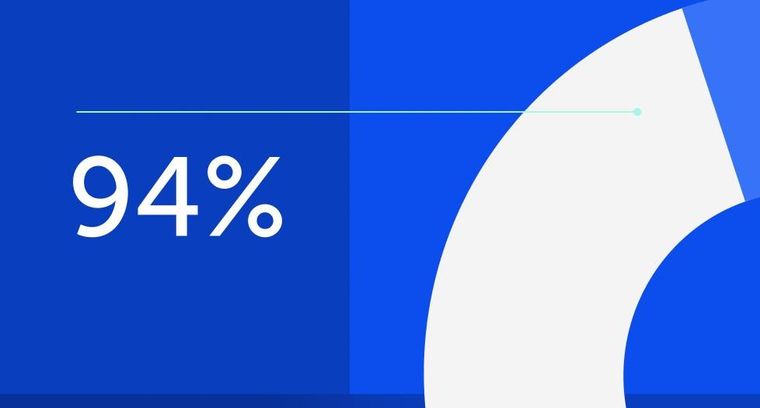
94% of researchers rate our articles as excellent or good
Learn more about the work of our research integrity team to safeguard the quality of each article we publish.
Find out more
REVIEW article
Front. Pediatr., 22 May 2023
Sec. Genetics of Common and Rare Diseases
Volume 11 - 2023 | https://doi.org/10.3389/fped.2023.1126209
Neural tube defects (NTDs) are serious congenital deformities of the nervous system that occur owing to the failure of normal neural tube closures. Genetic and non-genetic factors contribute to the etiology of neural tube defects in humans, indicating the role of gene-gene and gene-environment interaction in the occurrence and recurrence risk of neural tube defects. Several lines of genetic studies on humans and animals demonstrated the role of aberrant genes in the developmental risk of neural tube defects and also provided an understanding of the cellular and morphological programs that occur during embryonic development. Other studies observed the effects of folate and supplementation of folic acid on neural tube defects. Hence, here we review what is known to date regarding altered genes associated with specific signaling pathways resulting in NTDs, as well as highlight the role of various genetic, and non-genetic factors and their interactions that contribute to NTDs. Additionally, we also shine a light on the role of folate and cell adhesion molecules (CAMs) in neural tube defects.
Neural tube defects (NTDs) are the most prevalent serious human birth anomalies of the brain and spine that occur during embryogenesis (by the end of the 6th week of pregnancy). NTDs originate owing to the failure of the neurulation process, which represents the failure of the harmonized morphogenetic process involved in neural tube closure (1, 2). NTDs affect ten infants per 1,000 established pregnancies but this figure varies among different populations (3). The highest prevalence of NTDs has been reported in the Chinese population while the lowest prevalence is in Scandinavian countries (4–7). In India, the incidence of NTDs, especially in the northern part of the country, is approximately 7.8 per 1,000 births (8). NTDs are categorized into two kinds: open and closed NTDs. Open and closed NTDs have affected areas that are either exposed to the body surface or covered with skin, respectively. Anencephaly and spina bifida are the two most prevalent types of open NTDs that arise due to the failure of closure of neural tubes at cranial and spinal regions, respectively (Figure 1). Closed NTDs are classified based on the presence (lipomyelomeningocele, lipomyeloschisis, myelocystocele, and meningocele) or absence (caudal regression, dermal sinus, segmental, and spinal dysgenesis) of a subcutaneous mass (1). Studies based on population and family suggested an intricate etiology for NTDs that involves both genetic and environmental factors (9–13). Genetic mechanisms underlying NTDs are extremely complicated and follow multi-factorial inheritance that is regulated by the interaction of many genes and environmental factors (14). Harmonized gene programs are characteristic features of embryonic development, which is essential for normal neural tube formation. Alteration in harmonized gene programs involved in different signaling pathways (BMP, Wnt, Shh, FGF, TGFβ, etc.) culminates in NTDs (15–19). Recently, accumulating studies performed on genetic models and patients with NTDs revealed that the deregulation of multiple genes associated with signaling pathways, such as WNT, BMP, SHH, and retinoic acid (RA) signaling, culminates in NTDs (20–25). Hence, here we review what is known to date regarding altered genes associated with specific signaling pathways resulting in NTDs, as well as highlight the role of various genetic and non-genetic factors, and their interaction that contribute to NTDs. Additionally, we also shine a light on the role of folate and cell adhesion molecules (CAMs) in neural tube defects.
Figure 1. Diagrammatic representation of closure of the neural tube and the origin point for open neural tube defects.
The formation of the neural tube is a multistep, zipper-like, and discontinuous process regulated by multiple genes and is affected by environmental factors of the host. It involves gene-nutrients, gene-environment, and gene-gene interactions. Animal and clinical studies in the last five decades have recognized the etiology of neural tube defects, which comprise genetic, epigenetic, environmental, and nutritional factors (Table 1) (26–28). It is well-reported that genetic factors are responsible for 70% of the variance of neural tube defects (29).
(i) Non-genetic factors: Non-genetic factors indirectly affect the process of neural tube formation by modulating the gene functions that are discussed as follows:
a) Nutritional factors: The majority of congenital birth deformities occur especially in families with lower socioeconomic statuses, which leads to the evaluation of the involvement of nutritional factors in neural tube defects. It is well-reported that maternal nutrition plays a crucial role during the normal growth and development of the fetus. It can also affect the capacity of fertilization and the quality of gametes. An abundance of studies showed that polymorphism in folate metabolizing genes (MTRR and MTHFR) is associated with increased chances of non-disjunction (30–32). A low level of B-vitamin folate was noticed in fetuses with neural tube defects (33), inducing a clinical trial of folic acid supplementation to reduce the population burden of neural tube defects. A multi-centric randomized controlled trial showed that supplementation of folic acid mitigates (4mg/day) the occurrence of neural tube defects (34). Several other clinical trials confirmed the reduction of neural tube defects after the uptake of folic acid (35–37). Numerous previous studies suggested that the supplementation of folic acid reduced the occurrence of neural tube defects by 50%–70% (37, 38). Sub-optimal levels of folate may trigger neural tube defects in individuals who carry genetic mutations in the pax3 gene (39). Numerous experimental and clinical studies showed that alteration in purine and thymidylate biosynthesis is linked with the development of neural tube defects (40–42). A study conducted on curly tail mice showed that myo-inositol prevents cranial and spinal neural tube defects (43). Clinical studies reported no recurrence of neural tube defects in neonates with a combination of inositol and folic acid (44, 45). Previous studies demonstrated the relationship between Zn and neural tube defects (46, 47). The status of folate may also affect the gene expression associated with neurodevelopment as they influence histone modification and DNA methylation (48). Folate of maternal plasma affects the differential methylation of DNA in the newborn, leading to alterations of gene expression that eventually culminate in neural tube defects (49). Clinical data demonstrated that GNAS imprinting plays a crucial role in the regulation of folic acid metabolism during embryogenesis and that alteration in GNAS imprinting clusters leads to neural tube defects (50). Folate deficiency promotes the monoubiquitination of H2A histone, resulting in decreased expression of genes (Gata4, Cdx2, Pax6, and Nes) associated with neural tube closures in the embryonic stem cells of mice (51). However, the exact mechanisms underlying folate-deficiency-induced neural tube defects are still not known.
b) Hyperthermia: Elevated body temperature (>40°C) during the pregnancy is nominated as maternal hyperthermia and may happen because of fever, hot water baths, and the usage of saunas and hot tubs, causing developmental deformities (52, 53). In vivo and in vitro studies on different animal models showed that the neural tube is highly sensitive to elevated temperature (32). Hyperthermia influences multiple developmental processes such as cell differentiation, migration, apoptosis, and proliferation (32, 54). The impacts of heat stress on embryogenesis depend on the duration and dose of the heat exposure, strain, species, and stage of embryonic development (55, 56). Clinical case and animal studies reported the teratogenic and mutagenic effects of hyperthermia (56–60). Significant upregulation of expression of Cx43 mRNA (connexin 43) was observed in neural tubes, especially in heat-treated groups in contrast to the control, indicating a relationship between upregulated Cx43 mRNA and neural tube defects (53). Exposure to the influenza virus during the first trimester induces the risk of the development of neural tube defects (58). Nine case report studies clearly showed a clear relationship between maternal exposure to hyperthermia and elevated risk of neural tube defects (57). A study performed in California, United States, also observed similar effects of febrile illness and maternal fever on neural tube cases (61). A cohort study conducted on 23,491 women reported the association between maternal exposure to hyperthermia through various sources (hot water baths, hot tubs, fever, and sauna) and the risk of the development of neural tube defects (62). A combination of different sources of hyperthermia increased the risk of neural tube defects (59). A comparative study conducted on the population of the Texas-Mexico border showed that maternal exposure to hyperthermia during the first trimester enhanced the relative risk of development of neural tube defects by 3.6% (63). However, recent studies on pregnant women do not observe any fetal abnormalities after COVID-19 vaccination (64–68).
c) Pesticides: The population explosion increased the demand for the development of novel approaches to enhancing agricultural production to fulfill the increased demand, and these new methods were highly dependent on the utilization of pesticides. Continued and injudicious use of pesticides increased its residues in fruits, vegetables, cattle milk, cattle meat, food, and water, enhancing the risk of exposure to pregnant women. Several studies demonstrated the negative medical side effects caused by an enhanced and indiscriminate use of pesticides (69, 70). Several lines of evidence reported that pesticides contribute to significant developmental and reproductive disorders with carcinogenic and teratogenic capabilities (69, 71, 72). Several previous experimental and case reports indicated a connection between congenital disorders and pesticide exposure (69–71, 73, 74). A study performed in Washington, USA, showed the increased risk of development of neural tube defects post pesticide exposure (75). A case-control study demonstrated that pesticide exposure induced the developmental risk of neural tube defects (76). Another study performed on a case group found that there were 2 times greater chances of neural tube defects affecting pregnancies in individuals who were living 0.25 miles from agricultural fields or using pesticides at home (70). A population-based case-control study showed a marginal or zero developmental risk of neural tube defects post-pesticide exposure (63). Another study proved that other confounding factors (folate deficiency and low level of vitamin B12) may increase the developmental risk of neural tube defects on pesticide exposure. A systematic review showed that, due to the heavy usage of pesticides, the occurrence of neural tube defects in neonates is more prominent in developing countries such as those in the African continent (72). A case report study based on a questionnaire as directed by the WHO found an increased incidence of neural tube defects post-maternal exposure to pesticides (71). A study conducted on agriculture workers observed a higher incidence of congenital deformities in neonates (77).
d) Arsenic (As): Globally, the level of arsenic has increased due to metalworking industries, the combustion of coal, and the production of pesticides, resulting in contamination of inorganic arsenic in air, water, and soil. Approximately 95% of Arsenic absorption among Europeans is due to the consumption of arsenic-contaminated foods (48). Several lines of evidence reported the teratogenic and toxic properties of arsenic and found that it is an utmost risk for the development of neural tube defects (78–80). Numerous past studies reported that arsenic disrupts the placental structures, resulting in the disruption of the transport of nutrients and molecules (79–82). Animal and human studies showed that Arsenic induces neural tube defects because it triggers epigenetic alteration and gene mutation (80, 81). A case-control study based on GWAS recognized the 14 single nucleotide polymorphisms (SNP) expressed in neural tube defects pregnancies post arsenic toxicity (80). DNA methylation is a crucial process during the developmental period and is influenced by arsenic poisoning. Studies based on arsenic poisoning showed that it inhibits DNA methylation by reducing the activity of DNA methylase (1 and 3b) and S-adenosyl methionine (SAM) (48, 83, 84). Folate interacts with arsenic resulting in a reduction of arsenic in blood as well as an extensive efflux of folate (79). A case-control study conducted in Bangladesh showed that high efflux of folate owing to the interaction with arsenic increases the risk of neural tube defects (80). A case study that included 49 mothers and their neonates showed a clear relationship between arsenic levels in the environment and the placenta (82). The research also reported increased levels of lipid peroxidase and reduced glutathione in the blood and placenta, leading to increased oxidative damage. The states of Assam and Uttar Pradesh, in India, were dependent on rice and consumption of groundwater and developed arsenic belts between the regions, as is indicated by the high incidence of neural tube defects in these regions (78).
e) Polyaromatic hydrocarbons (PAHs): PAHs are environmental pollutants that arise through anthropogenic activities particularly owing to the incomplete combustion of wood, oil, coal, and petrol (85). PAHs have several medical side effects including enhanced risk of neural tube defects. A study conducted in the rural population of Shanxi province, China found that women with coal exposure (IAPCC) had a 60% enhanced risk of having newborns with neural tube defects in contrast to women without IAPCC exposure (86). Maternal occupational exposure to PAHs was found to enhance the risk of neonates with spina bifida amongst women with underweight or normal weight (87). Higher concentration of PAHs has been reported in the placenta in cases of neural tube defects (88). A woman with an elevated concentration in the serum was found to be associated with a high risk of neural tube defects in neonates (89). However, the molecular mechanisms underlying PAHs-induced neural tube defects are not well known. On the contrary, a recent study by Huang et al. (90), showed that reduced global DNA hypo-methylation could be one of the possible mechanisms underlying the increased risk of neural tube defects induced by PAHs.
f) Antibiotics: Antibiotics are employed to treat bacterial infections, such as acute cystitis and bacteriuria, experienced by pregnant women. Past studies revealed that antibiotics cause functional and physical deformities in the fetus or human embryo (91, 92). A study found that antibiotics prescribed for the management of urinary tract infections (UTIs) were linked with neural tube defects in neonates (93). Epidemiological reports observed that the trimethoprim drug increased the risk of both childbirth deformities and miscarriage (93–95). A population-based case-control study noted the association between the use of antibiotics during the first trimester and birth deformities in neonates (91). One study discovered the association between nitrofurantoin exposure during the first trimester and enhanced risk of birth defects in neonates (96). A population-based cohort study showed that gestational exposure to nitrofurantoin is marginally linked with developmental malformations (97). Antibiotics such as non-steroid anti-inflammatory drugs (NSAID), paracetamol, and opioids are prevalent drugs employed for the management of pain. Concurrent usage of opioid drugs and NSAIDs for the management of pain was found to be associated with a higher incidence of spina bifida in contrast to singular drug-mediated pain medication (98). Some of the studies also found similar outcomes with the usage of opioid drugs (99, 100). A study conducted in the USA reported the connection between the usage of anti-epileptic drugs and the incidence of cleft palate and spina bifida (101).
g) Trace Elements—Neural Tube Defects: Trace elements are chemical compounds in organisms that are required in minuscule amounts for physiological functions. Trace elements are divided into two groups: Essential trace elements (ETEs) and Non-essential trace elements. ETEs include Zn, Mn, Co, Mo, Fe, and Se; these trace elements play a key role in fetal and maternal health during pregnancy (102–104). Studies showed that ETEs are involved in cell function and differentiation, suggesting that ETEs play a key role in multiple physiological and cellular functions. Therefore, an alteration in the homeostasis of ETEs during pregnancy may lead to birth defects (105). Insufficient dietary intake of Fe is linked with a higher risk of spina bifida (106). Higher concentrations of Mn in maternal blood during pregnancy significantly increase the risk of NTDs (107). Studies have demonstrated that low selenium levels in maternal plasma and serum are associated with an enhanced risk of NTDs (108, 109). Several lines of evidence have demonstrated that lower concentrations of Zn in maternal serum and scalp hair are linked with increased risk for NTDs in offspring (110–112). However, some studies found that a higher concentration of Zn in maternal hair during the peri-conceptional period and nails during the third trimester is linked with elevated risk for NTDs (113, 114). Previous studies also observed the association between the level of Mo and Co and enhanced risk of NTDs in offspring (115, 116). Alkaline earth metals such as Ba, Th, and Cs also cause neural tube defects in children (117–119). Maternal exposure to Ba during the embryonic period leads to the development of NTDs in offspring (117). A case-control study has demonstrated the association between NTDs and Th levels (118). Another study led by Pi et al. (119), observed the association between Cs level and increased risk of NTDs (119).
(ii) Genetic factors: Neural tube defects are multi-factorial in origin (2, 120). Epidemiological evidence on humans showed that the genetic basis for neural tube defects is acquired from the positive concordance of neural tube defects from monozygotic twins in contrast to di-zygotic twins (121, 122). In mice, more than 400 genes are involved in the closure of the neural tube (123, 124), and approximately 191 NTD candidate genes are found in NTD fetuses (125). Although defects in neural tube closure occur more familiarly after one neural tube defect-affected pregnancy (recurrence rate is 1 in 20), the recurrence rate of neural tube defects does not exceed 10% even after two neural tube defect-affected pregnancies. These recurrence risks strongly indicate the involvement of multiple genes in neural tube defects. The risk of recurrence and pattern of inheritance of neural tube defects in the multiplex families do not follow the Mendelian law of inheritance (126). Some studies showed that both sex-influenced and maternal genetic factors contribute to the developmental risk of neural tube defects (127, 128). The estimated heritability rate in neural tube defects is approximately 60%, especially when multiple susceptible genes are involved (12). Animal models are very crucial in understanding the role of candidate genes in the development of neural tube defects because the process of neurulation is very similar in humans and mice. Several gene ablations that were responsible for neural tube defects in mice models echoed the few cases of neural tube defects observed in humans, such as Pax3 (paired box-3 protein) and Lrp6 (low-density lipoprotein receptor-related protein-6) (129, 130). Apart from animal models, next-generation sequencing (NGS) shines a new light on underlying molecular insight of genetic risk factors for neural tube defects that includes whole exome sequencing (WES), target panel sequencing (TPS), and whole genome sequencing (WGS). One research recognized the homozygous missense genetic ablation in the TRIM36 gene by using WGS, which is responsible for autosomal recessive anencephaly, particularly in Indian families (131). Another study identified the de novo damaging variants of anencephaly through WES (132). Ishida et al. (133), identified the 397 damaging variants of anencephaly cases through TPS, in which 21 variants out of the 397 had not been previously reported. A recent study used WGS to reveal the genetic mutation in non-coding regions that contributes to neural tube defects (134). Several studies have demonstrated the association between mutations in epigenetic regulators and enhanced risk of NTDs (28, 120, 135).
(iii) Epigenetic Factors: An epigenetic mechanism of gene regulation makes stable phenotypic changes without any change in the nucleotide sequence of DNA. Epigenetic regulators play a pivotal role in global gene regulation. Several studies have demonstrated the association between mutations in epigenetic regulators and enhanced risk of NTDs (28, 120, 135). Alterations in DNA methylation, chromatin remodeling, and histone modification may lead to an increased risk of NTDs (28, 136). It has been shown that DNA methylase 3A (DNMT3A) and DNMT3B are responsible for demethylating and remethylating the majority of the embryonic genome except for the imprinting region, while DNMT1 maintains the methylation pattern (137). Mice deficient in DNMT3A and DNMT3B had an increased risk of NTDs, indicating that appropriate remethylation is essential prior to implantation (138). Extensive studies have demonstrated the association of folate one-carbon metabolism with an elevated risk of NTDs owing to diminished methylation (139, 140). A study conducted on splotch embryos showed that enhanced methylation of H3K27 in neural crest cells leads to an increased risk of NTDs (141). Knockout mice of p300 (histone acetyltransferase enzyme) exhibited cranial NTDs, suggesting that it is essential for the closure of the neural tube (142). Studies have found that mutations in Gcn5 and Cited2 disrupt HAT activity and elevate the risk of NTDs (143, 144). Pharmacological inhibitors such as valproic acid and trichostatin-A demolish the regulation of acetylation that causes NTDs (145, 146). Mutations in histone deacetylase (hdac4 and sirt1) cause cranial NTDs (147, 148). Mutations in several chromatin remodeling enzymes are associated with NTDs (121, 149). Several studies showed that mutation in SMARCC1, CERCR2, BRD2, and SMARCA4 is linked with an enhanced risk of NTDs (150–153).
Neurulation occurs in two phases in mice and humans (primary and secondary) from embryonic day 8.5 to 10.5 (day 22–23 and 26–30 of gestation in humans) (154). The neural tube is an embryonic precursor that develops later into the spinal cord and brain through fine-tuned coordination of multiple signaling pathways, including planar cell polarity (PCP) signaling, sonic hedgehog (Shh) signaling, bone morphogenetic protein (BMP) signaling, inositol metabolism, retinoid signaling, canonical Wnt signaling, fibroblast growth factor (FGF) signaling, tumor growth factor (TGF-β) signaling, Notch signaling, receptor tyrosine kinase-like orphan receptor (ROR) signaling, and folate-methionine metabolic signaling pathway, during the time window that is required for closure of the neural tube (155). Genes associated with these signaling pathways are involved in epigenetic modifications (acetylation and methylation), organization of chromatin, regulation of the cell cycle, and actin cytoskeleton (156). The perturbation in genes and cross-talk between signaling pathways leads to the pathogenesis of neural tube defects (120) (Table 2), which are discussed as follows:
(i) Planar cell polarity (PCP) signaling pathway: PCP signaling is required for the closure of the boundary between the cervical and hindbrain; hence, it is nominated as a planar signaling pathway owing to its involvement in the coordinated polarized orientation of cells. Planar cell polarity was originally described in a Drosophila model as a signaling cascade that mediates its action without the requirement of β-catenin; so-called as a non-canonical Wnt signaling pathway and required for specification of plane polarity in epithelia, including compound eye and wing (156). PCP signaling pathway is highly conserved in vertebrates and involved in various developmental processes such as cellular and tissue polarity during morphogenesis and harmonized orientation of hair cells of the inner ear (156–159). Positioning cloning of Vangl2 in loop-tailed mutant mice that exhibited severe forms of neural tube defects (craniorachischisis) was the first evidence that shed a light on the role of PCP signaling pathway in the pathogenesis of neural tube defects (160, 161). Experimental studies showed that a double mutant of Fzd (Frizzled)-3 and -6 Dvl (disheveled)-1 and -2 protein contributes to the pathogenesis of craniorachischisis (162, 163). Several lines of experimental studies linked the other PCP-related genes (Srb1 and Ptk7) with the development of severe neural tube defects (161, 164). Genetic ablation in Sec24b contributes to the pathogenesis of neural tube defects (165). They also reported that the mutant form of Sec24b significantly enhances the prevalence of spina bifida by interacting with the LoF (loss of function) Vangl2 allele. Mutational studies showed that mutation of Sec24b, Ptk7, or Sdc4 contributes to craniorachischisis in combination with a heterozygous allele of Vangl2Lp/+ (161, 164, 166). Combination of Vangl2Lp/+ with genes (Fzd2+/−, Fzd1+/−, and Dvl3+/−) of the Wnt signaling pathway contributes to the risk of exencephaly (167, 168). Some other studies showed that PCP effector genes (Fuz or Intu) are also responsible for exencephaly (169–171). A mutational study on mice showed that genetic ablation in Smurf1/2 leads to PCP-related neural defects (172). The digenic combination of double knockout Vangl2 with Cthrc1 or cordonbleuC101 contributes to exencephaly (173, 174). Experimental mutational studies on mice demonstrated that Ptk7 (PCP genes) with Grh13 (non-PCP genes) develops spina bifida (164, 175) while with Cthrc1 develops exencephaly (175). A mutational study performed on mice models demonstrated the role of Celsr1in the pathogenesis of neural tube defects (176). A genetic study performed on a circle-tail mouse found that dysfunction of the Scrb1 (Scribb) gene contributes to neural tube defects (177). The candidate genes identified in the animal model provide the rationale for the recognition of orthologous genes involved in human neural tube defects. The Orthologue of Vangl2 was the first human gene of PCP signaling implicated in neural tube defects. A study conducted with Italian patients analyzed the role of Vangl2 and its paralogue Vangl (178) and reported on the three variants of Vangl: p.Val239Ile and p.Arg274Gln were involved in familial neural tube defects while p.Met328Thr was involved in sporadic cases of the disease. The p.Val239Ile mutation inhibited the interaction between Dvl proteins and Vangl1. Several clinical studies demonstrated the role of the Vangl1 gene in human neural tube defects (16, 179–183). Embryo with double heterozygous mutation of Vangl2Lp and Ptk7XST87 exhibited the development of spina bifida (184). Genetic studies also implicated the role of various genes (CELSR1–3, PRICKLE1, FZD6, LRP6, and SCRIB) in human neural tube defects (185–190). A missense mutation in ANKRD6 alters the reciprocal antagonism mechanisms between both Wnt signaling pathways involved in neurulation, resulting in NTDs (187). LRP6 is another candidate gene that encodes DIVESIN and functions as an antagonist on both Wnt signaling pathways (188). Genetic ablation of LRP6 leads to spina bifida (129). In another study, mutations in WDR34 impaired the PCP signaling pathway, increasing the risk of NTDs (191).
(ii) Canonical Wnt signaling pathway: Wnt/β-catenin signaling pathway is involved in anterior-posterior patterning during embryonic development and any perturbation in this process culminates in neural defects. Wnt signaling is also involved in the activation of the PCP signaling pathway through stimulation of Rho-dependent kinase (192). Altered expression of the Wnt signaling pathway leads to impairment in anterior-posterior patterning, resulting in NTDs (193). Genetic alteration in β-catenin with Pax3 contributes to spinal neural tube defects (194). Recently, one study conducted on a mouse model suggested that abnormal expression of Gcm1protein linked with the Wnt signaling pathway leads to neural tube defects (192). Habert et al. (195) observed the burden of deleterious SNPs associated with canonical Wnt signaling genes in patients with myelomeningocele. Several experimental studies reported the molecular switches, such as Ptk7 and Lrp6, that regulated the involvement of the Wnt signaling pathway (canonical and non-canonical) in the closure of neural tube defects (21, 196, 197). Some studies showed that Ptk7 mutation abrogates the targets of the canonical Wnt signaling pathway, resulting in failure of neural tube closure (198, 199). Another study conducted on animal models showed that Ptk7 and Lrp6 alter the activity of the canonical signaling pathway, resulting in neural tube defects (199). Exome sequencing analysis showed that mutations in ten Wnt genes are prominent among Mexican-American patients with myelomeningocele (21).
(iii) Sonic hedgehog (Shh) signaling pathway: Shh signaling pathway plays a crucial role in patterning, growth, and morphogenesis during embryonic development. It regulates the patterning of the ventral neural tube and its extension into the brain regions (200). Several lines of studies showed that genetic ablation in Ptc1 (patched) contributes to the failure of neural tube closure (201, 202). Negative mutation in Shh signaling inhibitory genes gives rise to neural tube defects (201–203). Some of the studies suggested that the overexpression of Smo and Shh proteins of Shh signaling may lead to the failure of neural tube closure (201, 202). Studies based on a knockout mouse model showed that Fkbp8 (FK506 binding protein-8) mutation leads to the development of spina bifida (204, 205). Mutation in many other genes of the Shh signaling pathway contributes to exencephaly (206–218). Some studies also implicated the mutation in the genes (Ptch1, Rab23, and Tulp3) of the Shh signaling pathway in the development of spina bifida and CRN (209–212, 217, 218). Another study showed that mutation in protein required for the function of cilia leads to impaired Shh signaling pathway, culminating with neural tube defects (17). Accumulating evidence on humans also showed that genetic ablation in the Shh signaling gene leads to the development of neural tube defects (219–221). Genetic ablation of the WDR34 gene impaired the Shh signaling pathway resulting in exencephaly (191).
(iv) BMP (bone morphogenetic protein) signaling pathway: BMPs are members of the TGF-β superfamily that acts as a morphogen, involved in the development, patterning, and function of the nervous system. It is needed for the development of dorsal neural tubes, especially for stimulation of dorsal neurons and neural crest cells (NCC) prior to neurulation. Animal and human studies showed that knockout mice with BMP4 and NOG (noggin) lead to neural tube defects (15, 19, 222–225). Evaluation of BMP4 and NOG showed that the genetic alteration in both genes resulted in neural tube defects in humans (222). Evidence from the knockout mouse model showed that mutation in Noggin culminates in exencephaly and spina bifida (15). Studies on genetic mouse models observed that BMP2 mutation culminates in premature as well as exaggerated bending of caudal neuropore and various cranial deformities (225, 226). Genetic studies performed on mouse models showed that double knockout of Bmpr1A and Bmpr1B leads to the development of holoprosencephaly (227–229). Genetic analysis based on the double mutant of Bmpr1A and Bmpr1B showed the existence of two kinds of holoprosencephaly (227). Embryo with Zic2 mutation leads to the development of spina bifida owing to the absence of DLHP required for closure of the neural tube in the lower region spinal cord (226).
(v) Retinoid signaling pathway: Retinoic acid, a derivative of vitamin-A, is crucial for the patterning of the spinal cord and hindbrain (229). An imbalance in the level of vitamin-A and retinoic acid has been implicated in birth defects including neural tube defects (230–232). Negative mutation in Raldh2 (key enzymes involved in retinoic acid synthesis), Cyp26a1 (key metabolizing enzyme), and retinoic receptors α and γ contributes to neural tube defects (233–235). A case-control study identified the association of variants of Raldh1A2, Cyp26A1, and CRABP1retinoic genes and neural tube defects in humans (230). Experimental studies on mouse models have shown that overexpression of retinoic acid leads to neural tube defects (236, 237). A recent study found that treatment of neural crest cells (NSCs) with all-trans-retinoic acid culminates in neural tube deformities (238).
(vi) Notch signaling pathway: The notch signaling pathway regulates the proliferation and differentiation of NSCs (neural crest cells) during embryonic development. These NSCs are required for the normal closure of the neural tube (2) and dysregulation of proliferation, migration, and differentiation of NSCs leads to brain anomalies (239, 240). Previous Studies observed that mutation in the genes Hes1, Hes3, and RBP-Jκ of the Notch signaling pathway contributes to neural tube anomalies (121, 149). A recent study observed that abnormal expression of N1 (Notch1) enforces the occurrence of neural tube deformities (238). A study conducted on embryonic stem cells showed that the double mutant embryo of CSL (CBF-1/Suppressor of hairless/Lag-1) displays the phenotypes of neural tube defects (241). Overexpression of Notch3 in the nervous system of mice has been implicated in exencephaly (242).
Folate is a water-soluble vitamin B that plays a crucial role in nucleotide synthesis and methylation pathway required for cellular proliferation and differentiation during embryonic development (Table 3) (19, 27). Several lines of evidence showed that genetic ablation in FOLR1, which encodes the protein required for folate transport, culminates in neural tube defects (243–245). However, mutations in FOLR2 and RFC (trans-membrane receptor) did not cause any congenital abnormalities (244, 246). A study led by Barber et al. (247), showed that the development of neural tubes will be delayed if an ample amount of nucleotide is not available in neuroepithelial cells, indicating the crucial role of folate during embryonic development. Experimental research conducted by Flemming et al. (248), on splotch mouse models supports this hypothesis. The authors concluded that mutation in the Pax3 gene leads to neural tube defects due to a deficiency of dTMP synthesis. Many studies on the splotch mouse model showed that supplementation with folic acid (FA) or thymidine ameliorates neural abnormalities (41, 248). Embryos with a null mutation in the SHMT1gene display an exencephaly similar to the one caused by maternal folate dietary deficiency (249, 250). Impairment in de novo synthesis of purine has been reported in homozygous knockout mice for the MTHFD1gene resulting in neural tube defects (40). However, this observation has not been reported in heterozygous mice for the MTHFD1 gene. A mouse model with a null mutation in the Cited2 gene exhibited exencephaly while this effect was reverted by FA supplementation (251). Previous reports have demonstrated that the proper functioning of methylation cycles is required for the normal closure of neural tubes (252, 253). A delay in the normal closure of neural tubes has been observed in chick embryos when the methylation cycle is inhibited by using inhibitors (254). Studies performed on mice showed that the Axd and Amt mutation contributes to the unresponsiveness to FA supplementation (255, 257). Several lines of experimental studies found that the perturbation in the methylation process owing to folate deficiency leads to a reduction in the normal closure of neural tubes (254, 257, 258). Exposure of an embryo to cycloleucine, an inhibitor of methylation, or Adox (oxidized adenosine), an inhibitor of S-adenosylhomocysteine hydrolase, leads to a delay in the neurulation process (257, 258). Previous reports showed that culturing the mouse embryo with a low concentration of methionine displays the phenotype of neural tube defects (259, 260). A study performed by Bjorklund et al. (261), hypothesized that the post-translation modification of cytoskeleton proteins might be involved in the abnormal closure of neural tubes. One of the studies showed that abnormal modification of actin protein leads to neural tube defects (259).
Table 3. Genes of folate-mediated pathway and cell adhesion molecules (CAM) linked with developmental risk of neural tube defects.
CAMs are groups of proteins found at the surface of a cell and are involved in the adhesion of the cell to cell or extracellular matrix; thus acting as a so-called molecular glue. They play a critical role in contact inhibition, cellular growth, and programmed cell death in fully developed animals (262). Apart from this, they also play an essential role in neurulation, cell-cell interaction, axon guidance, and cell migration during neural development (263). Experimental studies on humans and animals showed that mutation in genes associated with CAMs leads to neural tube defects (Table 3) (264–267). A study led by Deak et al. (265) observed the association between SNPs in the NCAM1 (neural-CAM-1) and neural tube defects. Fat1 is a cadherin molecule that is involved in the organization of the cytoskeleton at cell boundaries especially actin polymerization (267). Mutation in the gene of Fat1displays exencephaly, while Fat2 mutation did not cause exencephaly, however, a null mutation in both Fat1 and Fat2 enhanced the frequency of exencephaly in contrast to Fat1 alone (268). Existing literature showed that the mutation in integrins-α3/α6, perlecan, and laminin-α5 genes gives rise to neural tube defects (269–271). A lack of the ephrinA5 or EphA7 gene in mice led to neural tube defects (272–274). Another study reported that the null mutation in EphrinB1 displayed a higher incidence of exencephaly in heterozygous females in contrast to heterozygous males (275).
Neural tube defects are serious birth defects of the nervous system that occur because of an abnormal closure of the neural tube during embryonic development. Several lines of studies explored the mutated genes responsible for neural tube defects in humans and animals. However, the exact molecular mechanisms underlying neural tube defects are still not known. Advances in whole genome and exome sequencing in the near future may pierce our knowledge of the interactions between teratogens and their effects on the normal closure of neural tubes, leading to the understanding of the molecular mechanisms underlying neural tube defects.
SR conceptualized the subject, reviewed the literature, and wrote the draft manuscript. LL assisted in the manuscript preparation. SR and AS initiated the topic, designed the figures, and finalized the manuscript. SS and JK contributed in the revision, editing and proofreading of the final manuscript. All authors contributed to the article and approved the submitted version.
The authors declare that the research was conducted in the absence of any commercial or financial relationships that could be construed as a potential conflict of interest.
All claims expressed in this article are solely those of the authors and do not necessarily represent those of their affiliated organizations, or those of the publisher, the editors and the reviewers. Any product that may be evaluated in this article, or claim that may be made by its manufacturer, is not guaranteed or endorsed by the publisher.
1. Rossi A, Biancheri R, Cama A, Piatelli G, Ravegnani M, Tortori-Donati P. Imaging in spine and spinal cord malformations. Eur J Radiol. (2004) 50(2):177–200. doi: 10.1016/j.ejrad.2003.10.015
2. Copp AJ, Stanier P, Greene ND. Neural tube defects: recent advances, unsolved questions, and controversies. Lancet Neurol. (2013) 12(8):799–810. doi: 10.1016/S1474-4422(13)70110-8
3. Seidahmed MZ, Abdelbasit OB, Shaheed MM, Alhussein KA, Miqdad AM, Khalil MI, et al. Epidemiology of neural tube defects. Saudi Med J. (2014) 35(Suppl 1):S29. PMID: 25551106
4. Li Z, Ren A, Zhang L, Ye R, Li S, Zheng J, et al. Extremely high prevalence of neural tube defects in a 4-county area in Shanxi Province, China. Birth Defects Res A Clin Mol Teratol. (2006) 76(4):237–40. doi: 10.1002/bdra.20248
5. Li Z, Ren A, Zhang L, Guo Z, Li Z. A population-based case–control study of risk factors for neural tube defects in four high-prevalence areas of Shanxi province, China. Paediatr Perinat Epidemiol. (2006) 20(1):43–53. doi: 10.1111/j.1365-3016.2006.00694.x
6. Jacobsen P. Regional variations in neural tube defects and alfa-fetoprotein screening in Denmark 1983-88. Acta Obstet Gynecol Scand. (1996) 75(7):620–3. doi: 10.3109/00016349609054685
7. De Wals P, Rusen ID, Lee NS, Morin P, Niyonsenga T. Trend in prevalence of neural tube defects in Quebec. Birth Defects Res A Clin Mol Teratol. (2003) 67(11):919–23. doi: 10.1002/bdra.10124
8. Rai SK, Singh R, Pandey S, Singh K, Shinde N, Rai S, et al. High incidence of neural tube defects in Northern part of India. Asian J Neurosurg. (2016) 11(4):352. doi: 10.4103/1793-5482.175628
9. Frey L, Hauser WA. Epidemiology of neural tube defects. Epilepsia. (2003) 44:4–13. doi: 10.1046/j.1528-1157.44.s3.2.x
10. Mitchell LE. Epidemiology of neural tube defects. Am J Med Genet C Semin Med Genet. (2005) 35(1):88–94. doi: 10.1002/ajmg.c.30057
11. Lynch SA. Non-multifactorial neural tube defects. Am J Med Genet C Semin Med Genet. (2005) 135(1):69–76. doi: 10.1002/ajmg.c.30055
12. Manning SM, Jennings R, Madsen JR. Pathophysiology, prevention, and potential treatment of neural tube defects. Ment Retard Dev Disabil Res Rev. (2000) 6(1):6–14. doi: 10.1002/(SICI)1098-2779(2000)6:1%3C6::AID-MRDD2%3E3.0.CO;2-B
13. Gelineau-Van Waes J, Finnell RH. Importance of model organisms in understanding the biology and genetic basis of human nonsyndromic neural tube defects. Teratology. (2001) 64(4):177–80. doi: 10.1002/tera.1062
14. Phadke S, Agarwal M. Neural tube defects: a need for population-based prevention program. Indian J Hum Genet. (2012) 18(2):145. doi: 10.4103/0971-6866.100747
15. Stottmann RW, Berrong M, Matta K, Choi M, Klingensmith J. The BMP antagonist Noggin promotes cranial and spinal neurulation by distinct mechanisms. Dev Biol. (2006) 295(2):647–63. doi: 10.1016/j.ydbio.2006.03.051
16. Chen Z, Lei Y, Cao X, Zheng Y, Wang F, Bao Y, et al. Genetic analysis of Wnt/PCP genes in neural tube defects. BMC Med Genomics. (2018) 11(1):1–9. doi: 10.1186/s12920-018-0355-9
17. Murdoch JN, Copp AJ. The relationship between sonic Hedgehog signaling, cilia, and neural tube defects. Birth Defects Res A Clin Mol Teratol. (2010) 88(8):633–52. doi: 10.1002/bdra.20686
18. Diez del Corral R, Morales AV. The multiple roles of FGF signaling in the developing spinal cord. Front Cell Dev Biol. (2017) 5:58. doi: 10.3389/fcell.2017.00058
19. Meyers EA, Kessler JA. TGF-β family signaling in neural and neuronal differentiation, development, and function. Cold Spring Harbor Perspect Biol. (2017) 9(8):a022244. doi: 10.1101/cshperspect.a022244
20. Lee S, Gleeson JG. Closing in on mechanisms of open neural tube defects. Trends Neurosci. (2020) 43(7):519–32. doi: 10.1016/j.tins.2020.04.009
21. Hebert L, Hillman P, Baker C, Brown M, Ashley-Koch A, Hixson JE, et al. Burden of rare deleterious variants in WNT signaling genes among 511 myelomeningocele patients. PLoS One. (2020) 15(9):e0239083. doi: 10.1371/journal.pone.0239083
22. Kautto EA, Schieffer KM, McGrath S, Miller AR, Hernandez-Gonzalez ME, Choi S, et al. Expanding the clinical phenotype of FGFR1 internal tandem duplication. Mol Case Stud. (2022) 8(2):a006174. doi: 10.1101/mcs.a006174
23. Brooks ER, Islam MT, Anderson KV, Zallen JA. Sonic hedgehog signaling directs patterned cell remodeling during cranial neural tube closure. Elife. (2020) 9:e60234. doi: 10.7554/eLife.60234
24. Gonzalez-Gobartt E, Blanco-Ameijeiras J, Usieto S, Allio G, Benazeraf B, Martí E. Cell intercalation driven by SMAD3 underlies secondary neural tube formation. Dev Cell. (2021) 56(8):1147–63. doi: 10.1016/j.devcel.2021.03.023
25. Zou J, Wang F, Yang X, Wang H, Niswander L, Zhang T, et al. Association between rare variants in specific functional pathways and human neural tube defects multiple subphenotypes. Neural Dev. (2020) 15(1):1–5. doi: 10.1186/s13064-020-0138-9
26. Kondo A, Matsuo T, Morota N, Kondo AS, Okai I, Fukuda H. Neural tube defects: risk factors and preventive measures. Congenital Anom. (2017) 57(5):150–6. doi: 10.1111/cga.12227
27. Imbard A, Benoist JF, Blom HJ. Neural tube defects, folic acid and methylation. Int J Environ Res Public Health. (2013) 10(9):4352–89. doi: 10.3390/ijerph10094352
28. Greene ND, Stanier P, Moore GE. The emerging role of epigenetic mechanisms in the etiology of neural tube defects. Epigenetics. (2011) 6(7):875–83. doi: 10.4161/epi.6.7.16400
29. I LECK. Causation of neural tube defects: clues from epidemiology. Br Med Bull. (1974) 30(2):158–63. doi: 10.1093/oxfordjournals.bmb.a071187
30. O’Leary VB, Parle-McDermott A, Molloy AM, Kirke PN, Johnson Z, Conley M, et al. MTRR And MTHFR polymorphism: link to down syndrome? Am J Med Genet. (2002) 107(2):151–5. doi: 10.1002/ajmg.10121
31. Wong WY, Merkus HM, Thomas CM, Menkveld R, Zielhuis GA, Steegers-Theunissen RP. Effects of folic acid and zinc sulfate on male factor subfertility: a double-blind, randomized, placebo-controlled trial. Fertil Steril. (2002) 77(3):491–8. doi: 10.1016/S0015-0282(01)03229-0
32. Padmanabhan R. Etiology, pathogenesis and prevention of neural tube defects. Congenital Anom. (2006) 46(2):55–67. doi: 10.1111/j.1741-4520.2006.00104.x
33. Smithells RW, Sheppard S, Schorah CJ. Vitamin dificiencies and neural tube defects. Arch Dis Child. (1976) 51(12):944–50. doi: 10.1136/adc.51.12.944
34. Wald N, Sneddon J, Densem J, Frost C, Stone R. Prevention of neural tube defects: results of the medical research council vitamin study. Lancet. (1991) 338(8760):131–7. doi: 10.1016/0140-6736(91)90133-A
35. Berry RJ, Li Z, Erickson JD, Li S, Moore CA, Wang H, et al. Prevention of neural-tube defects with folic acid in China. N Engl J Med. (1999) 341(20):1485–90. doi: 10.1056/NEJM199911113412001
36. Czeizel AE, Dudás I, Paput L, Bánhidy F. Prevention of neural-tube defects with periconceptional folic acid, methylfolate, or multivitamins? Ann Nutr Metab. (2011) 58(4):263–71. doi: 10.1159/000330776
37. Czeizel AE, Dudas I. Prevention of the first occurrence of neural-tube defects by periconceptional vitamin supplementation. N Engl J Med. (1992) 327(26):1832–5. doi: 10.1056/NEJM199212243272602
38. MRC G. Vitamin study research, prevention of neural tube defects: results of the MRC vitamin study. Lancet. (1991) 338:132–7. doi: 10.1016/0140-6736(91)90133-A
39. Burren KA, Savery D, Massa V, Kok RM, Scott JM, Blom HJ, et al. Gene–environment interactions in the causation of neural tube defects: folate deficiency increases susceptibility conferred by loss of Pax3 function. Hum Mol Genet. (2008) 17(23):3675–85. doi: 10.1093/hmg/ddn262
40. Beaudin AE, Abarinov EV, Noden DM, Perry CA, Chu S, Stabler SP, et al. Shmt1 and de novo thymidylate biosynthesis underlie folate-responsive neural tube defects in mice. Am J Clin Nutr. (2011) 93(4):789–98. doi: 10.3945/ajcn.110.002766
41. Fleming A, Copp AJ. Embryonic folate metabolism and mouse neural tube defects. Science. (1998) 280(5372):2107–9. doi: 10.1126/science.280.5372.2107
42. Dunlevy LP, Chitty LS, Burren KA, Doudney K, Stojilkovic-Mikic T, Stanier P, et al. Abnormal folate metabolism in foetuses affected by neural tube defects. Brain. (2007) 130(4):1043–9. doi: 10.1093/brain/awm028
43. van Straaten HW, Copp AJ. Curly tail: a 50-year history of the mouse spina bifida model. Anat Embryol. (2001) 203(4):225–38. doi: 10.1007/s004290100169
44. Greene ND, Leung KY, Gay V, Burren K, Mills K, Chitty LS, et al. Inositol for the prevention of neural tube defects: a pilot randomised controlled trial. Br J Nutr. (2016) 115(6):974–83. doi: 10.1017/S0007114515005322
45. Cavalli P, Tonni G, Grosso E, Poggiani C. Birth defects res A clin mol teratol: effects of inositol supplementation in a cohort of mothers at risk of producing an NTD pregnancy. Altern Med Rev. (2011) 16(4):377–8. doi: 10.1002/brda.22853
46. Warkany J, Petering HG. Congenital malformations of the central nervous system in rats produced by maternal zinc deficiency. Teratology. (1972) 5(3):319–34. doi: 10.1002/tera.1420050307
47. Purandare SM, Ware SM, Kwan KM, Gebbia M, Bassi MT, Deng JM, et al. A complex syndrome of left-right axis, central nervous system and axial skeleton defects in Zic3 mutant mice. Development. (2002) 129(9):2293–302.
48. Isaković J, Šimunić I, Jagečić D, Hribljan V, Mitrečić D. Overview of neural tube defects: gene–environment interactions, preventative approaches and future perspectives. Biomedicines. (2022) 10(5):965. doi: 10.3390/biomedicines10050965
49. Joubert BR, Den Dekker HT, Felix JF, Bohlin J, Ligthart S, Beckett E, et al. Maternal plasma folate impacts differential DNA methylation in an epigenome-wide meta-analysis of newborns. Nat Commun. (2016) 7(1):1–8. doi: 10.1038/ncomms10577
50. Wang L, Chang S, Wang Z, Wang S, Huo J, Ding G, et al. Altered GNAS imprinting due to folic acid deficiency contributes to poor embryo development and may lead to neural tube defects. Oncotarget. (2017) 8(67):110797. doi: 10.18632/oncotarget.22731
51. Pei P, Yu J, Shen J, Li X, Wu J, Wang S, et al. Folate deficiency induced H2A ubiquitination to lead to downregulated expression of genes involved in neural tube defects. Epigenetics Chromatin. (2019) 12(1):1–9. doi: 10.1186/s13072-018-0245-6
52. Chen CP. Syndromes, disorders and maternal risk factors associated with neural tube defects (V). Taiwan J Obstet Gynecol. (2008) 47(3):259–66. doi: 10.1016/S1028-4559(08)60122-9
53. Zhang J, Chen FZ, Gao Q, Sun JH, Tian GP, Gao YM. Hyperthermia induces upregulation of connexin43 in the golden hamster neural tube. Birth Defects Res A Clin Mol Teratol. (2012) 94(1):16–21. doi: 10.1002/bdra.22852
54. Zhang T, Leng Z, Liu W, Wang X, Yan X, Yu L. Suppressed expression of mitogen-activated protein kinases in hyperthermia induced defective neural tube. Neurosci Lett. (2015) 594:6–11. doi: 10.1016/j.neulet.2015.03.046
55. Edwards MJ, Saunders RD, Shiota K. Effects of heat on embryos and foetuses. Int J Hyperthermia. (2003) 19(3):295–324. doi: 10.1080/0265673021000039628
56. Lundberg YW, Wing MJ, Xiong W, Zhao J, Finnell RH. Genetic dissection of hyperthermia-induced neural tube defects in mice. Birth Defects Res A Clin Mol Teratol. (2003) 67(6):409–13. doi: 10.1002/bdra.10044
57. Moretti ME, Bar-Oz B, Fried S, Koren G. Maternal hyperthermia and the risk for neural tube defects in offspring: systematic review and meta-analysis. Epidemiology. (2005):216–9. doi: 10.1097/01.ede.0000152903.55579.15
58. Luteijn JM, Brown MJ, Dolk H. Influenza and congenital anomalies: a systematic review and meta-analysis. Hum Reprod. (2014) 29(4):809–23. doi: 10.1093/humrep/det455
59. Salih MA, Murshid WR, Seidahmed MZ. Epidemiology, prenatal management, and prevention of neural tube defects. Saudi Med J. (2014) 35(Suppl 1):S15.25551106
60. Sandford MK, Kissling GE, Joubert PE. Neural tube defect etiology: new evidence concerning maternal hyperthermia, health and diet. Dev Med Child Neurol. (1992) 34(8):661–75. doi: 10.1111/j.1469-8749.1992.tb11502.x
61. Shaw GM, Todoroff K, Velie EM, Lammer EJ. Maternal illness, including fever, and medication use as risk factors for neural tube defects. Teratology. (1998) 57(1):1–7. doi: 10.1002/(SICI)1096-9926(199801)57:1%3C1::AID-TERA1%3E3.0.CO;2-6
62. Milunsky A, Ulcickas M, Rothman KJ, Willett W, Jick SS, Jick H. Maternal heat exposure and neural tube defects. JAMA. (1992) 268(7):882–5. doi: 10.1001/jama.1992.03490070064043
63. Suarez L, Felkner M, Hendricks K. The effect of fever, febrile illnesses, and heat exposures on the risk of neural tube defects in a Texas-Mexico border population. Birth Defects Res A Clin Mol Teratol. (2004) 70(10):815–9. doi: 10.1002/bdra.20077
64. Blakeway H, Prasad S, Kalafat E, Heath PT, Ladhani SN, Le Doare K, et al. COVID-19 vaccination during pregnancy: coverage and safety. Am J Obstet Gynecol. (2022) 226(2):236–e1. doi: 10.1016/j.ajog.2021.08.007
65. Shimabukuro TT, Kim SY, Myers TR, Moro PL, Oduyebo T, Panagiotakopoulos L, et al. Preliminary findings of mRNA COVID-19 vaccine safety in pregnant persons. N Engl J Med. (2021):2273–82. doi: 10.1056/NEJMoa2104983
66. Trostle ME, Limaye MA, Avtushka V, Lighter JL, Penfield CA, Roman AS. COVID-19 vaccination in pregnancy: early experience from a single institution. Am J Obstet Gynecol MFM. (2021) 3(6):100464. doi: 10.1016/j.ajogmf.2021.100464
67. Ruderman RS, Mormol J, Trawick E, Perry MF, Allen EC, Millan D, et al. Association of COVID-19 vaccination during early pregnancy with risk of congenital fetal anomalies. JAMA Pediatr. (2022c):717–9. doi: 10.1001/jamapediatrics.2022.0164
68. Pratama NR, Wafa IA, Budi DS, Putra M, Wardhana MP, Wungu CD. mRNA COVID-19 vaccines in pregnancy: a systematic review. PLoS One. (2022) 17(2):e0261350. doi: 10.1371/journal.pone.0261350
69. Kalra S, Dewan P, Batra P, Sharma T, Tyagi V, Banerjee BD. Organochlorine pesticide exposure in mothers and neural tube defects in offsprings. Reprod Toxicol. (2016) 66:56–60. doi: 10.1016/j.reprotox.2016.09.005
70. Brender JD, Felkner M, Suarez L, Canfield MA, Henry JP. Maternal pesticide exposure and neural tube defects in Mexican Americans. Ann Epidemiol. (2010) 20(1):16–22. doi: 10.1016/j.annepidem.2009.09.011
71. Gashaw A, Shine S, Yimer O, Wodaje M. Risk factors associated to neural tube defects among mothers who gave birth in North Shoa Zone Hospitals, Amhara Region, Ethiopia 2020: case control study. PLoS One. (2021) 16(4):e0250719. doi: 10.1371/journal.pone.0250719
72. Atlaw D, Tekalegn Y, Sahiledengle B, Seyoum K, Solomon D, Gezahegn H, et al. Magnitude and determinants of neural tube defect in Africa: a systematic review and meta-analysis. BMC Pregnancy Childbirth. (2021) 21(1):1–4. doi: 10.1186/s12884-021-03848-9
73. Makelarski JA, Romitti PA, Rocheleau CM, Burns TL, Stewart PA, Waters MA, et al. Maternal periconceptional occupational pesticide exposure and neural tube defects. Birth Defects Res A Clin Mol Teratol. (2014) 100(11):877–86. doi: 10.1002/bdra.23293
74. Baldacci S, Gorini F, Santoro M, Pierini A, Minichilli F, Bianchi F. Environmental and individual exposure and the risk of congenital anomalies: a review of recent epidemiological evidence. Epidemiol Prev. (2018) 42(3–4):1–34. doi: 10.19191/EP18.3-4.S1.P001.057
75. Sever LE, Hessol NA, Gilbert ES, McINTYRE JM. The prevalence at birth of congenital malformations in communities near the Hanford site. Am J Epidemiol. (1988) 127(2):243–54. doi: 10.1093/oxfordjournals.aje.a114800
76. Shaw GM, Wasserman CR, O’Malley CD, Nelson V, Jackson RJ. Maternal pesticide exposure from multiple sources and selected congenital anomalies. Epidemiology. (1999):60–6. doi: 10.1097/00001648-199901000-00011
77. Balarajan R, McDowall M. Congenital malformations and agricultural workers. Lancet. (1983) 321(8333):1112–3. doi: 10.1016/S0140-6736(83)91955-4
78. Amitai Y, Koren G. High risk for neural tube defects; the role of arsenic in drinking water and rice in Asia. Med Hypotheses. (2018) 119:88–90. doi: 10.1016/j.mehy.2018.07.018
79. Wlodarczyk BJ, Cabrera RM, Hill DS, Bozinov D, Zhu H, Finnell RH. Arsenic-induced gene expression changes in the neural tube of folate transport defective mouse embryos. Neurotoxicology. (2006) 27(4):547–57. doi: 10.1016/j.neuro.2006.02.005
80. Mazumdar M. Does arsenic increase the risk of neural tube defects among a highly exposed population? A new case–control study in Bangladesh. Birth Defects Res. (2017) 109(2):92–8. doi: 10.1002/bdra.23577
81. Han ZJ, Song G, Cui Y, Xia HF, Ma X. Oxidative stress is implicated in arsenic-induced neural tube defects in chick embryos. Int J Dev Neurosci. (2011) 29(7):673–80. doi: 10.1016/j.ijdevneu.2011.06.006
82. Shalat SL, Walker DB, Finnell RH. Role of arsenic as a reproductive toxin with particular attention to neural tube defects. J Toxicol Environ Health. (1996) 48(3):253–72. doi: 10.1080/009841096161320
83. Reichard JF, Puga A. Effects of arsenic exposure on DNA methylation and epigenetic gene regulation. Epigenomics. (2010) 2(1):87–104. doi: 10.2217/epi.09.45
84. Li Y, Tollefsbol TO. Impact on DNA methylation in cancer prevention and therapy by bioactive dietary components. Curr Med Chem. (2010) 17(20):2141–51. doi: 10.2174/092986710791299966
85. Abdel-Shafy HI, Mansour MS. A review on polycyclic aromatic hydrocarbons: source, environmental impact, effect on human health and remediation. Egypt J Petroleum. (2016) 25(1):107–23. doi: 10.1016/j.ejpe.2015.03.011
86. Li Z, Zhang L, Ye R, Pei L, Liu J, Zheng X, et al. Indoor air pollution from coal combustion and the risk of neural tube defects in a rural population in Shanxi Province, China. Am J Epidemiol. (2011) 174(4):451–8. doi: 10.1093/aje/kwr108
87. Langlois PH, Hoyt AT, Lupo PJ, Lawson CC, Waters MA, Desrosiers TA, et al. Maternal occupational exposure to polycyclic aromatic hydrocarbons and risk of oral cleft-affected pregnancies. Cleft Palate Craniofac J. (2013) 50(3):337–46. doi: 10.1597/12-104
88. Ren A, Qiu X, Jin L, Ma J, Li Z, Zhang L, et al. Association of selected persistent organic pollutants in the placenta with the risk of neural tube defects. Proc Natl Acad Sci USA. (2011) 108(31):12770–5. doi: 10.1073/pnas.1105209108
89. Wang B, Jin L, Ren A, Yuan Y, Liu J, Li Z, et al. Levels of polycyclic aromatic hydrocarbons in maternal serum and risk of neural tube defects in offspring. Environ Sci Technol. (2015) 49(1):588–96. doi: 10.1021/es503990v
90. Huang Y, Lin S, Jin L, Wang L, Ren A. Decreased global DNA hydroxymethylation in neural tube defects: association with polycyclic aromatic hydrocarbons. Epigenetics. (2019) 14(10):1019–29. doi: 10.1080/15592294.2019.1629233
91. Ailes EC, Gilboa SM, Gill SK, Broussard CS, Crider KS, Berry RJ, et al. National Birth Defects Prevention Study. Association between antibiotic use among pregnant women with urinary tract infections in the first trimester and birth defects, National Birth Defects Prevention Study 1997 to 2011. Birth Defects Res A Clin Mol Teratol. (2016) 106(11):940–9. doi: 10.1002/bdra.23570
92. Crider KS, Cleves MA, Reefhuis J, Berry RJ, Hobbs CA, Hu DJ. Antibacterial medication use during pregnancy and risk of birth defects: national birth defects prevention study. Arch Pediatr Adolesc Med. (2009) 163(11):978–85. doi: 10.1001/archpediatrics.2009.188
93. Andersen JT, Petersen M, Jimenez-Solem E, Broedbaek K, Andersen EW, Andersen NL, et al. Trimethoprim use in early pregnancy and the risk of miscarriage: a register-based nationwide cohort study. Epidemiol Infect. (2013a) 141(8):1749–55. doi: 10.1017/S0950268812002178
94. Andersen JT, Petersen M, Jimenez-Solem E, Rasmussen JN, Andersen NL, Afzal S, et al. Trimethoprim use prior to pregnancy and the risk of congenital malformation: a register-based nationwide cohort study. Obstet Gynecol Int. (2013b) 2013. doi: 10.1155/2013/364526
95. Hansen C, Andrade SE, Freiman H, Dublin S, Haffenreffer K, Cooper WO, et al. Trimethoprim–sulfonamide use during the first trimester of pregnancy and the risk of congenital anomalies. Pharmacoepidemiol Drug Saf. (2016) 25(2):170–8. doi: 10.1002/pds.3919
96. Goldberg O, Koren G, Landau D, Lunenfeld E, Matok I, Levy A. Exposure to nitrofurantoin during the first trimester of pregnancy and the risk for major malformations. J Clin Pharmacol. (2013) 53(9):991–5. doi: 10.1002/jcph.139
97. Nordeng H, Lupattelli A, Romøren M, Koren G. Neonatal outcomes after gestational exposure to nitrofurantoin. Obstet Gynecol. (2013) 121(2 PART 1):306–13. doi: 10.1097/AOG.0b013e31827c5f88
98. Interrante JD, Ailes EC, Lind JN, Anderka M, Feldkamp ML, Werler MM, et al. Risk comparison for prenatal use of analgesics and selected birth defects, National Birth Defects Prevention Study 1997–2011. Ann Epidemiol. (2017) 27(10):645–53. doi: 10.1016/j.annepidem.2017.09.003
99. Broussard CS, Rasmussen SA, Reefhuis J, Friedman JM, Jann MW, Riehle-Colarusso T, et al. Maternal treatment with opioid analgesics and risk for birth defects. Am J Obstet Gynecol. (2011) 204(4):314–e1. doi: 10.1016/j.ajog.2010.12.039
100. Yazdy MM, Mitchell AA, Tinker SC, Parker SE, Werler MM. Periconceptional use of opioids and the risk of neural tube defects. Obstet Gynecol. (2013) 122(4):838. doi: 10.1097/AOG.0b013e3182a6643c
101. Gilboa SM, Broussard CS, Devine OJ, Duwe KN, Flak AL, Boulet SL, et al. Influencing clinical practice regarding the use of antiepileptic medications during pregnancy: modeling the potential impact on the prevalences of spina bifida and cleft palate in the United States. Am J Med Genet C Semin Med Genet. (2011,) 157(3):234–46. doi: 10.1002/ajmg.c.30306
102. Lewicka I, Kocyłowski R, Grzesiak M, Gaj Z, Oszukowski P, Suliburska J. Selected trace elements concentrations in pregnancy and their possible role—literature review. Ginekol Pol. (2017) 88(9):509–14. doi: 10.5603/GP.a2017.0093
103. Rautiainen S, Manson JE, Lichtenstein AH, Sesso HD. Dietary supplements and disease prevention—a global overview. Nat Rev Endocrinol. (2016) 12(7):407–20. doi: 10.1038/nrendo.2016.54
104. Ward E. Addressing nutritional gaps with multivitamin and mineral supplements. Nutr J. (2014) 13(1):1. doi: 10.1186/1475-2891-13-72
105. Saghazadeh A, Mahmoudi M, Dehghani Ashkezari A, Oliaie Rezaie N, Rezaei N. Systematic review and meta-analysis shows a specific micronutrient profile in people with down syndrome: lower blood calcium, selenium and zinc, higher red blood cell copper and zinc, and higher salivary calcium and sodium. PLoS One. (2017) 12(4):e0175437. doi: 10.1371/journal.pone.0175437
106. Groenen PM, van Rooij IA, Peer PG, Ocké MC, Zielhuis GA, Steegers-Theunissen RP. Low maternal dietary intakes of iron, magnesium, and niacin are associated with spina bifida in the offspring. J Nutr. (2004) 134(6):1516–22. doi: 10.1093/jn/134.6.1516
107. Özel Ş, Ozyer S, Aykut O, Çinar M, Yılmaz OH, Caglar A, et al. Maternal second trimester blood levels of selected heavy metals in pregnancies complicated with neural tube defects. J Matern Fetal Neonatal Med. (2019) 32(15):2547–53. doi: 10.1080/14767058.2018.1441280
108. Cengiz B, Söylemez F, Öztürk E, Çavdar AO. Serum zinc, selenium, copper, and lead levels in women with second-trimester induced abortion resulting from neural tube defects: a preliminary study. Biol Trace Elem Res. (2004) 97:225–35. doi: 10.1385/BTER:97:3:225
109. Demir N, Başaranoğlu M, Huyut Z, Değer İ, Karaman K, Şekeroğlu MR, et al. The relationship between mother and infant plasma trace element and heavy metal levels and the risk of neural tube defect in infants. J Matern Fetal Neonatal Med. (2019) 32(9):1433–40. doi: 10.1080/14767058.2017.1408064
110. Cavdar AO, Bahceci M, Akar N, Dincer FN, Erten J. Maternal hair zinc concentration in neural tube defects in Turkey. Biol Trace Elem Res. (1991) 30:81–5. doi: 10.1007/BF02990344
111. Dey AC, Shahidullah M, Mannan MA, Noor MK, Saha L, Rahman SA. Maternal and neonatal serum zinc level and its relationship with neural tube defects. J Health Popul Nutr. (2010) 28(4):343. doi: 10.3329/jhpn.v28i4.6040
112. Yan L, Wang B, Li Z, Liu Y, Huo W, Wang J, et al. Association of essential trace metals in maternal hair with the risk of neural tube defects in offspring. Birth Defects Res. (2017) 109(3):234–43. doi: 10.1002/bdra.23594
113. Bergmann KE, Makosch G, Tews KH. Abnormalities of hair zinc concentration in mothers of newborn infants with spina bifida. Am J Clin Nutr. (1980) 33(10):2145–50. doi: 10.1093/ajcn/33.10.2145
114. Milunsky A, Morris JS, Jick H, Rothman KJ, Ulcickas M, Jick SS, et al. Maternal zinc and fetal neural tube defects. Teratology. (1992) 46(4):341–8. doi: 10.1002/tera.1420460405
115. Yin S, Wang C, Wei J, Wang D, Jin L, Liu J, et al. Essential trace elements in placental tissue and risk for fetal neural tube defects. Environ Int. (2020) 139:105688. doi: 10.1016/j.envint.2020.105688
116. Karacor T, Kirici P, Nacar MC, Bulbul M, Onderci M, Peker N. Evaluation of specific heavy metal levels of pregnant women complicated by neural tube defects. Ann Med Res. (2020) 27(2):551–99. doi: 10.1242/dev.129.9.2293
117. Wang C, Pi X, Chen Y, Wang D, Yin S, Jin L, et al. Prenatal exposure to barium and the occurrence of neural tube defects in offspring. Sci Total Environ. (2021) 764:144245. doi: 10.1016/j.scitotenv.2020.144245
118. Wang B, Pang Y, Zhang Y, Zhang L, Ye R, Yan L, et al. Thorium and fetal neural tube defects: an epidemiological evidence from large case-control study. Genes Environ. (2021) 43(1):1. doi: 10.1186/s41021-021-00227-w
119. Pi X, Wang D, Wang C, Li Z, Wang L, Yue W, et al. Placental concentrations of alkali metals and their associations with neural tube defects in offspring. Placenta. (2022) 121:46–52. doi: 10.1016/j.placenta.2022.02.020
120. Wolujewicz P, Ross ME. The search for genetic determinants of human neural tube defects. Curr Opin Pediatr. (2019) 31(6):739. doi: 10.1097/MOP.0000000000000817
121. Harris MJ, Juriloff DM. Mouse mutants with neural tube closure defects and their role in understanding human neural tube defects. Birth Defects Res A Clin Mol Teratol. (2007) 79(3):187–210. doi: 10.1002/bdra.20333
122. Salbaum JM, Kappen C. Neural tube defect genes and maternal diabetes during pregnancy. Birth Defects Res A Clin Mol Teratol. (2010) 88(8):601–11. doi: 10.1002/bdra.20680
123. Ross ME, Mason CE, Finnell RH. Genomic approaches to the assessment of human spina bifida risk. Birth Defects Res. (2017) 109(2):120–8. doi: 10.1002/bdra.23592
124. Blencowe H, Kancherla V, Moorthie S, Darlison MW, Modell B. Estimates of global and regional prevalence of neural tube defects for 2015: a systematic analysis. Ann N Y Acad Sci. (2018) 1414(1):31–46. doi: 10.1111/nyas.13548
125. Wallingford JB, Niswander LA, Shaw GM, Finnell RH. The continuing challenge of understanding, preventing, and treating neural tube defects. Science. (2013) 339(6123):1222002. doi: 10.1126/science.1222002
126. Bassuk AG, Kibar Z. Genetic basis of neural tube defects. Semin Pediatr Neurol (2009) 16(3):101–10. doi: 10.1016/j.spen.2009.06.001
127. Byrne J, Carolan S. Adverse reproductive outcomes among pregnancies of aunts and (spouses of) uncles in Irish families with neural tube defects. Am J Med Geneti A. (2006) 140(1):52–61. doi: 10.1002/ajmg.a.31049
128. Deak KL, Siegel DG, George TM, Gregory S, Ashley-Koch A, Speer MC, et al. Further evidence for a maternal genetic effect and a sex-influenced effect contributing to risk for human neural tube defects. Birth Defects Res A Clin Mol Teratol. (2008) 82(10):662–9. doi: 10.1002/bdra.20511
129. Lei Y, Fathe K, McCartney D, Zhu H, Yang W, Ross ME, et al. Rare LRP6 variants identified in spina bifida patients. Hum Mutat. (2015) 36(3):342–9. doi: 10.1002/humu.22750
130. Hart J, Miriyala K. Neural tube defects in Waardenburg syndrome: a case report and review of the literature. Am J Med Genet A. (2017) 173(9):2472–7. doi: 10.1002/ajmg.a.38325
131. Singh N, Kumble Bhat V, Tiwari A, Kodaganur SG, Tontanahal SJ, Sarda A, et al. A homozygous mutation in TRIM36 causes autosomal recessive anencephaly in an Indian family. Hum Mol Genet. (2017) 26(6):1104–14. doi: 10.1093/hmg/ddx020
132. Wang L, Ren A, Tian T, Li N, Cao X, Zhang P, et al. Whole-exome sequencing identifies damaging de novo variants in anencephalic cases. Front Neurosci. (2019) 13:1285. doi: 10.3389/fnins.2019.01285
133. Ishida M, Cullup T, Boustred C, James C, Docker J, English C, et al. A targeted sequencing panel identifies rare damaging variants in multiple genes in the cranial neural tube defect, anencephaly. Clin Genet. (2018) 93(4):870–9. doi: 10.1111/cge.13189
134. Aguiar-Pulido V, Wolujewicz P, Martinez-Fundichely A, Elhaik E, Thareja G, Abdel Aleem A, et al. Systems biology analysis of human genomes points to key pathways conferring spina bifida risk. Proc Natl Acad Sci USA. (2021) 118(51):e2106844118. doi: 10.1073/pnas.2106844118
135. Stolk L, Bouwland-Both MI, van Mill NH, Verbiest MM, Eilers PH, Zhu H, et al. Epigenetic profiles in children with a neural tube defect; a case-control study in two populations. PLoS One. (2013) 8(11):e78462. doi: 10.1371/journal.pone.0078462
136. Wilde JJ, Petersen JR, Niswander L. Genetic, epigenetic, and environmental contributions to neural tube closure. Annu Rev Genet. (2014) 48:583–611. doi: 10.1146/annurev-genet-120213-092208
137. Borgel J, Guibert S, Li Y, Chiba H, Schübeler D, Sasaki H, et al. Targets and dynamics of promoter DNA methylation during early mouse development. Nat Genet. (2010) 42(12):1093–100. doi: 10.1038/ng.708
138. Okano M, Bell DW, Haber DA, Li E. DNA Methyltransferases Dnmt3a and Dnmt3b are essential for de novo methylation and mammalian development. Cell. (1999) 99(3):247–57. doi: 10.1016/S0092-8674(00)81656-6
139. Scott JM, Weir DG, Molloy A, McPartlin J, Daly L, Kirke P. Folic acid metabolism and mechanisms of neural tube defects. Ciba foundation symposium 181-neural tube defects: neural tube defects: ciba foundation symposium 181. Chichester, UK: John Wiley & Sons, Ltd. (2007). p. 180–91.
140. Finnell RH, Blom HJ, Shaw GM. Does global hypomethylation contribute to susceptibility to neural tube defects? Am J Clin Nutr. (2010) 91(5):1153–4. doi: 10.3945/ajcn.2010.29534
141. Ichi S, Costa FF, Bischof JM, Nakazaki H, Shen YW, Boshnjaku V, et al. Folic acid remodels chromatin on Hes1 and Neurog2 promoters during caudal neural tube development. J Biol Chem. (2010) 285(47):36922–32. doi: 10.1074/jbc.M110.126714
142. Yao TP, Oh SP, Fuchs M, Zhou ND, Ch’ng LE, Newsome D, et al. Gene dosage–dependent embryonic development and proliferation defects in mice lacking the transcriptional integrator p300. Cell. (1998) 93(3):361–72. doi: 10.1016/S0092-8674(00)81165-4
143. Bu P, Evrard YA, Lozano G, Dent SY. Loss of Gcn5 acetyltransferase activity leads to neural tube closure defects and exencephaly in mouse embryos. Mol Cell Biol. (2007) 27(9):3405–16. doi: 10.1128/MCB.00066-07
144. Dunwoodie SL, Rodriguez TA, Beddington RS. Msg1 and Mrg1, founding members of a gene family, show distinct patterns of gene expression during mouse embryogenesis. Mech Dev. (1998) 72(1-2):27–40. doi: 10.1016/S0925-4773(98)00011-2
145. Finnell RH, Waes JG, Eudy JD, Rosenquist TH. Molecular basis of environmentally induced birth defects. Annu Rev Pharmacol Toxicol. (2002) 42(1):181–208. doi: 10.1146/annurev.pharmtox.42.083001.110955
146. Menegola E, Di Renzo F, Broccia ML, Prudenziati M, Minucci S, Massa V, et al. Inhibition of histone deacetylase activity on specific embryonic tissues as a new mechanism for teratogenicity. Birth Defects Res B Dev Reprod Toxicol. (2005) 74(5):392–8. doi: 10.1002/bdrb.20053
147. Vega RB, Matsuda K, Oh J, Barbosa AC, Yang X, Meadows E, et al. Histone deacetylase 4 controls chondrocyte hypertrophy during skeletogenesis. Cell. (2004) 119(4):555–66. doi: 10.1016/j.cell.2004.10.024
148. Cheng HL, Mostoslavsky R, Saito SI, Manis JP, Gu Y, Patel P, et al. Developmental defects and p53 hyperacetylation in Sir2 homolog (SIRT1)-deficient mice. Proc Natl Acad Sci USA. (2003) 100(19):10794–9. doi: 10.1073/pnas.1934713100
149. Harris MJ, Juriloff DM. An update to the list of mouse mutants with neural tube closure defects and advances toward a complete genetic perspective of neural tube closure. Birth Defects Res A Clin Mol Teratol. (2010) 88(8):653–69. doi: 10.1002/bdra.20676
150. Bultman S, Gebuhr T, Yee D, La Mantia C, Nicholson J, Gilliam A, et al. A Brg1 null mutation in the mouse reveals functional differences among mammalian SWI/SNF complexes. Mol Cell. (2000) 6(6):1287–95. doi: 10.1016/S1097-2765(00)00127-1
151. Banting GS, Barak O, Ames TM, Burnham AC, Kardel MD, Cooch NS, et al. CECR2, A protein involved in neurulation, forms a novel chromatin remodeling complex with SNF2l. Hum Mol Genet. (2005) 14(4):513–24. doi: 10.1093/hmg/ddi048
152. Kim JK, Huh SO, Choi H, Lee KS, Shin D, Lee C, et al. Srg3, a mouse homolog of yeast SWI3, is essential for early embryogenesis and involved in brain development. Mol Cell Biol. (2001) 21(22):7787–95. doi: 10.1128/MCB.21.22.7787-7795.2001
153. Gyuris A, Donovan DJ, Seymour KA, Lovasco LA, Smilowitz NR, Halperin AL, et al. The chromatin-targeting protein Brd2 is required for neural tube closure and embryogenesis. Biochim Biophys Acta. (2009) 1789(5):413–21. doi: 10.1016/j.bbagrm.2009.03.005
154. Pryor SE, Massa V, Savery D, Greene ND, Copp AJ. Convergent extension analysis in mouse whole embryo culture. In: Walker JM, editor. Planar cell polarity: Methods and protocols. (2012). p. 133–46. doi: 10.1007/978-1-61779-510-7_11
155. Copp AJ, Greene ND. Genetics and development of neural tube defects. J Pathol. (2010) 220(2):217–30. doi: 10.1002/path.2643
156. Greene ND, Stanier P, Copp AJ. Genetics of human neural tube defects. Hum Mol Genet. (2009) 18(R2):R113–29. doi: 10.1093/hmg/ddp347
157. Vladar EK, Antic D, Axelrod JD. Planar cell polarity signaling: the developing cell’s compass. Cold Spring Harbor Perspect Biol. (2009) 1(3):a002964. doi: 10.1101/cshperspect.a002964
158. Wallingford JB, Rowning BA, Vogeli KM, Rothbächer U, Fraser SE, Harland RM. Dishevelled controls cell polarity during Xenopus gastrulation. Nature. (2000) 405(6782):81–5. doi: 10.1038/35011077
159. Vandenberg AL, Sassoon DA. Non-canonical Wnt signaling regulates cell polarity in female reproductive tract development via van gogh-like 2. Development. (2009) 136(9):1559–70. doi: 10.1242/dev.034066
160. Kibar Z, Vogan KJ, Groulx N, Justice MJ, Underhill DA, Gros P. Ltap, a mammalian homolog of Drosophila Strabismus/Van Gogh, is altered in the mouse neural tube mutant Loop-tail. Nat Genet. (2001) 28(3):251–5. doi: 10.1038/90081
161. Murdoch JN, Doudney K, Paternotte C, Copp AJ, Stanier P. Severe neural tube defects in the loop-tail mouse result from mutation of Lpp1, a novel gene involved in floor plate specification. Hum Mol Genet. (2001) 10(22):2593–601. doi: 10.1093/hmg/10.22.2593
162. Juriloff DM, Harris MJ. A consideration of the evidence that genetic defects in planar cell polarity contribute to the etiology of human neural tube defects. Birth Defects Res A Clin Mol Teratol. (2012) 94(10):824–40. doi: 10.1002/bdra.23079
163. Murdoch JN, Damrau C, Paudyal A, Bogani D, Wells S, Greene ND, et al. Genetic interactions between planar cell polarity genes cause diverse neural tube defects in mice. Dis Model Mech. (2014) 7(10):1153–63. doi: 10.1242/dmm.016758
164. Lu X, Borchers AG, Jolicoeur C, Rayburn H, Baker JC, Tessier-Lavigne M. PTK7/CCK-4 Is a novel regulator of planar cell polarity in vertebrates. Nature. (2004) 430(6995):93–8. doi: 10.1038/nature02677
165. Merte J, Jensen D, Wright K, Sarsfield S, Wang Y, Schekman R, et al. Sec24b selectively sorts Vangl2 to regulate planar cell polarity during neural tube closure. Nat Cell Biol. (2010) 12(1):41–6. doi: 10.1038/ncb2002
166. Escobedo N, Contreras O, Muñoz R, Farías M, Carrasco H, Hill C, et al. Syndecan 4 interacts genetically with Vangl2 to regulate neural tube closure and planar cell polarity. Development. (2013) 140(14):3008–17. doi: 10.1242/dev.091173
167. Etheridge SL, Ray S, Li S, Hamblet NS, Lijam N, Tsang M, et al. Murine dishevelled 3 functions in redundant pathways with dishevelled 1 and 2 in normal cardiac outflow tract, cochlea, and neural tube development. PLoS Genet. (2008) 4(11):e1000259. doi: 10.1371/journal.pgen.1000259
168. Yu H, Smallwood PM, Wang Y, Vidaltamayo R, Reed R, Nathans J. Frizzled 1 and frizzled 2 genes function in palate, ventricular septum and neural tube closure: general implications for tissue fusion processes. Development. (2010) 137(21):3707–17. doi: 10.1242/dev.052001
169. Gray RS, Abitua PB, Wlodarczyk BJ, Szabo-Rogers HL, Blanchard O, Lee I, et al. The planar cell polarity effector Fuz is essential for targeted membrane trafficking, ciliogenesis and mouse embryonic development. Nat Cell Biol. (2009) 11(10):1225–32. doi: 10.1038/ncb1966
170. Heydeck W, Liu A. PCP Effector proteins inturned and fuzzy play nonredundant roles in the patterning but not convergent extension of mammalian neural tube. Dev Dyn. (2011) 240(8):1938–48. doi: 10.1002/dvdy.22696
171. Zeng H, Hoover AN, Liu A. PCP Effector gene Inturned is an important regulator of cilia formation and embryonic development in mammals. Dev Biol. (2010) 339(2):418–28. doi: 10.1016/j.ydbio.2010.01.003
172. Narimatsu M, Bose R, Pye M, Zhang L, Miller B, Ching P, et al. Regulation of planar cell polarity by Smurf ubiquitin ligases. Cell. (2009) 137(2):295–307. doi: 10.1016/j.cell.2009.02.025
173. Carroll EA, Gerrelli D, Gasca S, Berg E, Beier DR, Copp AJ, et al. Cordon-bleu is a conserved gene involved in neural tube formation. Dev Biol. (2003) 262(1):16–31. doi: 10.1016/S0012-1606(03)00323-3
174. Yamamoto S, Nishimura O, Misaki K, Nishita M, Minami Y, Yonemura S, et al. Cthrc1 selectively activates the planar cell polarity pathway of Wnt signaling by stabilizing the Wnt-receptor complex. Dev Cell. (2008) 15(1):23–36. doi: 10.1016/j.devcel.2008.05.007
175. Stiefel D, Shibata T, Meuli M, Duffy PG, Copp AJ. Tethering of the spinal cord in mouse fetuses and neonates with spina bifida. J Neurosurg. (2003) 99(2):206–13. doi: 10.3171/spi.2003.99.2.0206
176. Curtin JA, Quint E, Tsipouri V, Arkell RM, Cattanach B, Copp AJ, et al. Mutation of Celsr1 disrupts planar polarity of inner ear hair cells and causes severe neural tube defects in the mouse. Curr Biol. (2003) 13(13):1129–33. doi: 10.1016/S0960-9822(03)00374-9
177. Murdoch JN, Henderson DJ, Doudney K, Gaston-Massuet C, Phillips HM, Paternotte C, et al. Disruption of scribble (Scrb1) causes severe neural tube defects in the circletail mouse. Hum Mol Genet. (2003) 12(2):87–98. doi: 10.1093/hmg/ddg014
178. Kibar Z, Torban E, McDearmid JR, Reynolds A, Berghout J, Mathieu M, et al. Mutations in VANGL1 associated with neural-tube defects. N Engl J Med. (2007) 356(14):1432–7. doi: 10.1056/NEJMoa060651
179. Lei YP, Zhang T, Li H, Wu BL, Jin L, Wang HY. VANGL2 Mutations in human cranial neural-tube defects. N Engl J Med. (2010) 362(23):2232–5. doi: 10.1056/NEJMc0910820
180. Allache R, De Marco P, Merello E, Capra V, Kibar Z. Role of the planar cell polarity gene CELSR1 in neural tube defects and caudal agenesis. Birth Defects Res A Clin Mol Teratol. (2012) 94(3):176–81. doi: 10.1002/bdra.23002
181. Bartsch O, Kirmes I, Thiede A, Lechno S, Gocan H, Florian IS, et al. Novel VANGL1 gene mutations in 144 Slovakian, Romanian and German patients with neural tube defects. Mol Syndromol. (2012) 3(2):76–81. doi: 10.1159/000339668
182. Merello E, Mascelli S, Raso A, Piatelli G, Consales A, Cama A, et al. Expanding the mutational spectrum associated to neural tube defects: literature revision and description of novel VANGL1 mutations. Birth Defects Res A Clin Mol Teratol. (2015) 103(1):51–61. doi: 10.1002/bdra.23305
183. Wang L, Xiao Y, Tian T, Jin L, Lei Y, Finnell RH, et al. Digenic variants of planar cell polarity genes in human neural tube defect patients. Mol Genet Metab. (2018) 124(1):94–100. doi: 10.1016/j.ymgme.2018.03.005
184. Lei Y, Kim SE, Chen Z, Cao X, Zhu H, Yang W, et al. Variants identified in PTK7 associated with neural tube defects. Mol Genet Genomic Med. (2019) 7(4):e00584. doi: 10.1002/mgg3.584
185. Qiao X, Liu Y, Li P, Chen Z, Li H, Yang X, et al. Genetic analysis of rare coding mutations of CELSR1–3 in congenital heart and neural tube defects in Chinese people. Clin Sci. (2016) 130(24):2329–40. doi: 10.1042/CS20160686
186. De Marco P, Merello E, Rossi A, Piatelli G, Cama A, Kibar Z, et al. FZD6 Is a novel gene for human neural tube defects. Hum Mutat. (2012) 33(2):384–90. doi: 10.1002/humu.21643
187. Allache R, Wang M, De Marco P, Merello E, Capra V, Kibar Z. Genetic studies of ANKRD6 as a molecular switch between Wnt signaling pathways in human neural tube defects. Birth Defects Res A Clin Mol Teratol. (2015) 103(1):20–6. doi: 10.1002/bdra.23273
188. Allache R, Lachance S, Guyot MC, De Marco P, Merello E, Justice MJ, et al. Novel mutations in Lrp6 orthologs in mouse and human neural tube defects affect a highly dosage-sensitive Wnt non-canonical planar cell polarity pathway. Hum Mol Genet. (2014) 23(7):1687–99. doi: 10.1093/hmg/ddt558
189. Bosoi CM, Capra V, Allache R, Trinh VQ, De Marco P, Merello E, et al. Identification and characterization of novel rare mutations in the planar cell polarity gene PRICKLE1 in human neural tube defects. Hum Mutat. (2011) 32(12):1371–5. doi: 10.1002/humu.21589
190. Lei Y, Zhu H, Duhon C, Yang W, Ross ME, Shaw GM, et al. Mutations in planar cell polarity gene SCRIB are associated with spina bifida. PLoS One. (2013) 8(7):e69262. doi: 10.1371/journal.pone.0069262
191. Yin H, Peng R, Chen Z, Wang H, Zhang T, Zheng Y. WDR34 Mutation from anencephaly patients impaired both SHH and PCP signaling pathways. J Hum Genet. (2020) 65(11):985–93. doi: 10.1038/s10038-020-0793-z
192. Li J, Xie Q, Gao J, Wang F, Bao Y, Wu L, et al. Aberrant Gcm1 expression mediates Wnt/β-catenin pathway activation in folate deficiency involved in neural tube defects. Cell Death Dis. (2021) 12(3):1–6. 151. doi: 10.1038/s41419-020-03313-z
193. Stuhlmiller TJ, García-Castro MI. Current perspectives of the signaling pathways directing neural crest induction. Cell Mol Life Sci. (2012) 69:3715–37. doi: 10.1007/s00018-012-0991-8
194. Palmer AJ, Savery D, Massa V, Copp AJ, Greene ND. Genetic interaction of Pax3 mutation and canonical Wnt signaling modulates neural tube defects and neural crest abnormalities. Genesis. (2021) 59(11):e23445. 150. doi: 10.1002/dvg.23445
195. Weidinger G, Moon RT. When wnts antagonize wnts. J Cell Biol. (2003) 162(5):753–6. 152. doi: 10.1083/jcb.200307181
196. Bryja V, Andersson ER, Schambony A, Esner M, Bryjová L, Biris KK, et al. The extracellular domain of Lrp5/6 inhibits noncanonical wnt signaling in vivo. Mol Biol Cell. (2009) 20(3):924–36. doi: 10.1091/mbc.e08-07-0711
197. Andersson ER, Bryjova L, Biris K, Yamaguchi TP, Arenas E, Bryja V. Genetic interaction between Lrp6 and Wnt5a during mouse development. Dev Dyn. (2010) 239(1):237–45. doi: 10.1002/dvdy.22101
198. Puppo F, Thomé V, Lhoumeau AC, Cibois M, Gangar A, Lembo F, et al. Protein tyrosine kinase 7 has a conserved role in Wnt/β-catenin canonical signalling. EMBO Rep. (2011) 12(1):43–9. doi: 10.1038/embor.2010.185
199. Bin-Nun N, Lichtig H, Malyarova A, Levy M, Elias S, Frank D. PTK7 Modulates Wnt signaling activity via LRP6. Development. (2014) 141(2):410–21. doi: 10.1242/dev.095984
200. Ingham PW, McMahon AP. Hedgehog signaling in animal development: paradigms and principles. Genes Dev. (2001) 15(23):3059–87. doi: 10.1101/gad.938601
201. Zhang XM, Ramalho-Santos M, McMahon AP. Smoothened mutants reveal redundant roles for Shh and Ihh signaling including regulation of L/R asymmetry by the mouse node. Cell. (2001) 105(6):781–92. doi: 10.1016/S0092-8674(01)00385-3
202. Chiang C, Litingtung Y, Lee E, Young KE, Corden JL, Westphal H, et al. Cyclopia and defective axial patterning in mice lacking Sonic hedgehog gene function. Nature. (1996) 383(6599):407–13. doi: 10.1038/383407a0
203. Ybot-Gonzalez P, Cogram P, Gerrelli D, Copp AJ. Sonic hedgehog and the molecular regulation of mouse neural tube closure. Development. (2002) 129(10):2507–17. doi: 10.1242/dev.129.10.2507
204. Shirane M, Ogawa M, Motoyama J, Nakayama KI. Regulation of apoptosis and neurite extension by FKBP38 is required for neural tube formation in the mouse. Genes Cells. (2008) 13(6):635–51. doi: 10.1111/j.1365-2443.2008.01194.x
205. Wong RL, Wlodarczyk BJ, Min KS, Scott ML, Kartiko S, Yu W, et al. Mouse Fkbp8 activity is required to inhibit cell death and establish dorso-ventral patterning in the posterior neural tube. Hum Mol Genet. (2008) 17(4):587–601. doi: 10.1093/hmg/ddm333
206. Pan Y, Wang C, Wang B. Phosphorylation of Gli2 by protein kinase A is required for Gli2 processing and degradation and the Sonic Hedgehog-regulated mouse development. Dev Biol. (2009) 326(1):177–89. doi: 10.1016/j.ydbio.2008.11.009
207. Hsu CY, Chang NC, Lee MW, Lee KH, Sun DS, Lai C, et al. LUZP Deficiency affects neural tube closure during brain development. Biochem Biophys Res Commun. (2008) 376(3):466–71. doi: 10.1016/j.bbrc.2008.08.170
208. Huang Y, Roelink H, McKnight GS. Protein kinase A deficiency causes axially localized neural tube defects in mice. J Biol Chem. (2002) 277(22):19889–96. doi: 10.1074/jbc.M111412200
209. Goodrich LV, Milenkovic L, Higgins KM, Scott MP. Altered neural cell fates and medulloblastoma in mouse patched mutants. Science. (1997) 277(5329):1109–13. doi: 10.1126/science.277.5329.1109
210. Milenkovic L, Goodrich LV, Higgins KM, Scott MP. Mouse patched1 controls body size determination and limb patterning. Development. (1999) 126(20):4431–40. doi: 10.1242/dev.126.20.4431
211. Gunther T, Struwe M, Aguzzi A, Schughart K. Open brain, a new mouse mutant with severe neural tube defects, shows altered gene expression patterns in the developing spinal cord. Development. (1994) 120(11):3119–30. doi: 10.1242/dev.120.11.3119
212. Eggenschwiler JT, Espinoza E, Anderson KV. Rab23 is an essential negative regulator of the mouse Sonic hedgehog signalling pathway. Nature. (2001) 412(6843):194–8. doi: 10.1038/35084089
213. Eggenschwiler JT, Bulgakov OV, Qin J, Li T, Anderson KV. Mouse Rab23 regulates hedgehog signaling from smoothened to Gli proteins. Dev Biol. (2006) 290(1):1–2. doi: 10.1016/j.ydbio.2005.09.022
214. Echelard Y, Epstein DJ, St-Jacques B, Shen L, Mohler J, McMahon JA, et al. Sonic hedgehog, a member of a family of putative signaling molecules, is implicated in the regulation of CNS polarity. Cell. (1993) 75(7):1417–30. doi: 10.1016/0092-8674(93)90627-3
215. Cooper AF, Yu KP, Brueckner M, Brailey LL, Johnson L, McGrath JM, et al. Cardiac and CNS defects in a mouse with targeted disruption of suppressor of fused. Development. (2005) 132(19):4407–17. doi: 10.1242/dev.02021
216. Svärd J, Henricson KH, Persson-Lek M, Rozell B, Lauth M, Bergström Å, et al. Genetic elimination of Suppressor of fused reveals an essential repressor function in the mammalian Hedgehog signaling pathway. Dev Cell. (2006) 10(2):187–97. doi: 10.1016/j.devcel.2005.12.013
217. Ikeda A, Ikeda S, Gridley T, Nishina PM, Naggert JK. Neural tube defects and neuroepithelial cell death in Tulp3 knockout mice. Hum Mol Genet. (2001) 10(12):1325–34. doi: 10.1093/hmg/10.12.1325
218. Patterson VL, Damrau C, Paudyal A, Reeve B, Grimes DT, Stewart ME, et al. Mouse hitchhiker mutants have spina bifida, dorso-ventral patterning defects and polydactyly: identification of Tulp3 as a novel negative regulator of the Sonic hedgehog pathway. Hum Mol Genet. (2009) 18(10):1719–39. doi: 10.1093/hmg/ddp075
219. Roessler E, Belloni E, Gaudenz K, Jay P, Berta P, Scherer SW, et al. Mutations in the human Sonic Hedgehog gene cause holoprosencephaly. Nat Genet. (1996) 14(3):357–60. doi: 10.1038/ng1196-357
220. Roessler E, Du YZ, Mullor JL, Casas E, Allen WP, Gillessen-Kaesbach G, et al. Loss-of-function mutations in the human GLI2 gene are associated with pituitary anomalies and holoprosencephaly-like features. Proc Natl Acad Sci USA. (2003) 100(23):13424–9. doi: 10.1073/pnas.2235734100
221. Roessler E, Ma Y, Ouspenskaia MV, Lacbawan F, Bendavid C, Dubourg C, et al. Truncating loss-of-function mutations of DISP1 contribute to holoprosencephaly-like microform features in humans. Hum Genet. (2009) 125(4):393–400. doi: 10.1007/s00439-009-0628-7
222. Felder B, Stegmann K, Schultealbert A, Geller F, Strehl E, Ermert A, et al. Evaluation of BMP4 and its specific inhibitor NOG as candidates in human neural tube defects (NTDs). Eur J Hum Genet. (2002) 10(11):753–6. doi: 10.1038/sj.ejhg.5200875
223. Anderson MJ, Schimmang T, Lewandoski M. An FGF3-BMP signaling axis regulates caudal neural tube closure, neural crest specification and anterior-posterior axis extension. PLoS Genet. (2016) 12(5):e1006018. doi: 10.1371/journal.pgen.1006018
224. Stottmann RW, Klingensmith J. Bone morphogenetic protein signaling is required in the dorsal neural folds before neurulation for the induction of spinal neural crest cells and dorsal neurons. Dev Dyn. (2011) 240(4):755–65. doi: 10.1002/dvdy.22579
225. Castranio T, Mishina Y. Bmp2 is required for cephalic neural tube closure in the mouse. Dev Dyn. (2009) 238(1):110–22. doi: 10.1002/dvdy.21829
226. Ybot-Gonzalez P, Gaston-Massuet C, Girdler G, Klingensmith J, Arkell R, Greene ND, et al. Neural plate morphogenesis during mouse neurulation is regulated by antagonism of Bmp signalling. Development. (2007) 134(17):3203–11. doi: 10.1242/dev.008177
227. Fernandes M, Gutin G, Alcorn H, McConnell SK, Hébert JM. Mutations in the BMP pathway in mice support the existence of two molecular classes of holoprosencephaly. Development. (2007) 134(21):3789–94. doi: 10.1242/dev.004325
228. Qin L, Ahn KJ, Wine Lee L Jr, de Charleroy C, Crenshaw EB III. Analyses with double knockouts of the Bmpr1a and Bmpr1b genes demonstrate that BMP signaling is involved in the formation of precerebellar mossy fiber nuclei derived from the rhombic lip. PLoS One. (2019) 14(12):e0226602. doi: 10.1371/journal.pone.0226602
229. Li H, Zhang J, Chen S, Wang F, Zhang T, Niswander L. Genetic contribution of retinoid-related genes to neural tube defects. Hum Mutat. (2018) 39(4):550–62. doi: 10.1002/humu.23397
230. Lee LM, Leung CY, Tang WW, Choi HL, Leung YC, McCaffery PJ, et al. A paradoxical teratogenic mechanism for retinoic acid. Proc Natl Acad Sci USA. (2012) 109(34):13668–73. doi: 10.1073/pnas.1200872109
231. Iulianella A, Beckett B, Petkovich M, Lohnes D. A molecular basis for retinoic acid-induced axial truncation. Dev Biol. (1999) 205(1):33–48. doi: 10.1006/dbio.1998.9110
232. Maden M, Gale E, Kostetskii I, Zile M. Vitamin A-deficient quail embryos have half a hindbrain and other neural defects. Curr Biol. (1996) 6(4):417–26. doi: 10.1016/S0960-9822(02)00509-2
233. Niederreither K, Subbarayan V, Dollé P, Chambon P. Embryonic retinoic acid synthesis is essential for early mouse post-implantation development. Nat Genet. (1999) 21(4):444–8. doi: 10.1038/7788
234. Niederreither K, Abu-Abed S, Schuhbaur B, Petkovich M, Chambon P, Dollé P. Genetic evidence that oxidative derivatives of retinoic acid are not involved in retinoid signaling during mouse development. Nat Genet. (2002) 31(1):84–8. doi: 10.1038/ng876
235. Lohnes D, Mark M, Mendelsohn C, Dolle P, Dierich A, Gorry P, et al. Function of the retinoic acid receptors (RARs) during development (I). craniofacial and skeletal abnormalities in RAR double mutants. Development. (1994) 120(10):2723–48. doi: 10.1242/dev.120.10.2723
236. Sewell W, Kusumi K. Genetic analysis of molecular oscillators in mammalian somitogenesis: clues for studies of human vertebral disorders. Birth Defects Res, Part C. (2007) 81(2):111–20. doi: 10.1002/bdrc.20091
237. Liu D, Xue J, Liu Y, Gu H, Wei X, Ma W, et al. Inhibition of NRF2 signaling and increased reactive oxygen species during embryogenesis in a rat model of retinoic acid-induced neural tube defects. Neurotoxicology. (2018) 69:84–92. doi: 10.1016/j.neuro.2018.09.005
238. Chen N, Xu J, Zhang X, Li S, Zhu W, Cui H, et al. Effect of Notch1 on neural tube defects and neural stem cell differentiation induced by all-trans retinoic acid. Mol Med Rep. (2021) 23(3):1. doi: 10.3892/mmr.2021.11859
239. Lowery LA, Sive H. Strategies of vertebrate neurulation and a re-evaluation of teleost neural tube formation. Mech Dev. (2004) 121(10):1189–97. doi: 10.1016/j.mod.2004.04.022
240. Nikolopoulou E, Galea GL, Rolo A, Greene ND, Copp AJ. Neural tube closure: cellular, molecular and biomechanical mechanisms. Development. (2017) 144(4):552–66. doi: 10.1242/dev.145904
241. Main H, Radenkovic J, Jin SB, Lendahl U, Andersson ER. Notch signaling maintains neural rosette polarity. PLoS One. (2013) 8(5):e62959. doi: 10.1371/journal.pone.0062959
242. Lardelli M, Williams R, Mitsiadis T, Lendahl U. Expression of the Notch 3 intracellular domain in mouse central nervous system progenitor cells is lethal and leads to disturbed neural tube development. Mech Dev. (1996) 59(2):177–90. doi: 10.1016/0925-4773(96)00589-8
243. Blom HJ, Shaw GM, den Heijer M, Finnell RH. Neural tube defects and folate: case far from closed. Nat Rev Neurosci. (2006) 7(9):724–31. doi: 10.1038/nrn1986
244. Običan SG, Finnell RH, Mills JL, Shaw GM, Scialli AR. Folic acid in early pregnancy: a public health success story. FASEB J. (2010) 24(11):4167–74. doi: 10.1096/fj.10-165084
245. Piedrahita JA, Oetama B, Bennett GD, Van Waes J, Kamen BA, Richardson J, et al. Mice lacking the folic acid-binding protein Folbp1 are defective in early embryonic development. Nat Genet. (1999) 23(2):228–32. doi: 10.1038/13861
246. Taparia S, Gelineau-van Waes J, Rosenquist TH, Finnell RH. Importance of folate-homocysteine homeostasis during early embryonic development. Clin Chem Lab Med. (2007) 45(12):1717–27. doi: 10.1515/CCLM.2007.345
247. Barber RC, Lammer EJ, Shaw GM, Greer KA, Finnell RH. The role of folate transport and metabolism in neural tube defect risk. Mol Genet Metab. (199966(1):1–9. doi: 10.1006/mgme.1998.2787
248. Zhao R, Russell RG, Wang Y, Liu L, Gao F, Kneitz B, et al. Rescue of embryonic lethality in reduced folate carrier-deficient mice by maternal folic acid supplementation reveals early neonatal failure of hematopoietic organs. J Biol Chem. (2001) 276(13):10224–8. doi: 10.1074/jbc.C000905200
249. Wlodarczyk BJ, Tang LS, Triplett A, Aleman F, Finnell RH. Spontaneous neural tube defects in splotch mice supplemented with selected micronutrients. Toxicol Appl Pharmacol. (2006) 213(1):55–63. doi: 10.1016/j.taap.2005.09.008
250. Beaudin AE, Abarinov EV, Malysheva O, Perry CA, Caudill M, Stover PJ. Dietary folate, but not choline, modifies neural tube defect risk in Shmt1 knockout mice. Am J Clin Nutr. (2012) 95(1):109–14. doi: 10.3945/ajcn.111.020305
251. Christensen KE, Deng L, Leung KY, Arning E, Bottiglieri T, Malysheva OV, et al. A novel mouse model for genetic variation in 10-formyltetrahydrofolate synthetase exhibits disturbed purine synthesis with impacts on pregnancy and embryonic development. Hum Mol Genet. (2013) 22(18):3705–19. doi: 10.1093/hmg/ddt223
252. Barbera JP, Rodriguez TA, Greene ND, Weninger WJ, Simeone A, Copp AJ, et al. Folic acid prevents exencephaly in Cited2 deficient mice. Hum Mol Genet. (2002) 11(3):283–93. doi: 10.1093/hmg/11.3.283
253. Dunlevy LP, Burren KA, Chitty LS, Copp AJ, Greene ND. Excess methionine suppresses the methylation cycle and inhibits neural tube closure in mouse embryos. FEBS Lett. (2006) 580(11):2803–7. doi: 10.1016/j.febslet.2006.04.020
254. Dunlevy LP, Burren KA, Mills K, Chitty LS, Copp AJ, Greene ND. Integrity of the methylation cycle is essential for mammalian neural tube closure. Birth Defects Res A Clin Mol Teratol. (2006) 76(7):544–52. doi: 10.1002/bdra.20286
255. Afman LA, Blom HJ, Drittij MJ, Brouns MR, van Straaten HW. Inhibition of transmethylation disturbs neurulation in chick embryos. Dev Brain Res. (2005) 158(1-2):59–65. doi: 10.1016/j.devbrainres.2005.06.002
256. Essien FB, Wannberg SL. Methionine but not folinic acid or vitamin B-12 alters the frequency of neural tube defects in Axd mutant mice. J Nutr. (1993) 123(1):27–34. doi: 10.1093/jn/123.1.27
257. Narisawa A, Komatsuzaki S, Kikuchi A, Niihori T, Aoki Y, Fujiwara K, et al. Mutations in genes encoding the glycine cleavage system predispose to neural tube defects in mice and humans. Hum Mol Genet. (2012) 21(7):1496–503. doi: 10.1093/hmg/ddr585
258. Afman LA, Blom HJ, Van der Put NM, Van Straaten HW. Homocysteine interference in neurulation: a chick embryo model. Birth Defects Res A Clin Mol Teratol. (2003) 67(6):421–8. doi: 10.1002/bdra.10040
259. Chang H, Zhang T, Zhang Z, Bao R, Fu C, Wang Z, et al. Tissue-specific distribution of aberrant DNA methylation associated with maternal low-folate status in human neural tube defects. J Nutr Biochem. (2011) 22(12):1172–7. doi: 10.1016/j.jnutbio.2010.10.003
260. Coelho CN, Klein NW. Methionine and neural tube closure in cultured rat embryos: morphological and biochemical analyses. Teratology. (1990) 42(4):437–51. doi: 10.1002/tera.1420420412
261. Vanaerts LA, Blom HJ, Deabreu RA, Trijbels FJ, Eskes TK, Peereboom-Stegeman JH, et al. Prevention of neural tube defects by and toxicity of L-homocysteine in cultured postimplantation rat embryos. Teratology. (1994) 50(5):348–60. doi: 10.1002/tera.1420500506
262. Bjorklund NK, Gordon R. A hypothesis linking low folate intake to neural tube defects due to failure of post-translation methylations of the cytoskeleton. Int J Dev Biol. (2003) 50(2-3):135–41. doi: 10.1387/ijdb.052102nb
263. Harjunpää H, Llort Asens M, Guenther C, Fagerholm SC. Cell adhesion molecules and their roles and regulation in the immune and tumor microenvironment. Front Immunol. (2019) 10:1078. doi: 10.3389/fimmu.2019.01078
264. Gennarini G, Furley A. Cell adhesion molecules in neural development and disease. Mol Cell Neurosci. (2017) 81:1–3. doi: 10.1016/j.mcn.2017.03.010
265. Newgreen DF, Kerr RS, Minichiello J, Warren N. Changes in cell adhesion and extracellular matrix molecules in spontaneous spinal neural tube defects in avian embryos. Teratology. (1997) 55(3):195–207. doi: 10.1002/(SICI)1096-9926(199703)55:3%3C195::AID-TERA4%3E3.0.CO;2-1
266. Deak KL, Boyles AL, Etchevers HC, Melvin EC, Siegel DG, Graham FL, et al. SNPs in the neural cell adhesion molecule 1 gene (NCAM1) may be associated with human neural tube defects. Hum Genet. (2005) 117(2):133–42. doi: 10.1007/s00439-005-1299-7
267. Smith HM, Khairallah SM, Nguyen AH, Newman-Smith E, Smith WC. Misregulation of cell adhesion molecules in the Ciona neural tube closure mutant bugeye. Dev Biol. (2021) 480:14–24. doi: 10.1016/j.ydbio.2021.08.006
268. Tanoue T, Takeichi M. Mammalian Fat1 cadherin regulates actin dynamics and cell–cell contact. J Cell Biol. (2004) 165(4):517–28. doi: 10.1083/jcb.200403006
269. Badouel C, Zander MA, Liscio N, Bagherie-Lachidan M, Sopko R, Coyaud E, et al. Fat1 interacts with Fat4 to regulate neural tube closure, neural progenitor proliferation and apical constriction during mouse brain development. Development. (2015) 142(16):2781–91. doi: 10.1242/dev.123539
270. Miner JH, Cunningham J, Sanes JR. Roles for laminin in embryogenesis: exencephaly, syndactyly, and placentopathy in mice lacking the laminin α5 chain. J Cell Biol. (1998) 143(6):1713–23. doi: 10.1083/jcb.143.6.1713
271. Arikawa-Hirasawa E, Watanabe H, Takami H, Hassell JR, Yamada Y. Perlecan is essential for cartilage and cephalic development. Nat Genet. (1999) 23(3):354–8. doi: 10.1038/15537
272. De Arcangelis A, Mark M, Kreidberg J, Sorokin L, Georges-Labouesse E. Synergistic activities of alpha3 and alpha6 integrins are required during apical ectodermal ridge formation and organogenesis in the mouse. Development. (1999) 126(17):3957–68. doi: 10.1242/dev.126.17.3957
273. Holmberg J, Clarke DL, Frisén J. Regulation of repulsion versus adhesion by different splice forms of an Eph receptor. Nature. (2000) 408(6809):203–6. doi: 10.1038/35041577
274. Abdul-Aziz NM, Turmaine M, Greene ND, Copp AJ. EphrinA-EphA receptor interactions in mouse spinal neurulation: implications for neural fold fusion. Int J Dev Biol. (2004) 53(4):559–68. doi: 10.1387/ijdb.082777na
Keywords: neural tube defects (NTDs), gene, signaling pathway, genetic factors, folate
Citation: Rai S, Leydier L, Sharma S, Katwala J and Sahu A (2023) A quest for genetic causes underlying signaling pathways associated with neural tube defects. Front. Pediatr. 11:1126209. doi: 10.3389/fped.2023.1126209
Received: 10 January 2023; Accepted: 28 February 2023;
Published: 22 May 2023.
Edited by:
Shan Wang, Capital Institute of Pediatrics, ChinaReviewed by:
Qiu Xie, Peking Union Medical College Hospital (CAMS), China© 2023 Rai, Leydier, Sharma, Katwala and Sahu. This is an open-access article distributed under the terms of the Creative Commons Attribution License (CC BY). The use, distribution or reproduction in other forums is permitted, provided the original author(s) and the copyright owner(s) are credited and that the original publication in this journal is cited, in accordance with accepted academic practice. No use, distribution or reproduction is permitted which does not comply with these terms.
*Correspondence: Sunil Rai cy5yYWlAbXVhLmVkdQ== Anurag Sahu YW51cmFnc2FodWJodW5ldXJvc3VyZ2VyeUBnbWFpbC5jb20=
Specialty Section: This article was submitted to Genetics of Common and Rare Diseases, a section of the journal Frontiers in Pediatrics
Disclaimer: All claims expressed in this article are solely those of the authors and do not necessarily represent those of their affiliated organizations, or those of the publisher, the editors and the reviewers. Any product that may be evaluated in this article or claim that may be made by its manufacturer is not guaranteed or endorsed by the publisher.
Research integrity at Frontiers
Learn more about the work of our research integrity team to safeguard the quality of each article we publish.