- Department of Neonatology, Shanghai Children’s Hospital, School of Medicine, Shanghai Jiao Tong University, Shanghai, China
Objective: This study aims to investigate the effects of hyperoxia exposure on TGF-β1-induced endothelial-mesenchymal transition (EndoMT) and regulatory T cell (Treg)—mediated immunomodulation in human pulmonary microvascular endothelial cells (HPMECs), which could provide a theoretical basis for further studies of the pathogenesis of bronchopulmonary dysplasia (BPD).
Methods: A BPD cell model was established by exposing HPMECs to hyperoxia. Flow cytometry was used to isolate CD4 + CD3 + CD25 + CD127- Tregs from the peripheral blood samples of preterm infants. HPMECs were divided into four groups based on whether they were exposed to hyperoxia and/or co-cultured with Tregs. Quantitative reverse transcription-polymerase chain reaction (qRT-PCR) and enzyme-linked immunosorbent assay (ELISA) were used to test the expression levels of TGF-β1, α-SMA, Foxp3, IL-10, and reactive oxygen species (ROS).
Results: The results showed that the expression levels of TGF-β1 and α-SMA in HPMECs increased at 24 h, 48 h, and 72 h of hyperoxia exposure. In the co-culture group of HPMECs and Tregs, Foxp3 and IL-10 expressions decreased at 48 h and 72 h of hyperoxia exposure. ROS expression increased in the hyperoxia group of HPMECs at 24 h, 48 h, and 72 h of hyperoxia exposure, which were higher than those in the hyperoxia group of HPMECs and Tregs.
Conclusion: These findings suggest that hyperoxia exposure promotes EndoMT in HMPECs and inhibits the immunosuppressive effect of Tregs. Despite this, Tregs still seem could protect HPMECs from oxidative stress injury.
1 Introduction
Bronchopulmonary dysplasia (BPD) is a chronic lung disease (CLD) characterized by alveolar simplification and abnormal vascular growth following impaired lung development. It is proven to be associated with morbidity and mortality in preterm infants and significantly impacts the long-term quality of life in survivors (1). Hyperoxia exposure is one of the most important risk factors for BPD (2). Although a large number of studies have been conducted to investigate the pathogenesis of BPD, it is not yet fully understood.
Mesenchymal transition plays an important role in tissue fibrosis. It can promote a significant increase in fibroblasts and a significant decrease in microvascular density, leading to an accelerated fibrosis process (3, 4). TGF-β1 is a major driver of endothelial-to-mesenchymal transition (EndoMT) and epithelial-mesenchymal transition (EMT) and plays a crucial part in chronic obstructive pulmonary disease (COPD) and pulmonary fibrosis (4, 5). It was found that hyperoxia exposure in the BPD rat model induced EMT of alveolar epithelial cells (6, 7). However, the mechanism of EndoMT in the pathogenesis of BPD under hyperoxia exposure is unclear.
Moreover, it has been reported that the numbers of regulatory cells (Tregs) in the cord blood of BPD neonates and non-BPD neonates were significantly different, and the numbers of Tregs in the peripheral blood of BPD neonates changed dynamically during the disease process (8, 9). Treg is a subpopulation of CD4+ T cells with significant immunosuppressive effects that can suppress inflammation and autoimmune responses through the release of anti-inflammatory cytokines such as IL-10 (10). FOXP3 is one of the key transcription factors of Treg and is crucial for the immunosuppressive function of Treg (10, 11). The imbalance between anti-inflammatory and pro-inflammatory immune responses mediated by Tregs and Th17 has been reported to be related to the progression of COPD (10, 12).
Based on these findings, we hypothesized that the effects of hyperoxia exposure on EndoMT and Treg—mediated immunomodulation play important roles in the development of BPD. In this study, the human pulmonary microvascular endothelial cells (HPMECs) model exposed to hyperoxia was established to explore the function of EndoMT and Tregs in BPD, which could provide a theoretical basis for further studies of the molecular mechanism of BPD induced by hyperoxia exposure.
2 Methods
2.1 Participants and sample collection
The clinical data of preterm infants who were admitted to the Neonatology Department of Shanghai Children’s Hospital in October 2022 was collected. Inclusion criteria: (1) Gestational age (GA) at birth less than 32 weeks; (2) Hospital admission within 7 days after birth. Exclusion criteria: (1) Diagnosis of immunodeficiency disease; (2) Diagnosis of severe congenital malformations. The peripheral blood samples were collected around 7 days after birth for Treg isolation. The study was approved by the ethics committee of Shanghai Children’s Hospital (2022RY003) and informed consent was obtained from all the guardians.
2.2 Isolation of Tregs
Peripheral blood lymphocytes were isolated using the MACSprepTMPBMC isolation kit (MiltenyiBiotec, Germany). Anti-human CD3 antibody (317318, BioLegend, USA), anti-human CD4 antibody (300508, BioLegend, USA), anti-human CD25 antibody (302626, BioLegend, USA) and anti-human CD127 antibody (351312, BioLegend, USA) were used. The single-cell suspension was placed in tubes. 1 × 106 cells were taken from each tube to make a blank tube, a CD3 single-staining tube, a CD4 single-staining tube, a CD25 single-staining tube, and a CD127 single-staining tube by adding different antibodies respectively. Meanwhile, 3 × 107 cells were taken and all of the antibodies were added to prepare a whole staining tube. Each tube was centrifuged at 1,500 rpm for 5 min after adding 1 ml PBS. The supernatant was discarded and the 100–200 ul liquid was left for flow staining. In addition to the Blank tube, 1 ul anti-human CD16/32 antibody (130-059-901, Miltenyi, Germany) per tube was used to close the non-specific antigen binding sites on the cell surface. Flow-through antibodies were added based on the instructions. PBS was used to wash off unbound antibodies. CD3 + CD4 + CD25 + CD127- Treg population was sorted by a flow Cytometer (BD Biosciences FACSCalibur, USA). Tregs were then cultured at appropriate density in RPMI medium.
2.3 Establish of cell model
HPMECs (ScienCell, USA) were inoculated in six-well plates and cultured to an amount of approximately 8 × 106 cells. The concentration of Tregs was adjusted to 1 × 106/ml. HPMECs were co-cultured with Tregs for two days with a ratio of 10:1. The incubation conditions were 37°C and 5% CO2. The oxygen concentration of the hyperoxia groups was set to 95% and the oxygen concentration of the air groups was set to 21%. HPMECs were divided into four groups (H1, H2, HT1, and HT2) based on whether they were exposed to hyperoxia and/or co-cultured with Tregs (Table 1). The expression of each index was detected at different time points (24 h, 48 h and 72 h) of hyperoxia exposure.
2.4 Research methods
RNA extraction and quantitative reverse transcription-polymerase chain reaction (qRT-PCR) was performed to detect the expressions of genes on the mRNA level. TRIzol reagent (Invitrogen 15596-026, USA) was used for the extraction of RNA. The RNA quantity and quality were detected by NanoDrop 2,000 Spectrophotometer (Thermo Fisher Scientific, USA). Reverse transcription reactions were performed by referring to the instructions of the Reverse Transcription Kit (TaKaRa RR036B, Japan) to obtain cDNA samples. An ABI 7,500 thermal cycler (Applied Biosystems, Thermo Fisher Scientific, USA) using the following 2-step cycling program was performed for PCR: 95°C for 5 min, 40 cycles at 95°C for 15 s, 60°C for 20 s, and 72°C for 40 s. The PCR primers were provided by KeyGEN BioTECH company (China) (Table 2). GAPDH was used for normalization. The relative expression levels were analyzed by the 2−ΔΔCt method.
Enzyme-linked immunosorbent assay (ELISA) was performed to detect the expressions of genes on protein level. The TGF-β1 assay kit (mlbio: ml022522, China), α-SMA assay kit (mlbio: ml027532, China), ROS assay kit (KeyGEN BioTECH: KGT010-1, China), Foxp3 ELISA kit (mlbio: ml027353, China) and IL-10 ELISA kit (mlbio: ml064299, China) were used to test the expression of each index respectively. Standard wells, blank wells, and sample wells were set up respectively according to the instructions. 50 ul samples and 50 ul assay reagent were added to each well. The liquid in the wells was discarded after incubation for 30 min at 37°C. The chromogenic agent was added according to the instructions. Finally, optical density (OD) was measured sequentially at 450 nm using a Microplate reader (Rayto RT-6100, USA).
2.5 Statistical analysis
The results are presented as the mean ± standard deviation. All experiments were repeated 3 times. T-test was used to analyze data with the GraphPad Prism software (GraphPad, USA). P < 0.05 was considered statistically significant.
3 Results
3.1 General clinical information
A total of 14 preterm infants were involved in this study, including 6 male and 8 female infants with a GA of 29.2 ± 1.4 weeks and a Birth weight (BW) of 1,309.3 ± 246.6 g. Five of the 14 preterm infants developed BPD at 36 weeks postmenstrual age.
Peripheral blood samples were collected randomly from 5 of the 14 preterm infants with a GA of 30.2 ± 1.2 weeks and a BW of 1,570.0 ± 177.1 g. The 5 infants included 1 male and 4 females. One of the 5 preterm infants developed BPD at 36 weeks postmenstrual age.
3.2 Hyperoxia exposure increases expressions of TGF-β1 and α-SMA
Compared with those in the H1 group, TGF-β1 and α-SMA expressions at mRNA and protein levels in the H2 group increased significantly at 24 h, 48 h, and 72 h of hyperoxia exposure (Figure 1).
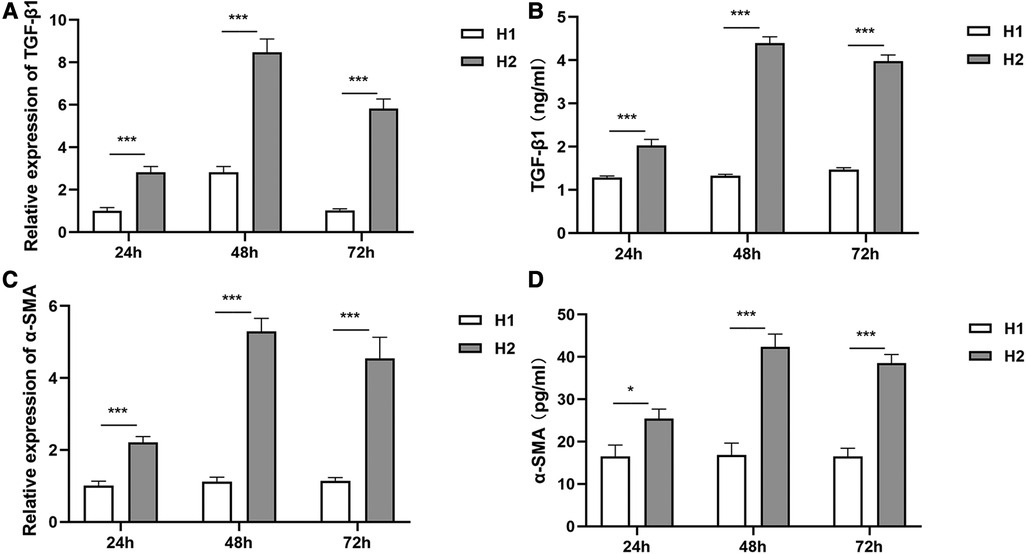
Figure 1 The expressions of TGF-β1 and α-SMA at different time points of hyperoxia exposure. (A,B) At 24 h, 48 h, and 72 h of hyperoxia exposure, TGF-β1 expressions at mRNA levels and protein levels in the H2 group (n = 3) were significantly higher than those in the H1 group (n = 3). (C,D) Compared with the H1 group (n = 3), α-SMA expressions in the H2 group (n = 3) were increased significantly at mRNA levels and protein levels at 24 h, 48 h, and 72 h of hyperoxia exposure. Three independent experimental repeats were performed. *P < 0.05, ***P < 0.001. H1, HPMECs incubated under conditions of 37°C, 5% CO2 and 21% O2; H2, HPMECs incubated under conditions of 37°C, 5% CO2 and 95% O2.
3.3 Isolation of Tregs from peripheral blood samples
The results of flow cytometry showed that the purity of monocytes in peripheral blood cells reached 90.5%–98.7%. CD4 + CD3+ T cells accounted for 40.9%–53.4% of the isolated monocytes, and CD25 + CD127- Treg accounted for 6.91%–11.9% of the total CD4 + CD3+ T cells isolated. Some of the results are shown in Figure 2.
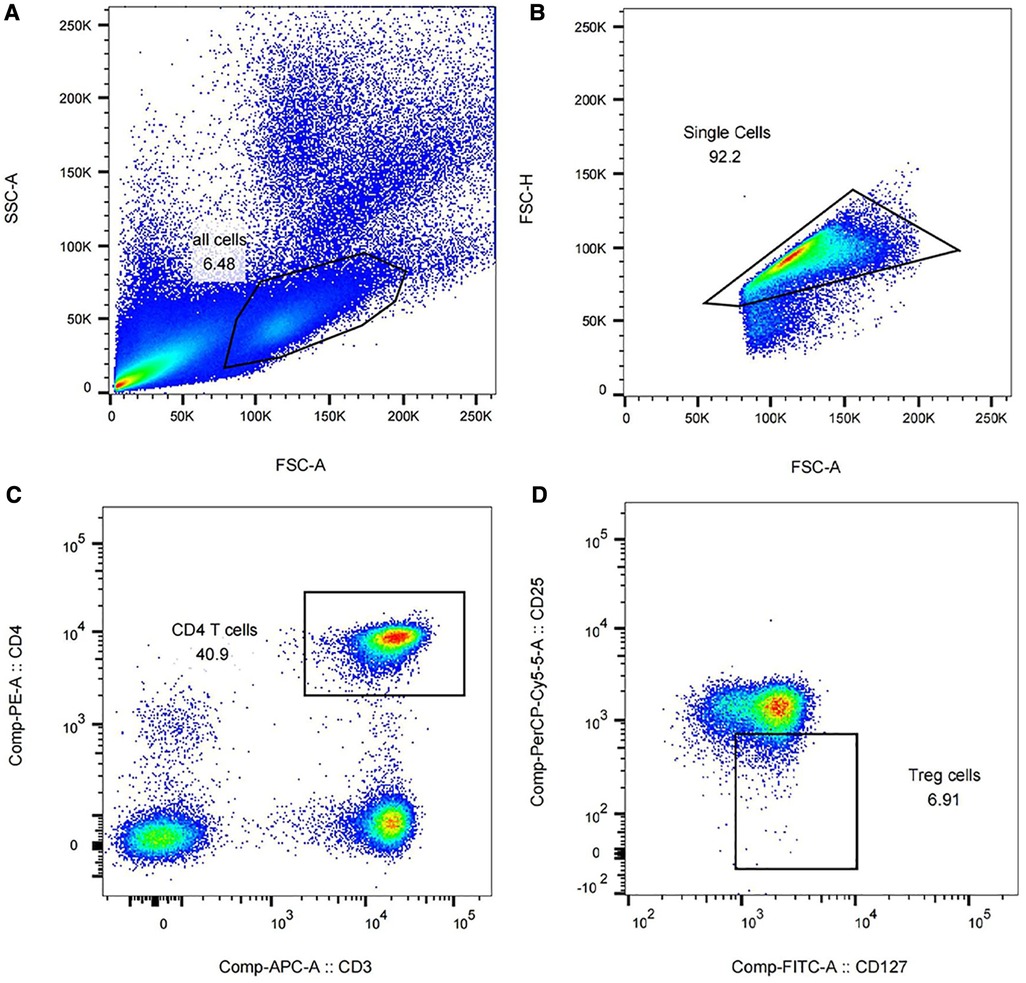
Figure 2 Isolation of Tregs in peripheral blood of preterm infants using flow cytometry (partial results). (A) Peripheral blood cells accounted for 6.48% of all peripheral blood components. (B) The proportion of monocytes in peripheral blood cells was 92.2%. (C) The proportion of CD4 + CD3+ T cells in monocytes was 40.9%. (D) CD25 + CD127- Treg accounted for 6.91% of CD4 + CD3+ T cells.
3.4 Hyperoxia exposure decreases expressions of Foxp3 and Il-10
No significant difference in the expressions of Foxp3 and IL-10 was detected between HT1 group and HT2 group at 24 h of hyperoxia exposure (Figure 3). However, at 48 h of hyperoxia exposure, the expressions of Foxp3 and IL-10 were significantly decreased in the HT2 group compared with those in the HT1 group (Figure 3). At 72 h of hyperoxia exposure, expressions of Foxp3 in the HT2 group were lower than those in the HT1 group (Figures 3A–C). Compared with that in HT1 group at 72 h of hyperoxia exposure, IL-10 expression at mRNA level in HT2 group decreased significantly, but its expression at the protein level showed a decreasing trend which is not statistically significant (Figures 3C,D).
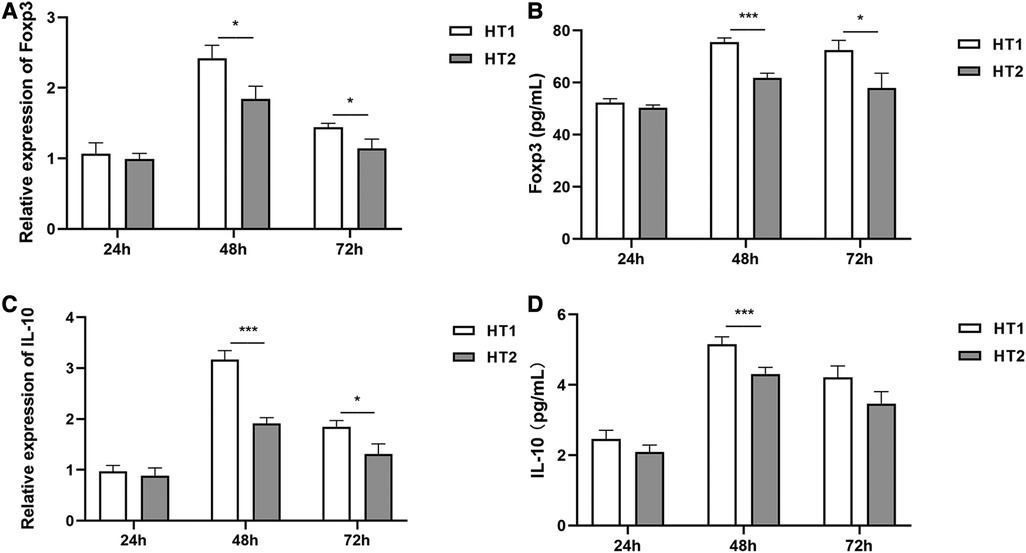
Figure 3 The expression of Foxp3 and IL-10 at different time points of hyperoxia exposure. (A,B): Foxp3 expressions showed no difference between the HT1 group (n = 3) and HT2 gourp (n = 3) at 24 h of hyperoxia exposure. However, at 48 h and 72 h of hyperoxia exposure, Foxp3 expressions decreased significantly in the HT2 group (n = 3) compared with those in the HT1 group (n = 3). (C) Although no significant difference was observed between the HT1 group (n = 3) and HT2 group (n = 3) after hyperoxia exposure for 24 h, IL-10 expressions at mRNA levels decreased significantly in the HT2 group (n = 3) compared with those in the HT1 group (n = 3) after hyperoxia exposure for 48 h and 72 h. (D) The results of ELISA showed that there was no difference in IL-10 expressions between the HT1 group (n = 3) and HT2 group (n = 3) at 24 h of hyperoxia exposure. Compared with that in the HT1 group (n = 3), a significant decrease was detected in the HT2 group (n = 3) at 48 h of hyperoxia exposure. The results also showed that compared with that in the HT1 group, the IL-10 expression in the HT2 group had no significant difference, but a downward trend at 72 h of hyperoxia exposure. Three independent experimental repeats were performed. *P < 0.05, ***P < 0.001. HT1, HPMECs and Tregs co-cultured under conditions of 37°C, 5% CO2 and 21% O2; HT2, HPMECs and Tregs co-cultured under conditions of 37°C, 5% CO2 and 95% O2.
3.5 Co-culture of HPMEC and Treg inhibits the increase of ROS expression under hyperoxia exposure
The results of ELISA showed that at 24 h, 48 h, and 72 h of hyperoxia exposure, ROS expressions of HPMECs in the H2 group were significantly higher than those in the H1 group (Figure 4). It was also observed that ROS expressions in HT2 group were significantly lower than that in H2 group at 24 h, 48 h and 72 h of hyperoxia exposure (Figure 4).
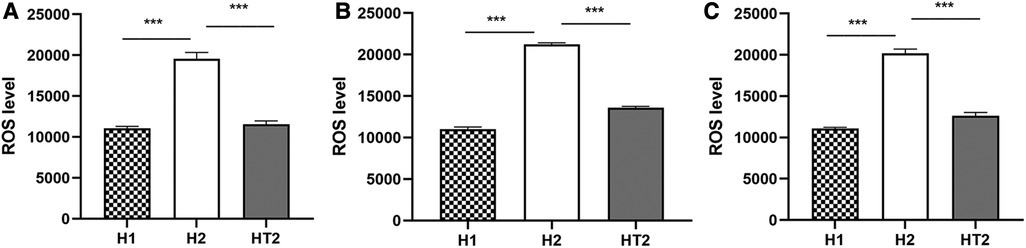
Figure 4 The expression of ROS detected by ELISA at different time points of hyperoxia exposure. (A–C) After hyperoxia exposure for 24 h, 48 h, and 72 h, the ROS expressions in the H2 group (n = 3) were significantly higher than those in the H1 group (n = 3) and HT2 group (n = 3). Three independent experimental repeats were performed. ***P < 0.001. H1, HPMECs incubated under conditions of 37°C, 5% CO2 and 21% O2; H2, HPMECs incubated under conditions of 37°C, 5% CO2 and 95% O2; HT2, HPMECs and Tregs co-cultured under conditions of 37°C, 5% CO2 and 95% O2.
4 Discussion
In this study, we found that the expressions of EndoMT-related indicators (TGF-β1 and α-SMA) were significantly increased in the hyperoxia-exposed HPMEC model. To further explore the interaction between Tregs and HPMECs under hyperoxia exposure, HPMECs were co-cultured with CD4 + CD3 + CD25 + CD127- Tregs which were isolated from the peripheral blood of preterm infants less than 32 weeks of GA. The findings showed that the expressions of Foxp3, a key transcription factor of Treg, and IL-10, an anti-inflammatory cytokine secreted by Treg, were both decreased after hyperoxia exposure, but Tregs still had a significant immunosuppressive effect on the increase of ROS expression induced by hyperoxia exposure.
The pathological feature of the new “BPD” is the retardation of lung development leading to simplification of alveolar structure and abnormities of pulmonary vascular development (1). HPMEC is crucial for the induction of pulmonary microangiogenesis and has important physiological functions such as constituting the endothelial barrier and secretion (13, 14). Pulmonary microvasculature actively promotes alveolar growth during lung development and helps maintain normal alveolar structure (13, 14). Our study found that TGF-β1 and α-SMA expressions increased significantly in the hyperoxia-exposed HPMEC cell model, confirming that hyperoxia exposure induces EndoMT in HPMECs. EndoMT can be triggered by different mechanisms in different cell types and cellular physiological or pathological states and involves different signaling pathways (15). EndoMT is also vital for embryonic development, malignancy, angiogenesis, inflammation, and fibrotic diseases (15). It has been reported that the EndoMT in HPMECs mediated by the TGF-β1/Smad3 pathway, and the EMT in lung epithelial cells can contribute to the development of COPD and pulmonary fibrosis (4, 5). It has also been found that EndoMT promotes pulmonary vascular remodeling in pulmonary arterial hypertension (15, 16). Therefore, EndoMT in HPMECs induced by hyperoxia exposure may contribute to the development of BPD.
T-cell counts and function have been reported to be involved in the development of BPD-associated lung injury (17–19). In our study, Tregs co-cultured with HPMECs were isolated from the peripheral blood of preterm infants with GA less than 32 weeks. A significant decrease in Foxp3 and IL-10 expressions under hyperoxia exposure was found, suggesting that hyperoxia exposure may inhibit the immunomodulatory function of Tregs. Treg is an important subpopulation of Th cells and is central to the maintenance of immune homeostasis (20). Foxp3 is a nuclear transcription factor of Treg, which is essential for Treg to exert immunosuppressive function (21, 22). Treg secretes immunosuppressive factors such as IL-10 and TGF-β, which are involved in a variety of chronic inflammation-mediated lung diseases (9, 23). Treg has the potential to shift from the anti-inflammatory phenotype to the pro-inflammatory phenotype under specific conditions (24). Treg of preterm infants exhibits distinct phenotypes and quantitative dynamics in the early postnatal period compared with that of term infants and adults (9). Lee and his collogues found that an imbalance between pro-inflammatory and anti-inflammatory immune responses due to a decrease in Treg numbers is associated with the development of COPD (25). Misra et al. collected cord blood samples from newborns at birth and found that Treg expression was significantly higher in the cord blood of preterm infants compared with that of term infants. They also found that the absolute number of Tregs in the cord blood of neonates with moderate BPD was significantly lower than that of patients with no or mild BPD (8). The results of Pagel et al. demonstrated that the number and immunosuppressive function of Tregs were significantly higher in preterm infants compared with that in term infants and adults. Their findings also showed that preterm infants with BPD had a significant increase in Treg frequency in the early postnatal period, with a peak at 4–10 days postnatal (9). The above results suggest that Treg plays an important role in the pathogenesis of BPD in preterm infants, but the dynamics and molecular mechanisms of Tregs in BPD are not yet clear, and more studies are needed.
In addition, hyperoxia exposure can lead to the release of ROS, causing severe lung injury (2, 23, 26). Previous studies have found that hyperoxia exposure induces oxidative stress in HPMECs, leading to increased cell edema, dysfunction, impaired survival, and abnormal growth (27, 28). Preterm infants are poorly tolerant to hyperoxia and more susceptible to ROS-mediated oxidative stress damage, which can cause lung injury and developmental impairment (29). Our findings demonstrated that hyperoxia exposure caused a significant increase in ROS expression in HPMECs, which was significantly decreased when HPMECs were co-cultured with Tregs. These findings indicated that Tregs had a protective effect on HPMECs under hyperoxia exposure.
In conclusion, these results indicate that hyperoxia exposure promotes the development of EndoMT in HPMEC and attenuates the immunosuppressive effect of Tregs, but Tregs still seem to have a protective effect against oxidative stress injury in HPMECs. Therefore, EndoMT and Tregs may play important roles in hyperoxia-induced lung injury in preterm infants with BPD. However, the molecular mechanism still needs further exploration.
Data availability statement
The original contributions presented in the study are included in the article/Supplementary Material, further inquiries can be directed to the corresponding author.
Ethics statement
The studies involving humans were approved by Ethics committee of Shanghai Children’s Hospital. The studies were conducted in accordance with the local legislation and institutional requirements. Written informed consent for participation in this study was provided by the participants’ legal guardians/next of kin.
Author contributions
YS: Conceptualization, Data curation, Formal Analysis, Writing – original draft. CY: Data curation, Formal Analysis, Writing – original draft. YiL: Data curation, Writing – original draft. YaL: Data curation, Writing – original draft. BW: Data curation, Writing – original draft. XG: Formal Analysis, Writing – review & editing. CC: Conceptualization, Methodology, Writing – review & editing.
Funding
The author(s) declare that no financial support was received for the research, authorship, and/or publication of this article.
Acknowledgments
The authors thank their preterm infants for participating in this study.
Conflict of interest
The authors declare that the research was conducted in the absence of any commercial or financial relationships that could be construed as a potential conflict of interest.
Publisher's note
All claims expressed in this article are solely those of the authors and do not necessarily represent those of their affiliated organizations, or those of the publisher, the editors and the reviewers. Any product that may be evaluated in this article, or claim that may be made by its manufacturer, is not guaranteed or endorsed by the publisher.
References
1. Schmidt AR, Ramamoorthy C. Bronchopulmonary dysplasia. Paediatr Anaesth. (2022) 32(2):174–80. doi: 10.1111/pan.14365
2. Giusto K, Wanczyk H, Jensen T, Finck C. Hyperoxia-induced bronchopulmonary dysplasia: better models for better therapies. Dis Model Mech. (2021) 14(2):dmm047753. doi: 10.1242/dmm.047753
3. Gong F, Zhao F, Gan XD. Celastrol protects TGF-beta1-induced endothelial-mesenchymal transition. J Huazhong Univ Sci Technolog Med Sci. (2017) 37(2):185–90. doi: 10.1007/s11596-017-1713-0
4. Qu YB, Zhang GH, Ji YX, Zhua HB, Lv CJ, Jiang WL. Protective role of gambogic acid in experimental pulmonary fibrosis in vitro and in vivo. Phytomedicine. (2016) 23(4):350–8. doi: 10.1016/j.phymed.2016.01.011
5. Hou W, Hu SY, Li C, Ma HB, Wang Q, Meng G, et al. Cigarette smoke induced lung barrier dysfunction, EMT, and tissue remodeling: a possible link between COPD and lung cancer. Biomed Res Int. (2019) 2019:2025636. doi: 10.1155/2019/2025636
6. Yang H, Fu J, Xue X, Yao L, Qiao L, Hou A, et al. Epithelial-mesenchymal transitions in bronchopulmonary dysplasia of newborn rats. Pediatr Pulmonol. (2014) 49(11):1112–23. doi: 10.1002/ppul.22969
7. Yang H, Fu J, Yao L, Hou A, Xue XD. Runx3 is a key modulator during the epithelial-mesenchymal transition of alveolar type II cells in animal models of BPD. Int J Mol Med. (2017) 40(5):1466–76. doi: 10.3892/ijmm.2017.3135
8. Thomas R, Qiao S, Yang X. Th17/Treg imbalance: implications in lung inflammatory diseases. Int J Mol Sci. (2023) 24(5):4865. doi: 10.3390/ijms24054865
9. Sakaguchi S, Mikami N, Wing JB, Tanaka A, Ichiyama K, Ohkura N. Regulatory T cells and human disease. Annu Rev Immunol. (2020) 38:541–66. doi: 10.1146/annurev-immunol-042718-041717
10. Lourenco JD, Ito JT, Martins MA, Tibério IFLC, Lopes FDTQDS. Th17/Treg imbalance in chronic obstructive pulmonary disease: clinical and experimental evidence. Front Immunol. (2021) 12:804919. doi: 10.3389/fimmu.2021.804919
11. Misra R, Shah S, Fowell D, Wang HY, Scheible K, Misra S, et al. Preterm cord blood CD4(+) T cells exhibit increased IL-6 production in chorioamnionitis and decreased CD4(+) T cells in bronchopulmonary dysplasia. Hum Immunol. (2015) 76(5):329–38. doi: 10.1016/j.humimm.2015.03.007
12. Pagel J, Twisselmann N, Rausch TK, Waschina S, Hartz A, Steinbeis M, et al. Increased regulatory T cells precede the development of bronchopulmonary dysplasia in preterm infants. Front Immunol. (2020) 11:565257. doi: 10.3389/fimmu.2020.565257
13. Gillich A, Zhang F, Farmer CG, Travaglini KJ, Tan SY, Gu M, et al. Capillary cell-type specialization in the alveolus. Nature. (2020) 586(7831):785–9. doi: 10.1038/s41586-020-2822-7
14. Eldridge L, Wagner EM. Angiogenesis in the lung. J Physiol. (2019) 597(4):1023–32. doi: 10.1113/JP275860
15. Piera-Velazquez S, Jimenez SA. Endothelial to mesenchymal transition: role in physiology and in the pathogenesis of human diseases. Physiol Rev. (2019) 99(2):1281–324. doi: 10.1152/physrev.00021.2018
16. Gorelova A, Berman M, Al GI. Endothelial-to-mesenchymal transition in pulmonary arterial hypertension. Antioxid Redox Signal. (2021) 34(12):891–914. doi: 10.1089/ars.2020.8169
17. Rosen D, Lee JH, Cuttitta F, Rafiqi F, Degan S, Sunday ME. Accelerated thymic maturation and autoreactive T cells in bronchopulmonary dysplasia. Am J Respir Crit Care Med. (2006) 174(1):75–83. doi: 10.1164/rccm.200511-1784OC
18. Angusamy S, Mansour T, Abdulmageed M, Han R, Schutte BC, LaPres J, et al. Altered thymocyte and T cell development in neonatal mice with hyperoxia-induced lung injury. J Perinat Med. (2018) 46(4):441–9. doi: 10.1515/jpm-2016-0234
19. Turunen R, Vaarala O, Nupponen I, Kajantie E, Siitonen S, Lano A, et al. Activation of T cells in preterm infants with respiratory distress syndrome. Neonatology. (2009) 96(4):248–58. doi: 10.1159/000220764
20. Zhu J. T helper cell differentiation, heterogeneity, and plasticity. Cold Spring Harb Perspect Biol. (2018) 10(10):a030338. doi: 10.1101/cshperspect.a030338
21. Barbi J, Pardoll D, Pan F. Treg functional stability and its responsiveness to the microenvironment. Immunol Rev. (2014) 259(1):115–39. doi: 10.1111/imr.12172
22. Savai R, Pullamsetti SS, Kolbe J, Bieniek E, Voswinckel R, Fink L, et al. Immune and inflammatory cell involvement in the pathology of idiopathic pulmonary arterial hypertension. Am J Respir Crit Care Med. (2012) 186(9):897–908. doi: 10.1164/rccm.201202-0335OC
23. Mittal M, Siddiqui MR, Tran K, Reddy SP, Malik AB. Reactive oxygen species in inflammation and tissue injury. Antioxid Redox Signal. (2014) 20(7):1126–67. doi: 10.1089/ars.2012.5149
24. Zhang W, Liu X, Zhu Y, Liu X, Gu YT, Dai XY, et al. Transcriptional and posttranslational regulation of Th17/Treg balance in health and disease. Eur J Immunol. (2021) 51(9):2137–50. doi: 10.1002/eji.202048794
25. Lee SH, Goswami S, Grudo A, Song LZ, Bandi V, Goodnight-White S, et al. Antielastin autoimmunity in tobacco smoking-induced emphysema. Nat Med. (2007) 13(5):567–9. doi: 10.1038/nm1583
26. Capasso L, Vento G, Loddo C, Tirone C, Iavarone F, Raimondi F, et al. Oxidative stress and bronchopulmonary dysplasia: evidences from microbiomics, metabolomics, and proteomics. Front Pediatr. (2019) 7:30. doi: 10.3389/fped.2019.00030
27. Stevens RP, Paudel SS, Johnson SC, Stevens T, Lee JY. Endothelial metabolism in pulmonary vascular homeostasis and acute respiratory distress syndrome. Am J Physiol Lung Cell Mol Physiol. (2021) 321(2):L358–76. doi: 10.1152/ajplung.00131.2021
28. Dolma K, Freeman AE, Rezonzew G, Payne GA, Xu X, Jilling T, et al. Effects of hyperoxia on alveolar and pulmonary vascular development in germ-free mice. Am J Physiol Lung Cell Mol Physiol. (2020) 318(2):L421–8. doi: 10.1152/ajplung.00316.2019
Keywords: regulatory T cell, endothelial-mesenchymal transition, human pulmonary microvascular endothelial cell, hyperoxia exposure, bronchopulmonary dysplasia
Citation: Sun Y, Yan C, Liu Y, Lin Y, Weng B, Gong X and Cai C (2024) Hyperoxia exposure promotes endothelial–mesenchymal transition and inhibits regulatory T cell function in human pulmonary microvascular endothelial cells. Front. Pediatr. 12: 1295868. doi: 10.3389/fped.2024.1295868
Received: 17 September 2023; Accepted: 4 July 2024;
Published: 23 July 2024.
Edited by:
Yusei Ohshima, University of Fukui, JapanReviewed by:
Alvaro Coronado Munoz, Children’s Hospital at Montefiore, United StatesRoopa Siddaiah, Penn State Milton S. Hershey Medical Center, United States
© 2024 Sun, Yan, Liu, Lin, Weng, Gong and Cai. This is an open-access article distributed under the terms of the Creative Commons Attribution License (CC BY). The use, distribution or reproduction in other forums is permitted, provided the original author(s) and the copyright owner(s) are credited and that the original publication in this journal is cited, in accordance with accepted academic practice. No use, distribution or reproduction is permitted which does not comply with these terms.
*Correspondence: Cheng Cai, Y2FpY2hlbmcyMDA0QDE2My5jb20=