PCL scaffolds for tissue engineering application. Sci Rep. (2022) 12(1):19935. doi: 10.1038/s41598-022-24275-6
12. Guzmán-Soria A, Moreno-Serna V, Canales DA, García-Herrera C, Zapata PA, Orihuela PA. Effect of electrospun PLGA/collagen scaffolds on cell adhesion, viability, and collagen release: potential applications in tissue engineering. Polymers (Basel). (2023) 15(5):1079. doi: 10.3390/polym15051079
13. Hu J, Ai B, Zhu S, Wang Z, Xia H, Jia W. Electrospun PLGA and PLGA/gelatin scaffolds for tubularized urethral replacement: studies in vitro and in vivo. J Biomater Appl. (2022) 36(6):956–64. doi: 10.1177/08853282211030904
14. Mahar R, Chakraborty A, Nainwal N, Bahuguna R, Sajwan M, Jakhmola V. Application of PLGA as a biodegradable and biocompatible polymer for pulmonary delivery of drugs. AAPS PharmSciTech. (2023) 24(1):39. doi: 10.1208/s12249-023-02502-1
15. Petrie K, Cox CT, Becker BC, MacKay BJ. Clinical applications of acellular dermal matrices: a review. Scars Burn Heal. (2022) 8:20595131211038313. doi: 10.1177/20595131211038313
16. Mousavi MS, Amoabediny G, Mahfouzi SH, Safiabadi Tali SH. Enhanced articular cartilage decellularization using a novel perfusion-based bioreactor method. J Mech Behav Biomed Mater. (2021) 119:104511. doi: 10.1016/j.jmbbm.2021.104511
17. Lv Y, Wang H, Li G, Zhao B. Three-dimensional decellularized tumor extracellular matrices with different stiffness as bioengineered tumor scaffolds. Bioact Mater. (2021) 6(9):2767–82. doi: 10.1016/j.bioactmat.2021.02.004
18. Schneider KH, Rohringer S, Kapeller B, Grasl C, Kiss H, Heber S, et al. Riboflavin-mediated photooxidation to improve the characteristics of decellularized human arterial small diameter vascular grafts. Acta Biomater. (2020) 116:246–58. doi: 10.1016/j.actbio.2020.08.037
19. Nie X, Chuah YJ, Zhu W, He P, Peck Y, Wang DA. Decellularized tissue engineered hyaline cartilage graft for articular cartilage repair. Biomaterials. (2020) 235:119821. doi: 10.1016/j.biomaterials.2020.119821
20. Crapo PM, Gilbert TW, Badylak SF. An overview of tissue and whole organ decellularization processes. Biomaterials. (2011) 32(12):3233–43. doi: 10.1016/j.biomaterials.2011.01.057
21. Gupta SK, Mishra NC, Dhasmana A. Decellularization methods for scaffold fabrication. Methods Mol Biol. (2018) 1577:1–10. doi: 10.1007/7651_2017_34
22. Funamoto S, Nam K, Kimura T, Murakoshi A, Hashimoto Y, Niwaya K, et al. The use of high-hydrostatic pressure treatment to decellularize blood vessels. Biomaterials. (2010) 31(13):3590–5. doi: 10.1016/j.biomaterials.2010.01.073
23. Sevastianov VI, Basok YB, Grigoriev AM, Nemets EA, Kirillova AD, Kirsanova LA, et al. Decellularization of cartilage microparticles: effects of temperature, supercritical carbon dioxide and ultrasound on biochemical, mechanical, and biological properties. J Biomed Mater Res A. (2023) 111(4):543–55. doi: 10.1002/jbm.a.37474
24. Goh SK, Bertera S, Olsen P, Candiello JE, Halfter W, Uechi G, et al. Perfusion-decellularized pancreas as a natural 3D scaffold for pancreatic tissue and whole organ engineering. Biomaterials. (2013) 34(28):6760–72. doi: 10.1016/j.biomaterials.2013.05.066
25. Xia W, Lin C, Tu Z, Li Y, Shen G. Preparation of laser microporous porcine acellular dermal matrix and observation of wound transplantation. Cell Tissue Bank. (2023) 24(1):191–202. doi: 10.1007/s10561-022-10023-7
26. Hogg P, Rooney P, Ingham E, Kearney JN. Development of a decellularised dermis. Cell Tissue Bank. (2013) 14(3):465–74. doi: 10.1007/s10561-012-9333-1
27. Dragúňová J, Kabát P, Babál P, Mrázová H, Boháč M, Krajčíová Ľ, et al. Development of a new method for the preparation of an acellular allodermis, quality control and cytotoxicity testing. Cell Tissue Bank. (2017) 18(2):153–66. doi: 10.1007/s10561-017-9625-6
28. Bera AK, Sriya Y, Pati F. Formulation of dermal tissue matrix bioink by a facile decellularization method and process optimization for 3D bioprinting toward translation research. Macromol Biosci. (2022) 22(8):e2200109. doi: 10.1002/mabi.202200109
29. Sobreiro-Almeida R, Quinteira R, Neves NM. Renal regeneration: the role of extracellular matrix and current ECM-based tissue engineered strategies. Adv Healthc Mater. (2021) 10(14):e2100160. doi: 10.1002/adhm.202100160
30. Sayk F, Bos I, Schubert U, Wedel T, Sievers HH. Histopathologic findings in a novel decellularized pulmonary homograft: an autopsy study. Ann Thorac Surg. (2005) 79(5):1755–8. doi: 10.1016/j.athoracsur.2003.11.049
31. Yang M, Chen CZ, Wang XN, Zhu YB, Gu YJ. Favorable effects of the detergent and enzyme extraction method for preparing decellularized bovine pericardium scaffold for tissue engineered heart valves. J Biomed Mater Res B Appl Biomater. (2009) 91(1):354–61. doi: 10.1002/jbm.b.31409
32. Faulk DM, Johnson SA, Zhang L, Badylak SF. Role of the extracellular matrix in whole organ engineering. J Cell Physiol. (2014) 229(8):984–9. doi: 10.1002/jcp.24532
33. Dadlani S. Porcine acellular dermal matrix: an alternative to connective tissue graft-a narrative review. Int J Dent. (2021) 2021:1652032. doi: 10.1155/2021/1652032
34. Gierek M, Łabuś W, Kitala D, Lorek A, Ochała-Gierek G, Zagórska KM, et al. Human acellular dermal matrix in reconstructive surgery-a review. Biomedicines. (2022) 10(11):2870. doi: 10.3390/biomedicines10112870
35. Bual R, Labares M Jr, Valle KDD, Pague J Jr, Bantilan ZC, Ducao PG, et al. Characterization of decellularized extracellular matrix from milkfish (chanos chanos) skin. Biomimetics (Basel). (2022) 7(4):213. doi: 10.3390/biomimetics7040213
36. Zhu M, Duan B, Hou K, Mao L, Wang X. A comparative in vitro and in vivo study of porcine- and bovine-derived non-cross-linked collagen membranes. J Biomed Mater Res B Appl Biomater. (2023) 111(3):568–78. doi: 10.1002/jbm.b.35174
37. Wang L, Hao C, Zhou B, Fu X, Li J, Wang Z, et al. Application of heparinized selective acellular sheepskin in wound-healing promotion of deep second degree burns. Wounds. (2016):WNDS20160929-3.27701124
38. Fosnot J, Kovach SJ 3rd, Serletti JM Acellular dermal matrix: general principles for the plastic surgeon. Aesthet Surg J. (2011) 31(7 Suppl):5S–12. doi: 10.1177/1090820X11417576
39. Xu N, Peng XL, Li HR, Liu JX, Cheng JS, Qi XY, et al. Marine-derived collagen as biomaterials for human health. Front Nutr. (2021) 8:702108. doi: 10.3389/fnut.2021.702108
40. Li D, Sun WQ, Wang T, Gao Y, Wu J, Xie Z, et al. Evaluation of a novel tilapia-skin acellular dermis matrix rationally processed for enhanced wound healing. Mater Sci Eng C Mater Biol Appl. (2021) 127:112202. doi: 10.1016/j.msec.2021.112202
41. Tao JP, Aakalu VK, Wladis EJ, Sobel RK, Freitag SK, Foster JA, et al. Bioengineered acellular dermal matrix spacer grafts for lower eyelid retraction repair: a report by the American academy of ophthalmology. Ophthalmology. (2020) 127(5):689–95. doi: 10.1016/j.ophtha.2019.11.011
42. Fahrenbach EN, Qi C, Ibrahim O, Kim JY, Alam M. Resistance of acellular dermal matrix materials to microbial penetration. JAMA Dermatol. (2013) 149(5):571–5. doi: 10.1001/jamadermatol.2013.1741
43. Chen J, Xu J, Wang A, Zheng M. Scaffolds for tendon and ligament repair: review of the efficacy of commercial products. Expert Rev Med Devices. (2009) 6(1):61–73. doi: 10.1586/17434440.6.1.61
44. Scheflan M, Allweis TM, Ben Yehuda D, Maisel Lotan A. Meshed acellular dermal matrix in immediate prepectoral implant-based breast reconstruction. Plast Reconstr Surg Glob Open. (2020) 8(11):e3265. doi: 10.1097/GOX.0000000000003265
45. Parikh RP, Tenenbaum MM, Yan Y, Myckatyn TM. Cortiva versus AlloDerm ready-to-use in prepectoral and submuscular breast reconstruction: prospective randomized clinical trial study design and early findings. Plast Reconstr Surg Glob Open. (2018) 6(11):e2013. doi: 10.1097/GOX.0000000000002013
46. Tognetti L, Pianigiani E, Ierardi F, Lorenzini G, Casella D, Liso FG, et al. The use of human acellular dermal matrices in advanced wound healing and surgical procedures: state of the art. Dermatol Ther. (2021) 34(4):e14987. doi: 10.1111/dth.14987
47. Xin ZC, Yang BC, Li M, Yuan YM, Cui WS, Tang Y, et al. Appllication of human acellular dermal matrix in surgical treatment of genitourinary disease. Beijing Da Xue Xue Bao Yi Xue Ban. (2019) 51(4):778–82. Chinese. doi: 10.19723/j.issn.1671-167X.2019.04.033
48. Zheng X, Chen Y, Dan N, Dan W, Li Z. Highly stable collagen scaffolds crosslinked with an epoxidized natural polysaccharide for wound healing. Int J Biol Macromol. (2021) 182:1994–2002. doi: 10.1016/j.ijbiomac.2021.05.189
49. Keane TJ, Swinehart IT, Badylak SF. Methods of tissue decellularization used for preparation of biologic scaffolds and in vivo relevance. Methods. (2015) 84:25–34. doi: 10.1016/j.ymeth.2015.03.005
50. Łabuś W, Kawecki M, Glik J, Maj M, Kitala D, Misiuga M, et al. Own experience from the use of a substitute of an allogeneic acellular dermal matrix revitalized with in vitro cultured skin cells in clinical practice. Pol Przegl Chir. (2015) 87(10):513–21. doi: 10.1515/pjs-2015-0097
51. Srivastava A, DeSagun EZ, Jennings LJ, Sethi S, Phuangsab A, Hanumadass M, et al. Use of porcine acellular dermal matrix as a dermal substitute in rats. Ann Surg. (2001) 233(3):400–8. doi: 10.1097/00000658-200103000-00015
52. Wang W, Huang WC, Zheng J, Xue C, Mao X. Preparation and comparison of dialdehyde derivatives of polysaccharides as cross-linking agents. Int J Biol Macromol. (2023) 236:123913. doi: 10.1016/j.ijbiomac.2023.123913
53. Chen L, Huang C, Zhong Y, Chen Y, Zhang H, Zheng Z, et al. Multifunctional sponge scaffold loaded with concentrated growth factors for promoting wound healing. iScience. (2022) 26(1):105835. doi: 10.1016/j.isci.2022.105835
54. Feng R, Dan N, Chen Y, Dan W. Crosslinking of dialdehyde heparin: a new strategy for improving the anticoagulant properties of porcine acellular dermal matrix. RSC Adv. (2022) 12(11):6811–20. doi: 10.1039/d1ra08982j
55. Cai R, Xiang H, Yang D, Lin KT, Wu Y, Zhou R, et al. Plasmonic AuPt@CuS heterostructure with enhanced synergistic efficacy for radiophotothermal therapy. J Am Chem Soc. (2021) 143(39):16113–27. doi: 10.1021/jacs.1c06652
56. Cifuentes J, Muñoz-Camargo C, Cruz JC. Reduced graphene oxide-extracellular matrix scaffolds as a multifunctional and highly biocompatible nanocomposite for wound healing: insights into characterization and electroconductive potential. Nanomaterials (Basel). (2022) 12(16):2857. doi: 10.3390/nano12162857
57. Fedoniuk LY, Kulyanda IS, Dovgalyuk AI, Lomakina YV, Kramar SB, Kulianda OO, et al. Morphological characteristics of acellular dermal matrix manufacturing. Wiad Lek. (2021) 74(3 cz 1):418–22. doi: 10.36740/WLek202103107
58. Song Z, Yang D, Yang J, Nie X, Wu J, Song H, et al. Abdominal wall reconstruction following resection of large abdominal aggressive neoplasms using tensor fascia lata flap with or without mesh reinforcement. Hernia. (2018) 22(2):333–41. doi: 10.1007/s10029-018-1738-8
59. Kirsner RS, Bohn G, Driver VR, Mills JL Sr, Nanney LB, Williams ML, et al. Human acellular dermal wound matrix: evidence and experience. Int Wound J. (2015) 12(6):646–54. doi: 10.1111/iwj.12185
60. Boháč M, Danišovič Ľ, Koller J, Dragúňová J, Varga I. What happens to an acellular dermal matrix after implantation in the human body? A histological and electron microscopic study. Eur J Histochem. (2018) 62(1):2873. doi: 10.4081/ejh.2018.2873
61. Mirzaei-Parsa MJ, Ghanbari H, Alipoor B, Tavakoli A, Najafabadi MRH, Faridi-Majidi R. Nanofiber-acellular dermal matrix as a bilayer scaffold containing mesenchymal stem cell for healing of full-thickness skin wounds. Cell Tissue Res. (2019) 375(3):709–21. doi: 10.1007/s00441-018-2927-6
62. Hodde JP, Record RD, Liang HA, Badylak SF. Vascular endothelial growth factor in porcine-derived extracellular matrix. Endothelium. (2001) 8(1):11–24. doi: 10.3109/10623320109063154
63. Fu Q, Cao YL. Use of tissue engineering in treatment of the male genitourinary tract abnormalities. J Sex Med. (2010) 7(5):1741–6. doi: 10.1111/j.1743-6109.2010.01708.x
64. Zhang X, Yang J, Li Y, Liu S, Long K, Zhao Q, et al. Functional neovascularization in tissue engineering with porcine acellular dermal matrix and human umbilical vein endothelial cells. Tissue Eng Part C Methods. (2011) 17(4):423–33. doi: 10.1089/ten.TEC.2010.0466
65. Kim J, Song SY, Lee SG, Choi S, Lee YI, Choi JY, et al. Treatment of human immunodeficiency virus-associated facial lipoatrophy with hyaluronic acid filler mixed with micronized cross-linked acellular dermal matrix. J Korean Med Sci. (2022) 37(5):e37. doi: 10.3346/jkms.2022.37.e37
66. Chang LS, Kim SH, Kim H, Ryu S, Choy YB, Kim SW. Evaluation of paste-type micronized acellular dermal matrix for soft tissue augmentation: volumetric and histological assessment in a mouse model. Aesthetic Plast Surg. (2023) 47(2):852–61. doi: 10.1007/s00266-022-03051-x
67. Kim J, Kim J, Lee YI, Lee JH. Treatment of linear morphea (en coup de sabre) with micronized acellular dermal matrix filler: a case report. Ann Dermatol. (2021) 33(4):373–6. doi: 10.5021/ad.2021.33.4.373
68. Yang J, Dang H, Xu Y. Recent advancement of decellularization extracellular matrix for tissue engineering and biomedical application. Artif Organs. (2022) 46(4):549–67. doi: 10.1111/aor.14126
69. Korneyev I, Ilyin D, Schultheiss D, Chapple C. The first oral mucosal graft urethroplasty was carried out in the 19th century: the pioneering experience of Kirill Sapezhko (1857–1928). Eur Urol. (2012) 62(4):624–7. doi: 10.1016/j.eururo.2012.06.035
70. Dubey D, Vijjan V, Kapoor R, Srivastava A, Mandhani A, Kumar A, et al. Dorsal onlay buccal mucosa versus penile skin flap urethroplasty for anterior urethral strictures: results from a randomized prospective trial. J Urol. (2007) 178(6):2466–9. doi: 10.1016/j.juro.2007.08.010
71. Patel S, Ziai K, Lighthall JG, Walen SG. Biologics and acellular dermal matrices in head and neck reconstruction: a comprehensive review. Am J Otolaryngol. (2022) 43(1):103233. doi: 10.1016/j.amjoto.2021.103233
72. Shaikh MS, Lone MA, Matabdin H, Lone MA, Soomro AH, Zafar MS. Regenerative potential of enamel matrix protein derivative and acellular dermal matrix for gingival recession: a systematic review and meta-analysis. Proteomes. (2021) 9(1):11. doi: 10.3390/proteomes9010011
73. Hassan AM, Asaad M, Seitz AJ, Liu J, Butler CE. Effect of wound contamination on outcomes of abdominal wall reconstruction using acellular dermal matrix: 14-year experience with more than 700 patients. J Am Coll Surg. (2021) 233(6):676–84. doi: 10.1016/j.jamcollsurg.2021.08.679
74. Kim TH, Wee SY. A new method for inverted nipple treatment with diamond-shaped dermal flaps and acellular dermal matrix: a preliminary study. Aesthetic Plast Surg. (2023) 47(3):998–1006. doi: 10.1007/s00266-022-03216-8
75. Skowronek K, Łabuś W, Stojko R, Kitala D, Sadłocha M, Drosdzol-Cop A. Application of acellular dermal matrix in gynaecology-a current review. J Clin Med. (2022) 11(14):4030. doi: 10.3390/jcm11144030
76. Park TH. Effectiveness of cross-linked acellular dermal matrix to correct post-traumatic enophthalmos. J Craniofac Surg. (2023) 34(5):e449–51. doi: 10.1097/SCS.0000000000009290
77. Salinas F, Robla D, Meana Á, Pevida M, Martinez Magide G, Sánchez Nuño C, et al. Novel technique of development of human derived acellular dermal matrix. Cell Tissue Bank. (2022) 23(2):385–94. doi: 10.1007/s10561-021-09954-4
78. Lin J, Hao JR, Jin J, Deng SM, Hu J, Na YQ. Homologous dermal acellular matrix graft for urethral reconstruction in man (report of 16 cases). Zhonghua Yi Xue Za Zhi. (2005) 85(15):1057–9. doi: 10.3760/j:issn:0376-2491.2005.15.013
79. Liu L, Liang DJ, Wang H, Wu TP, Li C, PingShen PF. Tissue engineering material for the replacement of urethral. Chin J Urol. (2001) 22:428–31. doi: 10.3760/j:issn:1000-6702.2001.07.014
80. Yang Y, Xu Y, Song Y, Xu AX, Wang XX, Hong BF. Usage of acellular dermal matrix allograft in surgical treatment of vesico-vaginal fistula and anterior urethral stricture: report of 9 cases. Zhonghua Yi Xue Za Zhi. (2007) 87(24):1693–4. doi: 10.3760/j:issn:0376-2491.2007.24.010
81. Tang X, Zhang X, Wu Y, Yin H, Du Y, Zhang X, et al. The clinical effects of utilizing allogeneic acellular dermal matrix in the surgical therapy of anterior urethral stricture. Urol Int. (2020) 104(11–12):933–8. doi: 10.1159/000510317
82. Bhargava S, Patterson JM, Inman RD, MacNeil S, Chapple CR. Tissue-engineered buccal mucosa urethroplasty-clinical outcomes. Eur Urol. (2008) 53(6):1263–9. doi: 10.1016/j.eururo.2008.01.061
83. El-Kassaby A, AbouShwareb T, Atala A. Randomized comparative study between buccal mucosal and acellular bladder matrix grafts in complex anterior urethral strictures. J Urol. (2008) 179(4):1432–6. doi: 10.1016/j.juro.2007.11.101
84. Sobhani S, Khaboushan AS, Jafarnezhad-Ansariha F, Azimzadeh A, Danesh Payeh M, Kajbafzadeh AM. Off-the-shelf acellular fetal skin scaffold as a novel alternative to buccal mucosa graft: the development and characterization of human tissue-engineered fetal matrix in rabbit model of hypospadiasis. Int Urol Nephrol. (2022) 54(9):2187–95. doi: 10.1007/s11255-022-03249-7
85. Yin JL, Wu Y, Yuan ZW, Gao XH, Chen HD. Advances in scarless foetal wound healing and prospects for scar reduction in adults. Cell Prolif. (2020) 53(11):e12916. doi: 10.1111/cpr.12916
86. Castillo V, Díaz-Astudillo P, Corrales-Orovio R, San Martín S, Egaña JT. Comprehensive characterization of tissues derived from animals at different regenerative stages: a comparative analysis between fetal and adult mouse skin. Cells. (2023) 12(9):1215. doi: 10.3390/cells12091215
87. Wu S, He R, Sun J, Zhao H. Acellular dermal matrix graft for ventral corporal lengthening orthoplasty in 2-stage proximal hypospadias repair. Transl Pediatr. (2021) 10(12):3151–8. doi: 10.21037/tp-21-372
88. Morgante D, Radford A, Abbas SK, Ingham E, Subramaniam R, Southgate J. Augmentation of the insufficient tissue bed for surgical repair of hypospadias using acellular matrix grafts: a proof of concept study. J Tissue Eng. (2021) 12:2041731421998840. doi: 10.1177/2041731421998840
89. Lin D, Wang G, Song H, Qu Y, Liu P, Liang H, et al. Use of acellular dermal matrix for urethroplasty coverage in proximal hypospadias repair: a pilot study. Adv Ther. (2020) 37(4):1425–35. doi: 10.1007/s12325-020-01254-9
90. Wang Y, Wang G, Hou X, Zhao Y, Chen B, Dai J, et al. Urethral tissue reconstruction using the acellular dermal matrix patch modified with collagen-binding VEGF in beagle urethral injury models. Biomed Res Int. (2021) 2021:5502740. doi: 10.1155/2021/5502740
91. Wu S, Ye C, Yang H, Chen B, Nie H, Li S. Application of allogeneic human acellular dermal matrix reduces the incidence of fistula in hypospadias repair. Front Pediatr. (2022) 10:774973. doi: 10.3389/fped.2022.774973
92. Ortac M, Ekerhult TO, Zhao W, Atala A. Tissue engineering graft for urethral reconstruction: is it ready for clinical application? Urol Res Pract. (2023) 49(1):11–8. doi: 10.5152/tud.2023.22226
93. Ribeiro-Filho LA, Sievert KD. Acellular matrix in urethral reconstruction. Adv Drug Deliv Rev. (2015) 82–83:38–46. doi: 10.1016/j.addr.2014.11.019
94. De Filippo RE, Yoo JJ, Atala A. Urethral replacement using cell seeded tubularized collagen matrices. J Urol. (2002) 168(4 Pt 2):1789–92; discussion 1792–3. doi: 10.1097/01.ju.0000027662.69103.72
95. Xue JD, Gao J, Fu Q, Feng C, Xie H. Seeding cell approach for tissue-engineered urethral reconstruction in animal study: a systematic review and meta-analysis. Exp Biol Med (Maywood). (2016) 241(13):1416–28. doi: 10.1177/1535370216640148
96. Fu JS, Zhou ZY, Xu SY. Bone marrow mesenchymal stem cells combined with acellular dermal matrix for repair of urethral injury. Zhongguo Zuzhi Gongcheng Yanjiu. (2018) 22(13):2075–80. doi: 10.3969/j.issn.2095-4344.0486
Keywords: acellular dermal matrix, tissue engineering, urethral strictures, hypospadias, regeneration
Citation: Aodi J, Ying L, Chengyang S and Hongfeng Z (2024) Acellular dermal matrix in urethral reconstruction. Front. Pediatr. 12:1342906. doi: 10.3389/fped.2024.1342906
Received: 22 November 2023; Accepted: 30 January 2024;
Published: 9 February 2024.
Edited by:
Sajid Sultan, Sindh Institute of Urology and Transplantation, PakistanReviewed by:
Lynn Woo, Cleveland Clinic, United States© 2024 Aodi, Ying, Chengyang and Hongfeng. This is an open-access article distributed under the terms of the Creative Commons Attribution License (CC BY). The use, distribution or reproduction in other forums is permitted, provided the original author(s) and the copyright owner(s) are credited and that the original publication in this journal is cited, in accordance with accepted academic practice. No use, distribution or reproduction is permitted which does not comply with these terms.
*Correspondence: Zhai Hongfeng emhhaWhvbmdmZW5nQHNpbmEuY29t
Disclaimer: All claims expressed in this article are solely those of the authors and do not necessarily represent those of their affiliated organizations, or those of the publisher, the editors and the reviewers. Any product that may be evaluated in this article or claim that may be made by its manufacturer is not guaranteed or endorsed by the publisher.
Research integrity at Frontiers
Learn more about the work of our research integrity team to safeguard the quality of each article we publish.