- 1Medicine and Surgery Department, University of Perugia, Perugia, Italy
- 2Allergy and Immunology Department, Rosario School of Medicine, National University of Rosario, Rosario, Argentina
- 3Health Sciences Institute, Autonomous University of Hidalgo State, Pachuca, Hidalgo, México
- 4Department of Pediatrics, Pulmonary and Allergy Disease Unit, G. Gaslini University Hospital, Genoa, Italy
- 5Department of Pediatric Pulmonology and Allergy, The Medical University Children's Hospital, Warszawa, Poland
- 6Department of Pediatrics, Unit of Pediatrics Pulmonology and Respiratory Endoscopy, G. Gaslini Hospital, Genoa, Italy
Respiratory tract infections (RTI) are mainly viral in origin and among the leading cause of childhood morbidity globally. Associated wheezing illness and asthma are still a clear unmet medical need. Despite the continuous progress in understanding the processes involved in their pathogenesis, preventive measures and treatments failed to demonstrate any significant disease-modifying effect. However, in the last decades it was understood that early-life exposure to microbes, may reduce the risk of infectious and allergic disorders, increasing the immune response efficacy. These results suggested that treatment with bacterial lysates (BLs) acting on gut microbiota, could promote a heterologous immunomodulation useful in the prevention of recurrent RTIs and of wheezing inception and persistence. This hypothesis has been supported by clinical and experimental studies showing the reduction of RTI frequency and severity in childhood after oral BL prophylaxis and elucidating the involved mechanisms. OM-85 is the product whose anti-viral effects have been most extensively studied in vitro, animal, and human cell studies and in translational animal infection/disease models. The results of the latter studies, describing the potential immune training-based activities of such BL, leading to the protection against respiratory viruses, will be reported. In response to human rhinovirus, influenza virus, respiratory syncytial virus and severe acute respiratory coronavirus-2, OM-85 was effective in modulating the structure and the functions of a large numbers of airways epithelial and immune cells, when administered both orally and intranasally.
Introduction
Respiratory tract infections (RTIs) are often associated with significant morbidity and, in childhood, represent one of the most common reasons of unscheduled medical visits and hospitalization (1, 2). Viruses are the predominant cause of such infections, but those of mixed etiology are not uncommon (3). However, RTIs often cause inappropriate antibiotic prescriptions, thus contributing to the development of antibiotic resistance, a major public health concern (3, 4). Infants and preschool children who experience severe respiratory virus infections, are at increased risk to develop airway hyperresponsiveness and eventually, loss of respiratory function later in life (5). Because of the paucity and not totally effective preventive measures and treatment, viral RTIs and their consequences such as wheezing illness and asthma, still represent an unmet medical need in children and other fragile populations (6–8). Over the last decades, attempts have been made to reduce the increased susceptibility to respiratory symptoms and morbidity, and epidemiological studies have demonstrated that living in a rural environment and some farming communities, may increase the efficiency of the immune responses against infectious pathogens and immune tolerance towards allergens (9–11). The evidence that early-life exposure to microbes, favoring the maturation and a healthy gut microbiota may have protective effects, has suggested that microbial-related products or metabolites such as prebiotics, probiotics, and bacterial lysates (BLs), could help prevent recurrent RTIs (rRTIs) and the onset of atopic disorders through “heterologous immunomodulation” of the body's natural defenses (9). These products may act on gut microbiota composition and indirectly exert immunomodulatory functions to provide an effective therapeutic option in patients with rRTIs (9–16). Among the several BLs available for clinical use, OM-85 is the product whose immunity training and anti-viral effects have been most extensively studied in in vitro animal and human cell studies and in translational animal infection/disease models (10, 17–19). The findings of the basic and applied studies designed to understand the biological mechanisms involved in those effects, will be summarized, and discussed in this review.
OM-85: composition and mode of action on immune effector cells not exposed to viruses
OM-85 comprises the lyophilised fractions of 21 bacterial strains of the following respiratory pathogens: Haemophilus influenzae, Streptococcus pneumoniae, Klebsiella pneumoniae subspecies pneumoniae and ozaenae, Staphylococcus aureus, Streptococcus pyogenes, Streptococcus sanguinis, and Moraxella catarrhalis (17). This drug, which possesses immune stimulating and regulating/anti-inflammatory properties, is indicated for the prevention of rRTIs in adults and children above 6 months of age (17–21). The rationale to use BLs in rRTIs prevention is based on the hypothesis of their ability to mimic the natural exposure to germs, promoting the maturation and the re-organization not only of the gut but also of the lung microbiota, and favoring the immune homeostasis, (i.e., the crosstalk between gut microbiota and immune system), in the airways via the gut-lung axis (17–19). The bacterial derived molecules of OM-85 act both, as pathogen-associated molecular patterns (PAMPs) on innate immunity and as antigens on adaptive immunity, which, reacting with pattern recognition receptors (PRRs) and other antigen receptor on immune effector and structural cells, activate both innate and adaptive immunity (19–21). The resulting heterologous mucosal immune responses regulate the anti-viral activities of dendritic cells (DCs), macrophages, CD4+ helper and CD8+ cytotoxic T-cells, regulatory T-cells, B-cells, natural killer (NK) cells against invading pathogens (19–22). DCs are major players in both innate and adaptive responses against viruses. Studies performed in vitro and ex vivo in experimental animals demonstrate that BLs can induce DC maturation, increasing the production of anti-viral cytokines/chemokines and the expression of surface molecules involved in antigen presentation (23–25). Through interaction with PRR receptors and activation of NF-kB and MAPK dependent pathways, in DCs cultures, OM-85 triggered the production of type I IFN-β, IL-8, CCL2, CCL20 and IFN-α, molecules involved in early immune reaction against viral infections, and of IL-12, a potent inducer of Th1 type lymphocytes (23, 25). After exposure to OM-85, a dose-dependent increase in the expression of MHC II, CD40, and CD86 surface proteins was also detected, molecules required for antigen presentation to T-cells (23). In blood monocyte and bone marrow-derived macrophage cultures, OM-85 increased IL-1, IL-2, and TNF-α release and oxygen radical production to kill TNF-α sensitive targets (26, 27). TNF-α is a cytokine which is produced by activated macrophages and possesses strong antiviral activity against human influenza viruses (IFV) (28). In mice, treatment with OM-85 induced a polyclonal activation of B-cells with the production of IFV- and Respiratory Syncytial Virus (RSV)-specific IgA and IgG in serum and bronchoalveolar lavage (BAL) of animals not previously exposed to these viruses (23). These virus-specific IgA and IgG were functionally active and significantly inhibited both IFV and RSV replication in vitro. Therefore, OM-85 could train innate immune cells which would be ready to cover broad heterologous protection against infections and, at the same time they could enhance the adaptive immune cells to mount a response following an infection.
Anti-viral activities of OM-85: in vitro and mice model results
Studies involving human epithelial cell cultures, i.e., primary bronchial epithelial cells (BECs) or epithelial cell lines, and human-relevant disease mice models were designed to evaluate the anti-viral activities of OM-85 against human rhinovirus (HRV), IFV, RSV, and Severe Acute Respiratory Syndrome-Coronavirus-2 (SARS-CoV-2).
HRV infections
HRV is an RNA virus known to be the etiologic agent of more than 50% of upper RTIs in humans worldwide. This virus is a leading cause of bronchiolitis in infants and the most common agent associated with wheezing illness in children and adults (29). Like all respiratory viruses, HRV recognized airway epithelial cells as the main target for infection. The primary function of the airway's epithelium is to act as a mechanical defensive barrier, aiding the maintenance of normal airway function. However, airway epithelial cells can also be actively involved in initiating and orchestrating immune and inflammatory responses through the expression of various receptors and effector proteins on their surface and the release of chemokines and cytokines, which recruit and activate inflammatory and immune effector cells (30). The intercellular adhesion molecule-1 (ICAM-1) is the major receptor for HRV-A and HRV-B, and the Cadherin related family member 3 (CDHR3) protein for HRV-C. Cell receptor binding is necessary or HRV to gain entry into the cells by endocytosis, whilst the interaction with Toll Like Receptor (TLR)2 on airway epithelial cell membranes and TLR3 and TLR7 in the endosomes and Melanoma Differentiation-Associated Protein 5 (MDA5) and retinoic acid-inducible gene I (RIG-I) in the cytoplasm modulates the RV-induced innate immune responses (30). In response to HRV infection, airway epithelial cells express chemokines such as CXCL-1, CXCL-5, and CXCL-8, growth factors, such as amphiregulin, epithelial cell growth factor (EGF) and epiregulin and a variety of Th2 cytokines.
OM-85 inhibited HRV infection in primary human BECs
To determine whether OM-85 could interfere with HRV infection, a study using primary human BECs of otherwise healthy controls, asthma, and COPD patients was performed (Figure 1A) (31). The preventive effect of OM-85 pre-incubation (10 μg/ml) on HRV infection of BEC and on became significant at 24 and 48 h, was detectable at similar levels in all three cell donor groups and was associated with an increased cell survival at day 1, 2 and 3 (Figure 1A). When evaluating the signaling pathways involved, it was shown that OM-85 induced the activation of Erk1/2 MAPK, significant at 30 and 60 min, preceded by the formation of intracellular cAMP, significantly within 15 min, which subsequently declined. OM-85 also significantly increased the expression of virus interacting proteins β-defensin and complement component C1q receptor, two cell surface molecules known to bind HRV and to help to kill the virus intracellularly (32, 33). In addition, it significantly reduced the HRV-induced ICAM-1 expression, the main adhesion site for both HRV type A and B (34), in BECs of asthma and COPD patients (Figure 1A). Inhibition of cAMP signaling, and Erk1/ 2 MAPK pathway activation prevented most of the anti-viral activities of OM-85 in human BECs, showing that this OBL acted through these three signaling routes. Finally, pre-incubation with OM-85 further induced the secretion of anti-viral cytokine interferon (IFN)-γ significantly by BECs of all three patient groups. It therefore appears that OM-85 can enhance BECs' capacity to directly counter viral infections, by engaging a variety of cell functions. The possibility that these effects could be translated in vivo, is an attractive hypothesis.
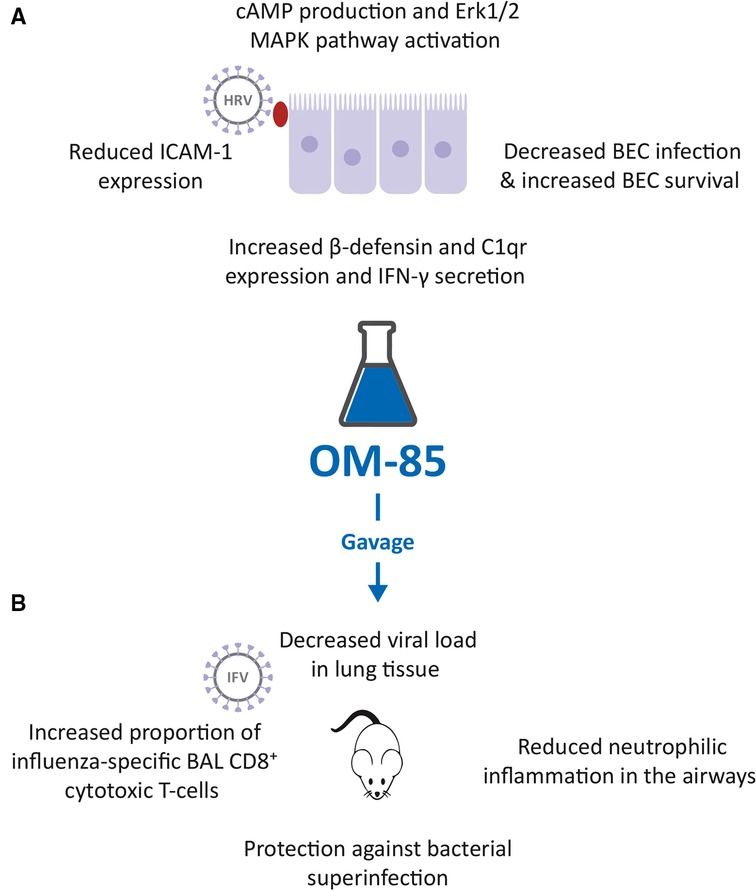
Figure 1. Inhibition of human rhinovirus (HRV) and influenza virus (IFV) infection by OM-85. (A). Effects of OM-85 on primary human bronchial epithelial cell (BEC) structural and functional changes, leading to decreased HRV infection and increased BEC survival. (B). Treatment of mice with OM-85 administered by gavage, decreased viral load in lung tissue, reduced neutrophilic inflammation in the airways, promoted the expansion of influenza-specific broncho alveolar lavage (BAL) fluid CD8+ cytotoxic T-cells and protected against bacterial superinfection, following IFV.
IFV infection
IFV is a major pathogen representing an ongoing health threat to several species, including humans. IFV infection, which is associated with a high risk of bacterial superinfection, is an important cause of respiratory morbidity and mortality in industrialized countries, despite the availability of anti-IFV specific vaccination (35). Upon infection with IFV the innate immunity plays a critical role in efficient and rapid control of viral infections, as well as in adaptive immunity initiation. The humoral immune system produces antibodies against different influenza antigens, whilst cell mediated immunity includes DC, CD4+ helper T cells and CD8 + cytotoxic T cells. Both humoral and cell mediated responses are the arms of adaptive immunity (35).
OM-85 counteracted IFV infection in mice and bacterial superinfection
The possible OM-85 “anti-viral” activities against IFV infections, shown by the in vitro study (23), were confirmed in a mouse model. In mice, infected with sub-lethal doses of IFV-A, prior administration by gavage of 7.2 mg of OM-85-active principle for 10 days, induced a highly significant decrease in lung tissue viral load, observed at day 5 post infection (Figure 1B) (23, 36). At the same study time, a reduction of neutrophilic inflammation into the airways was detected in treated mice, because of the early control of the viral infection. This reduced neutrophilia was associated with an increase proportion of influenza nucleoprotein (NP)-tetramer+ specific CD8+ cytotoxic T-cell proportions in BAL fluid at day 10 post infection, indicating the activation of the adaptive immune response (23, 36). In the same experimental model, mice were exposed to a sublethal dose of either Klebsiella pneumoniae or Streptococcus pneumoniae, on day 7 following influenza infection (23, 36). Pre-treatment with OM-85 also protected mice from bacteremia, at 24 h post infection, and significantly reduced all morbidity signs, i.e., weight loss, body temperature increase, and disease score, at day 12 post infection (Figure 1B). Thus, the anti-influenza-specific activation of the immune responses induced by OM-85 pre-treatment, was also associated with an enhancement of some specific anti-bacterial responses. These findings support the concept of boosting anti-viral immunity, to increase alertness and clearance efficacy against secondary bacterial infections.
RSV infection
RSV is a seasonal pathogen responsible for the highest percentage of severe bronchiolitis in pediatric patients but is also a major viral pathogen causing severe lung disease and exacerbations in the adult population, particularly among the elderly (37). The TLR4/CD14 complex is the main extracellular receptor recognizing RSV through binding with the F protein, while intracytoplasmic RIG-I, MDA5, and TLR3 PPRs recognize RSV transcripts and viral replication intermediates (29). The viral antigen recognition leads to nuclear factor-κB activation with production IFN-β, a type-I IFN, which initiates the production of IFN-α, CXCL8, IFN-λ and TNF-α, by airway epithelial cells and innate immune cells (29). These cytokines recruit and activate polymorphonuclear leukocytes and natural killer cells and also trigger airway epithelial cells programmed death, a mechanism limiting viral spread to neighboring cells (29). Airway dendritic cells carry viral antigens to regional lymph nodes and where they activate CD4+ T-cells, CD8 + cytotoxic T-cells and B-lymphocytes which migrate back to the infected airways to kill the virus (29).
Reduction of RSV-induced viral load, weight loss, and lung inflammation in mice
With the background that an intranasal (IN) administration of microbiota-derived acetate was protective against RSV (38), and that OM-85 effectively inhibited HRV infection of BEC (31), a study was designed to investigate the potential protective effect of IN administration of OM-85 in RSV infected mice (39). IN OM-85 treatment (1 mg diluted in 20 ml/nostril of saline solution) was delivered to specific pathogen-free (SPF) BALB/c mice following four different schedules: (i) starting 12 h after infection and given daily throughout 5 days post infection or (ii) starting 1 day, (iii) or 3 days or (iv) 5 days prior to IN infection with RSV strain A2. OM-85 treatment administered 12 h after infection did not modify outcomes of established RSV infection i.e., lung viral load, weight loss and airway inflammation. OM-85 IN treatment given 1 day prior to infection and, on the same day, 6 h later, protected against weight loss on day 2 post-infection, maintained the animal weight slightly above compared to controls, but did not protect from lung perivascular and peri bronchial inflammation, despite a trend towards decrease in viral load. OM-85 IN treatment given 3 days prior to infection protected against weight loss at days 2 and 3 post-infection, significantly reduced viral load, decreased perivascular and peri bronchial inflammation, and reduced IL-4 production in the lung. Finally, OM-85 IN treatment given 5 days prior to infection reduced weight loss, cellular infiltrates into the lungs and viral expression and replication in the airways, peri-bronchial and perivascular inflammation scores (Figure 2A). Reduction in inflammatory cells and structural damages in the lungs, significantly prevented the production of IL-4 and TNF-α, while increasing the synthesis of the anti-inflammatory cytokine IL-10 (39).
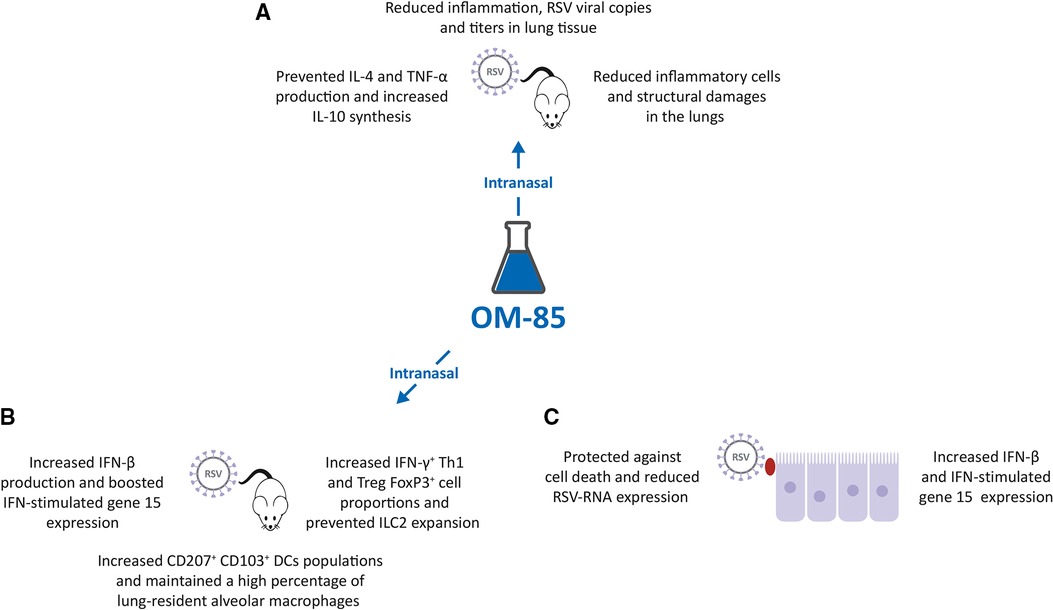
Figure 2. Inhibition of respiratory syncytial virus (RSV) infection by OM-85. (A,B). Intranasal (IN) treatment of Specific Pathogen Free (SPF) BALB/c mice with OM-85 reduced RSV infection in lung tissue promoting an effective innate and adaptive immune response. (C). Exposure of human airway cell line cultures to OM-85 protected against cell death and reduced RSV-RNA expression by increasing human interferon cytokine (IFN)-β and IFN-stimulated gene 15 expression.
Modulation of the lung immune response against RSV infection in mice
In these RSV-infected mice, IN treatment given 5 days prior to infection also significantly increased the proportion of the CD4+ IFN-γ+ Th1 cell and FoxP3+ Treg cell populations in the lung and prevented the RSV-induced increase of the innate lymphoid cell 2 (ILC2) proportions, cells known to be associated with Th2 type of immune response and with more severe RSV-bronchiolitis (Figure 2B) (39, 40). Moreover, the populations of tolerogenic CD207+ CD103+ DCs was increased in OM-85 pretreated RSV-infected mice, and in non-infected mice, thus suggesting a reprogramming of these DCs to promote less Th2 cell subtype activation (41). Finally, OM-85 treatment maintained a high percentage of lung-resident alveolar macrophages (AM), cells known to play an essential role in controlling RSV infection (42), increased the lung expression and production of the immunomodulator and IFN-β, and did boost the expression of the IFN-stimulated gene 15 (ISG15) (43–45). Finally, experiments were performed in vitro with human airway epithelial cells, a preeminent natural RSV target and important IFN-β producers (39). Pre-treated with OM-85 (7.5 mg/ml) protected A549 cell line cultures against cell death, reduced RSV-RNA expression in those cells, and increased IFN-β and ISG15 expression (Figure 2C). The protective role of OM-85 was abolished entirely in the absence of genes related to the type I IFN response, i.e., when A549 cells lacking the type I response pathway (IFNAR and RIG-I knockout cells) were used. Therefore, the broad protective effect of OM-85 against RSV infection is at least partially mediated through the modulation of the INF response.
SARS-CoV-2 infection
COVID-19, the syndrome induced by SARS-CoV-2, is characterized by a wide spectrum of clinical manifestations ranging from a mild, self-limiting flu-like respiratory illness to life-threatening multiorgan failure and death (46). The severity of the clinical manifestation of SARS-CoV-2 infection is related to the viral virulence and load, but also to efficiency of the innate and adaptive immune response (47). The experimental results of recent publications from three independent groups suggest thar OM-85 could inhibit SARS-CoV-2 and a murine coronavirus infection and promote early innate immune training against the virus.
Downregulation of SARS-CoV-2 ACE2 receptor and TMPRSS2 transcription and expression
SARS-CoV-2 targets human cells through the receptor-binding domain of the structural Spike (S) protein specifically recognizing angiotensin-converting enzyme type 2 (ACE2) receptor protein (46, 47). Following binding to ACE2 receptor, proteolytic cleavage between the S1 and S2 subunits by the transmembrane protease serine subtype 2 (TMPRSS2) is required to facilitate the fusion of the viral and the host cellular membranes and for viral entry (46, 47). Studies in mice, cells derived from SARS-CoV-2 target organs and human epithelial cells, have demonstrated that exposure to OM-85 effectively inhibits the transcription of ACE2 and TMPRSS2 proteins (Figure 3A). In mice, treated intranasally with OM-85 (1 mg/treatment for 14 treatments, over 32 days) ACE2 and TMPRSS2 transcription was significantly inhibited at day 2, and day 2 and 7 respectively, after the last OM-85 treatment (48). Since OM-85 ability to modulate the functions of dendritic cells, depends on Myd88/Trif innate immune signaling (25), this pathway involvement was assessed in wild-type and Myd88−/−Trif−/− C57BL/6 mice. As few as four IN OM-85 treatments were sufficient to reduce by more than half ACE2 and TMPRSS2 transcription in cells isolated from the lungs of wild-type mice, whilst negligible changes were detected in Myd88−/−Trif−/− mice. Also, in Vero E6 cell, Calu-3 cell and Caco-2 cell line cultures, OM-85 (0.48 mg/ml) significantly inhibited ACE2 and TMPRSS2 transcription (48). These results were validated in human BEC lines (BEAS-2B and Nuli) and on primary human BECs, isolated from a healthy individual (49). After exposure for 24 and 48 h to OM-85 (1:50 dilution, in phosphate-buffered saline (PBS), significant inhibition in ACE2 and TMPRSS2 transcription was detected. In kinetic studies, reduction in ACE2 protein expression was shown at all time points (24,48,72 and 96 h), while the downregulating effects on TMPRSS2 protein expression became significant only at day 4 (49).
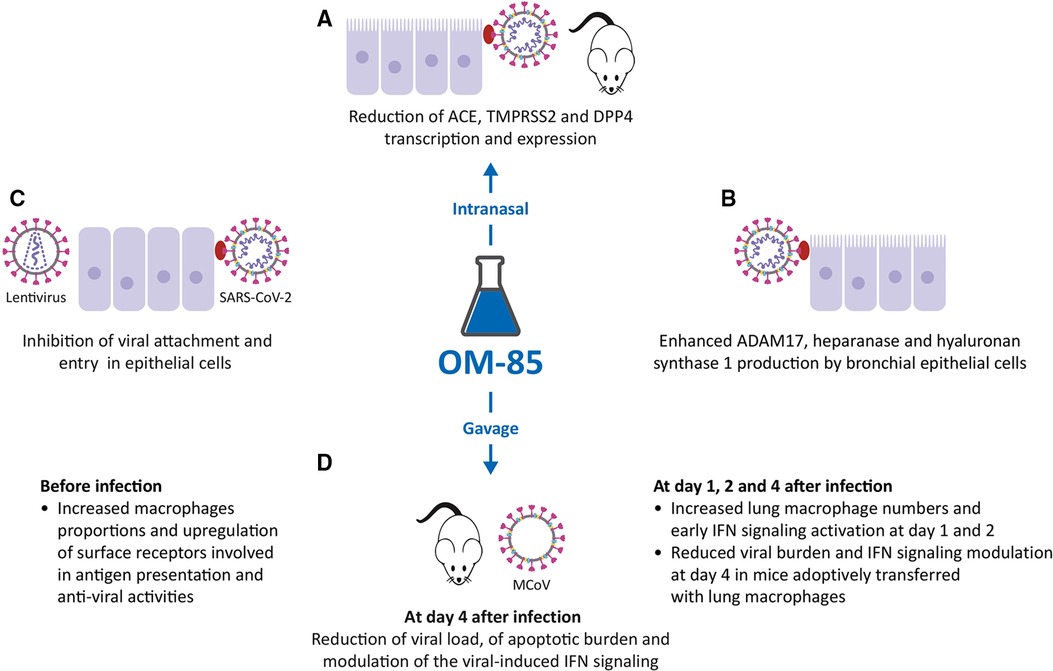
Figure 3. Inhibitory activity of OM-85 on coronavirus infections. (A). OM-85-induced inhibition of the transcription of ACE2, TMPRSS2 and DPP4, all proteins involved in Severe Acute Respiratory Syndrome-Coronavirus-2 (SARS-CoV-2) adhesion and infection in mice treated intranasally, and in epithelial cell cultures. (B). Increased synthesis of ADAM17, heparanase and hyaluronan synthase 1 by BECs. (C). Inhibition of SARS-CoV-2–pseudotyped particles, SARS-CoV-2 attachment, and entry in epithelial cells. (D). Effects of OM-85 administered by gavage in SPF mice: increase in macrophage proportions and early IFN signaling activation, reduction of viral load and apoptotic burden, modulation of the viral-induced IFN signaling leading to subsequent reduction of viral load.
Modulation of the expression of other BEC surface proteins involved in SARS-CoV-2 infection
In addition to ACE2 and TMPRSS2, other molecules expressed on the epithelial cell membrane are involved in SARS-CoV-2 infection. Therefore, the modulation of DPP4, ADAM17, heparanase and hyaluronan synthase 1 (Has-1) expression was evaluated, again in BEAS-2B, Nuli BEC lines, and primary human BECs (Figure 3B) (49). DPP4 is a serine exopeptidase expressed in several tissues, which can act as functional receptor by SARS-CoV-2 (50, 51) in experiments performed again in BEAS-2B and Nuli, and primary human BECs, daily treatment with OM-85 (1:50) significantly reduced the levels of DPP4 mRNA and DPP4 protein (i.e., transcription and expression) (49). In contrast with what was observed for ACE2, TMPRSS2 and DPP4, daily treatment with OM-85 significantly increased ADAM17 mRNA and protein levels at all time points (49). ADAM17 (a disintegrin and metalloproteinase domain 17) is engaged in the cleavage and release of ACE2 (52) and its upregulation was associate with an increase extracellular release of ACE2 in its soluble form (sACE2). Circulating sACE2 can bind to the SARS-CoV-2 virus effectively, acting as soluble decoy and blocking attachment to the membrane-bound receptor and virus entry (53, 54). An increase in heparanase secretion and of Has-1, was also demonstrated after OM-85 treatment (respectively at 1:50, and 1:100 or at 1:50 dilutions) (49). Heparanase is an enzyme that degrades polymeric heparan sulfate (HS) produced by epithelial cells (55). Expressed on the cell surface, HS serves as receptor for SARS-CoV-2 and facilitates the interaction between the S protein and its homing receptor ACE2 (56, 57). The upregulation of heparanase secretion was associated with a decrease of HS expression on the cell membrane, i.e., with a decrease of the SARS-CoV-2 binding sites availability. Finally, the increased expression of Has-1 in the presence of OM-85 was associated with an upregulation of the synthesis and secretion of hyaluronic acid (HA). HA is a non-sulphated glycosaminoglycan distributed throughout connective, epithelial and neural tissues (58). Being one of the chief components of the extracellular matrix, HA participates in a variety of processes, including the response to bacterial, fungal, and viral infections (59) and its antiviral and virucidal activity shown in vitro could be useful in fighting SARS-CoV-2 (60, 61).
Inhibition of virus attachment and cell entry
To investigate whether OM-85 could reduce SARS-CoV-2 S protein attachment to host cells, a recombinant histidine (His)-tagged S1 subunit comprising the SARS-CoV-2 receptor binding domain, was firstly used (48). Incubation with OM-85 (0.48 or 1.92 mg/ml) induced a dose-dependently significant reduction in S1 binding to Vero E6 and Calu-3 cells, but also to HEK293T stably transfected with human ACE2 (ACE2/HEK293T) (48) (Figure 3C). Moreover, OM-85 pretreatment (0.48 mg/ml) strongly inhibited the transduction by SARS-CoV-2–pseudotyped particles in Vero E6 cell but not in ACE2/HEK293T cells, because these ones do not downregulate ACE2 on OM-85 stimulation (48, 49). Therefore, the key event characterizing the OM-85–induced suppression of SARS-CoV-2 infection is the reduction of ACE2 expression. Finally, OM-85 (0.24–1.92 mg/ml) also suppressed Vero cells and Calu-3 cells infection by live SARS-CoV-2 (isolate USA-WA1/ 2020) (48).
Murine coronavirus (MCoV) infection
To study the cellular processes and the beneficial effects of OM-85 on viral infection and clearance as well as disease outcome a murine coronavirus (MCoV) model was designed by Salzmann M, and coworkers (62).
Promotion of lung macrophage anti-viral functions, reduction of viral and apoptotic burden and modulation of IFN signaling on days 4 and 10 after MCoV infection
In SPF mice receiving 7.2 mg OM-85 for 10 consecutive days by gavage before MCoV infection, a massive reduction of viral load was associated with enhanced viral clearance and downregulation of the apoptotic burden in lung tissue on days 4 and 10 (Figure 3D) (62). Strong activation of genes specifically linked to type 1 IFN signaling and innate immunity was observed but in OM-85-treated animals, the reduced viral stress starting at day 4, was associated with a significant downregulation of IFN-α, IFN-β, IFN-γ, and induced protein with tetratricopeptide repeats 1 (IFIT1) mRNA levels in lung tissue (62). RNA sequencing also indicated differences in antigen processing and presentation, and macrophage activation between treated and untreated mice. Pre-activation of the immune system by OM-85 did not magnify MCoV-induced injury because no adverse effects on lung pathology were detectable-.
Early increase in lung macrophage numbers, early IFN signaling activation before and reduced viral burden
Increase in CD11c positive interstitial lung macrophages before virus infection was shown, still retained 2 days after virus infection and associated with overexpression of mRNA levels of genes related to antigen presentation (MHC-II and CD86) and to C1q receptor already before exposure to the virus (62). In the first 2 days of the infection early interferon signaling was present, leading to increased IRF1, IFN-α and IFN-β expression in lung tissue and, at day 1, a significant increase was detected of the expression of the retinoic acid-inducible gene 1 (RIG-1), an intracellular receptor involved in the identification of RNA viruses (63). Moreover, mice receiving a transfer of monocytes and macrophages isolated from naive lung tissues before MCoV infection, showed, at day 4, a reduction in lung viral burden and in IFN-α, IFN-β and IFIT1 mRNA levels, effects like those induced by OM-85 treatment. Finally, early inhibition of IFN signaling with anti-IFNAR1 antibodies during MCoV infection, after OM-85 treatment, increased viral loads and persistent activation of IFN-related pathways at day 4 post-infection. Therefore OM-85 induced an early “innate immunity training”, leading to lung macrophage pre-activation, to enhanced and faster activation of IFN pathway and its downstream targets allowing for a faster viral clearance together with reduced tissue damage (62).
Discussion
Many studies and several reviews and meta-analyses have shown the clinical efficacy of OM-85 in the prevention of rRTIs in children (64–68), and in vulnerable adult populations (69–73, 74). More specifically, following oral administration of OM-85, reduction of infection incidence, duration, and severity as well as decreased antibiotic and drug courses have been reported in randomized clinical trials. In addition, this product has shown to reduce the risk for complications in children such as recurrent infectious wheezing episodes or asthma exacerbations (75). In adults with other chronic respiratory disease (e.g., chronic bronchitis, and COPD), OM-85 reduced the exacerbations triggered by respiratory infections (69, 74).
The underlying mechanisms for the potential immune training-based effects leading to protection against severe lower respiratory tract infections, are only partially understood. However, interesting new data came from a recent placebo-controlled trial in infants, showing that the OM-85-induced reduction in respiratory infection frequency and/or duration, was associated with immune changes indicative of innate immune training (76). Similar changes and effects have been described in the animal model studies included in the present review (39, 62). In the study by Troy and colleagues, human peripheral blood mononuclear cells obtained from infants, were exposed ex-vivo to OM-85 (or placebo) and then stimulated with the bacteria mimicking lipopolysaccharide (LPS) (Figure 4) (76). In cultures exposed to OM-85, upregulation of IFN signaling, was accompanied by, (i) network rewiring resulting in increased coordination of TLR4 expression with IFN pathway–associated genes (especially master regulator IRF7); ii) segregation of TNF and IFN-γ (which potentially synergize to exaggerate inflammatory sequelae) into separate expression modules; iii) reduced size/complexity of the main proinflammatory network module, containing, IL-1, IL-6, and CCL3. Finally, a reduced capacity for LPS-induced inflammatory cytokine IL-6 and TNF production in the OM-85 group was observed (76). Thus, immune training was associated with and attenuation of potentially pathogenic inflammation. The rationale behind the use of the oral route to administer immunomodulators for the prevention of respiratory conditions centers on the concept of the gut-lung immune axis (15–18). To modulate the inflammatory and immune-mediated responses, gut cells assimilate information directly from the local microorganisms and from the metabolites (e.g., short chain fatty acids) and cytokines they release. This modulation shapes immune responses locally but also at distal organs. Bacterial-derived products (PAMPs/antigens) and metabolites are processed by the gut DCs, which promote T cells and immunoglobulin-producing B cells differentiation and proliferation. Following immune challenges in the respiratory tract these cells, can move into the bloodstream, and traffic from the gut- associated lymphoid tissue (GALT) to the bronchial-associated lymphoid tissue (BALT) and to the airways, where they promote and modulate protective and anti-inflammatory responses (14, 15–18). BLs, such as OM-85, can promote immune homeostasis and anti-viral responses in patients with respiratory infections potentially acting on gut microbiota (77). Consistently, three studies summarized in this review showed the efficacy against IFV and MCoV infection of OM-85 administered to mice by gavage (23, 36, 62). In the first two studies, following administration of 7.2 mg of OM-85-active principle, for 10 days, an increase of influenza-specific NP-tetramer+ CD8+ cytotoxic T-cell proportions in BAL fluid were associated with a reduced viral replication and a faster viral clearance (23, 36). In the third study, a murine coronavirus (MCoV) model, orally gavage before viral infection with 7.2 mg OM-85 for 10 consecutive days, increased macrophage proportions and upregulated the expression of surface receptors involved in antigen presentation and anti-viral activities. An early IFN signaling activation was associated with the reduction of viral load and with modulation of a strong viral-induced IFN signaling in the “early convalescent” phase (62). In addition, four other studies reported the direct activity of low concentrations of OM-85 on airway epithelial cells (31, 39, 48, 49). In the first study, pre-incubation with OM-85 (10 μg/ml) of primary human BEC cultures infected with HRV, increased the expression of the two anti-viral proteins β-defensin and C1qR and the secretion of IFN-γ (31) and, at the same time, downregulated the expression of the ICAM-1. Significant inhibition of HRV replication through the cAMP and Erk1/2 MAP kinase pathway activation was detected (31). In the second study, pre-treatment with OM-85 (7.5 µg/ml) of human pulmonary epithelial cell cultures infected with RSV, increased IFN-β and ISG15 expression, decreased cell death and reduced RSV-RNA expression (39). In the third and fourth studies, preincubation of human bronchial epithelial cell lines with OM-85 (0.12, 0.24, 0.48, 0.96, or 1.92 mg/ml) modulated the expression of molecules involved in SARS-CoV-2 infection and inhibited virus attachment and cell entry (48, 49). The possibility that these direct effects on airway cells could be translated in vivo was addressed in the two studies that evaluated the efficacy of IN administration of OM-85 in mice infected with RSV and SARS-CoV-2 (39, 48). In the first study, IN administration of OM-85 (1 mg/treatment) for 5 days prior to RSV infection in BALB/c mice inhibited viral replication and reduced perivascular and peri bronchial inflammation in the lungs (39). The reduced viral load was associated with increased proportions of alveolar macrophages and a selective set of tolerogenic DCs, and with the expansion of Treg and Th1 lymphocyte expansion in the lung, contributing to a better Th1/Th2 lymphocyte balance and preventing ILC2 recruitment in the airways. The antiviral response was improved by the increased expression of IFN-β and of ISG15 in the lung. In the second study, IN treatment of mice with OM-85 (1 mg/treatment for 14 treatments, over 32 days) significantly inhibited ACE2 and TMPRSS2 transcription, at day 2 and at day 2 and 7 after the last OM-85 treatment, an activity that was Myd88/Trif innate immune signaling-dependent (48). Interestingly, a similar protocol involving OM-85 IN administration was used in mice asthma models (41). OM-85 administration (1 mg/treatment every 2 to 3 days, 14 times total), suppressed allergic asthma in different strains of mice, sensitized and challenged with ovalbumin or Alternaria. Among the multiple components of the innate and adaptive immune response targeted by OM-85 in that asthma study, preeminent was the epithelium/IL-33/ILC2 axis that is known to initiate type 2 inflammation in the airway mucosa also in RSV infection (78), and the CD103+CDs, whose Myd88/ Trif-dependent tolerogenic reprogramming was sufficient to transfer OM-85-induced asthma protection (41). Similarly, also in the RSV study the tolerogenic CD103+ DC population was significantly increased in OM-85 and in OM-85 + RSV-infected mice, and their expansion was and associated with the expansion of FoxP3+ Treg cells and Th1 lymphocytes in the lung, contributing to a better Th1/Th2 balance and to a prevention ILC2 recruitment in the airways (39). In an asthmatic mice model, established with OVA challenge, OM-85 increased the lung FoxP3+ Treg cell numbers, reduced the BAL eosinophil numbers, and downregulated both the IgE and Th2 pro-inflammatory cytokine production (79). Studies in children with rRTI have shown that OM–85 promotes immune system maturation via downregulation of Th2 lymphocyte activity, through the activation of FoxP3+ Treg cells, which are important for the immune response regulation and for viral clearance (17). Notably, in the two studies, OM-85 administration through the IN route resulted into significant inhibition of RSV replication and an almost complete asthma suppression at cumulative doses several fold lower than those reportedly used through the oral route.
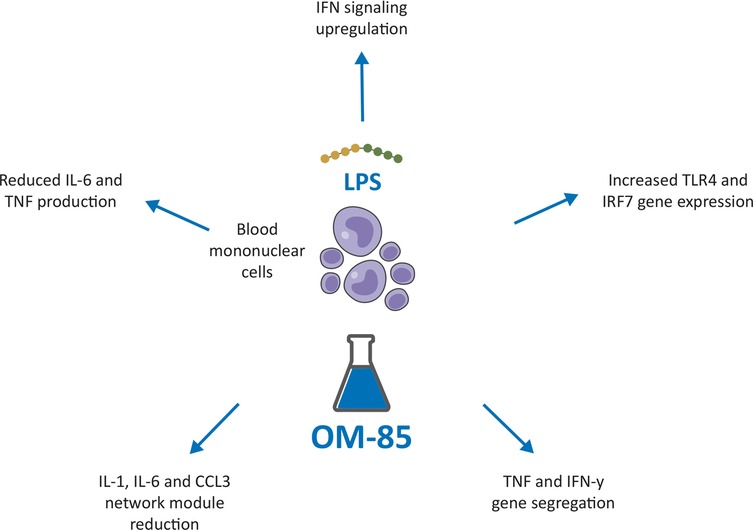
Figure 4. Potential immune training-based effects induced by OM-85 leading to protection against severe lower respiratory tract infections in children. LPS, Lipopolysaccaride; TLR 4, Toll Like Receptor; IRF1, Interferon Regulatory Factor 1.
Conclusions
As shown in pre-clinical research, OM-85 can promote an effective anti-viral activity, by modulating the structure and the functions of a large numbers of airways epithelial and immune cells, when administered orally, but also intranasally, as shown in several mice experiments. In addition, in line with the hygiene hypothesis and innate trained immunity concepts, it has been observed that oral bacterial-derived products might mimic the microbiota functions. There is some preliminary evidence that the product might also stabilize or rearrange the microbiota to promote a balanced immune state and respiratory health, but more data is needed. The positive effect of IN-administered OM-85 in regulating the response to infections and in asthma prevention, may reflect both the microbial stimulation that the BL provides at epithelial layer level and its direct access to the cellular and molecular networks that reside in the airway mucosa. The results of these studies which demonstrate that OM-85 can directly enhance anti-viral capacity through the engagement of a variety of cells, suggest that the administration of OM-85 in the airways could be a valuable option in clinical practice, a hypothesis that needs to be confirmed by future research and clinical trials.
Author contributions
SB: Literature research, manuscript drafting, review and approval; LA: Manuscript review and approval; JAOM: Manuscript review and approval; OS: Manuscript review and approval; WF: Manuscript review and approval; GAR: Literature research, manuscript drafting, review and approval, and figures design. All authors contributed to the article and approved the submitted version.
Conflict of interest
The authors declare that the research was conducted in the absence of any commercial or financial relationships that could be construed as a potential conflict of interest.
Publisher's note
All claims expressed in this article are solely those of the authors and do not necessarily represent those of their affiliated organizations, or those of the publisher, the editors and the reviewers. Any product that may be evaluated in this article, or claim that may be made by its manufacturer, is not guaranteed or endorsed by the publisher.
References
1. Grifn MR, Walker FJ, Iwane MK, Weinberg GA, Staat MA, Erdman DD. Epidemiology of respiratory infections in young children. Pediatr Infect Dis J. (2004) 23:188–92. doi: 10.1097/01.inf.0000144660.53024.64
2. Feldman C, Shaddock E. Epidemiology of lower respiratory tract infections in adults. Expert Rev Respir Med. (2019) 13:63–77. doi: 10.1080/17476348.2019.1555040
3. Brealey JC, Sly PD, Young PR, Chappell KJ. Viral bacterial co-infection of the respiratory tract during early childhood. FEMS Microbiol Lett. (2015) 362:fnv062. doi: 10.1093/femsle/fnv062
4. Shaver AL, Jacobs DM, LaMonte MJ, Noyes K. Antibiotic prescribing for acute respiratory tract infections in the United States outpatient setting. BMC Fam Pract. (2019) 20:91. doi: 10.1186/s12875-019-0980-1
5. Martinez FD. The connection between early life wheezing and subsequent asthma: the viral march. Allergol Immunopathol (Madr). (2009) 37:249–51. doi: 10.1016/j.aller.2009.06.008
6. Savitha MR, Nandeeshwara SB, Pradeep Kumar MJ, ul-Haque F, Raju CK. Modifiable risk factors for acute lower respiratory tract infections. Indian J Pediatr. (2007) 74:477–82. doi: 10.1007/s12098-007-0081-3
7. Frickmann H, Jungblut S, Hirche TO, Groß U, Kuhns M, Zautner AE. The influence of virus infections on the course of COPD. Eur J Microbiol Immunol (Bp). (2012) 2:176–85. doi: 10.1556/EuJMI.2.2012.3.2
8. Stokes JR, Bacharier LB. Prevention and treatment of recurrent viral-induced wheezing in the preschool child. Ann Allergy Asthma Immunol. (2020) 125:156–62. doi: 10.1016/j.anai.2020.05.018
9. Le Souëf P. Viral infections in wheezing disorders. Eur Respir Rev. (2018) 27:170133. doi: 10.1183/16000617.0133-2017
10. Feleszko W, Ruszczynski M, Zalewski BM. Non-specific immune stimulation in respiratory tract infections. Separating the wheat from the chaff. Paediatr Respir Rev. (2014) 15:200–6. doi: 10.1016/j.prrv.2013.10.006
11. Vercelli D. Mechanisms of the hygiene hypothesis–molecular and otherwise. Curr Opin Immunol. (2006) 18:733–7. doi: 10.1016/j.coi.2006.09.002
12. Man WH, de Steenhuijsen Piters WA, Bogaert D. The microbiota of the respiratory tract: gatekeeper to respiratory health. Nat Rev Microbiol. (2017) 15:259–70. doi: 10.1038/nrmicro.2017.14
13. Braido F, Tarantini F, Ghiglione V, Melioli G, Canonica GW. Bacterial lysate in the prevention of acute exacerbation of COPD and in respiratory recurrent infections. Int J Chron Obstruct Pulmon Dis. (2007) 2:335–45. PMID: 18229572; PMCID: PMC269519118229572
14. Dumas A, Bernard L, Poquet Y, Lugo-Villarino G, Neyrolles O. The role of the lung microbiota and the gut-lung axis in respiratory infectious diseases. Cell Microbiol. (2018) 20:e12966. doi: 10.1111/cmi.12966
15. Sencio V, Machado MG, Trottein F. The lung-gut axis during viral respiratory infections: the impact of gut dysbiosis on secondary disease outcomes. Mucosal Immunol. (2021) 14:296–304. doi: 10.1038/s41385-020-00361-8
16. Tsai YL, Lin TL, Chang CJ, Wu TR, Lai WF, Lu CC, et al. Probiotics, prebiotics, and amelioration of diseases. J Biomed Sci. (2019) 26:3. doi: 10.1186/s12929-018-0493-6
17. Esposito S, Soto-Martinez ME, Feleszko W, Jones MH, Shen KL, Schaad UB. Nonspecifc immunomodulators for recurrent respiratory tract infections, wheezing and asthma in children: a systematic review of mechanistic and clinical evidence. Curr Opin Allergy Clin Immunol. (2018) 18:198–209. doi: 10.1097/ACI.0000000000000433
18. Rossi GA, Pohunek P, Feleszko W, Ballarini S, Colin AA. Viral infections and wheezing-asthma inception in childhood: is there a role for immunomodulation by oral bacterial lysates? Clin Transl Allergy. (2020) 10:17. doi: 10.1186/s13601-020-00322-1
19. Esposito S, Jones MH, Feleszko W, Martell JAO, Falup-Pecurariu O, Geppe N, et al. Prevention of new respiratory episodes in children with recurrent respiratory infections: an expert consensus statement. Microorganisms. (2020) 8:1810. doi: 10.3390/microorganisms8111810
20. Manolova V, Flace A, Jeandet P, Bessler WC, Pasquali C. Biomarkers induced by the immunomodulatory bacterial extract OM-85: unique roles for Peyer's Patches and intestinal epithelial cells. J Clin Cell Immunol. (2017) 8:494. doi: 10.4172/2155-9899.1000494
21. Mauël J. Stimulation of immunoprotective mechanisms by OM-85 BV. A review of results from in vivo and in vitro studies. Respiration. (1994) 61(Suppl 1):8–15. doi: 10.1159/000196372
22. Ballarini S, Rossi GA, Principi N, Esposito S. Dysbiosis in pediatrics is associated with respiratory infections: is there a place for bacterial-derived products? Microorganisms. (2021) 9:448. doi: 10.3390/microorganisms9020448
23. Pasquali C, Salami O, Taneja M, Gollwitzer ES, Trompette A, Pattaroni C, et al. Enhanced mucosal antibody production and protection against respiratory infections following an orally administered bacterial extract. Front Med (Lausanne). (2014) 1:41. doi: 10.3389/fmed.2014.00041
24. Parola C, Salogni L, Vaira X, Scutera S, Somma P, Salvi V, et al. Selective activation of human dendritic cells by OM-85 through a NF-kB and MAPK dependent pathway. PLoS ONE. (2013) 8:e82867. doi: 10.1371/journal.pone.0082867
25. Dang AT, Pasquali C, Ludigs K, Guarda G. OM-85 is an immunomodulator of interferon-β production and inflammasome activity. Sci Rep. (2017) 7:43844. doi: 10.1038/srep43844
26. Mauël J. Macrophage activation by OM-85 BV. Respiration. (1992) 59(Suppl 3):14–8. doi: 10.1159/000196125
27. Huber M, Mossmann H, Bessler WG. Th1-orientated immunological properties of the bacterial extract OM-85-BV. Eur J Med Res. (2005) 10:209–17. PMID: 1594692215946922
28. Seo SH, Webster RG. Tumor necrosis factor alpha exerts powerful anti-influenza virus effects in lung epithelial cells. J Virol. (2002) 76:1071–6. doi: 10.1128/jvi.76.3.1071-1076.2002
29. Rossi GA, Colin AA. Infantile respiratory syncytial virus and human rhinovirus infections: respective role in inception and persistence of wheezing. Eur Respir J. (2015) 45:774–89. doi: 10.1183/09031936.00062714
30. Ganjian H, Rajput C, Elzoheiry M, Sajjan U. Rhinovirus and innate immune function of airway epithelium. Front. Cell. Infect. Microbiol. (2020) 10:277. doi: 10.3389/fcimb.2020.00277
31. Roth M, Pasquali C, Stolz D, Tamm M. Broncho vaxom (OM-85) modulates rhinovirus docking proteins on human airway epithelial cells via Erk1/2 mitogen activated protein kinase and cAMP. PLoS One. (2017) 12:e0188010. doi: 10.1371/journal.pone.0188010
32. Kumar NA, Kunnakkadan U, Thomas S, Johnson JB. In the crosshairs: RNA viruses OR complement? Front Immunol. (2020) 11:573583. doi: 10.3389/fimmu.2020.573583
33. Wilson SS, Wiens ME, Smith JG. Antiviral mechanisms of human defensins. J Mol Biol. (2013) 425:4965–80. doi: 10.1016/j.jmb.2013.09.038
34. Bianco A, Whiteman SC, Sethi SK, Allen JT, Knight RA, Spiteri MA. Expression of intercellular adhesion molecule-1 (ICAM-1) in nasal epithelial cells of atopic subjects: a mechanism for increased rhinovirus infection? Clin Exp Immunol. (2000) 121:339–45. doi: 10.1046/j.1365-2249.2000.01301.x
35. Bahadoran A, Lee SH, Wang SM, Manikam R, Rajarajeswaran J, Raju CS, et al. Immune responses to influenza virus and its correlation to age and inherited factors. Front Microbiol. (2016) 7:1841. doi: 10.3389/fmicb.2016.01841
36. Rossi GA, Bessler W, Ballarini S, Pasquali C. Evidence that a primary antiviral stimulation of the immune response by OM-85 reduces susceptibility to a secondary respiratory bacterial infection in mice. Ital J Pediatr. (2018) 44:112. doi: 10.1186/s13052-018-0569-7
37. Tin Tin Htar M, Yerramalla MS, Moïsi JC, Swerdlow DL. The burden of respiratory syncytial virus in adults: a systematic review and meta-analysis. Epidemiol Infect. (2020) 148:e48. doi: 10.1017/S0950268820000400
38. Antunes KH, Stein RT, Franceschina C, da Silva EF, de Freitas DN, Silveira J, et al. Short-chain fatty acid acetate triggers antiviral response mediated by RIG-I in cells from infants with respiratory syncytial virus bronchiolitis. EBioMedicine. (2022) 77:103891. doi: 10.1016/j.ebiom.2022.103891
39. Antunes KH, Cassão G, Santos LD, Borges SG, Poppe J, Gonçalves JB, et al. Airway administration of bacterial lysate OM-85 protects mice against respiratory syncytial virus infection. Front Immunol. (2022) 13:867022. doi: 10.3389/fimmu.2022.867022
40. Vu LD, Siefker D, Jones TL, You D, Taylor R, DeVincenzo J, et al. Elevated levels of type 2 respiratory innate lymphoid cells in human infants with severe respiratory syncytial virus bronchiolitis. Am J Respir Crit Care Med. (2019) 200:1414–23. doi: 10.1164/rccm.201812-2366OC
41. Pivniouk V, Gimenes–Junior JA, Ezeh P, Michael A, Pivniouk O, Hahn S, et al. Airway administration of OM–85, a bacterial lysate, blocks experimental asthma by targeting dendritic cells and the epithelium/IL– 33/ILC2 axis. J Allergy Clin Immunol. (2021) 149:943–56. doi: 10.1016/j.jaci.2021.09.013
42. Makris S, Bajorek M, Culley FJ, Goritzka M, Johansson C. Alveolar macrophages can control respiratory syncytial virus infection in the absence of type I interferons. J Innate Immun. (2016) 8:452–63. doi: 10.1159/000446824
43. González-Navajas JM, Lee J, David M, Raz E. Immunomodulatory functions of type I interferons. Nat Rev Immunol. (2012) 12:125–35. doi: 10.1038/nri3133
44. Ospelnikova TP, Isaeva EI, Kolodyaznaya LV, Kozulina IS, Andreeva SA, Poloskov VV, et al. Antiviral activity of the interferon beta 1a. Vopr Virusol. (2015) 60:24–8. PMID: 2702491327024913
45. Perng YC, Lenschow DJ. ISG15 In antiviral immunity and beyond. Nat Rev Microbiol. (2018) 16:423–39. doi: 10.1038/s41579-018-0020-5
46. Rossi GA, Sacco O, Mancino E, Cristiani L, Midulla F. Differences and similarities between SARS-CoV and SARS-CoV-2: spike receptor-binding domain recognition and host cell infection with support of cellular serine proteases. Infection. (2020) 48:665–9. doi: 10.1007/s15010-020-01486-5
47. Wiersinga WJ, Rhodes A, Cheng AC, Peacock SJ, Prescott HC. Pathophysiology, transmission, diagnosis, and treatment of coronavirus disease 2019 (COVID-19): a review. JAMA. (2020) 324:782–93. doi: 10.1001/jama.2020.12839
48. Pivniouk V, Pivniouk O, DeVries A, Uhrlaub JL, Michael A, Pivniouk D, et al. The OM-85 bacterial lysate inhibits SARS-CoV-2 infection of epithelial cells by downregulating SARS-CoV-2 receptor expression. J Allergy Clin Immunol. (2022) 149:923–33. doi: 10.1016/j.jaci.2021.11.019
49. Fang L, Zhou L, Tamm M, Roth M. OM-85 Broncho-Vaxom®, a bacterial lysate, reduces SARS-CoV-2 binding proteins on human bronchial epithelial cells. Biomedicines. (2021) 9:1544. doi: 10.3390/biomedicines9111544
50. Lambeir AM, Durinx C, Scharpé S, De Meester I. Dipeptidyl-peptidase IV from bench to bedside: an update on structural properties, functions, and clinical aspects of the enzyme DPP IV. Crit Rev Clin Lab Sci. (2003) 40:209–94. doi: 10.1080/713609354
51. Strollo R, Pozzilli P. DPP4 Inhibition: preventing SARS-CoV-2 infection and/or progression of COVID-19? Diabetes Metab Res Rev. (2020) 36:e33300. doi: 10.1002/dmrr.3330
52. Black RA, Rauch CT, Kozlosky CJ, Peschon JJ, Slack JL, Wolfson MF, et al. A metalloproteinase disintegrin that releases tumour-necrosis factor-alpha from cells. Nature. (1997) 385:729–33. doi: 10.1038/385729a0
53. Healy EF, Lilic M. A model for COVID-19-induced dysregulation of ACE2 shedding by ADAM17. Biochem Biophys Res Commun. (2021) 573:158–63. doi: 10.1016/j.bbrc.2021.08.040
54. Jing W, Procko E. ACE2-based Decoy receptors for SARS coronavirus 2. Proteins. (2021) 89:1065–78. doi: 10.1002/prot.26140
55. Vlodavsky I, Ilan N, Naggi A, Casu B. Heparanase: structure, biological functions, and inhibition by heparin-derived mimetics of heparan sulfate. Curr. Pharm. Des. (2007) 13:2057–73. doi: 10.2174/138161207781039742
56. Kinaneh S, Khamaysi I, Karram T, Hamoud S. Heparanase as a potential player in SARS-CoV-2 infection and induced coagulopathy. Biosci Rep. (2021) 41:BSR20210290. doi: 10.1042/BSR20210290
57. Clausen TM, Sandoval DR, Spliid CB, Pihl J, Perrett HR, Painter CD, et al. SARS-CoV-2 infection Depends on cellular heparan sulfate and ACE2. Cell. (2020) 183:1043–57. doi: 10.1016/j.cell.2020.09.033
59. Zamboni F, Wong CK, Collins MN. Hyaluronic acid association with bacterial, fungal, and viral infections: can hyaluronic acid be used as an antimicrobial polymer for biomedical and pharmaceutical applications? Bioact Mater. (2022) 19:458–73. doi: 10.1016/j.bioactmat.2022.04.023
60. Claus-Desbonnet H, Nikly E, Nalbantova V, Karcheva-Bahchevanska D, Ivanova S, Pierre G, et al. Polysaccharides and their derivatives as potential antiviral molecules. Viruses. (2022) 14:426. doi: 10.3390/v14020426
61. Cermelli C, Cuoghi A, Scuri M, Bettua C, Neglia RG, Ardizzoni A, et al. In vitro evaluation of antiviral and virucidal activity of a high molecular weight hyaluronic acid. Virol J. (2011) 8:141. doi: 10.1186/1743-422X-8-141
62. Salzmann M, Haider P, Kaun C, Brekalo M, Hartmann B, Lengheimer T, et al. Innate immune training with bacterial extracts enhances lung macrophage recruitment to protect from betacoronavirus infection. J Innate Immun. (2021) 22:1–13. doi: 10.1159/000519699
63. Rehwinkel J, Gack MU. RIG-I-like receptors: their regulation and roles in RNA sensing. Nat Rev Immunol. (2020) 20:537–51. doi: 10.1038/s41577-020-0288-3
64. Yin J, Xu B, Zeng X, Shen K. Broncho-Vaxom in pediatric recurrent respiratory tract infections: a systematic review and meta-analysis. Int Immunopharmacol. (2018) 54:198–209. doi: 10.1016/j.intimp.2017.10.032
65. Cardinale F, Lombardi E, Rossi O, Bagnasco D, Bellocchi A, Menzella F. Epithelial dysfunction, respiratory infections and asthma: the importance of immunomodulation. A focus on OM-85. Expert Rev Respir Med. (2020) 14:1019–26. doi: 10.1080/17476348.2020.1793673
66. Steurer-Stey C, Lagler L, Straub DA, Steurer J, Bachmann LM. Oral purified bacterial extracts in acute respiratory tract infections in childhood: a systematic quantitative review. Eur J Pediatr. (2007) 166:365–76. doi: 10.1007/s00431-006-0248-3
67. Schaad UB. OM-85 BV, an immunostimulant in pediatric recurrent respiratory tract infections: a systematic review. World J Pediatr. (2010) 6:5–12. doi: 10.1007/s12519-010-0001-x
68. Del-Rio-Navarro BE, Espinosa Rosales F, Flenady V, Sienra-Monge JJ. Immunostimulants for preventing respiratory tract infection in children. Cochrane Database Syst Rev. (2006) 4:CD004974. doi: 10.1002/14651858.CD004974.pub2
69. Pan L, Jiang XG, Guo J, Tian Y, Liu CT. Effects of OM-85 BV in patients with chronic obstructive pulmonary disease: a systematic review and meta-analysis. J Clin Pharmacol. (2015) 55:1086–92. doi: 10.1002/jcph.518
70. Orcel B, Delclaux B, Baud M, Derenne JP. Oral immunization with bacterial extracts for protection against acute bronchitis in elderly institutionalized patients with chronic bronchitis. Eur Respir J. (1994) 7:446–52. doi: 10.1183/09031936.94.07030446
71. Collet JP, Shapiro P, Ernst P, Renzi T, Ducruet T, Robinson A. Effects of an immunostimulating agent on acute exacerbations and hospitalizations in patients with chronic obstructive pulmonary disease. The PARI-IS study steering committee and research group. Prevention of acute respiratory infection by an immunostimulant. Am J Respir Crit Care Med. (1997) 156:1719–24. doi: 10.1164/ajrccm.156.6.9612096
72. Solèr M, Mütterlein R, Cozma G, Swiss-German OM-85 Study Group. Double-blind study of OM-85 in patients with chronic bronchitis or mild chronic obstructive pulmonary disease. Respiration. (2007) 74:26–32. doi: 10.1159/000093933
73. Tielemans C, Gastaldello K, Husson C, Marchant A, Delville JP, Vanherweghem JL, et al. Efficacy of oral immunotherapy on respiratory infections in hemodialysis patients: a double-blind, placebo-controlled study. Clin Nephrol. (1999) 51:153–60. PMID: 1009988810099888
74. Huang Y, Pei Y, Qian Y, Yao Z, Chen C, Du J, et al. A meta-analysis on the efficacy and safety of bacterial lysates in chronic obstructive pulmonary disease. Front Med (Lausanne). (2022) 9:877124. doi: 10.3389/fmed.2022.877124
75. de Boer GM, Żółkiewicz J, Strzelec KP, Ruszczyński M, Hendriks RW, Braunstahl GJ, et al. Bacterial lysate therapy for the prevention of wheezing episodes and asthma exacerbations: a systematic review and meta-analysis. Eur Respir Rev. (2020) 29(158):190175. doi: 10.1183/16000617.0175-2019
76. Troy NM, Strickland D, Serralha M, de Jong E, Jones AC, Read J, et al. Protection against severe infant lower respiratory tract infections by immune training: mechanistic studies. J Allergy Clin Immunol. (2022) 150:93–103. doi: 10.1016/j.jaci.2022.01.001
77. Esposito S, Ballarini S, Argentiero A, Ruggiero L, Rossi GA and Principi N Microbiota profiles in preschool children with respiratory infections: modifications induced by the oral bacterial lysate OM-85. Front. Cell. Infect. Microbiol. 2022;12: 789436.doi: 10.3389/fcimb.2022.789436
78. Rossi GA, Ballarini S, Salvati P, Sacco O, Colin AA. Alarmins and innate lymphoid cells 2 activation: a common pathogenetic link connecting respiratory syncytial virus bronchiolitis and later wheezing/asthma? Pediatr Allergy Immunol. (2022) 33:e13803. doi: 10.1111/pai.13803
Keywords: respiratory infections, bacterial lysates, OM-85, HRV, RSV, IFV, SARS-CoV-2, trained immunity
Citation: Ballarini S, Ardusso L, Ortega Martell JA, Sacco O, Feleszko W and Rossi GA (2022) Can bacterial lysates be useful in prevention of viral respiratory infections in childhood? The results of experimental OM-85 studies. Front. Pediatr. 10:1051079. doi: 10.3389/fped.2022.1051079
Received: 22 September 2022; Accepted: 24 October 2022;
Published: 21 November 2022.
Edited by:
Milos Jesenak, Comenius University, SlovakiaReviewed by:
Kamil Piotr Janeczek, Medical University of Lublin, PolandTiziana Corsello, University of Texas Medical Branch, United States
Charu Rajput, Temple University, United States
© 2022 Ballarini, Ardusso, Ortega Martell, Sacco, Feleszko and Rossi. This is an open-access article distributed under the terms of the Creative Commons Attribution License (CC BY). The use, distribution or reproduction in other forums is permitted, provided the original author(s) and the copyright owner(s) are credited and that the original publication in this journal is cited, in accordance with accepted academic practice. No use, distribution or reproduction is permitted which does not comply with these terms.
*Correspondence: Giovanni A. Rossi Z2lvdmFubmlyb3NzaUBnYXNsaW5pLm9yZw==
Specialty Section: This article was submitted to Pediatric Pulmonology, a section of the journal Frontiers in Pediatrics