- 1Department of Pediatrics, University of Miami Miller School of Medicine, Miami, FL, United States
- 2Batchelor Children’s Research Institute, University of Miami Miller School of Medicine, Miami, FL, United States
- 3Holtz Children’s Hospital, Jackson Memorial Medical Center, Miami, FL, United States
- 4Interdisciplinary Stem Cell Institute, University of Miami Miller School of Medicine, Miami, FL, United States
Extracellular vesicles (EVs) are a heterogeneous group of nano-sized membranous structures increasingly recognized as mediators of intercellular and inter-organ communication. EVs contain a cargo of proteins, lipids and nucleic acids, and their cargo composition is highly dependent on the biological function of the parental cells. Their cargo is protected from the extracellular environment by the phospholipid membrane, thus allowing for safe transport and delivery of their intact cargo to nearby or distant target cells, resulting in modification of the target cell's gene expression, signaling pathways and overall function. The highly selective, sophisticated network through which EVs facilitate cell signaling and modulate cellular processes make studying EVs a major focus of interest in understanding various biological functions and mechanisms of disease. Tracheal aspirate EV-miRNA profiling has been suggested as a potential biomarker for respiratory outcome in preterm infants and there is strong preclinical evidence showing that EVs released from stem cells protect the developing lung from the deleterious effects of hyperoxia and infection. This article will review the role of EVs as pathogenic messengers, biomarkers, and potential therapies for neonatal lung diseases.
1. Introduction
Airway cells are often exposed to microbes, environmental insults such as hyperoxia, hypoxia, and mechanical stimuli. These ecological cues induce airway injury, inflammatory responses, and repair processes in the respiratory system. Coordinated intercellular communication is required to maintain lung homeostasis. However, constant exposure to these environmental insults can damage the epithelial barrier leading to excessive inflammatory responses and lung pathology. In the last decade, extracellular vesicles (EVs) have been recognized as important mediators of lung homeostasis and disease (1).
EVs are nano-sized particles characterized based on their physical properties such as size (small EVs are <200 nm and large or medium EVs are >200 nm) or density (low, middle or high), biochemical composition (CD63+/CD81− EVs, Annexin A5 EVs, etc.) and description of conditions or cells of origin (lung epithelial cell-derived EVs, podocyte-derived EVs, hypoxia-induced EVs, etc.) (2). EVs contain a cargo of cell-specific lipids, proteins, metabolites, and nucleotides that influence the molecular and functional properties of neighboring and distant target cells (2).
EVs are also categorized based on how they are generated (2). EVs generated by directly budding of the cell plasma membrane have been termed microvesicles, and these are typically 100–1,000 nm in size (3). On the other hand, exosomes (30–100 nm in diameter) are formed from exocytosis of intraluminal vesicles (ILVs). ILVs are generated by endocytosis of cellular cargo (proteins, lipids, metabolites, nucleotides), forming endosomes and subsequently multivesicular bodies (MVBs). MVBs are transported to the plasma membrane through the cytoskeletal and microtubule network. They undergo fusion with the plasma membrane and secretion of ILVs into the extracellular space as exosomes (4). This is regulated by various signaling mechanisms and stimuli, including receptor activation by adenosine triphosphate (ATP) and lipopolysaccharide (LPS) (5, 6). The process also involves the assembly of SNAREs (soluble N-ethylmaleimide-sensitive fusion protein attachment protein receptors) complexes, which draw opposing membranes together to create the energy required for membrane fusion (7). Microvesicles are released through the outward budding and fission of the plasma membrane; this is calcium dependent and associated with cytoskeleton remodeling (3, 8–10).
Specific combinations of proteins and lipids such as tetraspanins, adhesion molecules, glycoproteins, cholesterol, sphingomyelin, and antigen presenting molecules are present on the surface of EVs (2). The exact composition is however dependent on the EV cellular origin, pathogenic conditions, and the mechanism of biogenesis (2). These proteins and lipids influence cellular transport, target cell identification and reception, cargo sorting, and cell programming (8).
EVs are produced by almost all cell types in the respiratory tract (11). Cell types already studied include alveolar type II pneumocytes, pulmonary vascular endothelial cells (PVECs), macrophages, mast cells, and fibroblasts. Under stress such as infection, oxidative stress, and mechanical stress, EVs released by injured lung cells contribute to the development of lung pathologies (12). In addition, lung cell-derived EVs may serve as biomarkers for lung disease risk and severity (11). We will review the mechanisms by which EVs induce lung pathology, the role of EVs as biomarkers in both adult and neonatal lung diseases, and the potential of EVs as vehicles for drug delivery (Figure 1).
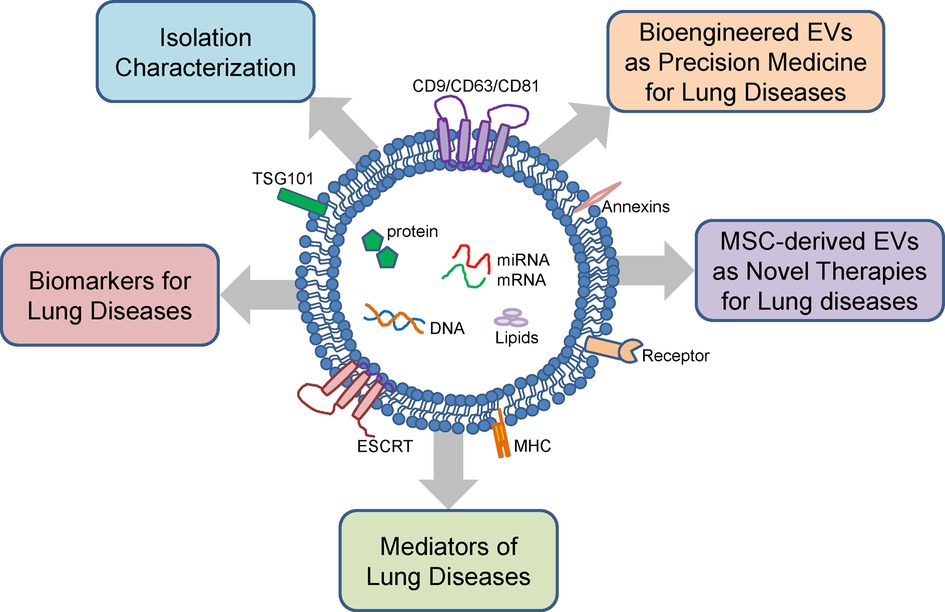
Figure 1. Structure, cargo and function of extracellular vesicles. Extracellular vesicles (EVs) are composed of a lipid bilayer containing transmembrane proteins with cargo consisting of proteins, mRNA, miRNA, DNA, and lipids. EVs can be isolated from various body fluids and have diverse sizes ranging from 100 to 1,000 nm. EVs isolated from the lung fluids and peripheral blood can be used as biomarkers for neonatal lung diseases. EVs have also been linked to the pathogenesis neonatal lung diseases. Mesenchymal stromal cell (MSC)-derived EVs and bioengineered EVs are potential novel therapies for neonatal lung diseases.
2. EV isolation
The EV membrane is composed of a phospholipid bilayer containing major histocompatibility complex molecules and tetraspanins. A major challenge of EV research however is achieving high purity EVs while maintaining their integrity and biological activity. Table 1 summarizes common methods of EV isolation.
Ultracentrifugation is considered the gold standard. It utilizes extremely high centrifugal forces to separate EVs from other biological particles, is affordable and requires little technical skill. However, ultracentrifugation as a purification method is time-consuming, prone to contamination by other particles of similar weight and density, and EV integrity and bioactivity may not be preserved after ultracentrifugation (13, 14, 21).
Ultrafiltration employs physical filters of varying pore sizes and properties like application of electric charge and transmembrane pressure. Ultrafiltration can also be combined with other techniques such as low-speed centrifugation, ultracentrifugation, or size-exclusion chromatography (15). The process of ultrafiltration is simple to perform. However, there are limitations to sample processing – low-yield, membranes clogging, damage to EVs, types of samples that can be ultrafiltered, and the process of ultrafiltration is time-consuming (9).
Size-exclusion chromatography (SEC) is an increasingly popular technique that involves running samples through porous beads leading to separation of molecules by size. EVs isolated by SEC are biologically intact, making this method ideal for functional research (16). When used in conjunction with ultracentrifugation, EV yield and purity are significantly increased (17). The polymer precipitation method utilizes reagents such as polyethylene glycol (PEG) to cause precipitation of EVs, allowing isolation of EVs by simple centrifugation, making it cost-effective and efficient, but prone to contamination (18).
The immunoaffinity technique is done by priming a medium with target antibodies to bind with specific surface antigens or receptors present on EVs of interest. This isolates EVs with high purity, but this method is costly and difficult to sustain. Membrane-based separation methods isolate EVs through binding of membrane hydrophilic phosphate of EVs to metal oxides or the negatively charged membranes to positively charged molecules. This method is high yield, efficient and has high purity rates (19).
Microfluidic platforms are sophisticated networks utilizing various methods of purification organized in a miniature device. Purification methods include immunoaffinity, membrane-based filtration, nanowire trapping, acoustic nanofiltration, deterministic lateral displacement, and viscoelastic flow sorting. Microfluidic devices can achieve high throughput, high yield and high purity EVs, but there is a lack of standardization of devices contributing to heterogeneity of results reported by multiple investigators utilizing various devices (20).
3. EV characterization
Analyzing the particle size, morphology and biocomposition of EVs by multiple, complementary techniques is critical in evaluating the likelihood that biomarkers or functions are associated with EVs and not other co-isolated materials (2).
The International Society for Extracellular Vesicles has proposed the Minimal Information for Studies of Extracellular Vesicles-2018 (MISEV2018) guidelines, which recommends that the source and preparation of the EV must be described quantitatively (2). MISEV2018 also recommends using techniques that provide images of single EVs at high resolution such as electron microscopy, using single particle analysis techniques that estimate biophysical features of EVs, and assessing the topology of EV-associated components (2). The commonly used EV characterization techniques are listed in Table 2.
Dynamic light scattering can be used to measure particle size, but analysis is limited when EVs of various sizes are present, and this cannot be used for functional analysis (22, 23). High resolution flow cytometry is a reliable and popular technique that enables structural analysis, quantification, and functional EV characterization (26). Nanoparticle tracking is a method by which the concentration, size distribution and particle velocity of EVs are measured. In nanoparticle tracking, specific antigens can also be identified with fluorescent tagged antibodies, providing more functional information (22). Atomic force microscopy is a technique that provides outputs of EV quantity, morphology, structural and functional analysis at a molecular level. This technique also preserves the integrity and bioactivity of EVs (24). Electron microscopy (EM) can also be used for structural characterization of EVs. EVs can be visualized with transmission EM, with a characteristic cup-shaped appearance of EVs due to dehydration during sample processing (25). Cryo-EM, on the other hand, allows for visualization of intact EVs without dehydration, enabling ultrastructural analysis of EV membranes and contents (31). EV membrane and cargo components can be analyzed with techniques according to molecule type, such as Western Blot and mass spectroscopy for proteins, and microarray and next generation sequencing for DNA or RNA (27–30).
Given the wide range of techniques available for both isolation and characterization of EVs with varying qualities, and with numerous research studies focusing on EVs that have been reported and are ongoing, there is a need for standardization of research protocols and techniques to maximize knowledge-sharing and productivity of the scientific community. Efforts are being made through the International Society for Extracellular Vesicles (ISEV) to create task forces and research guidelines to overcome these challenges (32, 33).
4. EVs in the pathogenesis of lung diseases
Increasing evidence indicates that EVs play essential roles in the pathogenesis of various adult lung diseases, including acute lung injury (ALI), acute respiratory distress syndrome (ARDS), asthma, chronic obstructive pulmonary disease (COPD), and pulmonary hypertension. The involvement of EVs in neonatal lung diseases has also been reported in bronchopulmonary dysplasia (BPD), but much less is known.
4.1. EVs and adult lung diseases
ALI and ARDS are devastating and rapidly progressive respiratory disorders that are characterized by disruption of the integrity of alveolar and vascular endothelial barriers (34–36). In response to inflammatory stimuli, EVs and microparticles (MPs) are released from circulating inflammatory cells, damaged PVECs, and epithelial cells (37). In preclinical models, PVEC-derived EVs induce significant lung injury, as demonstrated by alveolar-capillary barrier failure, lung edema, and neutrophil infiltration in mice (38). These pathological effects are linked to and presumably at least in part mediated by the detrimental effects of PVEC-derived EVs on endothelial function. In ALI models, PVEC-derived EVs induce a reduction in endothelial nitric oxide (NO) production and an increased release of lung inflammatory cytokines (39).
Alveolar macrophage derived EVs are also abundant in the bronchoalveolar lavage fluid (BALF) in animal models of ALI. They are capable of inducing inflammatory responses both in vivo and in vitro (37, 40–42). Alveolar macrophage derived EVs trigger EV release by epithelial cells and neutrophils and deliver high concentrations of TNF-α to alveolar epithelial cells, leading to increased production of keratinocyte-derived chemokine and intercellular adhesion molecule-1 (37, 40–42), inducing a vicious cycle of inflammatory injury.
Alveolar epithelial cell derived EVs are also important mediators of ALI. In hyperoxia-induced ALI, alveolar epithelial cell-derived EVs are increased in BALF and serum (43) and they activate proinflammatory responses in systemic and pulmonary macrophages leading to disease progression (44).
COPD is characterized by severe airway inflammation and subsequent lung parenchymal damage. Mononuclear/macrophage-derived EVs rich in inflammatory mediators such as cytokines, chemokines, adhesion molecules, and proteases have been linked to alveolar wall destruction and emphysema, the hallmarks of COPD (11, 45). Endothelial-derived microparticles can promote the progression of COPD by inducing apoptosis of neighboring health endothelial cells upon delivery of inflammatory cargo (46). Epithelial-derived EVs have also been linked to the pathogenesis of COPD. Cigarette smoke stimulates human bronchial epithelial cells to release EVs enriched in full-length CYR61/CTGF/NOV family 1 (CCN1) protein that not only mediates IL-18 induced inflammation but also helps maintain lung homeostasis by increasing the levels of vascular endothelial growth factor (VEGF) (47). Cigarette smoke extract-induced human bronchial epithelial cell-derived EVs promote myofibroblast differentiation of lung fibroblasts, leading to the development of fibrosis (48). Cigarette smoke-exposed lung epithelial cells also release EVs that contain pro-inflammatory cytokines and Wnt-5a into the circulation, and these EVs can reach distant cells and organs (49).
EVs are also implicated in the pathogenesis of pulmonary hypertension. Patients with pulmonary arterial hypertension (PAH) have increased endothelial-derived CD62e microparticles in their pulmonary arterial blood (50). PAH patients also have increased microparticles positive for endothelial PECAM and VE-cadherin in their plasma samples (51). In monocrotaline-induced PAH, lung- and plasma-derived small-sized EVs isolated from monocrotaline-exposed mice induce PAH in healthy mice (52). EVs from PAH mice and patients contain elevated levels of miR-19b, miR-20a, miR-20b, and miR-145, known to target bone morphogenesis protein receptor signaling, apoptosis, and cell proliferation. EVs from the lungs of PAH mice reduce apoptosis of PVECs (53). Furthermore, EVs released by PVECs from PAH mice convert healthy bone marrow-derived endothelial progenitor cells into a pathological progenitor phenotype. These cells induce pulmonary vascular remodeling when injected into the lungs of healthy mice (54).
4.2. EVs and bronchopulmonary dysplasia (BPD)
BPD is the most common adverse outcome of extreme prematurity (55). It is the result of antenatal injury to the developing lung combined with repetitive and multiple post-natal insults, including oxygen therapy and ventilation, leading to alveolar simplification and vascular rarefaction (55). Not much is, however, known about the role of EVs in BPD pathogenesis. Genschmer and collaborators compared the function of EVs derived from BALF from BPD and non-BPD infants in a murine model (56). Intriguingly, mice that received intranasal BPD-derived EVs had significant alveolar hypoplasia and right ventricular hypertrophy, suggesting a role for EVs in BPD pathogenesis (56).
Recently, Lal et al. also demonstrated that the tracheal aspirate of infants with severe BPD had higher EV particle concentrations as compared to control infants, and the majority of these EVs were derived from epithelial cells (57). EVs shed from hyperoxia and LPS-exposed epithelial cells had reduced miR-876-3p. Gain of miR-876-3p in murine models attenuated hyperoxia and LPS-induced alveolar simplification, highlighting a potential critical role of lung epithelial cell-derived EV-miRNAs in the pathogenesis of BPD (57). miRNAs are non-coding RNAs that bind to sequences in the 3′ untranslated region (3′UTR) of target mRNA, resulting in the destruction of target mRNA or its repression (58).
Recently, our laboratory investigated the critical role of circulating EVs from hyperoxia-exposed and mechanical ventilated newborn rats in inducing brain injury in healthy newborn rats (59, 60). In the hyperoxia model, newborn rats were exposed to room air or 85% oxygen for two weeks, and circulating EVs were isolated from the plasma of these rats. Fluorescence activated cell sorting (FACS) and Western blot analyses demonstrated that the EVs from hyperoxia-exposed rats contain increased levels of both surfactant C (SPC) and gasdermin D (GSDMD), a key executor of inflammasome-induced cell pyroptosis. When these EVs were adoptively transferred into healthy newborn rats by intra-tail vein injection, they were taken up by the lung and brain. In the lung, the EVs from the hyperoxia-exposed rats induced inflammation, indicated by increased inflammatory cell infiltration in the alveolar airspaces and expression of inflammatory cytokines and chemokines. Furthermore, alveolarization and vascular density were drastically reduced in the lungs that received EVs from hyperoxia-exposed rats. In vitro experiments with PVECs demonstrated reduced cell proliferation and increased cell death when cultured with EVs from hyperoxia-exposed rats (59). Upon examining the brain, EVs from hyperoxia-exposed rats induced brain inflammation by activating microglia and increasing expression of pro-inflammatory cytokines. These changes were associated with increased cell death in the cortex, subventricular zone, and subgranular zone. Additionally, in vitro experiments showed that neural stem cells (NSC) had decreased proliferation and increased cell death when cultured with EVs from hyperoxia-exposed rats (59). EVs from cultured hyperoxia-exposed lung epithelial cells induced pyroptosis in NSC (59). This data revealed a novel lung-brain crosstalk mediated by lung epithelial-derived EVs in both lung and brain injury.
This EV-mediated lung-brain crosstalk was further investigated in mechanical ventilation-associated brain injury in newborn rat models (60). We demonstrated that injurious mechanical ventilation induced similar markers of inflammation and pyroptosis, such as IL-1β and activated caspase-1/GSDMD in both lung and brain, in addition to inducing microglial activation and cell death in the brain (60). EVs isolated from neonatal rats with ventilator-induced lung injury had increased caspase-1. Adoptive transfer of these EVs into healthy newborn rats led to neuroinflammation with microglial activation and activation of caspase-1 and GSDMD in the brain, similar to that observed in neonatal rats that were mechanically ventilated (60). Thus, circulating EVs can contribute to brain injury and possibly poor neurodevelopmental outcomes in preterm infants exposed to hyperoxia and mechanical ventilation (60).
5. EVs as biomarkers for lung diseases
The stability of EVs is a potential advantage over traditional biomarkers. Traditional biomarkers such as proteins and RNA molecules are often unstable and susceptible to degradation over time, making them less reliable for diagnostic purposes. In contrast, EVs are surrounded by a protective lipid membrane that helps to stabilize their contents, including proteins, nucleic acids, and other molecular components (61). Proteomic and phosphoproteomic studies conducted on EVs from different cell types have suggested that they transport a diverse range of biologically relevant molecules, such as lipids, carbohydrates, RNAs, and some are believed to exhibit heterogeneity in composition, which is dependent on their cellular origin (62). EVs can carry specific proteins or RNA molecules that are unique to lung diseases. For example, sputum of patients with severe asthma has elevated levels of miR-142-3p, miR-629-3p, and miR-223-3p (63), and sputum-derived EVs from idiopathic pulmonary fibrosis (IPF) patients show an aberrant expression of miR-142-3p, miR-33a-5p, and let-7d-5p compared to healthy subjects (64).
There are few reports that EV-miRNAs can be used as biomarkers for BPD (65). In the study by Lal et al., EV miR876-3p was a potential biomarker for severe BPD in preterm infants. Decreased expression of EV miR-876-3p at birth predicted the future development of severe BPD in ELBW infants (57). This study established the predictive potential and causative role of microbiota-regulated miR-876-3p in severe BPD (57).
More recently, Ransom et al. characterized tracheal aspirate EVs in preterm infants between 22- and 35-week gestational age. Across all gestational ages, the majority of tracheal aspirate EVs expressed epithelial and immune cell markers. Moreover, infants who developed BPD had increased CD14+ EVs in their first tracheal aspirate obtained within 24 h of birth (66).
6. EVs as therapies for neonatal lung diseases
Mesenchymal stromal cells (MSCs) have regenerative properties and it is increasingly known that MSC-derived EVs replicate many of the beneficial effects of MSCs. EVs may also be bioengineered for drug delivery and genetically modified to carry specific target molecules. Although these therapeutic strategies are in the early stage of development, the prospect of using them in newborn infants is encouraging.
6.1. Stem cell derived EVs for newborn lung diseases
MSCs are efficacious in neonatal lung injury models (67–69). The pleiotropic properties of these cells make them particularly attractive and given their paracrine-mediated mechanism of action, MSC-derived EVs have been investigated as potential therapies.
In an experimental model of chorioamnionitis, antenatal administration of MSC-EVs reduced placental inflammation, and preserved lung structure, suggesting that antenatal MSC-EVs are efficacious in alleviating the deleterious effects of intrauterine inflammation. In experimental pre-eclampsia, MSC-EVs restore placental vascularity and preserve neonatal lung structure (70). In experimental BPD models, MSC-derived EVs restore alveolar structure, prevent lung vascular rarefaction, and alleviate PH by altering macrophage polarization, reprogramming bone marrow myeloid cells and increasing pro-angiogenic signaling pathways (70–77).
We recently compared the therapeutic efficacy of intra-tracheal (IT) and intravenously (IV) delivered MSC-EVs in a preclinical model of BPD. We demonstrated that systemically and IT delivered MSC-EVs have similar beneficial effects in experimental BPD (78). This finding is promising as IV MSC-EVs may also have beneficial effects on the developing brain (79). Another important question which we recently sought to address is the duration of MSC-EV therapeutic effects in experimental BPD. We administered MSC-EVs to neonatal pups with hyperoxia-induced BPD on postnatal day 3 and followed the pups into young adulthood (78). We found that one dose of MSC-EVs at postnatal day 3 had persistent beneficial effects at three month follow up (78). Importantly, late administration of MSC-EVs in an established BPD model was also found to partially reverse lung injury (79, 80). Clinical trials are now on the horizon but identifying the ideal patient will be critical.
6.2. Engineered EVs
EVs are also being investigated as “drug vehicles” (81). The ability of EVs to target a particular tissue or cell could be used to deliver drugs to intended targets while avoiding off-targets selectively (81). The “drug cargo” is selectively loaded into the EVs and the EVs are engineered to have specific properties to enhance their targeting and biomimetic features (82, 83). The lower number of transmembrane proteins, such as MHC complexes on their surface, make EVs less immunogenic than their parental source (84, 85). In addition, EVs do not replicate after injection. Thus, EVs are less likely to transfer latent viral pathogens or enable tumor generation (86). Compared to synthetic drug carriers, the intrinsic ability of EVs to cross cell barriers and penetrate tissues gives them an advantage (87). Synthetic drug carriers such as polymeric micelles and lipid nanoparticles cause high toxicity and immunogenicity compared to EVs (88). As therapeutic EVs are derived from benign biological or autologous sources, they are less likely to induce adverse effects.
Harnessing these unique properties of EVs to develop smart drug delivery systems with enhanced targeting, safety and pharmacokinetics has however been challenging (89). One study showed that that after intravenous injection, EVs are rapidly distributed and retained in the liver, spleen, gastrointestinal tract and lungs (90). Another study however showed rapid clearance of plasma-derived EVs following intravenous administration, with a half-life of approximately 7 min (91). Moving forward, more studies will be needed to understand EV circulation kinetics, biodistribution, cell tropism, and intracellular trafficking routes as the cellular origin, dose and route of administration may affect EV biodistribution pattern (92).
Other obstacles such as low isolation yield, the lack of purification protocols, large-scale clinical grade production, parental cell-dependent composition, and inefficient drug payload of the EVs continue to hamper the therapeutic ability of EVs (93). To improve de novo EV yield and therapeutic efficacy, re-engineering of the parental cell has been done through genome modification, stimulation with exogenous biomolecules and specific environmental factors (93). Bioreactors are also being extensively used to scale up the production of cell-based therapy and EVs. Bioreactors provide well-controlled nutrients, uniform culture conditions and biomimetic stimuli to regulate cell growth, differentiation and tissue development (94). While bioengineering of the parental cell predictably loads only a small proportion of the modified content into EVs, direct modification of isolated EVs may be another strategy to enrich EVs (95). For example, hydrophobically modified small interfering RNAs efficiently load into EVs upon coincubation, without altering EV size or integrity (96). Active EV loading can also be done by electroporation, sonication, extrusion, freeze-thawing and by surfactant-assisted loading, where surfactant saponin disrupts the membrane and increases its permeability (97).
Another option currently being investigated is the development of artificial EVs, namely the top-down and bottom-up approaches. The top-down approach is based on the disruption of the cultured cells to produce membrane fragments that will be used to form vesicles, while retaining the same membrane features of the initial cell (98). The bottom-up approach starts from small components of molecular building blocks to create complex structures, namely synthetic EVs (99).
7. Conclusion
We presented the evidence for lung-derived EVs as novel biomarkers and mediators for neonatal lung diseases and the potential for MSC-derived EVs as novel therapeutic modalities for neonatal lung diseases. Many of the studies discussed in this review are preclinical investigations that require successful translation from the bench to the bedside. Given that lung diseases are among the most common complications in preterm infants, with few effective therapies, it is crucial to continue discovering and understanding how EVs contribute to neonatal lung diseases and how to harness EVs to prevent and treat neonatal lung diseases. The incredible features of EVs in terms of their biocompatibility, cargo loading, cellular uptake, and escaping the immune system make them an appealing therapeutic strategy, but determining the ideal patient, route, dosing and timing will be essential to move forward. Procurement of EVs from physiologically relevant environments, the ability to scale up their manufacturing, optimize their biodistribution, and in vivo kinetics will also be crucial (93). This will contribute immensely to increasing the potential of EVs as acellular nanoscale therapeutics for neonatal lung diseases.
Author contributions
SW, MB, JD, KW, AT, AS, KY: conceived, drafted and reviewed the final submitted version of the manuscript. All authors contributed to the article and approved the submitted version.
Conflict of interest
The authors declare that the research was conducted in the absence of any commercial or financial relationships that could be construed as a potential conflict of interest.
Publisher's note
All claims expressed in this article are solely those of the authors and do not necessarily represent those of their affiliated organizations, or those of the publisher, the editors and the reviewers. Any product that may be evaluated in this article, or claim that may be made by its manufacturer, is not guaranteed or endorsed by the publisher.
References
1. Raposo G, Stahl PD. Extracellular vesicles: a new communication paradigm? Nat Rev Mol Cell Biol. (2019) 20:509–10. doi: 10.1038/s41580-019-0158-7
2. Théry C, Witwer KW, Aikawa E, Alcaraz MJ, Anderson JD, Andriantsitohaina R, et al. Minimal information for studies of extracellular vesicles 2018 (MISEV2018): a position statement of the international society for extracellular vesicles and update of the MISEV2014 guidelines. J Extracell Vesicles. (2018) 7:1535750. doi: 10.1080/20013078.2018.1535750
3. Heijnen HF, Schiel AE, Fijnheer R, Geuze HJ, Sixma JJ. Activated platelets release two types of membrane vesicles: microvesicles by surface shedding and exosomes derived from exocytosis of multivesicular bodies and alpha-granules. Blood. (1999) 94:3791–9. doi: 10.1182/blood.V94.11.3791
4. Harding C, Heuser J, Stahl P. Receptor-mediated endocytosis of transferrin and recycling of the transferrin receptor in rat reticulocytes. J Cell Biol. (1983) 97:329–39. doi: 10.1083/jcb.97.2.329
5. Bianco F, Pravettoni E, Colombo A, Schenk U, Möller T, Matteoli M, et al. Astrocyte-derived ATP induces vesicle shedding and IL-1 beta release from microglia. J Immunol. (2005) 174:7268–77. doi: 10.4049/jimmunol.174.11.7268
6. Gambim MH, de Oliveira do Carmo A, Marti L, Veríssimo-Filho S, Lopes LR, Janiszewski M. Platelet-derived exosomes induce endothelial cell apoptosis through peroxynitrite generation: experimental evidence for a novel mechanism of septic vascular dysfunction. Critical Care. (2007) 11:R107. doi: 10.1186/cc6133
7. Fukuda R, McNew JA, Weber T, Parlati F, Engel T, Nickel W, et al. Functional architecture of an intracellular membrane t-SNARE. Nature. (2000) 407:198–202. doi: 10.1038/35025084
8. Colombo M, Raposo G, Théry C. Biogenesis, secretion, and intercellular interactions of exosomes and other extracellular vesicles. Annu Rev Cell Dev Biol. (2014) 30:255–89. doi: 10.1146/annurev-cellbio-101512-122326
9. Taylor J, Azimi I, Monteith G, Bebawy M. Ca(2+) mediates extracellular vesicle biogenesis through alternate pathways in malignancy. J Extracell Vesicles. (2020) 9:1734326. doi: 10.1080/20013078.2020.1734326
10. Nabhan JF, Hu R, Oh RS, Cohen SN, Lu Q. Formation and release of arrestin domain-containing protein 1-mediated microvesicles (ARMMs) at plasma membrane by recruitment of TSG101 protein. Proc Natl Acad Sci U S A. (2012) 109:4146–51. doi: 10.1073/pnas.1200448109
11. Kubo H. Extracellular vesicles in lung disease. Chest. (2018) 153:210–6. doi: 10.1016/j.chest.2017.06.026
12. Kesimer M, Scull M, Brighton B, DeMaria G, Burns K, O'Neal W, et al. Characterization of exosome-like vesicles released from human tracheobronchial ciliated epithelium: a possible role in innate defense. FASEB J. (2009) 23:1858–68. doi: 10.1096/fj.08-119131
13. Coughlan C, Bruce KD, Burgy O, Boyd TD, Michel CR, Garcia-Perez JE, et al. Exosome isolation by ultracentrifugation and precipitation and techniques for downstream analyses. Curr Protoc Cell Biol. (2020) 88:e110. doi: 10.1002/cpcb.110
14. Jeppesen DK, Hvam ML, Primdahl-Bengtson B, Boysen AT, Whitehead B, Dyrskjot L, et al. Comparative analysis of discrete exosome fractions obtained by differential centrifugation. J Extracell Vesicles. (2014) 3:25011. doi: 10.3402/jev.v3.25011
15. Benedikter BJ, Bouwman FG, Vajen T, Heinzmann ACA, Grauls G, Mariman EC, et al. Ultrafiltration combined with size exclusion chromatography efficiently isolates extracellular vesicles from cell culture media for compositional and functional studies. Sci Rep. (2017) 7:15297. doi: 10.1038/s41598-017-15717-7
16. Sidhom K, Obi PO, Saleem A. A review of exosomal isolation methods: is size exclusion chromatography the best option? Int J Mol Sci. (2020) 21(18):6466. doi: 10.3390/ijms21186466
17. Wei R, Zhao L, Kong G, Liu X, Zhu S, Zhang S, et al. Combination of size-exclusion chromatography and ultracentrifugation improves the proteomic profiling of plasma-derived small extracellular vesicles. Biol Proced Online. (2020) 22:12. doi: 10.1186/s12575-020-00125-5
18. Martinez-Greene JA, Hernandez-Ortega K, Quiroz-Baez R, Resendis-Antonio O, Pichardo-Casas I, Sinclair DA, et al. Quantitative proteomic analysis of extracellular vesicle subgroups isolated by an optimized method combining polymer-based precipitation and size exclusion chromatography. J Extracell Vesicles. (2021) 10:e12087. doi: 10.1002/jev2.12087
19. Deregibus MC, Figliolini F, D'Antico S, Manzini PM, Pasquino C, De Lena M, et al. Charge-based precipitation of extracellular vesicles. Int J Mol Med. (2016) 38:1359–66. doi: 10.3892/ijmm.2016.2759
20. Gao F, Jiao F, Xia C, Zhao Y, Ying W, Xie Y, et al. A novel strategy for facile serum exosome isolation based on specific interactions between phospholipid bilayers and TiO2. Chem Sci. (2019) 10:1579–88. doi: 10.1039/C8SC04197K
21. Linares R, Tan S, Gounou C, Arraud N, Brisson AR. High-speed centrifugation induces aggregation of extracellular vesicles. J Extracell Vesicles. (2015) 4:29509. doi: 10.3402/jev.v4.29509
22. Filipe V, Hawe A, Jiskoot W. Critical evaluation of nanoparticle tracking analysis (NTA) by NanoSight for the measurement of nanoparticles and protein aggregates. Pharm Res. (2010) 27:796–810. doi: 10.1007/s11095-010-0073-2
23. Palmieri V, Lucchetti D, Gatto I, Maiorana A, Marcantoni M, Maulucci G, et al. Dynamic light scattering for the characterization and counting of extracellular vesicles: a powerful noninvasive tool. J Nanopart Res. (2014) 16:2583. doi: 10.1007/s11051-014-2583-z
24. Sharma S, LeClaire M, Gimzewski JK. Ascent of atomic force microscopy as a nanoanalytical tool for exosomes and other extracellular vesicles. Nanotechnol. (2018) 29:132001. doi: 10.1088/1361-6528/aaab06
25. Rikkert LG, Nieuwland R, Terstappen L, Coumans FAW. Quality of extracellular vesicle images by transmission electron microscopy is operator and protocol dependent. J Extracell Vesicles. (2019) 8:1555419. doi: 10.1080/20013078.2018.1555419
26. Headland SE, Jones HR, D’Sa AS, Perretti M, Norling LV. Cutting-edge analysis of extracellular microparticles using ImageStream(X) imaging flow cytometry. Sci Rep. (2014) 4:5237. doi: 10.1038/srep05237
27. Contreras-Naranjo JC, Wu HJ, Ugaz VM. Microfluidics for exosome isolation and analysis: enabling liquid biopsy for personalized medicine. Lab Chip. (2017) 17:3558–77. doi: 10.1039/C7LC00592J
28. De Sousa KP, Rossi I, Abdullahi M, Ramirez MI, Stratton D, Inal JM. Isolation and characterization of extracellular vesicles and future directions in diagnosis and therapy. Wiley Interdiscip Rev Nanomed Nanobiotechnol. (2023) 15:e1835. doi: 10.1002/wnan.1835
29. Tiwari S, Kumar V, Randhawa S, Verma SK. Preparation and characterization of extracellular vesicles. Am J Reprod Immunol. (2021) 85:e13367. doi: 10.1111/aji.13367
30. Hartjes TA, Mytnyk S, Jenster GW, van Steijn V, van Royen ME. Extracellular vesicle quantification and characterization: common methods and emerging approaches. Bioengineering. (2019) 6:7. doi: 10.3390/bioengineering6010007
31. Morandi MI, Busko P, Ozer-Partuk E, Khan S, Zarfati G, Elbaz-Alon Y, et al. Extracellular vesicle fusion visualized by cryo-electron microscopy. PNAS Nexus. (2022) 1:156. doi: 10.1093/pnasnexus/pgac156
32. Royo F, Thery C, Falcon-Perez JM, Nieuwland R, Witwer KW. Methods for separation and characterization of extracellular vesicles: results of a worldwide survey performed by the ISEV rigor and standardization subcommittee. Cells. (2020) 9:1955. doi: 10.3390/cells9091955
33. Lai JJ, Chau ZL, Chen SY, Hill JJ, Korpany KV, Liang NW, et al. Exosome processing and characterization approaches for research and technology development. Advanced Science. (2022) 9:e2103222. doi: 10.1002/advs.202103222
34. Mohan A, Agarwal S, Clauss M, Britt NS, Dhillon NK. Extracellular vesicles: novel communicators in lung diseases. Respir Res. (2020) 21:175. doi: 10.1186/s12931-020-01423-y
35. Shah T, Qin S, Vashi M, Predescu DN, Jeganathan N, Bardita C, et al. Alk5/Runx1 signaling mediated by extracellular vesicles promotes vascular repair in acute respiratory distress syndrome. Clin Transl Med. (2018) 7:19. doi: 10.1186/s40169-018-0197-2
36. Hu Q, Zhang S, Yang Y, Yao JQ, Tang WF, et al. Extracellular vesicles in the pathogenesis and treatment of acute lung injury. Mil Med Res. (2022) 9:61. doi: 10.1186/s40779-022-00417-9
37. Mahida RY, Matsumoto S, Matthay MA. Extracellular vesicles: a new frontier for research in acute respiratory distress syndrome. Am J Respir Cell Mol Biol. (2020) 63:15–24. doi: 10.1165/rcmb.2019-0447TR
38. Buesing KL, Densmore JC, Kaul S, Pritchard KA Jr, Jarzembowski JA, Gourlay DM, et al. Endothelial microparticles induce inflammation in acute lung injury. J Surg Res. (2011) 166:32–9. doi: 10.1016/j.jss.2010.05.036
39. Densmore JC, Signorino PR, Ou J, Hatoum OA, Rowe JJ, Shi Y, et al. Endothelium-derived microparticles induce endothelial dysfunction and acute lung injury. Shock. (2006) 26:464–71. doi: 10.1097/01.shk.0000228791.10550.36
40. McVey MJ, Maishan M, Blokland KEC, Bartlett N, Kuebler WM. Extracellular vesicles in lung health, disease, and therapy. Am J Physiol Lung Cell Mol Physiol. (2019) 316:L977–89. doi: 10.1152/ajplung.00546.2018
41. Li H, Meng X, Liang X, Gao Y, Cai S. Administration of microparticles from blood of the lipopolysaccharide-treated rats serves to induce pathologic changes of acute respiratory distress syndrome. Exp Biol Med. (2015) 240:1735–41. doi: 10.1177/1535370215591830
42. Soni S, Wilson MR, O'Dea KP, Yoshida M, Katbeh U, et al. Alveolar macrophage-derived microvesicles mediate acute lung injury. Thorax. (2016) 71:1020–9. doi: 10.1136/thoraxjnl-2015-208032
43. Moon HG, Cao Y, Yang J, Lee JH, Choi HS, Jin Y. Lung epithelial cell-derived extracellular vesicles activate macrophage-mediated inflammatory responses via ROCK1 pathway. Cell Death Dis. (2015) 6:e2016. doi: 10.1038/cddis.2015.282
44. Lee H, Zhang D, Laskin DL, Jin Y. Functional evidence of pulmonary extracellular vesicles in infectious and noninfectious lung inflammation. J Immunol. (2018) 201:1500–9. doi: 10.4049/jimmunol.1800264
45. Takahashi T, Kubo H. The role of microparticles in chronic obstructive pulmonary disease. Int J Chron Obstruct Pulmon Dis. (2014) 9:303–14. doi: 10.2147/COPD.S38931
46. Letsiou E, Bauer N. Endothelial extracellular vesicles in pulmonary function and disease. Curr Top Membr. (2018) 82:197–256. doi: 10.1016/bs.ctm.2018.09.002
47. Moon HG, Kim SH, Gao J, Quan T, Qin Z, Osorio JC, et al. CCN1 secretion and cleavage regulate the lung epithelial cell functions after cigarette smoke. Am J Physiol Lung Cell Mol Physiol. (2014) 307:L326–37. doi: 10.1152/ajplung.00102.2014
48. Fujita Y, Araya J, Ito S, Kobayashi K, Kosaka N, Yoshioka Y, et al. Suppression of autophagy by extracellular vesicles promotes myofibroblast differentiation in COPD pathogenesis. J Extracell Vesicles. (2015) 4:28388. doi: 10.3402/jev.v4.28388
49. Feller D, Kun J, Ruzsics I, Rapp J, Sarosi V, Kvell K, et al. Cigarette smoke-induced pulmonary inflammation becomes systemic by circulating extracellular vesicles containing Wnt5a and inflammatory cytokines. Front Immunol. (2018) 9:1724. doi: 10.3389/fimmu.2018.01724
50. Amabile N, Heiss C, Chang V, Angeli FS, Damon L, Rame EJ, et al. Increased CD62e(+) endothelial microparticle levels predict poor outcome in pulmonary hypertension patients. J Heart Lung Transplant. (2009) 28:1081–6. doi: 10.1016/j.healun.2009.06.005
51. Amabile N, Heiss C, Real WM, Minasi P, McGlothlin D, Rame EJ, et al. Circulating endothelial microparticle levels predict hemodynamic severity of pulmonary hypertension. Am J Respir Crit Care Med. (2008) 177:1268–75. doi: 10.1164/rccm.200710-1458OC
52. Aliotta JM, Pereira M, Wen S, Dooner MS, Del Tatto M, Papa E, et al. Exosomes induce and reverse monocrotaline-induced pulmonary hypertension in mice. Cardiovasc Res. (2016) 110:319–30. doi: 10.1093/cvr/cvw054
53. Aliotta JM, Pereira M, Amaral A, Sorokina A, Igbinoba Z, Hasslinger A, et al. Induction of pulmonary hypertensive changes by extracellular vesicles from monocrotaline-treated mice. Cardiovasc Res. (2013) 100:354–62. doi: 10.1093/cvr/cvt184
54. Aliotta JM, Pereira M, Wen S, Dooner MS, Del Tatto M, Papa E, et al. Bone marrow endothelial progenitor cells are the cellular mediators of pulmonary hypertension in the murine monocrotaline injury model. Stem Cells Transl Med. (2017) 6:1595–606. doi: 10.1002/sctm.16-0386
55. Thébaud B, Goss KN, Laughon M, Whitsett JA, Abman SH, Steinhorn RH, et al. Bronchopulmonary dysplasia. Nat Rev Dis Primers. (2019) 5:78. doi: 10.1038/s41572-019-0127-7
56. Genschmer KR, Russell DW, Lal C, Szul T, Bratcher PE, Noerager BD, et al. Activated PMN exosomes: pathogenic entities causing matrix destruction and disease in the lung. Cell. (2019) 176:113–26. doi: 10.1016/j.cell.2018.12.002
57. Lal CV, Olave N, Travers C, Rezonzew G, Dolma K, Simpson A, et al. Exosomal microRNA predicts and protects against severe bronchopulmonary dysplasia in extremely premature infants. JCI Insight. (2018) 3:e93994. doi: 10.1172/jci.insight.93994
58. Hu G, Drescher K, Chen X. Exosomal miRNAs: biological properties and therapeutic potential. Front Genet. (2012) 3:56. doi: 10.3389/fgene.2012.00056
59. Ali A, Zambrano R, Duncan MR, Chen S, Luo S, Yuan H, et al. Hyperoxia-activated circulating extracellular vesicles induce lung and brain injury in neonatal rats. Sci Rep. (2021) 11:8791. doi: 10.1038/s41598-021-87706-w
60. Chavez L, Meguro J, Chen S, de Paiva VN, Zambrano R, Eterno JM, et al. Circulating extracellular vesicles activate the pyroptosis pathway in the brain following ventilation-induced lung injury. J Neuroinflammation. (2021) 18:310. doi: 10.1186/s12974-021-02364-z
61. Sivanantham A, Jin Y. Impact of storage conditions on EV integrity/surface markers and cargos. Life. (2022) 12:697. doi: 10.3390/life12050697
62. Guiot J, Struman I, Louis E, Louis R, Malaise M, Njock MS. Exosomal miRNAs in lung diseases: from biologic function to therapeutic targets. J Clin Med. (2019):8(9):1345. doi: 10.3390/jcm8091345
63. Mortaz E, Alipoor SD, Varahram M, Jamaati H, Garssen J, Mumby SE, et al. Exosomes in severe asthma: update in their roles and potential in therapy. Biomed Res Int. (2018) 2018:2862187. doi: 10.1155/2018/2862187
64. Njock MS, Guiot J, Henket MA, Nivelles O, Thiry M, Dequiedt F, et al. Sputum exosomes: promising biomarkers for idiopathic pulmonary fibrosis. Thorax. (2019) 74:309–12. doi: 10.1136/thoraxjnl-2018-211897
65. Schiller EA, Cohen K, Lin X, El-Khawam R, Hanna N. Extracellular vesicle-microRNAs as diagnostic biomarkers in preterm neonates. Int J Mol Sci (2023) 24:2622. doi: 10.3390/ijms24032622
66. Ransom MA, Bunn KE, Negretti NM, Jetter CS, Bressman ZJ, Sucre JMS, et al. Developmental trajectory of extracellular vesicle characteristics from the lungs of preterm infants. Am J Physiol Lung Cell Mol Physiol. (2023) 324:L385–92. doi: 10.1152/ajplung.00389.2022
67. Augustine S, Avey MT, Harrison B, Locke T, Ghannad M, Moher D, et al. Mesenchymal stromal cell therapy in bronchopulmonary dysplasia: systematic review and meta-analysis of preclinical studies. Stem Cells Transl Med. (2017) 6:2079–93. doi: 10.1002/sctm.17-0126
68. Haaften Tv, Byrne R, Bonnet S, Rochefort GY, Akabutu J, Bouchentouf M, et al. Airway delivery of mesenchymal stem cells prevents arrested alveolar growth in neonatal lung injury in rats. Am J Respir Crit Care Med. (2009) 180:1131–42. doi: 10.1164/rccm.200902-0179OC
69. Moreira A, Winter C, Joy J, Winter L, Jones M, Noronha M, et al. Intranasal delivery of human umbilical cord Wharton’s jelly mesenchymal stromal cells restores lung alveolarization and vascularization in experimental bronchopulmonary dysplasia. Stem Cells Transl Med. (2020) 9:221–34. doi: 10.1002/sctm.18-0273
70. Abele AN, Taglauer ES, Almeda M, Wilson N, Abikoye A, Seedorf GJ, et al. Antenatal mesenchymal stromal cell extracellular vesicle treatment preserves lung development in a model of bronchopulmonary dysplasia due to chorioamnionitis. Am J Physiol Lung Cell Mol Physiol. (2022) 322:L179–90. doi: 10.1152/ajplung.00329.2021
71. Taglauer ES, Fernandez-Gonzalez A, Willis GR, Reis M, Yeung V, Liu X, et al. Antenatal mesenchymal stromal cell extracellular vesicle therapy prevents preeclamptic lung injury in mice. Am J Respir Cell Mol Biol. (2022) 66:86–95. doi: 10.1165/rcmb.2021-0307OC
72. Willis GR, Reis M, Gheinani AH, Fernandez-Gonzalez A, Taglauer ES, Yeung V, et al. Extracellular vesicles protect the neonatal lung from hyperoxic injury through the epigenetic and transcriptomic reprogramming of myeloid cells. Am J Respir Crit Care Med. (2021) 204:1418–32. doi: 10.1164/rccm.202102-0329OC
73. Chaubey S, Thueson S, Ponnalagu D, Alam MA, Gheorghe CP, Aghai Z, et al. Early gestational mesenchymal stem cell secretome attenuates experimental bronchopulmonary dysplasia in part via exosome-associated factor TSG-6. Stem Cell Res Ther. (2018) 9:173. doi: 10.1186/s13287-018-0903-4
74. Porzionato A, Zaramella P, Dedja A, Guidolin D, Bonadies L, Macchi V, et al. Intratracheal administration of mesenchymal stem cell-derived extracellular vesicles reduces lung injuries in a chronic rat model of bronchopulmonary dysplasia. Am J Physiol Lung Cell Mol Physiol. (2021) 320:L688–L704. doi: 10.1152/ajplung.00148.2020
75. Porzionato A, Zaramella P, Dedja A, Guidolin D, Van Wemmel K, Macchi V, et al. Intratracheal administration of clinical-grade mesenchymal stem cell-derived extracellular vesicles reduces lung injury in a rat model of bronchopulmonary dysplasia. Am J Physiol Lung Cell Mol Physiol. (2019) 316:L6–L19. doi: 10.1152/ajplung.00109.2018
76. Ahn SY, Park WS, Kim YE, Sung DK, Sung SI, Ahn JY, Chang YS. Vascular endothelial growth factor mediates the therapeutic efficacy of mesenchymal stem cell-derived extracellular vesicles against neonatal hyperoxic lung injury. Exp Mol Med. (2018) 50:1–12. doi: 10.1038/s12276-018-0055-8
77. Wang J, Zhang A, Huang F, Xu J, Zhao M. MSC-EXO and tempol ameliorate bronchopulmonary dysplasia in newborn rats by activating HIF-1α. Pediatr Pulmonol. (2023) 58:1367–79. doi: 10.1002/ppul.26317
78. Sharma M, Bellio MA, Benny M, Kulandavelu S, Chen P, Janjindamai C, et al. Mesenchymal stem cell-derived extracellular vesicles prevent experimental bronchopulmonary dysplasia complicated by pulmonary hypertension. Stem Cells Transl Med. (2022) 11:828–40. doi: 10.1093/stcltm/szac041
79. Lithopoulos MA, Strueby L, O'Reilly M, Zhong S, Möbius MA, Eaton F, et al. Pulmonary and neurologic effects of mesenchymal stromal cell extracellular vesicles in a multifactorial lung injury model. Am J Respir Crit Care Med. (2022) 205:1186–201. doi: 10.1164/rccm.202012-4520OC
80. Willis GR, Fernandez-Gonzalez A, Reis M, Yeung V, Liu X, Ericsson M, et al. Mesenchymal stromal cell-derived small extracellular vesicles restore lung architecture and improve exercise capacity in a model of neonatal hyperoxia-induced lung injury. J Extracell Vesicles. (2020) 9:1790874. doi: 10.1080/20013078.2020.1790874
81. Reddy SK, Ballal AR, Shailaja S, Seetharam RN, Raghu CH, Sankhe R, et al. Small extracellular vesicle-loaded bevacizumab reduces the frequency of intravitreal injection required for diabetic retinopathy. Theranostics. (2023) 13:2241–55. doi: 10.7150/thno.78426
82. Susa F, Limongi T, Dumontel B, Vighetto V, Cauda V. Engineered extracellular vesicles as a reliable tool in cancer nanomedicine. Cancers. (2019) 11(12):1979. doi: 10.3390/cancers11121979
83. Clemmens H, Lambert DW. Extracellular vesicles: translational challenges and opportunities. Biochem Soc Trans. (2018) 46:1073–82. doi: 10.1042/BST20180112
84. Shigemoto-Kuroda T, Oh JY, Kim DK, Jeong HJ, Park SY, Lee HJ, et al. MSC-derived extracellular vesicles attenuate immune responses in two autoimmune murine models: type 1 diabetes and uveoretinitis. Stem Cell Rep. (2017) 8:1214–25. doi: 10.1016/j.stemcr.2017.04.008
85. Lai RC, Tan SS, Teh BJ, Sze SK, Arslan F, de Kleijn DP, et al. Proteolytic potential of the MSC exosome proteome: implications for an exosome-mediated delivery of therapeutic proteasome. Int J Proteomics. (2012) 2012:971907. doi: 10.1155/2012/971907
86. Murphy DE, de Jong OG, Brouwer M, Wood MJ, Lavieu G, Schiffelers RM, et al. Extracellular vesicle-based therapeutics: natural versus engineered targeting and trafficking. Exp Mol Med. (2019) 51:1–12. doi: 10.1038/s12276-019-0223-5
87. Herrmann IK, Wood MJA, Fuhrmann G. Extracellular vesicles as a next-generation drug delivery platform. Nat Nanotechnol. (2021) 16:748–59. doi: 10.1038/s41565-021-00931-2
88. Witwer KW, Wolfram J. Extracellular vesicles versus synthetic nanoparticles for drug delivery. Nat Rev Mater. (2021) 6:103–6. doi: 10.1038/s41578-020-00277-6
89. Gangadaran P, Li XJ, Lee HW, Oh JM, Kalimuthu S, Rajendran RL, et al. A new bioluminescent reporter system to study the biodistribution of systematically injected tumor-derived bioluminescent extracellular vesicles in mice. Oncotarget. (2017) 8:109894–914. doi: 10.18632/oncotarget.22493
90. Wiklander OP, Nordin JZ, O'Loughlin A, Gustafsson Y, Corso G, Mager I, et al. Extracellular vesicle in vivo biodistribution is determined by cell source, route of administration and targeting. J Extracell Vesicles. (2015) 4:26316. doi: 10.3402/jev.v4.26316
91. Matsumoto A, Takahashi Y, Chang HY, Wu YW, Yamamoto A, Ishihama Y, et al. Blood concentrations of small extracellular vesicles are determined by a balance between abundant secretion and rapid clearance. J Extracell Vesicles. (2020) 9:1696517. doi: 10.1080/20013078.2019.1696517
92. Lu M, Huang Y. Bioinspired exosome-like therapeutics and delivery nanoplatforms. Biomaterials. (2020) 242:119925. doi: 10.1016/j.biomaterials.2020.119925
93. Man K, Brunet MY, Jones MC, Cox SC. Engineered extracellular vesicles: tailored-made nanomaterials for medical applications. Nanomaterials. (2020) 10(9):1838. doi: 10.3390/nano10091838
94. Stephenson M, Grayson W. Recent advances in bioreactors for cell-based therapies. F1000Res. (2018) 7:F1000. doi: 10.12688/f1000research.12533.1
95. Didiot MC, Hall LM, Coles AH, Haraszti RA, Godinho BM, Chase K, et al. Exosome-mediated delivery of hydrophobically modified siRNA for huntingtin mRNA silencing. Mol Ther. (2016) 24:1836–47. doi: 10.1038/mt.2016.126
96. Fuhrmann G, Serio A, Mazo M, Nair R, Stevens MM. Active loading into extracellular vesicles significantly improves the cellular uptake and photodynamic effect of porphyrins. J Control Release. (2015) 205:35–44. doi: 10.1016/j.jconrel.2014.11.029
97. Villata S, Canta M, Cauda V. EVs and bioengineering: from cellular products to engineered nanomachines. Int J Mol Sci. (2020) 21:6048. doi: 10.3390/ijms21176048
98. Garcia-Manrique P, Gutierrez G, Blanco-Lopez MC. Fully artificial exosomes: towards new theranostic biomaterials. Trends Biotechnol. (2018) 36:10–4. doi: 10.1016/j.tibtech.2017.10.005
Keywords: extracellular vesicle, neonatal lung disease, bronchopulmonary dysplasia, mesenchymal stem cell (MSC), biomarkers
Citation: Wu S, Benny M, Duara J, Williams K, Tan A, Schmidt A and Young KC (2023) Extracellular vesicles: pathogenic messengers and potential therapy for neonatal lung diseases. Front. Pediatr. 11:1205882. doi: 10.3389/fped.2023.1205882
Received: 14 April 2023; Accepted: 31 May 2023;
Published: 16 June 2023.
Edited by:
Chung-Ming Chen, Taipei Medical University, TaiwanReviewed by:
Dinesh Upadhya, Manipal Academy of Higher Education, IndiaGiuseppina Milano, Centre Hospitalier Universitaire Vaudois (CHUV), Switzerland
© 2023 Wu, Benny, Duara, Williams, Tan, Schmidt and Young. This is an open-access article distributed under the terms of the Creative Commons Attribution License (CC BY). The use, distribution or reproduction in other forums is permitted, provided the original author(s) and the copyright owner(s) are credited and that the original publication in this journal is cited, in accordance with accepted academic practice. No use, distribution or reproduction is permitted which does not comply with these terms.
*Correspondence: Karen C. Young a3lvdW5nM0BtZWQubWlhbWkuZWR1