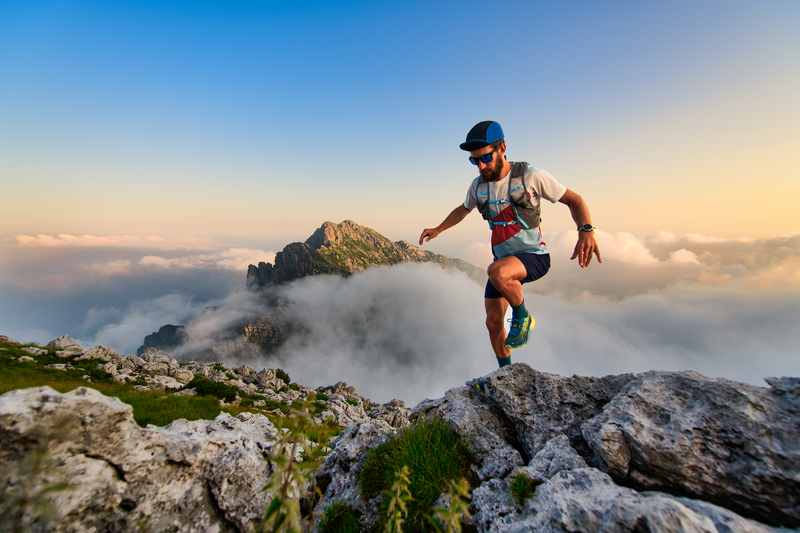
94% of researchers rate our articles as excellent or good
Learn more about the work of our research integrity team to safeguard the quality of each article we publish.
Find out more
REVIEW article
Front. Pediatr. , 21 September 2021
Sec. Neonatology
Volume 9 - 2021 | https://doi.org/10.3389/fped.2021.706012
This article is part of the Research Topic Perinatal Assessment of Biomarkers in Invasive and Non-Invasive Procedures of Biological Fluid Collection View all 21 articles
Circular RNAs (circRNAs) generated by back-splicing are the vital class of non-coding RNAs (ncRNAs). Circular RNAs are highly abundant and stable in eukaryotes, and many of them are evolutionarily conserved. They are blessed with higher expression in mammalian brains and could take part in the regulation of physiological and pathophysiological processes. In addition, premature birth is important in neurodevelopmental diseases. Brain damage in preterm infants may represent the main cause of long-term neurodevelopmental disorders in surviving babies. Until recently, more and more researches have been evidenced that circRNAs are involved in the pathogenesis of encephalopathy of premature. We aim at explaining neuroinflammation promoting the brain damage. In this review, we summarize the current findings of circRNAs properties, expression, and functions, as well as their significances in the neurodevelopmental impairments, white matter damage (WMD) and hypoxic-ischemic encephalopathy (HIE). So we think that circRNAs have a direct impact on neurodevelopment and brain injury, and will be a powerful tool in the repair of the injured immature brain. Even though their exact roles and mechanisms of gene regulation remain elusive, circRNAs have potential applications as diagnostic biomarkers for brain damage and the target for neuroprotective intervention.
The damage throughout perinatal period has a profound impact on brain development which is rather essential. Compared with term infants, brain damage in preterm infants, especially the very low birth weight (VLBW) infants, tends to represent the main cause of higher mortality and long-term adverse neurodevelopmental manifestations, including but not limited to motor and sensory problems, decreased cognitive development, and behavior disorders (1, 2). Generally speaking, the lower the gestational age (GA) and birth weight (BW) are, alone or combined, the higher the morbidity of brain damage is, and so is the mortality (3). A previous study has shown that premature birth does play an important role (4). Prematurity may greatly affect the integrity of brain structure and function, as well as health and development of further life (5). Thus, brain damage in preterm infants has grown into a crucial public health issue worldwide. Fortunately, early interventions could be helpful for regeneration and repair of the premature brain.
As is known to all, circular RNAs (circRNAs) have been confirmed to serve as important roles in the pathogenesis of various diseases (6). Currently, researchers have mostly studied circRNAs, and they demonstrated that circRNAs possibility participate in central nervous system (CNS) development and brain damage via excitotoxicity, apoptosis, especially the excessive neuroinflammation (7, 8). For example, circPTK2/miR-29b-SOCS-1-JAK2/STAT3-IL-1β axis could modulate microglia-induced neuronal apoptosis (9). Even though the biological importance of circRNAs has been shown, we know little about expression patterns and biological functions during brain injury in babies who are born prematurely. As a consequence, we mainly summarize the progress achieved in this research field to have a better understanding of the molecular etiology in brain development and damage of preterm infants, and then contribute circRNAs as novel agents for early detection, early diagnosis, early treatment, and even prognosis of circRNA-related diseases in the CNS.
Circular RNAs, one class of non-coding RNAs (ncRNAs), can be mainly divided into three categories, containing intron circRNAs, exon-intron circRNAs, and exon circRNAs (10). The first two subtypes remain in nucleus and could alter primary genes, while the last, the predominant one, then localize and function in the cytoplasm after being transferred from the nucleus through a length-dependent pathway (11, 12). Back splicing and linear splicing could independently produce circRNAs and mRNAs. Most circRNAs are generated from protein-coding genes by the reverse connection of the downstream 5′ splice site with the upstream 3′ splice site. Via the specific splicing, circRNAs are single-strained and blessed with covalently closed-loop structure for lack of 5′ and 3′ ends and poly(A) tail whose appearance is totally different from corresponding linear RNAs (Figure 1A) (13, 14).
Figure 1. Characteristics and functions of circRNAs. (A) Characteristics of circRNAs. circRNAs generated through back splicing can be divided into intron circRNAs, exon-intron circRNAs, and exon circRNAs. The first two kinds remain in nucleus, while exon circRNAs then localize to cytoplasm to play roles. (B) Functions of circRNAs. CircRNAs could serve as miRNA sponges, interact with RBP, and translate functional proteins to take part in important biological activities.
In spite that the production of circRNAs is not efficient in a high rate any more, they are rather resistant to degradation mediated by ribonuclease R (RNase R) for lack of free terminals. As a result, this class of RNAs owns transcript half-life exceeding 48 h longer than most of linear RNAs in cells (15, 16). For example, certain circRNA has six times resistance as many as that of linear transcript after RNase R disposition (17). On the one hand, it is the unique characteristic that renders circRNAs extraordinary stable, which makes it possible for circRNAs to impress the information efficiently and to be ideal diagnostic biomarkers for certain diseases. On the other hand, circRNAs generally accumulate in cytoplasm for free of degeneration, indicating that circRNAs may function in regulation of cellular functions and gene expression.
A majority of circRNAs are evolutionarily conserved in mammals (18, 19). The conservation of circRNAs, at least to a certain extent, is correlated with the complementary sequences in introns flanking exons in both species, instead of the circRNA sequences themselves (20, 21). On this account, the conserved expression of circRNAs in mammals suggests the similarity of their biological process in biogenesis, rather than side products of precursor RNAs.
Circular RNAs are widespread found in all kingdoms of life. Their expression is validated in RNA samples from almost all of the organs and tissues, such as brain, testis, kidney, spleen, liver, heart, bone marrow, smooth, skeletal muscles, and so on (22–24). Owing to better stability, these small molecules wrapped in exosomes gain the ability to cross the blood-brain barrier (BBB) and then enter the circulatory system with their original patterns in those of brain (25). Some scientists found 104,388, 96,675, and 82,321 circRNAs from humans, macaques, and mice, respectively, and the average proportion of each species successfully assembled into full-length transcripts was 72.6% (23). This large-scale study also confirmed that circRNAs were tissue-specific and appropriately 20% were expressed in the brain. That is to say, circRNAs are more significantly abundant, dynamically expressed in the mammalian brain tissue than in any other organs (26, 27). This discovery may demonstrate potential functions during nervous system development.
Generally speaking, circRNAs overall transcript levels in brain steadily grow during the developmental and aging phases, from embryonic to adulthood (28, 29). Circular RNAs expression during four different developmental stages (2, 6, 21, and 104 weeks) in rat brain tissues was previously studied, showing an increase in abundance as rats aged (30). However, the reality is that the expression and distribution of certain circRNAs are likely to vary from development to development (31). Some circRNAs are downregulated while the others are in upregulation with maturation (32). In general, this phenomenon in detail remains unclear.
What's more, the expression of circRNAs is not equally distributed throughout the brain, accompanying significant differences in various brain regions (11). Interestingly, circRNAs are highly enriched in olfactory bulb, prefrontal cortex, hippocampus, and cerebellum (33). Besides, the transcriptions of genes that major in producing circRNAs upon the differentiation of complex morphological neurons are dramatically enhanced, indicating that highly differentiated cells contain significantly higher levels of circRNAs (34–36). And circRNAs are unevenly expressed in diverse compartment of neurons, such as cell bodies and axons, but highly localized in synapses or other term. The levels of circRNAs in synapsis are modulated by neuronal activity and plasticity (37). All in all, circRNAs in neurons possess the characteristic of functional diversity as likely as not.
As stated above, circRNAs are dramatically enriched in CNS due to the following reasons. (A) CircRNAs are produced mainly from exons of protein-coding gene which are specific for neuronal and synaptic functions. (B) CircRNAs are unable to be identified by RNase R and get used to expressing in relatively high levels in cells. (C) On account that neurons are relatively stable cells and cell division rarely occurs, circRNAs theoretically accumulate during the process of development and aging.
The superior abundance and stability in the mammalian brain prompt many researchers to explore the roles of circRNAs in the CNS. Nonetheless, the mechanisms deep into the details by which circRNAs take participated in have not been completely identified. As is well known, microRNA (miRNA), small single-stranded, and ncRNA, could downregulate post-transcriptional expression by binding 3′-untranslated region of target messenger RNA (mRNA) to adjust the progression of brain development and diseases (38). RNA-binding proteins (RBPs) acting as regulators participate in the generation, alternative splicing, transfer of RNAs, as well as post-transcripted regulation (39). Previous researches have revealed that circRNAs could not only serve as miRNA sponges to competitively inhibit the transcriptional regulation of miRNAs, but also alter mRNAs expression via RBPs (Figure 1B) (39, 40). Sometimes, a circRNA includes at least two miRNA binding sites (41). For example, circRNA CDR1as is predominantly expressed in excitatory neurons and owns more than 70 conserved binding sites, one of which candidate is miR-7. Cdr1as sponging to miR-7 could directly promote miRNA depletion. After vanishing Cdr1as locus, miR-7 was destabilized and neuronal activity was then changed, causing electrophysiological and motor disorders in mice (42). For another example, circDYM that possesses the binding site for miR-9 has been demonstrated to inhibit miRNA activity so that HSP90 ubiquitination would occur and consequently suppress the microglial activation (43). Therefore, we can speculate that the cirRNA-miRNA-mRNA axis is a promising direction in the CNS. Furthermore, circHomer1α, a neuronal-enriched circRNA, interacting with neuronal RBP HuD could adjust the expression of synapses. And circHomer1α disorder has been found in the patients with bipolar disorder and schizophrenia (44). Last but not least, certain circRNAs might directly perform as templates for translation (Figure 1B) (45, 46). An early study found that an endogenous circRNA called circPINTexon2 was used to encode a tumor-suppressive peptide during the proliferation of glioblastoma cells, suggesting the potential indicator of this circRNA as diagnostic biomarker for brain tumor (47). However, how circRNAs are excellently translated into functional proteins in normal physiological or pathological conditions need to further clarify.
Cerebrovascular autoregulation is extraordinarily important to maintain the physiological function of brain. Newborns, especially those premature neonates with higher susceptibility, have imperfect cerebrovascular structures and regulation mechanisms (48, 49). When asphyxia happens, the cerebrovascular self-regulation is gradually lost, leading to cerebral edema and even worse, cerebral hemorrhage. It is demonstrated that cerebral hypoperfusion could result in white matter damage (WMD), BBB damage, neuronal oxidative stress, and cell death (50). Fortunately, rat models of ischemia reperfusion injury have suggested that circRNAs were sensitive to cerebral ischemia (40). So, we are aware that circRNAs expression may have a significant influence on CNS. Moreover, the disappearance of rather rich circRNAs can also be applied to mark diseases (14). And there will be a potential chance in changing the level of dysregulated circRNAs to improve brain damage.
Due to tissue and cellular structure specificity of circRNAs, increasing studies have been revealed that circRNAs are connected to neurological dysfunctions caused by brain damage (13, 51). Oligodendrocytes (OLs) and myelin have been demonstrated to downregulate in the course of WMD, along with functional loss of synaptic transmission, eventually leading to white matter atrophy (52–54). In addition, synapses play a vital role in information transfer between neurons, and are crucial to the complex signal processing and network in CNS (55, 56). Circular RNAs multiply in abundance during fetal development and the formation of brain structure occurs before birth (37). These may be the main causes of long-term neurological deficits reflected in surviving children of brain damage. To sum up, circRNAs have the potential chance to serve as diagnostic and prognostic indicators.
The early injury to the developing brain may cause circRNA disorder, recent, or long-term neurodevelopmental dysfunctions via diverse mechanisms. The circRNA Runx1t1, Pde5a, and Rtn4 three are differentially expressed in hippocampus and prefrontal cortex where learning and long-term memory take place, and their inhibition may perfect memory performance as well as cognitive function (57). Based on how circRNAs act in CNS, the relationship will be concluded between circRNAs and neurodevelopmental impairments in the aspects of intelligence, cognition and behavior, as well as others (Table 1).
Intellectual deficiency seriously affects the quality of life both of children themselves and their families. Circular RNA SATB2 from corpus callosum is significantly upregulated in the late stage of pregnancy (37). In a case report, the mutation of special AT-rich special sequence-binding protein 2 (SATB2) which encoded a DNA-binding protein can lead to severe intellectual disabilities in children, suggesting that SATB2 may play a disadvantageous role in nervous development (62). Furthermore, the relationship between SATB2 and learning and memory has been evidenced in mice experiments (58). And SATB2 directly activates transcription of forebrain embryonic zinc finger 2 and SRY-box 5 which are the essential genes for the development of subcerebral neurons (63). Collectively, more studies need performing to explain whether and how circSATB2 influences intellectuality.
The damage of cerebral gray and white matter has been confirmed to be closely related to cognitive abnormality in later life of preterm babies (64). Exon circCdh9, generated by Cdh9 gene, is validated to take part in autism spectrum disorder (ASD) (17). In other words, down-expressed circCdh9 may reflect cognitive problems. As we have mentioned before, circHomer1's original gene called HOMER1 was linked to psychiatric disorders (44). In addition, decreased levels of circHDAC9 in serum collected from cognitive impairment patients has been demonstrated, manifesting the connection between cognition and the steady-state levels of above transcripts (59).
Children with brain injury would exhibit abnormal behavior to some extent, such as attention deficit hyperactivity disorder (65). CircTLK1-knockout mice which lack certain circRNA expression in brain tissue, displayed reduced infarct volumes and neuronal damage, as well as significant improvements in neurobehavioral abnormalities. At the same time, circTLK1 sponging to miR-335-3p led to inhibited activity of miRNA, which aggravated subsequent neuronal injury (40). In consequence, the behavioral problems in children may be improved by regulation of related circRNAs expression.
Epilepsy with the higher risk in infants is a chronic neurological disease, of which temporal lobe epilepsy (TLE) is the most common type of epilepsy. As its name suggests, TLE seizures begin in the temporal lobe cortex and then abnormal cortical signals are exhibited, characterized by hippocampal sclerosis, neuronal death, gliosis, and so on (66, 67). However, little is known between circRNAs and TLE in synaptogenesis, ion channels, and neuroinflammation (68). A previous study showed the analyses of circRNAs profiles in human TLE. Although circ-EFCAB2 was remarkably increased, circ-DROSHA was dramatically down-regulated confirmed in quantitative PCR (60). Circ-EFCAB2 can combine with miR-485-5p in order to regulate calcium channel. Circ-DROSHA binding to miR-1252-5p decreases the level of alpha 2 subunit of Na+, K+-ATPase (ATP1A2) which has been evidenced to participate in reducing the extracellular K+ and then inhibiting neuron depolarization (69, 70). Another experiment interestingly demonstrated that overexpressed circ-DROSHA weakened the neural damage of the TLE cell model (71). A recent study also confirmed the altered circRNAs expression during epileptogenesis (61). If circRNAs could impair paradoxical discharge, TLE would be suppressed.
The brain damage in early life does influence the prognosis of affected children. Hirosuke and his colleagues investigated 3-year-old children's neurodevelopmental outcomes who were born <500 g. Through follow-up visits and data analyses of these survivals, their group made a great discovery that cystic periventricular leukomalacia, a severe category of brain damage in premature infants, was the high risk factor for neurological consequences (adjusted RR:1.40; 95%CI:1.13–1.73; P < 0.01) (4). So we will first focus on hypoxic-ischemic encephalopathy (HIE), an important component of the brain damage. The WMD and its related neuronal malformations are then discussed (Figure 2).
Figure 2. Circular RNAs in hypoxic-ischemic encephalopathy and white matter damage. (A) cZNF292 can act as a sponge of miR-22 and then participate in inflammation via wnt/β-catenin pathway of the oxygen-glucose deprivation/reperfusion (OGD/R) cell model. (B) In HIE rats, chr1: 200899066|201028171 can sponge miR-126a and miR-9a during apoptosis. circ-camk4 might participate in neuronal apoptosis by MAPK signaling pathway, which was showed through OGD/R neurons. (C) circ-003342 can sponge miR-143 to associate with vascular endothelium thereby regulate the blood-brain barrier in OGD/R brain microvascular endothelial cells. (D) hsa_circ_0001868, hsa_circ_0083176, hsa_circ_0018401, hsa_circ_0006357, and hsa_circ_0006716 are increased in premature infants serum to be biomarkers of white matter damage. (E) circSATB2 can regulate the extension of axons via animal and cell studies on schizophrenia.
Neonatal HIE refers to hypoxic-ischemic (HI) damage in brain caused by perinatal asphyxia and hypoxia, following a series of clinical neurological manifestations. What's worse, subsequent cascading reactions after cerebral ischemia-reperfusion may cause considerably severe brain damage (72). In other words, both cell death and apoptosis might to be caused by HI, which potentially leads to further secondary brain injury in adjacent tissues (73). In addition, such damages would alter the expression and release of circRNAs in cells. A current research has showed that circRNAs expression in neonatal rats following HI brain damage was greatly changed which included 20 upregulated and 46 downregulated circRNAs (74). In another mice model, circDLGAP4 overexpression significantly decreases infarct areas (75). Based on the above research results, circRNAs may serve as the potential role of novel treatment target in ischemic brain damage.
It is critical to appreciate that neuroinflammation is the main driver in injury to the developing brain and greatly amplified after HI insult. That is, inflammation inhibits conveying messages between neurons but increases the sensitivity of immature brains to hypoxic so that cerebral injury will be prolonged (76). What's more, oxidative stress is considered as the contributor to brain injury working in combination with inflammation (77). According to previous reports, microglia, astrocytes and endothelial cells activated by cerebral hypoperfusion can release reactive oxygen species (ROS) and toxicity of excitatory amino acids (78). And both excessive ROS and relative amino acids will further promote inflammatory cytokines and activate various cell signaling pathways, which in turn bring on immune responses, neuronal apoptosis and necrosis. This theory is widely applied in oxygen-glucose deprivation models (79). Through the neural stem cells experiment of oxygen-glucose deprivation/reperfusion (OGD/R)-induced injury, cZNF292 was over-expressed and then ORD/R injury exacerbated. Instead, cZNF292 had negative influence on miR-22, after which the insult was getting better by the way of wnt/β-catenin pathway (Figure 2A) (80). This signaling has been evidenced in importance for the differentiation of neurons and the formation of neural circuits, which are likely to be relevant to the arrangement of cerebrovascular and BBB (81). Therefore, this pathway will possibly have a huge impact on young brains.
Nevertheless, apoptosis is essential for the development of immature CNS. When the energy supply is significantly reduced, apoptotic cell death will occur, and at the same time, cell neurosis would take place in rather severe cases (49). Lin et al. made use of OGD/R model to analyze circRNAs in mouse hippocampal HT22 cells, and then speculated that several circRNAs may be involved in apoptosis, metabolism, and immune, which pathways have been widely studied in HIE (82). chr1: 200899066–201028171 can, respectively, sponge miR-126a and miR-9a to involve in hypoxia-induced neuronal apoptosis (Figure 2B) (11, 74). For another example, circ-camk4 could promote neuronal apoptosis, and might to act in MAPK signaling pathway which is related to the pathogenesis of cerebral ischemia reperfusion injury (Figure 2B) (83). Thus, apoptosis is regarded as a vital component of the neuronal cell death in neonatal HIE.
Blood-brain barrier selectively allows molecules to cross the barrier in order to protect the neonatal brains in physiological condition (84). Therefore, BBB damage is not only a consequence of but also a contributor to the progression of HI. Besides, cerebral microvascular endothelial cell dysfunction caused by cerebral ischemia-reperfusion is the initial phase of BBB destruction. It has been evidenced that certain circRNAs could improve vascular endothelial dysfunction (85). Modulation of circRNAs related to endothelial cell damage is beneficial to preserve the BBB integrity. For example, Liu et al. suggested that circRNAs may regulate calcium ion and facilitate signal transduction so as to modulate the function of vascular endothelium avoid cell swelling, and lysis (Figure 2C) (84). So, BBB protection has been regarded as a potential therapeutic strategy for ischemic encephalopathy. The molecular and cellular mechanism of HIE in BBB dysfunction remains to be elucidated. Similarly, the role of circRNAs in the pathogenesis of HIE is largely unknown. Consequently, further researches are needed to be done around circRNAs and BBB protection as well as inflammation in the developing brain.
White matter damage in premature infants is the predominant class of neonatal brain damage (86). It features high risk of disability, leading to severe impairments of neurological functions, such as cerebral palsy, cognitive impairment, epilepsy, and so on (52, 87). But even worse, for lack of specific clinical characteristics, its clinical diagnosis is largely depended on neuroimaging techniques which are called retrospective analyses. Cranial ultrasound (cUS) and MRI are the main methods for clinical diagnosis (88, 89). On the one hand, cUS is more convenient for the reason that it can be conducted in the ward. On the other hand, it's more suitable for cystic lesions and bigger focus while the vast majority of WMD is the diffuse type. As is known to all, MRI is not only used to analyze lesions' size, range, and location, but also as the gold standard of this disease (86). Nevertheless, it is the sedation before and during examination, and the movement to imaging department, as well as too much time spent on scanning, that make it more or less difficult to complete MRI, which very much result in delayed diagnosis for some severe patients.
Taking all the facts into consideration, there is an urgent need for early detection markers and novel therapeutic targets of WMD in preterm infants. Luckily, our team previously analyzed the whole blood samples of preterm infants who have had WMD and then identified that the expression profiles of circRNAs had greatly changed following brain injury (Figure 2D) (90). In hence, we suppose that circRNAs may act as an important regulator in the pathological changes and dysfunction of CNS.
At the same time, the white matter is featured in myelin, a critical component produced by OLs that facilitate the efficient transmission of electrical signals and then enable communication between gray matter neurons throughout various areas (91). Moreover, myelin can rapidly transfer action potential along axons, and myelination promoting synapses has been evidenced (92). As main cells in white matter between the GA of 24–30 weeks, premyelinating oligodendrocytes (pre-OLs) can be differentiated into mature OLs mainly after 32 weeks of gestation, which partially explains the highest incidence of WMD during this period (93). So selective pre-OL death seems to be the predominate process in diffuse WMD, eventually causing the failure of myelination in the developing white matter. Thus, pre-OL injury could also give rise to dysfunction of axonal development and ultimately axonal degeneration (65). Therefore, stimulating myelination and accelerating the recovery of white matter is possibly thought to account for effective treatments for WMD. However, little is known about circRNA function in pre-OL injury and repair during and after WMD. For this reason, whether circRNAs contribute to pre-OL damage in the course of WMD remains poorly understood.
Typically, loss of glia and axons has been acknowledged for many years to be the characteristic of cystic WMD (3). For preterm infants, the active axonal development in cerebral white matter could make nerve fibers particularly vulnerable. In CNS, the majority of axons cannot regenerate after injury, which likely explains irreversible neurological dysfunctions caused by CNS trauma (94). In addition to promote neuronal development, SATB2 regulates the extension of axons throughout the corpus callosum to participate in synaptic transmission (Figure 2E) (95).
Current researches of WMD have rarely involved the role of pro-inflammatory and anti-inflammatory in brain repair. Microglia, accounting for 10–20% of all glial cells, are the innate immune cells and the main regulator cells for the repair/regeneration of the CNS (96). Excessively activated microglia in white matter can destroy the axons and myelin sheaths of neurons, and ultimately lead to the declines in cognitive, motor, and other functions in various degrees. In other CNS diseases, circRNAs could regulate the progression through neuroinflammation. For instance, circHivep2 was evidenced in promoting microglia activation and the release of inflammatory factors via miR-181a-5p/SOCS2 pathway (97). Over-activated microglia may be harmful to axons and myelin in return. So, excessive inflammation could affect neuron development and inflammation is considered as the main cause in diffuse white matter lesions. If circRNAs could impair immoderate inflammation, WMI would be repressed.
The above reviewed findings emphasize the relevance of circRNAs as bioactive molecules regulating the neurodevelopmental conditions. The overall conclusions from the works presented here are: (A) Premature birth plays a major and early role in neurodevelopmental diseases; (B) Regulatory circRNAs expression and activity dynamics regulate, directly and/or indirectly, gene expression networks involved in brain damage; (C) CircRNAs have a direct impact on neurodevelopment and brain injury.
The detailed biogenesis of circRNAs remains complicated. The molecular and physiological functions of most circRNAs are still unknown, but there is a tendency that the focus in this area would generate tremendous growth due to the progressed methods of overexpression, knocking out, and knocking down in specific circRNAs. At present, researches are mostly focused on cerebroma and stroke in adults. Circular RNAs wrapped by exosomes or cell vesicles could pass through the incomplete BBB resulted from brain injury, so that circRNAs are available in peripheral blood. Circular RNAs have emerged as the new type of molecules with intriguing molecular functions and potential biomarkers of related diseases. For example, due to elevated levels in plasma, circPDS5B, and circCDC14A could be potentially valuable biomarkers for acute ischemic stroke and target for therapeutics in stroke outcomes. Overexpressed circ-CDC45 has been confirmed to be active in the progression of glioma proliferation and invasion, and predict its adverse prognosis (98). Especially, the BBB of premature infants with high permeability is imperfect, making circRNAs possible to be biomarkers of WMD.
In addition, the functional interaction between different classes of ncRNAs, such as circRNAs and corresponding miRNAs and RBPs, provides an additional layer of complexity, in which multiple circRNA-signaling pathways orchestrate the response to the cerebral injury. However, most of previous studies focused on a particular circRNA while ignore that various molecules are functionally altered in the CNS diseases. So, the diversity raises the challenge of aiming the regulation of circRNAs to better protect from brain damage. The cirRNA-miRNA-mRNA code is the focus of nowadays researches. Nevertheless, we can speculate that additional RNA classes present in the CNS can be affected by and associated to neurodevelopment, including highly abundant miRNAs.
The increasing roles of circRNAs in neurological diseases also drew our attention. Five circRNAs were found differentially expressed through RNA high-throughput sequencing (90). Preliminary work has shown that these circRNAs were highly correlated with WMD of preterm infants, and had better stability. In order to explore whether circRNAs can serve as WMD biomarkers, we plan to investigate the expression of certain circRNAs in peripheral blood and then analyze their effects by taking imaging examinations as the gold standard.
In spite of numerous studies in circRNAs, there remains a few researches in diseases associated with premature infants. All these results mentioned in this article demonstrate the unique prospect of circRNAs as clinical diagnosis markers and therapeutic targets, and neuroprotective agents for brain damage of preterm infants, but still need confirming in further continuous studies by the use of large quantities of animals, clinical samples and even patients. Early neurological interventions are closely related to the patient's quality of the rest of life. So, the application of circRNAs as neuro-biomarkers can be exploited in the near future, which could unbelievably make an increase in the possibility for premature infants to live better in the world.
QG was the primary author of the review. HL and JM assisted with writing and provided edits. All authors approved this version.
This work was supported in part by the Postgraduate Research and Practice Innovation Program of Jiangsu Province (SJCX20_0057) and in part by the Fundamental Research Funds for the Central Universities (3224002112D).
The authors declare that the research was conducted in the absence of any commercial or financial relationships that could be construed as a potential conflict of interest.
All claims expressed in this article are solely those of the authors and do not necessarily represent those of their affiliated organizations, or those of the publisher, the editors and the reviewers. Any product that may be evaluated in this article, or claim that may be made by its manufacturer, is not guaranteed or endorsed by the publisher.
We thank Haiqin Lu and Ping Zhu for helpful suggestions. And special thanks to the Department of Biobank, Zhongda Hospital, Southeast University for their supports in the review.
1. Wu Y, Stoodley C, Brossard-Racine M, Kapse K, Vezina G, Murnick J, et al. Altered local cerebellar and brainstem development in preterm infants. Neuroimage. (2020) 213:116702. doi: 10.1016/j.neuroimage.2020.116702
2. Chawanpaiboon S, Vogel JP, Moller AB, Lumbiganon P, Petzold M, Hogan D, et al. Global, regional, and national estimates of levels of preterm birth in 2014: a systematic review and modelling analysis. Lancet Glob Heal. (2019) 7:e37–46. doi: 10.1016/S2214-109X(18)30451-0
3. Schneider J, Miller SP. Preterm brain injury: white matter injury. Handb Clin Neurol. (2019) 162:155–72. doi: 10.1016/B978-0-444-64029-1.00007-2
4. Inoue H, Ochiai M, Sakai Y, Yasuoka K, Tanaka K, Ichiyama M, et al. Neurodevelopmental outcomes in infants with birth weight ≤ 500 g at 3 years of age. Pediatrics. (2018) 142:e20174286. doi: 10.1542/peds.2017-4286
5. Vogel JP, Chawanpaiboon S, Moller AB, Watananirun K, Bonet M, Lumbiganon P. The global epidemiology of preterm birth. Best Pract Res Clin Obstet Gynaecol. (2018) 52:3–12. doi: 10.1016/j.bpobgyn.2018.04.003
6. Liu N, Wang ZZ, Zhao M, Zhang Y, Chen NH. Role of non-coding RNA in the pathogenesis of depression. Gene. (2020) 735:144276. doi: 10.1016/j.gene.2019.144276
7. Mehta SL, Dempsey RJ, Vemuganti R. Role of circular RNAs in brain development and CNS diseases. Prog Neurobiol. (2020) 186:101746. doi: 10.1016/j.pneurobio.2020.101746
8. Kleaveland B, Shi CY, Stefano J, Bartel DP. A network of noncoding regulatory RNAs Acts in the mammalian brain. Cell. (2018) 174:350–62.e17. doi: 10.1016/j.cell.2018.05.022
9. Wang H, Li Z, Gao J, Liao Q. Circular RNA circPTK2 regulates oxygen-glucose deprivation-activated microglia-induced hippocampal neuronal apoptosis via miR-29b-SOCS-1-JAK2/STAT3-IL-1β signaling. Int J Biol Macromol. (2019) 129:488–96. doi: 10.1016/j.ijbiomac.2019.02.041
10. Costello A, Lao NT, Barron N, Clynes M. Reinventing the wheel: synthetic circular RNAs for mammalian cell engineering. Trends Biotechnol. (2020) 38:217–30. doi: 10.1016/j.tibtech.2019.07.008
11. Zhu H, Xing Z, Zhao Y, Hao Z, Li M. The role of circular RNAs in brain injury. Neuroscience. (2020) 428:50–9. doi: 10.1016/j.neuroscience.2019.12.018
12. Xiao MS, Ai Y, Wilusz JE. Biogenesis and functions of circular RNAs come into focus. Trends Cell Biol. (2020) 30:226–40. doi: 10.1016/j.tcb.2019.12.004
13. Qu X, Li Z, Chen J, Hou L. The emerging roles of circular RNAs in CNS injuries. J Neurosci Res. (2020) 98:1485–97. doi: 10.1002/jnr.24591
14. Patop IL, Kadener S. circRNAs in cancer. Curr Opin Genet Dev. (2018) 48:121–7. doi: 10.1016/j.gde.2017.11.007
15. Filippenkov IB, Kalinichenko EO, Limborska SA, Dergunova LV. Circular RNAs—one of the enigmas of the brain. Neurogenetics. (2017) 18:1–6. doi: 10.1007/s10048-016-0490-4
16. Zhang Y, Xue W, Li X, Zhang J, Chen S, Zhang JL, et al. The biogenesis of nascent circular RNAs. Cell Rep. (2016) 15:611–24. doi: 10.1016/j.celrep.2016.03.058
17. Gasparini S, Del Vecchio G, Gioiosa S, Flati T, Castrignano T, Legnini I, et al. Differential expression of hippocampal circular RNAs in the BTBR mouse model for autism spectrum disorder. Mol Neurobiol. (2020) 57:2301–13. doi: 10.1007/s12035-020-01878-6
18. Zhuo CJ, Hou WH, Jiang DG, Tian HJ, Wang LN, Jia F, et al. Circular RNAs in early brain development and their influence and clinical significance in neuropsychiatric disorders. Neural Regen Res. (2020) 15:817–23. doi: 10.4103/1673-5374.268969
19. Kristensen LS, Andersen MS, Stagsted LVW, Ebbesen KK, Hansen TB, Kjems J. The biogenesis, biology and characterization of circular RNAs. Nat Rev Genet. (2019) 20:675–91. doi: 10.1038/s41576-019-0158-7
20. Chen L, Wang F, Bruggeman EC, Li C, Yao B. CircMeta: a unified computational framework for genomic feature annotation and differential expression analysis of circular RNAs. Bioinformatics. (2020) 36:539–45. doi: 10.1093/bioinformatics/btz606
21. Li HM, Ma XL, Li HG. Intriguing circles: conflicts and controversies in circular RNA research. Wiley Interdiscip Rev RNA. (2019) 10:1–19. doi: 10.1002/wrna.1538
22. Lu S, Yang X, Wang C, Chen S, Lu S, Yan W, et al. Current status and potential role of circular RNAs in neurological disorders. J Neurochem. (2019) 150:237–48. doi: 10.1111/jnc.14724
23. Ji P, Wu W, Chen S, Zheng Y, Zhou L, Zhang J, et al. Expanded expression landscape and prioritization of circular RNAs in mammals. Cell Rep. (2019) 26:3444.e5–60.e5. doi: 10.1016/j.celrep.2019.02.078
24. Ottesen EW, Luo D, Seo J, Singh NN, Singh RN. Human survival motor neuron genes generate a vast repertoire of circular RNAs. Nucleic Acids Res. (2019) 47:2884–905. doi: 10.1093/nar/gkz034
25. Lu D, Xu AD. Mini Review: Circular RNAs as potential clinical biomarkers for disorders in the central nervous system. Front Genet. (2016) 7:1–5. doi: 10.3389/fgene.2016.00053
26. Gokool A, Anwar F, Voineagu I. The landscape of circular RNA expression in the human brain. Biol Psychiatry. (2020) 87:294–304. doi: 10.1016/j.biopsych.2019.07.029
27. Chen YJ, Chen CY, Mai TL, Chuang CF, Chen YC, Gupta SK, et al. Genome-wide, integrative analysis of circular RNA dysregulation and the corresponding circular RNAmicroRNA-mRNA regulatory axes in autism. Genome Res. (2020) 30:375–91. doi: 10.1101/gr.255463.119
28. Mahmoudi E, Cairns MJ. Circular RNAs are temporospatially regulated throughout development and ageing in the rat. Sci Rep. (2019) 9:1–12. doi: 10.1038/s41598-019-38860-9
29. Zucko D, Boris-Lawrie K. Circular RNAs are regulators of diverse animal transcriptomes: one health perspective. Front Genet. (2020) 11:1–16. doi: 10.3389/fgene.2020.00999
30. Zhou T, Xie X, Li M, Shi J, Zhou JJ, Knox KS, et al. Rat BodyMap transcriptomes reveal unique circular RNA features across tissue types and developmental stages. RNA. (2018) 24:1443–56. doi: 10.1261/rna.067132.118
31. Haque S, Ames RM, Moore K, Pilling LC, Peters LL, Bandinelli S, et al. circRNAs expressed in human peripheral blood are associated with human aging phenotypes, cellular senescence and mouse lifespan. GeroScience. (2020) 42:183–99. doi: 10.1007/s11357-019-00120-z
32. Lu CX, Sun XM, Li N, Wang WG, Kuang DX, Tong PF, et al. CircRNAs in the tree shrew (Tupaia belangeri) brain during postnatal development and aging. Aging (Albany NY). (2018) 10:833–52. doi: 10.18632/aging.101437
33. Rybak-Wolf A, Stottmeister C, GlaŽar P, Jens M, Pino N, Giusti S, et al. Circular RNAs in the mammalian brain are highly abundant, conserved, and dynamically expressed. Mol Cell. (2014) 58:870–85. doi: 10.1016/j.molcel.2015.03.027
34. Kondo MA, Mohan A, Mather KA. Going around in circles: deciphering the role of circular RNAs in neurodegenerative disease. Curr Opin Psychiatry. (2020) 33:141–7. doi: 10.1097/YCO.0000000000000582
35. Patop IL, Wüst S, Kadener S. Past, present, and future of circRNAs. EMBO J. (2019) 38:1–13. doi: 10.15252/embj.2018100836
36. Nicolet BP, Engels S, Aglialoro F, Van Den Akker E, Von Lindern M, Wolkers MC. Circular RNA expression in human hematopoietic cells is widespread and cell-type specific. Nucleic Acids Res. (2018) 46:8168–80. doi: 10.1093/nar/gky721
37. Chen BJ, Huang S, Janitz M. Changes in circular RNA expression patterns during human foetal brain development. Genomics. (2019) 111:753–8. doi: 10.1016/j.ygeno.2018.04.015
38. Paul S, Bravo Vázquez LA, Pérez Uribe S, Roxana Reyes-Pérez P, Sharma A. Current status of microRNA-based therapeutic approaches in neurodegenerative disorders. Cells. (2020) 9:1–26. doi: 10.3390/cells9071698
39. Zang J, Lu D, Xu A. The interaction of circRNAs and RNA binding proteins: an important part of circRNA maintenance and function. J Neurosci Res. (2020) 98:87–97. doi: 10.1002/jnr.24356
40. Wu F, Han B, Wu S, Yang L, Leng S, Li M, et al. Circular RNA TLK1 aggravates neuronal injury and neurological deficits after ischemic stroke via miR-335-3p/TIPARP. J Neurosci. (2019) 39:7369–93. doi: 10.1523/JNEUROSCI.0299-19.2019
41. Xie F, Zhao Y, Wang S D, Ma J, Wang X, Qian LJ. Identification, characterization, and functional investigation of circular RNAs in subventricular zone of adult rat brain. J Cell Biochem. (2019) 120:3428–37. doi: 10.1002/jcb.27614
42. Piwecka M, GlaŽar P, Hernandez-Miranda LR, Memczak S, Wolf SA, Rybak-Wolf A, et al. Loss of a mammalian circular RNA locus causes miRNA deregulation and affects brain function. Science. (2017) 357:eaam8526. doi: 10.1126/science.aam8526
43. Zhang Y, Du L, Bai Y, Han B, He C, Gong L, et al. CircDYM ameliorates depressive-like behavior by targeting miR-9 to regulate microglial activation via HSP90 ubiquitination. Mol Psychiatry. (2020) 25:1175–90. doi: 10.1038/s41380-018-0285-0
44. Zimmerman AJ, Hafez AK, Amoah SK, Rodriguez BA, Dell'Orco M, Lozano E, et al. A psychiatric disease-related circular RNA controls synaptic gene expression and cognition. Mol Psychiatry. (2020) 25:2712–27. doi: 10.1038/s41380-020-0653-4
45. Mahmoudi E, Kiltschewskij D, Fitzsimmons C, Cairns MJ. Depolarization-associated CircRNA regulate neural gene expression and in some cases may function as templates for translation. Cells. (2019) 9:25. doi: 10.3390/cells9010025
46. Sun J, Li B, Shu C, Ma Q, Wang J. Functions and clinical significance of circular RNAs in glioma. Mol Cancer. (2020) 19:1–18. doi: 10.1186/s12943-019-1121-0
47. Zhang M, Zhao K, Xu X, Yang Y, Yan S, Wei P, et al. A peptide encoded by circular form of LINC-PINT suppresses oncogenic transcriptional elongation in glioblastoma. Nat Commun. (2018) 9:4475. doi: 10.1038/s41467-018-06862-2
48. Zechariah A, Tran CHT, Hald BO, Sandow SL, Sancho M, Kim MSM, et al. Intercellular conduction optimizes arterial network function and conserves blood flow homeostasis during cerebrovascular challenges. Arterioscler Thromb Vasc Biol. (2020)40:733–50. doi: 10.1161/ATVBAHA.119.313391
49. Rocha-Ferreira E, Hristova M. Plasticity in the neonatal brain following hypoxic-ischaemic injury. Neural Plast. (2016) 2016 :4901014. doi: 10.1155/2016/4901014
50. Rodríguez M, Valez V, Cimarra C, Blasina F, Radi R. Hypoxic-ischemic encephalopathy and mitochondrial dysfunction: facts, unknowns, and challenges. Antioxidants Redox Signal. (2020) 33:247–62. doi: 10.1089/ars.2020.8093
51. Ma Y, Liu Y, Jiang Z. CircRNAs: a new perspective of biomarkers in the nervous system. Biomed Pharmacother. (2020) 128:110251. doi: 10.1016/j.biopha.2020.110251
52. Warnock A, Toomey LM, Wright AJ, Fisher K, Won Y, Anyaegbu C, et al. Damage mechanisms to oligodendrocytes and white matter in central nervous system injury: the australian context. J Neurotrauma. (2020) 37:739–69. doi: 10.1089/neu.2019.6890
53. van Tilborg E, de Theije CGM, van Hal M, Wagenaar N, de Vries LS, Benders MJ, et al. Origin and dynamics of oligodendrocytes in the developing brain: implications for perinatal white matter injury. Glia. (2018) 66:221–38. doi: 10.1002/glia.23256
54. Qin C, Liu Q, Hu ZW, Zhou LQ, Shang K, Bosco DB, et al. Microglial TLR4-dependent autophagy induces ischemic white matter damage via STAT1/6 pathway. Theranostics. (2018) 8:5434–51. doi: 10.7150/thno.27882
55. Holt CE, Martin KC, Schuman EM. Local translation in neurons: visualization and function. Nat Struct Mol Biol. (2019) 26:557–66. doi: 10.1038/s41594-019-0263-5
56. Diering GH, Huganir RL. The AMPA receptor code of synaptic plasticity. Neuron. (2018) 100:314–29. doi: 10.1016/j.neuron.2018.10.018
57. Chen BJ, Yang B, Janitz M. Region-specific expression of circular RNAs in the mouse brain. Neurosci Lett. (2018) 666:44–7. doi: 10.1016/j.neulet.2017.12.022
58. Jaitner C, Reddy C, Abentung A, Whittle N, Rieder D, Delekate A, et al. Satb2 determines miRNA expression and long-term memory in the adult central nervous system. Elife. (2016) 5:e17361. doi: 10.7554/eLife.17361
59. Lu Y, Tan L, Wang X. Circular HDAC9/microRNA-138/Sirtuin-1 pathway mediates synaptic and amyloid precursor protein processing deficits in Alzheimer's disease. Neurosci Bull. (2019) 35:877–88. doi: 10.1007/s12264-019-00361-0
60. Li J, Lin H, Sun Z, Kong G, Yan X, Wang Y, et al. High-throughput data of circular RNA profiles in human temporal cortex tissue reveals novel insights into temporal lobe epilepsy. Cell Physiol Biochem. (2018) 45:677–91. doi: 10.1159/000487161
61. Gomes-Duarte A, Bauer S, Venø MT, Norwood BA, Henshall DC, Kjems J, et al. Enrichment of circular RNA expression deregulation at the transition to recurrent spontaneous seizures in experimental temporal lobe epilepsy. Front Genet. (2021) 12:1–20. doi: 10.3389/fgene.2021.627907
62. Schwartz E, Wilkens A, Noon SE, Krantz ID, Wu Y, A de novo SATB2 mutation in monozygotic twins with cleft palate dental anomalies and developmental delay. Am J Med Genet Part A. (2017) 173:809–12. doi: 10.1002/ajmg.a.38071
63. McKenna WL, Ortiz-Londono CF, Mathew TK, Hoang K, Katzman S, Chen B. Mutual regulation between Satb2 and Fezf2 promotes subcerebral projection neuron identity in the developing cerebral cortex. Proc Natl Acad Sci USA. (2015) 112:11702–7. doi: 10.1073/pnas.1504144112
64. Serdar M, Herz J, Kempe K, Lumpe K, Reinboth BS, Sizonenko S V, et al. Fingolimod protects against neonatal white matter damage and long-term cognitive deficits caused by hyperoxia. Brain Behav Immun. (2016) 52:106–19. doi: 10.1016/j.bbi.2015.10.004
65. Volpe JJ. Brain injury in premature infants: a complex amalgam of destructive and developmental disturbances. Lancet Neurol. (2009) 8:110–24. doi: 10.1016/S1474-4422(08)70294-1
66. Thijs RD, Surges R, O'Brien TJ, Sander JW. Epilepsy in adults. Lancet. (2019) 393:689–701. doi: 10.1016/S0140-6736(18)32596-0
67. Pimentel-Silva LR, Casseb RF, Cordeiro MM, Campos BAG, Alvim MKM, Rogerio F, et al. Interactions between in vivo neuronal-glial markers, side of hippocampal sclerosis, and pharmacoresponse in temporal lobe epilepsy. Epilepsia. (2020) 61:1008–18. doi: 10.1111/epi.16509
68. Shao Y, Chen Y. Pathophysiology and clinical utility of non-coding RNAs in epilepsy. Front Mol Neurosci. (2017) 10:1–10. doi: 10.3389/fnmol.2017.00249
69. Castañeda MS, Zanoteli E, Scalco RS, Scaramuzzi V, Caldas VM, Reed UC, et al. A novel ATP1A2 mutation in a patient with hypokalaemic periodic paralysis and CNS symptoms. Brain. (2018) 141:3308–18. doi: 10.1093/brain/awy283
70. De Fusco M, Marconi R, Silvestri L, Atorino L, Rampoldi L, Morgante L, et al. Haploinsufficiency of ATP1A2 encoding the Na+/K+ pump α2 subunit associated with familial hemiplegic migraine type 2. Nat Genet. (2003) 33:192–6. doi: 10.1038/ng1081
71. Zheng D, Li M, Li G, Hu J, Jiang X, Wang Y, et al. Circular RNA circ_DROSHA alleviates the neural damage in a cell model of temporal lobe epilepsy through regulating miR-106b-5p/MEF2C axis. Cell Signal. (2021) 80:109901. doi: 10.1016/j.cellsig.2020.109901
72. Kleuskens DG, Gonçalves Costa F, Annink KV, van den Hoogen A, Alderliesten T, Groenendaal F, et al. Pathophysiology of cerebral hyperperfusion in term neonates with hypoxic-ischemic encephalopathy: a systematic review for future research. Front Pediatr. (2021) 9:631258. doi: 10.3389/fped.2021.631258
73. Thornton C, Leaw B, Mallard C, Nair S, Jinnai M, Hagberg H. Cell death in the developing brain after hypoxia-ischemia. Front Cell Neurosci. (2017) 11:248. doi: 10.3389/fncel.2017.00248
74. Jiang L, Li H, Fan Z, Zhao R, Xia Z. Circular RNA expression profiles in neonatal rats following hypoxic-ischemic brain damage. Int J Mol Med. (2019) 43:1699–708. doi: 10.3892/ijmm.2019.4111
75. Bai Y, Zhang Y, Han B, Yang L, Chen X, Huang R, et al. Circular RNA DLGAP4 ameliorates ischemic stroke outcomes by targeting miR-143 to regulate endothelial-mesenchymal transition associated with blood–brain barrier integrity. J Neurosci. (2018) 38:32–50. doi: 10.1523/JNEUROSCI.1348-17.2017
76. Chen X, Nakada S, Donahue JE, Chen RH, Tucker R, Qiu J, et al. Neuroprotective effects of inter-alpha inhibitor proteins after hypoxic-ischemic brain injury in neonatal rats. Exp Neurol. (2019) 317:244–59. doi: 10.1016/j.expneurol.2019.03.013
77. Wang P, Zhao M, Chen Z, Wu G, Fujino M, Zhang C, et al. Hydrogen gas attenuates hypoxic-ischemic brain injury via regulation of the MAPK/HO-1/PGC-1a pathway in neonatal rats. Oxid Med Cell Longev. (2020) 2020:6978784. doi: 10.1155/2020/6978784
78. Inaba T, Miyamoto N, Hira K, Ueno Y, Yamashiro K, Watanabe M, et al. Protective role of levetiracetam against cognitive impairment and brain white matter damage in mouse prolonged cerebral hypoperfusion. Neuroscience. (2019) 414:255–64. doi: 10.1016/j.neuroscience.2019.07.015
79. Yang B, Zang L, Cui J, Wei L. Circular RNA TTC3 regulates cerebral ischemia-reperfusion injury and neural stem cells by miR-372-3p/TLR4 axis in cerebral infarction. Stem Cell Res Ther. (2021) 12:1–11. doi: 10.1186/s13287-021-02187-y
80. Cao Y, Liu H, Zhang J, Dong Y. Circular RNA cZNF292 silence alleviates OGD/R-induced injury through up-regulation of miR-22 in rat neural stem cells (NSCs). Artif Cells Nanomed Biotechnol. (2020) 48:594–601. doi: 10.1080/21691401.2020.1725536
81. Hübner K, Cabochette P, Diéguez-Hurtado R, Wiesner C, Wakayama Y, Grassme KS, et al. Wnt/β-catenin signaling regulates VE-cadherin-mediated anastomosis of brain capillaries by counteracting S1pr1 signaling. Nat Commun. (2018) 9:4860 doi: 10.1038/s41467-018-07302-x
82. Lin SP, Ye S, Long Y, Fan Y, Mao HF, Chen MT, et al. Circular RNA expression alterations are involved in OGD/R-induced neuron injury. Biochem Biophys Res Commun. (2016) 471:52–6. doi: 10.1016/j.bbrc.2016.01.183
83. Zhang ZH, Wang YR, Li F, Liu XL, Zhang H, Zhu ZZ, et al. Circ-camk4 involved in cerebral ischemia/reperfusion induced neuronal injury. Sci Rep. (2020) 10:7012. doi: 10.1038/s41598-020-63686-1
84. Liu W, Jia C, Luo L, Wang HL, Min XL, Xu JH, et al. Novel circular RNAs expressed in brain microvascular endothelial cells after oxygen-glucose deprivation/recovery. Neural Regen Res. (2019) 14:2104–11. doi: 10.4103/1673-5374.262589
85. Min JW, Bu F, Qi L, Munshi Y, Kim GS, Marrelli SP, et al. Inhibition of calcium/calmodulin-dependent protein kinase kinase β is detrimental in hypoxia–ischemia neonatal brain injury. Int J Mol Sci. (2019) 20:2063. doi: 10.3390/ijms20092063
86. Agut T, Alarcon A, Cabañas F, Bartocci M, Martinez-Biarge M, Horsch S, et al. Preterm white matter injury: ultrasound diagnosis and classification. Pediatr Res. (2020) 87:37–49. doi: 10.1038/s41390-020-0781-1
87. Xiao D, Qu Y, Pan L, Li X, Mu D. MicroRNAs participate in the regulation of oligodendrocytes development in white matter injury. Rev Neurosci. (2018) 29:151–60. doi: 10.1515/revneuro-2017-0019
88. Hinojosa-Rodríguez M, Harmony T, Carrillo-Prado C, Van Horn JD, Irimia A, Torgerson C, et al. Clinical neuroimaging in the preterm infant: diagnosis and prognosis. NeuroImage Clin. (2017) 16:355–68. doi: 10.1016/j.nicl.2017.08.015
89. Mohammad K, Scott JN, Leijser LM, Zein H, Afifi J, Piedboeuf B, et al. Consensus approach for standardizing the screening and classification of preterm brain injury diagnosed with cranial ultrasound: a canadian perspective. Front Pediatr. (2021) 9:618236. doi: 10.3389/fped.2021.618236
90. Qiao L, Mo S, Zhou Y, Zhang Y, Li B, Wu S, et al. Circular RNA expression alteration in whole blood of premature infants with periventricular white matter damage. Genomics. (2020) 112:2875–85. doi: 10.1016/j.ygeno.2020.03.027
91. Philips T, Rothstein JD. Oligodendroglia: metabolic supporters of neurons. J Clin Invest. (2017) 127:3271–80. doi: 10.1172/JCI90610
92. Wang F, Yang YJ, Yang N, Chen XJ, Huang NX, Zhang J, et al. Enhancing oligodendrocyte myelination rescues synaptic loss and improves functional recovery after chronic hypoxia. Neuron. (2018) 99:689.e5–701.e5. doi: 10.1016/j.neuron.2018.07.017
93. van Tilborg E, Heijnen CJ, Benders MJ, van Bel F, Fleiss B, Gressens P, et al. Impaired oligodendrocyte maturation in preterm infants: potential therapeutic targets. Prog Neurobiol. (2016) 136:28–49. doi: 10.1016/j.pneurobio.2015.11.002
94. Mao S, Huang T, Chen Y, Shen L, Zhou S, Zhang S, et al. Circ-Spidr enhances axon regeneration after peripheral nerve injury. Cell Death Dis. (2019) 10:787. doi: 10.1038/s41419-019-2027-x
95. Whitton L, Apostolova G, Rieder D, Dechant G, Rea S, Donohoe G, et al. Genes regulated by SATB2 during neurodevelopment contribute to schizophrenia and educational attainment. PLoS Genet. (2018) 14:e1007515. doi: 10.1371/journal.pgen.1007515
96. Mallard C, Tremblay ME, Vexler ZS. Microglia and neonatal brain injury. Neuroscience. (2019) 405:68–76. doi: 10.1016/j.neuroscience.2018.01.023
97. Xiaoying G, Guo M, Jie L, Yanmei Z, Ying C, Shengjie S, et al. CircHivep2 contributes to microglia activation and inflammation via miR-181a-5p/SOCS2 signalling in mice with kainic acid-induced epileptic seizures. J Cell Mol Med. (2020) 24:12980–93. doi: 10.1111/jcmm.15894
Keywords: circular RNA, preterm infants, neurological impairment, white matter damage, hypoxic-ischemic encephalopathy
Citation: Gu Q, Liu H, Ma J, Yuan J, Li X and Qiao L (2021) A Narrative Review of Circular RNAs in Brain Development and Diseases of Preterm Infants. Front. Pediatr. 9:706012. doi: 10.3389/fped.2021.706012
Received: 14 May 2021; Accepted: 23 August 2021;
Published: 21 September 2021.
Edited by:
Diego Gazzolo, SS Annunziata Polyclinic Hospital, ItalyReviewed by:
Fahri Ovali, Istanbul Medeniyet University, TurkeyCopyright © 2021 Gu, Liu, Ma, Yuan, Li and Qiao. This is an open-access article distributed under the terms of the Creative Commons Attribution License (CC BY). The use, distribution or reproduction in other forums is permitted, provided the original author(s) and the copyright owner(s) are credited and that the original publication in this journal is cited, in accordance with accepted academic practice. No use, distribution or reproduction is permitted which does not comply with these terms.
*Correspondence: Lixing Qiao, cWlhb2xpeGluZ0BhbGl5dW4uY29t
Disclaimer: All claims expressed in this article are solely those of the authors and do not necessarily represent those of their affiliated organizations, or those of the publisher, the editors and the reviewers. Any product that may be evaluated in this article or claim that may be made by its manufacturer is not guaranteed or endorsed by the publisher.
Research integrity at Frontiers
Learn more about the work of our research integrity team to safeguard the quality of each article we publish.