- 1Lithuanian University of Health Sciences, Medical Academy, Pediatric Department, Kaunas, Lithuania
- 2Lithuanian University of Health Sciences, Medical Academy, Institute of Physiology and Pharmacology, Kaunas, Lithuania
- 3Rehabilitation Center “Palangos Linas”, Palanga, Lithuania
From the start of pandemics, children were described as the ones who were less affected by SARS-Cov-2 or COVID-19, which was mild in most of the cases. However, with the growing vaccination rate of the adult population, children became more exposed to the virus and more cases of severe SARS-CoV-2-induced ARDS are being diagnosed with the disabling consequences or lethal outcomes associated with the cytokine storm. Thus, we do hypothesize that some of the children could benefit from nervus vagus stimulation during COVID-19 ARDS through the inhibition of HMGB1 release and interaction with the receptor, resulting in decreased neutrophil accumulation, oxidative stress, and coagulopathy as well as lung vascular permeability. Moreover, stimulation through alpha-7 nicotinic acetylcholine receptors could boost macrophage phagocytosis and increase the clearance of DAMPs and PAMPs. Further rise of FGF10 could contribute to lung stem cell proliferation and potential regeneration of the injured lung. However, this stimulation should be very specific, timely, and of proper duration, as it could lead to such adverse effects as increased viral spread and systemic infection, especially in small children or infants due to specific pediatric immunity state and anatomical features of the respiratory system.
Introduction
Since the start of COVID-19 pandemics, children have been less addressed in the scientific investigations of SARS-CoV-2 infection as well as treatment implications. First, in comparison to adults' pediatric patients have been at lower risk for the severe course and lethal outcomes of COVID-19. Severe respiratory manifestations predominantly seen in adults were seldom documented in groups of pediatric patients who mostly tended to have an asymptomatic or mild form of infection (1–3). Mild COVID-19 in pediatric patients naturally leads to smaller hospitalization and death rates compared to sick adults. However, significant COVID-19 complications might occur in the pediatric age as well. With the increased mutation rate of SARS-CoV-2, the virus became more adaptable and targeted different age groups including children. Moreover, the pediatric population became more susceptible to SARS-CoV-2 infection due to increasing socialization, and more precautious enrollment in clinical vaccine trials resulting in later and slower vaccination rates (4). Overall, with the growing infectivity higher number of children are more likely to be severely ill, especially those with the risk factors (4). Still, the specific anti-SARS-CoV-2 treatment is lacking, and also, the pediatric population is less involved in the clinical trials for specific medication in case of severe COVID-19. Being a very specific part of the population with different immunity and distinct anatomy (e.g., respiratory tract) still under development over the whole childhood to the puberty, children require a specific glimpse into possible treatment applications during severe COVID-19 and SARS-CoV-2-induced acute lung injury (ALI).
Vagal nerve stimulation has been of interest in adult COVID-19 with a special focus on SARS-CoV-2-induced ALI. The basic concept behind is its anti-inflammatory activity with regard to cytokine storm reduction (5). Vagal nerve anti-inflammatory potential has been already shown in different chronic inflammatory processes, such as rheumatoid arthritis, systemic inflammation, acute postsurgical inflammatory response after lung lobectomy, or polymicrobial sepsis (6–9). In addition, in most the adult cases with a previous existing polymorbidity, COVID-19 is associated with decreased cardiac function. Thus, vagal stimulation can contribute to improvement via adjusting the sympathetic vagal imbalance (10). Children do have fewer cardiovascular and pulmonary comorbidities; thus, vagal nerve stimulation could be of interest emphasizing its immune-modulatory potential. First, it is demonstrated to limit dendritic cell recruitment into the lungs (11). Moreover, it can inhibit HMGB1 release, leading to a decrease of different pro-inflammatory cytokines. High-mobility group box protein 1 (HMGB1) has been studied as an initiating factor that participates in multiple inflammation-related diseases in adult and pediatric patients (12, 13). Research studies in the pediatric population show that many inflammatory diseases, including respiratory system-related diseases like pneumonia/bronchiolitis (14), influenza virus or respiratory syncytial virus (RSV)-induced infection, and lung injury (15, 16), correlate with increased levels of HMGB1. In the majority of cases, levels of HMGB1 are directly linked to the severity of the disease. Considering that SARS-CoV-2 might initiate inflammatory processes and induce ALI, HMGB1 could be used as a target for specific prevention and treatment option for pediatric COVID-19 and SARS-CoV-2-induced ALI. As nervus vagus activation can inhibit HMGB1 release, we hypothesize that vagal nerve stimulation could be of benefit in pediatric SARS-CoV-2 infection.
Hypothesis Validation
Acute Respiratory Diseases and Pathobiology of HMGB1
Different respiratory viruses, including SARS-CoV-2, target epithelial cells in the respiratory tract and through their cytopathic effect induces cellular death leading to a local release of different damage-associated molecular pattern proteins (DAMPs) together with pathogen-associated molecular pattern proteins (PAMPs) (17). High mobility group box 1 (HMGB1) is one of the most broadly studied DAMPs which is upstream of interleukin (IL) 6 release (13, 18). It plays an important role in different acute and chronic lung diseases as well as other inflammatory processes caused by various stimuli (19–21). HMGB1 plasma levels are shown to be associated with severity of the disease, survival, and mortality of bacterial pneumonia complicated by ARDS, meanwhile, treatment with HMGB1-specific antagonist revealed improved survival in preclinical animal models of acute inflammation (22–24). The significantly increased HMGB1 levels were detected in bronchoalveolar lavage fluid prior to the severe hyperoxia-induced lung injury (25). In a study of neonatal ARDS (NRDS), HMGB1 is considered a crucial indicator of disease severity and clinical outcomes (26, 27). Few studies proved that RSV, a leading cause of infant bronchiolitis, promotes HMGB1 release and respiratory epithelial cell necroptosis (28–30).
HMGB1 is a chromatin-linked, non-histonic protein, 99% identical to mammals with a cytokine activity on a cytosolic, nuclear and extracellular level (18). After damage and cellular death or via active secretion by stimulation of innate immune cells, excessive quantities of HMGB1 are released resulting in an inflammatory process together with enhanced cells, such as neutrophils or monocyte, recruitment, migration, and facilitated cell proliferation. Experimental studies demonstrated that HMGB1 plays a pivotal role in ALI through the recruitment of leucocytes into the lungs (25, 31). In addition, it induces neutrophil dysfunction in experimental sepsis models (32). Moreover, HMGB1 stimulates dendritic cell maturation and enhances antibody response (33, 34). The HMGB1 triggered inflammatory process is generated in two ways, i.e., binding HMGB1-specific receptors or forming complexes with DNA, RNA, or other DAMPs (35). Extracellular HMGB1 binds to Toll-like receptors (TLR), such as TLR3, TLR4, and receptors for the advanced glycation end product (RAGE). Thus, the P38 mitogen-activated protein kinase (MAPK), extracellular signal-regulated kinase 1/2 (ERK1/2), nuclear factor (NF)-kB, and other downstream pathways are activated which results in the secretion of tumor necrosis factor (TNF) α, IL-1β, IL6, transforming growth factor (TGF)-1β, platelet-derived growth factor (PDGF) and other molecules, leading to increased tissue damage and organ dysfunction (36, 37). HMGB1-RAGE/TLR4-axis has been attributed to a central role in influenza virus-induced infection models. Additionally, in experimental studies, HMGB1 antagonists demonstrated partial protection against influenza-induced lung injury as well as encephalopathy (38, 39), and prevention of necroptotic respiratory epithelial cell death (40). There are growing data regarding HMGB1 function in the regulation of autophagy and its diagnostic potential in ALI (41–43). HMGB1 is a late mediator causing cell apoptosis and autophagy via translocation of NF-kB inducing the production of various previously mentioned pro-inflammatory cytokines (44). Different cytokines further stimulate the release of HMGB1, thus, contributing to a positive feedback loop enhancing the inflammatory cascade (25, 45).
HMGB1 Role in SARS-CoV-2-Induced Lung Inflammation
Considering previous findings, we hypothesize that HMGB1 can be a crucial player in SARS-CoV-2-induced lung injury of adults as well as in pediatric patients. Indeed, a study by Chen et al. revealed that COVID-19 patients had higher plasma levels of HMGB1 compared to healthy volunteers (46). In SARS-CoV-2-induced respiratory tract infection, HMGB1 initiates inflammation via two different pathways. First, it triggers TLR4 leading to IL6 release. IL6 is one of the essential pro-inflammatory mediators in COVID-19 infection. Increased plasma levels of IL6 are detected in approximately half of patients with COVID-19 and are associated with the disease severity (47, 48). A study by Sivakorn et al. detected a significant correlation between HMGB1 and IL6 levels and other prognostic biomarkers, such as D-dimer and C-reactive protein (CRP) on admission to intensive care unit (ICU) (49). Even though, most children present asymptomatic or with the mild COVID-19 showing normal concentrations of IL6 (50), severely ill patients do have increased values of IL6 (51). Moreover, IL6 is frequently elevated in MISC – a multisystemic inflammatory response syndrome that is associated with a previous asymptomatic SARS-CoV-2 infection (52). A study by Abrams et al. demonstrated that higher IL6 levels together with other factors such as CRP or ferritin were linked to increased MISC admission to pediatric ICU, and IL-6 concentrations were related to shock (53). Taking into account above mentioned experimental data and clinical studies from the adult population, we speculate that HMGB1 could be an important contributor to pediatric COVID-19. The data are supported by the fact that HMGB1 is associated with RSV-induced bronchiolitis, neonatal ARDS, pediatric asthma, and pneumonia (12).
The second mechanism of HMGB1 action is to promote the expression of angiotensin-converting-enzyme 2 (ACE2) receptor on respiratory epithelial cells (13). ACE2 is a crucial host receptor for the entry of SARS-CoV-2 and is widely expressed in the human body, including naso- and oropharyngeal mucosa, and lower respiratory tract, e.g., alveolar epithelial cells. HMGB1 induces ACE2 expression via RAGE (54) which is important in different respiratory conditions as well as ALI/ARDS (55). As shown in a study by Pinto et al., increased expression of ACE2 correlates with SARS-CoV-2 induced disease severity and outcome (56). Few studies identified lower levels of ACE2 expression in children compared to adults, thus, it could partially explain milder COVID-19 cases in the pediatric population (57–59). However, some of the data show, that ACE2 expression could be ethnicity dependent (60). Moreover, ACE2 expression in children positive for SARS-CoV-2 was higher compared to negative ones (59, 61). Nevertheless, the ACE2 expression level was not related to viral loads (59). Thus, the mechanism of HMGB1-ACE2 could be of less importance in pediatric COVID, still, it could be of interest in severe SARS-CoV-2 induced ALI or in specific risk populations.
ALI, HMGB1, and Cholinergic System
A growing body of evidence shows the interplay between different immune cells, pro-inflammatory cytokines, and chemokines, and neural cells in the respiratory tract (5). Vagal afferents in the lung can identify different tissue-damaging factors (62) and contribute to the reduction of inflammation via e.g., the cholinergic anti-inflammatory pathway (63, 64). As the major sensory channel in the airway-brain axis, the vagus nerve regulates respiration, and respiratory defense and provides “feedback” to the brain (65). This process can be disturbed via ALI/ARDS. More data demonstrate an important role of the cholinergic system in ALI via inhibition or reduction of HMGB1. A recent study by Sitapara et al. revealed a potential therapeutic effect of alpha 7 nicotinic acetylcholine receptor (α7nAChR) agonist which attenuated hyperoxia-induced acute lung injury (HALI) by a significant decrease of HMGB1 levels in the airways and serum of mice (66). Nevertheless, the same study showed improved macrophage phagocytosis. Induction of α7nAChR demonstrated to prevent activation of NF-kB pathway leading to inhibition of HMGB1 secretion in in vitro cultured human macrophages (67). In addition, in experimental mouse models, vagal nerve stimulation reduced serum HMGB1 levels resulting in increased survival (68). The cholinergic anti-inflammatory pathway affects HMGB1 release and inhibits HMGB1 receptor-mediated activities. Acetylcholine and α7nAChR agonist constrained RAGE-induced endocytosis of HMGB1 complexes leading to downregulation of proinflammatory cytokines and pyroptosis (69). Moreover, α7nAChR signaling inhibits inflammasome activation, thus, reducing the release of HMGB1 together with IL-1α, IL-1β, and IL-18 (70) (Figure 1). In addition, vagal-α7nAChR stimulation promotes lung stem cell proliferation and differentiation in FGF-10 dependent manner (71). FGF-10 is important in mesenchymal stromal cell mobilization and lung inflammatory cytokines' reduction. Altogether, vagal-α7nAChR stimulation in pediatric patients could dampen SARS-COV-2-induced lung inflammation and promote lung tissue recovery via inhibition of HMGB1 and FGF-10 induction.
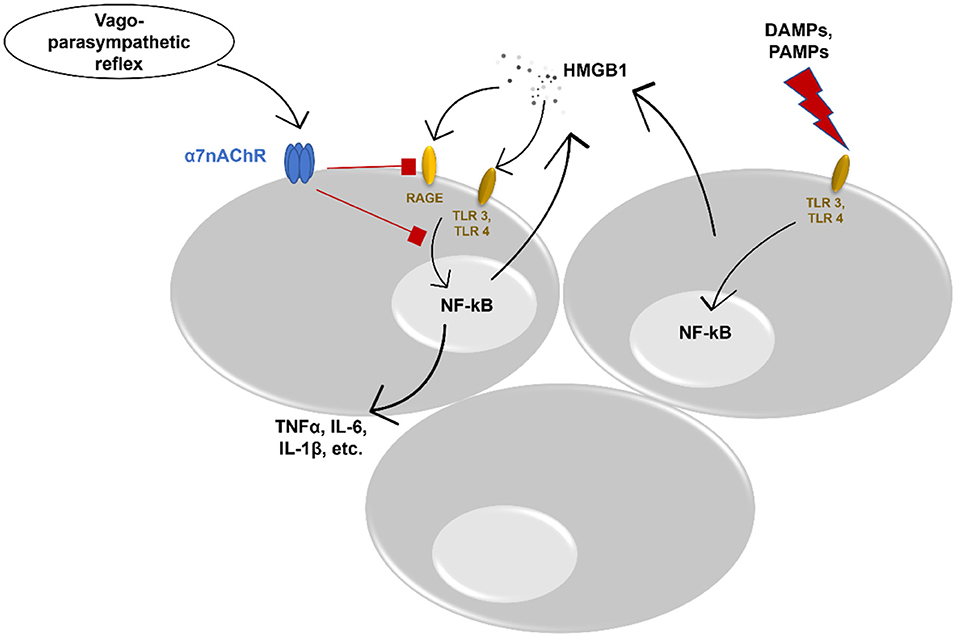
Figure 1. Potential anti-inflammatory effect of vagus nerve stimulation. DAMPs, danger associated molecular patterns; PAMPs, pathogen associated molecular patterns; TLR, toll-like receptor; NF-kB, nuclear factor kappa-light-chain-enhancer of activated B cells; HMGB1, High-mobility group box protein 1; RAGE, receptor for advanced glycation end product; TNFα, tumor necrosis factor alpha; IL, interleukin; α7nAChR, alpha 7 nicotinic acetylcholine receptor.
Application of Nervus Vagus Stimulation in Children
To date, different studies and protocols discuss and propose the best techniques and modes of vagal nerve stimulation (72). The duration and mode of nervus vagus stimulation seem to be pathology and age-dependent with a focus on a preferable effect. In addition, there are some other important confounding factors, such as gender, time of the day, and concomitant diseases of a patient. The majority of the studies apply transcutaneous, or percutaneous auricular nervus vagus stimulation associated with minimal side effects, such as local irritation or pain (72). A short-term auricular vagal stimulation has been urged for COVID-19 ALI patients as well (5, 73). Considering the pediatric population, transcutaneous auricular stimulation would be most suitable as this application is non-invasive with minimum transient side effects. Moreover, children do have less confounders, as they do have fewer comorbidities, thus, they are using fewer medications which could interfere with the beneficial effect of n. vagus stimulation or influence mode and duration of its application. Nevertheless, a lot of severely ill pediatric COVID-19 patients have different comorbidities (74, 75) and could be prescribed various medications, such as anti-inflammatory agents (non-steroid or glucocorticoids), etc. Thus, vagal stimulation modifications emphasizing different duration and frequencies with regard to the specific side effects and COVID-19 treatment applications might be taken into account. However, nervus vagus stimulation in neonates, premature babies, and infants, which correspond to another group of pediatric patients prone to severe COVID-19, could be challenging due to their anatomy, i.e., smaller ears. Overall, additional even non-invasive procedures applied in a healthcare environment can be stressful and children, especially smaller ones, can be less compliant compared to adults (72). Still, vagal stimulation has been successfully used in different pediatric conditions, such as nephrotic syndrome or epilepsy (76–79), showing its potential and opportunities in other pediatric diseases, including SARS-CoV-2-induced ALI. Another consideration should be with regard to voltage and frequency of nervus vagus stimulation. The higher voltage could be associated with pronounced bronchospasm (80), thus low voltages should be applied, especially in systemic nervus vagus stimulation, as specific fiber stimulation in case of lung pathology or specific stimulation of α7nAChR might be challenging. In addition, children do have different normal physiological heart rates (HR) and it is age-dependent. HR is shown to predict vagal nerve stimulation response (81), hence, it would be important to consider when applying vagal stimulation in children of different ages. The frequency and duration must be sufficient to decrease SARS-CoV-2-induced inflammation and be tolerable for a child. Higher voltages and longer duration can be associated with more side effects, including local pain. Currently, there is no clear duration and frequency for nervus vagus stimulation in acute pediatric conditions. Nevertheless, 2-h stimulation with 2 h off for few days (79) or once a day for 5 min (76) has been described, still, it could not be translated to COVID-19. Considering the pathophysiology and immunology of COVID-19, vagal stimulation might be started in a later phase of SARS-CoV-2-induced infection and for few days. However, there is increasing data that neuromodulation could benefit symptoms of long-covid (82) which is more frequent in asymptomatic children or children with mild disease (83), thus repeated stimulation could be applied to improve post-covid signs and symptoms.
Possible Risks of Vagal Nerve Stimulation in Pediatric COVID-19
In a variety of model systems, the vagus nerve acts as an anti-inflammatory. The majority of these counter-inflammatory actions have been attributed to macrophage nicotinic receptor activation (84–87). Little is known about the role of the vagal nerve in modulating the activity of other cells, such as CD4+ T cells and CD8+ cells, involved in an inflammatory response. SARS-CoV-2 infection may not be unique in this aspect as most the acute viral infection pathogens induce both CD4+ and CD8+ cells activation and proliferation (88). However, activation of T-cells might not be optimal in some patients with COVID-19, due to delayed or defective type I interferon (IFN) responses (89), potentially distorting T cell responses. Type I interferon (IFN-alpha and IFN-beta) is secreted by virus-infected cells (90), and this explains why viral load could have a major impact on the magnitude and quality of the T cell response (91). Besides, even though infants have a good cellular arsenal fighting against viral infections, they can still be underdeveloped (92). As an example, newborns do have diminished production of interferons which reaches adult levels only in children who are 1–2 years old. Furthermore, the production of other pro-inflammatory cytokines is decreased, and levels are rising with age, however, still lower than in adults (93). Additionally, neonates do present with an impaired macrophage response to IFN-gamma and neonatal monocytes and macrophages do have impaired response to multiple TLR ligands, which are crucial in anti-viral and initial pro-inflammatory response (94). Moreover, infants do have an attenuated capacity to generate memory CD4+ T cell response (94). Thus, they are physiologically and immunologically more prone to viral infections with a possibility of severe complications (e.g., pneumonia, ALI/ARDS). Reduced T cell response dependent on IFN also has been ascribed to the activation of the vagus nerve (95, 96). T cells play critical roles in pulmonary host defense against viral pathogens therefore stimulation of the vagal nerve would affect the initial response to the pathogen, which allows the virus to spread further bypassing the primary barrier. CD4+ (helper) cells help B cells to produce antibodies promoting the long-standing protective immunity (97), and, thus, the stimulation of the vagus nerve during the incubation period and in the early stages of the symptoms appearance of coronavirus disease is not recommended. One prominent feature of SARS-Co-V-2 infection is lymphopenia with the following decrease in the count of CD4+ T cells, CD8+ T cells, B cells, and natural killers (98, 99). Many respiratory viruses, such as influenza or human rhinovirus, cause transient lymphopenia, which lasts only 2–4 days after symptoms start (100), while COVID-19 associated lymphopenia may be more severe or persistent (101). The peripheral lymphopenia observed in patients with COVID-19 may reflect the recruitment of lymphocytes to the respiratory tract, although autopsy studies of patients' lungs were not identified excessive levels of lymphocytes in bronchoalveolar lavage fluid (102). The mechanism of lymphopenia in COVID-19 remains incompletely understood, therefore, there is a presumption that the reduction in the number of T cells may be related to the anti-inflammatory role of the vagus nerve.
Dendritic cells (DC) are potent antigen-presenting cells that respond to sites of injury and are crucial for the priming phase of the immune response (103). Vagal nerve stimulation produces an anti-inflammatory effect by inhibiting pulmonary DC recruitment to the lung and preventing ALI in animal models (11, 104). Neonatal DCs are less polyfunctional than those of adults, in response to TLR activation, therefore neonates and infants are much more susceptible to a wide variety of infections (105). As compared to adults, this higher susceptibility is speculated to reflect impairments in both innate and adaptive immunity (106). On activation, pulmonary DC produces a range of inflammatory mediators (107), including the cytokines interleukin (IL)-6 and transforming growth factor (TGF)-beta (108, 109). TGF is a key mediator of pulmonary edema in ALI as it is triggered locally by the αvβ6 integrin (110). DC cells are also known as the IFN factory of the immune system, equipped to detect viruses and able to produce IFN levels far exceeding those produced by infected cells or other immune cells (111). Understanding the density and activity of DCs in COVID-19 is critical due to their potential to significantly amplify the IFN activation, which also represents a risk for immunopathology (112). Moreover, there are findings suggesting reduced IFN signatures in patients with severe COVID-19 (113), in contrast to the early and strong IFN responses found in antiviral responses of mild SARS-CoV-2 infection (114). Based on these facts, it is conceivable that stimulation of the vagus nerve may weaken the antiviral barrier in adults, and may have an even greater effect in infancy, as most dendritic cells are immature (115).
The afferent and efferent vagus nerve, α7nAChR-expressing inflammatory cells, and the central vagal nucleus in the brain form an inflammatory reflex that could finely coordinate inflammation and immunity (116). Activation of this pathway is dependent on the level of inflammation in the local lung tissue (86), therefore the cholinergic anti-inflammatory pathway alleviates acute inflammation in turn preventing ALI (117). Agonists to α7nAChR reduce the release of extracellular HMGB1 and expression of the two main HMGB1 receptors RAGE and TLR4 and thus inhibit HMGB1-driven inflammation (118). It seems this cholinergic anti-inflammatory reflex is highly responsible for HMGB1 expression, and it is locally regulated, therefore the effect of external stimulation of the vagal nerve can be debatable. In addition, the study suggests, that vagus nerve stimulation significantly reduces TNF (tumor necrosis factor) levels in the spleen (94%) and liver (40%) but not in the lung (20%) (119), which explains external vagal stimulation influence is more systemic rather than local on leukocytes and it secreted cytokines level. Hence, it is possible, that the external vagus nerve stimulation may not be less effective in children, and the therapeutic effect might be achieved through the changes in systemic immunity.
Taken all together, nervus vagus stimulation could be an optimal therapeutic option in pediatric patients, however, a specific population of children should be selected, e.g., older children and adolescents could benefit more compared to neonates or infants due to their immune systems which is more mature and closer to adults. Moreover, a specific period of COVID-19 must be considered as well. Children could be less capable of effectively responding to vagal nerve stimulation during the early phase of SARS-CoV-2-induced infection and it can lead to a boosted viral spread with a systemic disease due to dampened antiviral response which is already immature.
Data Availability Statement
The original contributions presented in the study are included in the article/supplementary material, further inquiries can be directed to the corresponding author.
Author Contributions
LJ: hypothesis, editing, and supervision. LJ, MM, and G-CM: analysis, writing original draft, and review. All authors contributed to the article and approved the submitted version.
Conflict of Interest
The authors declare that the research was conducted in the absence of any commercial or financial relationships that could be construed as a potential conflict of interest.
Publisher's Note
All claims expressed in this article are solely those of the authors and do not necessarily represent those of their affiliated organizations, or those of the publisher, the editors and the reviewers. Any product that may be evaluated in this article, or claim that may be made by its manufacturer, is not guaranteed or endorsed by the publisher.
Acknowledgments
We do thank Prof. E. Kaniušas for invitation to contribute to this special issue of Frontiers in Physiology.
References
1. Cruz AT, Zeichner SL. COVID-19 in children: Initial characterization of the pediatric disease. Pediatrics. (2020) 145:e20200834. doi: 10.1542/peds.2020-0834
2. Dowell AC, Butler MS, Jinks E, Tut G, Lancaster T, Sylla P, et al. Children develop robust and sustained cross-reactive spike-specific immune responses to SARS-CoV-2 infection. Nat Immunol. (2021) 23:40–9. doi: 10.1038/s41590-021-01089-8
3. Jackson WM, Price JC, Eisler L, Sun LS, Lee JJ. COVID-19 in pediatric patients: a systematic review. J Neurosurg Anesthesiol. (2022) 34:141–7. doi: 10.1097/ANA.0000000000000803
4. Interim Statement on COVID-19 Vaccination for Children and Adolescents. Available online at: https://www.who.int/news/item/24-11-2021-interim-statement-on-covid-19-vaccination-for-children-and-adolescents (accessed January 23, 2022).
5. Kaniusas E, Szeles JC, Kampusch S, Alfageme-Lopez N, Yucuma-Conde D, Li X, et al. Non-invasive auricular vagus nerve stimulation as a potential treatment for Covid19-originated acute respiratory distress syndrome. Front Physiol. (2020) 11:890. doi: 10.3389/fphys.2020.00890
6. Hoover DB. Cholinergic modulation of the immune system presents new approaches for treating inflammation. Pharmacol Ther. (2017) 179:1–16. doi: 10.1016/j.pharmthera.2017.05.002
7. Salama M, Akan A, Mueller MR. Transcutaneous stimulation of auricular branch of the vagus nerve attenuates the acute inflammatory response after lung lobectomy. World J Surg. (2020) 44:3167–174. doi: 10.1007/s00268-020-05543-w
8. Kessler W, Diedrich S, Menges P, Ebker T, Nielson M, Partecke LI, et al. The role of the vagus nerve: modulation of the inflammatory reaction in murine polymicrobial sepsis. Mediators Inflamm. (2012) 2012:467620. doi: 10.1155/2012/467620
9. Kessler W, Traeger T, Westerholt A, Neher F, Mikulcak M, Müller A, et al. The vagal nerve as a link between the nervous and immune system in the instance of polymicrobial sepsis. Langenbecks Arch Surg. (2006) 391:83–7. doi: 10.1007/s00423-006-0031-y
10. Shinlapawittayatorn K, Chinda K, Palee S, Surinkaew S, Kumfu S, Kumphune S, et al. Vagus nerve stimulation initiated late during ischemia, but not reperfusion, exerts cardioprotection via amelioration of cardiac mitochondrial dysfunction. Heart Rhythm. (2014) 11:2278–87. doi: 10.1016/j.hrthm.2014.08.001
11. Lowry DM, Morishita K, Eliceiri BP, Bansal V, Coimbra R, Costantini TW. The vagus nerve alters the pulmonary dendritic cell response to injury. J Surg Res. (2014) 192:12–8. doi: 10.1016/j.jss.2014.06.012
12. Li B, Peng X, Li H, Chen F, Chen Y, Zhang Y, et al. The performance of the alarmin HMGB1 in pediatric diseases: from lab to clinic. Immun Inflamm Dis. (2021) 9:8–30. doi: 10.1002/iid3.370
13. Andersson U, Ottestad W, Tracey KJ. Extracellular HMGB1: a therapeutic target in severe pulmonary inflammation including COVID-19? Mol Med. (2020) 26:42. doi: 10.1186/s10020-020-00172-4
14. Chong SL, Lai OF, Castillo L, Yeo JG, Nadkarni N, Teoh OH, et al. Nasal high-mobility group box 1 and caspase in bronchiolitis. Pediatr Pulmonol. (2018) 53:1627–32. doi: 10.1002/ppul.24183
15. Yu B, Li X, Wan Q, Han W, Deng C, Guo C. High-mobility group box-1 protein disrupts alveolar elastogenesis of hyperoxia-injured newborn lungs. J Interferon Cytokine Res. (2016) 36:159–68. doi: 10.1089/jir.2015.0080
16. Manti S, Harford TJ, Salpietro C, Rezaee F, Piedimonte G. Induction of high-mobility group Box-1 in vitro and in vivo by respiratory syncytial virus. Pediatr Res. (2018) 83:1049–56. doi: 10.1038/pr.2018.6
17. Land WG. Role of DAMPs in respiratory virus-induced acute respiratory distress syndrome—with a preliminary reference to SARS-CoV-2 pneumonia. Genes Immun. (2021) 22:141–60. doi: 10.1038/s41435-021-00140-w
18. Andersson U, Yang H, Harris H. High-mobility group box 1 protein (HMGB1) operates as an alarmin outside as well as inside cells. Semin Immunol. (2018) 38:40–8. doi: 10.1016/j.smim.2018.02.011
19. Kang R, Chen R, Zhang Q, Hou W, Wu S, Cao L, et al. HMGB1 in health and disease. Mol Aspects Med. (2014) 40:1. doi: 10.1016/j.mam.2014.05.001
20. Andersson U, Tracey KJ. HMGB1 Is a therapeutic target for sterile inflammation and infection. Annu Rev Immunol. (2011) 29:139. doi: 10.1146/annurev-immunol-030409-101323
21. Street ME, Street ME. HMGB1: a possible crucial therapeutic target for COVID-19? Horm Res Paediatr. (2020) 93:73–5. doi: 10.1159/000508291
22. Tseng CC, Fang WF, Leung SY, Chen HC, Chang YC, Wang CC, et al. Impact of serum biomarkers and clinical factors on intensive care unit mortality and 6-month outcome in relatively healthy patients with severe pneumonia and acute respiratory distress syndrome. Dis Markers. (2014) 2014:804654. doi: 10.1155/2014/804654
23. Yang X, Cheng X, Tang Y, Qiu X, Wang Z, Fu G, et al. The role of type 1 interferons in coagulation induced by gram-negative bacteria. Blood. (2020) 135:1087–100. doi: 10.1182/blood.2019002282
24. Wang HL, Tsao SM, Bin YB, Chou YE, Yang SF. Circulating level of high mobility group box-1 predicts the severity of community-acquired pneumonia: Regulation of inflammatory responses via the c-Jun N-terminal signaling pathway in macrophages. Mol Med Rep. (2017) 16:2361–6. doi: 10.3892/mmr.2017.6892
25. Entezari M, Javdan M, Antoine DJ, Morrow DMP, Sitapara RA, Patel V, et al. Inhibition of extracellular HMGB1 attenuates hyperoxia-induced inflammatory acute lung injury. Redox Biol. (2014) 2:314–22. doi: 10.1016/j.redox.2014.01.013
26. Wang WX, Chen B, Zhang W, Zhang HR. Association between high-mobility group box 1 and neonatal respiratory distress syndrome. Zhongguo Dang Dai Er Ke Za Zhi. (2017) 19:398–401. doi: 10.7499/j.issn.1008-8830.2017.04.007
27. Influence of serum HMGB1 level on the incidence of respiratory Distress syndrome in neonates - PubMed. Available online at: https://pubmed.ncbi.nlm.nih.gov/30574754/ (accessed January 9, 2022).
28. Chen S, Yu G, Xie J, Tang W, Gao L, Long X, et al. High-mobility group box-1 protein from CC10 club cells promotes type 2 response in the later stage of respiratory syncytial virus infection. Am J Physiol Lung Cell Mol Physiol. (2019) 316:280–90. doi: 10.1152/ajplung.00552.2017
29. Hosakote YM, Brasier AR, Casola A, Garofalo RP, Kurosky A. Respiratory syncytial virus infection triggers epithelial HMGB1 release as a damage-associated molecular pattern promoting a monocytic inflammatory response. J Virol. (2016) 90:9618–31. doi: 10.1128/JVI.01279-16
30. Simpson J, Loh Z, Ullah MA, Lynch JP, Werder RB, Collinson N, et al. Respiratory syncytial virus infection promotes necroptosis and HMGB1 release by airway epithelial cells. Am J Respir Crit Care Med. (2020) 201:1358–71. doi: 10.1164/rccm.201906-1149OC
31. Huebener P, Pradere JP, Hernandez C, Gwak GY, Caviglia JM, Mu X, et al. The HMGB1/RAGE axis triggers neutrophil-mediated injury amplification following necrosis. J Clin Invest. (2015) 125:539–50. doi: 10.1172/JCI76887
32. Grégoire M, Tadié JM, Uhel F, Gacouin A, Piau C, Bone N, et al. Frontline Science: HMGB1 induces neutrophil dysfunction in experimental sepsis and in patients who survive septic shock. J Leukoc Biol. (2017) 101:1281–7. doi: 10.1189/jlb.5HI0316-128RR
33. Saenz R, Futalan D, Leutenez L, Eekhout F, Fecteau JF, Sundelius S, et al. TLR4-dependent activation of dendritic cells by an HMGB1-derived peptide adjuvant. J Transl Med. (2014) 12:211. doi: 10.1186/1479-5876-12-211
34. Messmer D, Yang H, Telusma G, Knoll F, Li J, Messmer B, et al. High mobility group box protein 1: an endogenous signal for dendritic cell maturation and Th1 polarization. J Immunol. (2004) 173:307–13. doi: 10.4049/jimmunol.173.1.307
35. Tian J, Avalos AM, Mao SY, Chen B, Senthil K, Wu H, et al. Toll-like receptor 9-dependent activation by DNA-containing immune complexes is mediated by HMGB1 and RAGE. Nat Immunol. (2007) 8:487–96. doi: 10.1038/ni1457
36. He ZW, Qin YH, Wang ZW, Chen Y, Shen Q, Dai SM. HMGB1 acts in synergy with lipopolysaccharide in activating rheumatoid synovial fibroblasts via p38 MAPK and NF- B signaling pathways. Mediators Inflamm. (2013) 2013:596716. doi: 10.1155/2013/596716
37. Pusterla T, Nèmeth J, Stein I, Wiechert L, Knigin D, Marhenke S, et al. Receptor for advanced glycation endproducts (RAGE) is a key regulator of oval cell activation and inflammation-associated liver carcinogenesis in mice. Hepatology. (2013) 58:363–73. doi: 10.1002/hep.26395
38. Okuma Y, Wake H, Teshigawara K, Takahashi Y, Hishikawa T, Yasuhara T, et al. Anti-high mobility group box 1 antibody therapy may prevent cognitive dysfunction after traumatic brain injury. World Neurosurg. (2019) 122:e864–71. doi: 10.1016/j.wneu.2018.10.164
39. Hatayama K, Nosaka N, Yamada M, Yashiro M, Fujii Y, Tsukahara H, et al. Combined effect of anti-high-mobility group box-1 monoclonal antibody and peramivir against influenza A virus-induced pneumonia in mice. J Med Virol. (2019) 91:361–9. doi: 10.1002/jmv.25330
40. Shirey KA, Lai W, Patel MC, Pletneva LM, Pang C, Kurt-Jones E, et al. Novel strategies for targeting innate immune responses to influenza. Mucosal Immunol. (2016) 9:1173–82. doi: 10.1038/mi.2015.141
41. Kang R, Livesey KM, Zeh HJ, Loze MT, Tang D. Metabolic Regulation by HMGB1-Mediated Autophagy and Mitophagy. Available online at: https://www.tandfonline.com/action/journalInformation?journalCode=kaup20 (accessed January 9, 2022).
42. Na I, Meng F, Kurgan L, Uversky VN. Autophagy-related intrinsically disordered proteins in intra-nuclear compartments. Mol Biosyst. (2016) 12:2798–817. doi: 10.1039/C6MB00069J
43. Qu L, Chen C, Chen Y, Li Y, Tang F, Huang H, et al. High-mobility group box 1 (HMGB1) and Autophagy in Acute Lung Injury (ALI): a review. Med Sci Monit. (2019) 25:1828–37. doi: 10.12659/MSM.912867
44. Liu X, Cao H, Li J, Wang B, Zhang P, Dong Zhang X, et al. Autophagy induced by DAMPs facilitates the inflammation response in lungs undergoing ischemia-reperfusion injury through promoting TRAF6 ubiquitination. Cell Death Differ. (2017) 24:683. doi: 10.1038/cdd.2017.1
45. Lee S, Piao C, Kim G, Kim JY, Choi E, Lee M. Production and application of HMGB1 derived recombinant RAGE-antagonist peptide for anti-inflammatory therapy in acute lung injury. Eur J Pharm Sci. (2018) 114:275–84. doi: 10.1016/j.ejps.2017.12.019
46. Chen L, Long X, Xu Q, Tan J, Wang G, Cao Y, et al. Elevated serum levels of S100A8/A9 and HMGB1 at hospital admission are correlated with inferior clinical outcomes in COVID-19 patients. Cell Mol Immunol. (2020) 17:992–4. doi: 10.1038/s41423-020-0492-x
47. Zhang ZL, Hou, YL, Li, DT, Li, FZ. Laboratory Findings of COVID-19: A Systematic Review and Meta-Analysis. Available online at: https://doi.org/10.1080/00365513.2020.1768587 (accessed January 9, 2022).
48. Han H, Ma Q, Li C, Liu R, Zhao L, Wang W, et al. Profiling Serum Cytokines in COVID-19 Patients Reveals IL-6 IL-10 are Disease Severity Predictors. (2020). Available from: https://doi.org/10.1080/22221751.2020.1770129 (accessed January 9, 2022).
49. Sivakorn C, Dechsanga J, Jamjumrus L, Boonnak K, Schultz MJ, Dondorp AM, et al. High mobility group box 1 and interleukin 6 at intensive care unit admission as biomarkers in critically Ill COVID-19 Patients. Am J Trop Med Hyg. (2021) 105:73–80. doi: 10.4269/ajtmh.21-0165
50. Soraya GV, Ulhaq ZS. Interleukin-6 levels in children developing SARS-CoV-2 infection. Pediatr Neonatol. (2020) 61:253. doi: 10.1016/j.pedneo.2020.04.007
51. Lu W, Yang L, Li X, Sun M, Zhang A, Qi S, et al. Early immune responses and prognostic factors in children with COVID-19: a single-center retrospective analysis. BMC Pediatr. (2021) 21:1–11. doi: 10.1186/s12887-021-02561-y
52. Diaz F, Bustos B R, Yagnam F, Karsies TJ, Vásquez-Hoyos P, Jaramillo-Bustamante JC, et al. Comparison of Interleukin-6 Plasma Concentration in Multisystem Inflammatory Syndrome in Children Associated With SARS-CoV-2 and Pediatric Sepsis. Front Pediatr. (2021) 9:1209. doi: 10.3389/fped.2021.756083
53. Abrams JY, Oster ME, Godfred-Cato SE, Bryant B, Datta SD, Campbell AP, et al. Factors linked to severe outcomes in multisystem inflammatory syndrome in children (MIS-C) in the USA: a retrospective surveillance study. Lancet Child Adolesc Health. (2021) 5:323–31. doi: 10.1016/S2352-4642(21)00050-X
54. Chen R, Huang Y, Quan J, Liu J, Wang H, Billiar TR, et al. HMGB1 as a potential biomarker and therapeutic target for severe COVID-19. Heliyon. (2020) 6:e05672. doi: 10.1016/j.heliyon.2020.e05672
55. Oczypok EA, Perkins TN, Oury TD. All the “RAGE” in lung disease: the receptor for advanced glycation endproducts (RAGE) is a major mediator of pulmonary inflammatory responses. Paediatr Respir Rev. (2017) 23:40–9. doi: 10.1016/j.prrv.2017.03.012
56. Pinto BGG, Oliveira AER, Singh Y, Jimenez L, Gonçalves ANA, Ogava RLT, et al. ACE2 Expression is increased in the lungs of patients with comorbidities associated with severe COVID-19. J Infect Dis. (2020) 222:556–63. doi: 10.1093/infdis/jiaa332
57. Li Q, Cao Z, Rahman P. Genetic variability of human angiotensin-converting enzyme 2 (hACE2) among various ethnic populations. Mol Genet Genomic Med. (2020) 8:e1344. doi: 10.1002/mgg3.1344
58. Bunyavanich S, Do A, Vicencio A. Nasal gene expression of angiotensin-converting enzyme 2 in children and adults. JAMA. (2020) 323:2427–9. doi: 10.1001/jama.2020.8707
59. Yonker LM, Neilan AM, Bartsch Y, Patel AB, Regan J, Arya P, et al. Pediatric severe acute respiratory syndrome coronavirus 2 (SARS-CoV-2): clinical presentation, infectivity, and immune responses. J Pediatr. (2020) 227:45. doi: 10.1016/j.jpeds.2020.08.037
60. Cardenas A, Rifas-Shiman SL, Sordillo JE, DeMeo DL, Baccarelli AA, Hivert MF, et al. DNA methylation architecture of the ACE2 gene in nasal cells of children. Sci Rep. (2021) 11:1–9. doi: 10.1038/s41598-021-86494-7
61. Hasan MR, Ahmad MN, Dargham SR, Zayed H, Hashemi A al, Ngwabi N, et al. Nasopharyngeal expression of angiotensin-converting enzyme 2 and transmembrane serine protease 2 in children within SARS-CoV-2-infected family clusters. Microbiol Spectr. (2021) 9:e0078321. doi: 10.1128/Spectrum.00783-21
62. Carr MJ, Undem BJ. Bronchopulmonary afferent nerves. Respirology. (2003) 8:291–301. doi: 10.1046/j.1440-1843.2003.00473.x
63. Huang Y, Zhao C, Su X. Neuroimmune regulation of lung infection and inflammation. QJM. (2019) 112:483–7. doi: 10.1093/qjmed/hcy154
64. Akella A, Deshpande SB. Vagal efferent stimulation protects against Mesobuthus tamulus venom-induced acute respiratory distress syndrome in rats. Toxicon. (2015) 108:189–201. doi: 10.1016/j.toxicon.2015.10.013
65. Chang RB, Strochlic DE, Williams EK, Umans BD, Liberles SD. Vagal sensory neuron subtypes that differentially control breathing. Cell. (2015) 161:622–33. doi: 10.1016/j.cell.2015.03.022
66. Sitapara RA, Gauthier AG, Valdés-Ferrer SI, Lin M, Patel V, Wang M, et al. The α7 nicotinic acetylcholine receptor agonist, GTS-21, attenuates hyperoxia-induced acute inflammatory lung injury by alleviating the accumulation of HMGB1 in the airways and the circulation. Mol Med. (2020) 26:63. doi: 10.1186/s10020-020-00177-z
67. Wang H, Liao H, Ochani M, Justiniani M, Lin X, Yang L, et al. Cholinergic agonists inhibit HMGB1 release and improve survival in experimental sepsis. Nat Med. (2004) 10:1216–21. doi: 10.1038/nm1124
68. Pavlov VA, Ochani M, Yang LH, Gallowitsch-Puerta M, Ochani K, Lin X, et al. Selective α7-nicotinic acetylcholine receptor agonist GTS-21 improves survival in murine endotoxemia and severe sepsis. Crit Care Med. (2007) 35:1139–44. doi: 10.1097/01.CCM.0000259381.56526.96
69. Yang H, Liu H, Zeng Q, Imperato GH, Addorisio ME, Li J, et al. Inhibition of HMGB1/RAGE-mediated endocytosis by HMGB1 antagonist box A, anti-HMGB1 antibodies, and cholinergic agonists suppresses inflammation. Mol Med. (2019) 25:1–13. doi: 10.1186/s10020-019-0081-6
70. Lu B, Kwan K, Levine YA, Olofsson PS, Yang H, Li J, et al. α7 nicotinic acetylcholine receptor signaling inhibits inflammasome activation by preventing mitochondrial DNA release. Mol Med. (2014) 20:350–8. doi: 10.2119/molmed.2013.00117
71. Chen X, Zhao C, Zhang C, Li Q, Chen J, Cheng L, et al. Vagal-α7nAChR signaling promotes lung stem cells regeneration via fibroblast growth factor 10 during lung injury repair. Stem Cell Res Ther. (2020) 11:230. doi: 10.1186/s13287-020-01757-w
72. Farmer AD, Strzelczyk A, Finisguerra A, Gourine A v., Gharabaghi A, Hasan A, et al. International consensus based review and recommendations for minimum reporting standards in research on transcutaneous vagus nerve stimulation (Version 2020). Front Hum Neurosci. (2021) 14:568051. doi: 10.3389/fnhum.2020.568051
73. Azabou E, Bao G, Bounab R, Heming N, Annane D. Vagus nerve stimulation: a potential adjunct therapy for COVID-19. Front Med. (2021) 8:493. doi: 10.3389/fmed.2021.625836
74. Kazi MA, Roychowdhury S, Ghosh S, Mahapatra MK, Bhakta S, Konar MC, et al. Characteristics and predictors of outcomes of critically Ill children with SARS-CoV-2 infection - the PICU experience. J Pediatr. (2022). doi: 10.1016/j.jped.2021.12.006
75. Shekerdemian LS, Mahmood NR, Wolfe KK, Riggs BJ, Ross CE, McKiernan CA, et al. Characteristics and outcomes of children with coronavirus disease 2019 (COVID-19) infection admitted to US and canadian pediatric intensive care units. JAMA Pediatr. (2020) 174:868–73. doi: 10.1001/jamapediatrics.2020.1948
76. Merchant K, Zanos S, Datta-Chaudhuri T, Deutschman CS, Sethna CB. Transcutaneous auricular vagus nerve stimulation (taVNS) for the treatment of pediatric nephrotic syndrome: a pilot study. Bioelect Med. (2022) 8:1–8. doi: 10.1186/s42234-021-00084-6
77. Sourbron J, Klinkenberg S, Kessels A, Schelhaas HJ, Lagae L, Majoie M. Vagus nerve stimulation in children: a focus on intellectual disability. Eur J Paediatr Neurol. (2017) 21:427–40. doi: 10.1016/j.ejpn.2017.01.011
78. Ji T, Yang Z, Liu Q, Liao J, Yin F, Chen Y, et al. Vagus nerve stimulation for pediatric patients with intractable epilepsy between 3 and 6 years of age: Study protocol for a double-blind, randomized control trial. Trials. (2019) 20:1–10. doi: 10.1186/s13063-018-3087-4
79. Kovacic K, Hainsworth K, Sood M, Chelimsky G, Unteutsch R, Nugent M, et al. Neurostimulation for abdominal pain-related functional gastrointestinal disorders in adolescents: a randomised, double-blind, sham-controlled trial. Lancet Gastroenterol Hepatol. (2017) 2:727–37. doi: 10.1016/S2468-1253(17)30253-4
80. Hoffmann TJ, Simon BJ, Zhang Y, Emala CW. Low voltage vagal nerve stimulation reduces bronchoconstriction in guinea pigs through catecholamine release. Neuromodulation. (2012) 15:527. doi: 10.1111/j.1525-1403.2012.00454.x
81. Geng D, Liu X, Wang Y, Wang J. The effect of transcutaneous auricular vagus nerve stimulation on HRV in healthy young people. PLoS ONE. (2022) 17:e0263833. doi: 10.1371/journal.pone.0263833
82. Verbanck P, Am C, Burton F, Nagant C, Cheron G. Transcutaneous auricular Vagus Nerve Stimulation (tVNS) can reverse the manifestations of the long-COVID syndrome: a pilot study. Adv Neurol Neurosci Res. (2021) 2:100011. doi: 10.51956/ANNR.100011
83. Say D, Crawford N, McNab S, Wurzel D, Steer A, Tosif S. Post-acute COVID-19 outcomes in children with mild and asymptomatic disease. Lancet Child Adolesc Health. (2021) 5:e22–3. doi: 10.1016/S2352-4642(21)00124-3
84. Meroni E, Stakenborg N, Viola MF, Boeckxstaens GE. Intestinal macrophages and their interaction with the enteric nervous system in health and inflammatory bowel disease. Acta Physiol (Oxf). (2019) 225:e13163. doi: 10.1111/apha.13163
85. Fonseca RC, Bassi GS, Brito CC, Rosa LB, David BA, Araújo AM, et al. Vagus nerve regulates the phagocytic and secretory activity of resident macrophages in the liver. Brain Behav Immun. (2019) 81:444. doi: 10.1016/j.bbi.2019.06.041
86. Li S, Qi D, Ni LJ, Yu DX, Xin WD. Vagus nerve stimulation enhances the cholinergic anti-inflammatory pathway to reduce lung injury in acute respiratory distress syndrome via STAT3. Cell Death Discovery. (2021) 7:63. doi: 10.1038/s41420-021-00431-1
87. Floto RA, Smith KGC. The vagus nerve, macrophages, and nicotine. Lancet. (2003) 361:1069–70. doi: 10.1016/S0140-6736(03)12902-9
88. Chen Z, John Wherry E. T cell responses in patients with COVID-19. Nat Rev Immunol. (2020) 20:529–36. doi: 10.1038/s41577-020-0402-6
89. Blanco-Melo D, Nilsson-Payant BE, Liu WC, Uhl S, Hoagland D, Møller R, et al. Imbalanced Host Response to SARS-CoV-2 Drives Development of COVID-19. Cell. (2020) 181:1036. doi: 10.1016/j.cell.2020.04.026
90. Malmgaard L. Induction and regulation of IFNs during viral infections. J Interferon Cytokine Res. (2004) 24:439–54. doi: 10.1089/1079990041689665
91. Yin SW, Zhou Z, Wang JL, Deng YF, Jing H, Qiu Y. Viral loads, lymphocyte subsets and cytokines in asymptomatic, mildly and critical symptomatic patients with SARS-CoV-2 infection: a retrospective study. Virol J. (2021) 18:1–9. doi: 10.1186/s12985-021-01597-x
92. Soldatou A, Davies EG. Respiratory virus infections in the immunocompromised host. Paediatr Respir Rev. (2003) 4:193–204. doi: 10.1016/S1526-0542(03)00061-7
93. Upham JW, Lee PT, Holt BJ, Heaton T, Prescott SL, Sharp MJ, et al. Development of interleukin-12-producing capacity throughout childhood. Infect Immun. (2002) 70:6583. doi: 10.1128/IAI.70.12.6583-6588.2002
94. Maródi L. Innate cellular immune responses in newborns. Clin Immunol. (2006) 118:137–44. doi: 10.1016/j.clim.2005.10.012
95. Karimi K, Bienenstock J, Wang L, Forsythe P. The vagus nerve modulates CD4+ T cell activity. Brain Behav Immun. (2010) 24:316–23. doi: 10.1016/j.bbi.2009.10.016
96. Rosas-Ballina M, Olofsson PS, Ochani M, Valdés-Ferrer SI, Levine YA, Reardon C, et al. Acetylcholine-synthesizing T cells relay neural signals in a vagus nerve circuit. Science. (2011) 334:98. doi: 10.1126/science.1209985
97. Chen K, Kolls JK. T cell–mediated host immune defenses in the lung. Annu Rev Immunol. (2013) 31:605. doi: 10.1146/annurev-immunol-032712-100019
98. Kuri-Cervantes L, Pampena MB, Meng W, Rosenfeld AM, Ittner CAG, Weisman AR, et al. Comprehensive mapping of immune perturbations associated with severe COVID-19. Sci Immunol. (2020) 5:eabd7114. doi: 10.1126/sciimmunol.abd7114
99. Giamarellos-Bourboulis EJ, Netea MG, Rovina N, Akinosoglou K, Antoniadou A, Antonakos N, et al. Complex immune dysregulation in COVID-19 patients with severe respiratory failure. Cell Host Microbe. (2020) 27:992. doi: 10.1016/j.chom.2020.04.009
100. McClain MT, Park LP, Nicholson B, Veldman T, Zaas AK, Turner R, et al. Longitudinal analysis of leukocyte differentials in peripheral blood of patients with acute respiratory viral infections. J Clin Virol. (2013) 58:689–95. doi: 10.1016/j.jcv.2013.09.015
101. Zou ZY, Ren D, Chen RL, Yu BJ, Liu Y, Huang JJ, et al. Persistent lymphopenia after diagnosis of COVID-19 predicts acute respiratory distress syndrome: a retrospective cohort study. Eur J Inflam. (2021) 19:1–10. doi: 10.1177/20587392211036825
102. Liao M, Liu Y, Yuan J, Wen Y, Xu G, Zhao J, et al. Single-cell landscape of bronchoalveolar immune cells in patients with COVID-19. Nat Med. (2020) 26:842–4. doi: 10.1038/s41591-020-0901-9
103. Hilligan KL, Ronchese F. Antigen presentation by dendritic cells and their instruction of CD4+ T helper cell responses. Cell Mol Immunol. (2020) 17:587–99. doi: 10.1038/s41423-020-0465-0
104. Valdes-Ferrer S, Rosas-Ballina M, Olofsson P, Chavan S, Tracey K. Vagus nerve stimulation produces an anti-inflammatory monocyte phenotype in blood. J Immunol. (2010) 184:138.25. Available online at: https://www.jimmunol.org/content/184/1_Supplement/138.25/tab-article-info
105. Klein JO, Baker CJ, Remington JS, Wilson CB. Current concepts of infections of the fetus and newborn infant. Infect Dis Fetus Newborn Infant. (2006) 3−25. doi: 10.1016/B0-72-160537-0/50003-7
106. Wynn JL, Levy O. Role of innate host defenses in susceptibility to early-onset neonatal sepsis. Clin Perinatol. (2010) 37:307–37. doi: 10.1016/j.clp.2010.04.001
107. Cook PC, MacDonald AS. Dendritic cells in lung immunopathology. Semin Immunopathol. (2016) 38:449–60. doi: 10.1007/s00281-016-0571-3
108. Desch AN, Henson PM, Jakubzick C. v. pulmonary dendritic cell development and antigen acquisition. Immunol Res. (2013) 55:178–86. doi: 10.1007/s12026-012-8359-6
109. Gubernatorova EO, Gorshkova EA, Namakanova OA, Zvartsev R v., Hidalgo J, Drutskaya MS, et al. Non-redundant functions of IL-6 produced by macrophages and dendritic cells in allergic airway inflammation. Front Immunol. (2018) 9:2718. doi: 10.3389/fimmu.2018.02718
110. Pittet JF, Griffiths MJD, Geiser T, Kaminski N, Dalton SL, Huang X, et al. TGF-β is a critical mediator of acute lung injury. J Clin Investig. (2001) 107:1537. doi: 10.1172/JCI11963
111. Fitzgerald-Bocarsly P, Feng D. The role of type I interferon production by dendritic cells in host defense. Biochimie. (2007) 89:843. doi: 10.1016/j.biochi.2007.04.018
112. Ho Lim C, Ito M. Monocyte and dendritic cell defects in COVID-19. Nat Cell Biol. (2021) 23:445–7. doi: 10.1038/s41556-021-00685-y
113. Hadjadj J, Yatim N, Barnabei L, Corneau A, Boussier J, Smith N, et al. Impaired type I interferon activity and inflammatory responses in severe COVID-19 patients. Science. (2020) 369:718–24. doi: 10.1126/science.abc6027
114. Carvalho T, Krammer F, Iwasaki A. The first 12 months of COVID-19: a timeline of immunological insights. Nat Rev Immunol. (2021) 21:245–56. doi: 10.1038/s41577-021-00522-1
115. Upham JW, Rate A, Rowe J, Kusel M, Sly PD, Holt PG. Dendritic Cell immaturity during infancy restricts the capacity to express vaccine-specific T-cell memory. Infect Immun. (2006) 74:1106. doi: 10.1128/IAI.74.2.1106-1112.2006
116. Yang X, Zhao C, Gao Z, Su X. A novel regulator of lung inflammation and immunity: pulmonary parasympathetic inflammatory reflex. QJM. (2014) 107:789–92. doi: 10.1093/qjmed/hcu005
117. Andersson U. The cholinergic anti-inflammatory pathway alleviates acute lung injury. Mol Med. (2020) 26:1–4. doi: 10.1186/s10020-020-00184-0
118. Yang H, Wang H, Andersson U. Targeting Inflammation Driven by HMGB1. Front Immunol. (2020) 11:484. doi: 10.3389/fimmu.2020.00484
Keywords: pediatric, SARS-CoV-2, COVID-19, ALI, HMGB1
Citation: Jankauskaite L, Malinauskas M and Mickeviciute G-C (2022) HMGB1: A Potential Target of Nervus Vagus Stimulation in Pediatric SARS-CoV-2-Induced ALI/ARDS. Front. Pediatr. 10:884539. doi: 10.3389/fped.2022.884539
Received: 28 February 2022; Accepted: 11 April 2022;
Published: 11 May 2022.
Edited by:
Alenka Gagro, Children's Hospital Zagreb, CroatiaReviewed by:
Domenica Nathaly Cevallos Robalino, University of the Americas, EcuadorJosko Markic, University Hospital Split, Croatia
Copyright © 2022 Jankauskaite, Malinauskas and Mickeviciute. This is an open-access article distributed under the terms of the Creative Commons Attribution License (CC BY). The use, distribution or reproduction in other forums is permitted, provided the original author(s) and the copyright owner(s) are credited and that the original publication in this journal is cited, in accordance with accepted academic practice. No use, distribution or reproduction is permitted which does not comply with these terms.
*Correspondence: Lina Jankauskaite, TGluYS5KYW5rYXVza2FpdGVAbHNtdW5pLmx0